- 1Department of Biology and Environmental Science, Linnaeus University, Kalmar, Sweden
- 2Department of Aquatic Resources, Institute of Freshwater Research, SLU, Drottningholm, Sweden
Migration strategies in fishes comprise a rich, ecologically important, and socioeconomically valuable example of biological diversity. The variation and flexibility in migration is evident between and within individuals, populations, and species, and thereby provides a useful model system that continues to inform how ecological and evolutionary processes mold biodiversity and how biological systems respond to environmental heterogeneity and change. Migrating fishes are targeted by commercial and recreational fishing and impact the functioning of aquatic ecosystems. Sadly, many species of migrating fish are under increasing threat by exploitation, pollution, habitat destruction, dispersal barriers, overfishing, and ongoing climate change that brings modified, novel, more variable and extreme conditions and selection regimes. All this calls for protection, sustainable utilization and adaptive management. However, the situation for migrating fishes is complicated further by actions aimed at mitigating the devastating effects of such threats. Changes in river connectivity associated with removal of dispersal barriers such as dams and construction of fishways, together with compensatory breeding, and supplemental stocking can impact on gene flow and selection. How this in turn affects the dynamics, genetic structure, genetic diversity, evolutionary potential, and viability of spawning migrating fish populations remains largely unknown. In this narrative review we describe and discuss patterns, causes, and consequences of variation and flexibility in fish migration that are scientifically interesting and concern key issues within the framework of evolution and maintenance of biological diversity. We showcase how the evolutionary solutions to key questions that define migrating fish—whether or not to migrate, why to migrate, where to migrate, and when to migrate—may depend on individual characteristics and ecological conditions. We explore links between environmental change and migration strategies, and discuss whether and how threats associated with overexploitation, environmental makeovers, and management actions may differently influence vulnerability of individuals, populations, and species depending on the variation and flexibility of their migration strategies. Our goal is to provide a broad overview of knowledge in this emerging area, spur future research, and development of informed management, and ultimately promote sustainable utilization and protection of migrating fish and their ecosystems.
Introduction
Migratory fish showcase a scientifically interesting example of biological diversity that is of considerable ecological and socioeconomic importance (Leggett, 1977; Lynch et al., 2016; Oke and Hendry, 2019). Given the alarming situation for migratory fish worldwide, there is a need for a better knowledge and understanding of the patterns, causes and consequences of variation of their migratory behavior. Important challenges addressed in this contribution include to identify how different ecological drivers influence the evolution and variation in migratory behavior, and to illuminate how genetic polymorphism, developmental plasticity, and intra-individual flexibility of migratory behavior influence the response, and ability of individuals, populations and species to cope with environmental change (Figure 1).
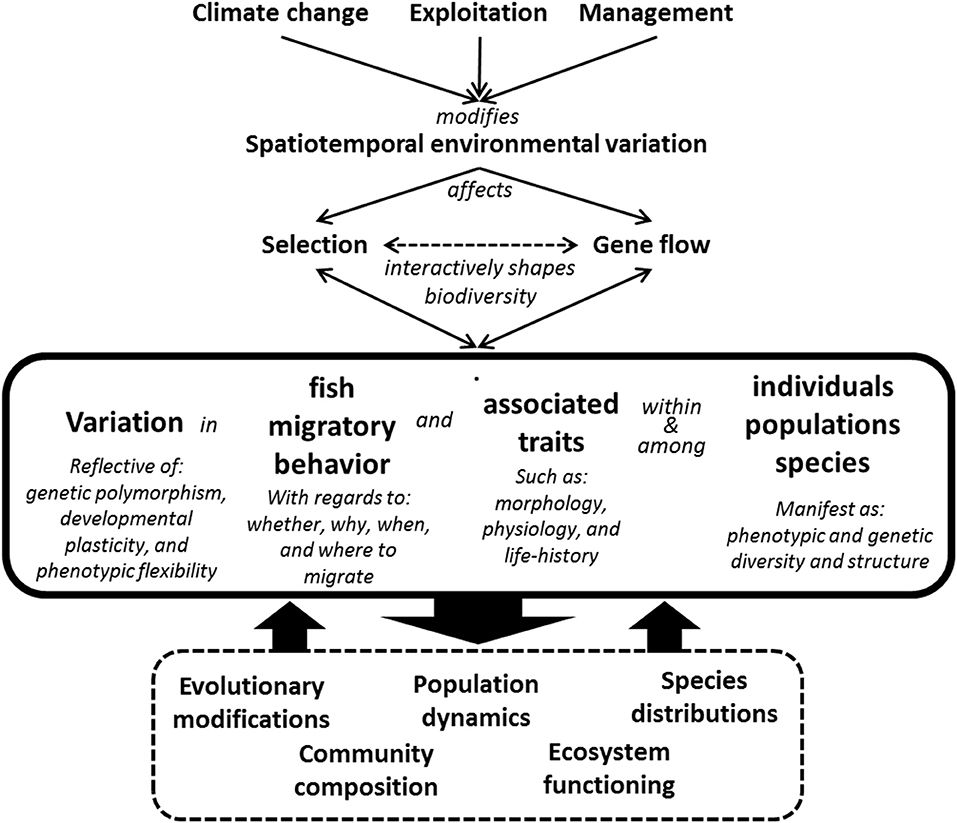
Figure 1. Overview of causes and consequences of variation and flexibility in fish migration. Fish migratory behaviors and associated phenotypic traits vary within and among individuals, populations and species. The phenotypic differences are reflective of the combined contributions of genetic differences, developmental plasticity, and phenotypic flexibility. The costs and benefits of migratory behaviors are context dependent in that they vary according to individual attributes (see Table 1) and are influenced by interactive ecological and evolutionary processes in response to spatiotemporal environmental heterogeneity. Climate change and anthropogenic activities result in environmental makeovers and can further modify the opportunities for migration, the direction and strength of selection, genetic diversity, and structure, population dynamics, species distributions, and community composition. Patterns of variation in fish migratory behaviors thus represent the outcome of complex and dynamic eco-evolutionary feed-back loops.
What's at Stake?
The variation and flexibility in migration strategies in fishes that move between habitats to fulfill competing needs provides a rich and fascinating example of how biological diversity manifests between and within communities, species, populations and individuals (Leggett, 1977; Roff, 1988; Lucas and Baras, 2001; Nathan et al., 2008; Mehner, 2012; Brönmark et al., 2014). As such, fish offer good model systems for investigating how biological systems respond to and cope with environmental heterogeneity and change. Being important predators, competitors, and prey to other species, migrating fish affect the functioning of lakes, rivers, coastal ecosystems and open oceans (Post et al., 2008; Brodersen et al., 2015; Donadi et al., 2017). In some areas, migrating fish represent important “vectors” by transferring nutrients or pathogens between habitats, as in the case of mass-migration and post-spawning death of Pacific salmon that brings energy from resource rich marine habitats to less productive rivers (Naiman et al., 2002). Migrating fish also comprise an important resource of considerable socioeconomic value targeted by commercial and recreational fisheries throughout the world (Oke and Hendry, 2019).
What Are the Key Hazards to Migrating Fish?
Migrating fish are under threat by habitat modification, fragmentation and destruction of spawning and nursery habitats, pollution, and overexploitation (Waldman et al., 2016; Forseth et al., 2017). Apart from immediate negative effects associated with declining populations, changes in distribution ranges, and local extinctions (Dudgeon et al., 2006), exploitation can induce long-term evolutionary shifts in behaviors, individual growth trajectories and life-history strategies. These in turn may affect the recruitment, size-structure and dynamics of populations (Beacham, 1983; Kuparinen and Merilä, 2007; Uusi-Heikkila et al., 2008; Lowerre-Barbieri et al., 2017).
Perhaps counterintuitively, migrating fish are potentially also under threat by various management actions. Even efforts designed to compensate for overexploitation and mitigate the devastating effects of dispersal barriers via removal of dams, construction of fishways, compensatory breeding and supplemental stocking may have unintentional and unforeseen negative consequences. For example, alterations in river connectivity caused by the building and removal of dams or the construction of fishways may bring about changes in community composition and species interactions (Ngor et al., 2018), and in rapid loss of local adaptations (Thompson et al., 2019). Connectivity changes can also affect the directions and rates of gene flow with consequences for genetic diversity and inter-population hybridization (Lynch, 1991; McClelland and Naish, 2007; Whitlock et al., 2013; Rius and Darling, 2014). Similar to fisheries induced evolution (Kuparinen and Merilä, 2007), the altered severity of migration caused by constructed fishways may influence the characteristics of successful migrants and impose selection and evolutionary shifts in traits that directly define migration or dispersal capacity, as well as in other traits that may impair population growth (as discussed and exemplified below).
Selection that gives rise to local adaptations generally reduces phenotypic and genetic variance. This can be detrimental because diversity brings many benefits. Theory and empirical evidence concur that flexibility and variance reducing bet-hedging strategies within individuals and genotypes can increase geometric mean fitness in changing and heterogeneous environments (Slatkin, 1974; Seger and Brockmann, 1987; Forsman et al., 2007). Earlier work unanimously show that among-individual variation contributes to improved establishment, more stable populations, and reduced extinction risk of populations and species, via complementarity and/or variance reducing effects (Hughes et al., 2008; Simberloff, 2009; Forsman, 2014; Forsman and Wennersten, 2016; Des Roches et al., 2018). Lastly, portfolio effects associated with variation among populations across environments or with high species diversity may increase stability, productivity and resilience of species and ecosystems (Schindler et al., 2010, 2015; Waldman et al., 2016; Hui et al., 2017; Lowerre-Barbieri et al., 2017).
Designing adaptive management for protection and sustainable utilization of migrating fish is complicated by ongoing climate change that brings changes in salinity, temperature, precipitation, sea surface levels, and species distribution ranges (Roessig et al., 2004; IPCC, 2013, 2018; Reusch et al., 2018; Cheng et al., 2019), thereby resulting in modified, novel, more variable and extreme selection regimes (Parmesan and Yohe, 2003; Root et al., 2003; Forsman et al., 2016b). The situation for migratory fish is worsened by the challenges (e.g., increased harvesting and habitat destruction) that accompany the increasing demands of a growing human population.
Questions Addressed in This Review
An important task for research is to investigate how the key hazards outlined above disrupt eco-evolutionary processes and the diversity of migrating fish. Scientific output on fish migration has grown tremendously from <100 papers per year prior to 1970 to nearly 3300 papers in 2018 (Figure 2A). The portion of studies addressing aspects of variation and flexibility among and within populations or individuals is relatively low (<10%), but this emerging field has increased 7-fold from <50 papers per year prior to 1990 to > 350 papers per year in 2018 (Figure 2B). This growing appreciation of the potential importance of flexible migration strategies in fish is comparable to that in other organism groups (Figure 2C), and evident also relative to total research output (Figure 2D).Given the rich literature on variation and flexibility in fish migration (Figure 2) it is impossible to provide an all-inclusive summary of current knowledge, and there are already more than 300 reviews touching on various facets of this emerging area. Previous reviews typically focus on specific hypothesis, biomes, taxa, or migratory behaviors to summarize knowledge within a restricted area.
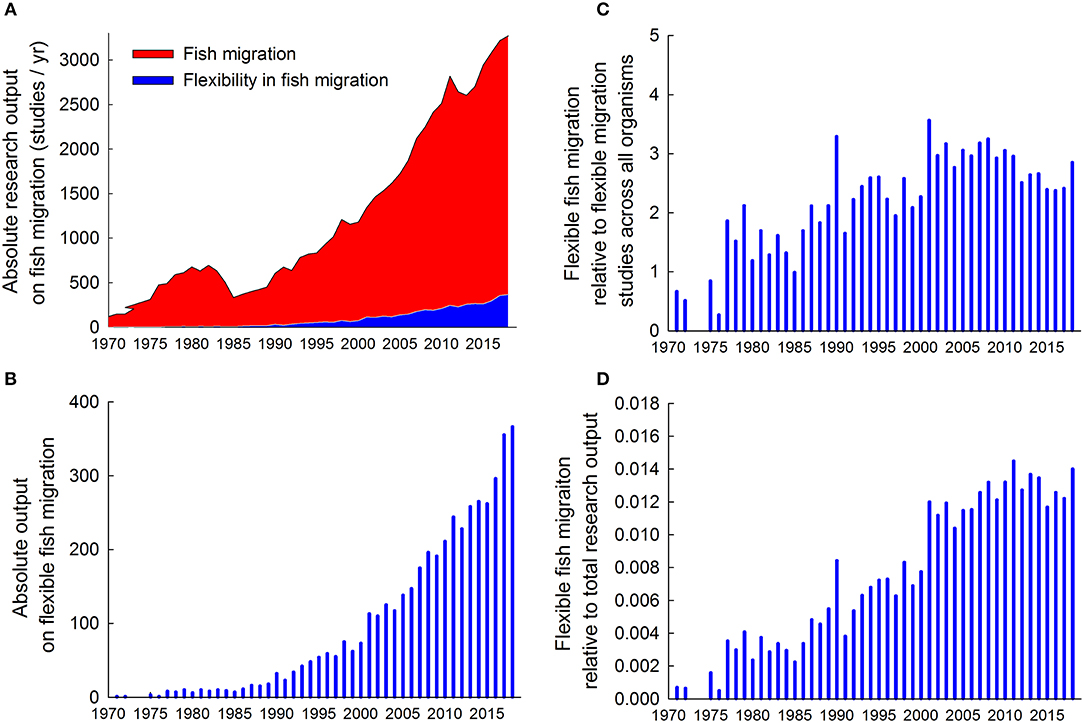
Figure 2. Trends in research output on fish migration. (A) Absolute research output measured as publications on fish migration (red1) and on flexibility in fish migration (blue2) published per year up to December 2018. (B) Absolute research output on flexibility in fish migration2 published per year. (C) Relative research output on flexibility in fish migration expressed as percentage of research output on flexibility in migration summed across all organisms3. (D) Relative research output on flexibility in fish migration2 expressed as percentage of total research output summed across all scientific disciplines4. Data extracted from a topic search conducted 18 February 2019 from all databased in ISI Web of Science using the following search strings:
1((migrat* OR *dromous OR dispers*) AND (fish*)), generated 61,591 papers.
2((migrat* OR *dromous OR dispers*) AND (fish*) AND (plastic* OR flexib* OR partial OR alternat*)), generated 4,967 papers.
3((migrat* OR *dromous OR dispers*) AND (plastic* OR flexib* OR partial OR alternat*)), generated 172,489 papers.
4(“in”), generated 54,424,812 papers.
In this narrative review we provide a broad overview, in which we describe and discuss aspects of variation and flexibility in fish migration of basic scientific interest that concern key issues within the framework of evolution and maintenance of biological diversity (Figure 1). We consider key questions (whether or not to migrate, why to migrate, where to migrate, and when to migrate?) that define migrating fish and other organisms (Nathan et al., 2008), and exemplify how the evolutionary solutions to these questions may vary and change depending on ecological conditions, environmental settings, and individual characteristics. In particular, we explore links between environmental change, and migration strategies, and discuss whether and how threats associated with overexploitation, environmental makeovers, and management actions are likely to differently influence individuals, populations and species depending on the variation and flexibility of their migration strategies. In so doing, we aim to advance knowledge, spur future research and critical evaluation of management strategies, to ultimately promote sustainable utilization and protection of migrating fish and their ecosystems. The disproportionate attention given to different subsections below reflects our subjective interests and concerns, not necessarily the relative importance or state of knowledge.
Variation in Fish Migration–What's at Stake?
Fish migration encompasses a broad range of behaviors and life-history strategies by which individuals, populations and species cope with challenges associated with different scales of temporal and spatial environmental heterogeneity (Figure 1). The growing literature (Figure 2) has resulted in a rich flora of terms and phrases pertaining to various aspects of fish migration (Myers, 1949; Lucas and Baras, 2001; Secor and Kerr, 2009). The increasing interest in developmental plasticity and phenotypic flexibility has also been accompanied by numerous classifications and definitions (Piersma and Drent, 2003; West-Eberhard, 2003; O'Connor et al., 2014; Forsman, 2015; Senner et al., 2015). Below we provide a brief overview and reintroduce some definitions and key concepts related to variation and flexibility of fish migration.
Definitions and Key Concepts in Fish Migration
Migration involves bi-directional large- or small-scale movements by individuals between habitats that fulfill competing needs that may occur within and between different life-stages. The habitats and resources that maximize growth, survival and reproductive success during different life history phases are typically separated in time and space (Gross et al., 1988). Migration is often interpreted as an adaptive response, although discriminating adaptive optimal migration solutions from “non-adaptive” movements induced by external or internal stressors can be difficult. Benefits from migratory movements may come in the forms of refuge from predators, access to resources, or strategic positioning of gametes in locations that offer advantageous conditions for the developing embryos and offspring. Potential costs include the energy expenditure associated with moving, predation risk, osmoregulation, erroneous navigation, and impaired reproductive success owing to genetic incompatibility associated with inter-population hybridization.
Migration tactics vary between species, among populations, and among individuals within populations. In “Migration of Freshwater Fishes,” Lucas and Baras (2001) define migration as: “a strategy of adaptive value, involving movement of part or all of a population in time, between discrete sites existing in an n-dimensional hypervolume of biotic and abiotic factors, usually but not necessarily involving predictability or synchronicity in time, since inter individual variation is a fundamental component of populations.” However, the classification and understanding of fish migration is complicated further by an intra-individual component of variation, meaning that migration strategies can change also over an individual's life. Despite the extensive variability, some general migration patterns can be discerned.
Main Migration Modes
Fish migration modes can be described on the basis of the fresh- and salt water biomes used (Figure 3). These include holobiotic lifestyles, meaning that the fish spend their entire lifespan in either salt or fresh water, and amphibiotic lifestyles, meaning that the fish move between water bodies with different salinities (Lucas and Baras, 2001).
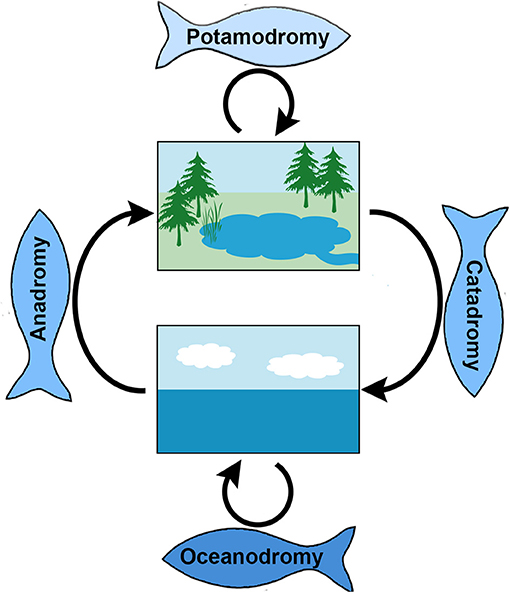
Figure 3. Spawning migration modes in fish classified based on the use of biomes (freshwater vs. brackish or saline). Oceanodromous fishes live and migrate to feed and reproduce wholly in the sea (lower box). Potamodromous fishes migrate between reproductive areas and feeding grounds entirely within fresh water (upper box). Diadromous fishes migrate between fresh and salt water to complete their life cycle; these are classified as anadromous if they spend most time in freshwater but migrate to marine environments to reproduce, and as catadromous if they instead spend most time in the sea and migrate into freshwater to reproduce. The figure was created in Adobe Photoshop CC 2015 v. 16.0.1.
