- Department of Ecology and Evolutionary Biology, University of Colorado, Boulder, CO, United States
Some herbivorous insects sequester chemicals from their host plants, which can serve as a defense against predators. However, sequestration can also be costly, impacting immunological responses important for other types of natural enemies, such as parasites and pathogens. These costs could also vary across herbivorous insect development, as both immune function and sequestration abilities change, although few studies have assessed variation in the cost of sequestration across life stages. The buckeye, Junonia coenia (Nymphalidae), is a specialist butterfly that sequesters iridoid glycosides from its host plants, including the introduced weed, Plantago lanceolata (Plantaginaceae). To determine the immunological costs of sequestration and how the immune response varies with host plant and across development, we reared caterpillars on a native host plant, Mimulus guttatus (Phrymaceae), which does not contain iridoid glycosides, and on P. lanceolata, which does. We assayed immune function across 3rd, 4th, and 5th instar caterpillars, estimating both hemocyte density and the ability of hemocytes to encapsulate foreign bodies by challenging the immune system with nylon filaments. For caterpillars reared on P. lanceolata, we then explored the relationships between iridoid glycoside sequestration and immune function across instars. We found that while hemocyte density tended to increase with instar regardless of host plant, caterpillars reared on P. lanceolata had lower encapsulation ability, and encapsulation decreased with increasing sequestration of iridoid glycosides, though patterns varied between instars and experimental periods. Interestingly, immune challenged caterpillars sequestered more iridoid glycosides than unchallenged caterpillars, suggesting that caterpillars responded to immune challenge by sequestering or retaining more iridoids, even though that may decrease their ability to encapsulate. These results suggest that sequestration can have important consequences for immune function across caterpillar development, and that the incorporation of novel hosts may affect defense against natural enemies.
Introduction
Herbivorous insects are exposed to many different natural enemies throughout their development, including predators, parasites, parasitoids, and pathogens (Rosenheim, 1998; Vidal and Murphy, 2018), and employ a variety of strategies to defend themselves against these enemies. For example, some insects use chemical defenses that are sequestered from the plants on which they feed (Brower et al., 1968; Bowers, 1990). However, while sequestration can be an effective defense against predators, the process of sequestration can also be costly (Bowers, 1992; Hartmann, 2004; Smilanich et al., 2009a; Zvereva and Kozlov, 2016). Sequestration or detoxification of plant secondary metabolites (PSMs) may involve several processes to store or metabolize the compounds; for example, compounds may be rendered harmless by the activity of gut enzymes (Dobler et al., 2011), or the compounds may be transferred from the gut to certain organs for storage (Poreddy et al., 2015). The mechanisms required for those processes are energetically costly and may impact other physiological processes. For instance, the immune system functions to target parasites, parasitoids, and pathogens that have entered the insect's body (Barbosa and Caldas, 2007) using specific immune cells to target and kill foreign bodies (Beckage, 2008). However, while a substantial amount of research has been conducted on the chemical defenses of herbivorous insects, until recently there has been less focus on how sequestration might impact immune function (see Smilanich et al., 2009a).
Ecoimmunology is an interdisciplinary field combining ecology and immunology that has been advancing because of its applications in fields such as disease ecology and biological control (Stanley et al., 2012). Smilanich et al. (2009a) showed that larvae feeding on plants with high amounts of PSMs have weaker immune responses compared to those fed on plants with lower amounts, suggesting that host plant chemistry is important not only for insect chemical defenses, but also for the effectiveness of the immune responses. This raises the possibility of trade-offs in defense strategies against multiple natural enemies, if the costs of sequestering PSMs outweigh the benefits of immune defenses or vice versa. Such trade-offs may become even more important in the presence of ecological perturbations, such as human-induced environmental change, which can lead to changes in host plant quality (Jamieson et al., 2015; Decker et al., 2018), range shifts of host plants or natural enemies (Jeffs and Lewis, 2013), the introduction of novel hosts (Jahner et al., 2011), and subsequently insect defense against natural enemies (Gherlenda et al., 2016).
Moreover, as an insect develops, the suite of natural enemies encountered often changes, necessitating different strategies in response to attacking enemies (Stamp, 1986). For example, younger and smaller stages may be more susceptible to predators (Memmott et al., 2000) and their immune system may not be fully developed until later stages (Brodeur and Vet, 1995; Gillespie et al., 1997), when parasitoids and pathogens may be more frequently encountered. Insects have an innate immune system with both humoral and cellular defenses, the latter consisting of three components, phagocytosis, nodule formation, and encapsulation (Strand, 2008). The cellular responses are primarily used to defend against enemies that have invaded the insect, such as parasitoid eggs, parasites, and pathogens (Beckage, 2008). Early larval instars have been shown to have weaker immune responses, suggesting that one component of the immune response, hemocyte production and differentiation may be important later in development (Brodeur and Vet, 1995; Gillespie et al., 1997). The activity of the immune response itself may also vary across development. For example, in Bombyx mori (Bombycidae), hemocyte adhesion, and subsequently phagocytosis have been shown to increase against invaders as larvae age (Wago and Ichikawa, 1979). Given that selective pressures from natural enemies change over larval development, larvae could exhibit stage-specific investment in chemical and immune defensive strategies. Previous studies have investigated how sequestering PSMs can affect insect herbivore preference and performance, parasitoid success, and immune response (Dyer, 1995; Smilanich et al., 2009b). However, changes in immune responses over insect development and how it relates to PSM sequestration has been little studied.
To determine how the sequestration of PSMs impacts the immune function of caterpillars across development, we reared larvae of the sequestering specialist, Junonia coenia (Nymphalidae) (common buckeye) on two hosts: one, an introduced weed (Cavers et al., 1980), containing sequesterable PSMs (Plangato lanceolata, Plantaginaceae) and the other, a native host plant species, without (Mimulus guttatus, Phrymaceae). We then compared immune functions of different instar caterpillars between host plants, and explored the relationship between sequestration and immune function across caterpillar development. Specifically we asked: (1) does the immune response of this specialist caterpillar change with larval instar, (2) if so, does the host plant on which caterpillars feed affect this response, and (3) does the relationship between sequestration and immune function vary across caterpillar development.
Methods
Study System
Junonia coenia (Nymphalidae), the common buckeye, is a specialist butterfly whose larvae feed on a variety of host plant species, most of which contain a specific group of PSMs, iridoid glycosides (Bowers, 1984). Iridoid glycosides (hereafter IGs) serve as feeding stimulants for the larvae and as oviposition stimulants for adult females (Bowers, 1984; Bowers and Puttick, 1986; Pereyra and Bowers, 1988; Bowers et al., 1992a). Larvae have been recorded feeding on plants in five different families containing IGs: Scrophulariaceae, Plantaginaceae, Verbenaceae, Acanthaceae, and Cornaceae (Bowers, 1984). Previous research has shown that J. coenia larvae sequester relatively high levels of IGs, ranging from 10 to 20% in newly molted individuals (Bowers and Collinge, 1992) and making them unpalatable to potential predators (Bowers, 1992).
Plantago lanceolata (Plantaginaceae, hereafter Plantago) is a common weed incorporated into the diet of many native North American insect herbivores, including buckeyes (Bowers and Collinge, 1992). It is native to Eurasia but has been distributed all over the world (Cavers et al., 1980). Plantago contains two IGs, aucubin and catalpol (Duff et al., 1965), that can be deterrent to generalist herbivores and non-adapted specialist herbivores (Bowers and Puttick, 1988). Buckeye larvae also feed on the native yellow monkey flower, Mimulus guttatus (Phrymaceae, hereafter Mimulus), which is found in wetlands across western North America and is their only host plant that does not contain IGs (Kooiman, 1970; Holeski et al., 2010). Although, Mimulus does contain phenylpropanoid glycosides; one of these, verbascoside, serves as a feeding stimulant for buckeye larvae, and can also be deterrent to generalist herbivores (Holeski et al., 2013).
