- 1Environmental Laboratory, U.S. Army Engineer Research and Development Center, Vicksburg, MS, United States
- 2Contractor to Environmental Laboratory, U.S. Army Engineer Research and Development Center, Vicksburg, MS, United States
An increasing abundance of projects demonstrate that coastal management strategies that align engineering and ecological objectives can deliver a wide range benefits. Better understanding how these strategies fare under stress is crucially important, including in comparison to more conventional coastal engineering approaches, in order to inform where they might be a viable alternative or complement to conventional coastal storm risk management. In particular, the prospect that these strategies may be able to recover from disturbances and adapt to better survive future disturbances with minimal or no intervention is compelling. However, no formal accounting method exists to assess how ecosystem-based approaches contribute to the resilience of coastal systems, that is, their ability to prepare, absorb, recover, and adapt from stressors. An assessment rubric is developed and demonstrated for Engineering With Nature® projects and limitations and ways forward are discussed.
Introduction
The co-existence and also clash of human activity and ecosystems at the interface of the land and ocean raise questions about how best to achieve balance among multiple coastal management objectives while maximizing benefits. Thinking about how to preserve human activity (e.g., shipping, tourism, energy exploration, fisheries, and others) in spite of hazards associated with coastal zones (i.e., energetic storms and inundation by rising sea levels) while reducing conflict with ecosystems is evolving. This is in part because highly engineered systems that interface with natural forces have sometimes proven brittle and while stronger, more robust solutions exist, they may come with exorbitant costs and may only delay brittle failure. At a time when coastal infrastructure is in need of investment and updating, decision makers have the opportunity to invest in natural and nature-based infrastructure to increase resilience and provide cost-effective critical services (Sutton-Grier et al., 2018). Efforts to better align the delivery of engineering objectives with environmental and social objectives abound in the United States, Europe, and are burgeoning worldwide (Bridges et al., 2018). They are demonstrating that strategies to enhance ecosystems and leverage natural processes can deliver a wide array of environmental, social, and economic benefits in a cost-effective manner. However, there is an enduring research need to examine how they perform under stress; for example, determining how natural and nature-based systems perform with respect to resilience goals has not been sufficiently explored. This paper examines the challenge of quantifying resilience benefits of coastal projects, develops an assessment method suited to existing projects, and discusses ways forward to meet the challenge.
USACE and the Nation’s Coastlines
These questions are salient to the United States Army Corps of Engineers (USACE), which plays an important role in coastal management in the United States, as the coastal zone is where its missions are often applicable and overlapping. The USACE is responsible for conducting and operating civil works projects to maintain the navigability of the nation’s waterways to support economic activity, reduce riverine and coastal flooding risk to minimize property damage and loss, and restore and manage aquatic ecosystems. The work to achieve these missions exists in tandem with complex natural and human dynamics including sediment processes, natural hazard risk prediction, and cost benefit trade-offs, among others complicating factors.
The USACE and other organizations are exploring new approaches to achieving agency missions in response to these present-day realities, a trend that is in line with the evolving policy and practice of incorporating nature-based approaches in Europe as well (Nesshöver et al., 2017). Engineering With Nature® (EWN®) is an initiative of the USACE that aims to investigate, demonstrate, and support the design of projects that meet engineering and mission objectives but also seeks to provide environmental benefits and enhance long-term sustainability. EWN efforts take a variety of forms to support water infrastructure projects, but share that they pursue “intentional alignment of natural and engineering processes to efficiently and sustainably deliver economic, environmental, and social benefits through collaboration” (Bridges et al., 2018). The overarching strategy is to use natural processes to achieve engineering objectives or to use human design to emulate natural features and functions, better aligning projects with nature and yielding greater value by addressing multiple objectives.
An important motivation for the EWN initiative is to more fully account for the array of environmental, economic, and social benefits that are generated by USACE projects (Foran et al., 2018), and to promote designs that can achieve numerous co-benefits. The initiative does so by enumerating benefits that may not be fully accounted for in common applications of 1983 Economic and Environmental Principles and Guidelines for Water and Related Land Implementation Studies and made generally on the basis of economic valuation (Narayan et al., 2017). The range of benefits of EWN projects include flood risk reduction, recreation, wildlife habitat, fish habitat, reduced shoreline erosion, navigation safety, plant habitat, and aesthetic value, among others (Bridges et al., 2014).
A co-benefit of increasing interest to the USACE and other organizations is resilience1, which can be derived from EWN projects (Bridges and Chasten, 2016). Resilience benefit to communities is expected to emerge from the enhancement of coastal ecosystems, the intention of which is both anthropocentric and eco-centric. The two categories are not mutually exclusive (Sutton-Grier et al., 2015), as has been articulated by the concept of “ecosystem goods and services” – that humans benefit directly or indirectly from the existence and functioning of ecosystems (Fisher et al., 2009). There is a vast body of literature that seeks to define and enumerate the benefits that humans derive from functioning ecosystems, especially so that they can enter systems of formal accounting that accompany assignment of economic valuation to projects (see literature review by Tazik et al., 2013). While the philosophy of EWN intuitively intersects with the concept of resilience, a formal approach does not exist for aligning resilience outcomes with EWN projects, as difficulty arises from how resilience should be applied via ecosystems for different types of hazards, which occur on different time scales, threaten systems with different stress thresholds, and have institutionally entrenched methods for managing them.
