- 1Department of Biological Sciences, Florida International University, Miami, FL, United States
- 2Department of Biology, McGill University, Montreal, QC, Canada
- 3Abt. Verhaltensphysiologie Philippstr, Institut für Biologie, Humboldt-Universität zu Berlin, Berlin, Germany
Eavesdropping by electroreceptive predators poses a conflict for weakly electric fish, which depend on their Electric Organ Discharge (EOD) signals both for navigation and communication in the dark. The EODs that allow weakly electric fish to electrolocate and communicate in the dark may attract electroreceptive predators such as catfishes and Electric Eels. These predators share with their prey the synapomorphy of passive electric sense supported by ampullary electroreceptors that are highly sensitive to low-frequency electric fields. Any low-frequency spectral components of the EOD make weakly electric fish conspicuous and vulnerable to attack from electroreceptive predators. Accordingly, most weakly electric fish shift spectral energy upwards or cloak low-frequency energy with compensatory masking signals. Subadults and females in particular emit virtually no low-frequency energy in their EODs, whereas courting males include a significant low-frequency component, which likely attracts females, but makes the signals conspicuous to predators. Males of species that coexist with the most predators tend to produce the least low-frequency signal energy, expressing sexual dimorphism in their signals in less risky ways. In these respects, electric signals follow the classic responses to opposing forces of natural and sexual selection, as exemplified in the visual signals of guppies and the acoustic signals of Túngara frogs. Unique to electric fish is that the electric signal modifications that help elude detection by electroreceptive predators are additions to the basal signal rather than losses of attractive components. These enhancements that enable crypsis are energetically costly, but have also provided the evolutionary substrates for subsequent sexual selection and species identity characters.
Introduction
Reproductive signals may be subject to balancing selection wherein sexual selection favors extravagant signals, while natural selection by predators provides a moderating counterforce (Maan and Seehausen, 2011). Researchers have documented a catalog of mechanisms by which animals reduce the problem of hostile eavesdroppers. Among these, shifting signal modes or signal frequencies are the most common ways to evade eavesdroppers of mate attraction signals. Hostile eavesdropping by predators has been speculated to lead to evolutionary arms races, wherein the signaler shifts its signal frequency out of the sensory range of the predator, and the predator falls under selection to shift its sensory range to continue detecting its prey (Verrell, 1991; Zuk and Kolluru, 1998). Such pressures can become extreme; unable to win a sensory arms race against parasitoid flies which locate field crickets by sound (Cade, 1975; Robert et al., 1992; Adamo et al., 1995), crickets in Hawaii evolved defective wing combs that render them totally silent in areas where the flies are common (Zuk et al., 2006).
Electric communication was discovered just over half a century ago (Lissmann, 1958), and is less well-studied than the other sensory modalities. However, electric communication signals appear to parallel the trends found for visual and acoustic signals with regard to the balance between conspicuous and cryptic signal forms. These patterns constitute the classic signature of opposing pressures between sexual selection and predation as forces shaping the signals. This research area remains fertile for discovery. The limited sensory physiology data on electric signals suggests vulnerability of some electric waveforms to predation, but the critical sensory information derives from study of temperate catfish species (rev. Finger, 1986) and are lacking for electrosensory systems of key tropical predators. Females of many extant electric fish species appear less conspicuous to predators than males, but we have no field observations showing preferential predation on males based on their signals. Stomach contents of electroreceptive predatory species sometimes suggests specialization on electric fish, but we do not have data showing that the predation is cued by the electric signals. Many electroreceptive predators have been overfished in their native habitat (Petrere et al., 2004), and their scarcity has made them hard to lure in the field with electric stimuli. Despite the gaps in our collective knowledge, in this paper we lay out the evidence we do have, that signals of electric fish have been, and continue to be, shaped by electroreceptive predators.
Electrogenic fish have evolved repeatedly, including torpedoes, skates, stargazers, gymnotiforms, several independent catfish lineages, and mormyroids (Bennett, 1970, 1971a; Bass, 1986; Hagedorn et al., 1990; Baron, 1994; Baron et al., 1994; Alves-Gomes, 2001). The Gymnotiformes radiated in South America, reaching from Mexico to Argentina (Albert and Crampton, 2005). The unrelated Mormyroidea (Osteoglossiformes) are found across sub-Saharan Africa, with the Mormyridae having radiated particularly broadly in western Africa (Sullivan et al., 2000). The Gymnotiformes and the Mormyroidea make up the weakly electric fishes, with each group counting around 200 species.
Weakly electric fish generate low-voltage electric fields that image the fish's surroundings in darkness (e.g., Engelmann et al., 2008) and allow these animals to communicate over short distances in support of sexually-selected behaviors serving courtship, male competition, and territorial defense (e.g., Henninger et al., 2018). The electric organ discharge (EOD) waveforms of many extant freshwater species have complex, polyphasic voltage waveforms (Hopkins and Heiligenberg, 1978; Hopkins, 1980). These waveforms appear to bear the signatures of historic selection for predator avoidance, mate attraction, and species isolation (Feulner et al., 2008, 2009; Arnegard et al., 2010; Crampton et al., 2011; Waddell et al., 2016). A cogent argument has been made that multiple signal phases reduced or eliminated the low-frequency power in the signals in response to selection by electroreceptive predators, providing the key substrates for subsequent evolution of sexual signaling and species isolation mechanisms (Stoddard, 1999, 2002a).
Electroreceptive Predators
Although uncommon as a mode of communication, electric fields do not constitute a private channel. Catfishes (order Siluriformes) are the sister group to the gymnotiform electric fishes. Although few catfish produce electric fields, all share electroreceptors by descent, creating the potential (no pun intended) for hostile electroreceptive eavesdropping by the piscivorous catfish species (Kalmijn, 1974). Catfish have radiated widely in the Neotropics and Sub-Saharan Africa, sympatric with the radiations of gymnotiform and mormyroid lineages of weakly electric fishes, respectively (Sullivan et al., 2006; Armbruster, 2011; Day et al., 2013). Although the diets of catfish are diverse, the large piscivorous species are ideally poised to shape the signals of sympatric weakly electric fish (Merron, 1993; Duque and Winemiller, 2003; Petrere et al., 2004).
All electroreceptive fishes express some sort of cutaneous ampullary electroreceptor (Bennett, 1971b; Bullock, 1982; Zakon, 1986). Ampullary electroreceptors of freshwater teleosts are highly sensitive to low-frequency electric fields (rev. Finger, 1986; Peters et al., 2007) ranging from 0 Hz (DC) up to approximately 100 Hz (Figure 1; Peters and Buwalda, 1972; Dunning, 1973). These data are derived from temperate catfish of the genus Ictalurus, and a gymnotiform electric fish of the genus Gymnotus, neither of which are predators of weakly electric fish. Similarity in the physiological response of their ampullary electroreceptors suggests a general vulnerability of EODs with low-frequency energy to piscivores with ampullary electroreceptors, but physiological studies should be conducted to confirm this assumption; limited anatomical data suggest that catfish that hunt weakly electric fish may have evolved higher frequency electroreception (Andres et al., 1988).
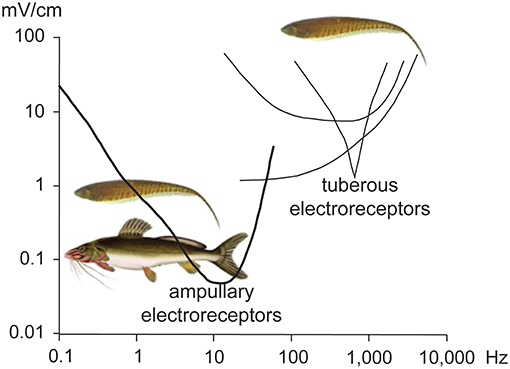
Figure 1. Tuning curves of the two general types of electroreceptors. All electroreceptive fishes have some sort of ampullary electroreceptors, which are extremely sensitive to low-frequency electric fields. Gymnotiform and mormyrid electric fishes have also evolved tuberous electroreceptors which are less sensitive and tuned to higher frequencies to detect the fish's own electric field and electric fields of nearby conspecifics. The ampullary receptor curve was recorded from a Gymnotus sp. (Dunning, 1973), and the tuberous receptor curves were recorded from Brachyhypopomus occidentalis (Shumway and Zelick, 1988). Adapted from Stoddard (2002a) with permission of the publisher.
