- 1Department of Chemistry, Biology, and Environmental Sciences, Nara Women’s University, Nara, Japan
- 2Department of Bioscience, Graduate School of Science and Technology, Kwansei Gakuin University, Sanda-shi, Japan
In traditional population models of microbial ecology, there are two central players: producers and consumers (including decomposers that depend on organic carbon). Producers support surface ecosystems by generating adenosine triphosphate (ATP) from sunlight, part of which is used to build new biomass from carbon dioxide. In contrast, the productivity of subsurface ecosystems with a limited supply of sunlight must rely on bacteria and archaea that are able generate ATP solely from chemical or electric energy to fix inorganic carbon. These “light-independent producers” are frequently not included in traditional food webs, even though they are ubiquitous in nature and interact with one another through the utilization of the by-products of others. In this review, we introduce theoretical approaches based on population dynamics that incorporate thermodynamics to highlight characteristic interactions in the microbial community of subsurface ecosystems, which may link community structures and ecosystem expansion under conditions of a limited supply of sunlight. In comparison with light-dependent producers, which compete with one another for light, the use of Gibbs free energy (chemical energy) can lead cooperative interactions among light-independent producers through the effects of the relative quantities of products and reactants on the available chemical energy, which is termed abundant resource premium. The development of a population theory that incorporates thermodynamics offers fundamental ecological insights into subsurface microbial ecosystems, which may be applied to fields of study such as environmental science/engineering, astrobiology, or the microbial ecosystems of the early earth.
Introduction
By the look of terrestrial ecosystems, life on Earth first appears to consist of abundant plant and animal communities. However, upon closer observation, there exists rich and diverse communities of fungi, bacteria, and archaea, many of which are not readily visible. According to Bar-On et al. (2018), the total biomass of the earth’s ecosystems is approximately 550 gigatons of carbon (Gt C) with an estimated 81.8% of plants, 12.7% of bacteria, 2.2% of fungi, and 1.3% of archaea, whereas animals only comprise 0.4%. They also reported that approximately 70 Gt C of biomass is present in the marine sub-seafloor sediments and the oceanic crust, as well as terrestrial substratums deeper than 8 m, which is dominated by bacteria and archaea. Total bacterial and archaeal cell numbers are estimated to be upwards of 1 × 1030 cells with nearly 3/4 residing deep in the subsurface (Flemming and Wuertz, 2019). Explorations of ocean drilling research projects have revealed that bacterial and archaeal communities are present at depths of 2.5 km below the ocean floor (Parkes et al., 1994; Ciobanu et al., 2014; Inagaki et al., 2015; Glombitza et al., 2016; Trembath-Reichert et al., 2017; Tanikawa et al., 2018). These findings suggest that there are widespread microbial communities in the subsurface realm that are not normally perceived.
Life cannot function without a constant supply of energy. As producers, plants harness sunlight as their primary energy source. However, in subsurface ecosystems where the availability of light is limited, the primary energy source would be derived from geochemical origins rather than solar energy. For example, hydrothermal vent systems are the natural plumbing systems that supply chemical substances as energy sources (e.g., hydrogen gas, methane, and dissolved iron) from the interior of the Earth. Unlike animals that depend solely on aerobic respiration for ATP generation, bacteria and archaea have evolved diverse metabolic processes that enable them to harness a variety of chemical reactions that use energy sources from geochemical origins (Table 1). These organisms synthesize ATP without relying on sunlight or organic substrates to produce their own biomass from inorganic carbon. By extending the ecological concept of producers to these microbes, they can be called “light-independent producers,” which would include denitrifiers, nitrifiers, iron oxidizers/reducers, sulfate reducers, methanotrophs, methanogens, etc. These functional (or trait-based) groups may include species that depends on organic substrate as energy source, but microbes harnessing the reactions listed in Table 1 are independent of organic substrate. Some light-independent producers may use molecular oxygen, although this may also be a photosynthetic by-product.
The presence of light-independent producers is not limited to subsurface ecosystems and also occurs in general and extreme environments. Due to their access to chemical substances, they are often observed in the interfaces between oxic (oxygen plentiful) and anoxic (oxygen-free) environments, and can move energy-source reactions forward. These environments are temporarily or spatially created, even in surface ecosystems such as sediment-water interfaces, soil aggregates, and biofilms (e.g., see review by Lau et al., 2018). Some light-independent producers can tolerate extreme pH or temperature conditions and are widely observed in mine drainage, soda lakes, hot springs, polar regions, and hydrothermal vents (Sorokin and Kuenen, 2005; Sattley and Madigan, 2006; Martin et al., 2008; Kimura et al., 2011). Although the contribution of these microbial communities to ecosystem productivity in surface ecosystems may be less important than the primary productivity of plants, light-independent producers drive important biogeochemical cycles by catalyzing energy-source reactions (Falkowski et al., 2008; Burgin et al., 2011).
The potential for subsurface primary production that is independent of solar energy has been suggested by several reviews (Gold, 1992; Stevens, 1997; Colman et al., 2017), but there are relatively few studies available that deal with the population dynamics and interspecific interactions among light-independent producers. The interspecific interactions among these producers are often associated with the use of chemical substances involved in energy-source reactions. Understanding these interactions requires not only knowledge of the biological interactions, but also the chemical interactions and energy that depends on the presence of the chemical substances in the system. Therefore, in this review, we introduce theoretical approaches based on population dynamics, which incorporates thermodynamics to highlight characteristic interactions in microbial communities in subsurface ecosystems on an energetic basis.