Conventional and Nature-Based Approaches to Coastal Risk Management
For coastal communities, flood hazard stems from the occurrence of storm surge and heavy precipitation that accompany coastal storms and loss of land mass to subsidence, coastal erosion, and sea level rise (Neumann et al., 2014). Coastal engineering is intended to provide defense against coastal flood risk and is designed to protect built infrastructure and human life from exposure to the full extent of coastal hazards (floodwaters, wind, etc.). The necessity of coastal defense is evident. In 2010, 39% of the 313 million people in the United States lived in coastal counties and 52% lived in coastal watershed counties; additionally, economies in coastal communities account for approximately $8.3 trillion in goods and services (National Oceanic and Atmospheric Administration [NOAA], 2019). However, an approach that relies predominantly on conventional structural measures to exclude floodwater and dissipate wave energy (e.g., with seawalls and breakwaters) is being called into question for several reasons. Among them are that achieving desired levels of protection is increasingly costly and that coastal structures can interfere with natural dynamics that maintain coastlines and land elevation (Temmerman et al., 2013) and can impact coastal ecosystems (Gittman et al., 2016).
Ecosystem-based coastal protection measures may be a viable alternative or complement to conventional coastal storm risk management and are being explored as such. Effective risk management reduces the parameters of risk, which is conceptualized as the product of hazard, vulnerability, and consequence (Willows et al., 2003). In their cost-benefit analysis of defense measures, Reguero et al. (2018) defines risk mitigation benefit in the context of coastal disasters as being derived from (1) hazard reduction via wave and surge attenuation, (2) physical protection from floods; and/or (3) physical exposure aversion. In their review, Shepard et al. (2011) found salt marsh protections that correspond with Reguero et al. (2018) risk mitigation benefits: wave attenuation as measured by reductions in wave height, and shoreline stabilization as measured by accretion, lateral erosion reduction, and marsh surface elevation change. Shepard et al. (2011) concludes that coastal ecosystems should be mobilized and protected by policy makers for economic and societal benefits. Further, studies that employ modeling aim to translate wave and storm surge attenuation to into efficacy and expected reduction in damages (Barbier and Enchelmeyer, 2014; Barbier, 2015; Vuik et al., 2016; Narayan et al., 2017; Reguero et al., 2018). Pontee et al. (2016) provides a small survey of diverse nature-based projects and reports lessons learned while Saleh and Weinstein (2016) review the literature on tidal wetlands, thin-layer placement, and living shorelines and also reports mixed results from a coastal protection perspective. Few studies, however, have compared the actual performance of ecosystem-based coastal protection to conventional measures. Gittman et al. (2014) compared the performance of North Carolina shoreline protection measures during Hurricane Irene (a category 1 hurricane) and found that marshes with and without sills suffered less erosion than bulkheads. More direct studies are needed to build the evidence base of where and under what circumstances ecosystem-based approaches can outperform or supplement their conventional counterparts.
Despite increasing efforts to mitigate the impact of natural disasters, losses suffered continues to rise (National Oceanic and Atmospheric Administration [NOAA], 2018). In fact, this reality is the primary motivation for United States and worldwide policies that set resilience management objectives (Bakkensen et al., 2017; Linkov and Trump, 2019); the inadequacy of risk management alone to reduce losses points to the need for new objectives related to maintaining system functions to their greatest extent in spite of disruptive events. In 2013, the USACE Chief of Engineers charged the Coastal Engineering Research Board (CERB) to strategize integrating risk reduction and resilience into Corps practices (Rosati et al., 2015). In their definition of resilience, the National Academy of Sciences (NAS) (National Research Council [NRC], 2012) stress the abilities to prepare and plan for, absorb, recover from, and more successfully adapt to adverse events. These cycles correlate with the definition of resilience considered by the CERB (Rosati et al., 2015) and capture the temporal dimension of resilience (Linkov et al., 2013). Action and infrastructure generally assigned to flood risk mitigation contribute to preparation for weather events and reduction of damages but do not extend to recovery and adaptation. Therefore, efforts to meet resilience objectives seek measures that improve systems along the entire lifecycle of a disaster. An assertion about the potential of ecosystem-based coastal defense to contribute to resilience has emerged and warrants further investigation, particularly given that it is already being considered as an alternative to conventional engineering solutions for the reasons described above (Spalding et al., 2014).
Defining Resilience for the Purpose of Measurement
Answering the question of how coastal ecosystems and ecosystem-based approaches to coastal management contribute to the resilience of a system requires teasing out and delineating the “system” and what it should be resilient to. In other words, the “resilience of what, to what” as was posited by Carpenter et al. (2001) almost two decades ago. Resilience broadly concerns how systems respond to stress, both acute and chronic. While many fields have expressed that the concept may be useful for describing and managing systems (communities, infrastructure, psychology, ecosystems, etc.), its adoption as a management objection has raised various debatable points: what constitutes a resilient outcome; can the resilience of a system to stress be predicted; are there attributes or indicators of a resilient system; what conditions or actions will foster resilient outcomes; and others.