Weakly electric fish have evolved additional classes of tuberous electroreceptors, tuned to the fish's own EOD and used in active electroreception and communication (revs. Hopkins, 1981, 1995). Tuberous electroreceptors respond to higher frequencies than ampullary electroreceptors, albeit with less sensitivity (Figure 1; Bennett, 1971b).
The key electroreceptive predators of weakly electric fishes appear to be their sister group Siluriformes, the catfishes. Stomach content analyses reveal that piscivorous catfishes of the Neotropical family Pimelodidae regularly prey upon weakly electric gymnotiforms (Reid, 1983; Duque and Winemiller, 2003; Petrere et al., 2004). The African Sharptooth Catfish, Clarias gariepinus has been documented actively hunting mormyrid electric fish (Merron, 1993). The Electric Eel (Electrophorus electricus), a strongly electric gymnotiform species, has been observed stalking weakly electric gymnotiforms in the field (Westby, 1988). In the lab, both Sharptooth Catfish and Electric Eels spontaneously attack electrodes playing signals of weakly electric fish, favoring those EODs that contain energy in the spectral range of ampullary electroreceptors (Hanika and Kramer, 1999, 2000; Stoddard, 1999). Stomach contents of Electric Eels collected in the Mamiraua reserve of Brazil included weakly-electric gymnotiforms along with a wide variety of aquatic vertebrates and invertebrates, suggesting no particular specialization on gymnotiform prey (W. Crampton, pers. com.). We conclude that piscivorous catfish are likely the primary electroreceptive predators of weakly electric fish, and that Electric Eels are opportunistic predators.
EOD Properties and Detectability by Predators
The waveform of the EOD determines its spectral energy content and thus its capacity to attract predators. The simplest EOD waveform is a monophasic pulse, resembling a single-period cosine rising off a zero-volt baseline. The energy spectrum of a single monophasic EOD pulse is dominated by low frequencies (Figure 2), which should make it particularly detectable by ampullary electroreceptors and thus attractive to electroreceptive predators. Monophasic pulsed EODs appear to be the ancestral condition in most or all electric fishes, both weak and strong (Bass, 1986; Kirschbaum, 1995; Alves-Gomes, 2001). Electric signals that have emerged in disparate fish lineages including skates, stargazers, mormyroids, gymnotiforms, and even a few catfishes, first appeared as monophasic pulsed EODs, except for synodontid catfish which make EODs from a modified swim bladder (Bass, 1986; Hagedorn et al., 1990; Baron et al., 1994; Sullivan et al., 2000; Boyle et al., 2014). Larval gymnotiforms and mormyrids produce monophasic EODs, gradually replacing them with polyphasic pulses that shift the frequency upwards as their electric organs mature through development (Figure 3; Franchina, 1997; Kirschbaum and Schugardt, 2002; Stoddard, 2002a; Crampton et al., 2011). Monophasic pulsed EODs are rare within the gymnotiform and mormyrid lineages, likely because their low-frequency bias attracts predators. Where monophasic species do occur, monophasy appears to be derived rather than plesiomorphic (Alves-Gomes and Hopkins, 1997; Arnegard et al., 2010; Lovejoy et al., 2010; De Santana and Crampton, 2011; Alda et al., 2013; Crampton et al., 2013, 2016). Evolutionary reemergence of monophasy in weakly electric fish is surprising, given the conspicuousness of monophasic signals to electroreceptive predators. Several monophasic Gymnotus species reside in low-predation refugia, and another in anoxic floating meadows not easily penetrated by predatory catfish (Crampton et al., 2013). By contrast, a common monophasic species from the Amazon, Brachyhypopomus bennetti, is subject to heavy tail-grazing, but does not change its EOD shape when the tail is damaged, a possible advantage for species discrimination in mixed-species assemblages (Sullivan et al., 2013), a recognized force shaping evolution of signal forms in electric fish (Crampton et al., 2011). The Electric Eel, which may be basal to the gymnotiform clade (Tagliacollo et al., 2016), retains a monophasic pulsed EOD. It can signal in the low-frequency spectrum with impunity because it can also generate a separate high-voltage discharge strong enough to repel any predator that tries to eat it (Faraday, 1839; Catania, 2016).
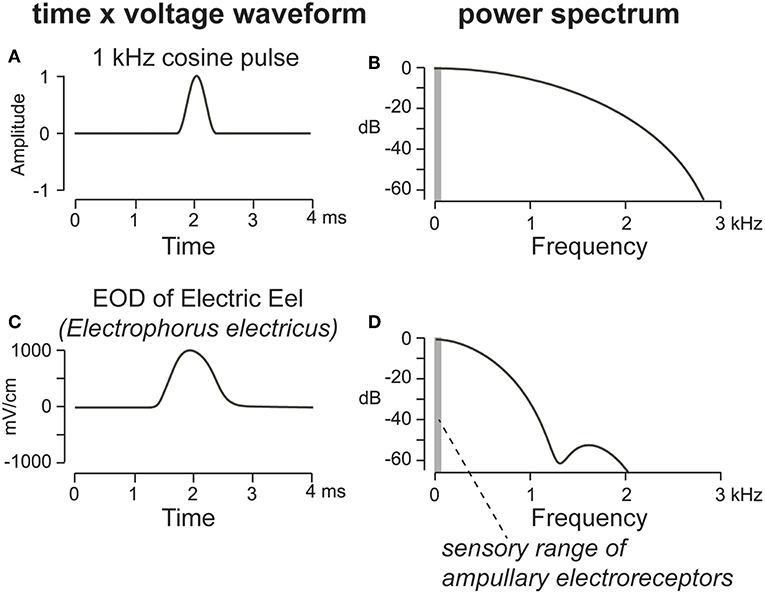
Figure 2. Shown here is a single-period cosine wave (A), its corresponding power spectrum (B), and the monophasic pulse EOD of an Electric Eel (C), the low-voltage signal it produces for electrolocation and communication, with its power spectrum (D). The energy spectrum of a monophasic EOD pulse (B or D) is dominated by low frequencies in the sensory range of ampullary electroreceptors (vertical gray bar) which should make it readily detectable by electroreceptive predators, including catfish and Electric Eels. Adapted from Stoddard and Markham (2008) with permission of the publisher.
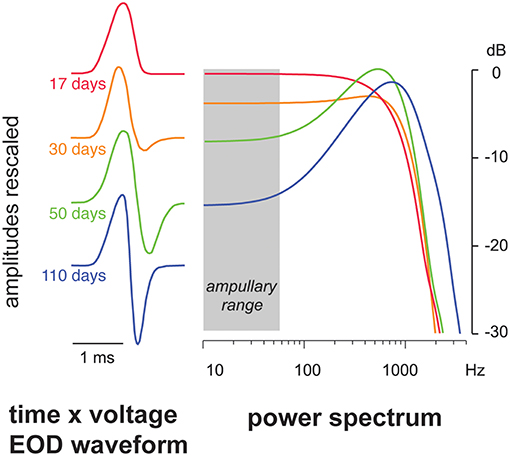
Figure 3. EOD ontogeny of the gymnotiform electric fish Brachyhypopomus gauderio (Franchina, 1997). The larval fish's initial EOD is a simple monophasic pulse with abundant energy in the spectral range of the ampullary electroreceptors (gray bar). Over the next 2 months the EOD transforms into a symmetrical biphasic pulse that suppresses the low-frequency spectrum and reduces detectability by ampullary electroreceptors. This transformation should reduce risk of predation from electroreceptive predators. Adapted from Stoddard and Markham (2008) with permission of the publisher.