Energy and Nutritional Groups
To expand our understanding of energy in ecosystems and how organisms use this energy for growth, we first summarize the concepts of energy and nutritional groups. Organisms, especially microorganisms, can be classified into primary nutritional groups based on the direct source of energy and carbon (Figure 1). As the definition of nutritional groups is somewhat ambiguous, we clarify them in light of recent research regarding bacterial and archaeal communities.
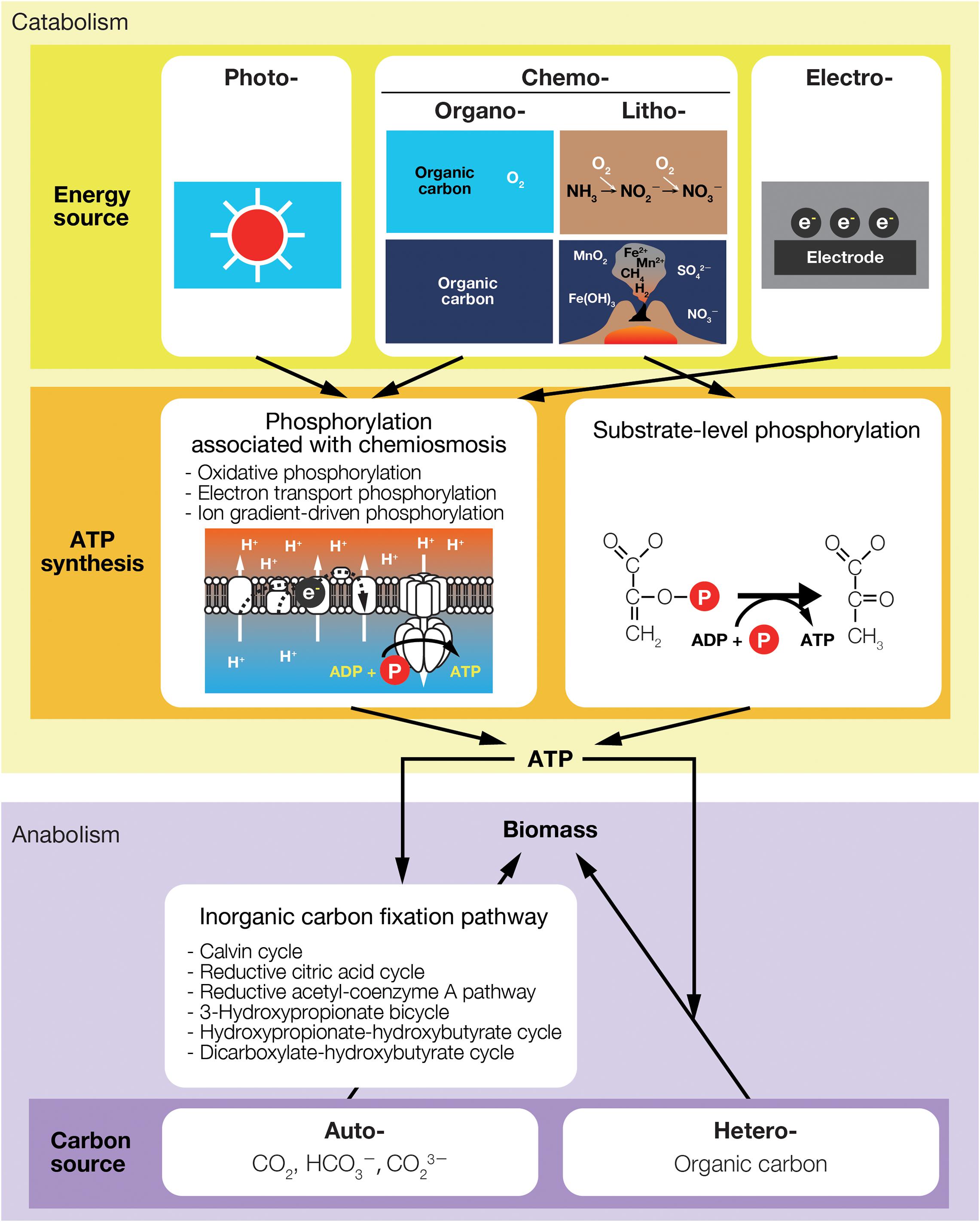
Figure 1. Primary nutritional groups and types of energy sources, ATP synthesis, and carbon sources for life. The names of primary nutritional groups are built by combining the Greek terms meaning the direct source of energy [photo (light), chemo (chemical), or electro (electric)] and carbon [auto (using inorganic carbon) or hetero (using organic carbon)] and “troph(s)” meaning nourishment. Chemo-(auto or hetero)-trophs that use organic carbon for energy-source reactions (e.g., aerobic respirators and fermentation bacteria) are especially termed chemo-organo-(auto or hetero)-trophs and others that only use inorganic substances for energy-source reactions (e.g., the reactions listed in Table 1) are termed chemo-litho-(auto or hetero)-trophs.