A large and growing body of literature works through these questions (e.g., see Walker and Cooper, 2011; Liao, 2012; Kress et al., 2016; Timpane-Padgham et al., 2017) and includes efforts to describe similarities, differences and interactions between the resilience of engineered and ecological systems (Holling, 1996; Angeler et al., 2018). Key differences stem from the nature of the objectives of the respective disciplines - the study and practice of engineering is prescriptive and assumes that resilience is normative whereas ecology is descriptive and more agnostic about stability. Hence, engineered systems are deliberately managed to achieve and maintain a single stable state and are generally recovered back to their original state following damage whereas ecological systems are observed to be capable of multiple states of variable stability (Pendall et al., 2010; Meerow et al., 2016). The two types of systems are not necessarily discrete, as has been recognized by resilience typologies such as socio-ecological resilience (Davidson et al., 2016).
The goal of our current research effort is to develop and demonstrate a rubric that is generally applicable to EWN projects and other ecosystem-based coastal features that vary greatly in their form and function. In terms of formulating an assessment method for EWN projects, bounding the problem serves to reduce some of the indeterminacy of the concept of resilience benefits of coastal ecosystems. Resilience is considered primarily with respect to the well-being of proximal communities in the presence of coastal hazards. More specifically, resilience is expected to emerge from:
• Buffering the built environment from/reducing the impact of coastal stressors and shocks by absorbing energy;
• Persistence of coastal features (including by robustness to impact, recovery and adaptation), mainly via sediment processes (stabilizing and/or accreting sediment); and
• Adaptability of landforms by natural or human means.
By using the resilience considerations mentioned above, a rubric was developed and applied to EWN projects as an attempt to better understand the performance of EWN projects in terms of their contributions to coastal ecosystem management.
Case Study Resilience Evaluation
Methodology
Since 2010, over 250 civil engineering projects in both coastal and inland environments in the United States and worldwide have employed practices consistent with EWN principles. Project details are documented and accessible via the EWN Project Mapper (ProMap), an online database and map viewer developed by the USACE, including site descriptions, associated infrastructure project types, engineering features, project benefits, and links to supplemental resources2. Project information in ProMap is sourced from a variety of resources, and thus content quality and quantity varies considerably among projects. For this reason, not all project descriptions contained the data and other information necessary to perform the evaluation; information was adequately detailed for 89 coastal projects, the majority of which are marine. Each was reviewed to determine the specific engineering strategies that were implemented. The engineering strategies were then summarized as a list of 27 feature types (Figure 1). Feature types are generally defined by feature’s coastal engineering form (e.g., breakwater, levee, and groin) and the specific aspect that indicates that the engineering involves nature (e.g., incorporates habitat opportunities, beneficially re-uses dredged sediment, relies on the functioning of natural elements to achieve engineering objectives). Each project was assigned at least one feature type (note that the number of projects that