- 1School of Natural Sciences, University of Tasmania, Hobart, TAS, Australia
- 2Menzies Institute for Medical Research, University of Tasmania, Hobart, TAS, Australia
- 3ARC Centre of Excellence for Australian Biodiversity and Heritage (CABAH), University of Tasmania, Hobart, TAS, Australia
Biodiverse environments contribute to human health through a wide range of ecosystem services: from providing food and medicines to filtering our air and water. Exposure to biodiverse, airborne microbial communities (aerobiomes) contributes to the development of healthy human-immune function. This overlooked but potentially powerful ecosystem service is akin to nature’s provision of traditional medicines and pharmaceutical compounds. But urban environments appear to support less diverse aerobiomes, suppressing this ecosystem service and potentially contributing to urban-associated diseases through altered immune function. Here, we synthesize the known relationships between aerobiome biodiversity and health and present the experimentally demonstrated mechanisms that connect aerobiome exposure to immune function. We then summarize what is currently known about the effect of urbanization on aerobiomes and identify several important knowledge gaps in this field, including a lack of rigorous, experimental, multi-scale studies demonstrating the mechanistic pathways between urbanization, altered aerobiomes and human health. We offer practical approaches that can close these knowledge gaps and will facilitate the transfer of knowledge and technology between microbiologists, urban ecologists and public-health practitioners. This synthesis should stimulate interdisciplinary research efforts to advance our understanding of how urbanization is impacting aerobiome ecosystem services, and what that means for human health.
Background
Biodiverse ecosystems provide health benefits across all categories of ecosystem services. Biodiverse environments supply provisioning services including food, fresh water, medicine, fiber and clean energy, and exciting research has been revealing the powerful health benefits of supporting, regulating and cultural ecosystem services as well. For example, biodiverse communities can filter pollutants and toxins from our air and water, and mitigate flooding, climate change and infectious disease transmission (MEA, 2005). Interacting with biodiverse environments also provides opportunities for socialization, cognitive restoration and physical activity that have cascading effects on physical, cognitive, and emotional health (Aerts et al., 2018; Lai et al., 2019).
An emerging field examines the way microbial communities (e.g., bacteria, fungi, protozoans, and viruses) move across biomes and in particular, how humans acquire microbes from the natural environment (Clarke et al., 2020). This exchange has direct implications for human health because of the intimate relationship between the microbiome and multiple health outcomes. For example, our own internal microbiomes have been linked to diseases as disparate as depression (Foster and McVey Neufeld, 2013), irritable bowel disease (Knights et al., 2013), and autism (Lammert et al., 2018). Internal microbiomes are strongly influenced by the environmental microbial communities to which we are exposed (e.g., through food, soil, air) (Braun-Fahrländer et al., 2002; Ege et al., 2011; Claesson et al., 2012; Liddicoat et al., 2020), and thus environmental microbiome exposure has important implications for human health outcomes. Aerial microbiomes, or aerobiomes, are particularly important as our skin, nasal, oral and respiratory surfaces are constantly exposed to the cocktail of microorganisms present in the atmosphere; people living in cities inhale approximately 108 microbial genomes every day (Després et al., 2007), with direct implications for the health of urban residents.
Urban environments typically have limited vegetated areas (“green spaces”), and altered and degraded ecosystems which are less able to provide ecosystem services (MEA, 2005). This loss of ecosystem services has direct and indirect effects on human health (Figure 1). In cities, for example, reduced regulating services contributes to higher levels of air pollution, and less green spaces means less opportunities for outdoor recreation (a cultural service). These reduced ecosystem services also impact biotic communities with indirect impacts on health. Cities can have a homogenizing impact on animal (Johnston et al., 2014; Concepción et al., 2015; Morelli et al., 2016) and microbial communities (Alenius et al., 2009; Schnorr et al., 2014; Qian et al., 2017; Flies et al., 2020) though the effect on plants is less clear (Concepción et al., 2015; Groffman et al., 2017; Kondratyeva et al., 2020). Exposure to less biodiverse microbial communities appears to negatively impact immune development and has been tied to increased levels of allergies and asthma and other urban-associated diseases (Ege et al., 2011; Hanski et al., 2012; Rook, 2013; Ruokolainen et al., 2015; von Hertzen et al., 2015; Flies et al., 2019). It is likely that reduced diversity in aerial microbiome exposure is a key component of this phenomenon; however, this remains understudied because aerial microbiomes are harder to measure than the microbes of living organisms, surfaces, soil or water. If we are to protect sensitive populations from the impacts of reduced diversity in urban aerial microbiomes (UAMs), and design cities to better maintain this ecosystem service, we require a much stronger understanding of how cities affect aerial microbiota and what that means for human health.