In ecology, energy flow has often been treated as a process that occurs in parallel with carbon flow. This is true for organisms that use carbon substrates as energy sources, however, the energy sources of microorganisms are not necessarily sunlight or carbon substrates. Energy is converted to ATP and then used for biomass synthesis. Producers use a part of ATP in the process of converting inorganic carbon to organic carbon (biomass). In addition to energy and carbon sources, we introduce the processes of ATP synthesis and carbon assimilation, which may highlight the differences between the carbon and energy needs of living organisms.
Classifications Based on Energy Source
In traditional textbooks, the direct energy source for life is described as either being sunlight or chemical reactions. Organisms that use sunlight are called phototrophs and those that harness energy from chemical reactions are called chemotrophs. According to these definitions, animals and fungi are classified as chemotrophs. Some chemotrophs use organic carbon for energy-source reactions (e.g., aerobic respirators and fermentation bacteria), and others only use inorganic substances (e.g., the reactions listed in Table 1). The former are termed chemoorganotrophs and the latter as chemolithotrophs. In addition to light and chemical energy, some microbes have been found to gain energy directly from electrons in the form of electric energy (Lovley, 2006, 2008; Thrash and Coates, 2008; Torres et al., 2010). As these electrons are supplied from the progression of the oxidation-reduction reaction (the redox reaction, a type of chemical reaction that involves the transfer of electrons), the distinction between chemical and electric energy gain is somewhat vague. Electron flow is also generated during photosynthesis and aerobic respiration, and is essential for one of the two ATP synthesizing processes described below.
ATP Synthesis
No matter which energy form is used, life eventually converts energy into ATP by adding one phosphate group to adenosine diphosphate (ADP), in a process called phosphorylation. Phosphorylation (i.e., ATP synthesis) in living organisms is achieved by two different biochemical pathways. Phosphorylation can occur via the direct transfer of a phosphate group from a phosphorylated intermediate metabolic compound to ADP. This is known as substrate-level phosphorylation and is exemplified by glycolysis (the first pathway of aerobic respiration) and fermentation (Figure 1). ADP-ribose-derived ATP synthesis in the cell nucleus might be considered as this type of phosphorylation (Wright et al., 2016). Alternatively, phosphorylation can be achieved by the electron flow generated from an energy-source reaction. The electron flow eventually creates ion gradients across membranes, or chemiosmosis, which enables ATP synthase to synthesize ATP (Mitchell, 1961; Dimroth, 1987; Russell, 2007; especially see Müller and Hess, 2017 for a discussion on the terms describing this type of phosphorylation). In this paper, we have termed the latter type as “phosphorylation associated with chemiosmosis.” In terms of energy production, substrate-level phosphorylation is generally less efficient than phosphorylation associated with chemiosmosis. However, the faster substrate-consumption rate of substrate-level phosphorylation can surpass phosphorylation associated with chemiosmosis under some conditions (Vazquez et al., 2010; Zheng, 2012).
Photosynthesis and aerobic respiration partly share similar phosphorylation processes, which are associated with chemiosmosis of hydrogen ions when electrons move down the electron transport chain. For light reactions occurring in plants, electrons are released after the light absorption by photosynthetic pigments and drive chemiosmosis across the thylakoid membrane. Similarly, most energy-source reactions, including aerobic reactions and the reactions listed in Table 1, are involved in electron transfers and, therefore, categorized as redox reactions that can be spontaneously carried out in the absence of sunlight. For example, during aerobic respiration, 24 moles of electrons are translocated from 1 mole of glucose to oxygen:
where e– indicates an electron. The overall reaction of Eq. (1c) is the well-known equation for aerobic respiration, which is the sum of the oxidation reaction (Eq. (1a)) that involves the loss of electrons from an electron donor (C6H12O6) and the reduction reaction (Eq. (1b)) that is involved with the gain of electrons of an electron acceptor (O2). Although the electron flow involved in Eq. 1 is eventually eliminated from Eq. 1c, this flow is used to generate ATP throughout chemiosmosis in mitocondria.
In general, chemical substances that act as electron acceptors are plentiful in oxic environments, while those that act as electron donors are abundant under anoxic conditions. Surface ecosystems have unique oxic environments where plants constantly supply the abundant organic substrate, which acts as an electron donor. In contrast, both electron acceptors and donors (except for organic substrates) come into play in oxic-anoxic interfaces and can supply energy for light-independent producers.
Classification Based on the Carbon Source
Some fraction of the energy stored as ATP is used to maintain the metabolism of an individual, while some is allocated to the growth of the population. Population growth is often quantified as the amount of carbon that is newly added to the overall population. For nutritional classifications, the source of carbon groups organisms into autotrophs that fix inorganic carbon to build biomass and heterotrophs that use organic carbon to synthesize biomass. Note that the classification of organisms according to carbon source is made based only on the form of carbon used as building blocks. It is independent of the direct energy source (light, chemical, or electric) to generate ATP, even though the carbon may be used in the energy-source reaction. The Calvin-Benson-Bassham (CBB) cycle is the only carbon-fixation pathway in eukaryotes (algae and plants). Some autotrophic bacteria also fix carbon dioxide in the CBB cycle, while others have evolved unique, inorganic carbon-fixation pathways (Berg et al., 2010; Berg, 2011; Hügler and Sievert, 2011).
