- 1Key Laboratory of Agricultural Water Resources, Hebei Laboratory of Agricultural Water-Saving, Center for Agricultural Resources Research, Institute of Genetics and Developmental Biology, Chinese Academy of Sciences, Shijiazhuang, China
- 2College of Advanced Agricultural Sciences, University of Chinese Academy of Sciences, Beijing, China
- 3College of Life Sciences, Hebei Agricultural University, Baoding, China
- 4State Key Laboratory of Plant Cell and Chromosome Engineering, Center for Agricultural Resources Research, Institute of Genetics and Developmental Biology, The Innovative Academy of Seed Design, Chinese Academy of Sciences, Shijiazhuang, China
Plant meristems are responsible for producing all post-embryonic organs during organogenesis. While the shoot apical meristem (SAM) maintains its meristematic property throughout the life of a plant, the floral meristem (FM) undergoes precise processes of initiation, maintenance and termination to ensure proper reproductive development and metagenesis. Plant meristem maintenance and termination are controlled by hierarchical genetic networks. While most of the genes in these networks have specific roles in particular processes, some genes have dual roles in SAM maintenance and FM termination through their interactions with different partners. Here, we summarize the molecular mechanisms of these dual-function regulators important for both SAM maintenance and FM termination and discuss the functions of WOX genes mediated gene regulatory networks on meristem maintenance and termination in different species.
Shoot Apical Meristem and Floral Meristem
Whereas animals complete the majority of organogenesis and body plan formation during embryogenesis, plants establish the shoot apical meristem (SAM) and root apical meristem (RAM) in the mature embryo (Jürgens, 2001; Lau et al., 2012; Bishopp and Bennett, 2014). During post-embryonic development, the RAM establishes the entire root system, and the SAM gives rise to the above-ground structures, such as leaves, stems and flowers (Miwa et al., 2009; Kaufmann et al., 2010; Satbhai et al., 2015). After the transition from vegetative development to reproductive development, the floral meristems (FMs) are produced in the axils of cryptic bracts at the flanking regions of SAM that is also called Inflorescence Meristem (IM) after floral transition (Figures 1A–C; Chandler, 2012). Therefore, the FM, IM and other secondary meristm such as axillary meristems (AMs) are three types of SAM. Subsequently, FMs generate all of the floral organs, such as sepals, petals, stamens and carpels in Arabidopsis (Figure 1D). Plant meristems consist of groups of undifferentiated cells that continually initiate new organ primordia, and these undifferentiated cells are produced from the limited number of stem cells within the meristem (Groß-Hardt and Laux, 2003; Laux, 2003; Sozzani and Iyer-Pascuzzi, 2014).
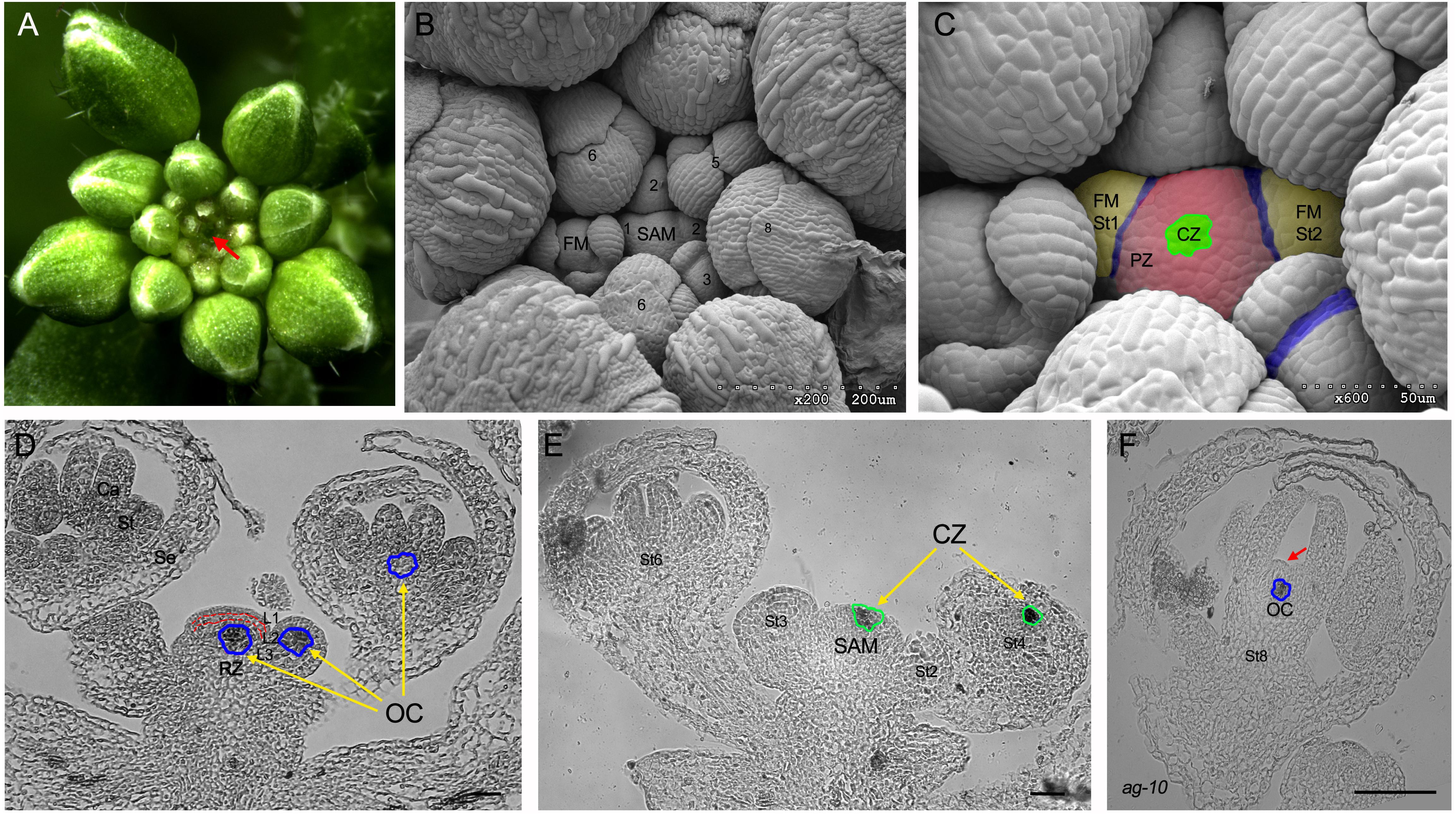
Figure 1. The shoot apical meristem (SAM) and floral meristems (FM) of Arabidopsis. (A) Top view of the inflorescence. The SAM is localized at the center and marked by a red arrow. (B,C) Overhead view of the SAM and FM. Numbers indicate the developmental stage of flower (Smyth et al., 1990). The central zone (CZ) and peripheral zone (PZ) are labeled by green and magenta color, respectively. The FMs are labeled by pale yellow color. The meristem–organ boundaries are marked in blue. (D,E) The longitudinal sections of SAM and FM. The organizing center (OC), in which the WUS is expressed, is marked by the blue cycles. The CZ that is marked by the expression of CLV3 detected by in situ hybridization is labeled by green circles. RZ, rib zone; L1, L1 cell layer; L2, L2 cell layer; Se, sepal; St, stamen; Ca, carpel. (F) In situ assay to show prolonged WUS expression (marked by blue circle) in one indeterminate floral bud at stage 8 of ag-10, a weak agamous allele, and the indeterminate meristem is marked by a red arrow. (D–F) Scale bar = 50 μm.
The SAM and FM of the model plant Arabidopsis (Figure 1A) have similar dome-like structure characterized by the typical tunica/corpus structure found in angiosperms (Figures 1B–E). The outer tunica consists of two single cell layers, the epidermal cells (L1) and subepidermal cells (L2), which divide anticlinally to the surface of the meristem; the inner corpus layer is a cluster of cells without clearly oriented divisions (Figure 1D; Carles and Fletcher, 2003). From the center outward, the tunica is divided into the central zone (CZ), which harbors a group of stem cells expressing the stem cell marker gene CLAVATA3 (CLV3) (Laux, 2003), and the peripheral zone (PZ), where the descendants of stem cells are displaced and recruited to generate new lateral organ primorida or FMs (Figure 1E). Stem cells are a group of pluripotent undifferentiated cells with two distinct capabilities: maintenance through self-renewal and the steady production of precursor cells to form differentiated tissues (Laux, 2003). The CZ is sustained by the underlying organizing center (OC), a part of the rib zone (RZ) underneath the CZ (Mayer et al., 1998; Alvarez-Buylla et al., 2010). The OC cells are characterized by WUSCHEL (WUS) gene expression (Figure 1D).
During development, successful initiation of lateral organ primordia, in which cells are differentiated into specific cell-type of distinct organ like leaf or flower, requires the formation of meristem-to-organ boundary zone to separate the newly formed entity from the rest of plan body (Aida and Tasaka, 2006). The boundary zone is morphologically visible as concave groove with a saddle-shaped surface due to the local growth repression (Figure 1C). Cells in the boundary have distinctive property compared to the surrounding cells with reduced rates of cell division, elongated shapes, and unique gene expression program (Breuil-Broyer et al., 2004; Zadnikova and Simon, 2014). Null mutants of boundary-specific genes often display organ fusion, but also impaired organ development and phyllotaxis patterning, indicating that the boundary zone acts as organizing center to control adjacent organ development (Laufs et al., 2004; Zadnikova and Simon, 2014; Wang et al., 2016). Therefore, the dynamic maintenance of meristem depends on the balance between meristem self-renewal and lateral organ formation.