To understand how weakly electric fish have shifted their signal frequencies away from the sensory range of predators, we first consider how electric field waveforms of different shapes distribute energy across the spectrum (Figure 4). The key feature is symmetry of the energy around the zero-volt baseline (Stoddard and Markham, 2008); any imbalance in the mean voltage will create a net-DC shift, which necessarily creates a low-frequency component that will stimulate ampullary electroreceptors of predators. A continuous sine wave, centered evenly around zero volts (Figure 4A), has energy at the fundamental frequency only, thus a 1,000 Hz sinewave has energy only at 1,000 Hz. As an electric field, this waveform would be imperceptible to ampullary electroreceptors of piscivorous predators, which are tuned much lower in frequency (Figure 1). However, if the same sine wave is offset up or down in amplitude from a center of zero volts (Figure 4B), a new spectral component emerges at 0 Hz that is readily detectable by ampullary electroreceptors. A single-period sinusoid centered around zero volts (Figure 4D) has a broader spectrum than the continuous sine wave, but little low-frequency energy, so a pulsed EOD with such a waveform would be undetectable by ampullary electroreceptors. As with the continuous sine wave, any DC asymmetry in the single-period sinusoid would likewise add energy to the low end of the spectrum, making the signal readily detectable by the ampullary system. The most extreme case of DC asymmetry is a monophasic EOD pulse (Figures 2, 4C).
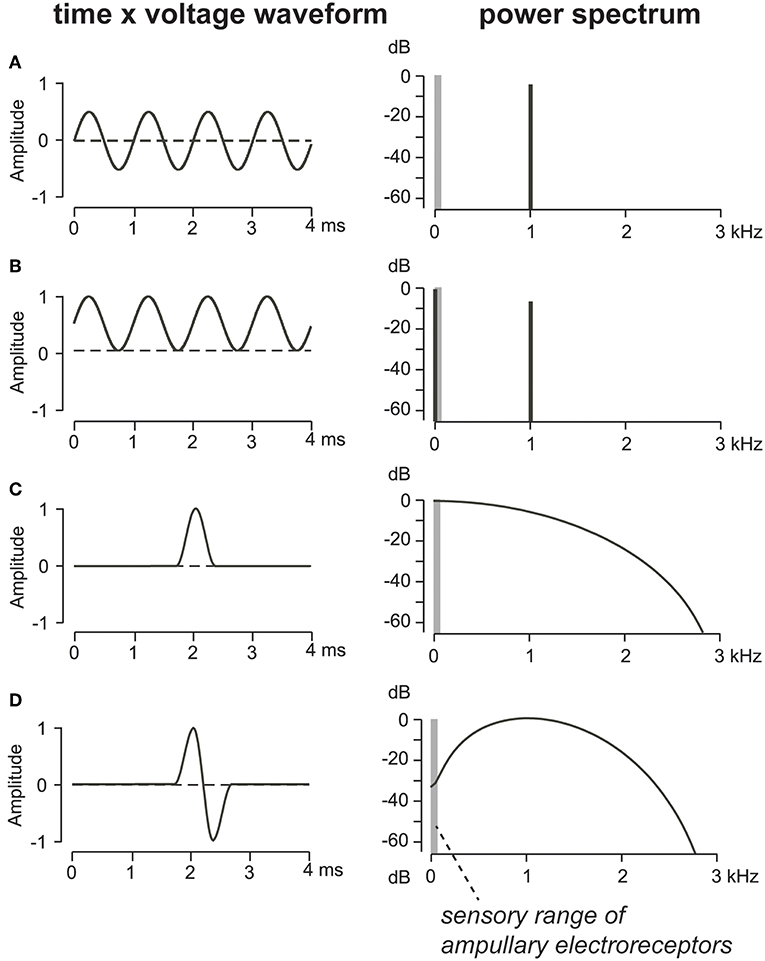
Figure 4. (A) A pure 1,000 Hz sine wave centered symmetrically around zero Volt amplitude has no low-frequency energy in the range of ampullary electroreceptors (vertical gray bar). (B) That same sine wave with a DC-offset has signal energy in the low-frequency range of the ampullary system. (C) An offset cosine pulse, resembling a monophasic EOD, has abundant low-frequency energy in the spectral range of ampullary electroreceptors. (D) A single-pulse sinusoid with a 1,000 Hz fundamental frequency has a much broader spectrum than the continuous sine wave, but does show some suppression of low-frequency energy. Adapted from Stoddard and Markham (2008) with permission of the publisher.
Actual EODs (Figure 5) distribute energy the same way as the canonical trigonometric waveforms. “Wave EODs” are strings of EOD pulses timed to produce a continuous sinusoid. The individual EODs making up a wave EOD can be sinusoidal pulses, as produced by the fish in the family Apteronotidae, or can be cosine-like pulses, offset by a DC current to balance symmetrically around 0 V, as produced by fish in the family Sternopygidae (Figure 5A). These DC-balanced wave EODs have no energy in the sensory range of ampullary electroreceptors and are expected to reduce predation by electroreceptive predators (Stoddard, 2002a), though nobody has tested electroreceptive predators for their ability to detect wave-type EODs.
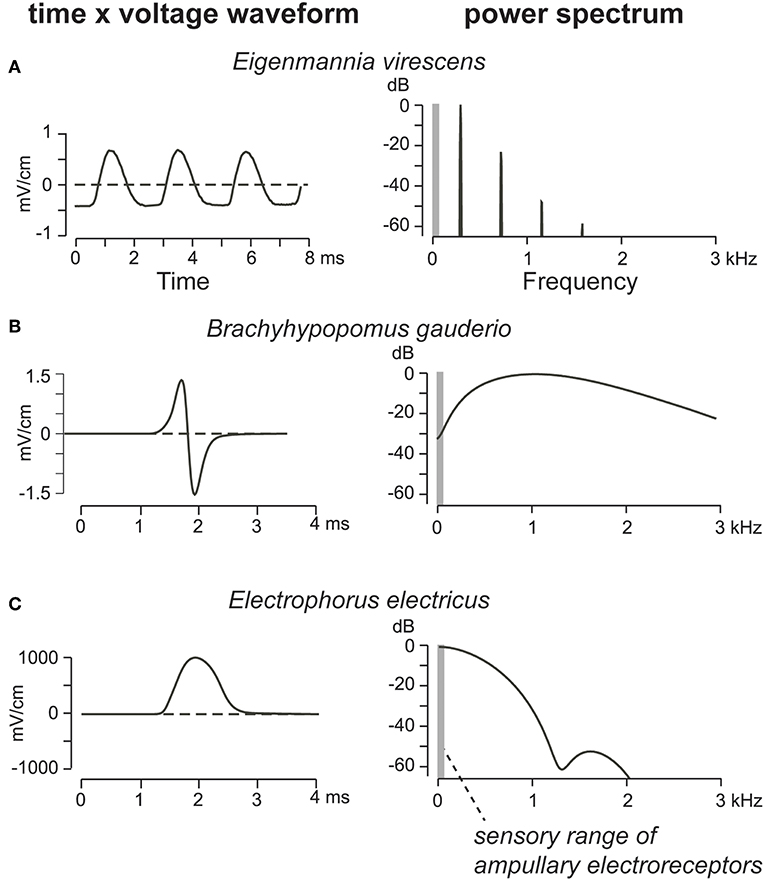
Figure 5. EODs of three gymnotiform electric fish species and their corresponding power spectra. (A) Wave EOD of the Glass Knifefish, Eigenmannia virescens (Sternopygidae), is a continuous series of monophasic pulses, offset by a DC current to resemble a sine wave. The EOD is balanced around 0 Volts DC, and thus has no energy in the spectral range of ampullary electroreceptors. (B) The EOD of a female Brachyhypopomus gauderio (Hypopomidae) resembles a symmetric single-period sinusoid. Some low-frequency energy is present, but much less than (C) in the monophasic pulse EOD of the Electric Eel, Electrophorus electricus (Gymnotidae). Adapted from Stoddard and Markham (2008) with permission of the publisher.