Experimental Design
To explore how a novel host plant impacts sequestration and immune function, we used greenhouse grown Mimulus and Plantago as alternate hosts for buckeye caterpillars. Plants were grown from seed in a growth chamber at 25° Celsius until large enough to be transferred to a greenhouse where the temperature ranged from 10° to 21°C. Plants were watered daily and fertilized with a 24-8-16 water soluble fertilizer (Miracle-Gro, The Scotts Company, LLC) twice per week. Given that chemical analyses indicated Plantago plants were very low in IGs when the experiment was first conducted in January (designated Experiment 1) (see Results), we repeated the entire experiment in September (designated Experiment 2) when plants had higher IG levels, allowing for qualitative comparison between times when host plants are low or high in IG concentrations.
Caterpillars for the experiment were reared from a source colony originating from adult butterflies collected around Sacramento, California. The adults were kept in 60 × 60 × 60 cm butterfly tents (BugDorm), where they were allowed to mate and oviposit on either Plantago or Mimulus plants. First instar larvae were collected from the host plants and placed into clear, round plastic petri dishes, 14 cm in diameter, and placed in a growth chamber kept on a 14:10 LD cycles, at 25°C light and 20°C dark. When larvae molted to the second instar, individuals were put into 60 ml plastic cups with plastic lids in order to monitor when they molted into third instar to begin immune assays.
Immune Assays
We compared two metrics of the immune response between caterpillars reared on each host plant: hemocyte density and encapsulation. Hemocytes are the primary cellular response of the insect immune system (Lavine and Strand, 2002), and all hemocyte types are involved in the immune response (Strand et al., 2006). Encapsulation is the process by which hemocytes in the insect's body cavity attach to foreign objects (Pech and Strand, 1996) too large to be killed by phagocytosis (Gillespie et al., 1997). After hemocytes adhere to the foreign object, they die and melanize (Smilanich et al., 2009b), killing cells (such as parasitoid eggs or pathogens) by asphyxiation and with cytotoxic molecules (Nappi and Christensen, 2005). Encapsulation can thus be measured by quantifying the melanization on the foreign object using photo imaging software (Smilanich et al., 2009b).
Once larvae reared on each of the two host plants reached the middle of the appropriate instar (~2 days after molting to third, fourth, or fifth; N = 15 on each host plant/instar combination), they were weighed to the nearest 0.01 mg, then placed into a freezer at −29°C for 1–2 min in order to slow the caterpillar's movement while being assayed (Smilanich et al., 2009b). Each caterpillar was then placed on a watch glass so that it was lying on its right side, and Parafilm used to hold the caterpillar in place. A clean insect pin was used to make a small hole in a designated spot between the 4th and 5th proleg in each caterpillar. After removing the pin, we sampled 10 uL of hemolymph using a Gilson Pipet set to 10 microliters. For some smaller 3rd instars where 10 uL was unable to be collected (due to small size), we sampled 5 uL. Hemolymph samples were immediately added to an equal amount of anticoagulant, and stored on ice until hemocytes were counted after filament insertion (see below). Anticoagulant was prepared using 0.684 grams of EDTA, 0.346 grams of citric acid, and 180 mL of phosphate-buffered saline (as in Smilanich et al., 2018). We counted all hemocytes using a hemocytometer (Sigma-Aldrich Bright-Line) under a compound light microscope following Triggs and Knell (2012), and calculated cell density per mL of hemolymph accordingly.
To measure encapsulation we inserted nylon filaments (Figure 1) as a proxy for parasitoid eggs or larvae (Smilanich et al., 2009b). Filaments were made using 0.20 mm diameter monofilament fishing line (Berkley Trilene XL Smooth Casting). The filaments were prepared by first lightly sanding a length of fishing line, then knots were tied along it and 2 mm lengths trimmed from the edge of each knot. An alternate method was also used in January, where sections of sanded monofilament were heated next to a butane lighter to melt and expand one end, then trimmed to 2 mm. Immediately after hemolymph was drawn from each caterpillar, a single filament was inserted into the wound by sliding it in laterally just under the cuticle of the caterpillar. The expanded end of the monofilament or knot held these inserts in place while the caterpillars were returned to the growth chamber, allowed to feed on their respective host plant, and given 24 h for encapsulation to occur (Smilanich et al., 2009b). Afterward, the filament was removed, and either placed in 70% ethanol in a 1.5 mL eppendorf tube in Experiment 1 (January) or immediately frozen (no ethanol) in Experiment 2 (September).
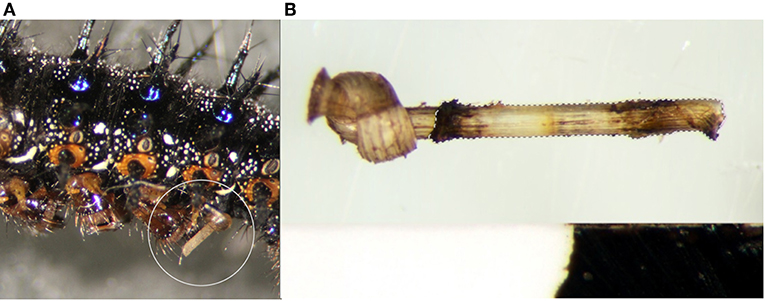
Figure 1. Nylon filaments were inserted just beneath the cuticle (A) behind the 4th abdominal proleg and imaged after 24 h (B) to measure the degree of melanization. Hashed lines represent the area of filament within the body of the caterpillar that was quantified.
We photographed filaments using a Canon Rebel XTi DSLR camera (Canon, U.S.A, Inc) mounted on a dissecting microscope. All photographs were taken at 16x magnification, and analyzed using Adobe Photoshop CC 2017 (Abobe, Inc.). In January, photographs were analyzed by setting the image mode to grayscale with 0 as white and 255 as black. The 1.5 mm tail end of the nylon monofilament was selected using the “magic wand” tool so that only the part that was inserted into the caterpillar was measured, and then we calculated the mean white value using the histogram tool. In September, we included a black and white standard with each filament during imaging to help standardize imaging measurements. The black and white standard was created using a small section of acid free archival, alcohol proof label paper, half of which was covered in black India ink. For each image, we set the black and white balance based on this standard, and measured the entire length of filament within the body of the caterpillar. We then calculated mean white values as above. Given that the mean measures the average amount of white per pixel across the filament, the inverse of the mean represents the average darkness of the filament; the darker the color the higher the degree of encapsulation (Smilanich et al., 2009b). We used this mean as a quantitative estimate of immune response.
IG Quantification and Sequestration
After 24 h of feeding and immediately after removing filaments, all caterpillars fed Plantago were placed in 1.5 mL of 95% methanol in 2 mL centrifuge tubes and frozen. To prepare samples for IG quantification, larvae were removed from the freezer and transferred into 15 mL glass test tubes. Whole caterpillars were ground with sand in five mL of methanol, left for 24 h for IGs to extract, then all particulates were filtered, and the remaining methanol evaporated (Knerl and Bowers, 2013). We then added 1 mL of 0.500 mg/mL phenyl-β-D-glucopyranoside (PBG) as an internal standard and 3 mL of water. To remove lipophilic substances, samples were treated with 3 ml of ether; vortexed, and the water and ether layers separated by centrifuging the samples for 4 min. The ether layer (containing lipophilic substances) was discarded (Bowers and Stamp, 1997) and the process repeated three times. The water layer, containing IGs and sugars, was evaporated, and then 1.00 ml of methanol was added and the sample left overnight. A 0.100 ml aliquot was then removed, the methanol evaporated, and the sample derivatized using Tri-Sil Z (Sigma Aldrich Chemical Company) (Bowers and Stamp, 1997).