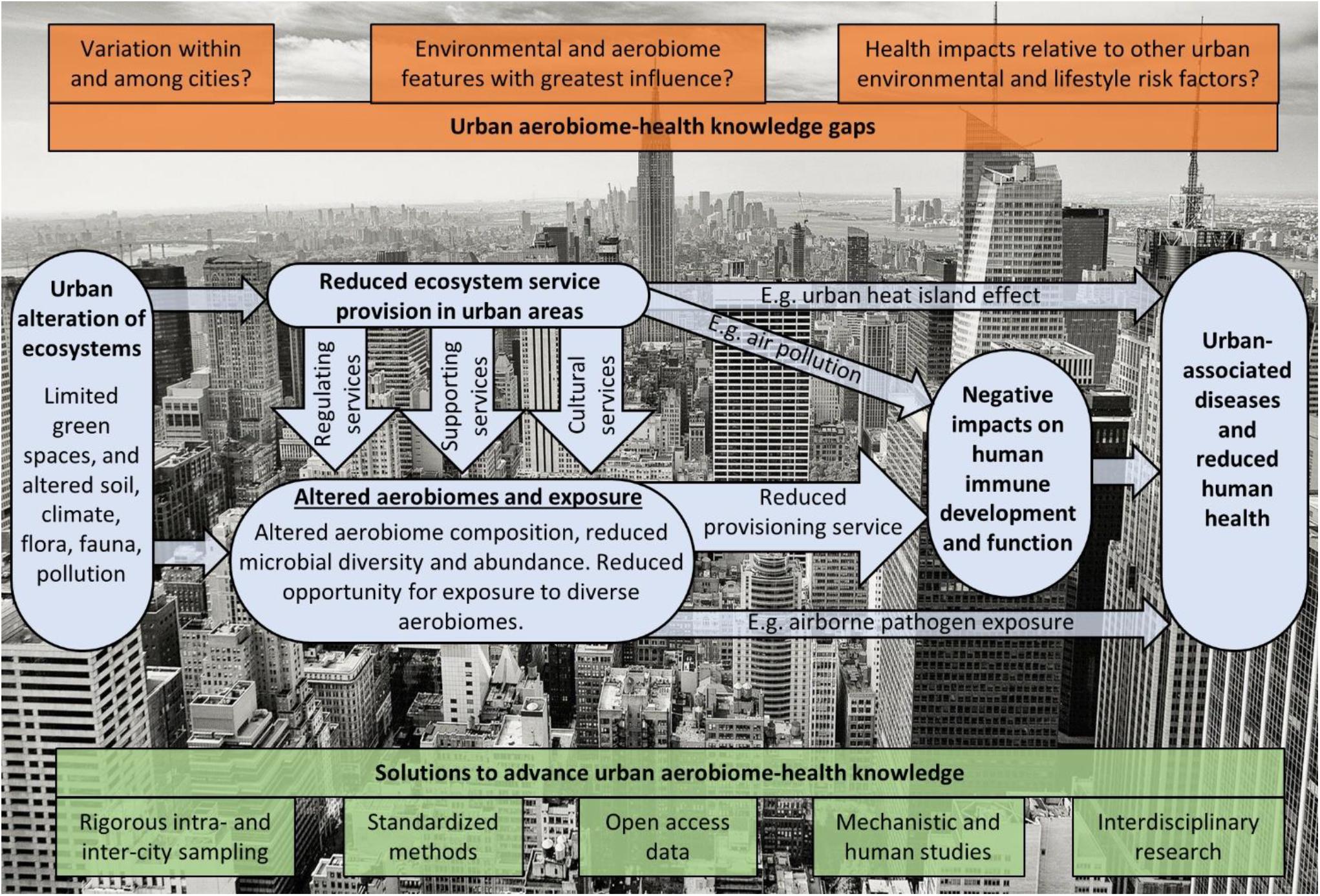
Figure 1. A conceptual diagram of how urbanization alters ecosystem services, aerobiomes and human health. Urban areas generally have less vegetation (green spaces) and altered ecosystems that result in reductions to many ecosystem services with direct and indirect impacts on health. Both reductions in regulating services (e.g., resulting in reduced air quality/greater air pollution) and supporting services (e.g., resulting in reduced maintenance of genetic diversity) impact the composition, and microbial diversity and abundance of aerobiomes. Additionally, less biodiverse and/or less extensive green spaces in cities limits outdoor recreation opportunities (a cultural ecosystem service). This reduced exposure to biodiverse aerobiomes for urban-dwelling people stifles the provisioning service that biodiverse microbiome exposure has on healthy immune development and function, leading to poor health outcomes. We highlight knowledge gaps in red and potential solutions to advance this field in green.
The last few decades have brought a revolution in microbiome techniques, which provide a promising avenue for advancing understandings and applications of biodiverse aerial microbiome exposure to improve human immune function and health. However, aerial microbiome studies have been published disparately in climate, ecology, medical, or occupational health and safety journals, among others, and these divergent fields have been developing knowledge and technical advances separately. There is a clear need for increased awareness of the important linkages between aerobiome composition, urbanization and human health as an ecosystem service to stimulate research activity and to ensure aerobiomes’ inclusion in urban design. We seek to contribute to that effort through a synthesis of the knowledge and gaps in (1) aerial microbiome impacts on human health, (2) urbanization impacts on aerial microbiomes, (3) urban aerial microbiome (UAM) methodology, and (4) how future UAM studies can better resolve these knowledge gaps.
Aerial Microbiomes and Human Health: Knowledge and Gaps
Clearly, contact with airborne pathogens can cause disease (Kim et al., 2018): SARS-CoV-2, influenza, anthrax, tuberculosis and other pathogens can be airborne. Furthermore, exposure to high levels of specific fungi or bacteria can contribute to allergies (Jaakkola et al., 2010), asthma (Karvala et al., 2010; Tham et al., 2014 and citations therein) and systemic disease (Cronholm, 1980; Rylander, 2006; Smit et al., 2006).
However, there is emerging experimental evidence that, in contrast to the effect of exposure to microbial communities with high levels of specific pathogenic or allergenic microbes, exposure to biodiverse microbial communities is an important ecosystem service that can reduce allergy, asthma and inflammatory responses. The concept that biodiversity benefits immune development is called the biodiversity hypothesis (Hanski et al., 2012; Rook, 2013; von Hertzen et al., 2015, 2011). A growing body of research has now established support for a linkage between exposure to diverse soil microbiomes and healthy immune system development (Wall et al., 2015; Li et al., 2018) and some of the mechanisms involved. For example, Ottman et al. (2018) demonstrated that soil-acquired microbes alleviate TH2-driven inflammation, a key aspect of allergic diseases. Similar mechanistic pathways are likely to operate with respect to aerobiome exposure (Hanski et al., 2012; Rook, 2013), particularly given the intimate connections between soil microbiome and aerial microbiome composition (Barberán et al., 2015; Mhuireach et al., 2016).
The mechanistic connections between aerial microbial diversity and immune function remain much less studied than those for the soil microbiota but are beginning to be clarified through experimental studies. Table 1 presents some of the immune mechanisms demonstrated to be altered by the diversity and/or abundance of aerobiome exposure. To date, the number of studies testing mechanistic linkages between aerobiome exposure and immune response is small, and most use in vitro or in-mouse models. Furthermore, most use collected or settled dust (from bedrooms or mattresses) as a proxy for airborne dusts and the affiliated microbes. While this has been demonstrated to be an indicative proxy for environmental exposure to airborne microbes (Ege et al., 2011), more precise exposure information could be acquired with filtered personal sampling devices (Wang et al., 2015) or real-time testing of aerobiomes (Nasir et al., 2019) would help link microbial exposure with the immune system response. Nonetheless, the evidence to date clearly demonstrates connections between characteristics of the aerial microbiome (e.g., the diversity and/or abundance of microbes) and immune system proxies that are consistent with a protective immune system effect.