“Pulse EODs” have a variable duty cycle, with inter-EOD intervals longer than the EOD pulses themselves. Pulse EODs of many species are simple, single-period sinusoids, but others are made up of 1–5 wavelets (Rodriguez-Cattaneo et al., 2008; Gallant et al., 2011). Such variety is even seen within a genus. During development, pulse-type EODs develop more phases and become DC-balanced (Franchina, 1997; Crampton et al., 2011) until they have little to no energy in the sensory range of ampullary electroreceptors (Figure 3). Captive playback studies have shown that DC-balanced pulse-type EODs are not attractive to electroreceptive predators, whereas those with DC-asymmetry are highly attractive to these predators, both catfish and Electric Eel (Hanika and Kramer, 1999, 2000; Stoddard, 1999). Field playbacks have been attempted by various researchers without success, probably because electroreceptive predators are sparse, and because EODs of most species are detectable only over short distances from the source fish (e.g., 1–1.5 m; Hopkins et al., 1990; Hanika and Kramer, 2000) compared to cues detected over longer distances such as odor plumes or turbulence wakes behind the prey (Pohlmann et al., 2001).
Both in gymnotiforms and mormyrids, breeding males of several genera produce pulse EODs that extend one EOD phase to create DC-asymmetry (e.g., Hopkins, 1999; Stoddard, 2002b), particularly at night when courtship and spawning occur. This DC-asymmetry destroys the electric crypsis of the multiphasic waveform by diverting significant energy to the spectrum detected by ampullary electroreceptors. The presence of breeding females elicits dynamic 2nd phase extension by male Brachyhypopomus gauderio (Gavassa et al., 2013) and a corresponding boost in low-frequency energy (Figure 6). This sexual dimorphism is further enhanced at night by a circadian rhythm in the hormones that regulate waveform shape (Stoddard et al., 2003, 2007; Markham and Stoddard, 2005; Markham et al., 2009a), in part through trafficking of ion channels (Markham et al., 2009b). The transiently DC-imbalanced EODs of these pulse-type male electric fish may thus bear an increased risk of detection by electroreceptive predators and could therefore serve as indicators of male quality, consistent with the handicap mechanism proposed by Zahavi (1975).
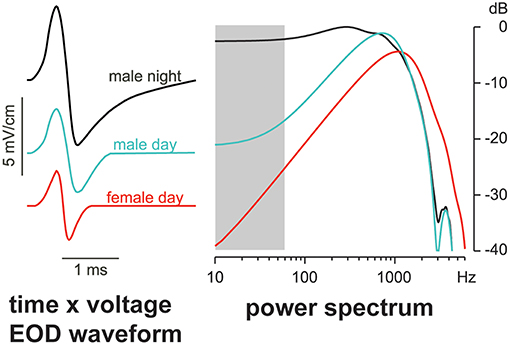
Figure 6. Male Brachyhypopomus gauderio extend the second phase of the EOD, creating DC-asymmetry, which boosts energy in the frequency range of ampullary electroreceptors (gray bar). Accordingly, males have more low-frequency energy than females, and even more at night when they further enhance the signal. Adapted from Stoddard and Markham (2008) with permission of the publisher.
Predation Density and EOD Properties in Natural Populations
If electroreceptive predators have a shaping effect on the EODs of weakly electric fish, one would predict differences in EOD waveform and power spectrum between populations exposed to strong versus weak predation pressure. Such evidence has recently been uncovered in a study of the pulse-type gymnotiform species Brachyhypopomus occidentalis in Panama. These electric fish are found in nearly all Atlantic- and Pacific-slope drainages of Panama (Picq et al., 2014). A comparison of the divergence pattern of the EODs of male B. occidentalis from different drainages with the divergence pattern of neutral genetic markers is consistent with a sizable effect of genetic drift on EOD evolution (Picq et al., 2016). Within-drainage variation in EOD properties suggested, however, that additional factors are likely shaping the waveform as well. Strong variation in the percentage of fish found with damaged and regenerating tails pointed at differences in predation pressure between streams within the same drainage. The amount of tail damage in each stream corresponded with stream counts of a piscivorous pimelodid catfish, Rhamdia quelen, a potential electroreceptive predator of B. occidentalis (Tran, 2014). Further, B. occidentalis from streams with higher incidence of catfish produced a less-extended EOD second phase with upward shifted power spectrum compared to fish from streams within the same drainage that had fewer catfish (Figure 7). To compare susceptibility to detection by predators equipped with an ampullary electrosense, Tran (2014) measured the frequencies of peak power of the EOD for B. occidentalis from a low-predation and a high-predation stream in each of three drainages (data from males and females were pooled for each drainage, as their EODs did not differ significantly in peak frequency). Compared to the EOD of B. occidentalis from low-predation streams, the frequency of peak power was significantly reduced in animals from the high-predation streams (mean differences in peak frequency between fish from the high- and low-predation populations were between 130 and 320 Hz; ANCOVA, p < 0.001). The reduced low-frequency power of fish exposed to relatively high predation pressure is consistent with the hypothesis that electroreceptive predators have been shaping the EOD properties of weakly electric fishes in the direction of greater waveform symmetry. Whether the within-drainage differences in EOD properties found in Panamanian B. occidentalis are indeed heritable or result from individually plastic response to presence of predators or predation remains to be determined.
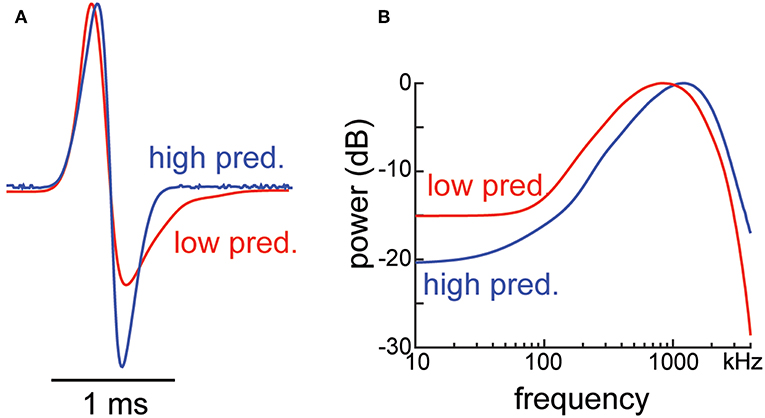
Figure 7. (A) B. occidentalis from streams in Panama with lower incidence of piscivorous catfish (red line) produced EODs with a more-extended second phase than animals in streams with high incidence of catfish (blue line). (B) The power spectra of a typical EOD from a low-catfish stream (red line) has more energy in the spectral range of ampullary electroreceptors than the EOD of a male from a high catfish stream (blue line).
Energetic Costs of Producing DC-Balanced EODs
Electric fish with DC-symmetrical EODs pay an energetic cost for the ionic mechanisms that reduce or eliminate the low-frequency energy in the signals. Species that make DC-symmetrical, pulsed EODs do so by adding phases to the initial monophasic EOD. Each phase would seem to add linearly to the cost of signal production, however, the action potentials underlying the additional phases temporally overlap one another within and between electrogenic cells (electrocytes) in the electric organs (Bennett, 1961, 1970, 1971a; Caputi et al., 1994; Stoddard et al., 1999). Temporal overlap results in cancellation of significant amounts of energy invested in electrogenesis (Figure 8) (Stoddard and Markham, 2008; Markham and Stoddard, 2013; Markham and Zakon, 2014). We postulate that reduction of predation pressure by balancing the DC energy in the EOD is worth the energetic expense of losing most of that energy to temporal overlap. Multiple EOD phases also allow for greater species specificity during mate selection, which appears to be another selective force shaping electric waveforms (Crampton et al., 2011).