To measure the iridoid glycoside content of the plant material fed to the larvae, we sampled leaves from five different Plantago plants in January and 10 different plants in September. Each set of leaves was dried at 50°C for 48 h, ground to a fine powder, and 25 mg weighed and extracted in methanol for 24 h. Plant sample preparation was then identical to caterpillar sample preparation. IG concentrations were reported as percent dry weight because of the variation in water content in plants and caterpillars (Knerl and Bowers, 2013). We calculated a dry weight conversion factor for 3rd, 4th, and 5th instar caterpillars, by weighing sets of 10 larvae fresh, then again after drying at 50°C for 48 h. We then multiplied the fresh weights of all caterpillars by the respective conversion factor to estimate dry weight.
We used gas chromatography (GC) to quantify IG concentrations of source plants of Plantago used for feeding and for caterpillars reared on Plantago during the experiment, following standard protocols (Bowers and Stamp, 1997). For IG quantification, we used an Agilent 7890A gas chromatograph equipped with a DB-1 column (30 m, 0.320 mm, 0.25 μm particle size), using flame-ionization detection. The temperature program used an initial temperature of 200°C held for 1 min, followed by a 3 min increase to 260°C, which was held for 8 min, followed by a 3 min increase to a final temperature of 320°C, held for 10 min. Amounts of aucubin and catalpol were quantified using ChemStation B-03-01 software after calibration using a standard containing known amounts of PBG, aucubin, and catalpol.
Sequestration and Immune Challenge
Since caterpillars store IGs in hemolymph (Bowers, 2003), removing hemolymph for counting hemocytes could directly reduce IGs in immune-challenged caterpillars. Therefore, we conducted two additional experiments to determine the impacts of our immune treatments on IG concentration. For the first, during both immune experiments (Experiment 1 and Experiment 2), we reared 15 additional caterpillars per instar on Plantago to serve as a comparative unmanipulated control for IG quantification. These were reared in the same way as other experimental groups but were not assayed for an immune response, thus no hemolymph was removed. In Experiment 1(January), these unchallenged controls were set up immediately after the initial experiment, and thus plants fed to those larvae could differ somewhat in their IG content compared to plants fed to caterpillars used for the immune assays (see Quintero et al., 2014) leading to differences in caterpillar IG content, although differences were likely to be small. In Experiment 2 (September), therefore, we set up the experiment so that unchallenged trials were conducted simultaneously with challenged trials.
In a third experiment (Experiment 3), we wanted to examine the consequences of hemolymph sampling and the immune assays for caterpillar sequestration in more detail. Therefore, the following March, we used 5th instar caterpillars fed on Plantago and had a control with three different immune assay treatments (N = 15 for each treatment). The first treatment group had 10 uL hemolymph drawn and was then immediately frozen for IG analysis to determine the direct effect of sampling hemolymph; the second had 10 ul hemolymph drawn and was then allowed 24 h to feed and recover before being frozen for IG analyses, allowing us to account for compensation for IG loss over 24 h; the third was treated as in the immune experiment, with 10 uL hemolymph drawn, a nylon filament inserted, and the caterpillar allowed 24 h to feed before removing the filament and freezing for IG analyses, to test for response to immune challenge. The control group was reared alongside other treatments but frozen without any immune assay. We expected immediate hemolymph draws should reduce IG concentration relative to unassayed controls. If caterpillars compensated for IG loss, they should sequester the same or more IGs after 24 h. Adding in the nylon filament should allow us to detect if immune challenge alters sequestration, either reducing or increasing IG concentrations relative to 24 h post-hemolymph draw treatments.
Statistical Analyses
All analyses were conducted in R version 3.51 (R. Core Team, 2017) and separate analyses were conducted for January and September. We compared hemocyte density between host plants and instars using two-way analysis of variance (ANOVA), with host plant, instar, and their interaction as fixed effects. We log-transformed hemocyte density to meet the assumption of normality. Given that hemocyte density should be predictive of encapsulation, we included it as a factor in analyses of melanization. To determine if host plant affected the relationship between hemocyte density and melanization, we analyzed melanization using a full-factorial ANCOVA, including host plant, and instar as main effects, hemocyte density as a covariate, and all two-way and three-way interactions. For caterpillars reared on Plantago, we compared the percent total IG sequestration and the proportion of IGs sequestered that was catalpol across larval instars using separate one-way ANOVAs, with larval instar as a fixed factor. We logit transformed both metrics of sequestration to meet the assumption of normality. To determine the effects of IG sequestration on immune response across development, we analyzed melanization of caterpillars fed Plantago using ANCOVA, with total IG concentration, cell density, and instar as main effects, and all two- and three-way interactions. We used only total percent IGs sequestered in ANCOVAs, given that Experiment 1 indicated that the proportion of catalpol sequestered was negatively correlated with total IG sequestration (T41 = −3.07, p = 0.004, r = −0.43). To determine if changes in sequestered IGs were indeed the results of immune challenge, we compared the different levels of immune treatment with ANOVA and Tukey Post Hoc tests. We included challenge type as a fixed effect, and logit transformed all IG measures. Seven caterpillars that died as a result of immune challenge during the experiment were excluded from analyses of melanization, given that they were unable to mount an immune response.
Results
Immune Assays
Experiment 1 (January)
Hemocyte density varied across larval development in January, increasing from third to fifth instar [Figure 2A, F(1, 84) = 17.75, p < 0.001] and was 36% higher in individuals fed Plantago vs. Mimulus [Figure 2A, F(1, 84) = 8.34, p = 0.005]. However, there was no significant interaction between instar and host plant [F(1, 84) = 0.601, p = 0.550], suggesting that the effect of host plant on hemocyte density was consistent across caterpillar development. Despite consistently higher hemocyte density when fed Plantago, there was no main effect of host plant on melanization and no overall relationship between hemocyte density and melanization (see Table 1). However, melanization varied significantly between instars and there was a significant interaction between instar and host plant (Figure 2B), wherein melanization increased across instars in caterpillars reared on Mimulus, but not when reared on Plantago. There was also a significant interaction between instar and hemocyte density, where melanization increased with increasing hemocyte density in third and fourth instars but not in fifth (Figure 2C). However, there was no interaction between host plant and hemocyte density and no three-way interaction between host plant, instar, and hemocyte density, suggesting little indirect effect of host plant on immune function through altered hemocyte function.
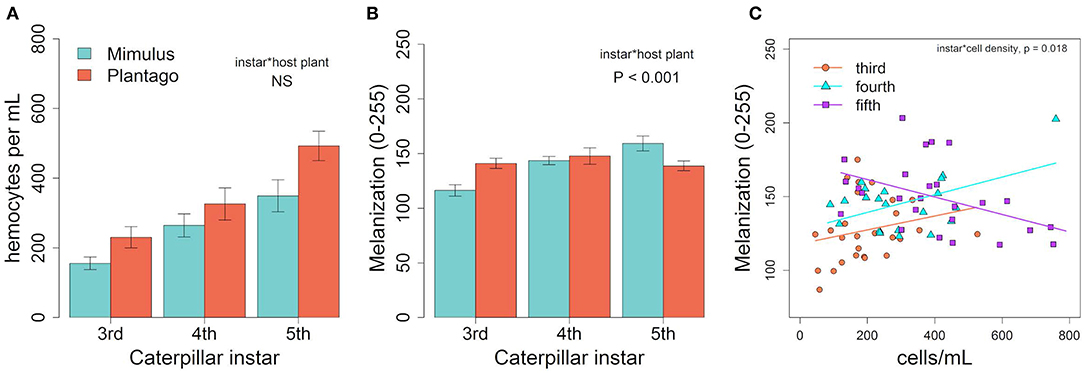
Figure 2. In January, (A) hemocyte density varied by instar and was higher on Plantago though the interaction was not significant. (B) There was significant interaction between host plant and instar, suggesting melanization increased with instar when fed Mimulus but did not change when fed Plantago. (C) A significant interaction between instar and hemocyte density suggested that melanization increased with cell density in third and fourth instars, but decreased with increasing cell density in fifth instar caterpillars. Lines represent predicted linear relationships for each instar. Bars represent ± 1 SE.