In Arabidopsis, IM, a type of SAM, is indeterminate with its ability to generate new organ throughout the life of a plant, while FM, another type of SAM, undergoes precise processes of initiation, maintenance and termination to ensure proper reproductive development and metagenesis (Sablowski, 2007). During the lifecycle, once a certain number of fruits are produced, all meristem activity arrests coordinately (termed global proliferative arrest, or GPA) to promote subsequent fruit filling and plant death. Studies showed that both signals from seeds/flowers and the age pathway regulate the GPA of SAM (Hensel et al., 1994; Wuest et al., 2016; Balanzà et al., 2018). After floral transition, the FMs are produced and specified from the flanking of IM under the control of cascaded gene regulatory networks (GRNs) mediated by the FM identity genes, LEAFY (LFY) and APETALA1 (AP1) (Liu C. et al., 2009; Liu et al., 2013; Chandler, 2012). Subsequently, the FM identity genes, LFY and AP1, induce the expression of floral organ identity genes that act in a combinatorial manner, termed ABC model, to control floral organ specification at differently developmental stages (Smyth et al., 1990; Coen and Meyerowitz, 1991; Weigel and Meyerowitz, 1994). Briefly, A class genes specify sepals, and A together with B class genes specify petals, while B and C class genes act together leading to stamen development, and C function alone controls carpel formation. In addition, A and C class genes act in an antagonistic manner (Coen and Meyerowitz, 1991). At last, the formation of the innermost carpel primordia is followed by the genetically programmed termination of FMs, termed floral determinacy: it ensures the production of a fixed number of floral organs and subsequent gametogenesis.
Prolonged or enhanced FM activity results in an increased number of floral organs, increased whorls and even extra organs borne on supernumerary whorls due to prolonged stem cell activity (Lenhard et al., 2001; Prunet et al., 2009; Sun et al., 2009; Liu et al., 2011; Figure 1F). Genetic analysis showed that the termination of FM is not simple via the differentiation meristem cells into carpel cells since the mutants with 4th whorl carpels are still indeterminate with additional tissue growing inside (Sun et al., 2009; Ji et al., 2011; Liu et al., 2011). Moreover, unlike the GPA of SAM, the temporally precise regulation of the FM ensures that it is terminated at a particular developmental stage (e.g., stage 6 in Arabidopsis) under the control of complex GRNs (Cao et al., 2015; Xu et al., 2019). Therefore, the SAM maintenance and FM determinacy are two distinctly developmental processes.
Genetic Regulation of SAM Maintenance and FM Determinacy
Using forward genetics approaches, researchers have characterized numerous genes that are components of the regulatory networks that maintain SAM activity or terminate FM activity (Cao et al., 2015; Gaillochet and Lohmann, 2015; Soyars et al., 2016; Lee et al., 2019). Since meristems rely on stem cells as their source, FM have similar mechanisms to maintain the stem cell pool as other types of SAM. Similar to animal stem cells, plant stem cells are maintained by stem cell niches (Sablowski, 2004; Zheng and Liu, 2019). WUS encodes a homeodomain-containing transcription factor (TF) that is essential for the stem cells maintenance (Mayer et al., 1998; Figure 1D). In the wus mutant, the SAM fails to properly generate leaf primordia, and the FM is depleted before the production of carpel primordia, due to the rapid consumption of the stem cells contained therein (Laux et al., 1996). In contrast, ectopic WUS expression can endow somatic cells with stem cell properties (Zuo et al., 2002; Gallois et al., 2004; Xu et al., 2005). These findings demonstrate that WUS is critical for the establishment and maintenance of meristems. Further mechanistic analysis uncovered that WUS moves to the overlying stem cells in the CZ to directly induce CLV3, which encodes a secreted peptide (Yadav et al., 2011; Daum et al., 2014; Figure 1E). CLV3 subsequently binds to the plasma membrane-localized CLV1 or CLV2/CORYNE(CRN) receptor complex to inhibit WUS expression (Soyars et al., 2016). Thus, the WUS/CLV3 negative feedback loop fine-tunes the stem cell pool of the SAM and FM.
Dynamic SAM maintenance is determined by the rates of stem cell proliferation and organ primordia formation. The boundary cells express a set of distinctive TFs that play important roles to locally repress cell proliferation and cell division through crosstalk with the meristem genes and organ primordia specific genes (Heisler and Ohno, 2014; Zadnikova and Simon, 2014). To date, numbers of meristem-to-organ boundary-specific regulators are well studied including NAC family TFs such as CUP-SHAPED COTYLEDON1/2/3 (CUC1/2/3), MYC-domain TFs such as LATERAL ORGAN FUSION1/2 (LOF1/2), and LATERAL ORGAN BOUNDARIES DOMAIN (LBD) family TFs such as JAGGED LATERAL ORGANS (JLO) and LATERAL ORGAN BOUNDARIES (LOB) (Wang et al., 2016). They modulate the boundary establishment as well as organ primordia specification and meristem maintenance through genetic interaction with meristem-specific genes such as SHOOT MERISTEMLESS (STM) and WUS, and organ primordial-specific genes like ASYMMETRIC LEAVES1/2 (AS1/2) and TEOSINTE BRANCHED1/CYCLOIDEA/PCF1 (TCP) genes (Zadnikova and Simon, 2014). However, the molecular mechanisms by which these regulators control cell proliferation and differentiation in the boundaries are still not understood.
At the early stages of flower development, the maintenance and termination of FM activity are highly coordinated with the formation and specification of floral organ to ensure the successful flower development (Smyth et al., 1990; Alvarez-Buylla et al., 2010). Spatially expanded FM activity associated with an increased stem cell population leads to the production of extra floral organs. Temporally enhanced FM activity due to prolonged stem cell maintenance, known as FM indeterminacy, gives rise to supernumerary whorls with extra organs (Prunet et al., 2009; Figure 1F). The WUS/CLV feedback loop maintain the FM similarly to the way they maintain the SAM, but the regulatory loop alone is incompetent to precisely terminate the FM. AGAMOUS (AG), a C-class MADS domain-containing TF, is a central positive regulator of FM determinacy. The null ag mutant displays a flower-in-flower phenotype: the entire fourth whorl of the primary flower is replaced by a new flower bud that in turn produces a new abnormal flower due to the continuous maintenance of stem cells in the FM center (Bowman et al., 1989, 1991; Lenhard et al., 2001). This demonstrated that AG has dual-function in the flower development: floral organ (stamen and carpal) identity and FM termination. At stage 3 of floral development (Smyth et al., 1990), AG expression is induced by WUS and LFY at the center of the FM, and WUS expression is turned off at stage 6, resulting in FM determinacy (Lohmann et al., 2001). AG indirectly represses WUS expression through KNUCKLES (Sun et al., 2009, 2019), and AG also directly represses WUS through the TERMINAL FLOWER2 (TFL2)-AG complex that triggers chromatin loop formation at the WUS locus (Liu et al., 2011; Guo et al., 2018). As a central hub in this network, AG is regulated by numerous factors at the transcriptional, post-transcriptional and protein level through genetic and epigenetic mechanisms (Cao et al., 2015; Xu et al., 2019).
To date, many genes have been characterized in terms of their functions in either SAM maintenance or FM determinacy, while other genes have dual roles in both processes through their genetic interactions with different partners. The following section summarizes recent findings on these dual-function genes with different roles in SAM maintenance and FM determinacy.
Individual Factors Involved in Both SAM Maintenance and FM Determinacy
MicroRNA172 (miR172) – APETALA2 (AP2) Module
MicroRNAs (miRNAs) are a class of endogenous 20–24 nt non-coding RNAs. They are encoded by miRNA genes (MIR) and processed by DICER-LIKE (DCL) RNase III endonucleases (Margis et al., 2006; Nozawa et al., 2012). Through complementary base pairing, miRNAs guide post-transcriptional regulation of their targets, by either transcript cleavage or translational inhibition, and many of these targets are TFs (D’Ario et al., 2017; Yu et al., 2017b; Liu et al., 2018). Accordingly, miRNAs play dominant roles in plant development and growth. The five MIR172 genes in Arabidopsis produce three unique mature miR172 species that accumulate in different organs during plant development. When plants are transferred from short-day to long-day to induce flowering, miR172 abundance increases in the SAM (Wollmann et al., 2010), where SQUAMOSA PROMOTER BINDING PROTEIN-LIKE (SPL) proteins, the targets of miR156, promote the expression of miR172 (Wu et al., 2009). In turn, miR172 represses a family of AP2-like TFs, including APETALA2 (AP2), TARGET OF EAT 1 (TOE1), TOE2, TOE3, SCHLAFMÜTZE (SMZ), and SCHNARCHZAPFEN (SNZ), to regulate phase transitions and SAM activity (Aukerman and Sakai, 2003; Chen, 2004; Mathieu et al., 2009). It was well studied that the miR156/SPLs module controls the juvenile-to-adult transition by fine-tuning the miR172/AP2 module (Wang et al., 2009; Wu et al., 2009). At the same time, AP2 directly binds to individual MIR156 and MIR172 loci to promote MIR156 expression and repress MIR172 expression (Yant et al., 2010; Figure 2A). miR172 abundance is high during early floral development then gradually decreases from stage 3 onward. In these later stages, it is mainly detected in the fourth whorl, with the highest levels in the center of the FM, where it represses its target genes AP2 and TOE3 to maintain FM activity (Chen, 2004; Wollmann et al., 2010; Jung et al., 2014). Mutations in genes affecting miR172 biosynthesis or accumulation, such as HUA ENHANCER 1 (HEN1) and CARPEL FACTORY (CAF), result in increased AP2 protein levels and loss of FM termination (Jacobsen et al., 1999; Chen et al., 2002). Additionally, a mutation in POWERDRESS (PWR) could enhance the weakly indeterminate ag-10 allele. PWR promotes the expression of MIR172a, b, and c, but not MIR172d and e, while a mutation in mature miR172d could enhance the determinacy defects of ag-10 in an AP2-dependent manner, showing that the transcriptional diversification of the MIR172 family may make the floral determinacy regulatory network more robust (Yumul et al., 2013; Figure 2B).