Oceanodromous fishes live and migrate wholly in the sea (Myers, 1949; Lucas and Baras, 2001). Well-known examples include small prey fish such as sardine (Sardina pilchardus), anchoveta (Engraulis encrasicolus), herring (Clupea harengus) but also larger fishes at higher trophic levels, pelagic species with wide distributions such as tuna, sailfish, marlin, swordfish, sharks, and rays that undertake variable but often long-distance migrations for feeding or reproduction.
Potamodromous fishes migrate between natal areas and feeding grounds entirely within fresh water. Although these fish typically migrate relatively shorter distances, these movements across habitats within freshwater may be just as important for survival, growth and reproduction as the typically larger scale migrations partaken by oceanodromous or diadromous species. There are also potamodromous species with extensive migrations; spawning migration distances of 300 km have been recorded for the endangered Colorado pike minnow of the Colorado River system (Lucas and Baras, 2001).
Diadromous fishes migrate between fresh and salt water environments to complete different parts of their life cycle (Lucas and Baras, 2001; Griffiths, 2006, 2010) (Figure 3). Catadromous fish spend the majority of the time feeding and growing in freshwaters and migrate into the saline sea water as adults to reproduce. Famous examples are the freshwater eels of the genus Anguilla, including the iconic European eel (Anguilla anguilla L.), which spawns in the Sargasso Sea and whose offspring drift across the Atlantic Ocean to the coasts and freshwaters of Europe and North Africa where they will grow and mature, before returning to the Sargasso Sea to reproduce (Moyle, 2004; Aoyama, 2009). Another catadromous species is the Indo-Pacific barramundi (Lates calcarifer) that inhabits rivers before returning to the river mouths or estuaries to spawn, and where the larvae and juveniles live in the associated brackish temporary swamps (Russell and Garrett, 1983). Anadromous fishes spend the majority of the time feeding and growing in the sea and move into freshwater to reproduce. Well-known examples can be found among salmonids, such as Atlantic salmon (Salmo salar) that exploit the rich resources of the ocean as adults, only to return to the natal river or stream to reproduce. Additional examples include various species of Pacific salmon, striped bass (Morone saxatilis), and sea lampreys (Petromyzon marinus) (Moyle, 2004; Silva et al., 2014).
Not all fish species fall easily into the above categories. Species showing pronounced intraspecific variation include some salmonids (S. trutta), the three-spine stickleback (Gasterosteus aculeatus), and the northern pike (Esox lucius) in which different populations of the same species can be classified as belonging to at least two of the oceanodromous, potamodromous, and the anadromous lifestyles (Jonsson and Jonsson, 1993; Fleming, 1996; Lucas and Baras, 2001; Dodson et al., 2013; Forsman et al., 2015; Leitwein et al., 2016). There is also extensive variation in timing and distance of migration among species and populations (McDowall, 1997; Hendry and Day, 2005; Kuparinen and Merilä, 2009; Griffiths, 2010; Seamons and Quinn, 2010; Kovach et al., 2015; Forsman and Berggren, 2017; Bloom et al., 2018).
Spawning Migration
While fish migration takes countless shapes and can be described based on utilization of different biomes (Figure 3), it is sometimes fruitful to analyze and classify them from a functional viewpoint. In principle, the main drivers of large scale fish migrations are to reproduce, find food, and avoid enemies. Although any habitat shifts must be interpreted as representing the outcome of these competing needs, fish migrations are typically classified based on reproduction.
Spawning-, reproductive- or breeding migrations involve the movements of reproductively mature fish from foraging areas to a location where they will place their gametes. For a spawning environment to be productive, it should provide abiotic and biotic conditions that are favorable for the development and survival of fertilized eggs, embryos, hatched larvae, and young juveniles (Lowerre-Barbieri et al., 2017) (Figure 1). Because of differential needs and demands depending on size and age, the nursery habitat progressively becomes suboptimal. As the fish grow larger and older, they eventually leave the nursery grounds in favor of more productive foraging grounds where they likely join the adult population. Spawning migration may involve the crossing of the borders between fresh, brackish, and saline water bodies, but can occur within such biomes, for example between or within lakes and rivers (Figures 3, 4).
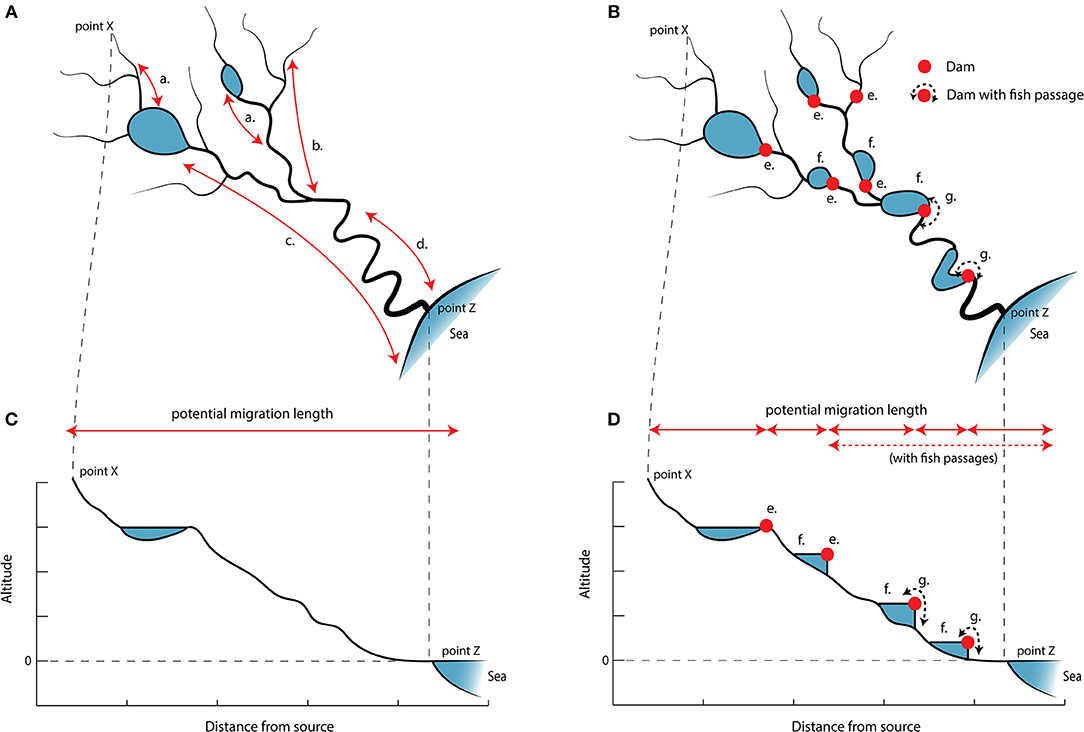
Figure 4. Variation in fish migration and consequences of exploitation and management actions. (A) A river system showing the principal modes of migration for fish inhabiting the system. Fish migrate (a) between lakes and rivers, (b) between larger and smaller parts of the river, (c) between sea and lakes, and (d) between the sea and river. (B) When dams (e) are added to a river system, previous migration routes become disconnected and the total amount of freely available habitat fragments becomes smaller. Damming structures create impoundments (f) that store water, converting lotic habitats to lentic habitats. Connectivity can be partially restored by adding fish passages (g) that enable fish to pass obstacles, but the impoundments created upstream of damming structures persist even though fish passages are built. The height profiles (C,D) drawn from point X to point Z illustrate how damming changes the large-scale structure of a river system to a series of steps. The potential migration length for freshwater fish in the river system is severely shortened, limiting access to areas that may provide better opportunities for growth, survival, or reproduction for fish.
Homing Behavior and Navigation
Some fish display homing behavior. After having reached maturity, the adults may return to spawn where they were born. In iteroparous species, the adults may reuse the same spawning grounds for multiple reproductive cycles (e.g., Tibblin et al., 2016b). Homing is not an obligatory part of fish migratory behavior (Lucas and Baras, 2001). However, it can allow for evolution of genetic structure, local adaptations, and divergence of early life-history traits among subpopulations that use different spawning areas, and thereby reinforce the benefits of homing (Jensen et al., 2008; Kavanagh et al., 2010; Petersson, 2015; Tibblin et al., 2015, 2016a; Berggren et al., 2016; Mäkinen et al., 2016; Sunde et al., 2018a). This showcases how varying environmental conditions and behaviors can shape biodiversity even on small spatial scales.
The mechanism(s) involved in navigation, identification and habitat recognition that allow for homing behavior in fish have been reviewed elsewhere (Lucas and Baras, 2001; Odling-Smee and Braithwaite, 2003; Keefer and Caudill, 2014; Petersson, 2015). Receptors sensitive to electric and geomagnetic fields, light, temperature, olfactory and visual cues together with information based on landmarks, water flow, and sound seem to be involved to various degrees by different species (Lucas and Baras, 2001; Keefer and Caudill, 2014).
Below, we illustrate how migratory behavior may vary among and within species of fish (Table 1). We also exemplify how variation and flexibility in migratory behavior may be associated with, and possibly depend on, spatiotemporal environmental heterogeneity and vary according to individual characteristics (Table 1; Figure 1).
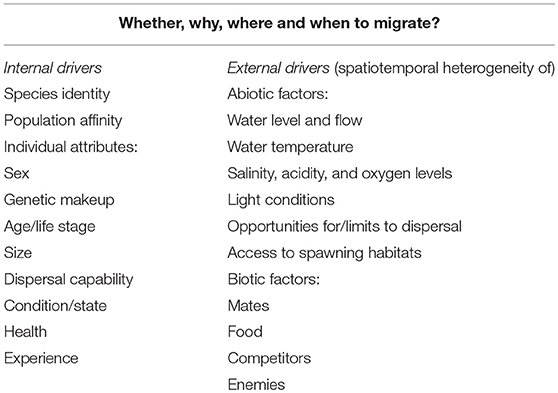
Table 1. Overview of potential correlates and putative internal (left column) and external (right column) drivers of variation and flexibility in migration behavior in fishes.
Variation Among Species
Patterns and strategies of migration vary extensively among species with regards to function (e.g., spawning, feeding, and refuge from predators and other environmental stressors), migration mode (diadromous, potamodromous and oceanodromous), mode of parity (semelparous-iteroparous), timing of migratory events (phenology), and migratory distance (McDowall, 1997; Griffiths, 2010; Seamons and Quinn, 2010; Mehner, 2012; Forsman and Berggren, 2017; Nilsson et al., 2019). As for diadromy, inter-specific comparisons have uncovered that anadromous species predominate in temperate latitudes where productivity in freshwater is generally lower than in marine environments whereas catadromy dominates in tropical latitudes where the highest productivity is found in freshwater habitats (Gross et al., 1988; McDowall, 1997). Similarly among potamodromous fish, many species utilize rivers as spawning and nursery grounds whereas foraging occurs in more productive areas such as lakes. Drivers other than productivity are also important in shaping the mode or direction of migration in fish (Bloom and Lovejoy, 2014). Recent evidence from a comparative analysis indicate that across Clupeiformes (anchovies, herring, shad and allies) diadromous species are larger than non-diadromous species, whereas no association was found with trophic position (Bloom et al., 2018).
With regards to migration timing, Kovach et al. (2015) report that temporal trends in the direction of the shift in the median migration date, as well as in duration and inter-annual variation in migration timing are highly variable across species and populations of Pacific salmon. The drivers resulting in the diversity of migration strategies seen across fish species are poorly understood but presumed to be the result of improved access to resources in a patchy system or decreased predation. With regards to distance, pike, and goliath catfish (Brachyplatystoma rousseauxii) offer an example of a striking difference in freshwater migration distance between anadromous species. Both species inhabit coastal estuarine areas and migrate to spawning locations upstream. For pike, maximum migration distance in freshwater is probably <50 km (Larsson et al., 2015), whereas the goliath catfish that spawns in the western Amazon travels 11,500 km, the longest fish freshwater migration in the world (Barthem et al., 2017). In the catadromous Anguilla eels, migration distances from freshwater to the marine spawning areas range from 750 to > 8,000 km (see Table S1 in Forsman and Berggren, 2017). Results from a comparative analysis indicate that the evolutionary increments of migration distances in Anguilla have been accompanied by shifts in dispersal enhancing phenotypic traits, such as larger body size (Forsman and Berggren, 2017). Phylogenetic comparative analysis also point to a role of thermal biology for migration distance. Watanabe et al. (2015) showed that species that are able to maintain red muscles warmer than ambient temperatures swim faster and have longer annual migration distances compared with similar sized species of fish without red muscle endothermy (i.e., the vast majority of fishes).
Even though different species may share the same modes of migration there can be differences in where and when alternative strategies (residents and anadromous) are sympatric or allopatric. For example, salmonid and esocid species are phylogenetically relatively close (Rondeau et al., 2014), and their resident and anadromous populations are partially sympatric (Craig, 1996; Fleming, 1996; Quinn, 2005; Jonsson and Jonsson, 2011; Skov and Nilsson, 2017). However, salmonids are sympatric during spawning and early life-stages in the recruitment habitat, whereas in esocids the resident and anadromous phenotypes are sympatric during the adult life-stage in the foraging habitat (Engstedt et al., 2010; Forsman et al., 2015; Tibblin et al., 2015).
Insights about the causes and consequences of migration behavior in fishes might be gained by studying species and populations that do not migrate, or do so to a lesser extent. This opens for phylogeny based comparative approaches (Felsenstein, 1985) that may inform about large scale evolutionary dynamics of migration behavior in fishes. Although tedious to perform, the compilation and analysis of data within a phylogenetic framework may pay dividends in the long run. For example, such approaches may uncover how migratory behavior data deficiency is distributed across and within different clades of fishes, and thereby help identify taxa and geographic regions in particular need of further investigation. Given a sufficient number of independent evolutionary modifications, phylogenetic comparative approaches can help identify why certain species migrate whereas others do not (McDowall, 1997; Bloom et al., 2018). Apart from uncovering associations of migration behaviors with external environmental factors, there is potential for phylogeny based comparisons to reveal whether evolutionary shifts in migration have been accompanied by correlated modifications of morphological, physiological, or behavioral phenotypic dimensions (Watanabe et al., 2015; Forsman and Berggren, 2017; Bloom et al., 2018) (Figure 1).
The diversity of migration behaviors among species outlined above is impressive. However, identifying generality is complicated by the extensive variation seen also within species.
Variation Among Populations
There is considerable variation in spawning migratory patterns among populations within species (Jonsson and Jonsson, 1993; Griffiths, 2006; Dodson et al., 2013). Different populations have different evolutionary histories, and are exposed to different selection pressures depending on the environment they inhabit (Berggren et al., 2016; Sunde et al., 2018a). Accordingly, different populations can adopt different migration tactics and, in some cases, display a level of variation that is comparable to that observed between species. In several salmonid species (e.g., S. salar, S trutta, O. mykiss, O. tshawytscha, and Salvelinus alpinus) populations differ in migration mode (ranging from anadromous, potamodromous to residents in either streams or lakes) (Jonsson and Jonsson, 1993; Fleming, 1996; Lucas and Baras, 2001; Dodson et al., 2013; Leitwein et al., 2016), and the number of migratory events vary according to mode of parity (Unwin et al., 1999; Narum et al., 2008; Seamons and Quinn, 2010; Dodson et al., 2013). This large-scale variability at the population level has been attributed to life-history evolution being shaped by stage-specific mortality and resource availability (McDowall, 1997; Kindsvater et al., 2016). Variation in migration modes among populations has also been documented in cyprinids, esocids, gasterosteids, gadids, and percids (Nordahl et al., (in press); Lucas and Baras, 2001; Tibblin et al., 2012).
Populations commonly vary also in the timing and distance of migratory events (Kinnison et al., 2001; Hodgson and Quinn, 2002; Quinn, 2005; Kuparinen and Merilä, 2009; Kennedy and Crozier, 2010; Jonsson and Jonsson, 2011). This has been suggested to reflect in part phenotypic flexibility (Forsman, 2015) in response to environmental conditions (e.g., temperature, precipitation, light regime and water flow) along latitudinal and altitudinal gradients and local climate (Hodgson and Quinn, 2002; Dodson et al., 2013), but a growing body of evidence suggests that genetic components are also involved (Skov et al., 2010; Plantalech manel-la et al., 2011; Kovach et al., 2012; Thompson et al., 2019). Crossin et al. (2004) showed that migratory distance of populations of sockeye salmon (O. nerka) within the Fraser river ranged from <100 km to >1,100 km, and that the severity of migration (distance and elevation) was associated with higher densities of somatic energy and a more fusiform, streamlined body shape. A similar pattern has been documented in roach (Rutilus rutilus) with migratory populations having a more slender body shape than resident ones (Chapman et al., 2015).
An important task for future research is to determine whether the occurrence of populations with different migration strategies within a species buffers against environmental challenges. Predictions from theory, evidence from manipulation experiments, and results from comparative analyses concur that populations and species with higher phenotypic and genetic diversity are better able to cope with environmental changes and less extinction prone (Hughes et al., 2008; Bolnick et al., 2011; Wennersten and Forsman, 2012; Forsman, 2014, 2015; Forsman and Wennersten, 2016). However, it has not yet been systematically investigated whether these benefits apply also to variation and flexibility of migratory behavior in fishes. To achieve this, information on migration behaviors must first be compiled for multiple populations and species. The large number of studies of variation and flexibility in fish migration identified by our literature search (Figure 2) opens for such future systematic reviews and for meta-analytical approaches that can be used to summarize information, identify patterns, and evaluate potential drivers of variation in migration mode, migration timing, and migration distance among populations (Gurevitch et al., 2018). Results from such endeavors may also help identify the need for and inform population specific management and conservation efforts.
Variation Among and Within Individuals
Variation in migratory behaviors among individuals within populations can also provide insights into the underlying mechanisms and functional significance of migration (Wilson, 1998). Spawning migrating and resident phenotypes sometimes coexist within the same population, a population level phenomenon called partial migration (Brodersen et al., 2007; Chapman et al., 2011a; Dodson et al., 2013; Brönmark et al., 2014). Such partial migration may offer good opportunities to study both the causes and consequences of migration, and suggests that sometimes not migrating is adaptive for an individual in an otherwise migratory population, and further that partial migration is an evolutionary stable strategy. Whether individuals chose to migrate or not is influenced by numerous interacting environmental variables (e.g., resource availability, predation risk, water flow and temperature) and individual characteristics (e.g., growth rate, size, age, lipid content, life history stage, personality, and previous reproduction efforts), as well as by genetic variation in the sensitivity to the external and internal cues (Chapman et al., 2011a,b; Skov et al., 2011; Dodson et al., 2013; Brönmark et al., 2014) (Table 1; Figure 1). For example, Olsson et al. (2006) showed that migration could be environmentally induced by translocating individuals between two habitat patches that differed in density and opportunities for individual growth.