According to these two classifications, plants and phytoplankton are photo-autotrophs, while animals and most fungi are chemo-heterotrophs. In subsurface ecosystems where there is no light, microbial communities are composed of chemo-autotrophs and chemo-heterotrophs. Here, we exclude discussions of photo-heterotrophic bacteria, but this nutritional group may be abundant and comprise approximately 10% of the microbial community in the euphotic zone in the upper ocean (Kolber et al., 2001). Some bacteria and archaea are known for their versatile metabolic strategies, which enable a cell to use both inorganic and organic carbon as building blocks, or the different types of energy source reactions (Eiler, 2006; Hügler and Sievert, 2011). These organisms that can switch metabolic strategies in response to environmental conditions are called mixotrophs.
For surface ecosystems, the primary and basic energy source is light. Plants and phytoplankton as producers harness light energy to synthesize ATP, part of which is used to fix carbon dioxide (inorganic carbon) into biomass. The direct energy source of consumers and decomposers is chemical as they generate ATP from aerobic respiration, a reaction using oxygen and organic substrates. Since both of these substrates are the by-products of photosynthesis, the original energy source for consumers and decomposers is light energy. Although consumers and decomposers also allocate organic carbon to the building blocks of biomass, carbon flows are a good indicator of energy flow within feeding interactions because it is closely linked with the energy transfer among organisms that use organic substrates as their energy source.
In contrast, the ultimate energy source of subsurface ecosystems should be chemical energy (including electrical energy), which may not rely on the supply of organic substrates as the photosynthetic by-product from surface ecosystems. Direct or indirect carbon transfers from light-independent producers to chemoorganoheterotrophs that belong to the same nutritional group as animals can also be linked to energy transfers through the microbial community. However, the energy consumption of light-independent producers and the consequential interspecific interactions among them highlights the unique relationship between population growth and energy, which can be understood as the use of energy as opposed to organic carbon.
Characteristic Interspecific Interactions Among Light- Independent Producers
Of the interspecific interactions of light-dependent producers, resource competition is an important factor that determines growth, fitness, and productivity. Although the ultimate limiting resource for light-dependent producers can be the availability of essential nutrients (primarily nitrogen and phosphorus as the building blocks of biomass), water, and/or space, only those interactions associated with the availability of light energy as a limiting resource is discussed here. However, it should be noted that the availability of nutrients and water affect the ability of light-dependent producers to capture light energy. For example, a phosphorus deficiency in chloroplast stroma inhibits ATP synthase activity (Takizawa et al., 2008; Karlsson et al., 2015; Carstensen et al., 2018).
There is experimental, observational, and theoretical evidence indicating that plants and phytoplankton may specialize in separate light niches in response to different light environments (Litchman, 2000; Litchman and Klausmeier, 2001; Silvertown, 2004; Six et al., 2007; Sterck et al., 2011). Although some shade-tolerant plants may acquire new niches due to the presence of others, plants reside in the same canopy are often competitive and they never increase the light intensity, or light energy (Figure 2A).
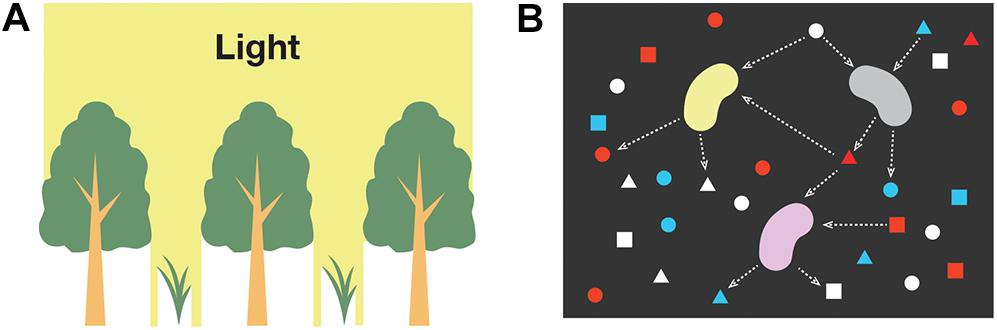
Figure 2. Interspecific interactions associated with the utilization of (A) light and (B) chemical energy.
The availability of light energy in a terrestrial ecosystem is mainly determined by the amount of sunlight received at the Earth’s surface. An example of a model that describes the availability of light, L, under a canopy is,
where L0 is the light intensity at the top of the canopy (the light available prior to any consumption), ci is the light adsorption coefficient of species i, and Bi is the biomass of species i. f describes the total light consumption by the existing species, which is a decreasing function with a maximum value of 1 that holds when no plants exist (Grovar, 1997). Any increase in Bi is associated with the consumption of light and, thus, decreases the availability of light and inhibits the light-dependent growth rate of other species.