are grouped into feature type is in parentheses next to feature type labels in Figure 1). For example, the Vermillion Bay Oyster Reef Restoration project in New Orleans, LA, United States utilized reef modules to restore oyster reefs and provide a habitat for fish. This engineering feature type was categorized as “breakwater constructed with modified concrete blocks that allow habitat growth opportunities”. Feature types were formulated to group similar projects while retaining a sufficient level of detail to be informative about how project designs intend to achieve multiple and synergistic objectives.
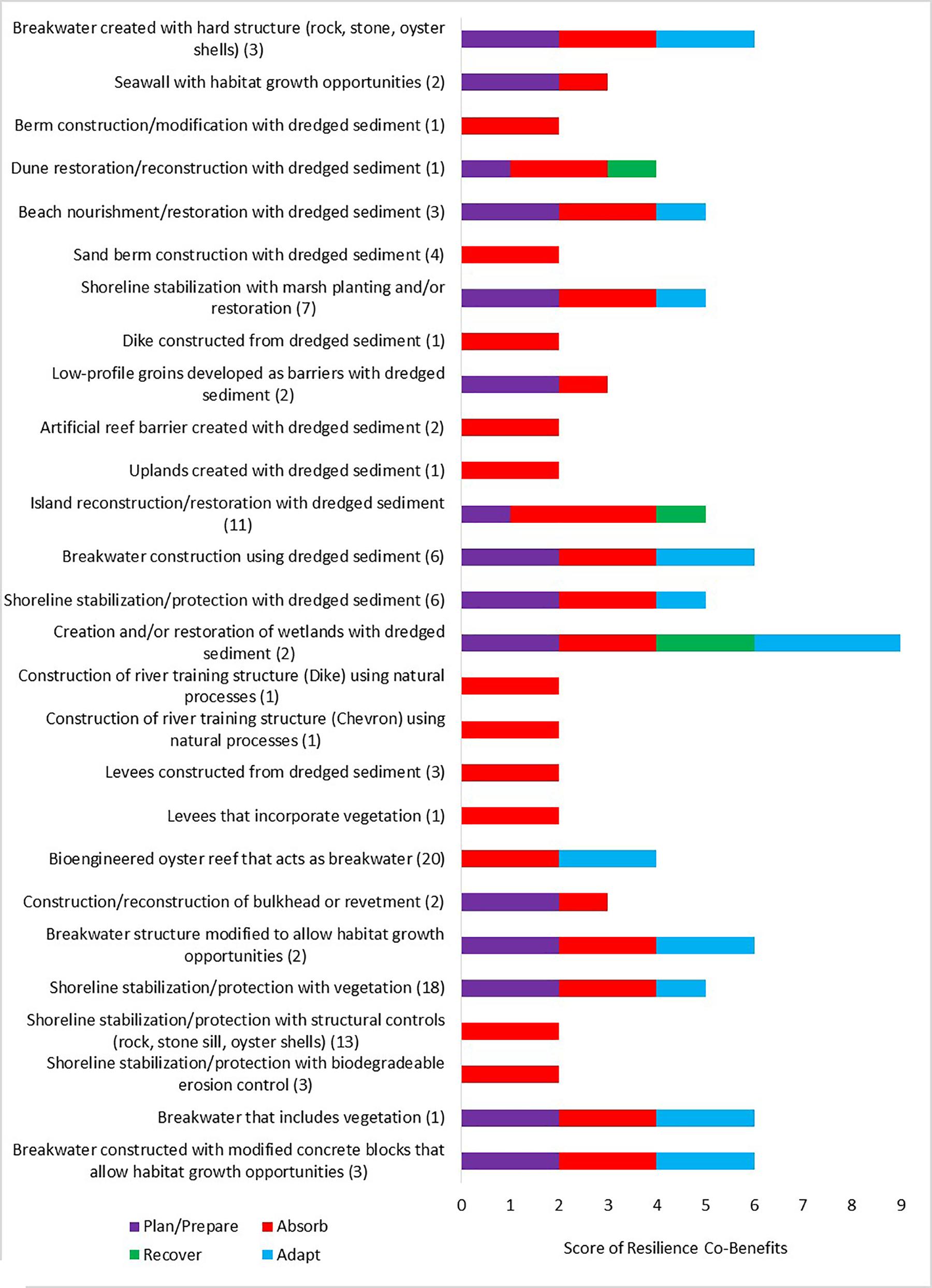
Figure 1. Resilience scores of EWN features types. Number in parentheses indicates how many projects were classified as being of that feature type.
Each engineering feature type was evaluated for its contribution to (1) resilience and (2) USACE business lines (i.e., flood risk management, navigation, ecosystem restoration). A rubric was developed for assessing the resilience contribution of EWN feature types. It disaggregates resilience into four phases of the disaster lifecycle as defined by the NAS (National Research Council [NRC], 2012) – plan/prepare, absorb, recover, and adapt – and suggested as appropriate for capturing the temporal dimension of resilience (Linkov et al., 2013). Indicators of resilience were developed to capture the expected ability of feature types to prepare for and absorb shocks, recover from damages, and adapt to better prepare for future conditions and shocks. Indicators were derived from a report of the Environmental Defense Fund (EDF) that used literature support and expert judgment to evaluate the strengths, known weaknesses, uncertainties, and suitable conditions of engineered coastal features as methods for risk reduction (Cunniff and Schwartz, 2015). That report summarizes the outcomes of a workshop of 19 subject matter experts including scientists, engineers, program managers, and financiers that provided insight into the performance of natural infrastructure and nature-based features and supplemented with a review of literature on the same subject. In order to create an assessment rubric, all of the beneficial qualities of coastal features were assigned to a NAS resilience category. Descriptions in the EDF report generally contained terms that could be aligned to a temporal scale of disturbances. Similar benefits were summarized into indicator statements so they could be applied to the EWN projects (Table 1). The coastal features evaluated in Cunniff and Schwartz (2015) and the feature types used in this research are generally similar and therefore the beneficial qualities (i.e., indicators) were assumed to be extendable to EWN project evaluation. A tally was generated for feature type to quantify how many indicators of resilience each was judged to possess. As an example, the Hart-Miller Island, which is located near the mouths of Back and Middle rivers near Baltimore, MA, United States, was developed with placement of dredged material. The island was categorized as a “barrier island” and barrier islands have characteristics that align with all of the indicators of absorbing stress and shocks: dissipate wave energy from coastal storm surges, protects the shoreline from erosion, and acts as a wind break for the adjacent community. Project-level information contributed to the appropriate designation of feature type(s) and feature types were assessed in aggregate. Some of the weaknesses identified in the EDF report are included in the discussion section of this paper.
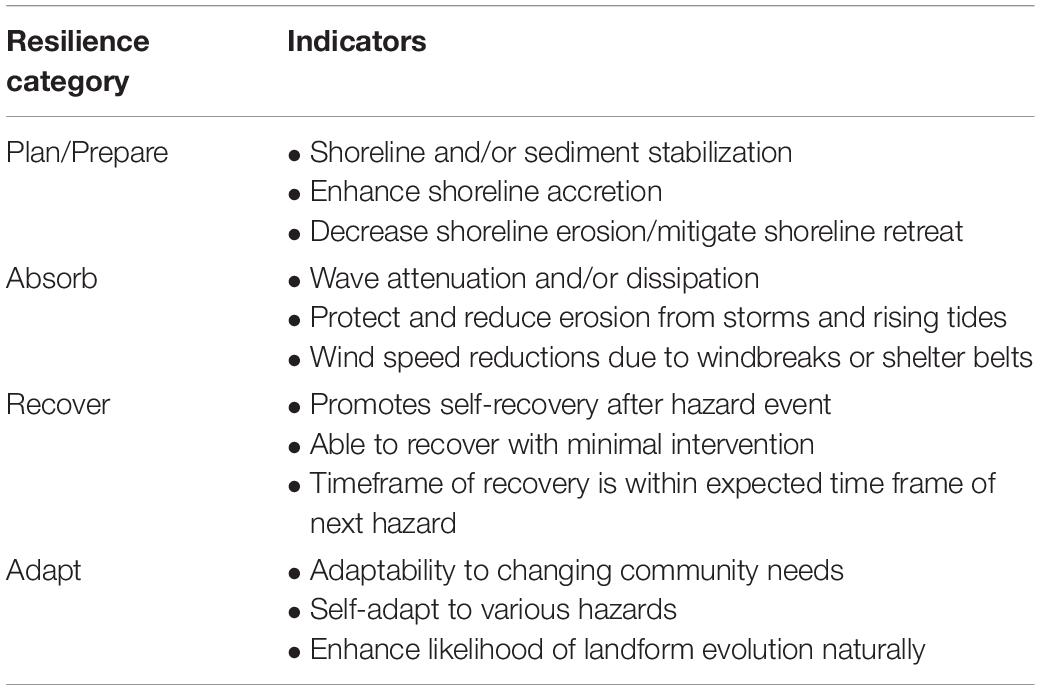
Table 1. Resilience Indicators [adapted from Cunniff and Schwartz (2015)].