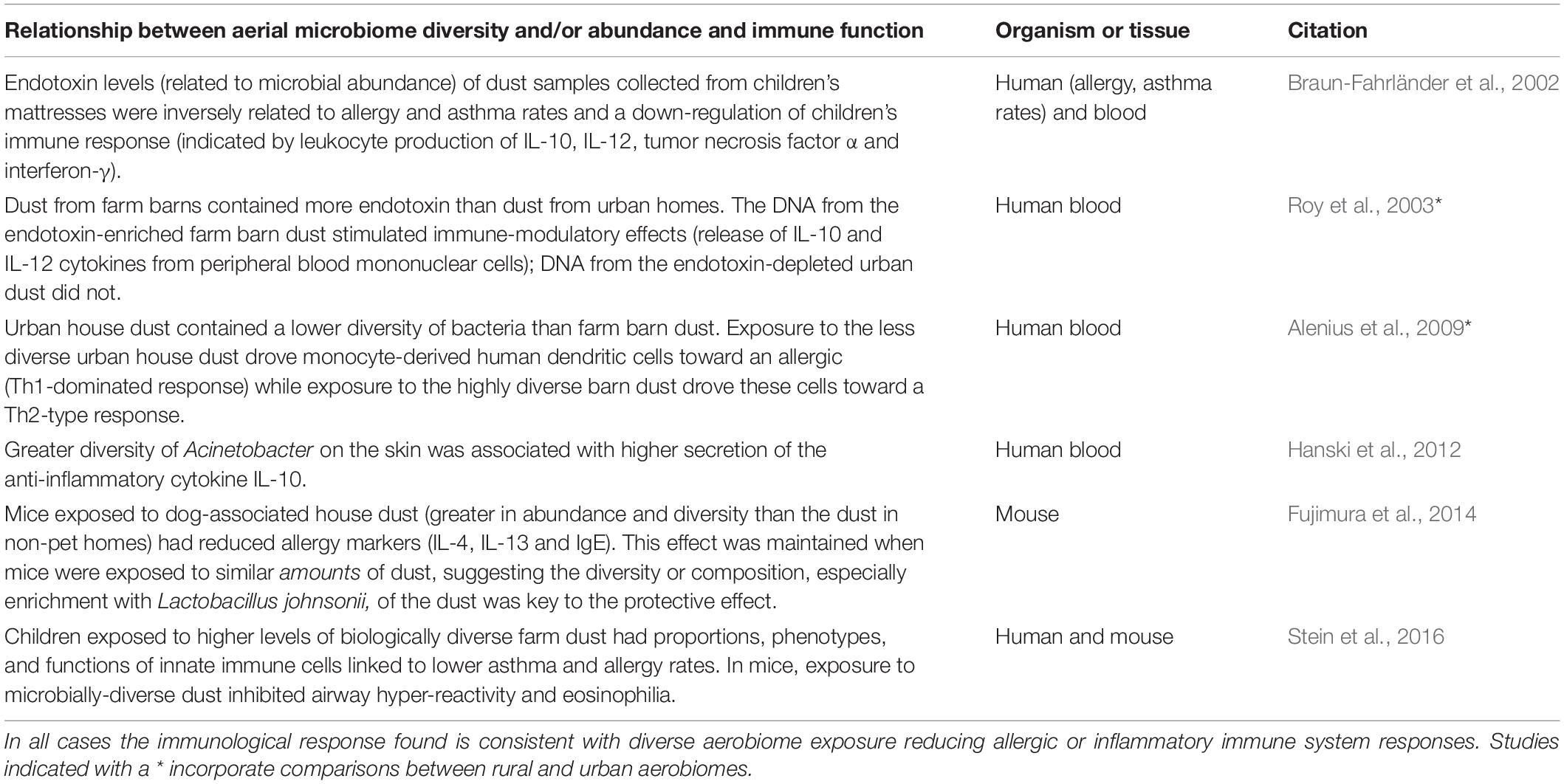
Table 1. Selected experimental studies demonstrating the immunological effect of exposure to diverse aerial microbial communities in mice and humans.
However, the characteristics of airborne microbial communities are shaped by the environment and cities appear to be a homogenizing force for aerial and human microbiomes (Alenius et al., 2009; Schnorr et al., 2014; Ruokolainen et al., 2015; Ying et al., 2015; Qian et al., 2017; Flies et al., 2020). In line with biodiversity hypothesis predictions, cities have higher rates of allergies, asthma and other immune dysfunction diseases (Flies et al., 2019). The few experimental studies comparing how human immune function responds differently to urban and rural aerial microbiomes (a subset of those in Table 1) have found UAM exposure to drive immune function toward a more allergic response (Roy et al., 2003; Alenius et al., 2009). Specifically, Roy et al. (2003) found that dust from urban homes had lower endotoxin levels (associated with bacteria) than dust from rural homes, and that this led to altered immune-modulatory effects. Alenius et al. (2009) reported that exposure to (less biodiverse) urban house dust drove monocyte-derived human dendritic cells toward an allergic (Th1-dominated response), while exposure to (more biodiverse) farm barn dust drove these cells toward a Th2-type response. These outcomes reinforce that exposure to biodiverse airborne microbiomes, such as those typically found in rural areas, can support healthy (i.e., less allergic, less inflammatory) immune function.
Overall, these studies suggest that diverse aerobiome exposure is an important ecosystem service with direct implications for human health. However, many knowledge gaps remain: the evidence base is limited and more research is needed to tease apart the impacts of aerobiome abundance and diversity, and the role of community composition. For example, is it specifically diversity that is important, or is it the species/genera that occur in biodiverse communities? We also need to know more about the importance of age and frequency of aerobiome exposure, “trade-offs” in the potentially positive and negative health impacts of exposure to some aerobiome components, and how urban environmental factors like air pollutants interact with aerobiomes. All of these questions must be answered before these concepts can be applied to support urban design for healthy aerobiome exposure.
How Urbanisation Impacts Aerial Microbiomes: Knowledge and Gaps
Land use has a strong impact on aerial-microbiome composition, which changes substantially with urbanization. In urban locations, aerial bacteria include species common in soils and plants as well as those associated with important human pathogens: Actinobacteria, Proteobacteria, Acidobacteria, Cyanobacteria (which includes chloroplasts), Firmicutes, and Burkholderiales among others are all found in urban air (Shaffer and Lighthart, 1997; Brodie et al., 2007; Bowers et al., 2011a,b; Bertolini et al., 2013). However, UAMs are neither static nor uniform, adding complexity to efforts to unpack exactly how, where and why urbanization impacts the aerobiome and thus the ecosystem services it can provide.
Spatially, UAMs can vary at fine scales (<50 m) due to differences in land use and land cover (Shaffer and Lighthart, 1997; Chandra Mouli et al., 2005; Lymperopoulou et al., 2016; Mhuireach et al., 2016). Each microbial component of the UAM has a distinct source: for example, soil, leaf surfaces, man-made materials, and feces in urban parks contribute to the UAM and these sources vary at fine scales across a cityscape (Bowers et al., 2011b; Lymperopoulou et al., 2016; Mhuireach et al., 2016). For example, parking lots can have substantially more Acetobacteraceae, which oxidize sugar and ethanol to acid, than urban parks, which have more Acidobacteriaceae, a common soil microbe – although the human health implications of this difference remain unclear (Mhuireach et al., 2016). The intra-city distribution of vegetation also has an important effect; aerial microbial and fungal communities that exist above vegetated areas are distinct from those above non-vegetated urban areas, even at a proximity of 50 m (Adams et al., 2013; Mhuireach et al., 2016). Overall, urban land use thus strongly mediates both urban aerobiome diversity and ecosystem service provision at fine intra-city spatial scales.