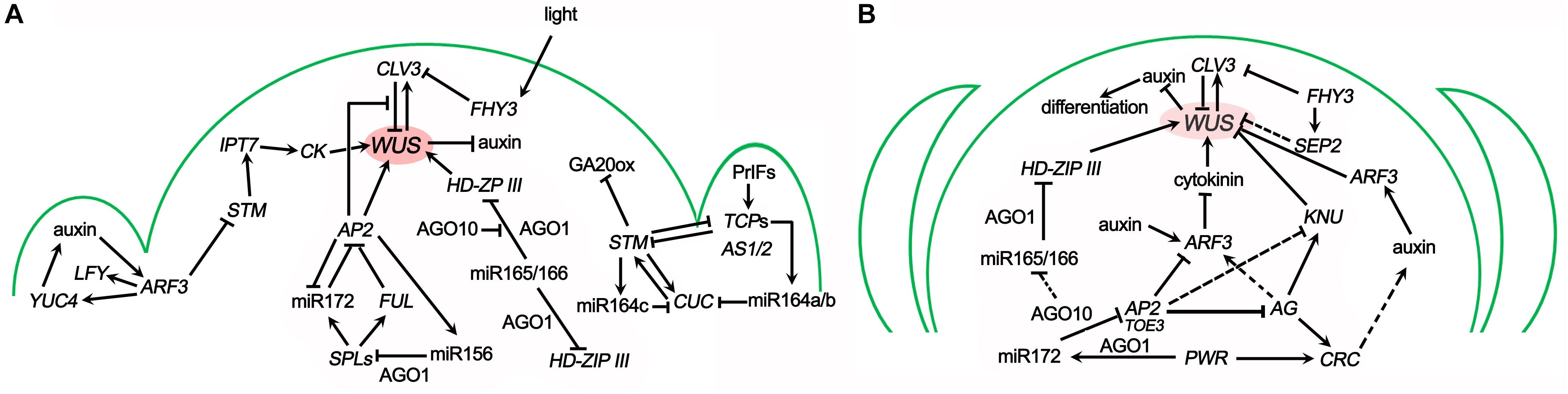
Figure 2. Genetic pathways in the SAM maintenance (A) and FM determinacy (B). The arrows represent the activation of gene expression, while the flat arrows represent its repression. The OC is shown in magenta. The genetic interactions are introduced in the text.
AP2 has numerous roles in floral transition, floral organ patterning, stem cell maintenance and seed development (Bowman et al., 1989; Ohto et al., 2005; Wurschum et al., 2006; Yant et al., 2010). At vegetative stage, AP2 is highly expressed in incipient leaf primordia, but its transcript levels are low in the SAM and the center of the FM after stage 2 (Wurschum et al., 2006; Wollmann et al., 2010). In early floral development, AP2 transcripts are concentrated in the sepal and petal primordia and partially overlap with MIR172 transcript in the third whorl. At later stages, AP2 is abundant in the developing petals, stamen filaments and the gynoecium, consistent with its multiple roles in floral development (Wollmann et al., 2010).
The semi-dominant I28 mutant, which harbors a dominant-negative AP2 allele, exhibits reduced SAM size, premature termination of the SAM and differentiation of the stem cells as in the wus mutant. At the early seedling stage, WUS and CLV3 expression are disrupted in the SAM of I28. Functional and genetic analysis revealed that AP2 promotes SAM maintenance either by repressing CLVs signaling or by promoting WUS expression independently of the AG pathway (Wurschum et al., 2006). Meanwhile, a recent study showed that the MADS-box gene FRUITFULL (FUL) promotes meristem arrest through direct AP2 repression. ful and ap2-170, in which the microRNA binding site of miR172 is mutated, have delayed coordinated arrest of all meristems, or GPA, that correlates with the repression of WUS expression. Induction of the miR172-resistant version of AP2, AP2170, in arrested plants reactivated the SAM and normal flower development, highlighting the important role of AP2 in SAM maintenance (Balanzà et al., 2018; Figure 2A). Since AP2 is clearly expressed in the emerging leaf primordia but hard to be detected in the SAM, how AP2 regulate WUS expression is unclear. A possibility is that AP2 could regulate WUS expression non-cell-autonomously.
In the ABC model of flower development (Weigel and Meyerowitz, 1994), AP2 functions as an A-class gene that acts antagonistically with AG, specifies the perianth organs and restricts AG expression to the inner two whorls (Drews et al., 1991). Specifically, AP2 directly binds to the second intron of AG to repress AG expression in the outer two whorls (Wollmann et al., 2010; Yant et al., 2010; Dinh et al., 2012). In the ag mutant, AP2 does not expand to the center of the FM, and miR172 accumulation is unaffected, indicating that AP2 is mainly regulated by miR172, however, another study showed that AG misexpression with the 35S promoter counteracted AP2 in the outer two whorls (Zhao et al., 2007; Wollmann et al., 2010). Transgenic lines expressing miR172-resistant versions of AP2 and TOE3 were found to exhibit floral organ identity defects and severe FM indeterminacy phenotypes (Zhao et al., 2007; Jung et al., 2014). In addition, AP2 physically interacts with TOE3 in vivo, and it inhibits both KNU and AG expression to promote WUS expression and FM activity (Zhao et al., 2007; Yant et al., 2010; Huang et al., 2017). At the same time, AP2 also directly represses the expression of AUXIN RESPONSE FACTOR3 (ARF3) to regulate WUS expression (see below) (Liu et al., 2014; Figure 2B).
Argonaute1 (AGO1) and Argonaute10 (AGO10)
Through sequence complementarity with their target transcripts, mature miRNAs that are loaded into the RNA-induced silencing complex (RISC) direct the repression of their targets (Rogers and Chen, 2013). The PAZ and PIWI domain–containing AGO proteins are the central component of the RISC complex. miRNAs are sorted into different AGO proteins according to their 5′-nucleotide (Mi et al., 2008). Of the 10 AGO proteins in Arabidopsis, AGO1 is required for the functions of most miRNAs, and AGO1 deficiency results in pleiotropic developmental phenotypes (Bohmert et al., 1998; Vaucheret et al., 2004; Mallory and Vaucheret, 2010). AGO10, also known as ZWILLE (ZLL)/PINHEAD (PNH), is the closet paralog of AGO1 and is specifically required for SAM maintenance (Moussian et al., 1998; Vaucheret, 2008). While AGO1 is ubiquitously expressed (Bohmert et al., 1998), AGO10 expression is restricted to the provasculature underneath the SAM and the adaxial side of lateral organ primordia, where AGO10 and AGO1 have overlapping functions (Moussian et al., 1998; Lynn et al., 1999). While ago1 mutants display pleiotropic defects of general plant architecture including single stem and rare axillary meristems (Bohmert et al., 1998), ago10 seedlings have an empty apex, a pinhead-like structure or a solitary leaf, and these mutant phenotypes clearly indicate the importance of AGO10 in SAM establishment and maintenance (Moussian et al., 1998; Tucker et al., 2008).
In the SAM, five class III homeodomain-leucine zipper (HD-ZIP III) TF genes are expressed: PHABULOSA (PHB), PHAVOLUTA (PHV), REVOLUTA (REV), CORONA (CNA), and ATHB8. These genes are targeted by miR165/166 and play important roles in organ polarity, SAM establishment and maintenance as well as FM termination. rev phb and rev phb phv mutants fail to establish a SAM and often produce single pin-like cotyledons, while gain-of-function phb-D and rev-D mutants have enlarged meristems (Talbert et al., 1995; Byrne, 2006). The transcripts of HD-ZIP III genes are targets of miR165/166 that is restricted to the abaxial side of organ primordia and excluded from the SAM (Liu Q. et al., 2009). miR165/166 overexpression dramatically reduces the transcript levels of all five HD-ZIP III genes, resulting in a range of phenotypes from an enlarged SAM to loss of the SAM (Jung and Park, 2007; Zhou et al., 2007). Thus, fine-tuning of the miR165/166–HD-ZIP III module by AGO1 and AGO10 is important for SAM maintenance. While miR165/166 are mainly loaded into AGO1 to repress HD-ZIP III gene expression and several genetic analyses showed that AGO1 and AGO10 have complementary functions in SAM maintenance (Tucker et al., 2008; Mallory et al., 2009), AGO10 misexpression leads to increased levels of HD-ZIP III mRNAs that are degraded by AGO1 (Liu Q. et al., 2009). Subsequently, the detailed biochemical studies revealed that AGO10 binds miR165/6 with higher affinity than AGO1 and promotes miR165/6 degradation in its restricted expressing tissue (Yu et al., 2017a). Thus, acting as a decoy AGO1 protein, AGO10 competitively binds miR165/166 to protect the HD-ZIP III transcripts from post-transcriptional regulation, thereby maintaining SAM activity, in line with the predominant distribution patterns of AGO10 in the SAM (Zhu et al., 2011; Aichinger et al., 2012; Zhang and Zhang, 2012).
Genetic analysis showed that AGO10 activity in the FM contrasts its positive role in SAM maintenance. Mutations in AGO1 and AGO10 enhance the FM indeterminacy of ag-10 indicate that AGO1 and AGO10 functional redundantly promote stem cell termination in the FM (Ji et al., 2011), and both AGO1 and AGO10 can bind miR172 and miR165/166 to regulate AP2 and HD-ZIP III gene expression, respectively. While AGO1 mainly mediates the activities of miR165/166, AGO10 sequesters miR165/6 and represses its accumulation to fine-tune HD-ZIP III gene expression (Yu et al., 2017a). Correspondingly, reduced HD-ZIP III expression and increased AP2 expression result in prolonged floral stem cell activity in the ago10 mutant (Ji et al., 2011). The expression of HD-Zip III genes needs to be precisely controlled to achieve successful FM determinacy since both of HD-ZIP III reduction by over-expression of miR165/166 and mis-expression of HD-Zip III overexpression by rendering them resistant to miR165/166 led to prolonged floral stem cell activity (Ji et al., 2011). Thus, both AGO1 and AGO10 are required for SAM establishment and maintenance as well as FM determinacy, with distinct roles in these processes (Figure 2).
Shoot Meristemless (STM)
STM, a class-I KNOX gene, encodes a mobile TALE homeodomain TF that is essential for SAM establishment and maintenance (Long et al., 1996). Unlike WUS, STM is expressed throughout the SAM, where it suppresses cell differentiation, but is down-regulated in incipient organ primordial (Scofield et al., 2018). Loss-of-function stm mutants have compromised SAM formation and defective SAM organization, as evidenced by the fused cotyledon phenotype, due to the rapid consumption of the entire SAM (Clark et al., 1996; Endrizzi et al., 1996).
Ectopic expression of STM at leaf primordia suppresses cell differentiation and maintains the potential to form additional lateral outgrowths (Lenhard et al., 2002). Genetic analysis has revealed that ectopic expression of STM and WUS could trigger ectopic organogenesis, while they function in different pathway in meristem regulation (Byrne et al., 2002; Lenhard et al., 2002). Although the stm and wus mutants display similar developmental defects of SAM organization, the meristem arrest phenotypes of them differ from each other, in which meristematic cells are consumed into developing organs in stm mutants but are retained in a non-meristematic state in wus (Endrizzi et al., 1996; Laux et al., 1996), indicating that STM function is required to prevent meristematic cells from adopting organ-specific cell fates, whereas WUS is critical for the stem cell pool maintenance.