An additional set of indicators was developed to account for the contributions of EWN projects to select USACE civil works programs (Table 2), which are essentially civil works objectives, with the goal of gaining insight into which types of USACE projects might have the most resilience contribution. Three business lines were chosen for the assessment: navigation management, environmental restoration, and a combination of flood management and coastal storm risk reduction, the latter two being distinguishable programs that have overlapping missions and benefits. The indicators of which business line(s) a project services were developed based on literature from the USACE including a technical report on the use of natural-based features to support coastal resilience (Bridges et al., 2015).
Each of the 27 EWN features types was assessed along the 12 resilience indicators and 10 USACE business line indicators. Feature types receive a binary 0–1 score for each indicator, where 1 was assigned primarily on whether the EDF report attributed a particular kind of benefit to a feature. Scores were summed for resilience and business line, respectively, such that total scores are the number of indicators each feature type was found to have. Each EWN project received the same score as their respective feature types. Some EWN projects implemented more than one of the 27 engineering features listed in Figure 1 and therefore received points for meeting the same indicator more than once. In these instances, the score received for that specific indicator was normalized to 1.
Results
Results of the resilience assessment of EWN features indicates that they tended to score higher in the planning/prepare, absorb, and adapt aspects of resilience and lower in their ability to recover. As indicated in Figure 1, only 3 of 27 feature types had any indicator of recoverability: dune restoration/reconstruction with dredged sediment; island reconstruction/restoration with dredged sediment; and creation and/or restoration of wetlands with dredged sediment. All 27 feature types had some indication of ability to absorb shocks whereas 15 met indicators for the ability to plan/prepare and 11 had indicators of being able to adapt.
A pairwise comparison between each of the indicators of USACE business lines and indicators of resilience, as shown in Figure 2, illustrates how many EWN feature types achieve a specific USACE civil works objective and contribute to resilience, as it was assessed in this study. The graph sums the number of resilience benefits that can be attributed to features that have an indicator of a business line. Each feature can have up to 12 “points,” as that is the number of resilience indicators used in the assessment rubric. The results show that projects that incorporated beneficial use of dredged sediment scored highest in the resilience assessment. Projects that employed structures that attenuate wave energy and projects that constructed or restored land masses scored the second and third highest in the resilience scoring, respectively. The pairwise comparison indicates where there are opportunities to use civil work projects to achieve the types of benefits that comprise the resilience assessment.
Discussion and Conclusion
The connection between EWN and resilience, in both the engineering and ecological sense, is intuitive to some extent, but is in need of a more formal connection between management and outcomes as well as a method for enumeration. The challenge of drawing clear links between the two lies as much with the indeterminacy of resilience as a concept (Kurth et al., 2018) as with the diverse and growing experience of EWN practitioners. A solution lies partly in establishing appropriate performance metrics and associated monitoring schemes to establish the relative success of EWN. It is important to note, however, that a lack of a formal accounting process to measure the connection between EWN and resilience should not stifle efforts to maximize the co-benefits of USACE civil works projects, which have a rich history of success (Bridges et al., 2018). Progress in ecosystem management and engineering is often achieved through learning-by-doing and adaptive strategies (Walters and Holling, 1990).
Questions remain about the extent to which or under what circumstances EWN projects might outperform more traditional coastal engineering approaches. The prospect of features that can recover from disturbances and adapt to better survive future disturbances with minimal or no intervention is compelling particularly in comparison to conventional infrastructure, which has little to no capacity to do so. Both have thresholds beyond which they cannot perform as intended and a tradeoff likely exists between the capacity to perform one function (e.g., be robust to a high energy storm) with the capacity to perform another (e.g., regenerate and migrate). Formally conducting a tradeoff analysis will require more data about how coastal features perform under stress and shocks. Questions that arose during this project were ones such as: what level of disturbance would permanently undermine the self-healing capability of an engineered natural feature, at least to return to the intended structure and function? And for how long does a nature-based feature need to be managed before it is fully functional and self-sustaining? Nesshöver et al. (2017) offer some considerations to maximize the success and utility of nature-based solutions and accept certain realities, which are useful for confronting the questions we raise. They suggest that implementation should include provisions for knowledge creation and concurrent social and technical innovation, as well as clear definition of success criteria and target objectives within the multifunctional role of nature, among others.