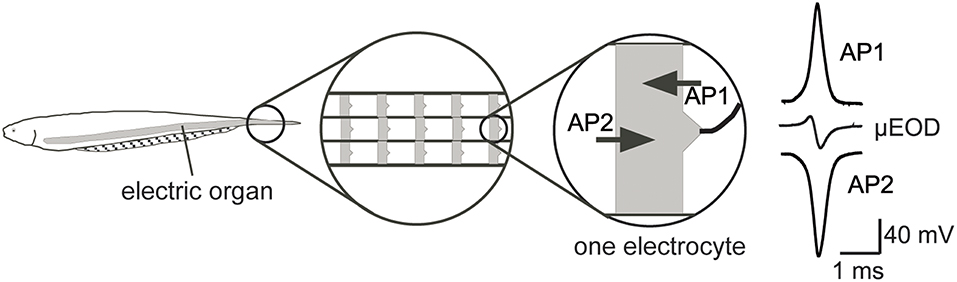
Figure 8. The biphasic EODs of B. gauderio and others are produced at the level of the single electrocyte by a pair of action potentials (APs) driving sodium currents in opposite directions (AP1 headward, AP2 tailward). The action potentials are temporally offset, which produces a biphasic micro-EOD when the two currents sum outside the cell. The temporal offset is only partial, however, so the current from each action potential partially cancels out the other. Thus, the 2nd EOD phase that balances the EOD to reduce predation risk also wastes significant energy. Adapted from Stoddard (2002a), Markham and Stoddard (2005), and Stoddard and Markham (2008) with permission of the publisher.
Parallels to Other Systems
Microgeographic patterns of electric signal crypsis among B. occidentalis in Panama parallel the predator-driven pattern seen in Trinidadian guppies (Poecilia reticulata). Male guppies have more conspicuous patterning than females, particularly so in streams, or even parts of streams, where predators are less frequent (Endler, 1991). Guppies translocated between high- and low-predation areas quickly evolved color patterns that balanced sexual attractiveness against the prevailing predation risk (Reznick et al., 1997). Female preferences for male EODs have not been explored yet in B. occidentalis. Gravid females of the related species, B. gauderio, preferred larger over smaller males, when given a choice (Curtis and Stoddard, 2003). The larger males had EOD pulses of larger amplitude and longer duration than smaller males, the latter feature being consistent with female preference for signals with more low-frequency power. Playback experiments with gravid females are needed to assess whether the females base their preference on EOD amplitude and/or duration or whether they evaluate male size independently of the EOD. Similar experiments with B. occidentalis may permit quantifying the trade-off between sexual and natural selection in a lower-dimensional system than the visual communication of guppies, where sexual selection appears to push male coloration in different directions depending not only on the specific habitat conditions but also the starting trait of the particular lineage under selection (Kemp et al., 2018).
Division of electric signal energy between the high-frequency band detected by tuberous electroreceptors and the low-frequency band detected by ampullary electroreceptors of conspecifics and predatory eavesdroppers alike, bears a parallel to mate attraction calls of the Túngara frog complex Physalaemus spp. of the Neotropics. Frogs have two auditory organs, the basilar papilla and amphibian papilla, with high and low frequency tuning, respectively (Zakon and Wilczynski, 1988). Male Túngara frogs produce a “whine” call for mate attraction. However, when competing with conspecific males, male Túngara frogs add lower-frequency “chuck” call components with energy focused in the frequency band of the basilar papilla, which makes them more attractive to conspecific females, but also to predatory bats and parasitic flies (Ryan et al., 1990; Ryan, 1992; Bernal et al., 2006; Page and Ryan, 2008). We see a similar pattern in males of the electric fish, B. gauderio, which enhance their low-frequency power output in the presence of either sex, but boost their signal amplitude only when competing males are present (Franchina et al., 2001; Gavassa et al., 2013). Increasing EOD amplitude is expected to increase predation risk by expanding the space over which predators can detect the emitter. A second effect of the boost in amplitude is the added energetic expense of producing larger currents flowing across the electrocyte membranes (Salazar and Stoddard, 2008; Markham et al., 2009b). Whereas the energetic cost of male Túngara frogs producing whines, and increasing the rate of whine production, was assessed many years ago (Bucher et al., 1982), the cost of adding chucks to whines has, to our knowledge, not been estimated.
Future Directions
Although predatory catfish are believed to shape the evolution of electric signals in the dominant groups of electric fish, tuning curves of ampullary receptors in the catfish, the presumed dominant predatory drivers, have been characterized only in temperate catfish of North America (Dijkgraa, 1968; Peters and Buwalda, 1972; Bullock, 1979; Finger, 1986; Peters et al., 1997; Eeuwes et al., 2001; Collin and Whitehead, 2004). A detailed study of sensory physiology and predation on electric fish by Neotropical pimelodid catfishes would be a useful addition to our understanding. Likewise, although we find strong signatures of sensory drive on electric signals by electroreceptive predators, the microgeographic differences in EOD spectrum seen in male electric fish in Panama (Tran, 2014) could result from either evolutionary or developmental response to predation. Resolution of this question could prove interesting. Finally, we have assumed that the dangerous low-frequency components of male electric courtship signals are attractive to conspecific females (Stoddard, 2002a,b), but no studies have tested this assumption directly. Such studies are tricky: female electric fish are finicky subjects for mate choice studies, and low-frequency, low-intensity electric fields require special care to measure and regulate.
Author Contributions
PS and RK wrote the manuscript. AT and RK conducted the predation study in Panama.
Funding
RK was supported by the Natural Sciences and Engineering Research Council of Canada (NSERC Discovery grant 2014-05364). AT was funded, in part, through a fellowship from the Fonds de recherche du Québec – Nature et Technologies. Preparation of the manuscript was supported by NSF grant IOS-1457173 to PS.
Conflict of Interest Statement
The authors declare that the research was conducted in the absence of any commercial or financial relationships that could be construed as a potential conflict of interest.
Acknowledgments
Thanks to Michael Markham for contribution of data for Figure 8.
References
Adamo, S. A., Robert, D., and Hoy, R. R. (1995). Effects of a tachinid parasitoid, Ormia ochracea, on the behavior and reproduction of its male and female field cricket hosts (Gryllus spp.). J. Insect Physiol. 41, 269–277. doi: 10.1016/0022-1910(94)00095-X
Albert, J. S., and Crampton, W. G. R. (2005). “Diversity and phylogeny of neotropical electric fishes (Gymnotiformes),” in Electroreception, eds T. H. Bullock, C. D. Hopkins, A. N. Popper, and R. R. Fay (New York, NY: Springer), 360–409. doi: 10.1007/0-387-28275-0_13
Alda, F., Picq, S., León, L. F. D., González, R., Walz, H., Bermingham, E., et al. (2013). First record of Gymnotus henni (Albert, Crampton and Maldonado, 2003) in Panama: phylogenetic position and electric signal characterization. Check List 9, 655–659. doi: 10.15560/9.3.655
Alves-Gomes, J. (2001). The evolution of electroreception and bioelectrogenesis in teleost fish: a phylogenetic perspective. J. Fish Biol. 58, 1489–1511. doi: 10.1111/j.1095-8649.2001.tb02307.x
Alves-Gomes, J., and Hopkins, C. D. (1997). Molecular insights into the phylogeny of mormyriform fishes and the evolution of their electric organs. Brain Behav. Evolu. 49, 324–350. doi: 10.1159/000113001
Andres, K. H., von Düring, M., and Petrasch, E. (1988). The fine structure of ampullary and tuberous electroreceptors in the South American blind catfish Pseudocetopsis spec. Anat. Embryol. 177, 523–535. doi: 10.1007/BF00305139
Armbruster, J. W. (2011). “Global catfish biodiversity,” in Conservation, Ecology, and Management of Catfish: The Second International Symposium, 2010, Vol. 77 (Bethesda, MD), 15–37.
Arnegard, M. E., McIntyre, P. B., Harmon, L. J., Zelditch, M. L., Crampton, W. G., Davis, J. K., et al. (2010). Sexual signal evolution outpaces ecological divergence during electric fish species radiation. Am. Nat. 176, 335–356. doi: 10.1086/655221
Baron, V. D. (1994). African Clarias catfish emits long-lasting weak electric pulses. Experientia 50, 644–647. doi: 10.1007/BF01952864
Baron, V. D., Morshnev, K. S., Olshansky, V. M., and Orlov, A. A. (1994). Electric organ discharges of two species of African catfish (Synodontis) during social behaviour. Anim. Behav. 48, 1472–1475. doi: 10.1006/anbe.1994.1387
Bass, A. H. (1986). “Electric organs revisited,” in Electroreception, eds T. H. Bullock and W. Heiligenberg (New York, NY: Wiley), 13–70.