Interspecific interactions via the use of chemical energy also significantly affect the growth of light-independent producers, although not always in a negative manner. The Gibbs energy, G, in thermodynamics is a measure of the availability of chemical energy in a system. The amount of Gibbs energy depends on all of the chemical substances present in a system and is described as:
where nj is the number of moles of substance j, and denotes the partial molar Gibbs energy, or chemical potential, when temperature, pressure, and the amount of all other substances are held constant. μj depends not only on the substance j, but on other substances in the system, as the interaction of substances affects the availability of chemical potential μj. Unlike the change of L in Eq. (2), which is described by direct consumption by plant species, the change in G can only be indirectly described by changes in the molar concentrations of reactants and products associated with the progression of the energy-source reaction (Figure 2B).
The Gibbs energy change of a reaction, ΔrG, is an important and useful thermodynamic property that enables us to estimate the availability of chemical energy for microbes under a given environmental condition. ΔrG denotes the amount of chemical energy dissipated from a reaction on a kJ per mole basis. Hence, a negative ΔrG (i.e., −ΔrG) denotes the maximum available energy for species when it catalyzes one mole of reaction to dissipate energy. If species 1 generates energy from the following overall reaction, then:
where X1 and X2 are two different compounds (or two different redox states) composed of the same element. X2 has more electrons than X1. In Eq. (4c), B2 serves as an electron donor and A1 serves as an electron acceptor. The negative Gibbs energy change of this reaction is
where −ΔrG° is the standard Gibbs energy change of reaction 1 (kJ mol–1), R is the gas constant (8.31 × 10–3 kJ K–1 mol–1), T is the absolute temperature (K), and {Xj} is the activity of Xj. Activity can be approximated as the molar concentration when the interaction forces of the substances can be ignored: {Xj} ≈ nj/v, where v is the volume. ° indicates the standard-state condition where all reactants and products (chemical substances utilized for and released from the energy-source reaction) have activity = 1. −ΔrG° is given by,
where ΔfGx° denotes the Gibbs energy change accompanied by the x formation (here, x = A1, A2, B1, or B2). ΔfGx° is the relative level of Gibbs energy of x from all of the reference states of the elements composing x [e.g., the relative level of Gibbs energy of CH4 in comparison with C(solid) as graphite and H2 (gas)]. ΔfGx° is a constant that is intrinsic to substance x under standard conditions. As ΔrG° is the difference between the sum of ΔfG° for the by-products and the sum of ΔfG° for the reactants, it is a reaction-specific quantity that is determined by the combination of reactants and by-products. ΔfGx° values are available from the CHNOSZ package for R1. The second term of −ΔrG in the right-hand side of Eq. (4d) indicates the effect of the relative quantities of products and reactants on the available chemical energy. This is termed “abundant resource premium” (ARP) (Seto and Iwasa, 2019a) and is especially important for the growth of light-independent producers, which results in characteristic mutualistic interactions, or obligately mutualistic metabolism.
The growth of light-independent producers always decreases the total amount of Gibbs energy of a system; similarly, the growth of light-dependent producers reduces the availability of light. However, unlike light consumption by plant species, which often results in competition, the progression of reaction 1 does not always decrease the availability of chemical energy for other light-independent producers. When species 2 harnesses a reaction that utilizes A2 we get,
where the negative Gibbs energy change of reaction 2 is
To distinguish ΔrG in Eqs. (4d) and (5b), from here we will use the symbol ΔrGi, where subscript i denotes the reaction number. Because the supply of A2 increases −ΔrG2 due to the presence of the ARP term, species 2 may generate more energy when species 1 exists (Figure 2B). The consumption of A2 also increases −ΔrG1, which consequentially increases the energy gain of species 1. Hence, the consumption of the by-product of the energy-harvesting reaction can lead to mutualism between species 1 and 2 owing to the presence of ARP. An example of a mutualistic interaction which is similar to this type occurs between ammonia-oxidizers and nitrite-oxidizers in Table 1 [the combination of reactions (VIII) and (X)], which are microbes interacting via the supply and consumption of nitrite ion (NO2−). Although these microbes compete for oxygen, one can predict that the presence of a nitrite-oxidizer may increase the energy acquisition per reaction of an ammonia-oxidizer under oxic conditions where the availability of oxygen may not limit the growth of nitrite-oxidizer and ammonia-oxidizer. These microbes have been confirmed to occur in clusters and be in frequent contact with each other within sludge flocs (Mobarry et al., 1996).
A more intuitive example of the mutualism between light-independent producers that completes material recycling within their energy-source reaction is a combination of Eq. (4c) and
where the negative Gibbs energy change of reaction 3 is
An example can be found in the iron cycle between Fe2+ and Fe oxides (e.g., the combination of reaction (IV) and (XI) or (XIII) in Table 1) such as Fe (OH)3 that is readily formed in the presence of Fe3+ at a circumneutral pH. Experimental studies have reported the possibility of micro-scale bacterial iron cycling at oxic-anoxic interfaces (Emerson and Revsbech, 1994; Sobolev and Roden, 2002; Roden et al., 2004). Another example is the sulfate cycle, which is closely associated with the methane and nitrogen cycles [e.g., the combination of reactions (II) and (XV) in Table 1] (Lau et al., 2016).