The demands that resilience, as an objective, place on consequent management efforts are numerous; resilience arises from systems that possess several capabilities, which cannot realistically be performed by singular components. EWN features may be powerful additions to a portfolio of system components that enhance resilience. An example would be complementary combinations of approaches that utilize conventional infrastructure along with restored or created natural infrastructure such as a salt marsh or oyster reef (e.g., Toft et al., 2014). Disaggregating the capabilities that can be fulfilled by EWN projects may help capture this reality and allow these projects to enter into the realm of resilience assessment. For example, the Resilience Matrix is a framework proposed by Linkov et al. (2013) and demonstrated by Fox-Lent et al. (2015) that structures the capabilities of a system that support it to maintain its performance quality despite stressors and shocks. Resilience assessment of this kind would place EWN projects alongside other elements that act in tandem to sustain a system. A formal accounting method to assess how EWN projects contribute to a system’s ability to prepare, respond, absorb, and adapt to different hazards would improve the enumeration of the benefits that can be derived from these projects and improve decision making about enhancing the resilience of coastal systems.
This paper provides a critical look at the role that EWN projects can serve in potentially enhancing the resilience of communities via coastal ecosystems. In the absence of consistent project documentation, in part due to varied nature of EWN project types and monitoring data, a qualitative resilience assessment rubric was developed. However, as with many ex ante assessments, it is difficult to know if projects possess the abilities as they are assessed (i.e., they have not been put to the test or if tested, have not been documented).
Some important considerations are raised where the primary intention of establishing and restoring ecosystems is the creation of resilience co-benefits for adjacent communities and built infrastructure. Some are that resilient ecosystems naturally fluctuate to states that might deliver less or different benefits than that of the original state; natural recovery of ecosystems from disturbance takes place on vastly different time scales than is ideal for the intended object of their protection; and that successful establishment of healthy ecosystems can be compromised by nearby human disturbances. In general, a better understanding of the coupled feedback loops between human and ecological coastal systems would be informative for maximizing benefits for both. For example, the ability of coastal ecosystems to buffer human systems from the impact of coastal processes may be dependent on the human systems managing the level of stress they place on ecosystems so that thresholds of what they can endure are not exceeded. Stated more simply, conserving and enhancing the natural system can support the resilience of coastal communities.
Data Availability Statement
The datasets generated for this study are available on request to the corresponding author.
Author Contributions
MK and RA identified the research need, conceived of the research design, and wrote the manuscript. RA carried out the analysis. IL supervised the project. BS provided the critical feedback. TB contributed to framing.
Funding
Funding for the project was received from the Engineer Research and Development Center Dredging Operations Technical Support Program. The findings discussed herein do not necessarily reflect those of the United States Army Corps of Engineers.
Conflict of Interest
The Engineering With Nature Initiative is a registered trademark of the United States Department of the Army.
The authors declare that the research was conducted in the absence of any commercial or financial relationships that could be construed as a potential conflict of interest.
Footnotes
- ^ The culmination of many events motivates USACE’s work to enhance resilience: lessons learned from devastating hurricanes such as Katrina and Sandy, Department of Defense (DoD) and executive branch policies emphasizing continuity of critical infrastructure functions, and greater recognition that uncertainty, complexity, and changing conditions complicate our ability to meet various objectives. The 2016 Resilience Initiative Roadmap establishes that resilience thinking should be implemented USACE-wide and work to mainstream the concept into USACE operations is ongoing.
- ^ Link to EWN ProMap: https://ewn.el.erdc.dren.mil/ProMap/index.html.
References
Angeler, D. G., Allen, C. R., Garmestani, A., Pope, K. L., Twidwell, D., and Bundschuh, M. (2018). Resilience in environmental risk and impact assessment: concepts and measurement. Bull. Environ. Contam. Toxicol. 101, 543–548. doi: 10.1007/s00128-018-2467-5
Bakkensen, L. A., Fox-Lent, C., Read, L. K., and Linkov, I. (2017). Validating resilience and vulnerability indices in the context of natural disasters. Risk Anal. 37, 982–1004. doi: 10.1111/risa.12677
Barbier, E. B. (2015). Valuing the storm protection service of estuarine and coastal ecosystems. Ecosyst. Serv. 11, 32–38. doi: 10.1016/j.ecoser.2014.06.010
Barbier, E. B., and Enchelmeyer, B. S. (2014). Valuing the storm surge protection service of US Gulf Coast wetlands. J. Environ. Econ. Policy 3, 167–185. doi: 10.1080/21606544.2013.876370
Bridges, T. S., Wagner, P. W., Burks-Copes, K. A., Bates, M. E., Collier, Z. A., and Fischenich, C. J., et al. (2015). “Use of Natural and Nature-Based Features (NNBF) for Coastal Resilience,” ERDC SR-15-1. Vicksburg, MS: US Army, Engineer Research and Development Center. Available online at: https://http://el.erdc.usace.army.mil/
Bridges, T. S., Bourne, E. M., King, J. K., Kuzmitski, H. K., Moynihan, E. B., and Suedel, B. C. (2018). Engineering With Nature: An atlas. ERDC/EL SR-18-8. Vicksburg, MS: U.S. Army Engineer Research and Development Center.
Bridges, T. S., and Chasten, M. A. (2016). Engineering with nature advancing system resilience and sustainable development. Military Eng. 699, 53–54.