In iteroparous species that engage in multiple migratory spawning events there is potential for phenotypic flexibility (Forsman, 2015), such that individuals change and modify their migratory behavior (Brodersen et al., 2014). Intra-individual flexibility in migratory behavior has recently received increased scientific attention (Figure 2), especially in birds. Evidence is accumulating that flexibility is key to cope with the challenges associated with anthropogenic impacts such as climate change and exploitation (Arnaud et al., 2013; Winkler et al., 2014). Yet, individual flexibility in migratory behavior and timing of fish remains largely overlooked (Tibblin et al., 2016b). Studies of roach, an iteroparous species that displays partial migration, suggest that individuals are consistent rather than flexible across years in whether to migrate or not, thus implying that residency and migration can be stable strategies (Brodersen et al., 2014). This consistency can either be attributed to genetic differences or to initial plasticity, possibly caused by variation in somatic condition, followed by canalization with the latter gaining some support in the roach system (Brodersen et al., 2014).
With regards to phenotypic correlates of timing of spawning migration (Table 1; Figure 1), a common pattern is that males migrate, and subsequently arrive in the spawning habitat, earlier than females (Morbey and Ydenberg, 2001; Tibblin et al., 2016b), possibly because males strive to maximize the number of mating opportunities. Migratory timing may also be associated with body size. Larger size is associated with early migration in both juvenile and adult life-stages of salmonids (Heim et al., 2016; Jonsson et al., 2017), but Tibblin et al. (2016b) report the opposite pattern in pike. Reversible phenotypic flexibility can be selected for and evolve in environments that change throughout an individual's lifetime. Models predict that organisms that are long-lived relative to the rate and frequency of environmental changes should be more flexible, compared with short-lived organisms. It has been suggested that causality may be bidirectional because flexibility itself may select for longevity. Simulation models suggest that under highly auto-correlated environmental fluctuations, reversible flexibility should coevolve with lifespan (Ratikainen and Kokko, 2019). To our knowledge, it has not yet been investigated whether reversible flexibility in migration strategies is more common in long-lived species of fish.
Besides the long-term and often larger scale seasonal migratory movements between areas used for breeding and non-breeding purposes, many fish engage in migrations at smaller spatial, and temporal scales (Lucas and Baras, 2001; Mehner, 2012). Daily migratory movements for utilizing reoccurring and predictable windows of available resources and favorable conditions are particularly common. Many marine-, brackish-, and freshwater fish show such diel vertical migrations, rising to the surface to feed during night and diving to deeper layers during the day (reviewed in Lucas and Baras, 2001; Mehner, 2012). Some species instead utilize the near surface waters during the daytime and return to bottom layers in the evenings to feed. Other proximate triggers of vertical migrations include light intensity and water temperature, and ultimate drivers encompass bioenergetics efficiency, foraging opportunities and predator avoidance (Mehner, 2012; Nordahl et al., 2019). Horizontal fish migrations include movements between shallow, inshore littoral areas and offshore pelagic areas performed by fishes in larger lakes. Such horizontal movements are often cyclical on a daily basis, with shifts from offshore to inshore areas at night, or in the reverse direction. It is generally believed that such rhythmical diel shifts are driven by a trade-off between foraging and avoiding being fed upon (Lucas and Baras, 2001; Mehner, 2012).
Migrating between water bodies also offers a means to buffer against changing external physicochemical conditions, maintain internal homeostasis and regulate body temperature to conserve energy expenditure or to maximize aspects of performance (Reynolds and Casterlin, 1980; Nakamura et al., 2015; Pépino et al., 2015; Nordahl et al., 2018, 2019). Observations of diel horizontal migrations in juvenile coho salmon (Oncorhynchus kisutch) indicate that individuals that moved to warmer habitats after feeding processed their food more quickly and grew faster compared with individuals that adopted other behaviors (Armstrong et al., 2013). A behavioral study of pike has shown that individuals surface during daytime and seek out deeper waters during night in the summer, whereas the direction is reversed during winter, thus pointing to a possible role of sun basking (Nordahl, 2018; Nordahl et al., 2019).
A recent study of carp (Cyprinus carpio) demonstrates that sun basking close to the surface during sunny conditions enables fish to increase their body temperature above that of the ambient water, and further indicates that the temperature excess gained by basking enabled the fish to grow faster (Nordahl et al., 2018), thereby putting the individual in a favorable situation compared to those not expressing this behavior. The discovery that sun basking can offer thermoregulatory benefits even in aquatic environments (Nordahl et al., 2018, 2019) is likely to spur future research and may ultimately change the way we think about fish ecology and evolution, in particular with regards to behaviors and migrations.
Longitudinal studies have contributed with knowledge regarding how migratory behavior may be modified in response to environmental cues (Table 1; Figure 1). Forsythe et al. (2012a) and Forsythe et al. (2012b) studied associations between external factors and individual timing of spawning migration in lake sturgeon (Acipenser fulvescens) across 8 years and showed that individuals adjust their timing according to lunar cycle, water flow and temperature. These last results might be interpreted as an indication that flexibility is adaptive, but firm evidence to that effect is scarce, mainly for logistical reasons (Forsman, 2015). However, a recent study of pike migratory behavior has shed some light on this matter. Data on recapture rates of pike suggests that the timing of arrival to the spawning area is under stabilizing viability selection, and that individuals that are more flexible in their timing during the 1st years survive longer compared with less flexible individuals (Tibblin et al., 2016b). Besides extensive research on how abiotic cues influence migratory timing it has been proposed that timing may be modulated by social interactions. Work by Berdahl et al. (2017) suggests that migratory timing in sockeye salmon was better explained by social interactions (group migration) than by abiotic cues such as temperature and river flow. Environmental influences aside, there is evidence emerging that timing can be under genetic control and undergo rapid evolutionary change (Thompson et al., 2019). There is also potential for variation among individuals in the timing of spawning migration to contribute to population genetic structure; isolation by time rather than isolation by distance (Hendry and Day, 2005). Whether isolation by time is a common driver of genetic divergence and adaptation in fish, and whether differences in the timing of spawning migration contributes more or less to population structure in different species depending on their life-history (e.g., discrete or overlapping generations) remains to be investigated.
Phenotypic Correlates of Migratory Performance
A plethora of studies have aimed to identify phenotypic correlates of swimming performance and the evolution of adaptations facilitating migratory behavior. Collectively, results point to important roles of morphological (e.g., body size, body shape, number of vertebrae, spool width, and size and shape of fins) and physiological traits that influence aspects of performance (e.g., swimming capacity, acceleration, endurance, and ability to sustain high water velocities), and of behavioral (boldness, and latency to pass obstacles) traits (Webb, 1975; Swain, 1992; McDowall et al., 1994; Fleming, 1996; McDowall, 2003; Crossin et al., 2004; Haugen et al., 2008; Long et al., 2011; Chapman et al., 2015; Podgorniak et al., 2016, 2017; Tibblin et al., 2016a; Forsman and Berggren, 2017; Hall, 2018; Aguirre et al., 2019). There is also potential for indirect evolutionary responses of phenotypic dimensions that are genetically or developmentally correlated with dispersal enhancing traits (see “Construction of Fishways”).
On Genetic Polymorphism, Developmental Plasticity and Phenotypic Flexibility
The differences in migration behaviors, or any other traits, between species, populations, and among individuals within populations discussed above may be seen as representing the combined outcomes of underlying genetic polymorphisms, developmental plasticity and phenotypic flexibility (Piersma and Drent, 2003; West-Eberhard, 2003; O'Connor et al., 2014; Forsman, 2015; Senner et al., 2015). The concept of phenotypic plasticity is deceptively simple, and has been previously defined in numerous ways by different authors [see for instance Box 1 in Whitman and Agrawal (2009)]. The consequences of plasticity and flexibility for the performance and success of individuals, populations and species continue to attract a growing interest (see Figure 1 in Forsman, 2015). Here, we distinguish between irreversible developmental plasticity and reversible intra-individual phenotypic flexibility (Piersma and Drent, 2003; Forsman, 2015).
Developmental plasticity is used primarily for irreversible phenotypic variation in traits of individuals (or genotypes) that result from environmentally induced modifications of development and growth (Stearns, 1989). Developmental plasticity can also involve mechanisms that operate across generations. When the phenotype is induced by the female parent, the plasticity is usually referred to as maternal effects (Roff, 1997; Mousseau and Fox, 1998). Cross generational plasticity can also be mediated by the male parent (e.g., Kekalainen et al., 2018).
Phenotypic flexibility is used for reversible changes within individuals of labile, context-dependent physiological, morphological, or life-history traits (Piersma and Drent, 2003; Forsman, 2015). It is applicable also to behavioral traits, for instance as a result of previous history, learning, and experience, or adjustments to external conditions that influence current responses and behaviors in given situations (Dingemanse et al., 2010; Tuomainen and Candolin, 2011; Snell-Rood, 2013).
Plasticity and flexibility are not fundamentally distinct from genetic polymorphisms (Leimar et al., 2006; Forsman, 2015). Crossing norms of reaction, when different genotypes display different phenotypic responses to environmental change, are manifestations of underlying genetic polymorphisms (Pigliucci, 2001; West-Eberhard, 2003). It is often difficult to disentangle the contribution of genetic and non-genetic sources of variation. Demonstrations of trait heritability alone do not provide conclusive evidence that differences among individuals or populations have a genetic basis. Conversely, failure to demonstrate a role of developmental plasticity for a given trait in response to a given environmental factor does not necessarily imply that the trait is insensitive also to other factors.
As we have seen, variation in fish migratory behaviors manifests at different hierarchical levels and at different spatiotemporal scales, and can be of genetic and/or environmental origin. In the following sections, we discuss how this may contribute to the viability of species and resilience of ecosystems. Safeguarding against key hazards requires management actions that do not raze, but promote variance-coping mechanisms. Unfortunately, management and conservation actions aimed to mitigate the devastating effects of key hazards for migrating fish can themselves disrupt natural processes and threaten biodiversity, as discussed below.
Key Hazards and How They Disrupt the Natural Processes That Underlie Diversity
The environmental heterogeneity that has shaped evolution of fish migration behaviors is modified by anthropogenic activities and climate change. Threats associated with such makeovers, overexploitation and management actions may differently influence individuals, populations and species depending on their migration strategies (Figure 1). The variance reducing effects that diversity at different hierarchical levels of biological organization have on productivity (see Introduction for references) must inform decision making regarding utilization and protection of migratory fish and the ecosystem services they provide.
On the Roles of Exploitation, Environmental Makeovers, and Management Actions
Dams and Hydroelectric Power Plants
Damming is a major threat to migratory fish, biodiversity, and ecosystem functioning. Damming is one of the most widespread environmental alterations of river ecosystems, affecting about half of all large river systems globally (Nilsson et al., 2005; Grill et al., 2015). Consequences include habitat fragmentation, loss and degradation, and changed hydrological regimes. Fragmentation resulting from damming in rivers is particularly troublesome because aquatic organisms are limited to linear pathways and cannot find another route unless one is provided. River systems comprise diverse communities of fish with many migration modes, partaken on different spatial and temporal scales and between different habitats (Figure 4A). Dams and other obstacles reduce river connectivity and hinder both small and large migratory movements for most species (Figure 4B). Although likely to be more common than recorded in the scientific literature, there are examples indicating that dams and inability to migrate cause local extirpations of populations (Winston et al., 1991; Holmquist et al., 1998; Morita and Yamamoto, 2002; Locke et al., 2003). Obstacles can potentially also constrict larger scale migrations such as poleward or altitudinal range shifts that many species are undertaking to evade effects of climate change (Comte and Grenouillet, 2013).
When connection between freshwater and marine habitats is removed, the persistence of anadromous species depends on whether they can switch to a more resident strategy. Species that would have utilized the ocean as foraging grounds but gets landlocked may change to a freshwater resident behavior or disappear from the freshwater system altogether. Such switches may lead to evolutionary divergence. For example, comparisons of juvenile alewives (Alosa pseudoharengus) have shown that anadromous life history forms are more robust compared with fish in landlocked freshwater resident populations that have a more fusiform body shape, pointing to a parallel divergence mediated by shifts in zooplankton prey (Jones et al., 2013). Catadromous species that utilize freshwater habitats as foraging and nursery grounds may get locked out in the ocean and extirpated from inaccessible freshwater systems (Harris et al., 2016). Potamodromous species are also affected by migration barriers (Branco et al., 2017) (Figure 4B) as most species migrate between habitats used for growth, survival or reproduction (Lucas and Baras, 2001). Fish with flexible migration strategies are likely more persistent during such severe environmental makeovers as they may adjust migratory behaviors to novel regimes.
Habitat Fragmentation, Conversion and Loss
Obstacles can be definitive or partial dispersal barriers, depending on the severity of the obstacle and the swimming capabilities of the fish. Naturally, obstacles are often harder to traverse in the upstream direction, while weirs and spillways may allow for some downstream dispersal. This unidirectional dispersal constricts gene flow in the upstream direction and reduces genetic diversity in the upstream direction (Gouskov et al., 2016; Van Leeuwen et al., 2018). Small populations upstream of dams, with no possibility for immigration or recolonization from downstream populations, may also be extirpated (Morita and Yamamoto, 2002). Depending on the severity of the upstream and downstream barriers, movement becomes restricted and gene flow between fragments reduced. This can lead to population differentiation among fragments and manifest as local population structures between barriers (Van Leeuwen et al., 2018). Reductions in the number, size, and type of available habitats (Figure 4C vs. Figure 4D) will reduce the size of the local populations that can be sustained between barriers, with consequences for genetic diversity, divergence, and viability of populations (Carim et al., 2016).
Inundation, the creation of reservoirs upstream dams (Figures 4B,D), can impact river communities (Geist, 2011) and cause a shift from lotic to lentic fish assemblages. Impoundments upstream of damming structures persist despite attempts to restore connectivity through fish passage solutions (Figures 4B,D), and the lentic habitats created upstream can in themselves continue to pose large migratory challenges (Jepsen et al., 1998; Olsson and Greenberg, 2004). As a consequence of complete or partial conversion of lotic to lentic habitats by inundation (Figure 4), lotic habitats also become less frequent and spaced further apart (Aarts et al., 2004), reducing available suitable habitats for species that depend on running waters. Local populations whose structure and temporal dynamics is governed by meta-population processes may be particularly sensitive to river fragmentation (Rieman and Dunham, 2000) because damming increases isolation of “islands.”
Construction of Fishways
Management actions to alleviate the negative impacts on migrating fish of impaired connectivity, river fragmentation and habitat destruction discussed above include the fitting of fauna passage solutions to damming structures and compensatory breeding, both of which may also have undesirable consequences. A fishway is a type of passage that, usually, consists of engineering solutions that reroute part of the water around obstacles to offer an alternative migration route and “free passage” for the fish (Birnie-Gauvin et al., 2018), a goal that is practically unreachable because fishways themselves entail a barrier of sorts. A more grounded goal would be that fishways should enable a wide range of genotypes and phenotypes to pass, such that populations can maintain their evolutionary potential. The innate tradition of fishway retrofitting to avoid negatively affecting the damming structures or the hydroelectric power production generally results in compromised designs and a performance that is suboptimal.
Although fishways improve possibilities for spawning migration (Gouskov et al., 2016; Tamario et al., 2018), they seldom result in the desired level of connectivity restoration (Brown et al., 2013; Foulds and Lucas, 2013; Birnie-Gauvin et al., 2018; Silva et al., 2018; Tamario et al., 2019) and are not fully and equally permeable for all species, ages and phenotypes (Haugen et al., 2008; Volpato et al., 2009; Birnie-Gauvin et al., 2018). The altered severity of migration caused by fishways, and other types of partial dispersal barriers (Newton et al., 2018), may thus impose selection by favoring certain phenotypes and disfavoring others, and thereby impact on the phenotypic and genetic composition (Figure 5). Fishways that are harsh to traverse may cause size selection with evolutionary consequences similar to that of size-selective fishing, with average size and variation in sizes decreasing over time (Haugen et al., 2008; Maynard et al., 2017). The loss of phenotypic diversity can be surprisingly rapid and observable over just a few decades (Haugen et al., 2008). Similarly, fish passage solutions for eels usually consist of ramps lined with a homogeneous climbing substrate that may favor the sinusoid movements and climbing performance of eels of a certain size (Podgorniak et al., 2017). Podgorniak et al. (2017) report that eels upstream of fish passage solutions showed less variation in size than eels below, and that different climbing substrates may select for different sizes. Such climbing substrates vary widely in their efficiency (Watz et al., 2019), and ignorance of best technical solutions in management likely leads to reduced fishway performance, stronger selection, and higher culling of variation.
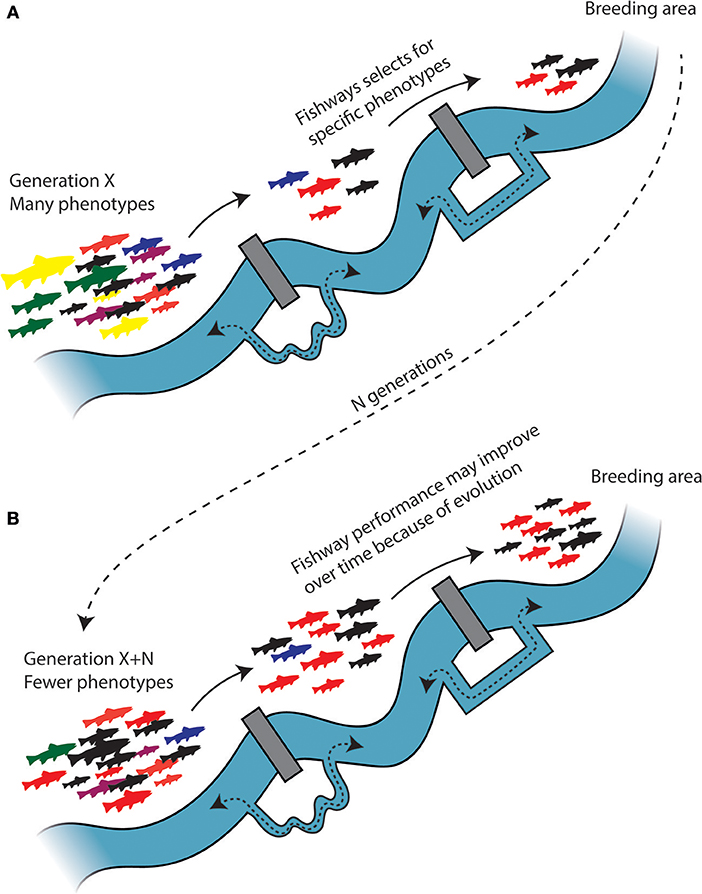
Figure 5. Fishways impose selection and can induce evolutionary modifications of spawning migrating fish. (A) The spawning migrating fish have to pass two barriers equipped with fishways to arrive at the breeding area. Fishways are generally inefficient and can either relax selection or impose selection by favoring certain phenotypes (here represented by red and black) that are more proficient at traversing fishways, gradually reducing phenotypic diversity after each passage. Due to selection and spatial sorting, the breeding population will consist mostly of red and black phenotypes, and as a result of assortative mating the relative frequency of these phenotypes will increase and the phenotypic and genetic diversity in the population will decrease over time (N generations). (B) The increase in relative frequency of passage proficient phenotypes may, at least in theory, improve fishway performance. The loss of standing genetic variation, however, will reduce the adaptive potential, and viability of the population.