Population Dynamics Under Available Chemical-Energy Limitations
The availability of chemical energy changes in response to changes in the amount of chemical substances in the surrounding environment, however, this effect has been neglected in population dynamics models in traditional microbial ecology. The population growth of light-dependent producers (plants and phytoplankton) is often limited by the availability of energy. Similarly, the population dynamics of light-independent producers are often limited by the acquisition rate of chemical energy. Interestingly, modeling this provides a framework for understanding mutualistic interactions among light-independent producers, which has not been previously recognized in the ecological sciences. In a previous paper, we introduced a microbial population dynamics model in relation to energy production per reaction that included the ARP term (Seto and Iwasa, 2019a). For a microbial population that harvests energy from reaction i,
where Fi denotes the biomass-specific catalytic rate of the energy-source reaction, qi is the biomass yield of species i for a given energy gain from ATP that is generated from an energy-source reaction, ci is the fraction of energy that can be used for ATP synthesis (0 < ci < 1) and excludes energy expenditure such as loss by heat transfer, and mi is the rate of energy loss that occurs to maintain cellular activity. For simplicity, we call xi the population density of the ith species, but it is more appropriate to call it the ith functional group, as multiple species typically share the same energy-source reaction. The term in curly brackets in Eq. (7a) describes the biomass-specific rate of the net energy gain. A reaction requiring two substrates (an electron acceptor and an electron donor) may be simultaneously limited by the low availability of both substrates. There are several proposed models that encompass dual-limitations from the abundance of electron donors and acceptors (Bae and Rittmann, 1996; Jin and Bethke, 2003, 2007; LaRowe et al., 2012). Here we have adopted the simplest model—the double-Monod model. For Eq. (4c) (reaction 1) it is described as,
where r1 is the maximum microbial catalytic rate of reaction 1, and KX is the half-saturation constant for X.
The growth of chemoheterotrophs (e.g., aerobic bacteria and fermentation bacteria) is often described by the Monod equation, where the growth rate of microbial population x appears to be limited solely by the availability of the concentration of the organic substrate R:
Equation (7a) can be approximated as Eq. (8) if the following three conditions are satisfied: (i) the decreasing rate – mx is added to Eq. (8), (ii) the concentration of one of the two substrates is significantly higher than its half-saturation constant such that the corresponding factor is replaced by 1, and (iii) the effect of ARP on −ΔrG can be ignored because of the high value of −ΔrG°. Assumptions (ii) and (iii) are plausible for aerobic bacteria and fermentation bacteria. The incubation of aerobic bacteria is usually conducted under aerobic conditions (i.e., high oxygen concentration), whereas fermentation bacteria only require a single organic substrate, which is used to generate energy from substrate-level phosphorylation. Additionally, the energy-source reaction that uses the organic substrate generally has a high −ΔrG°. The relative abundance of reactants and by-products can affect −ΔrG only when the contribution of ARP on −ΔrG is so large that −ΔrG cannot be approximated as −ΔrG°. When the availability of reactants is significantly higher than products, for example ({A1}{B2})/({A2}{B1}) = 106, ARP is approximately 33.5 kJ mol–1 at 1 bar and 298.15 K. The ARP increases with increasing temperature if all other variables and parameters are fixed. However, the activities of chemical substances and −ΔrG° often depend on temperature sensitivity, which may occasionally decrease −ΔrG with increasing temperature. As shown in Eq. 4e, the value of −ΔrG° is determined by the combination of reactants and products under constant temperature and pressure conditions. The −ΔrG° of light-independent producers is typically lower than 1000 kJ mol–1, whereas −ΔrG° can exceed 2000 kJ mol–1 for aerobic respiration when glucose is used as an electron donor. This suggests that the ARP term does not affect the growth dynamics of microbes that harness aerobic respiration, but in the case of light-independent producers, it may have a significant effect on microbial growth and steady-state behavior.
Due to the ARP effect, the model’s behaviors are qualitatively different from those observed in traditional microbial population growth models that follow the Monod-type equation (Seto and Iwasa, 2019a). The steady-state population density of the Monod-type equation increases with increasing ri and decreasing KX, as these changes increase the resource-utilizing ability or the resource consumption rate of the species. Meanwhile, the steady population density of the population dynamics that follow Eq. (7) is maximized at an intermediate ri and KX. This is because the increase in the resource-utilizing ability positively affects population growth when the abundance of the reactants is relatively larger than that of the by-products, but it negatively affects the energy acquisition per reaction as by-products accumulate in the surroundings, or −ΔrG becomes low.
The unique steady-state response of light-independent producers to ri and KX may provide them with a distinctive resource-utilizing strategy. If several species compete for the same resource, the steady-state analysis of the Monod-type population dynamics model predicts that the species that can deplete the abundance of resources to the lowest level will exclude others at the steady-state (R∗-rule; Tilman, 1982), which may act as a major selective force for many microbial species. Following the R∗-rule, when all of the species have the same parameter values except for r, only the species with the highest r will eventually dominate the system and maintain the highest population-density level. In contrast, in our model, if several species harness the same energy-source reaction with the same parameter values except for r coexist within a system, the species with the highest r will also exhibit the fastest growth rate and dominate the system, but will maintain a relatively lower population density. Modeling studies have predicted that a small population is more strongly threatened by stochastic processes than a large population if the population is isolated, which would subsequently cause local extinction (Matthies et al., 2004). This implies that subsurface ecosystems may shape a unique evolution of specialization in the resource utilization of light-independent producers.