Weather and season also have a major influence on aerial microbiomes (Brodie et al., 2007; Bowers et al., 2011a,b; Woo et al., 2013; Rathnayake et al., 2017). In particular, total fungal and bacterial counts are often higher after rain events (Chandra Mouli et al., 2005) and during the summer (Bertolini et al., 2013; Niazi et al., 2015) or autumn (fungi; Frohlich-Nowoisky et al., 2009), compared to the winter in temperate environments. However, despite the documented importance of temporal variation, many UAM studies either leave it unaddressed, or poorly account for it in their study design, and thus the implications for ecosystem services remains a key gap in UAM research.
Importantly, despite significant inter-site and temporal variability, UAMs appear to be, in general, less diverse than rural aerial microbiomes (Flies et al., 2020): for example, one study of aerial bacterial diversity in samples of fine particulate matter reported a Shannon-Weaver diversity index of 1.07 for urban samples compared to 1.25 for rural aerial samples (Després et al., 2007). Microbial abundance also varies across habitats within cities, between cities, and between urban and natural areas (Shaffer and Lighthart, 1997; Burrows et al., 2009; Lymperopoulou et al., 2016).
Overall, the evidence suggests that urbanization leads to (a) less diverse UAMs, regardless of whether the metric used is richness and/or a diversity index (Flies et al., 2020); and (b) altered composition. With respect to the latter, there is some evidence that UAMs tend to have fewer bacterial taxa that have been associated with protective immune effects (e.g., Vestergaard et al., 2018); however, we lack sufficient evidence to make robust generalizations about compositional trends. With respect to both diversity and composition, many questions remain about how, where and why these effects are manifested. Addressing these gaps with rigorous multi-scale, eco-epidemiological studies (Flies et al., 2018b) will be vital if we are to understand how to protect UAM ecosystem services.
In summary, UAM composition is strongly dependent on the different ways that urbanization impacts (a) soil, (b) plant and animal communities, (c) climate (e.g., urban heat island effect), (d) the abundance of soil, water and man-made objects (e.g., concrete) exposed to the air and (e) the amount and size of particulate matter in the air. However, because of the spatial and temporal complexity within and among cities, it is not yet clear the precise effects these components of urbanization have on UAM composition and therefore health. As we describe in the following section, this is further confounded by the diversity of methods used to collect, process and analyze UAM samples. This will be important to clarify so urban design can promote UAMs that contribute to healthier resident human populations.
Unifying UAM Methodology to Fill Knowledge Gaps
Sample Collection
Achieving a robust, systematic and representative sampling regime has been – and remains - a core challenge in characterizing and determining the drivers of UAM diversity and composition in a way that enables application for healthy urban design. The extent of the challenge derives from the size range and morphological diversity of the microbes, their vulnerability to sampling damage, and the spatial and temporal variability of UAMs. Together, these factors mean that there is no single optimal sampling method that is appropriate for all aerial microbiome studies. Instead, there has been a proliferation of sampling devices, each of which have their own advantages, disadvantages and limitations.
Aerial microbiome samplers (typically termed bioaerosol samplers) can be categorized into several main functional types (Supplementary Figure S1, see Ghosh et al., 2015; Haig et al., 2016; Lindsley et al., 2017 for comprehensive overviews). Within each functional type, samplers tend to have a set of common advantages and limitations (see Supplementary Table S1). For example, filters tend to have high collection efficiencies, but poor potential for the maintenance of biological viability. However, even within categories, the available samplers are highly diverse and can have variable sampling characteristics (such as flow rate, collection efficiency, and collection medium; Kesavan and Sagripanti, 2015; Wang et al., 2015; Haig et al., 2016). A comprehensive list of bioaerosol samplers, together with their particle size range and application suitability (culture, microscopy or immunological/chemical/other assay) is provided in Lindsley et al. (2017).
Sampler choice will strongly affect the outcomes of aerial microbiome studies. Results from studies using different samplers are not easily comparable, and in some cases inappropriate sampler choice may render a study’s conclusions invalid. This is particularly important in the context of infectious disease monitoring; Kesavan and Sagripanti (2015), for example, show that inadequate sampler selection can result in a serious underestimate of risk. Although sampling technologies are continuing to advance and evolve, standardization remains unlikely given that sampler choice will remain guided by study design and aims. However, the obstacle of different sample collection techniques for understanding UAMs for ecosystem service provision can be minimized if techniques for downstream genetic and statistical analysis are unified and data sharing is implemented.
Genetic Analysis
Traditionally, all bioaerosol studies were done using culture and/or microscopic identification. Today, unless specifically interested in the live bacteria/fungi (e.g., Urbaniak et al., 2018), genetic techniques better demonstrate the diversity of microbial communities (Mbareche et al., 2017). However, the method of DNA extraction can have a significant impact on the microorganisms that get amplified and therefore appear to characterize the community (Willner et al., 2012; Mbareche et al., 2017). For example, extraction kits that use a mechanical bead-beating step, rather than purely enzymatic and chemical lysis, have been found to improve detection of more resilient microbes (e.g., Staphylococcus, Streptococcus) and pollen in samples (Willner et al., 2012) which is beneficial for UAM studies. During the gene amplification and sequencing, most studies use the same gene regions to search for the species and communities of interest (e.g., 16S for bacteria/archaea, ITS for fungi etc.) but the segment of the gene amplified varies, as do the primers used, and sequencing read depths, all of which can influence apparent community composition (Jovel et al., 2016). For maximum comparative potential, we suggest a unification of genetic methods, for example using the detailed online protocols from the Earth Microbiome Project (Gilbert et al., 2014) and adhering to Caporaso et al. (2012) for standard sequencing methodology.
Statistical Analysis of UAM Communities
Another challenge of UAM studies is the diversity of statistical ways to characterize microbial communities, and the drivers shaping them. Studies designed to compare microbial communities often use a combination of simple community metrics (e.g., alpha and beta diversity), phylogenetic distances (e.g., UniFrac), statistical models, and visualizations.
If genetic techniques are used, the phylogenetic relatedness of “species” [i.e., operational taxonomic units (OTUs), or amplicon sequence variants (ASVs)] within a community can be calculated and different communities can be compared using UniFrac distinctness or the Bray-Curtis Dissimilarity index. There are also many approaches for visualizing compositional similarities across communities, typically underpinned by cluster-based ordination approaches like non-metric multidimensional scaling (NMDS; Kembel et al., 2012), principal components analysis (PCA; Bertolini et al., 2013) and constrained analysis of principle coordinates (CAP; Mhuireach et al., 2016) to group/split sites along multiple (dis)similarity axes. Some of these statistical models can uncover environmental effects on compositional differences (Erb-Downward et al., 2012; Mhuireach et al., 2016).