Bridges, T. S., Lillycrop, J., Wilson, J. R., Fredette, T. J., Suedel, B., Banks, C. J., et al. (2014). Engineering with nature promotes triple-win outcomes. Terra Et Aqua. 135, 17–23.
Carpenter, S., Walker, B., Anderies, J., and Abel, N. (2001). From metaphor to measurement: resilience of what to what? Ecosystems 4:765. doi: 10.1007/s10021-001-0045-9
Cunniff, S., and Schwartz, A. (2015). Performance of Natural Infrastructure and Nature-based Measures as Coastal Risk Reduction Features. Environmental Defense Fund. Available online at: https://www.edf.org/sites/default/files/summary_ni_literature_compilation_0.pdf
Davidson, J., Jacobson, C., Lyth, A., Dedekorkut-Howes, A., Baldwin, C., Ellison, J., et al. (2016). Interrogating resilience: toward a typology to improve its operationalization. Ecol. Soc. 21:27. doi: 10.5751/ES-08450-210227
Fisher, B., Turner, R. K., and Morling, P. (2009). Defining and classifying ecosystem services for decision making. Ecol. Econ. 68, 643–653. doi: 10.1016/j.ecolecon.2008.09.014
Foran, C. M., Burks-Copes, K. A., Berkowitz, J., Corbino, J., and Suedel, B. C. (2018). Quantifying wildlife and navigation benefits of a dredging beneficial use project in the lower atchafalaya river: a demonstration of engineering with nature®. Integr. Environ. Assess. Manage. 14, 759–768. doi: 10.1002/ieam.4084
Fox-Lent, C., Bates, M. E., and Linkov, I. (2015). A matrix approach to community resilience assessment: an illustrative case at Rockaway Peninsula. Environ. Syst. Decis. 35, 209–218. doi: 10.1007/s10669-015-9555-4
Gittman, R. K., Popowich, A. M., Bruno, J. F., and Peterson, C. H. (2014). Marshes with and without sills protect estuarine shorelines from erosion better than bulkheads during a Category 1 hurricane. Ocean Coast. Manag. 102, 94–102. doi: 10.1016/j.ocecoaman.2014.09.016
Gittman, R. K., Scyphers, S. B., Smith, C. S., Neylan, I. P., and Grabowski, J. H. (2016). Ecological consequences of shoreline hardening: a meta-analysis. Bioscience 66, 763–773. doi: 10.1093/biosci/biw091
Holling, C. S. (1996). Engineering Resilience Versus Ecological Resilience. Engineering Within Ecological Constraints. Washington, D.C: National Academy Press.
Kress, M., Touzinsky, K. F., Vuxton, E. A., Greenfeld, B., Lillycrop, L. S., and Rosati, J. D. (2016). Alignment of U. S. ACE civil works missions to restore habitat and increase environmental resiliency. Coast. Manag. 44, 193–208. doi: 10.1080/08920753.2016.1160203
Kurth, M. H., Keenan, J. M., Sasani, M., and Linkov, I. (2018). Defining resilience for the US building industry. Build. Res. Inform. 47, 480–492.
Liao, K. (2012). A theory on urban resilience to floods—a basis for alternative planning practices. Ecol. Soc. 17:48. doi: 10.5751/ES-05231-170448
Linkov, I., Eisenberg, D. A., Bates, M. E., Chang, D., Convertino, M., Allen, J. H., et al. (2013). Measurable resilience for actionable policy. Environ. Sci. Technol. 47, 10108–10110. doi: 10.1021/es403443n
Meerow, S., Newell, J. P., and Stults, M. (2016). Defining urban resilience: a review. Landsc. Urban Plann. 147, 38–49. doi: 10.1016/j.landurbplan.2015.11.011
Narayan, S., Beck, M. W., Wilson, P., Thomas, C., Guerrero, A., Shepard, C., et al. (2017). The value of coastal wetlands for flood damage reduction in the Northeastern USA. Sci. Rep. 7:9463. doi: 10.1038/s41598-017-09269-z
National Research Council [NRC]. (2012). Disaster Resilience: A National Imperative. Washington, DC: The National Academies Press.
National Oceanic and Atmospheric Administration [NOAA]. (2018). Data from: U.S. Billion-Dollar Weather and Climate Disasters. National Centers for Environmental Information. Available online at: https://www.ncdc.noaa.gov/billions/ (accessed May 20, 2019).
National Oceanic and Atmospheric Administration [NOAA] (2019). Data from: Economics, and Demographics. Office for Coastal Management. Available online at: https://coast.noaa.gov/states/fast-facts/economics-and-demographics.html (accessed May 20, 2019).
Nesshöver, C., Assmuth, T., Irvine, K. N., Rusch, G., Waylen, K. A., Delbaere, B., et al. (2017). The science, policy and practice of nature-based solutions: an interdisciplinary perspective. Sci. Total Environ. 579, 1215–1227. doi: 10.1016/j.scitotenv.2016.11.106
Neumann, J. E., Emanuel, K., Ravela, S., Ludwig, L., and Kirshen, P. (2014). Joint effects of storm surge and sea-level rise on US Coasts: new economic estimates of impacts, adaptation, and benefits of mitigation policy. Clim. Chang. 129, 337–349. doi: 10.1007/s10584-014-1304-z
Pendall, R., Foster, K. A., and Cowell, M. (2010). Resilience and regions: building understanding of the metaphor. Cambridge J. Regions Econ. Soc. 3, 71–84. doi: 10.1093/cjres/rsp028
Pontee, N., Narayan, S., Beck, M. W., and Hosking, A. H. (2016). Nature-based solutions: lessons from around the world. Proc. Instit. Civil Eng. Maritime Eng. 169, 29–36.