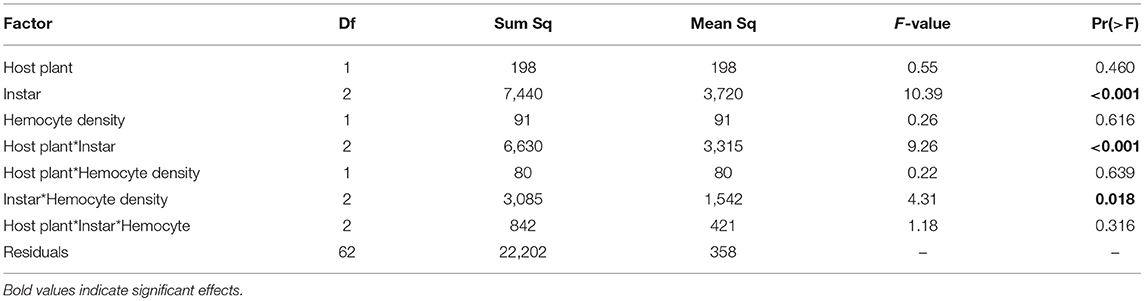
Table 1. Results from ANCOVA in the January experiment included a main effect of caterpillar developmental instar on melanization, as well as interactions between host plant and instar and instar and hemocyte density.
Experiment 2 (September)
Hemocyte density was 23% higher on average in September than January (373 vs. 303 cells per mL on average, respectively) and results differed. We found no differences in hemocyte density between caterpillar instars (F(2, 84) = 2.00, p = 0.142), no effect of host plant [F(1, 84) = 0.85, p = 0.359], and no interaction between host plant and caterpillar instar [Figure 3A, F(2, 84) = 0.29, p = 0.749, Figure 3A]. While there was no main effects of either host plant or instar on melanization, there was a significant interaction between host plant and instar (Table 2), with 5th instars having 16% less melanization on average when raised on Plantago vs. Mimulus, a pattern similar to January (Figure 3B). However, we found no other two-way or three-way interactions (Table 2).
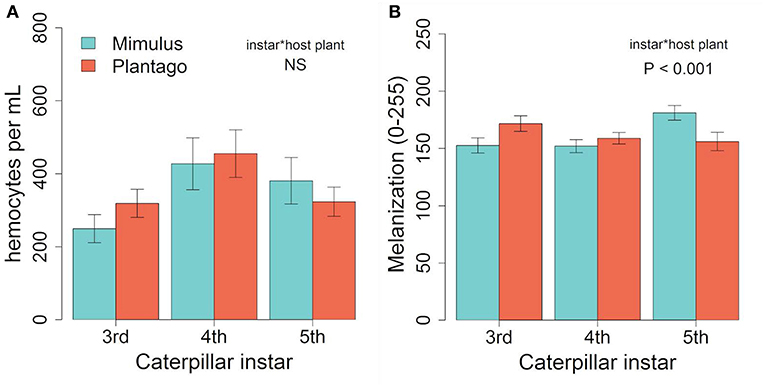
Figure 3. In September, (A) we found no significant effects of host plant or instar on hemocyte density. However, similar to January (B) melanization varied across instars when reared on Mimulus but not when reared on Plantago. Bars represent ± 1 SE.
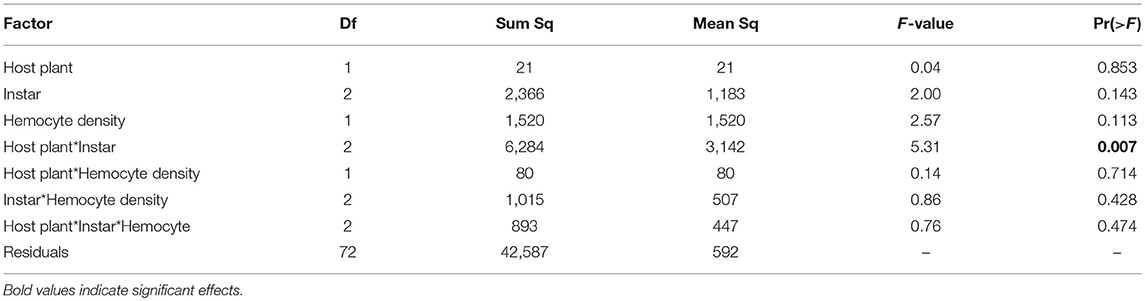
Table 2. In September ANCOVA indicated only an interaction between host plant and caterpillar developmental instar on melanization.
IG Sequestration in Plantago-Reared Caterpillars
Experiment 1 (January)
Caterpillars sequestered both aucubin and catalpol and in concentrations more than twice that of Plantago plants (Figure 4A). In January, the percent dry weight total IGs sequestered varied across larval instars [F(2, 42) = 28.03, P < 0.001], with 4th instars sequestering the highest levels of IGs, over three times the concentrations of 3rd instars, and more than 80% higher than 5th instars (Figure 4A). ANCOVA revealed no direct effects of IG sequestration on hemocyte density or melanization (Table 3). However, there was a significant interaction between total IG sequestration and hemocyte density on melanization, but the relationships did not vary by caterpillar instar as there were no two- or three-way interactions. Immune challenged caterpillars sequestered a mean of 76% more total IGs per dry weight than unchallenged caterpillars [F(1, 84) = 14.13, P < 0.001, Figure 4A]. Although sequestration varied by instar [F(2, 84) = 22.26, P < 0.001], and there was a significant interaction between instar and immune challenge [F(2, 84) = 9.06, P < 0.001], with fourth instars sequestering over two and half times more IGs than controls, much more than either third or fifth instars (Figure 4). The proportion of total IGs sequestered that was catalpol was also 35% lower in immune challenged compared to unchallenged caterpillars [F(1, 82) = 20.28, P < 0.001]. Though the proportion of catalpol varied by instar [F(2, 82) = 14.5, P < 0.001], and there was a significant interaction between instar and immune challenge [F(2, 82) = 6.29, P = 0.003], with third instars sequestering similar proportions between challenged and control caterpillars (0.37 and 0.38, respectively), and fourth and fifth instars sequestering roughly half the proportion of catalpol when immune challenged (0.15 vs. 0.29 and 0.17 vs. 0.37, respectively, Figure 4A).
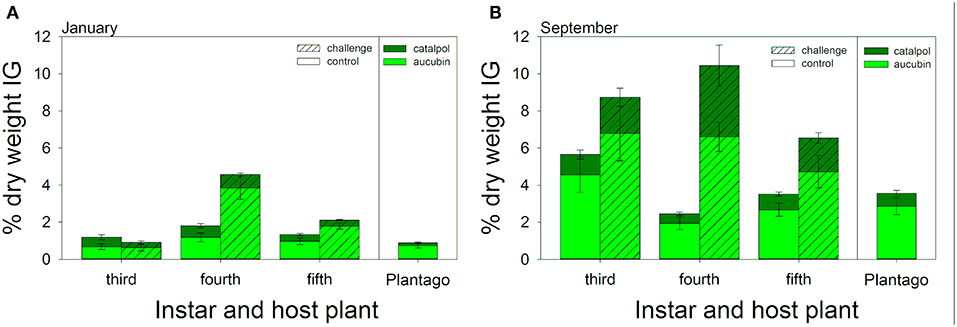
Figure 4. Composite figures illustrate multiple significant interactions between caterpillar instar and immune challenge on both total iridoid glycoside sequestrations (bar height) and the proportion of sequestered IGs that was catalpol (dark green). Results from the January (A) and September (B) experiments are included: right panels illustrate chemistry of sampled Plantago used for feeding during each experimental period. Bars represent ± 1 SE. See Results for statistical tests.