Selection on migratory performance may have evolutionary consequences that extend beyond the phenotypic dimensions that directly influence migratory capacity. This is because phenotype sorting on dispersal enhancing traits may result in indirect correlated responses and induce evolutionary transitions in morphological, physiological, behavioral, and reproductive life-history traits that are developmentally, functionally or genetically associated with the traits directly involved in migration and dispersal (Lande and Arnold, 1983; Roff, 1997; Walsh and Lynch, 2012), and that may impair population growth. For example, a study on the effect of body length and arrival timing on reproductive success in wild pink salmon (O. gorbuscha) indicated that these traits are under stabilizing selection (Dickerson et al., 2005). Therefore, if small size enhances the ability to overcome migration obstacles [as in Maynard et al. (2017); Newton et al. (2018)], this might not only impact the evolutionary trajectory for body length but also population productivity. Changes in the severity of migration may thus have consequences similar to fisheries induced evolution (Kuparinen and Merilä, 2007).
Because fishways and other partial barriers can be difficult to find and pass through, migrating fish may be delayed (McLaughlin et al., 2013; Newton et al., 2018). Longer delays may lead to aggregations that promote disease transmission, create predatory hotspots, and leave individuals with less energy available for reproduction (McLaughlin et al., 2013). Tagging studies suggest that low attraction is often a limiting factor (Dodd et al., 2017), partly because fish rely on water flow dynamics as a cue to initiate upstream migration and to find the fishways (Hall, 2018). Mismatches between fishway operation (van Leeuwen et al., 2016) and the evolved migratory timing may have consequences for both individuals (e.g., late arrival, suboptimal conditions for breeding, not finding a partner) and populations (loss of adaptation of migratory timing) (Dickerson et al., 2005). For example, populations may become reproductively isolated by utilizing the same spawning grounds at different times (Quinn et al., 2000). Delays associated with passing of fishways can potentially cause admixture between temporally isolated subpopulations.
As for recommendations, there should be less focus on the number of fish passing, and more focus on maintaining diverse and viable fish populations (Birnie-Gauvin et al., 2018; Silva et al., 2018). Designing optimal fish passage solutions is complicated by the differential demands of different species, life-history stages and phenotypes (Birnie-Gauvin et al., 2018). There is a growing concern that fishways may relax selection or select for phenotypic dimensions, such as certain life-stages or sizes (Haugen et al., 2008; Maynard et al., 2017) or boldness (Cote et al., 2010), and trait value combinations that are different from those that are beneficial in un-manipulated water courses (Newton et al., 2018) (Figure 5). When deciding on the design and operation of fishway passages, it is important to consider that selectivity may apply to each of the approach, entry and passage components, as well as to post-passage behaviors and performances (Silva et al., 2018). The phenotypic and genetic structure of fish populations may be further influenced by selection operating on individuals as they embark on the downstream journey to complete their life-cycle in the lake or sea. We have in mind the risky and often deadly passage through the created impoundments as well as turbines of hydroelectric power-plants (Jepsen et al., 1998; Calles et al., 2010). If the phenotypic trait values that are favored by selection on juveniles during this downstream passage are different from those that are favored in spawning migrating adults during the upstream journey then this will magnify the variance reducing effect (comparable to stabilizing selection), which can detrimentally impact long-term population persistence. Perhaps the key question regarding connectivity restoration is whether the persistence of the dam or migration barrier is at all defendable, and whether it can be removed instead of installing inherently imperfect fishways? With barrier removal comes also the complex issue of how the capacity for re-colonization and range expansion may vary among species depending on migratory behavior and life-history characteristics (Pess et al., 2014), and the possible establishment of invasive migratory species, such as the sea lamprey, that may disrupt local communities (Smith and Tibbles, 1980; McLaughlin et al., 2013).
Captive Breeding, Supplemental Stocking and Aquaculture
The release of captive reared fishes might be seen as a quick and feasible fix for declining wild fish stocks to compensate for overfishing and losses due to dam construction (Hórreo, 2015), but it does not come without problems. Releasing large numbers of captive-bred fishes might expose wild fish populations to elevated competition and predation, and it can do so even if the stocked fish do not reproduce in the wild, as exemplified by escapes of farmed S. salar in Norway (Anonymous, 1999). The escaped farmed fish have low reproductive success (Fleming et al., 1996), and probably do not replace what they destroy neither in numbers nor quality of offspring. An example from the North American west coast further indicates that the consequences of stocked fish may vary according to environmental conditions. Levin et al. (2001) report that the productivity of wild Chinook salmon (O. tshawytscha) was affected by the interaction between ocean conditions and the number of stocked hatchery spring chinooks. Nickelson (2003) reports on a similar negative relationship between hatchery spawners and wild productivity in coho salmon (O. kisutch).
Captive breeding has the advantage over wild reproduction that fewer parental fishes are needed for producing a certain number of juveniles of a certain age. However, captive breeding programs rarely use a sufficient number of breeding individuals, and studies indicate that the genetic variation declines in populations exposed to repeated captive breeding (Hansen et al., 2001; Säisä et al., 2003) thereby reducing their performance and adaptability in the wild (Araki et al., 2007). In addition, released captive reared fish, and escapers from aquaculture cages, may interbreed with wild stocks and result in genetic admixture.
Genetic Admixture
Migration behavior may result in reproductive interactions between fishes from different populations. Mixing of previously separated gene pools, admixture (Lynch, 1991), can occur both between species and between populations within species. Intraspecific admixture may be a natural outcome of dispersal and non-natal adult straying (Keefer and Caudill, 2014). It can also result from anthropogenic activities, including management actions aimed at protecting biodiversity, such as removal of migration barriers, installation of fishways, compensatory breeding, supplementary stocking, and translocations (Gjedrem et al., 1991; Berg et al., 1997; Søndergaard et al., 2000; McClelland and Naish, 2007; Seddon et al., 2007; Frankham, 2008; Service USFW, 2012).
Admixture will increase the genetic diversity in the receiving population, but fitness consequences can vary from positive to negative. By contributing new alleles and enabling creation of novel genotypes and haplotypes, admixture can counteract inbreeding depression, conceal deleterious recessive alleles, and result in heterosis (Lynch, 1991; Fenster and Galloway, 2000; Keller and Waller, 2002; Facon et al., 2005; Drake, 2006; Lavergne and Molofsky, 2007; Weeks et al., 2011). Conversely, the introduction of new genetic material can dilute favorable alleles, break up co-adapted gene complexes (Lynch, 1991; Rhymer and Simberloff, 1996; Fenster and Galloway, 2000; Edmands, 2007; Verhoeven et al., 2011; Whitlock et al., 2013) and reduce fertility and offspring viability (Gilk et al., 2004; Turner et al., 2012; Sunde and Forsman, 2016), thereby impairing population performance (Fleming et al., 2000; McGinnity et al., 2003).
The outcome of admixture affects both the genetic diversity within populations and genetic differentiation between populations, which might have consequences for the viability, and adaptability of the populations and species (McGinnity et al., 2009). From a management perspective it is therefore problematic that the direction and magnitude of responses to admixture can differ between species (Hardiman and Culley, 2010; Molofsky et al., 2014; Rollinson et al., 2014), among populations within species (Escobar et al., 2008; Tortajada et al., 2010; Hufford et al., 2012; Sunde and Forsman, 2016; Tinnert et al., 2016; Shi et al., 2018), and even vary depending on the sex of the immigrants (Sunde et al., 2018b). That the effects of admixture can be sex-specific (Sunde et al., 2018b) might impact on dispersal behavior; if the responses to admixture depend on the sex of the immigrant, it is likely that the impact on spawning migratory behavior may also differ between the sexes. Predicting the outcome of admixture is further complicated by that responses can differ also between generations (Huff et al., 2011; Tinnert et al., 2016) and environments (Lynch, 1991; McClelland and Naish, 2007).
Evolutionary divergence following reproductive isolation can occur in just a few generations (Christie et al., 2012; Thompson et al., 2019), and is thus potentially relevant for recent population sub-divisions. Anadromous fish populations that have been split into reproductively isolated subpopulations due to migration barriers have resulted in the evolution of freshwater forms in landlocked subpopulations (e.g., McDowall, 1997; Littrell et al., 2018). Evolutionary divergence and population genetic structure may also be modified by admixture associated with captive breeding programs, stocking, and escapes of farmed individuals (Christie et al., 2014), and this can affect migratory behavior and evolution of populations that have not previously been much influenced by gene flow.
Admixture is likely more important for species and populations that display spawning migration, compared with resident forms (Keefer and Caudill, 2014) for example owing to non-natal adult straying (Keefer and Caudill, 2014). Spawning migrating species and populations may also be affected more strongly by admixture resulting from management actions. Comparisons of dispersal probability between wild and captive-bred individuals have generated mixed results; some studies report that captive bred individuals are more likely to disperse, while others have found that wild individuals are more dispersive [reviewed in Quinn (1993)]. Jonsson et al. (2003) showed that wild populations have a higher probability of homing and that captive-bred individuals tend to stray more. Studies investigating whether and how migratory behavior is affected by hybridization between different migratory forms are scarce. However, Saint-Pé et al. (2018) investigated genetic structure and spatial patterns of admixture in brown trout (S. trutta) within a small watershed in France, and report that dispersal was admixture-biased. In conclusion, populations can differentiate rapidly, selection can modify migratory behavior, and admixture between different migratory forms can impact on dispersal probability, population differentiation and genetic structure of migratory fish. Besides the immediate relevance for the understanding and management of biodiversity, this has implications for the productivity, functioning and resilience of ecosystems.
Overfishing and Fishing Regulations
Overfishing is a major threat to aquatic biodiversity globally (Pauly et al., 1998; Jackson et al., 2001). In addition to aggregations in spawning habitats, migratory species may be particularly vulnerable to overfishing through aggregations during migration (Allan et al., 2005). This is especially relevant to anadromous and catadromous species that pass through confined waterbodies represented by streams on their way toward the spawning habitats. It is plausible that species, populations and individuals may be differently affected by fisheries harvest depending on migration patterns and fishing regulations regarding timing (Diaz Pauli and Sih, 2017). For instance, it may result in skewed harvesting of migratory phenotypes in comparison to resident phenotypes, and ultimately change population dynamics and evolutionary trajectories. Fisheries may also impose differential mortality due to variation in timing and size of migratory fish. For example, it is a common practice to regulate fisheries with closed seasons (Wilen, 1985), and such actions might render early or late migrants disproportionately vulnerable to fisheries. If timing co-varies with body size (Tibblin et al., 2016b; Jonsson et al., 2017; Morita, 2019), regulations involving closed seasons may also translate into size-selective mortality, with potentially dramatic ecological and evolutionary side effects (Kuparinen and Merilä, 2007). Together, this calls for adaptive fisheries management where variation in migratory behavior is incorporated in management strategies and actions to prevent loss of biodiversity and unique migratory patterns. For example, given the protective variance reducing portfolio effect that population and life history diversity may have in exploited species, such as sockeye salmon, it will be important to minimize the homogenizing effects that hatcheries may have on genetic structure and to protect weak and declining populations from exploitation. This is essential both because it can stabilize productivity of individual species (Schindler et al., 2010), and because there can be a critical threshold for the number of populations below which regional extinction is likely (Hui et al., 2017). Maintaining options and portfolios for organism and their ecosystems is a means of spreading the risk and maintaining productivity, biodiversity and ecosystem functioning in the face of future uncertainties (Schindler et al., 2015; Waldman et al., 2016; Lowerre-Barbieri et al., 2017).
Responses to Changing Water Temperatures, Sea Surface Fluctuations, and Salinity Gradients Associated With Climate Change
Climate change constitutes a major threat to biodiversity in both terrestrial and aquatic environments. Environmental conditions (hydro geography, temperature, precipitation, ice coverage, sea surface levels, acidity, flow regimes, currents, and salinity gradients) are changing rapidly worldwide due to ongoing global warming (Mackenzie et al., 2007; IPCC, 2013, 2018; Cheng et al., 2019). In the wake of climate change, organisms will be exposed not only to increasing averages but also to more variable and extreme conditions (IPCC, 2018), with changes in both the strength and direction of selection over time. While there is little doubt that climate change is happening, it remains unclear how biodiversity and ecosystem services will be affected—particularly in aquatic systems that are less well studied compared with terrestrial systems (see Figure 1 in Forsman et al., 2016a).
Altered water temperatures, sea surface levels, flow regimes, and salinity gradients may modify the opportunities for dispersal and affect connectivity among populations (Figure 1). This too may induce changes in the timing of events, local adaptations, and distribution shifts, potentially with far reaching implications and indirect effects mediated via species interactions, modified community species compositions and altered ecosystem functioning.
Distribution Shifts
Because of limited potential for temperature regulation, body temperatures of fish generally conform closely to surrounding temperatures. Some species of fish [such as tunas (Scombroidei) and sharks (Lamniformes) (Dickson and Graham, 2004), and the opah (Lampris guttatus) (Wegner et al., 2015)] can evade the temperature boundaries of ambient water by generating and conserving metabolic heat internally, but this capacity is restricted to about 0.1% of the known fish species (Dickson and Graham, 2004). Most fish instead rely on external heat from the environment and on behavioral thermoregulation, including both larger scale migrations between colder and warmer environments and smaller scale vertical movements involved in sun basking and when fish take advantage of temperature differences among strata in stratified lakes and oceans, to regulate their internal temperature (May, 1979; Reynolds and Casterlin, 1980; Hertz et al., 1993; Gillooly et al., 2002; Mehner, 2012; Ma et al., 2018; Nordahl et al., 2018, 2019).
Mobile organisms (including fishes) may respond to temporal environmental changes (or altered demands) by dispersing to habitats with more suitable conditions, which might ultimately result in range expansions, distribution shifts (Parmesan and Yohe, 2003; Root et al., 2003; Cooke et al., 2004; Perry et al., 2005; Forsman et al., 2016b) and spatiotemporal modifications of migration routes (Crozier and Hutchings, 2014). That climate change is driving poleward distribution shifts in marine fish species that attempt to escape warm waters and enables fishes that cannot tolerate too cold water to colonize new regions complicates management, governance, and international fishing regulations. For example, recent modeling results suggest that the system for allocating fish stocks is being outpaced by the movement of fish species in response to climate change (Pinsky et al., 2018).
Phenology Shifts
In fish, changes in the timing of adult migration and reproduction, age at maturity and in age at juvenile migration seem to be common responses to temperature shifts (Crozier and Hutchings, 2014). Cooke et al. (2004) report that the timing of peak upriver spawning migration of sockeye salmon in the Fraser River shifted forward more than 6 weeks from 1995 through 2002, and that the earlier migration was associated with higher pre-spawning mortality. Such temporal shifts in the onset of spawning migration in salmonids are typically interpreted as responses to climate change. However, it has also been suggested that it might instead reflect a fisheries-induced evolutionary response because late-spawning brood lines are being fished for longer time periods (Morita, 2019). Predictions regarding future changes of migration timing in the face of global warming are further complicated by the heterogeneity in long-term shifts in migration timing seen across species and populations of Pacific salmon, with some postponing and others migrating earlier (Kovach et al., 2015). Environmental challenges in the form of warmer waters and altered flow velocities associated with climate change may also directly influence locomotor performance and the costs of migration, moderate energetic trade-offs, and limit the amount of resources available for other facets of the reproductive cycle (Fenkes et al., 2016).
An investigation of an anadromous pike population in the Baltic Sea shows that the timing of arrival to the spawning area may vary among years by as much as 3 weeks. Despite this year-to-year flexibility, the relative timing of spawning migration differed considerably and in a consistent manner among individuals (Tibblin et al., 2016b). Whether this variation has a genetic component remains unknown, but estimates of repeatability point to an upper bound of heritability of about 0.25 (Tibblin et al., 2016b), indicating that evolutionary responses to selection on timing of spawning in pike are possible. That the timing of spawning migration in pike is highly flexible, with individuals fine-tuning migratory timing between years (Tibblin et al., 2016b), indicates that temporal behavioral adjustments are used to ensure that embryos and larvae develop when temperature conditions are favorable. Such phenotypic flexibility may buffer populations against rapid unpredictable environmental changes and potentially prevent the loss of genetic diversity (Wennersten and Forsman, 2012; Forsman, 2015).
Adaptations of Migratory Fish to Changing Conditions
Migrating fishes cross habitat borders and move along environmental gradients (Figure 3). Individuals are exposed to environmental changes also if they remain for prolonged periods within a given habitat or limited area. Depending on the spatial and temporal scales of the environmental changes relative to the dispersal capacity, generation time and reproductive mode of the organisms, this may maintain a diversity of specialists within populations, or promote the evolution of generalist strategies that perform reasonably well across a range of environments (Levins, 1968; Kassen, 2002; Forsman et al., 2011). Generalist strategies include plastic or flexible phenotypes that adjust to conditions via developmental modifications or reversible intra-individual physiological or behavioral modifications (Pigliucci, 2001; West-Eberhard, 2003; Forsman, 2015; Tibblin et al., 2016b).
With regards to early life-history traits, it has been demonstrated in some species of spawning migrating fish that populations with a history of exposure to different thermal conditions during incubation of eggs and embryos display local adaptations and respond differently to temperature manipulations in the laboratory. Examples include cold water specialist salmonids such as brown trout (Salmo trutta) (Jensen et al., 2008) and grayling (Thymallus thymallus) (Kavanagh et al., 2010; Thomassen et al., 2011; Mäkinen et al., 2016), and temperate latitude species such as pike (Sunde et al., in press). Given that early and late spawning phenotypes coexist within populations (Tibblin et al., 2016b), and that the offspring produced by early arrivers likely develop in lower temperatures, it can be hypothesized that correlational selection (Arnold and Wade, 1984; Forsman and Appelqvist, 1998) on the combination of spawning timing and temperature tolerance has favored the evolution of genetic covariance and phenotypic integration between these behavioral and physiological traits. This hypothesis could be evaluated by comparing temperature related performance of eggs and embryos produced by gametes collected from adults arriving at the beginning and toward the end of the spawning period. Temperature related adaptations may potentially reinforce reproductive isolation by time (Hendry and Day, 2005), and contribute to further population structuring.
Spawning migrating fish not only have to cope with changing water temperatures. Global warming also brings modifications in sea surface levels and salinity regimes that may be particularly challenging for some anadromous species. Depending on the altitude of spawning areas proximate to the sea, sea surface fluctuations may result in that saline or brackish water temporarily enters the freshwater areas used for spawning and early life-history stages (Sunde et al., 2018a). A recent study suggests that different anadromous populations of pike in the Baltic Sea vary in their ability to cope with fluctuating salinity levels, and that the effects of salinity differed among families within populations, consistent with the notion that intra-population genetic variation for developmental plasticity offers buffering capacity and adaptive potential (Sunde et al., 2018a). Changing conditions in the areas used for reproduction can also affect population size and density, with consequences for intensity of competition, cannibalism, sexual selection, and for population genetic diversity and structure that together may influence the relative success of alternative migration strategies.