The challenge in this field is then comparing across studies; simple abundance and diversity metrics can be easy to compare but they (or especially their errors and sample sizes, required for formal meta-analysis) are not always reported and are influenced by the bioinformatics pipeline used. Accurately comparing communities across studies or locations would require access to the complete metagenomic data used to calculate community metrics. These sorts of big-data comparisons are only possible when all metagenomic data is made open access, as is done with the Earth Microbiome Project (Gilbert et al., 2014) and the Biomes of Australian Soil Environments (Bissett et al., 2016). We encourage other researchers to make their data available through these platforms for across-study comparisons and a clarification of general trends.
Future Directions and Conclusion
Exposure to biodiverse aerial microbial communities can provide an under-recognized ecosystem service for human health. Urbanization appears to be reducing the diversity of airborne microbes in cities (Flies et al., 2020), with potentially important impacts on asthma, allergies and urban-associated diseases (Flies et al., 2019). Fortunately, the actions that could increase the biodiversity of UAMs and human exposure to them rely on increasing urban biodiversity overall and human interaction with urban green spaces, both of which have co-benefits for many urban ecosystem services and health. Actions that could improve exposure to biodiverse UAMs include the creation of biodiverse urban green spaces (Flies et al., 2017, 2018a), restoration of urban habitat (Mills et al., 2017), and public health policies that encourage active use of biodiverse urban green spaces. Given the intimate linkages between the soil and aerial microbiomes, specific efforts to cultivate diverse soil microbiota in both public and private urban green spaces could provide further benefits (Wall et al., 2015; Li et al., 2018). All of these policies are likely to be highly beneficial, though we note that much more research into the linkages between urbanization and UAM composition is needed if we are to develop specific policies to support UAM ecosystem service provision.
With this article we intend to raise awareness about the role of airborne microbiomes as an ecosystem service for health to facilitate a closing of the knowledge gaps in this field (Figure 1). We have outlined how exposure to different types of aerial microbial communities influence immune function in mice and in humans, with microbial diversity as an important link (Ege et al., 2011; Hanski et al., 2012). However, whether it is the diversity of the microbes per se or the species or (functional) families of microbes contained in biodiverse microbiomes, or if biodiversity is an indicator of other characteristics of the microbiome is yet unclear. We encourage studies investigating the features of urban landscapes that influence UAM biodiversity and composition, and further examination of how various features of aerial microbiomes impact human health.
Differences in methodology have made it difficult to make inter-study comparisons of microbial communities to understand how communities differ across space, time and methodologies and to connect those differences with human health. Along with further studies that compare aerial microbiomes across a diversity of land uses, geographies and urban regions, while controlling for critical confounding variables like weather, it is crucial that studies make their data open access so as to facilitate cross-study comparisons using a common suite of standardized analytical methods and bioinformatics pipelines (Figure 1).
Importantly, though microbial diversity appears to benefit immune function (Ege et al., 2011; Hanski et al., 2012; Ruokolainen et al., 2015; Stein et al., 2016), this is unrecognized in many aerial microbiome fields and diversity is often not reported in analyses. Further experimental studies are needed to clarify how microbial abundance and microbiome composition impact human health and the immunological mechanisms driving those health effects. We encourage studies testing the impacts of exposure to UAMs with specific characteristics (microbial abundance, diversity, and community compositions) on immune characteristics in mouse models and human tissues. In order to best clarify the mechanisms involved, we recommend that such studies test the impact of targeted features of the aerobiome, rather than (or in addition to) simply comparing immune responses to urban and rural UAM samples. The specific characteristics of UAMs that support healthy immune system function are critical to clarify if we want to foster biodiverse UAMs that have a positive impact on health.
Author Contributions
EF conceptualized the manuscript and led the research and writing with PJ. EF, PJ, JB, and BB all contributed to writing, researching and editing the manuscript. All authors contributed to the article and approved the submitted version.
Funding
This work was funded by Australian Research Council grant FL160100101.
Conflict of Interest
The authors declare that the research was conducted in the absence of any commercial or financial relationships that could be construed as a potential conflict of interest.
Acknowledgments
We would like to acknowledge the support of the Australian Research Council for this work.
Supplementary Material
The Supplementary Material for this article can be found online at: https://www.frontiersin.org/articles/10.3389/fevo.2020.568902/full#supplementary-material
References
Adams, R. I., Miletto, M., Taylor, J. W., and Bruns, T. D. (2013). Dispersal in microbes: fungi in indoor air are dominated by outdoor air and show dispersal limitation at short distances. ISME J. 7, 1262–1273. doi: 10.1038/ismej.2013.28
Aerts, R., Honnay, O., and Van Nieuwenhuyse, A. (2018). Biodiversity and human health: mechanisms and evidence of the positive health effects of diversity in nature and green spaces. Br. Med. Bull. 127, 5–22. doi: 10.1093/bmb/ldy021
Alenius, H., Pakarinen, J., Saris, O., Andersson, M. A., Leino, M., Sirola, K., et al. (2009). Contrasting immunological effects of two disparate dusts - Preliminary observations. Int. Arch. Allergy Immunol. 149, 81–90. doi: 10.1159/000176310
Barberán, A., Ladau, J., Leff, J. W., Pollard, K. S., Menninger, H. L., Dunn, R. R., et al. (2015). Continental-scale distributions of dust-associated bacteria and fungi. Proc. Natl. Acad. Sci. U.S.A. 112, 5756–5761. doi: 10.1073/pnas.1420815112
Bertolini, V., Gandolfi, I., Ambrosini, R., Bestetti, G., Innocente, E., Rampazzo, G., et al. (2013). Temporal variability and effect of environmental variables on airborne bacterial communities in an urban area of Northern Italy. Appl. Microbiol. Biotechnol. 97, 6561–6570. doi: 10.1007/s00253-012-4450-0
Bissett, A., Fitzgerald, A., Meintjes, T., Mele, P. M., Reith, F., Dennis, P. G., et al. (2016). Introducing BASE: the biomes of australian soil environments soil microbial diversity database. Gigascience 5:11. doi: 10.1186/s13742-016-0126-5
Bowers, R. M., McLetchie, S., Knight, R., and Fierer, N. (2011a). Spatial variability in airborne bacterial communities across land-use types and their relationship to the bacterial communities of potential source environments. ISME J. 5, 601–612. doi: 10.1038/ismej.2010.167
Bowers, R. M., Sullivan, A. P., Costello, E. K., Collett, J. L., Knight, R., and Fierer, N. (2011b). Sources of bacteria in outdoor air across cities in the midwestern United States. Appl. Environ. Microbiol. 77, 6350–6356. doi: 10.1128/AEM.05498-11
Braun-Fahrländer, C., Riedler, J., Herz, U., Eder, W., Waser, M., Grize, L., et al. (2002). Environmental exposure to endotoxin and its relation to asthma in school-age children. N. Engl. J. Med. 347, 869–877. doi: 10.1056/NEJMoa020057
Brodie, E. L., DeSantis, T. Z., Parker, J. P. M., Zubietta, I. X., Piceno, Y. M., and Andersen, G. L. (2007). Urban aerosols harbor diverse and dynamic bacterial populations. Proc. Natl. Acad. Sci. U.S.A. 104, 299–304. doi: 10.1073/pnas.0608255104
Burrows, S. M., Elbert, W., Lawrence, M. G., and Pöschl, U. (2009). Bacteria in the global atmosphere – Part 1: review and synthesis of literature data for different ecosystems. Atmos. Chem. Phys. 9, 9263–9280.