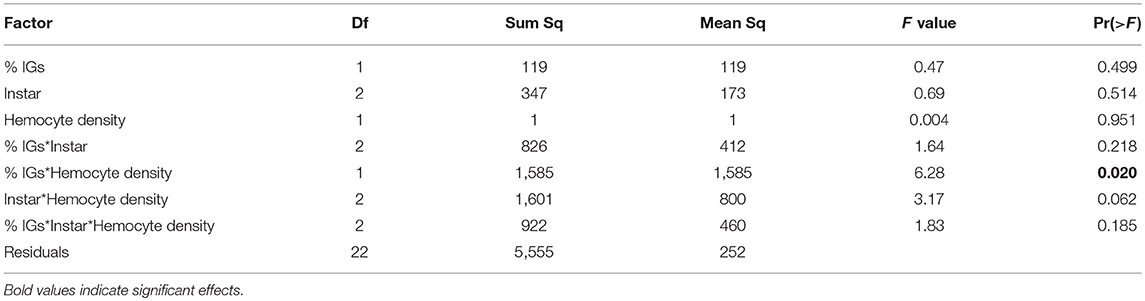
Table 3. For caterpillars reared on Plantago in January, ANCOVA showed an interaction between percent dry weight total IGs sequestered and hemocyte density on melanization.
Experiment 2 (September)
In September, caterpillars sequestered over 3.5 times more IGs than in January (8.89% dry weight on average compared to 2.53% dry weight in January). While IG sequestration was much higher, it was also more variable, and there was no significant difference in total IG sequestration between instars [F(2, 38) = 1.12, P = 0.336, Figure 4B]. Similar to January, ANCOVA revealed little direct effects of either instar, hemocyte density, or IG sequestration on melanization (Table 4). However, there was a significant interaction between sequestration, hemocyte density, and instar, suggesting that, although melanization tended to increase with hemocyte density, it declined with sequestration, and the relationship varied across caterpillar development. Similar to results from January, immune challenged caterpillars sequestered 75% more IGs than unchallenged controls [F(1, 86) = 9.02, P = 0.004] but the interaction between instar and immune challenge was not significant [F(1, 86) = 2.63, P = 0.110, Figure 4B]. The proportion of catalpol sequestered also varied across instars [F(1, 84) = 25.95, P < 0.001] and treatment [F(1, 84) = 19.41, P < 0.001], although there was a significant interaction between instar and immune challenge [F(1, 84) = 7.10, P = 0.009], driven mostly by 4th and 5th instars sequestering 43 and 17% higher proportions of catalpol, compared to 3rd instars which sequestered 19% lower proportion catalpol when immune challenged (Figure 4B).
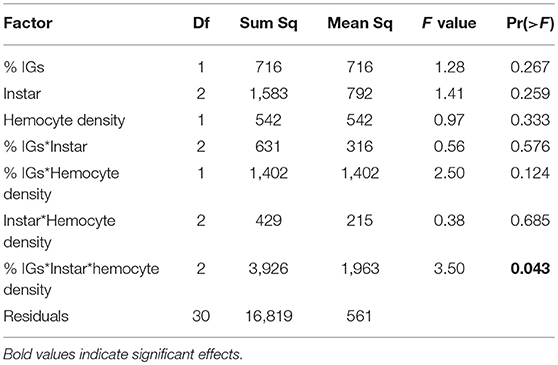
Table 4. For caterpillars reared on Plantago in September, ANCOVA revealed a three-way interaction between percent dry weight total IGs sequestered, hemocyte density, and caterpillar instar on melanization.
Experiment 3 (March)
In this experiment comparing the different immune challenge treatments with a control, sequestered IGs were generally low, although caterpillars sequestered IGs at nearly five times higher concentrations than the Plantago on which they were reared (Figure 5). We found a significant effect of immune challenge treatment on total IG sequestration [F(3, 57) = 6.43, P < 0.001, Figure 5], but not on the proportion of total IGs sequestered that was catalpol [F(3, 57) = 1.03, P = 0.387). Post-hoc tests showed that as expected, drawing hemolymph led to a 25% reduction in IG concentrations on average, although this difference was not significant (Figure 5). After 24 h IG concentrations were similar to controls, and these three treatments were not significantly different (Figure 5). Interestingly, when challenged with hemolymph withdrawal and insertion of a filament, caterpillars sequestered 47% more IGs than controls, which was a significant difference.
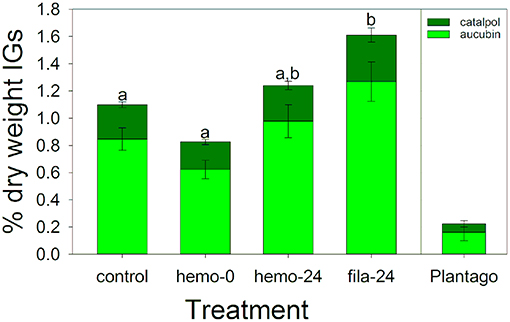
Figure 5. Iridoid glycoside sequestration during the March experiment testing the effects of immune challenge showed no significant difference in the proportion catalpol sequestered. However, total IG sequestration was similar between controls and caterpillars where hemolymph was sampled, but increase when immune challenged with a nylon filament. Letters are significant groups from a Tukey post-hoc test of % dry weight IGs. Bars represent ± 1 SE.
Discussion
Overall, we found that caterpillars reared on an introduced host had higher levels of cellular defenses than when reared on a native host, but that those defenses do not appear to translate into increased immune function. Moreover, the relationships between host plant, cellular defenses, and melanization vary through time, and are likely driven in part by sequestration of plan secondary metabolites. Still, these results suggest that introduced host plants can impact native herbivores through stage-specific immunological responses, and that variation in introduced host plant chemistry can mediate the relationships between sequestration and immune function. Interestingly, these results also indicated that immune challenge can change caterpillar sequestration abilities, suggesting that chemically-mediated defense against natural enemies could play some role in the potential switch to introduced hosts. That the patterns detected varied across the course of the study and corresponded to differences in host plant chemistry suggest that the ecological conditions that herbivores and their host plants experience likely have complex consequences for sequestration and defense.
In general, while stage-specific (e.g., Meylaers et al., 2007; Stoepler et al., 2013) and within stadium (e.g., Hoover et al., 2002; McNeil et al., 2010) immunity in insects has been observed, intra-annual variation and across different instars is less well-understood. The results from immune assays in Experiment 1 (January) suggested that immunity, as measured by hemocyte density, varies across instars, increasing with caterpillar development. In contrast, hemocyte density was 23% higher on average and much more variable in Experiment 2 (September), resulting in no significant differences between instars. This could be due in part to differences in caterpillar response to host plants between time periods: J. coenia larvae feeding on Plantago in January had higher hemocyte density than those feeding on Mimulus; although, in September, there were no differences. Host plant quality may vary across a growing season (Boege and Marquis, 2005; Hanley et al., 2007; Barton and Koricheva, 2010; Quintero et al., 2014) and differences between alternative hosts could be magnified by seasonal variation in host plant quality or chemistry. Alternatively, hemocyte density may not be the best metric of immunity. For example, Bauer et al. (1998) found no differences in hemocyte density across Pieris brassicae development, although total hemocytes increased with instar, in line with dramatic increases in hemolymph volume as caterpillars grew (Bauer et al., 1998). Still, the fact that encapsulation similarly changed in magnitude between time periods, with encapsulation being 13% higher in September, suggests that hemocyte density was an underlying mechanism driving immune function.