In the SAM, STM represses the expression of one target gene encoding GA20 oxidase (G20ox) enzyme that is required for phytohormone giberellic acid (GA) biosynthesis, to maintain low level of GA that stimulates growth by promote cell expansion. Exogenous GA treatment and constitutive GA signaling suppress STM gain-of-function phenotypes, whilst constitutive GA signaling mutant enhances the defects of weak stm mutants (Hay et al., 2002). At the same time, over-expression of cytokinin (CK), another phytohormone, biosynthetic ISOPENTENYL TRANSFERASE (IPT) genes and the exogenous application of CK can partially rescue the meristem defects of stm mutants, indicating that CK mediates the function of STM on meristem regulation. Further study showed that STM promotes IPT7 expression to increase CK activity in SAM, which contributes to the homeostasis of CK and WUS expression (see below) (Leibfried et al., 2005; Yanai et al., 2005). Therefore, STM may functions on both SAM organization and stem cell maintenance.
At the boundary zone, CUC1 is required for the boundary specification and restricted to express at boundary (Aida et al., 1999). STM binds and activates CUC1 expression, and CUC1 can directly activate STM expression to comprise a direct positive-feedback loop, which is attenuated by STM-induced miR164c (Hibara et al., 2003; Spinelli et al., 2011; Scofield et al., 2018). In the regulatory interactions, the movement of STM is important for the meristem function and the correct expression patterns of CUC1 and CUC2 at the boundary zone (Lucas et al., 1995; Kim et al., 2003; Balkunde et al., 2017). In the organ primordia, primordium identity factors (PrIFs) specify primordium identity and promote expression of TCP family genes, which repress the expression of KNOX genes in primordia by direct interaction with primordium-specific AS1/AS2 complex and CUC1 expression through miR164a/b, whilst STM represses T in the SAM (Byrne et al., 2002; Li et al., 2012; Scofield et al., 2018; Figure 2A). Therefore, the genetic interactions among these genes ensure the precise gene expression pattern and the boundary formation.
During flower development, STM is not expressed in FM founder cells or incipient FMs at the flanks of the SAM. However, STM is reactivated expression throughout the apical region of the FM proper but not in the basal domain that corresponds to the cryptic bract prior to floral patterning, shortly after the FM becomes distinct from the SAM, and then restricted to whorl 4 at late stages (Long and Barton, 2000). Ectopic expression of STM results in the formation of ectopic carpels, carpelloid organs and the conversion of ovules to carpels (Scofield et al., 2007). In the mild stm-2 mutant, the SAM terminates in flowers that lack a central gynoecium (Clark et al., 1996; Scofield et al., 2007). These findings indicated that STM is required for whorl 4 and/or carpel development in FMs. Recent study showed that STM is also required for the FM competence. Genetic analysis has revealed that STM and UNUSUAL FLORAL ORGANS (UFO), but in depend of AP1, genetically interact to specify FM identity and initiate the floral program by regulation of flower identity genes (Roth et al., 2018). These findings demonstrated the multiple-functions of STM on organ identity and meristem activity.
Auxin Response Factor3 (ARF3)
The phytohormone auxin and CK are critical for many plant growth and developmental processes including establishment, maintenance and termination of meristem (Schaller et al., 2015). Auxin is biosynthesized by YUCCA gene family and its signaling is mediated by two protein families: auxin response factors (ARFs) and Aux/IAA proteins, which induce global auxin response by regulate the expression of target genes (Reinhardt et al., 2000; Liscum and Reed, 2002; Cheng et al., 2006; Guilfoyle and Hagen, 2007; Vanneste and Friml, 2009). CK biosynthesis depends on the IPT gene family and LONELY GUY (LOG) gene family, respectively. After perceived by its receptors ARABIDOPSIS HISTIDINE KINASE2/3/4 (AHK2/3/4), CKs trigger the CK transcriptional response through B-type ARABIDOPSIS RESPONSEREGULATORs (ARRs) that are the TFs activated through phosphorylation by CK signaling, while A-types ARRs are negative regulators of CK signaling whose expression is induced by CK (Kieber and Schaller, 2014). In the SAM, auxin maxima are found at locations of primordia formation where it induces cellular differentiation and organ outgrowth, while CK maximum is found at the OC to promote the proliferation of undifferentiated cells (Schaller et al., 2015). CK is required for the activation of WUS expression in an AHK2/AHK4-dependent manner, while WUS represses the expression of several A-type ARR genes, such as ARR5/7/15, resulting in increased CK activity in the OC (Leibfried et al., 2005; Gordon et al., 2009; Zhao et al., 2010). ARF5/MONOPTEROS (MP) mediates the crosstalk between auxin and CK signaling required for SAM maintenance. Specifically, auxin induces the expression of ARF5/MP to repress ARR7/15 to fine tune CK activity and WUS expression (Zhao et al., 2010). Recent study showed that WUS, in turn, maintains low auxin signaling output in stem cells by reducing target genes expression through regulating the histone acetylation status of target loci (Ma et al., 2019). Thus, WUS keeps stem cell pool from auxin induced differentiation, while enhancing CK activity to sustain its expression.
ARF3, also known as ETTIN, is one of the 23 ARF family members in Arabidopsis (Guilfoyle and Hagen, 2007). ARF3 has numerous roles in plant development, including gynoecium morphogenesis, de novo organ regeneration, organ polarity and FM determinacy (Sessions et al., 1997; Nemhauser et al., 2000; Cheng et al., 2013; Liu et al., 2014). During de novo organ regeneration, ARF3 is highly expressed in the emerging SAM at early stage of SAM formation, but is ubiquitously expressed in the SAM at later stage of SAM formation, where ARF3 mediates the auxin response and directly represses the expression of the CK biosynthesis gene AtIPT5. Mutations in ARF3 lead to ectopic CK biosynthesis as well as disrupted stem cell initiation and meristem formation (Cheng et al., 2013). ARF3 protein is evenly distributed throughout the SAM and early FM, while ARF3 mRNA is abaxially distributed in the SAM and floral organ primordia (Liu et al., 2014; Simonini et al., 2017). Thus, the dynamic ARF3 distribution is required for its function on SAM formation and maintenance. Genome-wide analysis revealed that ARF3 interacts with its partners in an auxin-dependent manner that determines its repressor or activator roles (Simonini et al., 2017). At the flanking regions of the SAM, ARF3 may directly activate the expression of LFY to specified floral primordium fate, and YUC4 to induce auxin biosynthesis, and then promote cell differentiation (Schultz and Haughn, 1991; Cheng et al., 2006; Simonini et al., 2017). Simultaneously, ARF3 physically interacts with FIL to directly repress STM expression, and the resulting histone deacetylation promotes organogenesis (Chung et al., 2019). In addition, given that STM promote CK activity, ARF3 could fine-tune CK activity in SAM (Figure 2A).
Unlike the even distribution pattern of ARF3 observed in the SAM, ARF3 is concentrated in the OC of the FM. It overlaps with WUS and the CK receptor gene AHK4, indicating different roles of ARF3 in the FM and SAM (Liu et al., 2014). Genetic analysis showed that ARF3 promotes FM determinacy by repressing WUS expression. In this context, ARF3 is repressed by AP2 to mediate the functions of AP2 and AG in FM maintenance and termination (Liu et al., 2014). Detailed analysis of the underlying molecular mechanism revealed that during early FM development (stages 3–5), AG transiently represses ARF3 expression to de-repress the expression of IPT3/5/7 and cell cycle genes, which helps to maintain FM activity. At later FM stages (stages 5–6), AG and auxin increase ARF3 expression, while AP2 represses ARF3 expression. ARF3 directly inhibits IPT3/5/7 and AHK4 expression and indirectly inhibits the expression of LOG genes. This regulation by ARF3 represses CK activity in the OC and ensures proper temporal termination of WUS expression (Zhang et al., 2018). Moreover, ARF3 can bind to the WUS promotor in an AG-dependent manner to fine-tune WUS expression (Liu et al., 2014; Figure 2B). Recent studies showed that fine-tuning of auxin homeostasis is required for the FM determinacy and gynoecium formation. Locally increased auxin production rescued the FM indeterminacy phenotype of knu crc (crabs claw), which is supposed to be mediated by ARF3 (Yamaguchi et al., 2017, 2018).
FAR-RED Elongated Hypocotyl3 (FHY3)
Coordination of internal developmental cues, nutrients, hormones, and external environmental signals is important for the meristem maintenance and organogenesis in shoots and roots (Li et al., 2017). Light is one of the most important environmental signals for plant development and growth (Arsovski et al., 2012). In addition, light activates photosynthesis to provide energy by sucrose production. Recent studies found that Glucose energy signaling is essential to activate SAM and RM activity through activating target of rapamycin (TOR) kinase, while light induces auxin synthesis to promote SAM activity (Pfeiffer et al., 2016; Li et al., 2017).
At same time, plants have evolved a family of photoreceptors to perceive the light signal. Phytochrome A (phyA) is a key member with both specific and shared functions (Li et al., 2011). Light exposure triggers the transformation of phyA from the inactive Pr form to the active Pfr form, and it translocates into the nucleus with the help of FAR-RED ELONGATED HYPOCOTYL1 (FHY1) and its homolog FHY1-LIKE (FHL) (Casal et al., 2014). In Arabidopsis, FAR-RED ELONGATED HYPOCOTYLS3 (FHY3) directly actives the expression of FHY1 and FHL to promote phyA signaling (Lin et al., 2007). Additionally, FHY3 plays diverse roles in different plant developmental and physiological processes, such as circadian signaling, chloroplast biogenesis, chlorophyll biosynthesis and programmed cell death (Wang and Wang, 2015). Genome-wide gene expression profiling showed that under far-red (FR) light conditions, FHY3 mainly acts as a transcriptional activator to promote photomorphogenesis during the vegetative stage (Ouyang et al., 2011), but it acts primarily as transcriptional repressor in flower development (Li et al., 2016).
A genetic analysis uncovered the dual roles of FHY3 in SAM maintenance and FM determinacy (Li et al., 2016). Specifically, a mutation in FHY3 led to a smaller SAM size, and fhy3 enhanced the indeterminacy of ag-10, a weak ag allele. Additionally, wus was found to be epistatic to fhy3. Molecular analysis revealed that FHY3 directly represses CLV3 expression to regulate WUS expression in the SAM. When seedlings transition from dark to light conditions, CLV3 expression decreases in WT plants but not in the fhy3 and phyA mutants, indicating that FHY3 mediates the repressive effect of light on CLV3 expression (Li et al., 2016). The de-repressed expression of CLV3 in the fhy3 mutant results in reduced WUS expression and a small SAM size. On the other hand, during flower development, FHY3 directly actives SEPALLATA1 (SEP1) and SEP2 expression, in parallel to the FHY3-CLV3 pathway, to repress WUS expression and to promote FM determinacy (Li et al., 2016; Figure 2).