Future Directions
Scientific output on variation in fish migration has increased tremendously over the past 50 years (Figure 2). This review highlights patterns, causes, and consequences of variation and flexibility of migration behaviors that are of relevance for the understanding, protection, and sustainable utilization of migratory fishes and of their ecosystems (Figure 1). Despite extensive previous research (Figure 2), important knowledge gaps and unanswered questions remain that require future investigations. We briefly summarize these points and future directions below:
Research on fish migration has traditionally focused on a few species of high socio-economic importance, primarily salmonids. In most species and populations, the relative contributions of genetic and environmentally induced variation in migratory behavior remain unresolved. Knowledge of how internal attributes, social interactions, and environmental factors (Table 1; Figure 1) influence variation in migratory behavior, timing and distance among species, populations and individuals of fish (including many salmonids) is incomplete. However, recent technological developments in bio-logging have advanced the ability to obtain high-resolution data on fish movements, inform about internal and external drivers of movements, help illuminate the consequences of movements for individual performance and population fitness, and provide answers to the questions how, where, when, and why fish migrate (Nathan et al., 2008; Cooke et al., 2013; Wilmers et al., 2015; Nordahl et al., 2018, 2019; Lowerre-Barbieri et al., 2019). This may improve our understanding of diversity, and allow for more reliable predictions of the consequences that exploitation, management actions and climate change may have for migratory fish.
New insights into the causes, consequences, and evolutionary dynamics of migratory behavior in fishes might be gained by phylogeny based comparative approaches (Felsenstein, 1985; Bloom et al., 2018). Besides uncovering the distribution of data deficiency and identifying taxa and geographic regions in particular need of further investigation, phylogenetic comparisons can inform why certain species migrate whereas others do so to a lesser degree, and uncover associations of evolutionary shifts in migration behaviors with environmental factors and with morphological, physiological, or behavioral phenotypic dimensions (e.g., McDowall, 1997; Watanabe et al., 2015; Forsman and Berggren, 2017; Bloom et al., 2018).
Another potentially rewarding line of future research is to focus on variation among populations. Information on migration behaviors for multiple populations and species compiled using systematic reviews and meta-analytical approaches (Gurevitch et al., 2018) can illuminate patterns and evaluate potential drivers of variation in migration mode, migration timing, and migration distance among populations. Besides summarizing information and advancing knowledge, results can inform when the evidence is sufficient for adaptive population specific management and conservation efforts.
The timing of spawning migration varies considerably both among and within fish populations. Whether isolation by time (Hendry and Day, 2005) is a common driver of reproductive isolation, genetic divergence and adaptation in fish, and whether differences in the timing of spawning migration contribute more or less to population structure in different species depending on their life-history remains to be investigated.
Several studies have aimed at identifying phenotypic correlates of variation in migratory behavior and performance. However, we need to know more about how alterations in migratory challenges brought about by exploitation, management and climate change modifies the intensity and direction of selection and evolutionary shifts in dispersal related physiological, morphological, behavioral traits, and correlated life-history attributes.
Improved sequencing technologies have enabled genomic resources to be generated with increasing efficiency and speed, such that non-mainstream fish species can now be utilized as models. The NCBI (2019) database (http://www.ncbi.nlm.nih.gov/genome/browse/ - last accessed 18 February 2019) currently includes genome sequence assemblies for 258 fish species. Recent developments in genomic tools [e.g., RAD-sequencing, WGS, GWAS and SNP-genotyping (Andrews et al., 2016)] together with information for closely related species and populations in different environments may help identify genes and underlying genomic regions under selection involved in shaping the diversity of migratory behavior. There is also potential for such approaches to clarify the contributions of stochastic processes, gene flow, selection, and plasticity and to pinpoint the role of specific genes in shaping genetic structure and phenotypic evolution in migratory fishes (e.g., Barson et al., 2015; Momigliano et al., 2017; Thompson et al., 2019).
Changes in river connectivity alters selection pressures and may have implications for species interactions, community composition and ecosystem functioning. To evaluate the consequences that selection associated with fishway passages may have for spawning migrating fish requires a combination of methodological approaches, such as analysis of otolith elemental chemistry, collection of high-resolution spatiotemporal movement data using mark-recapture studies, telemetry, and passive integrative transponders, behavioral monitoring using fishways equipped with submerged cameras, as well as longitudinal and cross sectional comparisons of phenotype distributions (Nathan et al., 2008). When evaluating the design and operation of fishway passages, it is important to consider that selection may operate during each of the approach, entry, and passage components, as well as on post-passage behaviors and performances; it is the cumulative effect that may modify evolution of behavior, reproduction, population genetics and population dynamics. As the number of studies grows, the opportunities for systematic reviews and meta-analytical approaches to provide new insights will increase.
The directions and rates of genetic exchange between populations may change over time owing to natural processes, anthropogenic environmental makeovers and management actions. The consequences of genetic admixture can vary from positive to negative, affect genetic differentiation and diversity between and within populations, and ultimately influence viability and adaptability of populations and species (McClelland and Naish, 2007; McGinnity et al., 2009). An important question to consider is whether and how migratory strategies employed by the populations subjected to management might affect the outcome of admixture. Conversely, few (if any) studies have examined whether and how genetic admixture affects migratory behavior. It is therefore necessary to investigate the reciprocal feedback loop between migration and admixture, and how it may influence dynamics, genetic structuring, and viability of populations. Further, admixture effects can be sex-specific (Sunde et al., 2018b), and it might be hypothesized that this should impact on spawning migratory behavior. To our knowledge, however, it has not yet been examined whether sex-biased dispersal or migration is associated with sex-specific responses to admixture.
Water temperature influences migratory behavior and performance of fishes. Recent studies show that fish can reap thermoregulatory rewards and elevate their body temperature above that of ambient water by sun basking near the water surface (Nordahl et al., 2018, 2019). There is little doubt that the capacity for aquatic thermoregulation by sun basking is important for fish. However, more work is needed in this emerging area to clarify the consequences of sun basking for fish performance, migratory behavior, spatiotemporal distribution shifts, and whether and how it will modify predictions regarding responses to climate change.
To understand the effects that climate change may have on migratory fish, future research needs to expand beyond considering effects of the projected increase in average water temperatures. There is a need for better knowledge of how more extreme and fluctuating water temperatures and sea levels may affect the development of early life-history stages or the growth and survival of adult fish, and whether this influences the relative success of spawning migrating forms.
Lastly, we offer a cautionary note regarding management. It remains uncertain how human activities and climate change will influence environmental conditions and selective regimes for fish. A particular challenge when addressing consequences of climate change is to find the balance between realism and methodological tractability (Forsman et al., 2016a), and this applies also to the evaluation of management actions. It is difficult to foresee which genetic makeups, phenotypic trait value combinations, and behaviors that will be most successful in the future. To safeguard against this uncertainty, management actions should be designed to maintain genetic and phenotypic diversity with regards to migratory behavior, seasonal timing of reproduction, place of spawning, growth trajectories, size and age at maturity, and reproductive allocation strategies both among and within populations and species. Theory and empirical evidence concur that this may promote establishment success, reduce extinction risk, enable populations and species to cope with environmental change and adapt to novel conditions, and increase productivity, functioning and resilience of ecosystems (Hughes et al., 2008; Schindler et al., 2010, 2015; Bolnick et al., 2011; Wennersten and Forsman, 2012; Forsman, 2014, 2015; Forsman and Wennersten, 2016; Waldman et al., 2016; Lowerre-Barbieri et al., 2017). Perhaps future research should aim to develop a ‘best practice’ regarding adaptive management and how to safeguard against uncertainty; there might be good solutions waiting to be discovered.
Author Contributions
The first outline of the review was conceived of by AF. AF coordinated the writing, and revisions. All authors contributed to developing the idea and to the writing of separate sections of the first draft and provided feedback on earlier drafts and approved the final version for submission.
Funding
Funding was provided by the Swedish Research Council Formas (Dnr. 2017-00346 to AF, EP, and PT; Dnr. 2018-00605 to PT), Stiftelsen Oscar och Lili Lamms Minne (DO 2017-0050) to AF, EP, and PT, and by Linnaeus University (AF, PT).
Conflict of Interest Statement
The authors declare that the research was conducted in the absence of any commercial or financial relationships that could be construed as a potential conflict of interest.
Acknowledgments
We thank Brett Sandercock and Nathan Senner for the invitation to write this review. We would like to thank Oscar Nordahl and two reviewers for comments on an earlier version of the manuscript.
References
Aarts, B. G. W., Van Den Brink, F. W. B., and Nienhuis, P. H. (2004). Habitat loss as the main cause of the slow recovery of fish faunas of regulated large rivers in Europe: the transversal floodplain gradient. River Res. Appl. 20, 3–23. doi: 10.1002/rra.720
Aguirre, W. E., Young, A. G., Cucalón, R. V., Borders, T., Navarrete-Amaya, R., Valdiviezo-Rivera, J., et al. (2019). Vertebral number covaries with body form and elevation along the western slopes of the Ecuadorian Andes in the Neotropical fish genus Rhoadsia (Teleostei: Characidae). Biol. J. Linn. Soc. 126, 706–720. doi: 10.1093/biolinnean/blz002
Allan, J. D., Abell, R., Hogan, Z., Revenga, C., Taylor, B. W., Welcomme, R. L., et al. (2005). Overfishing of inland waters. Bioscience 55, 1041–1051. doi: 10.1641/0006-3568(2005)055[1041:OOIW]2.0.CO;2
Andrews, K. R., Good, J. M., Miller, M. R., Luikart, G., and Hohenlohe, P. A. (2016). Harnessing the power of RADseq for ecological and evolutionary genomics. Nat. Rev. Gen. 17, 81–92. doi: 10.1038/nrg.2015.28
Anonymous (1999). Till laks åt alle kan ingen gjere?—Om årsaker til nedgangen i de norske villaksbestandene og forslag til strategier og tiltak å bedre situasjonen (Salmon for everyone can not be done?—The causes for the decrease of wild salmon stocks in Norway and strategies and action for improving the situation). NOU 1999:9.
Aoyama, J. (2009). Life history and evolution of migration in catadromous eels (genus Anguilla). Aqua-BioSci. Monographs. 2, 1–42. doi: 10.5047/absm.2009.00201.0001
Araki, H., Cooper, B., and Blouin, M. S. (2007). Genetic effects of captive breeding cause a rapid, cumulative titness decline in the wild. Science 318, 100–103. doi: 10.1126/science.1145621
Armstrong, J. B., Schindler, D. E., Ruff, C. P., Brooks, G. T., Bentley, K. E., and Torgersen, C. E. (2013). Diel horizontal migration in streams: juvenile fish exploit spatial heterogeneity in thermal and trophic resources. Ecology 94, 2066–2075. doi: 10.1890/12-1200.1
Arnaud, C. M., Becker, P. H., Dobson, F. S., and Charmantier, A. (2013). Canalization of phenology in common terns: genetic and phenotypic variations in spring arrival date. Behav. Ecol. 24, 683–690. doi: 10.1093/beheco/ars214
Arnold, S. J., and Wade, M. J. (1984). On the measurement of natural and sexual selection: theory. Evolution 38, 709–719. doi: 10.1111/j.1558-5646.1984.tb00344.x
Barson, N. J., Aykanat, T., Hindar, K., Baranski, M., Bolstad, G. H., Fiske, P., et al. (2015). Sex-dependent dominance at a single locus maintains variation in age at maturity in salmon. Nature 528, 405–408. doi: 10.1038/nature16062
Barthem, R. B., Goulding, M., Leite, R. G., Cañas, C., Forsberg, B., Venticinque, E., et al. (2017). Goliath catfish spawning in the far western Amazon confirmed by the distribution of mature adults, drifting larvae and migrating juveniles. Sci. Rep. 7:41784. doi: 10.1038/srep41784
Beacham, T. D. (1983). Variability in median size and age at sexual maturity of Atlantic cod, Gadus morhua, on the Scotian shelf in the Northwest Atlantic Ocean. Fish Bull. 81, 303–321.
Berdahl, A., Westley, P. A. H., and Quinn, T. P. (2017). Social interactions shape the timing of spawning migrations in an anadromous fish. Anim. Behav. 126, 221–229. doi: 10.1016/j.anbehav.2017.01.020
Berg, S., Jeppesen, E., and Søndergaard, M. (1997). Pike (Esox lucius L.) stocking as a biomanipulation tool 1. Effects on the fish population in Lake Lyng, Denmark. Hydrobiologia 342, 311–318. doi: 10.1007/978-94-011-5648-6_32
Berggren, H., Nordahl, O., Tibblin, P., Larsson, P., and Forsman, A. (2016). Testing for local adaptation to spawning habitat in sympatric subpopulations of pike by reciprocal translocation of embryos. PLoS ONE 11:e0154488. doi: 10.1371/journal.pone.0154488
Birnie-Gauvin, K., Franklin, P., Wilkes, M., and Aarestrup, K. (2018). Moving beyond fitting fish into equations: progressing the fish passage debate in the Anthropocene. Aquat. Cons. Mar. Freshw. Ecosyst. 2018, 1–11. doi: 10.1002/aqc.2946
Bloom, D. D., Burns, M. D., and Schriever, T. A. (2018). Evolution of body size and trophic position in migratory fishes: a phylogenetic comparative analysis of Clupeiformes (anchovies, herring, shad and allies). Biol. J. Linn. Soc. 125, 302–314. doi: 10.1093/biolinnean/bly106
Bloom, D. D., and Lovejoy, N. R. (2014). The evolutionary origins of diadromy inferred from a time-calibrated phylogeny for Clupeiformes (herring and allies). Proc. Biol. Sci. 281:20132081. doi: 10.1098/rspb.2013.2081
Bolnick, D. I., Amarasekare, P., Araújo, M. S., Bürger, R., Levine, J. M., Novak, M., et al. (2011). Why intraspecific trait variation matters in community ecology. Trends Ecol. Evol. 26, 183–192. doi: 10.1016/j.tree.2011.01.009
Branco, P., Amaral, S. D., Ferreira, M. T., and Santos, J. M. (2017). Do small barriers affect the movement of freshwater fish by increasing residency? Sci. Tot. Envir. 581–582, 486–494. doi: 10.1016/j.scitotenv.2016.12.156
Brodersen, J., Bronmark, C., Skov, C., Nilsson, P. A., and Hansson, L.-A. (2007). “Seasonal migration in cyprinid fish: causes and consequences,” in Ecological Society of America Annual Meeting Abstracts (San Jose, CA).