Moreover, results from our encapsulation assays suggest that immune function, as measured through melanization, varied across caterpillar instars depending on host plant. In both Experiment 1 and 2, melanization increased across larval instars when reared on Mimulus, but not when reared on Plantago. The results were that third and fourth instar caterpillars feeding on Plantago had higher immune function than those feeding on Mimulus, while fifth instars fed Mimulus had the highest immune function and more so than fifth instars reared on Plantago. This suggests that tradeoffs in immune function across caterpillar development are likely host plant specific, although we detected no three-way interaction between host plant, instar, and hemocyte density in January or September. It should be noted that melanization is the result of both cellular and humoral responses of the insect immune system (Strand, 2008), although we did not measure humoral responses. However, changes in immune function over caterpillar development can be driven by hemocyte differentiation within the immune system occurring later in development (Brodeur and Vet, 1995; Gillespie et al., 1997), potentially an evolutionary response to pressure from parasitoids targeting larger caterpillars (Memmott et al., 2000). We found a significant interaction between instar and hemocyte density in January, but melanization actually decreased with hemocyte density in fifth instars, while increasing with hemocyte density in third and fourth instars. Hemocyte titers have been shown to decline during rapid growth of final caterpillar instars (Beetz et al., 2008). That fifth instars in Experiment 2 (September) had lower hemocyte densities, could suggest that fifth instars exhibit compensatory growth to immune challenge instead of investing in immune defense. Given that the development of immune function in our study varied as a result of host plant, one impact of introduced hosts could therefore be through altering stage-specific susceptibility to natural enemies.
Plant chemistry is a fundamental component of host quality for sequestering herbivores and can impact immunity, posing potential tradeoffs in defense against natural enemies (Gentry and Dyer, 2002). In buckeyes for example, Smilanich et al. (2009a) demonstrated that larvae fed on a diet with higher levels of iridoid glycosides are more poorly defended against parasitoids while being better protected against predators. The results from our study showed that IG sequestration varies across instars and time periods, and this can have implications for immune function, although relationships may be complex and vary through time. In general, IG sequestration was low in January, just 2.5% dry weight on average, compared to 8.9% dry weight on average in September. This was driven by extremely low IG concentrations of Plantago in January compared to September (0.89 vs. 4.8% dry weight IG, respectively), and lower than previous studies (Bowers and Stamp, 1992). By comparison, Lampert and Bowers (2010) found that buckeye larvae in the fourth instar sequestered 5 to 15% dry weight IGs, and larvae can sequester up to up to 25% of their dry weight (Theodoratus and Bowers, 1999). Regardless, in both January and September, fourth instar immune challenged caterpillars had the highest concentrations of IGs relative to third and fifth instars, which could suggest that the costs of sequestering IGs vary across caterpillar development. Moreover, the relationships between sequestration and immune function varied across time periods. We found little evidence of IGs directly impacting immune function in either time periods. However, when IGs were higher in September, we found a significant three-way interaction between sequestration, caterpillar instar, and hemocyte density on melanization. This interaction suggested that the effect of hemocyte density on melanization could be impacted by high IG sequestration and that any costs of sequestration on immune function likely vary across instars. This could be illustrative of stage-specific tradeoffs in sequestration and immune function.
Interestingly, we also found that immune challenged caterpillars from both Experiments 1 and 2 actually sequestered higher IG concentrations relative to unchallenged controls, and in all instars except third instars in Experiment 2 (Figure 4). Furthermore, it appears that this is not due to the methods we used, given the results of Experiment 3, in which we compared several different immune challenge treatments (Figure 5). The results of that experiment showed a short-term reduction in IGs due to hemolymph loss (although the difference was not significant), followed by a return to control levels after 24 h (during which time caterpillars were feeding). Most importantly, our results showed that when hemolymph was removed and a filament inserted (immune challenged), after 24 h caterpillars had sequestered significantly higher concentrations of IGs. The mechanism driving this is unclear; it could be due to compensatory feeding or an increase in active transport of IGs from the gut to the hemolymph. Alternatively, it may not be an adaptive response, but due to underlying regulatory pathways. For example, in pollen beetles injected with bacteria and yeast, there were concurrent upregulation of stress and detoxification-related genes along with known immune-related genes (Vogel et al., 2014). Still, that fourth instars exhibited higher responses than third and fifth, and in both the January and September experiments, suggests that potential costs associated with sequestration vary across caterpillar development. Further experiments to tease apart mechanisms driving such stage-specific tradeoffs in sequestration and defense could help improve our understanding of herbivore response to introduced hosts.
Without doubt, exotic plant species can have large impacts on ecological communities across the globe (Daehler, 1998; Mack et al., 2000), including through altering herbivore-host plant interactions (Bowers et al., 1992b), impacting the physiology, growth, and fitness of herbivores (Dyer, 1995; Knerl and Bowers, 2013), and with particularly large effects on larval stages with limited mobility (Graves and Shapiro, 2003). These in turn, can also affect tritrophic interactions, depending on how natural enemies respond to host plant mediated chemical defenses (Knerl and Bowers, 2013), but also potentially on how tradeoffs associated with chemical defenses vary across herbivore development. The results of this study suggest that the impacts of novel host chemistry across larval development are likely complex and dependent on variation in host plant chemistry, and on stage-specific relationships between sequestration and defense. Future studies manipulating host plant chemistry could help disentangle the mechanisms driving tradeoffs in defense strategies across caterpillar development and how introduced hosts impact tritrophic interactions.
Data Availability
The datasets generated for this study are available on request to the corresponding author.
Author Contributions
The initial research was conceptualized by ME and MB. ME and AC conducted experiments. AC conducted statistical tests and graphical representation of results. All authors contributed to manuscript preparation.
Funding
This research was supported by grants from the University of Colorado Undergraduate Research Opportunity Program, the Marion and Gordon Alexander Fellowship, and the National Science Foundation (award #IOS 1456338).
Conflict of Interest Statement
The authors declare that the research was conducted in the absence of any commercial or financial relationships that could be construed as a potential conflict of interest.
Acknowledgments
We thank N. Pepen and F. Hasandokht for assisting with experiments, A. Smilanich and N. Muchoney for advice on immunological assays, and the Bowers lab for comments and suggestions on the manuscript.
References
Barbosa, P., and Caldas, A. (2007). Do larvae of species in macrolepidopteran assemblages share traits that influence susceptibility to parasitism? Environ. Entomol. 36, 329–336. doi: 10.1093/ee/36.2.329
Barton, K. E., and Koricheva, J. (2010). The ontogeny of plant defense and herbivory: characterizing general patterns using meta-analysis. Am. Natural. 175, 481–493. doi: 10.1086/650722
Bauer, E., Trenczek, T., and Dorn, S. (1998). Instar-dependent hemocyte changes in Pieris brassicae after parasitization by Cotesia glomerata. Entomol. Exp. Et Appl. 88, 49–58. doi: 10.1046/j.1570-7458.1998.00345.x
Beckage, N. E. (2008). Epilogue: Pathways into the Future of Insect Immunology in Insect Immunity.331-336. San Diego, CA: Academic Press.
Beetz, S., Holthusen, T. K., Koolman, J., and Trenczek, T. (2008). Correlation of hemocyte counts with different developmental parameters during the last larval instar of the tobacco hornworm, Manduca sexta. Arch. Insect Biochem. Physiol. 67, 63–75. doi: 10.1002/arch.20221
Boege, K., and Marquis, R. J. (2005). Facing herbivory as you grow up: the ontogeny of resistance in plants. Trends Ecol. Evol. 20, 441–448. doi: 10.1016/j.tree.2005.05.001
Bowers, M. D. (1984). Iridoid glycosides and host-plant specificity in larvae of the buckeye butterfly, Junonia coenia (Nymphalidae). J. Chem. Ecol. 10, 1567–1577. doi: 10.1007/BF00988425
Bowers, M. D. (1990). “Recycling plant natural products for insect defense,” in Insect Defenses: Adaptive Mechanisms and Strategies of Prey and Predators, eds D. L. Evans and J. O. Schmidt (Albany, NY: SUNY Press), 353–386.