Conclusion and Future Perspectives
All postembryonic organs in plants develop from the stem cells that reside in the meristems. A key similarity between animal and plant stem cells is that the stem cell niche is critical for the maintenance of their activity (Heidstra and Sabatini, 2014; Zheng and Liu, 2019). The WUS homeobox (HB) TF belongs to the plant-specific WUS homeobox (WOX) protein family, one of a number of plant HB TF families that are characterized by the presence of a homeodomain. More broadly, this DNA-binding domain is important for developmental decisions in eukaryotes (van der Graaff et al., 2009). Phylogenetic and functional analyses have shown that WOX genes are conserved in euphyllophytes with distinct functions in a wide range of processes, particularly in the establishment, maintenance and termination of different types of meristems (Dodsworth, 2009; van der Graaff et al., 2009; Zhang et al., 2010; Costanzo et al., 2014; Liu and Xu, 2018). WUS homologs with conserved functions among angiosperms act in diverse and intricate regulatory networks (Dodsworth, 2009) in which some key players have dual roles in SAM maintenance and FM termination through their interactions with different partners (this review). However, there are many unanswered questions about how WOX members have come to function in diverse developmental processes over the course of evolution.
Poaceae (also called grasses) is one of the largest families of angiosperms containing many agriculturally important crops, such as rice, wheat, barley and maize (Grass Phylogeny Working Group et al., 2001). All grasses have a complex inflorescence composed of one, a few, or many spikelets produced by different type meristems. The IM of Arabidopsis is indeterminate and form two types meristems: indeterminate branch meristem and determinate FM. While the IM of wheat is determinate but the IMs of rice and maize are indeterminate, they produce determinate FMs (Zhang and Yuan, 2014). Therefore, the WOX genes mediated GRNs that are composed of homologs of key players in different species have distinct functions on meristem maintenance and FM determinacy through different molecular mechanisms. Phylogenetic analysis indicated, for example, that FHY3-like genes, encoding Mutator-like transposase-derived TFs, are widespread in angiosperms but not in other organisms (Lin et al., 2007), indicating that FHY3 is probably linked to the adaptive evolution of phyA (Mathews et al., 2003). Interestingly, although close orthologs of FHY3 are widespread in dicots, they are missing in monocot genomes (Lin et al., 2007). Thus, moving forward, comparative analyses of the functions of meristem-related genes in diverse plant species, particularly genes involved in SAM establishment and maintenance as well as FM determinacy, will be critical for an improved understanding of meristem evolution and conductive to agricultural production.
Author Contributions
WC and YG contributed equally to the composition of the manuscript. XL and LG contributed equally to the conceptualization of the manuscript. HZ prepared the manuscript figures.
Funding
This work was supported by National Key Special Programs for Breeding from the Ministry of Science and Technology (2016YFD0100401); the National Science Foundation of China (NSFC) (31970824); the Guangdong Provincial Key Laboratory for Plant Epigenetics (College of Life Sciences and Oceanography, Shenzhen University); and the State Key Laboratory of Plant Cell and Chromosome Engineering (PCCE-KF-2018-04).
Conflict of Interest
The authors declare that the research was conducted in the absence of any commercial or financial relationships that could be construed as a potential conflict of interest.
Acknowledgments
We thank Rae Eden Yumul for valuable advice and language editing. We apologize to those authors whose work could not be cited due to space limitations.
References
Aichinger, E., Kornet, N., Friedrich, T., and Laux, T. (2012). Plant stem cell niches. Annu. Rev. Plant Biol. 63, 615–636. doi: 10.1146/annurev-arplant-042811-105555
Aida, M., Ishida, T., and Tasaka, M. (1999). Shoot apical meristem and cotyledon formation during arabidopsis embryogenesis: interaction among the cup-shaped cotyledon and shoot meristemless genes. Development 126, 1563–1570.
Aida, M., and Tasaka, M. (2006). Morphogenesis and patterning at the organ boundaries in the higher plant shoot apex. Plant Mol. Biol. 60, 915–928. doi: 10.1007/s11103-005-2760-7
Alvarez-Buylla, E. R., Benitez, M., Corvera-Poire, A., Chaos Cador, A., de Folter, S., Gamboa de Buen, A.,, et al. (2010). Flower development. Arabidopsis Book 8:e0127.
Arsovski, A. A., Galstyan, A., Guseman, J. M., and Nemhauser, J. L. (2012). Photomorphogenesis. Arabidopsis Book 10:e0147.
Aukerman, M. J., and Sakai, H. (2003). Regulation of flowering time and floral organ identity by a microrna and its apetala2-like target genes. Plant Cell 15, 2730–2741. doi: 10.1105/tpc.016238
Balanzà, V., Martínez-Fernández, I., Sato, S., Yanofsky, M. F., Kaufmann, K., Angenent, G. C., et al. (2018). Genetic control of meristem arrest and life span in arabidopsis by a fruitfull-apetala2 pathway. Nat. Commun. 9:565. doi: 10.1038/s41467-018-03067-5
Balkunde, R., Kitagawa, M., Xu, X. M., Wang, J., and Jackson, D. (2017). Shoot meristemless trafficking controls axillary meristem formation, meristem size and organ boundaries in arabidopsis. Plant J. 90, 435–446. doi: 10.1111/tpj.13504
Bishopp, A., and Bennett, M. J. (2014). Hormone crosstalk: directing the flow. Curr. Biol. 24, R366–R368. doi: 10.1016/j.cub.2014.03.018
Bohmert, K., Camus, I., Bellini, C., Bouchez, D., Caboche, M., and Benning, C. (1998). Ago1 defines a novel locus of arabidopsis controlling leaf development. EMBO J. 17, 170–180. doi: 10.1093/emboj/17.1.170
Bowman, J. L., Smyth, D. R., and Meyerowitz, E. M. (1989). Genes directing flower development in arabidopsis. Plant Cell 1, 37–52. doi: 10.1105/tpc.1.1.37
Bowman, J. L., Smyth, D. R., and Meyerowitz, E. M. (1991). Genetic interactions among floral homeotic genes of arabidopsis. Development 112, 1–20.
Breuil-Broyer, S., Morel, P., de Almeida-Engler, J., Coustham, V., Negrutiu, I., and Trehin, C. (2004). High-resolution boundary analysis during Arabidopsis thaliana flower development. Plant J. 38, 182–192.
Byrne, M. E. (2006). Shoot meristem function and leaf polarity: the role of class III hd-zip genes. PLoS Genet. 2:e89. doi: 10.1371/journal.pgen.0020089
Byrne, M. E., Simorowski, J., and Martienssen, R. A. (2002). ASYMMETRIC LEAVES1 reveals knox gene redundancy in Arabidopsis. Development 129, 1957–1965.
Cao, X., He, Z., Guo, L., and Liu, X. (2015). Epigenetic mechanisms are critical for the regulation of wuschel expression in floral meristems. Plant Physiol. 168, 1189–1196. doi: 10.1104/pp.15.00230
Carles, C. C., and Fletcher, J. C. (2003). Shoot apical meristem maintenance: the art of a dynamic balance. Trends Plant Sci. 8, 394–401. doi: 10.1016/s1360-1385(03)00164-x
Casal, J. J., Candia, A. N., and Sellaro, R. (2014). Light perception and signalling by phytochrome a. J. Exp. Bot. 65, 2835–2845. doi: 10.1093/jxb/ert379
Chandler, J. W. (2012). Floral meristem initiation and emergence in plants. Cell. Mol. Life Sci. 69, 3807–3818. doi: 10.1007/s00018-012-0999-0
Chen, X., Liu, J., Cheng, Y., and Jia, D. (2002). HEN1 functions pleiotropically in Arabidopsis development and acts in c function in the flower. Development 129, 1085–1094.
Chen, X. M. (2004). A microrna as a translational repressor of apetala2 in arabidopsis flower development. Science 303, 2022–2025. doi: 10.1126/science.1088060
Cheng, Y., Dai, X., and Zhao, Y. (2006). Auxin biosynthesis by the yucca flavin monooxygenases controls the formation of floral organs and vascular tissues in Arabidopsis. Genes Dev. 20, 1790–1799. doi: 10.1101/gad.1415106
Cheng, Z. J., Wang, L., Sun, W., Zhang, Y., Zhou, C., Su, Y. H.,, et al. (2013). Pattern of auxin and cytokinin responses for shoot meristem induction results from the regulation of cytokinin biosynthesis by auxin response factor3. Plant Physiol. 161, 240–251. doi: 10.1104/pp.112.203166
Chung, Y., Zhu, Y., Wu, M. F., Simonini, S., Kuhn, A., Armenta-Medina, A.,, et al. (2019). Auxin response factors promote organogenesis by chromatin-mediated repression of the pluripotency gene shootmeristemless. Nat. Commun. 10:886. doi: 10.1038/s41467-019-08861-3
Clark, S. E., Jacobsen, S. E., Levin, J. Z., and Meyerowitz, E. M. (1996). The clavata and shoot meristemless loci competitively regulate meristem activity in Arabidopsis. Development 122, 1567–1575.