Brodersen, J., Chapman, B. B., Nilsson, P. A., Skov, C., Hansson, L.-A., and Brönmark, C. (2014). Fixed and flexible: coexistence of obligate and facultative migratory strategies in a freshwater fish. PLoS ONE 9:e90294. doi: 10.1371/journal.pone.0090294
Brodersen, J., Howeth, J. G., and Post, D. M. (2015). Emergence of a novel prey life history promotes contemporary sympatric diversification in a top predator. Nat. Commun. 6:8115. doi: 10.1038/ncomms9115
Brönmark, C., Hulthén, K., Nilsson, P. A., Skov, C., Hansson, L. A., Brodersen, J., et al. (2014). There and back again: migration in freshwater fishes. Can. J. Zool. 92, 467–479. doi: 10.1139/cjz-2012-0277
Brown, J. J., Limburg, K. E., Waldman, J. R., Stephenson, K., Glenn, E. P., Juanes, F., et al. (2013). Fish and hydropower on the U.S. Atlantic coast: failed fisheries policies from half-way technologies. Cons. Lett. 6, 280–286. doi: 10.1111/conl.12000
Calles, O., Olsson, I. C., Comoglio, C., Kemp, P. S., Blunden, L., Schmitz, M., et al. (2010). APPLIED ISSUES: size-dependent mortality of migratory silver eels at a hydropower plant, and implications for escapement to the sea. Freesw. Biol. 55, 2167–2180. doi: 10.1111/j.1365-2427.2010.02459.x
Carim, K. J., Eby, L. A., Barfoot, C. A., and Boyer, M. C. (2016). Consistent loss of genetic diversity in isolated cutthroat trout populations independent of habitat size and quality. Cons. Gen. 17, 1363–1376. doi: 10.1007/s10592-016-0867-9
Chapman, B. B., Brönmark, C., Nilsson, J.-Å., and Hansson, L.-A. (2011a). The ecology and evolution of partial migration. Oikos 120, 1764–1775. doi: 10.1111/j.1600-0706.2011.20131.x
Chapman, B. B., Hulthen, K., Blomqvist, D. R., Hansson, L.-A., Nilsson, J.-A., Brodersen, J., et al. (2011b). To boldly go: individual differences in boldness influence migratory tendency. Ecol. Lett. 14, 871–876. doi: 10.1111/j.1461-0248.2011.01648.x
Chapman, B. B., Hulthen, K., Bronmark, C., Nilsson, P. A., Skov, C., Hansson, L. A., et al. (2015). Shape up or ship out: migratory behaviour predicts morphology across spatial scale in a freshwater fish. J. Anim. Ecol. 84, 1187–1193. doi: 10.1111/1365-2656.12374
Cheng, L., Abraham, J., Hausfather, Z., and Trenberth, K. E. (2019). How fast are the oceans warming? Science 363, 128–129. doi: 10.1126/science.aav7619
Christie, M. R., Ford, M. J., and Blouin, M. S. (2014). On the reproductive success of early-generation hatchery fish in the wild. Evol. Appl. 7, 883–896. doi: 10.1111/eva.12183
Christie, M. R., Marine, M. L., French, R. A., and Blouin, M. S. (2012). Genetic adaptation to captivity can occur in a single generation. Proc. Natl. Acad. Sci. U.S.A. 109, 238–242. doi: 10.1073/pnas.1111073109
Comte, L., and Grenouillet, G. (2013). Do stream fish track climate change? Assessing distribution shifts in recent decades. Ecography 36, 1236–1246. doi: 10.1111/j.1600-0587.2013.00282.x
Cooke, S. J., Hinch, S. G., Farrell, A. P., Lapointe, M. F., Jones, S. R. M., Macdonald, J. S., et al. (2004). Abnormal migration timing and high en route mortality of Sockeye salmon in the Fraser River, British Columbia. Fisheries 29, 22–33. doi: 10.1577/1548-8446(2004)29[22:AMTAHE]2.0.CO;2
Cooke, S. J., Midwood, J. D., Thiem, J. D., Klimley, P., Lucas, M. C., Thorstad, E. B., et al. (2013). Tracking animals in freshwater with electronic tags: past, present and future. Anim. Biotel. 1:5. doi: 10.1186/2050-3385-1-5
Cote, J., Clobert, J., Brodin, T., Fogarty, S., and Sih, A. (2010). Personality-dependent dispersal : characterization, ontogeny and consequences for spatially structured populations. Phil. Trans. R. Soc. B. 365, 4065–4076. doi: 10.1098/rstb.2010.0176
Crossin, G. T., Hinch, S. G., Farrell, A. P., Higgs, D. A., Lotto, A. G., Oakes, J. D., et al. (2004). Energetics and morphology of sockeye salmon: effects of upriver migratory distance and elevation. J. Fish Biol. 65, 788–810. doi: 10.1111/j.0022-1112.2004.00486.x
Crozier, L. G., and Hutchings, J. A. (2014). Plastic and evolutionary responses to climate change in fish. Evol. Appl. 7, 68–87. doi: 10.1111/eva.12135
Des Roches, S., Post, D. M., Turley, N. E., Bailey, J. K., Hendry, A. P., Kinnison, M. T., et al. (2018). The ecological importance of intraspecific variation. Nat. Ecol. Evol. 2, 57–64. doi: 10.1038/s41559-017-0402-5
Diaz Pauli, B., and Sih, A. (2017). Behavioural responses to human-induced change: why fishing should not be ignored. Evol. Appl. 10, 231–240. doi: 10.1111/eva.12456
Dickerson, B. R., Brinck, K. W., Willson, M. F., Bentzen, P., and Quinn, T. P. (2005). Relative importance of salmon body size and arrival time at breeding grounds to reproductive success. Ecology 86, 347–352. doi: 10.1890/03-625
Dickson, K. A., and Graham, J. B. (2004). Evolution and consequences of endothermy in fishes. Phys. Biochem. Zool. 77, 998–1018. doi: 10.1086/423743
Dingemanse, N. J., Kazem, A. J. N., Réale, D., and Wright, J. (2010). Behavioural reaction norms: animal personality meets individual plasticity. Trends Ecol. Evol. 25, 81–89. doi: 10.1016/j.tree.2009.07.013
Dodd, J. R., Cowx, I. G., and Bolland, J. D. (2017). Efficiency of a nature-like bypass channel for restoring longitudinal connectivity for a river-resident population of brown trout. J. Environ. Manage. 204(Pt 1), 318–326. doi: 10.1016/j.jenvman.2017.09.004
Dodson, J. J., Aubin-Horth, N., Theriault, V., and Paez, D. J. (2013). The evolutionary ecology of alternative migratory tactics in salmonid fishes. Biol. Rev. 88, 602–625. doi: 10.1111/brv.12019
Donadi, S., Austin, Å. N., Bergström, U., Eriksson, B. K., Hansen, J. P., Jacobson, P., et al. (2017). A cross-scale trophic cascade from large predatory fish to algae in coastal ecosystems. Proc. R. Soc. B. 284:20170045. doi: 10.1098/rspb.2017.0045
Drake, J. M. (2006). Heterosis, the catapult effect and establishment success of a colonizing bird. Biol. Lett. 2, 304–307. doi: 10.1098/rsbl.2006.0459
Dudgeon, D., Arthington, A. H., Gessner, M. O., Kawabata, Z.-I., Knowler, D. J., Lévêque, C., et al. (2006). Freshwater biodiversity: importance, threats, status and conservation challenges. Biol. Rev. 81, 163–182. doi: 10.1017/S1464793105006950
Edmands, S. (2007). Between a rock and a hard place: evaluating the relative risks of inbreeding and outbreeding for conservation and management. Mol. Ecol. 16, 463–475. doi: 10.1111/j.1365-294X.2006.03148.x
Engstedt, O., Stenroth, P., Larsson, P., Ljunggren, L., and Elfman, M. (2010). Assessment of natal origin of pike (Esox lucius) in the Baltic Sea using Sr:Ca in otoliths. Env. Biol. Fish 89, 547–555. doi: 10.1007/s10641-010-9686-x
Escobar, J. S., Nicot, A., and David, P. (2008). The different sources of variation in inbreeding depression, heterosis and outbreeding depression in a metapopulation of Physa acuta. Genetics 180, 1593–1608. doi: 10.1534/genetics.108.092718
Facon, B., Jarne, P., Pointier, J. P., and David, P. (2005). Hybridization and invasiveness in the freshwater snail Melanoides tuberculata: hybrid vigour is more important than increase in genetic variance. J. Evol. Biol. 18, 524–535. doi: 10.1111/j.1420-9101.2005.00887.x
Felsenstein, J. (1985). Phylogenies and the comparative method. Am. Nat. 125, 1–15. doi: 10.1086/284325
Fenkes, M., Shiels, H. A., Fitzpatrick, J. L., and Nudds, R. L. (2016). The potential impacts of migratory difficulty, including warmer waters and altered flow conditions, on the reproductive success of salmonid fishes. Comp. Biochem. Physiol. A Mol. Integr. Physiol. 193, 11–21. doi: 10.1016/j.cbpa.2015.11.012
Fenster, C. B., and Galloway, L. F. (2000). Population differentiation in an annual legume: genetic architecture. Evolution 54, 1157–1172. doi: 10.1111/j.0014-3820.2000.tb00551.x
Fleming, I. A. (1996). Reproductive strategies of Atlantic salmon: ecology and evolution. Rev. Fish Biol. Fisher. 6, 379–416. doi: 10.1007/BF00164323
Fleming, I. A., Hindar, K., Mjølnerød, I. B., Jonsson, B., Balstad, T., and Lamberg, A. (2000). Lifetime success and interactions of farm salmon invading a native population. Proc. R. Soc. B. 267, 1517–1523. doi: 10.1098/rspb.2000.1173
Fleming, I. A., Jonsson, B., Gross, M. R., and Lamberg, A. (1996). An experimental study of the reproductive behaviour and success of farmed and wild Atlantic salmon (Salmo salar). J. Appl. Ecol. 33, 893–905. doi: 10.2307/2404960
Forseth, T., Barlaup, B. T., Finstad, B., Fiske, P., Gjøsæter, H., Falkegård, M., et al. (2017). The major threats to Atlantic salmon in Norway. ICES J. Mar. Sci. 74, 1496–1513. doi: 10.1093/icesjms/fsx020
Forsman, A. (2014). Effects of genotypic and phenotypic variation on establishment are important for conservation, invasion and infection biology. Proc. Natl. Acad. Sci. U.S.A. 111, 302–307. doi: 10.1073/pnas.1317745111
Forsman, A. (2015). Rethinking phenotypic plasticity and its consequences for individuals, populations and species. Heredity 115, 276–284. doi: 10.1038/hdy.2014.92
Forsman, A., Ahnesjö, J., and Caesar, S. (2007). Fitness benefits of diverse offspring in pygmy grasshoppers. Evol. Ecol. Res. 9, 1305–1318.
Forsman, A., and Appelqvist, S. (1998). Visual predators impose correlational selection on prey color pattern and behavior. Behav. Ecol. 9, 409–413. doi: 10.1093/beheco/9.4.409
Forsman, A., and Berggren, H. (2017). Can spatial sorting associated with spawning migration explain evolution of body size and vertebral number in Anguilla eels? Ecol. Evol. 7, 751–761. doi: 10.1002/ece3.2671
Forsman, A., Berggren, H., Åström, M., and Larsson, P. (2016a). To what extent can existing research help project climate change impacts on biodiversity in aquatic environments? A review of methodological approaches. J. Mar. Sci. Engin. 4:75. doi: 10.3390/jmse4040075
Forsman, A., Betzholtz, P. E., and Franzén, M. (2016b). Faster poleward range shifts in moths with more variable colour patterns. Sci. Rep. 6:36265. doi: 10.1038/srep36265
Forsman, A., Karlsson, M., Wennersten, L., Johansson, J., and Karpestam, E. (2011). Rapid evolution of fire melanism in replicated populations of pygmy grasshoppers. Evolution 65, 2530–2540. doi: 10.1111/j.1558-5646.2011.01324.x
Forsman, A., Tibblin, P., Berggren, H., Nordahl, O., Koch-Schmidt, P., and Larson, P. (2015). Pike Esox lucius as an emerging model organism for studies in ecology and evolutionary biology: a review. J. Fish Biol. 87, 472–479. doi: 10.1111/jfb.12712
Forsman, A., and Wennersten, L. (2016). Inter-individual variation promotes ecological success of populations and species: evidence from experimental and comparative studies. Ecography 39, 630–648. doi: 10.1111/ecog.01357
Forsythe, P. S., Crossman, J. A., Bello, N. M., Baker, E. A., and Scribner, K. T. (2012a). Individual-based analyses reveal high repeatability in timing and location of reproduction in lake sturgeon (Acipenser fulvescens). Can. J. Fish. Aquat. Sci. 69, 60–72. doi: 10.1139/f2011-132
Forsythe, P. S., Scribner, K. T., Crossman, J. A., Ragavendran, A., Baker, E. A., Davis, C., et al. (2012b). Environmental and lunar cues are predictive of the timing of river entry and spawning-site arrival in lake sturgeon Acipenser fulvescens. J. Fish Biol. 81, 35–53. doi: 10.1111/j.1095-8649.2012.03308.x
Foulds, W. L., and Lucas, M. C. (2013). Extreme inefficiency of two conventional, technical fishways used by European river lamprey (Lampetra fluviatilis). Ecol. Engin. 58, 423–433. doi: 10.1016/j.ecoleng.2013.06.038
Frankham, R. (2008). Genetic adaptation to captivity in species conservation programs. Mol. Ecol. 17, 325–333. doi: 10.1111/j.1365-294X.2007.03399.x
Geist, J. (2011). Integrative freshwater ecology and biodiversity conservation. Ecol. Indic. 11, 1507–1516. doi: 10.1016/j.ecolind.2011.04.002
Gilk, S. E., Wang, I. A., Hoover, C. L., Smoker, W. W., Taylor, S. G., Gray, A. K., et al. (2004). Outbreeding depression in hybrids between spatially separated pink salmon, Oncorhynchus gorbuscha, populations: marine survival, homing ability, and variability in family size. Environ. Biol. Fish 69, 287–297. doi: 10.1023/B:EBFI.0000022888.28218.c1
Gillooly, J. F., Charnov, E. L., West, G. B., Savage, V. M., and Brown, J. H. (2002). Effects of size and temperature on developmental time. Nature 417, 70–73. doi: 10.1038/417070a
Gjedrem, T., Gjøen, H. M., and Gjerde, B. (1991). Genetic origin of Norwegian farmed Atlantic salmon. Aquaculture 98, 41–50. doi: 10.1016/0044-8486(91)90369-I
Gouskov, A., Reyes, M., Wirthner-Bitterlin, L., and Vorburger, C. (2016). Fish population genetic structure shaped by hydroelectric power plants in the upper Rhine catchment. Evol. Appl. 9, 394–408. doi: 10.1111/eva.12339
Griffiths, D. (2006). Pattern and process in the ecological biogeography of European freshwater fish. J. Anim. Ecol. 75, 734–751. doi: 10.1111/j.1365-2656.2006.01094.x
Griffiths, D. (2010). Pattern and process in the distribution of North American freshwater fish. Biol. J. Linn. Soc. 100, 46–61. doi: 10.1111/j.1095-8312.2010.01404.x
Grill, G., Lehner, B., Lumsdon, A. E., MacDonald, G. K., Zarfl, C., and Reidy Liermann, C. (2015). An index-based framework for assessing patterns and trends in river fragmentation and flow regulation by global dams at multiple scales. Environ. Res. Lett. 10:015001. doi: 10.1088/1748-9326/10/1/015001
Gross, M. R., Coleman, R. M., and McDowall, R. M. (1988). Aquatic productivity and the evolution of diadromous fish migration. Science 239, 1291–1293. doi: 10.1126/science.239.4845.1291
Gurevitch, J., Koricheva, J., Nakagawa, S., and Stewart, G. (2018). Meta-analysis and the science of research synthesis. Nature 555:175. doi: 10.1038/nature25753
Hall, M. (2018). “To pass or not to pass, a question of shape – a migratory study of brown trout (Salmo trutta) and the possible sorting effects of a fishway,” in Independent Project Course, Master in Science, Second Level, 15 ECTS, Linnaeus University, Faculty of Health and Life Sciences, Department of Biology and Environmental Science (Kalmar).
Hansen, M. M., Ruzzante, D. E., Nielsen, E. E., and Mensberg, K.-L. D. (2001). Brown trout (Salmo trutta) stocking impact assessment using microsatellite DNA markers. Ecol. Appl. 11, 148–160. doi: 10.1890/1051-0761(2001)011[0148:BTSTSI]2.0.CO;2
Hardiman, N. A., and Culley, T. M. (2010). Reproductive success of cultivated Pyrus calleryana (rosaceae) and establishment ability of invasive, hybrid progeny. Am. J. Bot. 97, 1698–1706. doi: 10.3732/ajb.1000113
Harris, J. H., Kingsford, R. T., Peirson, W., and Baumgartner, L. J. (2016). Mitigating the effects of barriers to freshwater fish migrations : the Australian experience. Mar. Freshw. Res. 68, 614–628. doi: 10.1071/MF15284
Haugen, T. O., Aass, P., Stenseth, N. C., and Vøllestad, L. A. (2008). Changes in selection and evolutionary responses in migratory brown trout following the construction of a fish ladder. Evol. Appl. 1, 319–335. doi: 10.1111/j.1752-4571.2008.00031.x
Heim, K. C., Wipfli, M. S., Whitman, M. S., and Seitz, A. C. (2016). Body size and condition influence migration timing of juvenile Arctic grayling. Ecol. Freshw. Fish 25, 156–166. doi: 10.1111/eff.12199
Hendry, A. P., and Day, T. (2005). Population structure attributable to reproductive time: isolation by time and adaptation by time. Mol. Ecol. 14, 901–916. doi: 10.1111/j.1365-294X.2005.02480.x
Hertz, P. E., Huey, R. B., and Stevenson, R. D. (1993). Evaluating temperature regulation by field-active ectotherms: the fallacy of the inappropriate question. Am. Nat. 142, 796–818. doi: 10.1086/285573
Hodgson, S., and Quinn, T. P. (2002). The timing of adult sockeye salmon migration into fresh water: adaptations by populations to prevailing thermal regimes. Can. J. Zool. 80, 542–555. doi: 10.1139/z02-030
Holmquist, J. G., Schmidt-Gengenbach, J. M., and Yoshioka, B. B. (1998). High dams and marine-freshwater linkages: effects on native and introduced fauna in the Caribbean. Cons. Biol. 12, 621–630. doi: 10.1046/j.1523-1739.1998.96427.x
Hórreo, J. L. (2015). “Captive Breeding and Conservation,” in Evolutionary Biology of the Atlantic Salmon, eds T. Vladic and E. Petersson (Boca Raton, FA: CRC Press Taylor and Francis Group), 273–296. doi: 10.1201/b18721-19
Huff, D. D., Miller, L. M., Chizinski, C. J., and Vondracek, B. (2011). Mixed-source reintroductions lead to outbreeding depression in second-generation descendents of a native North American fish. Mol. Ecol. 20, 4246–4258. doi: 10.1111/j.1365-294X.2011.05271.x
Hufford, K. M., Krauss, S. L., and Veneklaas, E. J. (2012). Inbreeding and outbreeding depression in Stylidium hispidum: implications for mixing seed sources for ecological restoration. Ecol. Evol. 2, 2262–2273. doi: 10.1002/ece3.302
Hughes, A. R., Inouye, B. D., Johnson, M. T. J., Underwood, N., and Vellend, M. (2008). Ecological consequences of genetic diversity. Ecol. Lett. 11, 609–623. doi: 10.1111/j.1461-0248.2008.01179.x
Hui, C., Fox, G. A., and Gurevitch, J. (2017). Scale-dependent portfolio effects explain growth inflation and volatility reduction in landscape demography. Proc. Natl. Acad. Sci. U.S.A. 114, 12507–12511. doi: 10.1073/pnas.1704213114
IPCC (2013). Climate Change 2013: The Physical Science Basis. Contribution of Working Group I to the Fifth Assessment Report of the Intergovernmental Panel on Climate Change. Cambridge, New York, NY: Cambridge University Press.
IPCC (2018). “Global warming of 1.5°C,” in An IPCC Special Report on the Impacts of Global Warming of 1.5°C Above Pre-Industrial Levels and Related Global Greenhouse Gas Emission Pathways, in the Context of Strengthening the Global Response to the Threat of Climate Change, Sustainable Development, and Efforts to Eradicate Poverty, eds V. Masson-Delmotte, P. Zhai, H.O. Pörtner, D. Roberts, J. Skea, P.R. Shukla, A. Pirani, W. Moufouma-Okia, C. Péan, R. Pidcock, S. Connors, J.B.R. Matthews, Y. Chen, X. Zhou, M.I. Gomis, E. Lonnoy, T. Maycock, M. Tignor and T. Waterfield (Geneva: IPCC).
Jackson, J. B. C., Kirby, M. X., Berger, W. H., Bjorndal, K. A., Botsford, L. W., Bourque, B. J., et al. (2001). Historical overfishing and the recent collapse of coastal ecosystems. Science 293, 629–637. doi: 10.1126/science.1059199
Jensen, L. F., Hansen, M. M., Pertoldi, C., Holdensgaard, G., Mensberg, K.-L. D., and Loeschcke, V. (2008). Local adaptation in brown trout early life-history traits: implications for climate change adaptability. Proc. R. Soc. B. 275, 2859–2868. doi: 10.1098/rspb.2008.0870
Jepsen, N., Aarestrup, K., Økland, F., and Rasmussen, G. (1998). Survival of radio-tagged Atlantic salmon (Salmo salar L.) and trout (Salmo trutta L.) smolts passing a reservoir during seaward migration. Hydrobiologia 371/372, 347–353. doi: 10.1007/978-94-011-5090-3_39
Jones, A. W., Palkovacs, E. P., and Post, D. M. (2013). Recent parallel divergence in body shape and diet source of alewife life history forms. Evol. Ecol. 27, 1175–1187. doi: 10.1007/s10682-013-9650-2
Jonsson, B., Jonsson, M., and Jonsson, N. (2017). Influences of migration phenology on survival are size-dependent in juvenile Atlantic salmon (Salmo salar). Can. J. Zool. 95, 581–587. doi: 10.1139/cjz-2016-0136
Jonsson, B., and Jonsson, N. (1993). Partial migration: niche shift versus sexual maturation in fishes. Rev. Fish Biol. Fisher. 3, 348–365. doi: 10.1007/BF00043384
Jonsson, B., and Jonsson, N. (2011). Ecology of Atlantic Salmon and Brown Trout: Habitat as a Template for Life Histories. Basel: Springer. doi: 10.1007/978-94-007-1189-1
Jonsson, B., Jonsson, N., and Hansen, L. P. (2003). Atlantic salmon straying from the River Imsa. J. Fish Biol. 62, 641–657. doi: 10.1046/j.1095-8649.2003.00053.x
Kassen, R. (2002). The experimental evolution of specialists, generalists, and the maintenance of diversity. J. Evol. Biol. 15, 173–190. doi: 10.1046/j.1420-9101.2002.00377.x
Kavanagh, K. D., Haugen, T. O., Gregersen, F., Jernvall, J., and Vøllestad, L. A. (2010). Contemporary temperature-driven divergence in a Nordic freshwater fish under conditions commonly thought to hinder adaptation. BMC Evol. Biol. 10, 350–350. doi: 10.1186/1471-2148-10-350
Keefer, M. L., and Caudill, C. C. (2014). Homing and straying by anadromous salmonids: a review of mechanisms and rates. Rev. Fish Biol. Fisher. 24, 333–368. doi: 10.1007/s11160-013-9334-6
Kekalainen, J., Oskoei, P., Janhunen, M., Koskinen, H., Kortet, R., and Huuskonen, H. (2018). Sperm pre-fertilization thermal environment shapes offspring phenotype and performance. J. Exoper. Biol. 221:10.1242/jeb.181412. doi: 10.1242/jeb.181412
Keller, L. F., and Waller, D. M. (2002). Inbreeding effects in wild populations. Trends Ecol. Evol. 17, 230–241. doi: 10.1016/S0169-5347(02)02489-8
Kennedy, R. J., and Crozier, W. W. (2010). Evidence of changing migratory patterns of wild Atlantic salmon Salmo salar smolts in the River Bush, Northern Ireland, and possible associations with climate change. J. Fish Biol. 76, 1786–1805. doi: 10.1111/j.1095-8649.2010.02617.x
Kindsvater, H. K., Braun, D. C., Otto, S. P., and Reynolds, J. D. (2016). Costs of reproduction can explain the correlated evolution of semelparity and egg size: theory and a test with salmon. Ecol. Lett. 19, 687–696. doi: 10.1111/ele.12607
Kinnison, M. T., Unwin, M. J., Hendry, A. P., and Quinn, T. P. (2001). Migratory costs and the evolution of egg size and number in introduced and indigenous salmon populations. Evolution 55, 1656–1667. doi: 10.1111/j.0014-3820.2001.tb00685.x
Kovach, R. P., Ellison, S. C., Pyare, S., and Tallmon, D. A. (2015). Temporal patterns in adult salmon migration timing across southeast Alaska. Glob. Change Biol. 21, 1821–1833. doi: 10.1111/gcb.12829
Kovach, R. P., Gharrett, A. J., and Tallmon, D. A. (2012). Genetic change for earlier migration timing in a pink salmon population. Proc. R. Soc. B. 279, 3870–3878. doi: 10.1098/rspb.2012.1158
Kuparinen, A., and Merilä, J. (2007). Detecting and managing fisheries induced evolution. Trends Ecol. Evol. 22, 652–659. doi: 10.1016/j.tree.2007.08.011
Kuparinen, A., and Merilä, J. (2009). Variation in the timing of river entry of Atlantic salmon (Salmo salar L.) in the Baltic. Curr. Zool. 55, 342–349.