Caporaso, J. G., Lauber, C. L., Walters, W. A., Berg-Lyons, D., Huntley, J., Fierer, N., et al. (2012). Ultra-high-throughput microbial community analysis on the Illumina HiSeq and MiSeq platforms. ISME J. 6, 1621–1624. doi: 10.1038/ismej.2012.8
Chandra Mouli, P., Venkata Mohan, S., and Jayarama Reddy, S. (2005). Assessment of microbial concentrations of ambient air at semi-arid urban region: influence of meteorological factors. Environ. Res. 3, 139–149.
Claesson, M. J., Jeffery, I. B., Conde, S., Power, S. E., O’Connor, E. M., Cusack, S., et al. (2012). Gut microbiota composition correlates with diet and health in the elderly. Nature 488, 178–184. doi: 10.1038/nature11319
Clarke, L. J., Jones, P. J., Ammitzboll, H., Barmuta, L. A., Breed, M. F., Chariton, A., et al. (2020). Mainstreaming microbes across biomes. Bioscience 70, 589–596. doi: 10.1093/biosci/biaa057
Concepción, E. D., Moretti, M., Altermatt, F., Nobis, M. P., and Obrist, M. K. (2015). Impacts of urbanisation on biodiversity: the role of species mobility, degree of specialisation and spatial scale. Oikos 124, 1571–1582. doi: 10.1111/oik.02166
Cronholm, L. S. (1980). Potential health hazards from microbial aerosols in densely populated urban regions. Appl. Environ. Microbiol. 39, 6–12.
Després, V., Nowoisky, J., Klose, M., Conrad, R., Andreae, M. O., and Poschl, U. (2007). Characterization of primary biogenic aerosol particles in urban, rural, and high-alpine air by DNA sequence and restriction fragment analysis of ribosomal RNA genes. Biogeosciences 4, 1127–1141. doi: 10.5194/bg-4-1127-2007
Ege, M. J., Mayer, M., Normand, A.-C., Genuneit, J., Cookson, W. O. C. M., Braun-Fahrländer, C., et al. (2011). Exposure to environmental microorganisms and childhood asthma. N. Engl. J. Med. 364, 701–709. doi: 10.1056/NEJMoa1007302
Erb-Downward, J. R., Sadighi Akha, A. A., Wang, J., Shen, N., He, B., Martinez, F. J., et al. (2012). Use of direct gradient analysis to uncover biological hypotheses in 16S survey data and beyond. Sci. Rep. 2:774. doi: 10.1038/srep00774
Flies, E. J., Clarke, L. J., Brook, B. W., and Jones, P. (2020). Urbanisation reduces the abundance and diversity of airborne microbes - but what does that mean for our health? A systematic review. Sci. Total Environ. 738:140337. doi: 10.1016/j.scitotenv.2020.140337
Flies, E. J., Mavoa, S., Zosky, G. R., Mantzioris, E., Williams, C., Eri, R. D., et al. (2019). Urban-associated diseases: candidate diseases, environmental risk factors, and a path forward. Environ. Int. 133:105187.
Flies, E. J., Skelly, C., Lovell, R., Breed, M. F., Phillips, D., and Weinstein, P. (2018a). Cities, biodiversity and health: we need healthy urban microbiome initiatives. Cities Health 2, 143–150. doi: 10.1080/23748834.2018.1546641
Flies, E. J., Weinstein, P., Anderson, S. J., Koolhof, I., Foufopoulos, J., and Williams, C. R. (2018b). Ross river virus and the necessity of multiscale, eco-epidemiological analyses. J. Infect. Dis. 217, 807–815. doi: 10.1093/infdis/jix615
Flies, E. J., Skelly, C., Negi, S. S., Prabhakaran, P., Liu, Q., Liu, K., et al. (2017). Biodiverse green spaces: a prescription for global urban health. Front. Ecol. Environ. 15, 510–516. doi: 10.1002/fee.1630
Foster, J. A., and McVey Neufeld, K. A. (2013). Gut-brain axis: how the microbiome influences anxiety and depression. Trends Neurosci. 36, 305–312. doi: 10.1016/j.tins.2013.01.005
Frohlich-Nowoisky, J., Pickersgill, D. A., Despres, V. R., and Poschl, U. (2009). High diversity of fungi in air particulate matter. Proc. Natl. Acad. Sci. U.S.A. 106, 12814–12819.