Bennett, M. V. L. (1961). Modes of operation of electric organs. Ann. N. Y. Acad. Sci. 94, 458–509. doi: 10.1111/j.1749-6632.1961.tb35555.x
Bennett, M. V. L. (1970). Comparative physiology: electric organs. Annu. Rev. Physiol. 32, 471–528. doi: 10.1146/annurev.ph.32.030170.002351
Bennett, M. V. L. (1971a). “Electric organs,” in Fish Physiology, eds W. S. Hoar and D. J. Randall (London: Academic Press), 347–491. doi: 10.1016/S1546-5098(08)60051-5
Bennett, M. V. L. (1971b). “Electroreception,” in Fish Physiology, eds W. S. Hoar and D. J. Randal (New York, NY: Academic Press), 493–574. doi: 10.1016/S1546-5098(08)60052-7
Bernal, X. E., Rand, A. S., and Ryan, M. J. (2006). Acoustic preferences and localization performance of blood-sucking flies (Corethrella Coquillett) to túngara frog calls. Behav. Ecol. 17, 709–715. doi: 10.1093/beheco/arl003
Boyle, K. S., Colleye, O., and Parmentier, E. (2014). Sound production to electric discharge: sonic muscle evolution in progress in Synodontis spp. catfishes (Mochokidae). Proc. Biol. Sci. 281, 20141197. doi: 10.1098/rspb.2014.1197
Bucher, T. L., Ryan, M. J., and Bartholomew, G. A. (1982). Oxygen consumption during resting, calling, and nest building in the frog Physalaemus pustulosus. Physiol. Zool. 55, 10–22. doi: 10.1086/physzool.55.1.30158439
Bullock, T. (1982). Electroreception. Annu. Rev. Neurosci. 5, 121–170. doi: 10.1146/annurev.ne.05.030182.001005
Bullock, T. H. (1979). Processing of ampullary input in the brain: comparison of sensitivity and evoked responses among elasmobranch and siluriform fishes. J. Physiol. 75, 397–407.
Cade, W. H. (1975). Acoustically orienting parasitoids: fly phonotaxis to cricket song. Science 190, 1312–1313. doi: 10.1126/science.190.4221.1312
Caputi, A., Macadar, O., and Trujillo-Cenóz, O. (1994). Waveform generation in Rhamphichthys rostratus (L.) (Teleostei, Gymnotiformes). The electric organ and its spatiotemporal activation pattern. J. Comp. Physiol. A 174, 633–642. doi: 10.1007/BF00217384
Catania, K. C. (2016). Leaping eels electrify threats, supporting Humboldt's account of a battle with horses. Proc. Natl. Acad. Sci. U.S.A. 113:6979. doi: 10.1073/pnas.1604009113
Collin, S. P., and Whitehead, D. (2004). The functional roles of passive electroreception in non-electric fishes. Anim Biol. 54, 1–25. doi: 10.1163/157075604323010024
Crampton, W. G., Rodriguez-Cattaneo, A., Lovejoy, N. R., and Caputi, A. A. (2013). Proximate and ultimate causes of signal diversity in the electric fish Gymnotus. J. Exp. Biol. 216(Pt 13), 2523–2541. doi: 10.1242/jeb.083261
Crampton, W. G. R., de Santana, C. D., Waddell, J. C., and Lovejoy, N. R. (2016). Phylogenetic systematics, biogeography, and ecology of the electric fish genus Brachyhypopomus (Ostariophysi: Gymnotiformes). PLoS ONE 11:e0161680. doi: 10.1371/journal.pone.0161680
Crampton, W. G. R., Lovejoy, N. R., and Waddell, J. C. (2011). Reproductive character displacement and signal ontogeny in a sympatric assemblage of electric fish. Evolution 65, 1650–1666. doi: 10.1111/j.1558-5646.2011.01245.x
Curtis, C. C., and Stoddard, P. K. (2003). Mate preference in female electric fish, Brachyhypopomus pinnicaudatus. Anim. Behav. 66, 329–336. doi: 10.1006/anbe.2003.2216
Day, J. J., Peart, C. R., Brown, K. J., Friel, J. P., Bills, R., and Moritz, T. (2013). Continental diversification of an African catfish radiation (Mochokidae: Synodontis). Syst. Biol. 62, 351–365. doi: 10.1093/sysbio/syt001
De Santana, C. D., and Crampton, W. G. R. (2011). Phylogenetic interrelationships, taxonomy, and reductive evolution in the Neotropical electric fish genus Hypopygus (Teleostei, Ostariophysi, Gymnotiformes). Zool. J. Linn. Soc. 163, 1096–1156. doi: 10.1111/j.1096-3642.2011.00736.x
Dijkgraa, S. (1968). Electroreception in catfish Amiurus nebulosus. Experientia 24, 187–188. doi: 10.1007/BF02146979
Dunning, B. B. (1973). A quantitative and Comparative Analysis of the Tonic Electroreceptors of Gnathonemus, Gymnotus and Kryptopterus (Ph.D. Thesis). Minneapolis, MN: University of Minnesota.
Duque, A. B., and Winemiller, K. O. (2003). Dietary segregation among large catfishes of the Apure and Arauca Rivers, Venezuela. J. Fish Biol. 63, 410–427. doi: 10.1046/j.1095-8649.2003.00163.x
Eeuwes, L. B. M., Peters, R. C., Bretschneider, F., and Loos, W. J. G. (2001). Electroreception of catfish Ictalurus nebulosus in uniform and non-uniform DC fields: detection threshold and body length. Belg. J. Zool. 131, 73–78.
Endler, J. A. (1991). Variation in the appearance of guppy color patterns to guppies and their predators under different visual conditions. Vision Res. 31, 587–608. doi: 10.1016/0042-6989(91)90109-I
Engelmann, J., Bacelo, J., Metzen, M., Pusch, R., Bouton, B., Migliaro, A., et al. (2008). Electric imaging through active electrolocation: implication for the analysis of complex scenes. Biol. Cybern. 98, 519–539. doi: 10.1007/s00422-008-0213-5
Faraday, M. (1839). I. Experimental researches in electricity. - Fifteenth series. Notice of the character and direction of the electric force of the Gymnotus. Philos. Trans. R. Soc. Lond. 129, 1–12. doi: 10.1098/rstl.1839.0002
Feulner, P. G. D., Kirschbaum, F., and Tiedemann, R. (2008). Adaptive radiation in the Congo River: an ecological speciation scenario for African weakly electric fish (Teleostei; Mormyridae; Campylomormyrus). J. Physiol. 102, 340–346. doi: 10.1016/j.jphysparis.2008.10.002
Feulner, P. G. D., Plath, M., Engelmann, J., Kirschbaum, F., and Tiedemann, R. (2009). Electrifying love: electric fish use species-specific discharge for mate recognition. Biol. Lett. 5, 225–228. doi: 10.1098/rsbl.2008.0566
Finger, T. E. (1986). “Electroreception in catfish: behavior, anatomy, and physiology,” in Electroreception, eds T. H. Bullock and W. Heiligenberg (New York, NY: John Wiley and Sons Inc., 287–318.