Bowers, M. D. (1992). “The evolution of unpalatability and the cost of chemical defense in insects,” in Insect Chemical Ecology: an Evolutionary Approach, eds B. D Roitberg and M. B. Isman (New York, NY: Chapman and Hall),216–244.
Bowers, M. D. (2003). “Coevolution of insects and plants,” in Encyclopedia of Plant and Crop Science, eds M. Kogan and S. Rao (New York, NY: Marcel Dekker Inc), 289–291. doi: 10.1081/E-EPCS-120010481
Bowers, M. D., and Collinge, S. K. (1992). Fate of iridoid glycosides in different life stages of the buckeye, Junonia coenia (Lepidoptera, Nymphalidae). J. Chem. Ecol. 18, 817–831. doi: 10.1007/BF00988322
Bowers, M. D., Collinge, S. K., Gamble, S. E., and Schmitt, J. (1992a). Effects of genotype, habitat, and seasonal-variation on iridoid glycoside content of Plantago lanceolata (Plantaginaceae) and the implications for insect herbivores. Oecologia 91, 201–207. doi: 10.1007/BF00317784
Bowers, M. D., and Puttick, G. M. (1986). Fate of ingested iridoid glycosides in lepidopteran herbivores. J/ Chem. Ecol. 12, 169–178. doi: 10.1007/BF01045600
Bowers, M. D., and Puttick, G. M. (1988). Response of generalist and specialist insects to qualitative allelochemical variation. J. Chem. Ecol. 14, 319–334. doi: 10.1007/BF01022549
Bowers, M. D., and Stamp, N. E. (1992). Chemical variation within and between individuals of Plantago lanceolata (Plantaginaceae). J. Chem. Ecol. 18, 985–995. doi: 10.1007/BF00980057
Bowers, M. D., and Stamp, N. E. (1997). Fate of host-plant iridoid glycosides in lepidopteran larvae of Nymphalidae and Arctiidae. J. Chem. Ecol. 23, 2955–2965. doi: 10.1023/A:1022535631980
Bowers, M. D., Stamp, N. E., and Collinge, S. K. (1992b). Early stage of host range expansion by a specialist herbivore, Euphydryas phaeton (Nymphalidae). Ecology 73, 526–536. doi: 10.2307/1940758
Brodeur, J., and Vet, L. E. M. (1995). Relationships between parasitoid host-range and host-defense: a comparative-study of egg encapsulation in 2 related parasitoid species. Physiol. Entomol. 20, 7–12. doi: 10.1111/j.1365-3032.1995.tb00794.x
Brower, L. P., Ryerson, W. N., Coppinger, L., and Glazier, S. C. (1968). Ecological chemistry and palatability spectrum. Science 161, 1349–1350. doi: 10.1126/science.161.3848.1349
Cavers, P. B., Bassett, I. J., and Crompton, C. W. (1980). The biology of canadian weeds.47. Plantago lanceolata L. Can. J. Plant Sci. 60, 1269–1282. doi: 10.4141/cjps80-180
Daehler, C. C. (1998). The taxonomic distribution of invasive angiosperm plants: ecological insights and comparison to agricultural weeds. Biol. Conserv. 84, 167–180. doi: 10.1016/S0006-3207(97)00096-7
Decker, L. E., de Roode, J. C., and Hunter, M. D. (2018). Elevated atmospheric concentrations of carbon dioxide reduce monarch tolerance and increase parasite virulence by altering the medicinal properties of milkweeds. Ecol. Lett. 21, 1353–1363. doi: 10.1111/ele.13101
Dobler, S., Petschenka, G., and Pankoke, H. (2011). Coping with toxic plant compounds: the insect's perspective on iridoid glycosides and cardenolides. Phytochemistry 72, 1593–1604. doi: 10.1016/j.phytochem.2011.04.015
Duff, R. B., Bacon, J. S. D., Mundie, C. M., Farmer, V. C., Russell, J. D., and Forreste, Ar. (1965). Catalpol and methylcatalpol: naturally occurring glycosides in Plantago and Buddleia species. Biochem. J. 96, 1–5. doi: 10.1042/bj0960001
Dyer, L. A. (1995). Tasty generalists and nasty specialists? antipredator mechanisms in tropical lepidopteran larvae. Ecology 76, 1483–1496. doi: 10.2307/1938150
Gentry, G. L., and Dyer, L. A. (2002). On the conditional, nature of neotropical caterpillar defenses against their natural enemies. Ecology 83, 3108–3119. doi: 10.1890/0012-9658(2002)083[3108:OTCNON]2.0.CO;2
Gherlenda, A. N., Haigh, A. M., Moore, B. D., Johnson, S. N., and Riegler, M. (2016). Climate change, nutrition and immunity: effects of elevated CO2 and temperature on the immune function of an insect herbivore. J. Insect Physiol. 85, 57–64. doi: 10.1016/j.jinsphys.2015.12.002
Gillespie, J. P., Kanost, M. R., and Trenczek, T. (1997). Biological mediators of insect immunity. Annual Rev. Entomol. 42, 611–643. doi: 10.1146/annurev.ento.42.1.611
Graves, S. D., and Shapiro, A. M. (2003). Exotics as host plants of the California butterfly fauna. Biol. Conserv. 110, 413–433. doi: 10.1016/S0006-3207(02)00233-1
Hanley, M. E., Lamont, B. B., Fairbanks, M. M., and Rafferty, C. M. (2007). Plant structural traits and their role in anti-herbivore defence. Perspect. Plant Ecol. Evol. Syst. 8, 157–178. doi: 10.1016/j.ppees.2007.01.001
Hartmann, T. (2004). Plant-derived secondary metabolites as defensive chemicals in herbivorous insects: a case study in chemical ecology. Planta 219, 1–4. doi: 10.1007/s00425-004-1249-y
Holeski, L. M., Chase-Alone, R., and Kelly, J. K. (2010). The genetics of phenotypic plasticity in plant defense: trichome production in Mimulus guttatus. Am. Natural. 175, 391–400. doi: 10.1086/651300
Holeski, L. M., Keefover-Ring, K., Bowers, M. D., Harnenz, Z. T., and Lindroth, R. L. (2013). Patterns of phytochemical variation in Mimulus guttatus (yellow monkeyflower). J. Chem. Ecol. 39, 525–536. doi: 10.1007/s10886-013-0270-7
Hoover, K., Grove, M. J., and Su, S. (2002). Systemic component to intrastadial developmental resistance in Lymantria dispar to its baculovirus. Biol. Control 25, 92–98. doi: 10.1016/S1049-9644(02)00041-5
Jahner, J. P., Bonilla, M. M., Badik, K. J., Shapiro, A. M., and Forister, M. L. (2011). Use of exotic hosts by lepidoptera: widespread species colonize more novel hosts. Evolution 65, 2719–2724. doi: 10.1111/j.1558-5646.2011.01310.x
Jamieson, M. A., Schwartzberg, E. G., Raffa, K. F., Reich, P. B., and Lindroth, R. L. (2015). Experimental climate warming alters aspen and birch phytochemistry and performance traits for an outbreak insect herbivore. Glob Change Biol. 21, 2698–2710. doi: 10.1111/gcb.12842
Jeffs, C. T., and Lewis, O. T. (2013). Effects of climate warming on hostparasitoid interactions. Ecol. Entomol. 38, 209–218. doi: 10.1111/een.12026
Knerl, A., and Bowers, M. D. (2013). Incorporation of an introduced weed into the diet of a native butterfly: consequences for preference, performance and chemical defense. J. Chem. Ecol. 39, 1313–1321. doi: 10.1007/s10886-013-0355-3
Kooiman, P. (1970). Occurrence of iridoid glycosides in Scrophulariaceae. Acta Botan. Neerland. 19, 329–330. doi: 10.1111/j.1438-8677.1970.tb00655.x
Lampert, E. C., and Bowers, M. D. (2010). Host plant species affects the quality of the generalist Trichoplusia ni as a host for the polyembryonic parasitoid Copidosoma floridanum. Entomol. Exp. Appl. 134, 287–295. doi: 10.1111/j.1570-7458.2009.00956.x
Lavine, M. D., and Strand, M. R. (2002). Insect hemocytes and their role in immunity. Insect Biochem. Molecul. Biol. 32, 1295–1309. doi: 10.1016/S0965-1748(02)00092-9
Mack, R. N., Simberloff, D., Lonsdale, W. M., Evans, H., Clout, M., and Bazzaz, F. A. (2000). Biotic invasions: causes, epidemiology, global consequences, and control. Ecol. Appl. 10, 689–710. doi: 10.1890/1051-0761(2000)010[0689:BICEGC]2.0.CO;2
McNeil, J., Cox-Foster, D., Slavicek, J., and Hoover, K. (2010). Contributions of immune responses to developmental resistance in Lymantria dispar challenged with baculovirus. J. Insect Physiol. 56, 1167–1177. doi: 10.1016/j.jinsphys.2010.03.020
Memmott, J., Martinez, N. D., and Cohen, J. E. (2000). Predators, parasitoids and pathogens: species richness, trophic generality and body sizes in a natural food web. J. Anim. Ecol. 69, 1–15. doi: 10.1046/j.1365-2656.2000.00367.x
Meylaers, K., Freitak, D., and Schoofs, L. (2007). Immunocompetence of Galleria mellonella: sex- and stage-specific differences and the physiological during cost of mounting an immune response metamorphosis. J. Insect Physiol. 53, 146–156. doi: 10.1016/j.jinsphys.2006.11.003
Nappi, A. J., and Christensen, B. M. (2005). Melanogenesis and associated cytotoxic reactions: applications to insect innate immunity. Insect Biochem. Molecul. Biol. 35, 443–459. doi: 10.1016/j.ibmb.2005.01.014
Pech, L. L., and Strand, M. R. (1996). Granular cells are required for encapsulation of foreign targets by insect haemocytes. J. Cell Sci. 109, 2053–2060.