ARP-Driven Mutualistic Interactions
Understanding two-species population dynamics is the first step toward identifying the complex interspecific interactions among light-independent producers. We previously undertook mathematical analyses of two models, the structures of which are illustrated in Figure 3. These models deal with mutualistic interspecific interactions that occur through the use of different chemical forms of an element labeled as A1, A2, and A3 (Seto and Iwasa, 2019b, 2020), where a larger subscript number denotes having more electrons. Although light-independent producers can compete for the same resource(s) used in energy-source reactions, we focused on two cases of mutualism where, (i) species 2 uses the by-product of species 1 (the combination of Eqs. (4c) and (5a), i.e., reactions 1 and 2), and (ii) each species receives its own resources from the other species and provides them with the by-products as a resource (the combination of Eqs. (4c) and (6a), i.e., reactions 1 and 3). We call the former type of interspecific interaction a one-way interaction, and the latter a recycling interaction.
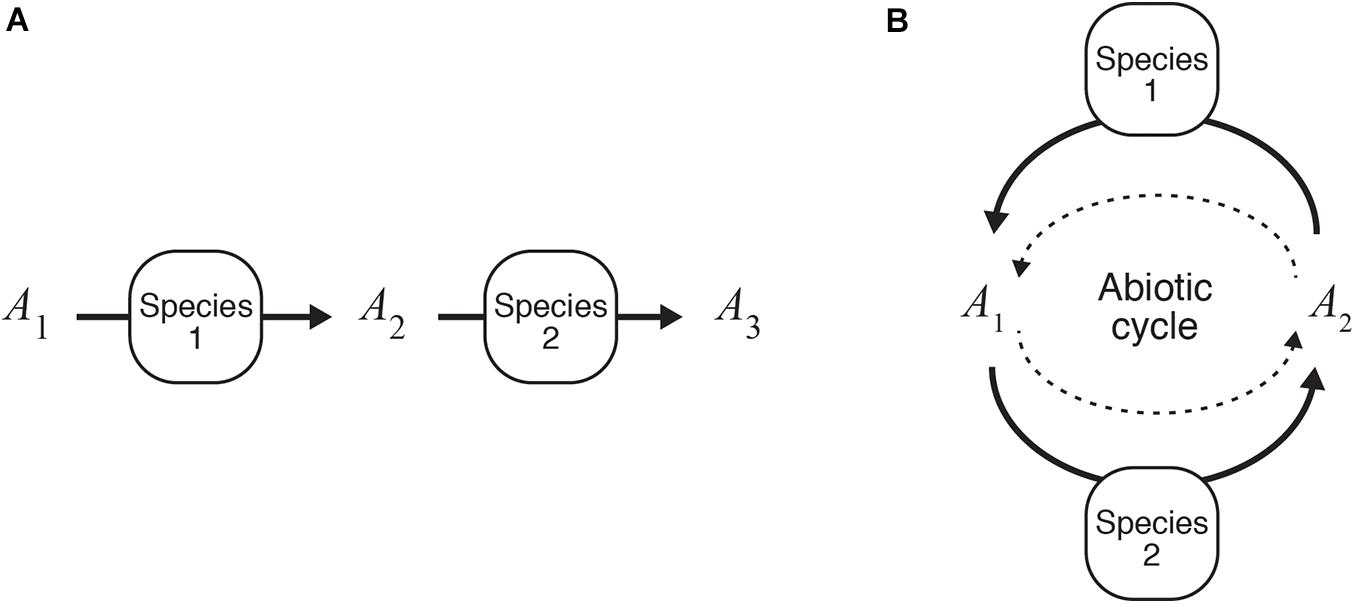
Figure 3. Mutualistic catabolic interactions. (A) One-way interactions (Seto and Iwasa, 2019b). (B) Recycling interactions (Seto and Iwasa, 2020).
For both models, the presence of species 2 increases the steady-state biomass of species 1 and expands the realized niche of species 1 from its fundamental niche. For the one-way interaction model, the interspecific interaction can only be commensalism in the absence of ARP (i.e., the second term on the right-hand side of −ΔrG is eliminated) because the presence of species 1, which supplies the resources for species 2, can expand the conditions for the survival of species 2 and its realized niche. This is a standard effect known as “niche construction” or “niche changing” (Erwin, 2008; Laland et al., 2016). The ARP term allows species 1 to increase the fitness of species 1 in the presence of species 2 because species 2 reduces the abundance of the by-product of species 1, resulting in the expansion of the realized niches of species 1. Hence, both species are able to live in conditions in which neither of them could survive alone. Meanwhile, for the recycling interaction model, the same tendency is true for a model with and without the ARP term. As confirmed by the analysis of the one-species model, an increase in the resource-utilizing ability of species in the two models eventually leads to a decline in steady-state population density. However, the presence of a mutualistic partner allows a species to keep its steady-state population density at a higher level than when it would if alone. This implies that a species with a higher resource-utilizing ability may be able to robustly survive in a system if a species that works in a mutually beneficial manner is present and in close proximity.
The mutualistic relationship observed between species 1 and 2 occurs when the population growth of both species depends on the amount of chemical energy including the ARP term. If the growth rate is limited by not the energy acquisition process (catabolism) but the biosynthetic process (anabolism), these two species may compete for essential nutrients to build biomass. This suggests that interspecific interactions among light-independent producers may change depending on which process ultimately limits the microbial growth rate.