Coen, E. S., and Meyerowitz, E. M. (1991). The war of the whorls: genetic interactions controlling flower development. Nature 353, 31–37. doi: 10.1038/353031a0
Costanzo, E., Trehin, C., and Vandenbussche, M. (2014). The role of wox genes in flower development. Ann. Bot. 114, 1545–1553. doi: 10.1093/aob/mcu123
D’Ario, M., Griffiths-Jones, S., and Kim, M. (2017). Small rnas: big impact on plant development. Trends Plant Sci. 22, 1056–1068. doi: 10.1016/j.tplants.2017.09.009
Daum, G., Medzihradszky, A., Suzaki, T., and Lohmann, J. U. (2014). A mechanistic framework for noncell autonomous stem cell induction in Arabidopsis. Proc. Natl. Acad. Sci. U.S.A. 111, 14619–14624. doi: 10.1073/pnas.1406446111
Dinh, T. T., Girke, T., Liu, X., Yant, L., Schmid, M., and Chen, X. (2012). The floral homeotic protein apetala2 recognizes and acts through an at-rich sequence element. Development 139, 1978–1986. doi: 10.1242/dev.077073
Dodsworth, S. (2009). A diverse and intricate signalling network regulates stem cell fate in the shoot apical meristem. Dev. Biol. 336, 1–9. doi: 10.1016/j.ydbio.2009.09.031
Drews, G. N., Bowman, J. L., and Meyerowitz, E. M. (1991). Negative regulation of the arabidopsis homeotic gene agamous by the apetala2 product. Cell 65, 991–1002. doi: 10.1016/0092-8674(91)90551-9
Endrizzi, K., Moussian, B., Haecker, A., Levin, J. Z., and Laux, T. (1996). The SHOOT MERISTEMLESS gene is required for maintenance of undifferentiated cells in Arabidopsis shoot and floral meristems and acts at a different regulatory level than the meristem genes WUSCHEL and ZWILLE. Plant J. 10, 967–979. doi: 10.1046/j.1365-313x.1996.10060967.x
Gaillochet, C., and Lohmann, J. U. (2015). The never-ending story: from pluripotency to plant developmental plasticity. Development 142, 2237–2249. doi: 10.1242/dev.117614
Gallois, J. L., Nora, F. R., Mizukami, Y., and Sablowski, R. (2004). Wuschel induces shoot stem cell activity and developmental plasticity in the root meristem. Genes Dev. 18, 375–380. doi: 10.1101/gad.291204
Gordon, S. P., Chickarmane, V. S., Ohno, C., and Meyerowitz, E. M. (2009). Multiple feedback loops through cytokinin signaling control stem cell number within the Arabidopsis shoot meristem. Proc. Natl. Acad. Sci. U.S.A. 106, 16529–16534. doi: 10.1073/pnas.0908122106
Grass Phylogeny Working Group, Barker, N. P., Clark, L. G., Davis, J. I., Duvall, M. R., Guala, G. F.,, et al. (2001). Phylogeny and subfamilial classification of the grasses (poaceae). Ann. Mo. Bot. Gard. 88, 373–457.
Groß-Hardt, R., and Laux, T. (2003). Stem cell regulation in the shoot meristem. J. Cell Sci. 116, 1659–1666. doi: 10.1242/jcs.00406
Guilfoyle, T. J., and Hagen, G. (2007). Auxin response factors. Curr. Opin. Plant Biol. 10, 453–460.
Guo, L., Cao, X., Liu, Y., Li, J., Li, Y., Li, D.,, et al. (2018). A chromatin loop represses WUSCHEL expression in Arabidopsis. Plant J. 94, 1083–1097. doi: 10.1111/tpj.13921
Hay, A., Kaur, H., Phillips, A., Hedden, P., Hake, S., and Tsiantis, M. (2002). The gibberellin pathway mediates knotted1-type homeobox function in plants with different body plans. Curr. Biol. 12, 1557–1565. doi: 10.1016/s0960-9822(02)01125-9
Heidstra, R., and Sabatini, S. (2014). Plant and animal stem cells: similar yet different. Nat. Rev. Mol. Cell Biol. 15, 301–312. doi: 10.1038/nrm3790
Heisler, M. G., and Ohno, C. (2014). Live-imaging of the Arabidopsis inflorescence meristem. Methods Mol. Biol. 1110, 431–440. doi: 10.1007/978-1-4614-9408-9_25
Hensel, L. L., Nelson, M. A., Richmond, T. A., and Bleecker, A. B. (1994). The fate of inflorescence meristems is controlled by developing fruits in Arabidopsis. Plant Physiol. 106, 863–876. doi: 10.1104/pp.106.3.863
Hibara, K., Takada, S., and Tasaka, M. (2003). Cuc1 gene activates the expression of sam-related genes to induce adventitious shoot formation. Plant J. 36, 687–696. doi: 10.1046/j.1365-313x.2003.01911.x
Huang, Z. G., Shi, T., Zheng, B. L., Yumul, R. E., Liu, X. G., You, C. J.,, et al. (2017). Apetala2 antagonizes the transcriptional activity of agamous in regulating floral stem cells in Arabidopsis thaliana. New Phytol. 215, 1197–1209. doi: 10.1111/nph.14151
Jacobsen, S. E., Running, M. P., and Meyerowitz, E. M. (1999). Disruption of an rna helicase/rnase III gene in Arabidopsis causes unregulated cell division in floral meristems. Development 126, 5231–5243.
Ji, L. J., Liu, X. G., Yan, J., Wang, W. M., Yumul, R. E., Kim, Y. J.,, et al. (2011). ARGONAUTE10 and ARGONAUTE1 regulate the termination of floral stem cells through two micrornas in Arabidopsis. PLoS Genet. 7:e1001358.
Jung, J. H., Lee, S., Yun, J., Lee, M., and Park, C. M. (2014). The mir172 target toe3 represses AGAMOUS expression during Arabidopsis floral patterning. Plant Sci. 215–216, 29–38. doi: 10.1016/j.plantsci.2013.10.010
Jung, J. H., and Park, C. M. (2007). MIR166/165 genes exhibit dynamic expression patterns in regulating shoot apical meristem and floral development in Arabidopsis. Planta 225, 1327–1338. doi: 10.1007/s00425-006-0439-1
Jürgens, G. (2001). Apical–basal pattern formation in Arabidopsis embryogenesis. EMBO J. 20, 3609–3616. doi: 10.1093/emboj/20.14.3609
Kaufmann, K., Pajoro, A., and Angenent, G. C. (2010). Regulation of transcription in plants: mechanisms controlling developmental switches. Nat. Rev. Genet. 11, 830–842. doi: 10.1038/nrg2885
Kieber, J. J., and Schaller, G. E. (2014). Cytokinins. Arabidopsis Book 12:e0168. doi: 10.1199/tab.0168
Kim, J. Y., Yuan, Z., and Jackson, D. (2003). Developmental regulation and significance of knox protein trafficking in Arabidopsis. Development 130, 4351–4362. doi: 10.1242/dev.00618
Lau, S., Slane, D., Herud, O., Kong, J., and Jürgens, G. (2012). Early embryogenesis in flowering plants: setting up the basic body pattern. Annu. Rev. Plant Biol. 63, 483–506. doi: 10.1146/annurev-arplant-042811-105507
Laufs, P., Peaucelle, A., Morin, H., and Traas, J. (2004). Microrna regulation of the cuc genes is required for boundary size control in Arabidopsis meristems. Development 131, 4311–4322. doi: 10.1242/dev.01320
Laux, T. (2003). The stem cell concept in plants: a matter of debate. Cell 113, 281–283. doi: 10.1016/s0092-8674(03)00312-x
Laux, T., Mayer, K. F. X., Berger, J., and Jurgens, G. (1996). The wuschel gene is required for shoot and floral meristem integrity in Arabidopsis. Development 122, 87–96.
Lee, Z. H., Hirakawa, T., Yamaguchi, N., and Ito, T. (2019). The roles of plant hormones and their interactions with regulatory genes in determining meristem activity. Int. J. Mol. Sci. 20:4065. doi: 10.3390/ijms20164065
Leibfried, A., To, J. P., Busch, W., Stehling, S., Kehle, A., Demar, M.,, et al. (2005). Wuschel controls meristem function by direct regulation of cytokinin-inducible response regulators. Nature 438, 1172–1175. doi: 10.1038/nature04270
Lenhard, M., Bohnert, A., Jurgens, G., and Laux, T. (2001). Termination of stem cell maintenance in Arabidopsis floral meristems by interactions between WUSCHEL and AGAMOUS. Cell 105, 805–814. doi: 10.1016/s0092-8674(01)00390-7
Lenhard, M., Jürgens, G., and Laux, T. (2002). The WUSCHEL and SHOOTMERISTEMLESS genes fulfil complementary roles in Arabidopsis shoot meristem regulation. Development 129, 3195–3206.
Li, D., Fu, X., Guo, L., Huang, Z., Li, Y., Liu, Y.,, et al. (2016). FAR-RED ELONGATED HYPOCOTYL3 activates SEPALLATA2 but inhibits CLAVATA3 to regulate meristem determinacy and maintenance in Arabidopsis. Proc. Natl. Acad. Sci. U.S.A. 113, 9375–9380. doi: 10.1073/pnas.1602960113
Li, J., Li, G., Wang, H., and Wang Deng, X. (2011). Phytochrome signaling mechanisms. Arabidopsis Book 9:e0148. doi: 10.1199/tab.0148
Li, X., Cai, W., Liu, Y., Li, H., Fu, L., Liu, Z.,, et al. (2017). Differential tor activation and cell proliferation in Arabidopsis root and shoot apexes. Proc. Natl. Acad. Sci. U.S.A. 114, 2765–2770.