Pereyra, P. C., and Bowers, M. D. (1988). Iridoid glycosides as oviposition stimulants for the buckeye butterfly, Junonia coenia (Nymphalidae). J. Chem. Ecol. 14, 917–928. doi: 10.1007/BF01018783
Poreddy, S., Mitra, S., Schöttner, M., Chandran, J., Schneider, B., Baldwin, I. T., et al. (2015). Detoxification of hostplant's chemical defence rather than its anti-predator co-option drives β-glucosidase-mediated lepidopteran counteradaptation. Nat. Commun. 6:8525. doi: 10.1038/ncomms9525
Quintero, C., Lampert, E. C., and Bowers, M. D. (2014). Time is of the essence: direct and indirect effects of plant ontogenetic trajectories on higher trophic levels. Ecology 95, 2589–2602. doi: 10.1890/13-2249.1
R. Core Team (2017). R: A Language and Environment For Statistical Computing. R Foundation for Statistical Computing. Vienna, Austria
Rosenheim, J. A. (1998). Higher-order predators and the regulation of insect herbivore populations. Ann. Rev. Entomol. 43, 421–447. doi: 10.1146/annurev.ento.43.1.421
Smilanich, A. M., Dyer, L. A., Chambers, J. Q., and Bowers, M. D. (2009a). Immunological cost of chemical defence and the evolution of herbivore diet breadth. Ecol. Lett. 12, 612–621. doi: 10.1111/j.1461-0248.2009.01309.x
Smilanich, A. M., Dyer, L. A., and Gentry, G. L. (2009b). The insect immune response and other putative defenses as effective predictors of parasitism. Ecology 90, 1434–1440. doi: 10.1890/08-1906.1
Smilanich, A. M., Langus, T. C., Doan, L., Dyer, L. A., Harrison, J. G., Hsueh, J., et al. (2018). Host plant associated enhancement of immunity and survival in virus infected caterpillars. J. Invert. Pathol. 151, 102–112. doi: 10.1016/j.jip.2017.11.006
Stamp, N. E. (1986). Physical constraints of defense and response to invertebrate predators by pipevine caterpillars Battus philenor Papilionidae. J. Lepidopterists' Soc. 40, 191–205.
Stanley, D., Haas, E., and Miller, J. (2012). Eicosanoids: exploiting insect immunity to improve biological control programs. Insects 3, 492–510. doi: 10.3390/insects3020492
Stoepler, T. M., Castillo, J. C., Lill, J. T., and Eleftherianos, I. (2013). Hemocyte density increases with developmental stage in an immune-challenged forest caterpillar. PLoS ONE 8:e0157882. doi: 10.1371/journal.pone.0070978
Strand, M. R. (2008). The insect cellular immune response. Insect Sci. 15, 1–14. doi: 10.1111/j.1744-7917.2008.00183.x
Strand, M. R., Beck, M. H., Lavine, M. D., and Clark, K. D. (2006). Microplitis demolitor bracovirus inhibits phagocytosis by hemocytes from Pseudoplusia includens. Arch. Insect Biochem. Physiol. 61, 134–145. doi: 10.1002/arch.20107
Theodoratus, D. H., and Bowers, M. D. (1999). Effects of sequestered iridoid glycosides on prey choice of the prairie wolf spider, Lycosa carolinensis. J. Chem. Ecol. 25, 283–295. doi: 10.1023/A:1020894729188
Triggs, A., and Knell, R. J. (2012). Interactions between environmental variables determine immunity in the Indian meal moth Plodia interpunctella. J. Anim. Ecol. 81, 386–394. doi: 10.1111/j.1365-2656.2011.01920.x
Vidal, M. C., and Murphy, S. M. (2018). Bottom-up vs. top-down effects on terrestrial insect herbivores: a meta-analysis. Ecol. Lett. 21, 138–150. doi: 10.1111/ele.12874
Vogel, H., Badapanda, C., Knorr, E., and Vilcinskas, A. (2014). RNA-sequencing analysis reveals abundant developmental stage-specific and immunity-related genes in the pollen beetle Meligethes aeneus. Insect Molecul Biol. 23, 98–112. doi: 10.1111/imb.12067
Wago, H., and Ichikawa, Y. (1979). Changes in the phagocytic rate during the larval development and manner of hemocytic reactions to foreign cells in Bombyx mori. Appl. Entomol. Zool. 14, 397–403. doi: 10.1303/aez.14.397
Keywords: immune, ecoimmunology, sequestration, iridoid, Plantago, Junonia
Citation: Carper AL, Enger M and Bowers MD (2019) Host Plant Effects on Immune Response Across Development of a Specialist Caterpillar. Front. Ecol. Evol. 7:208. doi: 10.3389/fevo.2019.00208
Received: 07 March 2019; Accepted: 20 May 2019;
Published: 07 June 2019.
Edited by:
Gina Marie Wimp, Georgetown University, United StatesReviewed by:
Kelli Hoover, Pennsylvania State University, United StatesLiza Holeski, Northern Arizona University, United States
Copyright © 2019 Carper, Enger and Bowers. This is an open-access article distributed under the terms of the Creative Commons Attribution License (CC BY). The use, distribution or reproduction in other forums is permitted, provided the original author(s) and the copyright owner(s) are credited and that the original publication in this journal is cited, in accordance with accepted academic practice. No use, distribution or reproduction is permitted which does not comply with these terms.
*Correspondence: Adrian L. Carper, YWRyaWFuLmNhcnBlckBjb2xvcmFkby5lZHU=