Reguero, B. G., Beck, M. W., Bresch, D. N., Calil, J., and Meliane, I. (2018). Comparing the cost effectiveness of nature-based and coastal adaptation: a case study from the Gulf Coast of the United States. PLoS One 13:e0192132. doi: 10.1371/journal.pone.0192132
Rosati, J. D., Touzinsky, K. F., and Lillycrop, W. J. (2015). Quantifying coastal system resilience for the US Army corps of engineers. Environ. Syst. Decis. 35, 196–208.
Saleh, F., and Weinstein, M. P. (2016). The role of nature-based infrastructure (NBI) in coastal resiliency planning: a literature review. J. Environ. Manage. 183, 1088–1098. doi: 10.1016/j.jenvman.2016.09.077
Shepard, C. C., Crain, C. M., and Beck, M. W. (2011). The protective role of coastal marshes: a systematic review and meta-analysis. PLoS One 6:e27374. doi: 10.1371/journal.pone.0027374
Spalding, M. D., Ruffo, S., Lacambra, C., Lacambra, C., Meliane, I., Hale, L. Z., et al. (2014). Teh role of ecosystems in coastal protection: Adapting to climate change and coastal hazards. Ocean. Coast. Manag. 90, 50–57. doi: 10.1016/j.ocecoaman.2013.09.007
Sutton-Grier, A. E., Gittman, R. K., Arkema, K. K., Bennett, R. O., Benoit, J., Blitch, S., et al. (2018). Investing in natural and nature-based infrastructure: building better along our coasts. Sustainability 10:523.
Sutton-Grier, A. E., Wowk, K., and Bamford, H. (2015). Future of our coasts: the potential for natural and hybrid infrastructure to enhance the resilience of our coastal communities, economies, and ecosystems. Environ. Sci. Policy 51, 137–148. doi: 10.1016/j.envsci.2015.04.006
Tazik, D. J., Cushing, J., Murray, E., and Wainger, L. (2013). Incorporating Ecosystem Goods and Services in Environmental Planning: A Literature Review of Definitions, Classification and Operational Approaches. Vicksburg: Engineer Research and Development Center.
Temmerman, S., Meire, P., Bouma, T. J., Herman, P. M. J., Ysebaert, T., and Huib, J. D. V. (2013). Ecosystem-based coastal defence in the face of global change. Nature 504, 79–83.
Timpane-Padgham, B. L., Beechie, T., and Klinger, T. (2017). A systematic review of ecological attributes that confer resilience to climate change in environmental restoration. PLoS ONE. 12:e0173812. doi: 10.1371/journal.pone.0173812
Toft, J. D., Ogston, A. S., Heerhartz, S. M., Cordell, J. R., and Flemer, E. E. (2014). Ecological response and physical stability of habitat enhancements along an urban armored shoreline. Ecol. Eng. 57, 97–108.
Vuik, V., Jonkman, S. N., Borsje, B. W., and Suzuki, T. (2016). Nature-based flood protection: the efficiency of vegetated foreshores for reducing wave loads on coastal dikes. Coast. Eng. 116, 42–56.
Walker, J., and Cooper, M. (2011). Genealogies of resilience from systems ecology to the political economy of crisis adaptation. Security Dial. 42, 143–160. doi: 10.1177/0967010611399616
Walters, C. J., and Holling, C. S. (1990). Large-scale management experiments and learning by doing. Ecology 71, 2060–2068. doi: 10.2307/1938620
Keywords: resilience, co-benefits, coastal management, natural infrastructure, risk
Citation: Kurth MH, Ali R, Bridges TS, Suedel BC and Linkov I (2020) Evaluating Resilience Co-benefits of Engineering With Nature® Projects. Front. Ecol. Evol. 8:149. doi: 10.3389/fevo.2020.00149
Received: 09 July 2019; Accepted: 04 May 2020;
Published: 01 June 2020.
Edited by:
Craig R. Allen, University of Nebraska–Lincoln, United StatesReviewed by:
Mauro Fois, University of Cagliari, ItalyRachel Kelley Gittman, East Carolina University, United States
Copyright © 2020 At least a portion of this work is authored by Margaret H. Kurth, Rahim Ali, Todd S. Bridges, Burton C. Suedel and Igor Linkov* on behalf of the U.S. Government and, as regards Dr. Kurth, Dr. Ali, Dr. Bridges, Dr. Suedel, and Dr. Linkov and the U.S. Government, is not subject to copyright protection in the United States. Foreign and other copyrights may apply. This is an open-access article distributed under the terms of the Creative Commons Attribution License (CC BY). The use, distribution or reproduction in other forums is permitted, provided the original author(s) and the copyright owner(s) are credited and that the original publication in this journal is cited, in accordance with accepted academic practice. No use, distribution or reproduction is permitted which does not comply with these terms.
*Correspondence: Igor Linkov, SWdvci5MaW5rb3ZAdXNhY2UuYXJteS5taWw=