Franchina, C. R. (1997). Ontogeny of the electric organ and the electric organ discharge in the weakly electric fish Brachyhypopomus pinnicaudatus (Teleostei, Gymnotiformes). J. Comp. Physiol. A 181, 111–119. doi: 10.1007/s003590050098
Franchina, C. R., Salazar, V. L., Volmar, C. H., and Stoddard, P. K. (2001). Plasticity of the electric organ discharge waveform of male Brachyhypopomus pinnicaudatus. II. Social effects. J. Comp. Physiol. A 187, 45–52. doi: 10.1007/s003590000176
Gallant, J. R., Arnegard, M. E., Sullivan, J. P., Carlson, B. A., and Hopkins, C. D. (2011). Signal variation and its morphological correlates in Paramormyrops kingsleyae provide insight into the evolution of electrogenic signal diversity in mormyrid electric fish. J. Comp. Physiol. A 197, 799–817. doi: 10.1007/s00359-011-0643-8
Gavassa, S., Roach, J. P., and Stoddard, P. K. (2013). Social regulation of electric signal plasticity in male Brachyhypopomus gauderio. J. Comp. Physiol. A 199, 375–384. doi: 10.1007/s00359-013-0801-2
Hagedorn, M., Womble, M., and Finger, T. E. (1990). Synodontid catfish: a new group of weakly electric fish. Brain Behav. Evolu. 35, 268–277. doi: 10.1159/000115873
Hanika, S., and Kramer, B. (1999). Electric organ discharges of mormyrid fish as a possible cue for predatory catfish. Naturwissenschaften 86, 286–288. doi: 10.1007/s001140050616
Hanika, S., and Kramer, B. (2000). Electrosensory prey detection in the African sharptooth catfish, Clarias gariepinus (Clariidae), of a weakly electric mormyrid fish, the bulldog (Marcusenius macrolepidotus). Behav. Ecol. Sociobiol. 48, 218–228. doi: 10.1007/s002650000232
Henninger, J., Krahe, R., Kirschbaum, F., Grewe, J., and Benda, J. (2018). Statistics of natural communication signals observed in the wild identify important yet neglected stimulus regimes in weakly electric fish. J. Neurosci. 38, 5456–5465. doi: 10.1523/JNEUROSCI.0350-18.2018
Hopkins, C. D. (1980). Evolution of electric communication channels of mormyrids. Behav. Ecol. Sociobiol. 7, 1–13. doi: 10.1007/BF00302513
Hopkins, C. D. (1981). The neuroethology of electric communication. Trends Neurosci. 4, 4–6. doi: 10.1016/0166-2236(81)90003-5
Hopkins, C. D. (1995). Convergent designs for electrogenesis and electroreception. Curr. Opin. Neurobiol. 5, 769–777. doi: 10.1016/0959-4388(95)80105-7
Hopkins, C. D. (1999). Design features for electric communication. J. Exp. Biol. 202(Pt 10), 1217–1228.
Hopkins, C. D., Comfort, N. C., Bastian, J., and Bass, A. H. (1990). Functional analysis of sexual dimorphism in an electric fish, Hypopomus pinnicaudatus, order Gymnotiformes. Brain Behav. Evolu. 35, 350–367. doi: 10.1159/000115880
Hopkins, C. D., and Heiligenberg, W. (1978). Evolutionary designs for electric signals and electroreceptors in gymnotoid fishes of Surinam. Behav. Ecol. Sociobiol. 3, 113–134. doi: 10.1007/BF00294985
Kalmijn, A. J. (1974). “The detection of electric fields from inanimate and animate sources other than electric organs,” in Electroreceptors and Other Specialized Receptors in Lower Vertebrates, ed A. Fessard (Berlin; Heidelberg: Springer-Verlag), 147–200. doi: 10.1007/978-3-642-65926-3_5
Kemp, D. J., Batistic, F. K., and Reznick, D. N. (2018). Predictable adaptive trajectories of sexual coloration in the wild: Evidence from replicate experimental guppy populations. Evolution 72, 2462–2477. doi: 10.1111/evo.13564
Kirschbaum, F. (1995). “Reproduction and development in mormyriform and gymnotiform fishes,” in Electric Fishes History and Behavior, ed P. Moller (London: Chapman and Hall), 267–301.
Kirschbaum, F., and Schugardt, C. (2002). Reproductive strategies and developmental aspects in mormyrid and gymnotiform fishes. J. Physiol. 96, 557–566. doi: 10.1016/S0928-4257(03)00011-1
Lissmann, H. W. (1958). On the function and evolution of electric organs in fish. J. Exp. Biol. 35, 156–191.
Lovejoy, N. R., Lester, K., Crampton, W. G., Marques, F. P., and Albert, J. S. (2010). Phylogeny, biogeography, and electric signal evolution of Neotropical knifefishes of the genus Gymnotus (Osteichthyes: Gymnotidae). Mol. Phylogenet. Evol. 54, 278–290. doi: 10.1016/j.ympev.2009.09.017
Maan, M. E., and Seehausen, O. (2011). Ecology, sexual selection and speciation. Ecol. Lett. 14, 591–602. doi: 10.1111/j.1461-0248.2011.01606.x
Markham, M. R., Allee, S. J., Goldina, A., and Stoddard, P. K. (2009a). Melanocortins regulate the electric waveforms of gymnotiform electric fish. Horm. Behav. 55, 306–313. doi: 10.1016/j.yhbeh.2008.11.002
Markham, M. R., McAnelly, M. L., Stoddard, P. K., and Zakon, H. H. (2009b). Circadian and social cues regulate ion channel trafficking. PLoS Biol. 7:e1000203. doi: 10.1371/journal.pbio.1000203
Markham, M. R., and Stoddard, P. K. (2005). Adrenocorticotropic hormone enhances the masculinity of an electric communication signal by modulating the waveform and timing of action potentials within individual cells. J. Neurosci. 25, 8746–8754. doi: 10.1523/JNEUROSCI.2809-05.2005
Markham, M. R., and Stoddard, P. K. (2013). Cellular mechanisms of developmental and sex differences in the rapid hormonal modulation of a social communication signal. Horm. Behav. 63, 586–597. doi: 10.1016/j.yhbeh.2013.02.004
Markham, M. R., and Zakon, H. H. (2014). Ionic nechanisms of microsecond-scale spike timing in single cells. J. Neurosci. 34, 6668–6678. doi: 10.1523/JNEUROSCI.0615-14.2014
Merron, G. S. (1993). Pack-hunting in two species of catfish, Clarias gariepinus and C. ngamensis, in the Okavango Delta, Botswana. J. Fish Biol. 43, 575–584. doi: 10.1006/jfbi.1993.1160
Page, R. A., and Ryan, M. J. (2008). The effect of signal complexity on localization performance in bats that localize frog calls. Anim. Behav. 76, 761–769. doi: 10.1016/j.anbehav.2008.05.006
Peters, R. C., Brans, R. J., Bretschneider, F., Versteeg, E., and Went, A. (1997). Converging electroreceptor cells improve sensitivity and tuning. Neuroscience 81, 297–301. doi: 10.1016/S0306-4522(97)00190-5
Peters, R. C., and Buwalda, R. J. A. (1972). Frequency response of the electroreceptors (“small pit organs”) of the catfish, Ictalurus nebulosus LeS. J. Comp. Physiol. 79, 29–38. doi: 10.1007/BF00693616
Peters, R. C., Eeuwes, L. B. M., and Bretschneider, F. (2007). On the electrodetection threshold of aquatic vertebrates with ampullary or mucous gland electroreceptor organs. Biol. Rev. 82, 361–373. doi: 10.1111/j.1469-185X.2007.00015.x
Petrere, M., Barthem, R. B., Cordoba, E. A., and Gomez, B. C. (2004). Review of the large catfish fisheries in the upper Amazon and the stock depletion of piraiba (Brachyplatystoma filamentosum Lichtenstein). Rev. Fish Biol. Fish 14, 403–414. doi: 10.1007/s11160-004-8362-7
Picq, S., Alda, F., Bermingham, E., and Krahe, R. (2016). Drift-driven evolution of electric signals in a Neotropical knifefish. Evolution 70, 2134–2144. doi: 10.1111/evo.13010
Picq, S., Alda, F., Krahe, R., and Bermingham, E. (2014). Miocene and Pliocene colonization of the Central American Isthmus by the weakly electric fish Brachyhypopomus occidentalis (Hypopomidae, Gymnotiformes). J. Biogeogr. 41, 1520–1532. doi: 10.1111/jbi.12309
Pohlmann, K., Grasso, F. W., and Breithaupt, T. (2001). Tracking wakes: the nocturnal predatory strategy of piscivorous catfish. Proc. Natl. Acad. Sci. U.S.A. 98, 7371–7374. doi: 10.1073/pnas.121026298
Reid, S. (1983). La biologia de los bagres rayados Pseudoplatystoma fasciatum y P. tigrinum en la cuenca del rio Apure - Venezuela. Revista UNELLEZ de Ciencia y Tecnologia 1, 13–41.