Li, Z., Li, B., Shen, W. H., Huang, H., and Dong, A. (2012). Tcp transcription factors interact with as2 in the repression of class-i knox genes in Arabidopsis thaliana. Plant J. 71, 99–107. doi: 10.1111/j.1365-313X.2012.04973.x
Lin, R., Ding, L., Casola, C., Ripoll, D. R., Feschotte, C., and Wang, H. (2007). Transposase-derived transcription factors regulate light signaling in Arabidopsis. Science 318, 1302–1305. doi: 10.1126/science.1146281
Liscum, E., and Reed, J. W. (2002). Genetics of aux/iaa and arf action in plant growth and development. Plant Mol. Biol. 49, 387–400. doi: 10.1007/978-94-010-0377-3_10
Liu, C., Teo, Z. W., Bi, Y., Song, S., Xi, W., Yang, X.,, et al. (2013). A conserved genetic pathway determines inflorescence architecture in arabidopsis and rice. Dev. Cell 24, 612–622. doi: 10.1016/j.devcel.2013.02.013
Liu, C., Xi, W., Shen, L., Tan, C., and Yu, H. (2009). Regulation of floral patterning by flowering time genes. Dev. Cell 16, 711–722. doi: 10.1016/j.devcel.2009.03.011
Liu, H., Yu, H., Tang, G., and Huang, T. (2018). Small but powerful: function of micrornas in plant development. Plant Cell Rep. 37, 515–528. doi: 10.1007/s00299-017-2246-5
Liu, Q., Yao, X., Pi, L., Wang, H., Cui, X., and Huang, H. (2009). The ARGONAUTE10 gene modulates shoot apical meristem maintenance and establishment of leaf polarity by repressing mir165/166 in Arabidopsis. Plant J. 58, 27–40. doi: 10.1111/j.1365-313X.2008.03757.x
Liu, W., and Xu, L. (2018). Recruitment of ic-wox genes in root evolution. Trends Plant Sci. 23, 490–496. doi: 10.1016/j.tplants.2018.03.011
Liu, X., Dinh, T. T., Li, D., Shi, B., Li, Y., Cao, X.,, et al. (2014). Auxin response factor 3 integrates the functions of agamous and apetala2 in floral meristem determinacy. Plant J. 80, 629–641. doi: 10.1111/tpj.12658
Liu, X. G., Kim, Y. J., Muller, R., Yumul, R. E., Liu, C. Y., Pan, Y. Y.,, et al. (2011). Agamous terminates floral stem cell maintenance in arabidopsis by directly repressing wuschel through recruitment of polycomb group proteins. Plant Cell 23, 3654–3670. doi: 10.1105/tpc.111.091538
Lohmann, J. U., Hong, R. L., Hobe, M., Busch, M. A., Parcy, F., Simon, R., et al. (2001). A molecular link between stem cell regulation and floral patterning in Arabidopsis. Cell 105, 793–803. doi: 10.1016/s0092-8674(01)00384-1
Long, J., and Barton, M. K. (2000). Initiation of axillary and floral meristems in Arabidopsis. Dev. Biol. 218, 341–353. doi: 10.1006/dbio.1999.9572
Long, J. A., Moan, E. I., Medford, J. I., and Barton, M. K. (1996). A member of the knotted class of homeodomain proteins encoded by the STM gene of Arabidopsis. Nature 379, 66–69. doi: 10.1038/379066a0
Lucas, W. J., Bouche-Pillon, S., Jackson, D. P., Nguyen, L., Baker, L., Ding, B.,, et al. (1995). Selective trafficking of knotted1 homeodomain protein and its mrna through plasmodesmata. Science 270, 1980–1983. doi: 10.1126/science.270.5244.1980
Lynn, K., Fernandez, A., Aida, M., Sedbrook, J., Tasaka, M., Masson, P.,, et al. (1999). The pinhead/zwille gene acts pleiotropically in Arabidopsis development and has overlapping functions with the argonaute1 gene. Development 126, 469–481.
Ma, Y., Miotk, A., Šutiković, Z., Ermakova, O., Wenzl, C., Medzihradszky, A.,, et al. (2019). Wuschel acts as an auxin response rheostat to maintain apical stem cells in Arabidopsis. Nat. Commun. 10:5093. doi: 10.1038/s41467-019-13074-9
Mallory, A., and Vaucheret, H. (2010). Form, function, and regulation of argonaute proteins. Plant Cell 22, 3879–3889. doi: 10.1105/tpc.110.080671
Mallory, A. C., Hinze, A., Tucker, M. R., Bouche, N., Gasciolli, V., Elmayan, T.,, et al. (2009). Redundant and specific roles of the argonaute proteins ago1 and zll in development and small rna-directed gene silencing. PLoS Genet. 5:e1000646. doi: 10.1371/journal.pgen.1000646
Margis, R., Fusaro, A. F., Smith, N. A., Curtin, S. J., Watson, J. M., Finnegan, E. J.,, et al. (2006). The evolution and diversification of dicers in plants. FEBS Lett. 580, 2442–2450. doi: 10.1016/j.febslet.2006.03.072
Mathews, S., Burleigh, J. G., and Donoghue, M. J. (2003). Adaptive evolution in the photosensory domain of phytochrome a in early angiosperms. Mol. Biol. Evol. 20, 1087–1097. doi: 10.1093/molbev/msg123
Mathieu, J., Yant, L. J., Murdter, F., Kuttner, F., and Schmid, M. (2009). Repression of flowering by the mir172 target smz. PLoS Biol. 7:e1000148. doi: 10.1371/journal.pbio.1000148
Mayer, K. F. X., Schoof, H., Haecker, A., Lenhard, M., Jurgens, G., and Laux, T. (1998). Role of WUSCHEL in regulating stem cell fate in the Arabidopsis shoot meristem. Cell 95, 805–815. doi: 10.1016/s0092-8674(00)81703-1
Mi, S., Cai, T., Hu, Y., Chen, Y., Hodges, E., Ni, F.,, et al. (2008). Sorting of small rnas into arabidopsis argonaute complexes is directed by the 5’ terminal nucleotide. Cell 133, 116–127. doi: 10.1016/j.cell.2008.02.034
Miwa, H., Kinoshita, A., Fukuda, H., and Sawa, S. (2009). Plant meristems: clavata3/esr-related signaling in the shoot apical meristem and the root apical meristem. J. Plant Res. 122, 31–39. doi: 10.1007/s10265-008-0207-3
Moussian, B., Schoof, H., Haecker, A., Jurgens, G., and Laux, T. (1998). Role of the zwille gene in the regulation of central shoot meristem cell fate during Arabidopsis embryogenesis. EMBO J. 17, 1799–1809. doi: 10.1093/emboj/17.6.1799
Nemhauser, J. L., Feldman, L. J., and Zambryski, P. C. (2000). Auxin and ettin in Arabidopsis gynoecium morphogenesis. Development 127, 3877–3888.
Nozawa, M., Miura, S., and Nei, M. (2012). Origins and evolution of microrna genes in plant species. Genome Biol. Evol. 4, 230–239. doi: 10.1093/gbe/evs002
Ohto, M. A., Fischer, R. L., Goldberg, R. B., Nakamura, K., and Harada, J. J. (2005). Control of seed mass by apetala2. Proc. Natl. Acad. Sci. U.S.A. 102, 3123–3128. doi: 10.1073/pnas.0409858102
Ouyang, X., Li, J., Li, G., Li, B., Chen, B., Shen, H.,, et al. (2011). Genome-wide binding site analysis of far-red elongated hypocotyl3 reveals its novel function in Arabidopsis development. Plant Cell 23, 2514–2535. doi: 10.1105/tpc.111.085126
Pfeiffer, A., Janocha, D., Dong, Y., Medzihradszky, A., Schone, S., Daum, G.,, et al. (2016). Integration of light and metabolic signals for stem cell activation at the shoot apical meristem. eLife 5:e17023.
Prunet, N., Morel, P., Negrutiu, I., and Trehin, C. (2009). Time to stop: flower meristem termination. Plant Physiol. 150, 1764–1772. doi: 10.1104/pp.109.141812
Reinhardt, D., Mandel, T., and Kuhlemeier, C. (2000). Auxin regulates the initiation and radial position of plant lateral organs. Plant Cell 12, 507–518. doi: 10.1105/tpc.12.4.507
Rogers, K., and Chen, X. (2013). Biogenesis, turnover, and mode of action of plant micrornas. Plant Cell 25, 2383–2399. doi: 10.1105/tpc.113.113159
Roth, O., Alvarez, J. P., Levy, M., Bowman, J. L., Ori, N., and Shani, E. (2018). The knoxi transcription factor shoot meristemless regulates floral fate in Arabidopsis. Plant Cell 30, 1309–1321. doi: 10.1105/tpc.18.00222
Sablowski, R. (2004). Plant and animal stem cells: conceptually similar, molecularly distinct? Trends Cell Biol. 14, 605–611. doi: 10.1016/j.tcb.2004.09.011
Sablowski, R. (2007). Flowering and determinacy in Arabidopsis. J. Exp. Bot. 58, 899–907. doi: 10.1093/jxb/erm002
Satbhai, S. B., Ristova, D., and Busch, W. (2015). Underground tuning: quantitative regulation of root growth. J. Exp. Bot. 66, 1099–1112. doi: 10.1093/jxb/eru529
Schaller, G. E., Bishopp, A., and Kieber, J. J. (2015). The yin-yang of hormones: cytokinin and auxin interactions in plant development. Plant Cell 27, 44–63.
Schultz, E. A., and Haughn, G. W. (1991). Leafy, a homeotic gene that regulates inflorescence development in Arabidopsis. Plant Cell 3, 771–781. doi: 10.1105/tpc.3.8.771
Scofield, S., Dewitte, W., and Murray, J. A. (2007). The KNOX gene SHOOT MERISTEMLESS is required for the development of reproductive meristematic tissues in Arabidopsis. Plant J. 50, 767–781. doi: 10.1111/j.1365-313x.2007.03095.x
Scofield, S., Murison, A., Jones, A., Fozard, J., Aida, M., Band, L. R.,, et al. (2018). Coordination of meristem and boundary functions by transcription factors in the shoot meristemless regulatory network. Development 145:dev157081.
Sessions, A., Nemhauser, J. L., McColl, A., Roe, J. L., Feldmann, K. A., and Zambryski, P. C. (1997). Ettin patterns the Arabidopsis floral meristem and reproductive organs. Development 124, 4481–4491.
Simonini, S., Bencivenga, S., Trick, M., and Ostergaard, L. (2017). Auxin-induced modulation of ettin activity orchestrates gene expression in Arabidopsis. Plant Cell 29, 1864–1882. doi: 10.1105/tpc.17.00389
Smyth, D. R., Bowman, J. L., and Meyerowitz, E. M. (1990). Early flower development in Arabidopsis. Plant Cell 2, 755–767.
Soyars, C. L., James, S. R., and Nimchuk, Z. L. (2016). Ready, aim, shoot: stem cell regulation of the shoot apical meristem. Curr. Opin. Plant Biol. 29, 163–168. doi: 10.1016/j.pbi.2015.12.002
Sozzani, R., and Iyer-Pascuzzi, A. (2014). Postembryonic control of root meristem growth and development. Curr. Opin. Plant Biol. 17, 7–12. doi: 10.1016/j.pbi.2013.10.005
Spinelli, S. V., Martin, A. P., Viola, I. L., Gonzalez, D. H., and Palatnik, J. F. (2011). A mechanistic link between STM and CUC1 during Arabidopsis development. Plant Physiol. 156, 1894–1904. doi: 10.1104/pp.111.177709
Sun, B., Xu, Y. F., Ng, K. H., and Ito, T. (2009). A timing mechanism for stem cell maintenance and differentiation in the Arabidopsis floral meristem. Genes Dev. 23, 1791–1804. doi: 10.1101/gad.1800409
Sun, B., Zhou, Y., Cai, J., Shang, E., Yamaguchi, N., Xiao, J.,, et al. (2019). Integration of transcriptional repression and polycomb-mediated silencing of wuschel in floral meristems. Plant Cell 31, 1488–1505. doi: 10.1105/tpc.18.00450
Talbert, P. B., Adler, H. T., Parks, D. W., and Comai, L. (1995). The revoluta gene is necessary for apical meristem development and for limiting cell divisions in the leaves and stems of Arabidopsis thaliana. Development 121, 2723–2735.