Lande, R., and Arnold, S. J. (1983). The measurement of selection on correlated characters. Evolution 37, 1210–1226. doi: 10.1111/j.1558-5646.1983.tb00236.x
Larsson, P., Tibblin, P., Koch-Schmidt, P., Engstedt, O., Nilsson, J., Nordahl, O., et al. (2015). Ecology, evolution, and management strategies of Northern pike populations in the Baltic Sea. Ambio 44 (Suppl. 3), S451–S461. doi: 10.1007/s13280-015-0664-6
Lavergne, S., and Molofsky, J. (2007). Increased genetic variation and evolutionary potential drive the success of an invasive grass. Proc. Natl. Acad. Sci. U.S.A. 104, 3883–3888. doi: 10.1073/pnas.0607324104
Leggett, W. C. (1977). The ecology of fish migrations. Ann. Rev. Ecol. Syst. 8, 285–308. doi: 10.1146/annurev.es.08.110177.001441
Leimar, O., Hammerstein, P., and VanDooren, T. J. M. (2006). A new perspective on developmental plasticity and the principles of adaptive morph determination. Am. Nat. 167, 367–376. doi: 10.1086/499566
Leitwein, M., Garza, J. C., and Pearse, D. E. (2016). Ancestry and adaptive evolution of anadromous, resident, and adfluvial rainbow trout (Oncorhynchus mykiss) in the San Francisco bay area: application of adaptive genomic variation to conservation in a highly impacted landscape. Evol. Appl. 10, 56–67. doi: 10.1111/eva.12416
Levin, P. S., Zabel, R. W., and Williams, J. G. (2001). The road to extinction is paved with good intentions: negative association of fish hatcheries with threatened salmon. Proc. Biol. Sci. 268, 1153–1158. doi: 10.1098/rspb.2001.1634
Littrell, K. A., Ellis, D., Gephard, S. R., MacDonald, A. D., Palkovacs, E. P., Scranton, K., et al. (2018). Evaluating the potential for prezygotic isolation and hybridization between landlocked and anadromous alewife (Alosa pseudoharengus) following secondary contact. Evol. Appl. 11, 1554–1566. doi: 10.1111/eva.12645
Locke, A., Hanson, J. M., Klassen, G. J., Richardson, S. M., and Aubé, C. I. (2003). The damming of the Petitcodiac River: species, populations, and habitats lost. Northeast. Natural. 10, 39–54. doi: 10.1656/1092-6194(2003)010[0039:TDOTPR]2.0.CO;2
Long, J. H., Krenitsky, N. M., Roberts, S. F., Hirokawa, J., de Leeuw, J., and Porter, M. E. (2011). Testing biomimetic structures in bioinspired robots: how vertebrae control the stiffness of the body and the behavior of fish-like swimmers. Integr. Comp. Biol. 51, 158–175. doi: 10.1093/icb/icr020
Lowerre-Barbieri, S., DeCelles, G., Pepin, P., Catalán, I. A., Muhling, B., Erisman, B., et al. (2017). Reproductive resilience: a paradigm shift in understanding spawner-recruit systems in exploited marine fish. Fish Fisher 18, 285–312. doi: 10.1111/faf.12180
Lowerre-Barbieri, S. K., Kays, R., Thorson, J. T., and Wikelski, M. (2019). The ocean's movescape: fisheries management in the bio-logging decade (2018–2028). ICES J. Mar. Sci. 76, 477–488. doi: 10.1093/icesjms/fsy211
Lucas, M. C., and Baras, E. (2001). Migration of Freshwater Fishes. Oxford: Blackwell Science Ltd. doi: 10.1002/9780470999653
Lynch, A. J., Cooke, S. J., Deines, A. M., Bower, S. D., Bunnell, D. B., Cowx, I. G., et al. (2016). The social, economic, and environmental importance of inland fish and fisheries. Environ. Rev. 24, 115–121. doi: 10.1139/er-2015-0064
Lynch, M. (1991). The genetic interpretation of inbreeding depression and outbreeding depression. Evolution 45, 622–629. doi: 10.1111/j.1558-5646.1991.tb04333.x
Ma, G., Bai, C.-M., Wang, X.-J., Majeed, M. Z., and Ma, C.-S. (2018). Behavioural thermoregulation alters microhabitat utilization and demographic rates in ectothermic invertebrates. Anim. Behav. 142, 49–57. doi: 10.1016/j.anbehav.2018.06.003
Mackenzie, B. R., Gislason, H., Möllmann, C., and Köster, F. W. (2007). Impact of 21st century climate change on the Baltic Sea fish community and fisheries. Glob. Change Biol. 13, 1348–1367. doi: 10.1111/j.1365-2486.2007.01369.x
Mäkinen, H., Papakostas, S., Vøllestad, L. A., Leder, E. H., and Primmer, C. R. (2016). Plastic and evolutionary gene expression responses are correlated in European grayling (Thymallus thymallus) subpopulations adapted to different thermal environments. J. Hered. 107, 82–89. doi: 10.1093/jhered/esv069
May, M. L. (1979). Insect thermoregulation. Ann. Rev. Entomol. 24, 313–349. doi: 10.1146/annurev.en.24.010179.001525
Maynard, G. A., Kinnison, M. T., and Zydlewski, J. D. (2017). Size selection from fishways and potential evolutionary responses in a threatened Atlantic salmon population. River. Res. Appl. 33, 1004–1015. doi: 10.1002/rra.3155
McClelland, E., and Naish, K. (2007). What is the fitness outcome of crossing unrelated fish populations? A meta-analysis and an evaluation of future research directions. Conserv. Gen. 8, 397–416. doi: 10.1007/s10592-006-9178-x
McDowall, M. (2003). Variation in vertebral number in galaxiid fishes, how fishes swim and a possible reason for pleomerism. Rev. Fish Biol. Fisher 13, 247–263. doi: 10.1023/B:RFBF.0000033121.97066.c1
McDowall, R. M. (1997). The evolution of diadromy in fishes (revisited) and its place in phylogenetic analysis. Rev. Fish Biol. Fisher 7, 443–462. doi: 10.1023/A:1018404331601
McDowall, R. M., Mitchell, C. P., and Brothers, E. B. (1994). Age at migration from the sea of juvenile galaxias in new-zealand (Pisces, galaxiidae). Bull. Mar. Sci. 54, 385–402.
McGinnity, P., Jennings, E., deEyto, E., Allott, N., Samuelsson, P., Rogan, G., et al. (2009). Impact of naturally spawning captive-bred Atlantic salmon on wild populations: depressed recruitment and increased risk of climate-mediated extinction. Proc. R. Soc. B 276, 3601–3610. doi: 10.1098/rspb.2009.0799
McGinnity, P., Prodöhl, P., Ferguson, A., Hynes, R., Maoiléidigh, N. Ó., Baker, N., et al. (2003). Fitness reduction and potential extinction of wild populations of Atlantic salmon, Salmo salar, as a result of interactions with escaped farm salmon. Proc. R. Soc. B 270, 2443–2450. doi: 10.1098/rspb.2003.2520
McLaughlin, R. L., Smyth, E. R. B., Castro-Santos, T., Jones, M. L., Koops, M. A., Pratt, T. C., et al. (2013). Unintended consequences and trade-offs of fish passage. Fish Fisher 14, 580–604. doi: 10.1111/faf.12003
Mehner, T. (2012). Diel vertical migration of freshwater fishes – proximate triggers, ultimate causes and research perspectives. Freesw. Biol. 57, 1342–1359. doi: 10.1111/j.1365-2427.2012.02811.x
Molofsky, J., Keller, S. R., Lavergne, S., Kaproth, M. A., and Eppinga, M. B. (2014). Human- aided admixture may fuel ecosystem transformation during biological invasions: theoretical and experimental evidence. Ecol. Evol. 4, 899–910. doi: 10.1002/ece3.966
Momigliano, P., Jokinen, H., Fraimout, A., Florin, A.-B., Norkko, A., and Merilä, J. (2017). Extraordinarily rapid speciation in a marine fish. Proc. Natl. Acad. Sci. U.S.A. 114, 6074–6079. doi: 10.1073/pnas.1615109114
Morbey, Y. E., and Ydenberg, R. C. (2001). Protandrous arrival timing to breeding areas: a review. Ecol. Lett. 4, 663–673. doi: 10.1046/j.1461-0248.2001.00265.x
Morita, K. (2019). Earlier migration timing of salmonids: an adaptation to climate change or maladaptation to the fishery? Can. J. Fish Aquat. Sci. 76, 475–479. doi: 10.1139/cjfas-2018-0078.
Morita, K., and Yamamoto, S. (2002). Effects of habitat fragmentation by damming on the persistence of stream-dwelling charr populations. Conserv. Biol. 16, 1318–1323. doi: 10.1046/j.1523-1739.2002.01476.x
Mousseau, T. A., and Fox, C. W. (1998). The adaptive significance of maternal effects. Trends Ecol. Evol. 13, 403–407. doi: 10.1016/S0169-5347(98)01472-4
Moyle, P. B. (2004). Fishes: An Introduction to Ichthyology. San Francisco, CA: Pearson Benjamin Cummings.
Myers, G. S. (1949). Usage of anadromous, catadromous and allied terms for migratory fishes. Copeia 1949, 89–97. doi: 10.2307/1438482
Naiman, R. J., Bilby, R. E., Schindler, D. E., and Helfield, J. M. (2002). Pacific salmon, nutrients, and the dynamics of freshwater and riparian ecosystems. Ecosystemes 5, 399–417. doi: 10.1007/s10021-001-0083-3
Nakamura, I., Goto, Y., Sato, K., and Hays, G. (2015). Ocean sunfish rewarm at the surface after deep excursions to forage for siphonophores. J. Anim. Ecol. 84, 590–603. doi: 10.1111/1365-2656.12346
Narum, S. R., Hatch, D., Talbot, A. J., Moran, P., and Powell, M. S. (2008). Iteroparity in complex mating systems of steelhead Oncorhynchus mykiss (Walbaum). J. Fish Biol. 72, 45–60. doi: 10.1111/j.1095-8649.2007.01649.x
Nathan, R., Getz, W. M., Revilla, E., Holyoak, M., Kadmon, R., Saltz, D., et al. (2008). A movement ecology paradigm for unifying organismal movement research. Proc. Natl. Acad. Sci. U.S.A. 105, 19052–19059. doi: 10.1073/pnas.0800375105
Newton, M., Dodd, J. A., Barry, J., Boylan, P., and Adams, C. E. (2018). The impact of a small-scale riverine obstacle on the upstream migration of Atlantic Salmon. Hydrobiologia 806, 251–264. doi: 10.1007/s10750-017-3364-3
Ngor, P. B., Oberdorff, T., Phen, C., Baehr, C., Grenouillet, G., and Lek, S. (2018). Fish assemblage responses to flow seasonality and predictability in a tropical flood pulse system. Ecosphere 9:e02366. doi: 10.1002/ecs2.2366
Nickelson, T. (2003). The influence of hatchery coho salmon (Oncorhynchus kisutch) on the productivity of wild coho salmon populations in Oregon coastal basins. Can. J. Fish. Aquat. Sci. 60, 1050–1056. doi: 10.1139/f03-091
Nilsson, C., Reidy, C. A., Dynesius, M., and Revenga, C. (2005). Fragmentation and flow regulation of the world ' s large river systems. Science 308, 405–408. doi: 10.1126/science.1107887
Nilsson, J., Flink, H., and Tibblin, P. (2019). Predator–prey role reversal may impair the recovery of declining pike populations. J. Anim. Ecol. 88, 927–939. doi: 10.1111/1365-2656.12981
Nordahl, O. (2018). Intraspecific diversity of pike (Esox lucius) in the Baltic Sea and new insights on thermoregulation in fish. Ph.D., Linnaeus University, Kalmar.
Nordahl, O., Koch-Schmidt, P., Sunde, J., Yildirim, Y., Tibblin, P., Forsman, A., et al. (in press). Genetic differentiation between and within ecotypes of pike (Esox lucius) in the Baltic Sea. Aquat. Conserv. Mar. Freshw. Ecosyst.
Nordahl, O., Koch-Schmidt, P., Tibblin, P., Forsman, A., and Larsson, P. (2019). Vertical movements of coastal pike (Esox lucius) – on the role of sun basking. Ecol. Freshw. Fish. doi: 10.1111/eff.12484. [Epub ahead of print].
Nordahl, O., Tibblin, P., Koch-Schmidt, P., Berggren, H., Larsson, P., and Forsman, A. (2018). Sun basking fish benefit from body temperatures that are higher than ambient water. Proc. R. Soc. B 285:20180639. doi: 10.1098/rspb.2018.0639
O'Connor, C. M., Norris, D. R., Crossin, G. T., and Cooke, S. J. (2014). Biological carryover effects: linking common concepts and mechanisms in ecology and evolution. Ecosphere 5:art28. doi: 10.1890/ES13-00388.1
Odling-Smee, L., and Braithwaite, V. A. (2003). The role of learning in fish orientation. Fish Fisher 4, 235–246. doi: 10.1046/j.1467-2979.2003.00127.x
Oke, K. B., and Hendry, A. P. (2019). Genetic insights into the past, present, and future of a keystone species. Proc. Natl. Acad. Sci. U.S.A. 116, 344–346. doi: 10.1073/pnas.1819789116
Olsson, I. C., and Greenberg, L. A. (2004). Partial migration in a landlocked brown trout population. J. Fish Biol. 65, 106–121. doi: 10.1111/j.0022-1112.2004.00430.x
Olsson, I. C., Greenberg, L. A., Bergman, E., and Wysujack, K. (2006). Environmentally induced migration: the importance of food. Ecol. Lett. 9, 645–651. doi: 10.1111/j.1461-0248.2006.00909.x
Parmesan, C., and Yohe, G. (2003). A globally coherent fingerprint of climate change impacts across natural systems. Nature 421:37–42. doi: 10.1038/nature01286
Pauly, D., Christensen, V., Dalsgaard, J., Froese, R., and Torres, F. (1998). Fishing down marine food webs. Science 279, 860–863. doi: 10.1126/science.279.5352.860
Pépino, M., Goyer, K., and Magnan, P. (2015). Heat transfer in fish: are short excursions between habitats a thermoregulatory behaviour to exploit resources in an unfavourable thermal environment? J. Exp. Biol. 218, 3461–3467. doi: 10.1242/jeb.126466
Perry, A. L., Low, P. J., Ellis, J. R., and Reynolds, J. D. (2005). Climate change and distribution shifts in marine fishes. Science 308, 1912–1915. doi: 10.1126/science.1111322
Pess, G. R., Quinn, T. P., Gephard, S. R., and Saunders, R. (2014). Re-colonization of Atlantic and Pacific rivers by anadromous fishes: linkages between life history and the benefits of barrier removal. Rev. Fish Biol. Fisher 24, 881–900. doi: 10.1007/s11160-013-9339-1
Petersson, E. (2015). “Homing and timing of reproduction,” in Evolutionary Biology of Atlantic Salmon, eds T. Vladić and E. Petersson (Boca Raton: CRC Press), 44–59.
Piersma, T., and Drent, J. (2003). Phenotypic flexibility and the evolution of organismal design. Trends Ecol. Evol. 18, 228–233. doi: 10.1016/S0169-5347(03)00036-3
Pigliucci, M. (2001). Phenotypic Plasticity Beyond Nature and Nurture. Baltimore: The John Hopkins University Press.
Pinsky, M. L., Reygondeau, G., Caddell, R., Palacios-Abrantes, J., Spijkers, J., and Cheung, W. W. L. (2018). Preparing ocean governance for species on the move. Science 360, 1189–1191. doi: 10.1126/science.aat2360
Plantalech manel-la, N., Chittenden, C. M., Okland, F., Thorstad, E. B., Davidsen, J. G., Sivertsgard, R., et al. (2011). Does river of origin influence the early marine migratory performance of Salmo salar? J. Fish Biol. 78, 624–634. doi: 10.1111/j.1095-8649.2010.02882.x
Podgorniak, T., Angelini, M., De Oliveira, E., Daverat, F., and Pierron, F. (2017). Selective pressure of fishways upon morphological and muscle enzymatic traits of migrating glass eels. Can. J. Fish. Aquat. Sci. 74, 445–451. doi: 10.1139/cjfas-2016-0110
Podgorniak, T., Blanchet, S., De Oliveira, E., Daverat, F., and Pierron, F. (2016). To boldly climb: behavioural and cognitive differences in migrating European glass eels. R. Soc. Open Sci. 3:150665. doi: 10.1098/rsos.150665
Post, D. M., Palkovacs, E. P., Schielke, E. G., and Dodson, S. I. (2008). Intrapsecific variation in a predator affects community structure and cascading trophic interactions. Ecology 89, 2019–2032. doi: 10.1890/07-1216.1
Quinn, T. P. (1993). A review of homing and straying of wild and hatchery-produced salmon. Fish. Res. 18, 29–44. doi: 10.1016/0165-7836(93)90038-9
Quinn, T. P. (2005). The Behavior and Ecology of Pacific Salmon and Trout. Bethesda, MA: Univeristy of Washington Press.