Fujimura, K. E., Demoor, T., Rauch, M., Faruqi, A. A., Jang, S., Johnson, C. C., et al. (2014). House dust exposure mediates gut microbiome Lactobacillus enrichment and airway immune defense against allergens and virus infection. Proc. Natl. Acad. Sci. U.S.A. 111, 805–810. doi: 10.1073/pnas.1310750111
Ghosh, B., Lal, H., and Srivastava, A. (2015). Review of bioaerosols in indoor environment with special reference to sampling, analysis and control mechanisms. Environ. Int. 85, 254–272. doi: 10.1016/j.envint.2015.09.018
Gilbert, J. A., Jansson, J. K., and Knight, R. (2014). The earth microbiome project: successes and aspirations. BMC Biol. 12:69. doi: 10.1186/s12915-014-0069-1
Groffman, P. M., Avolio, M., Cavender-Bares, J., Bettez, N. D., Grove, J. M., Hall, S. J., et al. (2017). Ecological homogenization of residential macrosystems. Nat. Ecol. Evol. 1:191. doi: 10.1038/s41559-017-0191
Haig, C. W., Mackay, W. G., Walker, J. T., and Williams, C. (2016). Bioaerosol sampling: sampling mechanisms, bioefficiency and field studies. J. Hosp. Infect. 93, 242–255. doi: 10.1016/j.jhin.2016.03.017
Hanski, I., Von Hertzen, L., Fyhrquist, N., Koskinen, K., Torppa, K., Laatikainen, T., et al. (2012). Environmental biodiversity, human microbiota, and allergy are interrelated. Proc. Natl. Acad. Sci. U.S.A. 109, 8334–8339. doi: 10.1073/pnas.1205624109
Jaakkola, J. J. K., Hwang, B.-F., and Jaakkola, M. S. (2010). Home dampness and molds as determinants of allergic rhinitis in childhood: a 6-year, population-based cohort study. Am. J. Epidemiol. 172, 451–459. doi: 10.1093/aje/kwq110
Johnston, E., Weinstein, P., Slaney, D., Flies, A. S., Fricker, S., and Williams, C. (2014). Mosquito communities with trap height and urban-rural gradient in Adelaide, South Australia: implications for disease vector surveillance. J. Vect. Ecol. 39, 48–55. doi: 10.1111/j.1948-7134.2014.12069.x
Jovel, J., Patterson, J., Wang, W., Hotte, N., O’Keefe, S., Mitchel, T., et al. (2016). Characterization of the gut microbiome using 16S or shotgun metagenomics. Front. Microbiol. 7:459. doi: 10.3389/fmicb.2016.00459
Karvala, K., Toskala, E., Luukkonen, R., Lappalainen, S., Uitti, J., and Nordman, H. (2010). New-onset adult asthma in relation to damp and moldy workplaces. Int. Arch. Occup. Environ. Health 83, 855–865. doi: 10.1007/s00420-010-0507-5
Kembel, S. W., Jones, E., Kline, J., Northcutt, D., Stenson, J., Womack, A. M., et al. (2012). Architectural design influences the diversity and structure of the built environment microbiome. ISME J. 6, 1469–1479. doi: 10.1038/ismej.2011.211
Kesavan, J., and Sagripanti, J. L. (2015). Evaluation criteria for bioaerosol samplers. Environ. Sci. Process. Impacts 17, 638–645. doi: 10.1039/c4em00510d
Kim, K. H., Kabir, E., and Jahan, S. A. (2018). Airborne bioaerosols and their impact on human health. J. Environ. Sci. 67, 23–35. doi: 10.1016/j.jes.2017.08.027
Knights, D., Lassen, K. G., and Xavier, R. J. (2013). Advances in inflammatory bowel disease pathogenesis: linking host genetics and the microbiome. Gut 62, 1505–1510. doi: 10.1136/gutjnl-2012-303954
Kondratyeva, A., Knapp, S., Durka, W., Kühn, I., Vallet, J., Machon, N., et al. (2020). Urbanization effects on biodiversity revealed by a two-scale analysis of species functional uniqueness vs. redundancy. Front. Ecol. Evol. 8:73. doi: 10.3389/fevo.2020.00073
Lai, H., Flies, E. J., Weinstein, P., and Woodward, A. (2019). The impact of green space and biodiversity on health. Front. Ecol. Environ. 17, 383–390. doi: 10.1002/fee.2077
Lammert, C. R., Frost, E. L., Bolte, A. C., Paysour, M. J., Shaw, M. E., Bellinger, C. E., et al. (2018). Cutting edge: critical roles for microbiota-mediated regulation of the immune system in a prenatal immune activation model of autism. J. Immunol. 201, 845–850. doi: 10.4049/jimmunol.1701755
Li, G., Sun, G. X., Ren, Y., Luo, X. S., and Zhu, Y. G. (2018). Urban soil and human health: a review. Eur. J. Soil Sci. 69, 196–215. doi: 10.1111/ejss.12518
Liddicoat, C., Sydnor, H., Cando-Dumancela, C., Dresken, R., Liu, J., Gellie, N. J. C., et al. (2020). Naturally-diverse airborne environmental microbial exposures modulate the gut microbiome and may provide anxiolytic benefits in mice. Sci. Total Environ. 701:134684. doi: 10.1016/j.scitotenv.2019.134684
Lindsley, W. G., Green, B. J., Blachere, F. M., Martin, S. B., Law, B. F., Jensen, P. A., et al. (2017). “Sampling and characterization of bioaerosols,” in NIOSH Manual of Analytical Methods, eds K. Ashley and P. F. O’Connor (Washington, DC: National Institute for Occupational Health and Safety), 82–112. doi: 10.1103/PhysRevB.71.165307
Lymperopoulou, D. S., Adams, R. I., and Lindow, S. E. (2016). Contribution of vegetation to the microbial composition of nearby outdoor air. Appl. Environ. Microbiol. 82, 3822–3833. doi: 10.1128/AEM.00610-16
Mbareche, H., Brisebois, E., Veillette, M., and Duchaine, C. (2017). Bioaerosol sampling and detection methods based on molecular approaches: No pain no gain. Sci. Total Environ. 599–600, 2095–2104. doi: 10.1016/j.scitotenv.2017.05.076
Mhuireach, G., Johnson, B. R., Altrichter, A. E., Ladau, J., Meadow, J. F., Pollard, K. S., et al. (2016). Urban greenness influences airborne bacterial community composition. Sci. Total Environ. 571, 680–687. doi: 10.1016/j.scitotenv.2016.07.037
Mills, J. G., Weinstein, P., Gellie, N. J. C., Weyrich, L. S., Lowe, A. J., and Breed, M. F. (2017). Urban habitat restoration provides a human health benefit through microbiome rewilding: the Microbiome rewilding hypothesis. Restor. Ecol. 25, 866–872. doi: 10.1111/rec.12610
Morelli, F., Benedetti, Y., Ibáñez-Álamo, J. D., Jokimäki, J., Mänd, R., Tryjanowski, P., et al. (2016). Evidence of evolutionary homogenization of bird communities in urban environments across Europe. Glob. Ecol. Biogeogr. 25, 1284–1293.