Reznick, D. N., Shaw, F. H., Rodd, F. H., and Shaw, R. G. (1997). Evaluation of the rate of evolution in natural populations of guppies (Poecilia reticulata). Science 275, 1934–1937. doi: 10.1126/science.275.5308.1934
Robert, D., Amoroso, J., and Hoy, R. R. (1992). The evolutionary convergence of hearing in a parasitoid fly and its cricket host. Science 258, 1135–1137. doi: 10.1126/science.1439820
Rodriguez-Cattaneo, A., Pereira, A. C., Aguilera, P. A., Crampton, W. G., and Caputi, A. A. (2008). Species-specific diversity of a fixed motor pattern: the electric organ discharge of Gymnotus. PLoS ONE 3:e2038. doi: 10.1371/journal.pone.0002038
Ryan, M. J. (1992). The Tungara Frog: A Study in Sexual Selection and Communication. Chicago, IL: University of Chicago Press.
Ryan, M. J., Fox, J. H., Wilczynski, W., and Rand, A. S. (1990). Sexual selection for sensory exploitation in the frog Physalaemus pustulosus. Nature 343, 66–67. doi: 10.1038/343066a0
Salazar, V. L., and Stoddard, P. K. (2008). Sex differences in energetic costs explain sexual dimorphism in the circadian rhythm modulation of the electrocommunication signal of the gymnotiform fish Brachyhypopomus pinnicaudatus. J. Exp. Biol. 211(Pt 6), 1012–20. doi: 10.1242/jeb.014795
Shumway, C. A., and Zelick, R. D. (1988). Sex recognition and neuronal coding of electric organ discharge waveform in the pulse-type weakly electric fish, Hypopomus occidentalis. J. Comp. Physiol. A 163, 465–478. doi: 10.1007/BF00604901
Stoddard, P. K. (1999). Predation enhances complexity in the evolution of electric fish signals. Nature 400, 254–256. doi: 10.1038/22301
Stoddard, P. K. (2002a). Electric signals: Predation, sex, and environmental constraints. Adv. Study Behav. 31, 201–242. doi: 10.1016/S0065-3454(02)80009-2
Stoddard, P. K. (2002b). The evolutionary origins of electric signal complexity. J. Physiol. Paris 96, 485–491. doi: 10.1016/S0928-4257(03)00004-4
Stoddard, P. K., and Markham, M. R. (2008). Signal cloaking by electric fish. Bioscience 58, 415–425. doi: 10.1641/B580508
Stoddard, P. K., Markham, M. R., and Salazar, V. L. (2003). Serotonin modulates the electric waveform of the gymnotiform electric fish Brachyhypopomus pinnicaudatus. J. Exp. Biol. 206, 1353–1362. doi: 10.1242/jeb.00252
Stoddard, P. K., Markham, M. R., Salazar, V. L., and Allee, S. (2007). Circadian rhythms in electric waveform structure and rate in the electric fish Brachyhypopomus pinnicaudatus. Physiol. Behav. 90, 11–20. doi: 10.1016/j.physbeh.2006.08.013
Stoddard, P. K., Rasnow, B., and Assad, C. (1999). Electric organ discharges of the gymnotiform fishes: III. Brachyhypopomus. J. Comp. Physiol. A 184, 609–630. doi: 10.1007/s003590050359
Sullivan, J., Zuanon, J., and Cox Fernandes, C. (2013). Two new species and a new subgenus of toothed Brachyhypopomus electric knifefishes (Gymnotiformes, Hypopomidae) from the central Amazon and considerations pertaining to the evolution of a monophasic electric organ discharge. ZooKeys 327, 1–34. doi: 10.3897/zookeys.327.5427
Sullivan, J. P., Lavoue, S., and Hopkins, C. D. (2000). Molecular systematics of the African electric fishes (Mormyroidea: Teleostei) and a model for the evolution of their electric organs. J. Exp. Biol. 203(Pt 4), 665–683.
Sullivan, J. P., Lundberg, J. G., and Hardman, M. (2006). A phylogenetic analysis of the major groups of catfishes (Teleostei: Siluriformes) using rag1 and rag2 nuclear gene sequences. Mol. Phylogenet. Evol. 41, 636–662. doi: 10.1016/j.ympev.2006.05.044
Tagliacollo, V. A., Bernt, M. J., Craig, J. M., Oliveira, C., and Albert, J. S. (2016). Model-based total evidence phylogeny of Neotropical electric knifefishes (Teleostei, Gymnotiformes). Mol. Phylogenet. Evol. 95, 20–33. doi: 10.1016/j.ympev.2015.11.007
Tran, A. (2014). The Effects of Predation on Electric Fish Signals (Masters Thesis). Montreal, QC: McGill University.
Verrell, P. A. (1991). Illegitimate exploitation of sexual signalling systems and the origin of species. Ethol. Ecol. Evolu. 3, 273–283. doi: 10.1080/08927014.1991.9525356
Waddell, J. C., Rodriguez-Cattaneo, A., Caputi, A. A., and Crampton, W. G. R. (2016). Electric organ discharges and near-field spatiotemporal patterns of the electromotive force in a sympatric assemblage of Neotropical electric knifefish. J. Physiol. 110 (3 Pt B), 164–181. doi: 10.1016/j.jphysparis.2016.10.004
Westby, G. W. M. (1988). The ecology, discharge diversity and predatory behaviour of gymnotiforme electric fish in the coastal streams of French Guiana. Behav. Ecol. Sociobiol. 22, 341–354.
Zahavi, A. (1975). Mate selection – selection for a handicap. J. Theor. Biol. 53, 205–214. doi: 10.1016/0022-5193(75)90111-3
Zakon, H., and Wilczynski, W. (1988). “The physiology of the VIIIth nerve,” in The Evolution of the Amphibian Auditory System, eds B. Fritzsch, M. J. Ryan, W. Wilczynski, T. Hetherington, and W. Walkowiak (New York, NY: Wiley), 125–155.
Zakon, H. H. (1986). “The electroreceptive periphery,” in Electroreception, eds T. H. Bullock and W. Heiligenberg (New York, NY: Wiley, 103–156.
Zuk, M., and Kolluru, G. R. (1998). Exploitation of sexual signals by predators and parasitoids. Q. Rev. Biol. 73, 415–438. doi: 10.1086/420412
Keywords: catfish, electroreception, Gymnotiformes, Mormyridae, predation, sensory drive, signal diversity
Citation: Stoddard PK, Tran A and Krahe R (2019) Predation and Crypsis in the Evolution of Electric Signaling in Weakly Electric Fishes. Front. Ecol. Evol. 7:264. doi: 10.3389/fevo.2019.00264
Received: 08 December 2018; Accepted: 24 June 2019;
Published: 10 July 2019.
Edited by:
Ximena E. Bernal, Purdue University, United StatesReviewed by:
Michael J. Pauers, Milwaukee Public Museum, United StatesDavid Ernesto Saenz, Texas A&M University, United States
Bruce A. Carlson, Washington University in St. Louis, United States
Kirk Winemiller, Texas A&M AgriLife Research, Texas A&M University, United States
Copyright © 2019 Stoddard, Tran and Krahe. This is an open-access article distributed under the terms of the Creative Commons Attribution License (CC BY). The use, distribution or reproduction in other forums is permitted, provided the original author(s) and the copyright owner(s) are credited and that the original publication in this journal is cited, in accordance with accepted academic practice. No use, distribution or reproduction is permitted which does not comply with these terms.
*Correspondence: Rüdiger Krahe, cnVlZGlnZXIua3JhaGVAaHUtYmVybGluLmRl