Quinn, T. P., Unwin, M. J., and Kinnison, M. T. (2000). Evolution of temporal isolation in the wild: genetic divergence in timing of migration and breeding by introduced chinook salmon populations. Evolution 54, 1372–1385. doi: 10.1111/j.0014-3820.2000.tb00569.x
Ratikainen, I. I., and Kokko, H. (2019). The coevolution of lifespan and reversible plasticity. Nat. Commun. 10:538. doi: 10.1038/s41467-019-08502-9
Reusch, T. B. H., Dierking, J., Andersson, H. C., Bonsdorff, E., Carstensen, J., Casini, M., et al. (2018). The Baltic Sea as a time machine for the future coastal ocean. Sci. Adv. 4:eaar8195. doi: 10.1126/sciadv.aar8195
Reynolds, W. W., and Casterlin, M. E. (1980). “The role of temperature in the environmental physiology of fishes,” in Environmental Physiology of Fishes, ed M. A. Ali (Boston, MA: Springer US), 497–518.
Rhymer, J. M., and Simberloff, D. (1996). Extinction by hybridization and introgression. Ann. Rev. Ecol. Syst. 27, 83–109. doi: 10.1146/annurev.ecolsys.27.1.83
Rieman, B. E., and Dunham, J. B. (2000). Metapopulations and salmonids: a synthesis of life history patterns and empirical observations. Ecol. Freshw. Fish 9, 51–64. doi: 10.1034/j.1600-0633.2000.90106.x
Rius, M., and Darling, J. A. (2014). How important is intraspecific genetic admixture to the success of colonising populations? Trends Ecol. Evol. 29, 233–242. doi: 10.1016/j.tree.2014.02.003
Roessig, J. M., Woodley, C. M., Cech, J. J., and Hansen, L. J. (2004). Effects of global climate change on marine and estuarine fishes and fisheries. Rev. Fish Biol. Fisher 14, 251–275. doi: 10.1007/s11160-004-6749-0
Roff, D. A. (1988). The evolution of migration and some life history parameters in marine fishes. Env. Biol. Fish 22, 133–146. doi: 10.1007/BF00001543
Rollinson, N., Keith, D. M., Houde, A. L. S., Debes, P. V., McBride, M. C., and Hutchings, J. A. (2014). Risk assessment of inbreeding and outbreeding depression in a captive-breeding program. Conserv. Biol. 28, 529–540. doi: 10.1111/cobi.12188
Rondeau, E. B., Minkley, D. R., Leong, J. S., Messmer, A. M., Jantzen, J. R., von Schalburg, K. R., et al. (2014). The genome and linkage map of the northern pike (Esox lucius): conserved synteny revealed between the Salmonid sister group and the Neoteleostei. PLoS ONE 9:e102089. doi: 10.1371/journal.pone.0102089
Root, T. L., Price, J. T., Hall, K. R., Schneider, S. H., Rosenzweig, C., and Pounds, J. A. (2003). Fingerprints of global warming on wild animals and plants. Nature 421, 57–60. doi: 10.1038/nature01333
Russell, D. J., and Garrett, R. N. (1983). Use by juvenile barramundi, Lates calcarifer (Bloch), and other fishes of temporary supralittoral habitats in a tropical estuary in Northern Australia. Mar. Freshw. Res. 34, 805–811. doi: 10.1071/MF9830805
Saint-Pé, K., Blanchet, S., Tissot, L., Poulet, N., Plasseraud, O., Loot, G., et al. (2018). Genetic admixture between captive-bred and wild individuals affects patterns of dispersal in a brown trout (Salmo trutta) population. Conserv. Gen. 19, 1269–1279. doi: 10.1007/s10592-018-1095-2
Säisä, M., Koljonen, M.-L., and Tähtinen, J. (2003). Genetic changes in Atlantic salmon stocks since historical times and the effective population size of a long-term captive breeding programme. Conserv. Gen. 4, 613–627. doi: 10.1023/A:1025680002296
Schindler, D. E., Armstrong, J. B., and Reed, T. E. (2015). The portfolio concept in ecology and evolution. Front. Ecol. Environ. 13, 257–263. doi: 10.1890/140275
Schindler, D. E., Hilborn, R., Chasco, B., Boatright, C. P., Quinn, T. P., Rogers, L. A., et al. (2010). Population diversity and the portfolio effect in an exploited species. Nature 465, 609–612. doi: 10.1038/nature09060
Seamons, T. R., and Quinn, T. P. (2010). Sex-specific patterns of lifetime reproductive success in single and repeat breeding steelhead trout (Oncorhynchus mykiss). Behav. Ecol. Sociobiol. 64, 505–513. doi: 10.1007/s00265-009-0866-7
Secor, D. H., and Kerr, L. A. (2009). Lexicon of life cycle diversity in diadromous and other fishes. Amer. Fisher Soc. Symp. 69, 537–556.
Seddon, P. J., Armstrong, D. P., and Maloney, R. F. (2007). Developing the science of reintroduction biology. Conserv. Biol. 21, 303–312. doi: 10.1111/j.1523-1739.2006.00627.x
Senner, N. R., Conklin, J. R., and Piersma, T. (2015). An ontogenetic perspective on individual differences. Proc. Biol. Sci. 282:20151050. doi: 10.1098/rspb.2015.1050
Service USFW (ed.). (2012). National Fish Passage Program - Creating Aquatic Possibilities. U.S.F.W. Service.
Shi, J., Joshi, J., Tielbörger, K., Verhoeven, K. J. F., and Macel, M. (2018). Costs and benefits of admixture between foreign genotypes and local populations in the field. Ecol. Evol. 8, 3675–3684. doi: 10.1002/ece3.3946
Silva, A. T., Lucas, M. C., Castro-Santos, T., Katopodis, C., Baumgartner, L. J., Thiem, J. D., et al. (2018). The future of fish passage science, engineering, and practice. Fish Fisher 19, 340–362. doi: 10.1111/faf.12258
Silva, S., Araújo, M. J., Bao, M., Mucientes, G., and Cobo, F. (2014). The haematophagous feeding stage of anadromous populations of sea lamprey Petromyzon marinus: low host selectivity and wide range of habitats. Hydrobiologia 734, 187–199. doi: 10.1007/s10750-014-1879-4
Simberloff, D. (2009). The role of propagule pressure in biological invasions. Ann. Rev. Ecol. Syst. 40, 81–102. doi: 10.1146/annurev.ecolsys.110308.120304
Skov, C., Aarestrup, K., Baktoft, H., Brodersen, J., Brönmark, C., Hansson, L.-A., et al. (2010). Influences of environmental cues, migration history, and habitat familiarity on partial migration. Behav. Ecol. 21, 1140–1146. doi: 10.1093/beheco/arq121
Skov, C., Baktoft, H., Brodersen, J., Brönmark, C., Chapman, B. B., Hansson, L.-A., et al. (2011). Sizing up your enemy: individual predation vulnerability predicts migratory probability. Proc. R. Soc. B 278, 1414–1418. doi: 10.1098/rspb.2010.2035
Skov, C., and Nilsson, P. A. (eds.). (2017). Biology and Ecology of Pike. (Boca Raton, FL: CRC Press; Taylor & Francis Group).
Smith, B., and Tibbles, J. (1980). Sea lamprey (Petromyzon marinus) in Lakes Huron, Michigan, and superior: history of invasion and control, 1936-78. Can. J. Fish. Aquat. Sci. 37, 1780–1801. doi: 10.1139/f80-222
Snell-Rood, E. C. (2013). An overview of the evolutionary causes and consequences of behavioural plasticity. Anim. Behav. 85, 1004–1011. doi: 10.1016/j.anbehav.2012.12.031
Søndergaard, M., Jeppesen, E., Jensen, J. P., and Lauridsen, T. (2000). Lake restoration in Denmark. Lakes Reserv. Sci. Pol. Managm. Syst. Use 5, 151–159. doi: 10.1046/j.1440-1770.2000.00110.x
Stearns, S. C. (1989). The evolutionary significance of phenotypic plasticity - phenotypic sources of variation among organisms can be described by developmental switches and reaction norms. Bioscience 39, 436–445. doi: 10.2307/1311135
Sunde, J., and Forsman, A. (2016). Mate choice is not consistent with short-term effects of intraspecific admixture in woodlice. Biol. J. Linn. Soc. 119, 359–369. doi: 10.1111/bij.12811
Sunde, J., Larsson, P., and Forsman, A. (in press). Adaptations of early development to local spawning temperature in anadromous populations of pike (Esox lucius). BMC Evol. Biol.
Sunde, J., Tamario, C., Tibblin, P., Larsson, P., and Forsman, A. (2018a). Variation in salinity tolerance between and within anadromous subpopulations of pike (Esox lucius). Sci. Rep. 8:22. doi: 10.1038/s41598-018-24805-1
Sunde, J., Tibblin, P., Larsson, P., and Forsman, A. (2018b). Sex-specific effects of outbreeding on offspring quality in pike (Esox lucius). Ecol. Evol. 8, 10448–10459. doi: 10.1002/ece3.4510
Swain, D. P. (1992). The functional basis of natural-selection for vertebral traits of larvae in the stickleback Gasterosteus aculeatus. Evolution 46, 987–997. doi: 10.1111/j.1558-5646.1992.tb00614.x
Tamario, C., Calles, O., Watz, J., Nilsson, P. A., and Degerman, E. (2019). Coastal river connectivity and the distribution of ascending juvenile European eel (Anguilla anguilla L.) - implications for conservation strategies regarding fish passage solutions. Aquat. Conserv. Mar. Freshw. Ecosyst. 29, 612–622. doi: 10.1002/aqc.3064
Tamario, C., Degerman, E., Donadi, S., Spjut, D., and Sandin, L. (2018). Nature-like fishways as compensatory lotic habitats. River Res. Appl. 34, 253–261. doi: 10.1002/rra.3246
Thomassen, G., Barson, N. J., Haugen, T. O., and Vøllestad, L. A. (2011). Contemporary divergence in early life history in grayling (Thymallus thymallus). BMC Evol. Biol. 11, 360–360. doi: 10.1186/1471-2148-11-360
Thompson, T. Q., Bellinger, M. R., O'Rourke, S. M., Prince, D. J., Stevenson, A. E., Rodrigues, A. T., et al. (2019). Anthropogenic habitat alteration leads to rapid loss of adaptive variation and restoration potential in wild salmon populations. Proc. Natl. Acad. Sci. U.S.A. 116, 177–186. doi: 10.1073/pnas.1811559115
Tibblin, P., Berggren, H., Nordahl, O., Larsson, P., and Forsman, A. (2016a). Causes and consequences of intra-specific variation in vertebral number. Sci. Rep. 6:26372. doi: 10.1038/srep26372
Tibblin, P., Forsman, A., Borger, T., and Larsson, P. (2016b). Causes and consequences of repeatability, flexibility and individual fine-tuning of migratory timing in pike. J. Anim. Ecol. 85, 136–145. doi: 10.1111/1365-2656.12439
Tibblin, P., Forsman, A., Koch-Schmidt, P., Nordahl, O., Johannessen, P., Nilsson, et al. (2015). Evolutionary divergence of adult body size and juvenile growth in sympatric subpopulations of a top predator in aquatic ecosystems. Am. Nat. 168, 98–110. doi: 10.1086/681597
Tibblin, P., Koch-Schmidt, P., Larsson, P., and Stenroth, P. (2012). Effects of salinity on growth and mortality of migratory and resident forms of Eurasian perch in the Baltic Sea. Ecol. Freshw. Fish 21, 200–206. doi: 10.1111/j.1600-0633.2011.00537.x
Tinnert, J., Berggren, H., and Forsman, A. (2016). Population-specific effects of interbreeding and admixture on reproductive decisions and offspring quality. Ann. Zool. Fennic 53, 55–68. doi: 10.5735/086.053.0205
Tortajada, A. M., Carmona, M. J., and Serra, M. (2010). Effects of population outcrossing on rotifer fitness. BMC Evol. Biol. 10:312. doi: 10.1186/1471-2148-10-312
Tuomainen, U., and Candolin, U. (2011). Behavioural responses to human-induced environmental change. Biol. Rev. 86, 640–657. doi: 10.1111/j.1469-185X.2010.00164.x
Turner, L. M., Schwahn, D. J., and Harr, B. (2012). Reduced male fertility is common but highly variable in form and severity in a natural House mouse hybrid zone. Evolution 66, 443–458. doi: 10.1111/j.1558-5646.2011.01445.x
Unwin, M. J., Kinnison, M. T., and Quinn, T. P. (1999). Exceptions to semelparity: postmaturation survival, morphology, and energetics of male chinook salmon (Oncorhynchus tshawytscha). Can. J. Fish. Aquat. Sci. 56, 1172–1181. doi: 10.1139/f99-045
Uusi-Heikkila, S., Wolter, C., Klefoth, T., and Arlinghaus, R. (2008). A behavioral perspective on fishing-induced evolution. Trends Ecol. Evol. 23, 419–421. doi: 10.1016/j.tree.2008.04.006
Van Leeuwen, C. H. A., Dalen, K., Museth, J., Junge, C., and Vøllestad, L. A. (2018). Habitat fragmentation has interactive effects on the population genetic diversity and individual behaviour of a freshwater salmonid fish. River Res. Appl. 34, 60–68. doi: 10.1002/rra.3226
van Leeuwen, C. H. A., Museth, J., Sandlund, O. T., Qvenild, T., and Vøllestad, L. A. (2016). Mismatch between fishway operation and timing of fish movements: a risk for cascading effects in partial migration systems. Ecol. Evol. 6, 2414–2425. doi: 10.1002/ece3.1937
Verhoeven, K. J. F., Macel, M., Wolfe, L. M., and Biere, A. (2011). Population admixture, biological invasions and the balance between local adaptation and inbreeding depression. Proc. R. Soc. B Biol. Sci. 278, 2–8. doi: 10.1098/rspb.2010.1272
Volpato, G. L., Barreto, R. E., Marcondes, A. L., Andrade Moreira, P. S., and de Barros Ferreira, M. F. (2009). Fish ladders select fish traits on migration–still a growing problem for natural fish populations. Mar. Freshw. Behav. Phys. 42, 307–313. doi: 10.1080/10236240903299177
Waldman, J., Wilson, K. A., Mather, M., and Snyder, N. P. (2016). A resilience approach can improve anadromous fish restoration. Fisheries 41, 116–126. doi: 10.1080/03632415.2015.1134501
Walsh, B., and Lynch, M. (2012). Evolution and Selection of Quantitative Traits. Oxford: Oxford University Press.
Watanabe, Y. Y., Goldman, K. J., Caselle, J. E., Chapman, D. D., and Papastamatiou, Y. P. (2015). Comparative analyses of animal-tracking data reveal ecological significance of endothermy in fishes. Proc. Natl. Acad. Sci. U.S.A. 112, 6104–6109. doi: 10.1073/pnas.1500316112
Watz, J., Nilsson, P. A., Degerman, E., Tamario, C., and Calles, O. (2019). Climbing the ladder: an evaluation of three different anguillid eel climbing substrata and placement of upstream passage solutions at migration barriers. Anim. Conserv. doi: 10.1111/acv.12485
Webb, P. W. (1975). Hydrodynamics and energetics of fish propulsion. Bull. Fisher Res. Board Can. 190, 1–156.
Weeks, A. R., Sgro, C. M., Young, A. G., Frankham, R., Mitchell, N. J., Miller, K. A., et al. (2011). Assessing the benefits and risks of translocations in changing environments: a genetic perspective. Evol. Appl. 4, 709–725. doi: 10.1111/j.1752-4571.2011.00192.x
Wegner, N. C., Snodgrass, O. E., Dewar, H., and Hyde, J. R. (2015). Whole-body endothermy in a mesopelagic fish, the opah, Lampris guttatus. Science 348, 786–789. doi: 10.1126/science.aaa8902
Wennersten, L., and Forsman, A. (2012). Population-level consequences of polymorphism, plasticity and randomized phenotype switching: a review of predictions. Biol. Rev. 87, 756–767. doi: 10.1111/j.1469-185X.2012.00231.x
West-Eberhard, M. J. (2003). Developmental Plasticity and Evolution. Oxford: Oxford University Press.
Whitlock, R., Stewart, G., Goodman, S., Piertney, S., Butlin, R., Pullin, A., et al. (2013). A systematic review of phenotypic responses to between-population outbreeding. Envir. Evid. 2:13. doi: 10.1186/2047-2382-2-13
Whitman, D. W., and Agrawal, A. A. (2009). “What is phenotypic plasticity and why is it important?” in Phenotypic Plasticity of Insects: Mechanisms and Consequences, eds D. W. Whitman and T. N. Ananthakrishnan (Boca Raton, FL: CRC Press), 1–63.
Wilen, J. E. (1985). Towards a theory of the regulated fishery. Mar. Res. Econ. 1, 369–388. doi: 10.1086/mre.1.4.42628868
Wilmers, C. C., Nickel, B., Bryce, C. M., Smith, J. A., Wheat, R. E., and Yovovich, V. (2015). The golden age of bio-logging: how animal-borne sensors are advancing the frontiers of ecology. Ecology 96, 1741–1753. doi: 10.1890/14-1401.1
Wilson, D. S. (1998). Adaptive individual differences within single populations. Phil. Trans. R. Soc. B 353, 199–205. doi: 10.1098/rstb.1998.0202
Winkler, D. W., Jørgensen, C., Both, C., Houston, A. I., McNamara, J. M., Levey, D. J., et al. (2014). Cues, strategies, and outcomes: how migrating vertebrates track environmental change. Mov. Ecol. 2:10. doi: 10.1186/2051-3933-2-10
Keywords: biodiversity, climate change, developmental plasticity, evolution, fish migration, fishway, phenotypic flexibility, spawning migration
Citation: Tamario C, Sunde J, Petersson E, Tibblin P and Forsman A (2019) Ecological and Evolutionary Consequences of Environmental Change and Management Actions for Migrating Fish. Front. Ecol. Evol. 7:271. doi: 10.3389/fevo.2019.00271
Received: 08 March 2019; Accepted: 28 June 2019;
Published: 10 July 2019.
Edited by:
Yolanda E. Morbey, University of Western Ontario, CanadaReviewed by:
Christer Brönmark, Lund University, SwedenSue Katherine Lowerre-Barbieri, University of Florida, United States
Copyright © 2019 Tamario, Sunde, Petersson, Tibblin and Forsman. This is an open-access article distributed under the terms of the Creative Commons Attribution License (CC BY). The use, distribution or reproduction in other forums is permitted, provided the original author(s) and the copyright owner(s) are credited and that the original publication in this journal is cited, in accordance with accepted academic practice. No use, distribution or reproduction is permitted which does not comply with these terms.
*Correspondence: Anders Forsman, YW5kZXJzLmZvcnNtYW5AbG51LnNl