Nasir, Z. A., Hayes, E., Williams, B., Gladding, T., Rolph, C., Khera, S., et al. (2019). Scoping studies to establish the capability and utility of a real-time bioaerosol sensor to characterise emissions from environmental sources. Sci. Total Environ. 648, 25–32. doi: 10.1016/j.scitotenv.2018.08.120
Niazi, S., Hassanvand, M. S., Mahvi, A. H., Nabizadeh, R., Alimohammadi, M., Nabavi, S., et al. (2015). Assessment of bioaerosol contamination (bacteria and fungi) in the largest urban wastewater treatment plant in the Middle East. Env. Sci Pollut Res. 22, 16014–16021. doi: 10.1007/s11356-015-4793-z
Ottman, N., Ruokolainen, L., Suomalainen, A., Sinkko, H., Karisola, P., Lehtimäki, J., et al. (2018). Soil exposure modifies the gut microbiota and supports immune tolerance in a mouse model. J. Allergy Clin. Immunol. 39, 48–55. doi: 10.1016/J.JACI.2018.06.024
Qian, L., Xie, J., Zhou, D., Hu, Y., Zhu, L., Zhao, R., et al. (2017). Impact of rural and urban environmental microbial exposure on intestinal microbiota in early infancy. Nanosci. Nanotechnol. Lett. 9, 761–769. doi: 10.1166/nnl.2017.2283
Rathnayake, C. M., Metwali, N., Jayarathne, T., Kettler, J., Huang, Y., Thorne, P. S., et al. (2017). Influence of rain on the abundance of bioaerosols in fine and coarse particles. Atmos. Chem. Phys. 17, 2459–2475. doi: 10.5194/acp-17-2459-2017
Rook, G. A. W. (2013). Regulation of the immune system by biodiversity from the natural environment: an ecosystem service essential to health. Proc. Natl. Acad. Sci. U.S.A. 110, 18360–18367. doi: 10.1073/pnas.1313731110
Roy, S. R., Schiltz, A. M., Marotta, A., Shen, Y., and Liu, A. H. (2003). Bacterial DNA in house and farm barn dust. J. Allergy Clin. Immunol. 112, 571–578. doi: 10.1016/S0091-6749(03)01863-3
Ruokolainen, L., Hertzen, L., Von Fyhrquist, N., Laatikainen, T., Lehtom, J., Auvinen, P., et al. (2015). Green areas around homes reduce atopic sensitization in children. Allergy 70, 195–202. doi: 10.1111/all.12545
Rylander, R. (2006). Endotoxin and occupational airway disease. Curr. Opin. Allergy Clin. Immunol. 6, 62–66. doi: 10.1097/01.all.0000202356.83509.f7
Schnorr, S. L., Candela, M., Rampelli, S., Centanni, M., Consolandi, C., Basaglia, G., et al. (2014). Gut microbiome of the Hadza hunter-gatherers. Nat. Commun. 5:3654. doi: 10.1038/ncomms4654
Shaffer, B. T., and Lighthart, B. (1997). Survey of culturable airborne bacteria at four diverse locations in Oregon: urban, rural, forest, and coastal. Microb. Ecol. 34, 167–177. doi: 10.1007/s002489900046
Smit, L. A. M., Wouters, I. M., Hobo, M. M., Eduard, W., Doekes, G., and Heederik, D. (2006). Agricultural seed dust as a potential cause of organic dust toxic syndrome. Occup. Environ. Med. 63, 59–67. doi: 10.1136/oem.2005.021527
Stein, M. M., Hrusch, C. L., Gozdz, J., Igartua, C., Pivniouk, V., Murray, S. E., et al. (2016). Innate immunity and asthma risk in Amish and Hutterite farm children. N. Engl. J. Med. 375, 411–421. doi: 10.1056/NEJMoa1508749
Tham, R., Dharmage, S. C., Taylor, P. E., Katelaris, C. H., Vicendese, D., Abramson, M. J., et al. (2014). Outdoor fungi and child asthma health service attendances. Pediatr. Allergy Immunol. 25, 439–449. doi: 10.1111/pai.12257
Urbaniak, C., Sielaff, A. C., Frey, K. G., Allen, J. E., Singh, N., Jaing, C., et al. (2018). Detection of antimicrobial resistance genes associated with the international space station environmental surfaces. Sci. Rep. 8, 1–13. doi: 10.1038/s41598-017-18506-4
Vestergaard, D. V., Holst, G. J., Basinas, I., Elholm, G., Schlünssen, V., Linneberg, A., et al. (2018). Pig farmers’ homes harbor more diverse airborne bacterial communities than pig stables or suburban homes. Front. Microbiol. 9:870. doi: 10.3389/fmicb.2018.00870
von Hertzen, L., Beutler, B., Bienenstock, J., Blaser, M., Cani, P. D., Eriksson, J., et al. (2015). Helsinki alert of biodiversity and health. Ann. Med. 47, 1–8. doi: 10.3109/07853890.2015.1010226
von Hertzen, L., Hanski, I., and Haahtela, T. (2011). Natural immunity. Biodiversity loss and inflammatory diseases are two global megatrends that might be related. EMBO Rep. 12, 1089–1093. doi: 10.1038/embor.2011.195
Wall, D. H., Nielsen, U. N., and Six, J. (2015). Soil biodiversity and human health. Nature 528, 69–76. doi: 10.1038/nature15744
Wang, C. H., Chen, B. T., Han, B. C., Liu, A. C. Y., Hung, P. C., Chen, C. Y., et al. (2015). Field evaluation of personal sampling methods for multiple bioaerosols. PLoS One 10:120308. doi: 10.1371/journal.pone.0120308
Willner, D., Daly, J., Whiley, D., Grimwood, K., Wainwright, C. E., and Hugenholtz, P. (2012). Comparison of DNA extraction methods for microbial community profiling with an application to pediatric bronchoalveolar lavage samples. PLoS One 7:e34605. doi: 10.1371/journal.pone.0034605
Woo, A. C., Brar, M. S., Chan, Y., Lau, M. C. Y., Leung, F. C. C., Scott, J. A., et al. (2013). Temporal variation in airborne microbial populations and microbially- derived allergens in a tropical urban landscape. Atmos. Environ. 74, 291–300. doi: 10.1016/j.atmosenv.2013.03.047
Keywords: urban, aerial, microbial, health, bioaerosol, airborne, exposure, biodiversity
Citation: Flies EJ, Jones P, Buettel JC and Brook BW (2020) Compromised Ecosystem Services From Urban Aerial Microbiomes: A Review of Impacts on Human Immune Function. Front. Ecol. Evol. 8:568902. doi: 10.3389/fevo.2020.568902
Received: 02 June 2020; Accepted: 02 October 2020;
Published: 22 October 2020.
Edited by:
Teodoro Semeraro, University of Salento, ItalyReviewed by:
Loren B. Byrne, Roger Williams University, United StatesMarzia Vergine, University of Salento, Italy
Copyright © 2020 Flies, Jones, Buettel and Brook. This is an open-access article distributed under the terms of the Creative Commons Attribution License (CC BY). The use, distribution or reproduction in other forums is permitted, provided the original author(s) and the copyright owner(s) are credited and that the original publication in this journal is cited, in accordance with accepted academic practice. No use, distribution or reproduction is permitted which does not comply with these terms.
*Correspondence: Emily J. Flies, RW1pbHkuRmxpZXNAdXRhcy5lZHUuYXU=