Tucker, M. R., Hinze, A., Tucker, E. J., Takada, S., Jurgens, G., and Laux, T. (2008). Vascular signalling mediated by zwille potentiates wuschel function during shoot meristem stem cell development in the Arabidopsis embryo. Development 135, 2839–2843. doi: 10.1242/dev.023648
van der Graaff, E., Laux, T., and Rensing, S. A. (2009). The wus homeobox-containing (wox) protein family. Genome Biol. 10:248. doi: 10.1186/gb-2009-10-12-248
Vanneste, S., and Friml, J. (2009). Auxin: a trigger for change in plant development. Cell 136, 1005–1016. doi: 10.1016/j.cell.2009.03.001
Vaucheret, H. (2008). Plant argonautes. Trends Plant Sci. 13, 350–358. doi: 10.1016/j.tplants.2008.04.007
Vaucheret, H., Vazquez, F., Crete, P., and Bartel, D. P. (2004). The action of argonaute1 in the mirna pathway and its regulation by the mirna pathway are crucial for plant development. Genes Dev. 18, 1187–1197. doi: 10.1101/gad.1201404
Wang, H., and Wang, H. (2015). Multifaceted roles of fhy3 and far1 in light signaling and beyond. Trends Plant Sci. 20, 453–461. doi: 10.1016/j.tplants.2015.04.003
Wang, J. W., Czech, B., and Weigel, D. (2009). Mir156-regulated spl transcription factors define an endogenous flowering pathway in Arabidopsis thaliana. Cell 138, 738–749. doi: 10.1016/j.cell.2009.06.014
Wang, Q., Hasson, A., Rossmann, S., and Theres, K. (2016). Divide et impera: boundaries shape the plant body and initiate new meristems. New Phytol. 209, 485–498. doi: 10.1111/nph.13641
Weigel, D., and Meyerowitz, E. M. (1994). The abcs of floral homeotic genes. Cell 78, 203–209. doi: 10.1016/0092-8674(94)90291-7
Wollmann, H., Mica, E., Todesco, M., Long, J. A., and Weigel, D. (2010). On reconciling the interactions between apetala2, mir172 and agamous with the abc model of flower development. Development 137, 3633–3642. doi: 10.1242/dev.036673
Wu, G., Park, M. Y., Conway, S. R., Wang, J. W., Weigel, D., and Poethig, R. S. (2009). The sequential action of mir156 and mir172 regulates developmental timing in Arabidopsis. Cell 138, 750–759. doi: 10.1016/j.cell.2009.06.031
Wuest, S. E., Philipp, M. A., Guthorl, D., Schmid, B., and Grossniklaus, U. (2016). Seed production affects maternal growth and senescence in Arabidopsis. Plant Physiol. 171, 392–404. doi: 10.1104/pp.15.01995
Wurschum, T., Gross-Hardt, R., and Laux, T. (2006). APETALA2 regulates the stem cell niche in the Arabidopsis shoot meristem. Plant Cell 18, 295–307. doi: 10.1105/tpc.105.038398
Xu, Y. F., Yamaguchi, N., Gan, E. S., and Ito, T. (2019). When to stop: an update on molecular mechanisms of floral meristem termination. J. Exp. Bot. 70, 1711–1718. doi: 10.1093/jxb/erz048
Xu, Y. Y., Wang, X. M., Li, J., Li, J. H., Wu, J. S., Walker, J. C.,, et al. (2005). Activation of the wus gene induces ectopic initiation of floral meristems on mature stem surface in Arabidopsis thaliana. Plant Mol. Biol. 57, 773–784. doi: 10.1007/s11103-005-0952-9
Yadav, R. K., Perales, M., Gruel, J., Girke, T., Jonsson, H., and Reddy, G. V. (2011). Wuschel protein movement mediates stem cell homeostasis in the Arabidopsis shoot apex. Genes Dev. 25, 2025–2030. doi: 10.1101/gad.17258511
Yamaguchi, N., Huang, J., Tatsumi, Y., Abe, M., Sugano, S. S., Kojima, M.,, et al. (2018). Chromatin-mediated feed-forward auxin biosynthesis in floral meristem determinacy. Nat. Commun. 9:5290. doi: 10.1038/s41467-018-07763-0
Yamaguchi, N., Huang, J., Xu, Y., Tanoi, K., and Ito, T. (2017). Fine-tuning of auxin homeostasis governs the transition from floral stem cell maintenance to gynoecium formation. Nat. Commun. 8:1125. doi: 10.1038/s41467-017-01252-6
Yanai, O., Shani, E., Dolezal, K., Tarkowski, P., Sablowski, R., Sandberg, G.,, et al. (2005). Arabidopsis knoxi proteins activate cytokinin biosynthesis. Curr. Biol. 15, 1566–1571. doi: 10.1016/j.cub.2005.07.060
Yant, L., Mathieu, J., Dinh, T. T., Ott, F., Lanz, C., Wollmann, H.,, et al. (2010). Orchestration of the floral transition and floral development in Arabidopsis by the bifunctional transcription factor apetala2. Plant Cell 22, 2156–2170. doi: 10.1105/tpc.110.075606
Yu, Y., Ji, L., Le, B. H., Zhai, J., Chen, J., Luscher, E.,, et al. (2017a). Argonaute10 promotes the degradation of mir165/6 through the sdn1 and sdn2 exonucleases in Arabidopsis. PLoS Biol. 15:e2001272. doi: 10.1371/journal.pbio.2001272
Yu, Y., Jia, T., and Chen, X. (2017b). The ‘how’ and ‘where’ of plant micrornas. New Phytol. 216, 1002–1017. doi: 10.1111/nph.14834
Yumul, R. E., Kim, Y. J., Liu, X. G., Wang, R. Z., Ding, J. H., Xiao, L. T.,, et al. (2013). Powerdress and diversified expression of the mir172 gene family bolster the floral stem cell network. PLoS Genet. 9:e1003218.
Zadnikova, P., and Simon, R. (2014). How boundaries control plant development. Curr. Opin. Plant Biol. 17, 116–125. doi: 10.1016/j.pbi.2013.11.013
Zhang, D., and Yuan, Z. (2014). Molecular control of grass inflorescence development. Annu. Rev. Plant Biol. 65, 553–578. doi: 10.1146/annurev-arplant-050213-040104
Zhang, K., Wang, R., Zi, H., Li, Y., Cao, X., Li, D.,, et al. (2018). Auxin response factor3 regulates floral meristem determinacy by repressing cytokinin biosynthesis and signaling. Plant Cell 30, 324–346. doi: 10.1105/tpc.17.00705
Zhang, X., Zong, J., Liu, J., Yin, J., and Zhang, D. (2010). Genome-wide analysis of WOX gene family in rice, sorghum, maize, Arabidopsis and poplar. J. Integr. Plant Biol. 52, 1016–1026. doi: 10.1111/j.1744-7909.2010.00982.x
Zhang, Z., and Zhang, X. (2012). Argonautes compete for mir165/166 to regulate shoot apical meristem development. Curr. Opin. Plant Biol. 15, 652–658. doi: 10.1016/j.pbi.2012.05.007
Zhao, L., Kim, Y., Dinh, T. T., and Chen, X. (2007). Mir172 regulates stem cell fate and defines the inner boundary of APETALA3 and PISTILLATA expression domain in Arabidopsis floral meristems. Plant J. 51, 840–849. doi: 10.1111/j.1365-313x.2007.03181.x
Zhao, Z., Andersen, S. U., Ljung, K., Dolezal, K., Miotk, A., Schultheiss, S. J.,, et al. (2010). Hormonal control of the shoot stem-cell niche. Nature 465, 1089–1092. doi: 10.1038/nature09126
Zheng, Y., and Liu, X. (2019). Review: chromatin organization in plant and animal stem cell maintenance. Plant Sci. 281, 173–179. doi: 10.1016/j.plantsci.2018.12.026
Zhou, G. K., Kubo, M., Zhong, R., Demura, T., and Ye, Z. H. (2007). Overexpression of mir165 affects apical meristem formation, organ polarity establishment and vascular development in Arabidopsis. Plant Cell Physiol. 48, 391–404. doi: 10.1093/pcp/pcm008
Zhu, H., Hu, F., Wang, R., Zhou, X., Sze, S. H., Liou, L. W.,, et al. (2011). Arabidopsis argonaute10 specifically sequesters mir166/165 to regulate shoot apical meristem development. Cell 145, 242–256. doi: 10.1016/j.cell.2011.03.024
Keywords: SAM maintenance, FM determinacy, miRNAs, AGO1/10, ARF3, AP2, FHY3, STM
Citation: Chang W, Guo Y, Zhang H, Liu X and Guo L (2020) Same Actor in Different Stages: Genes in Shoot Apical Meristem Maintenance and Floral Meristem Determinacy in Arabidopsis. Front. Ecol. Evol. 8:89. doi: 10.3389/fevo.2020.00089
Received: 03 December 2019; Accepted: 18 March 2020;
Published: 03 April 2020.
Edited by:
Annette Becker, University of Giessen, GermanyReviewed by:
Toshiro Ito, Nara Institute of Science and Technology (NAIST), JapanBenoit Landrein, UMR5667 Reproduction et Developpement des Plantes (RDP), France
Harley M. Smith, Agriculture and Food, Commonwealth Scientific and Industrial Research Organisation (CSIRO), Australia
Copyright © 2020 Chang, Guo, Zhang, Liu and Guo. This is an open-access article distributed under the terms of the Creative Commons Attribution License (CC BY). The use, distribution or reproduction in other forums is permitted, provided the original author(s) and the copyright owner(s) are credited and that the original publication in this journal is cited, in accordance with accepted academic practice. No use, distribution or reproduction is permitted which does not comply with these terms.
*Correspondence: Xigang Liu, eGdsaXVAc2p6aWFtLmFjLmNu; Lin Guo, Z3VvbGluQHNqemlhbS5hYy5jbg==
†These authors have contributed equally to this work