The presence of a mutually beneficial partner increases the rate of the energy-source reaction, which may drive the geochemical process that proceeds very slowly in the absence of light-independent producers. Species 1 in Figure 3B is able to survive only in the presence of species 2 that sufficiently accelerates the reaction from A1 to A2 when it is unlikely to carry forward abiotically. A reaction spontaneously proceeds with releasing energy when ΔrG < 0 (i.e., −ΔrG > 0). However, the rate of reaction is not determined by the value of ΔrG, but, rather, it depends on activation energy and environmental factors (e.g., temperature, coexisting chemical substances, water availability, and pH), which affect the intensity of the competition between abiotic and microbial reactions (Kirby et al., 1999; Melton et al., 2014; Liu et al., 2017). Microbes, like enzymes in biochemical systems, can often accelerate the reaction rate by lowering the energetic barrier required for the reaction to commence. For instance, in the presence of the iron-oxidizing bacteria Thiobacillus ferrooxidans, the iron oxidation rate increases up to several orders of magnitude in comparison with the rate under abiotic controls (Lacey and Lawson, 1970; Nordstrom, 1985).
In contrast, rapid abiotic reactions may inhibit the growth of microbes that compete for the same resources. Under neutral pH conditions, it is hypothesized that the growth of iron-oxidizing bacteria is constrained under low oxygen conditions, where microbial iron oxidation can outcompete rapid abiotic iron oxidation (Druschel et al., 2008). Micro-scale iron recycling between iron-oxidizing and -reducing bacteria may enable iron-oxidizing bacteria to overcome abiotic iron oxidation.
Discussion
The Productivity of Subsurface Microbial Ecosystems
The presence of abundant microbial biomass in subsurface ecosystems implies there is considerable productivity of light-independent producers. However, research on the productivity of subsurface microbial ecosystems has been advanced only recently due to limited access to the subsurface biosphere.
Estimations of the productivity of hydrothermal vent ecosystems based on calculations of ΔrG have suggested that the productivity of light-independent producers contributes only a small fraction of the total photosynthetic biomass produced in the oceans (McCollom and Shock, 1997; McCollom, 2007). The vast biomass stock, despite low productivity in the subsurface biosphere, may have three explanations. First, the energy sources of subsurface ecosystems may be heavily dependent on organic substrates supplied by surface ecosystems. The dominance of heterotrophic microbes that use organic carbon to build biomass has been confirmed for some sedimentary subsurface ecosystems (Biddle et al., 2006; Morono et al., 2011). Second, microorganisms might adapt or acclimate to energetic stress by lowering the maintenance energy (m in our model). To thrive under limited access to energy sources, microbes in the subsurface have often been observed to reduce maintenance energy by switching their metabolic modes; and, as a result, the mean cell-doubling time may then range from a few years to thousands of years (Jørgensen and Boetius, 2007; Valentine, 2007; Hoehler and Jørgensen, 2013). Finally, microbes under low oxygen environments require less energy to synthesize biomass than those under oxygen-rich conditions, which may result in relatively high biomass conversion efficiency (q in our model) in the subsurface biosphere. Nitrogen and sulfur sources in oxygen-rich conditions often exist as NO3– and SO42–. To generate amino acids as building blocks of biomass, NO3– and SO42– should be converted into ammonia and hydrogen sulfide ions, and, in the presence of oxygen, more energy is required to carry out these reactions (McCollom and Amend, 2005).
An interesting question is whether the biodiversity-ecosystem functioning (BEF) relationship in subsurface microbial ecosystems is similar across surface and subsurface ecosystems. Controlled experiments in terrestrial ecosystems have shown increased plant biomass and primary productivity with increasing plant diversity (Hector et al., 1999; Tilman et al., 2001), whereas a mechanistic model that incorporates a food-web structure has demonstrated that plant biomass does not always increase with plant diversity (Thébault and Loreau, 2003). Studies examining BEF relationships for soil microorganisms reported somewhat contradictory results; some studies emphasized the link between the physiological or functional diversity and ecosystem functions, especially process rates or activities (e.g., nitrification rate and denitrification activity), in soil microorganisms (Balser and Firestone, 2005; Bell et al., 2005; Webster et al., 2005; Philippot et al., 2013), and the other demonstrated the irrelevance of microbial diversity to ecosystem functions (especially carbon mineralization) (Balser and Firestone, 2005; Wertz et al., 2006). Ecosystem functions of microbes are often evaluated by the process rates of carbon and nitrogen, but as we introduced so far, the energy-source reactions of light-independent producers drive the flows of not only carbon and nitrogen but also other elements such as iron and sulfur. This may result in the underestimation of the effect of microbial diversity on their ecosystem functioning. The extension of our model would help to provide a theoretical framework for understanding the BEF relationship for light-independent producers and overall subsurface microbial ecosystems, including heterotrophic microbes. It would be interesting to address whether or how much the supply of organic substrates from surface ecosystems controls the productivity and biodiversity of these ecosystems, as suggested previously (Hubalek et al., 2016; Magnabosco et al., 2018).
Ecology of Light-Independent Producers as an Interdisciplinary Field
The application of a population dynamics model to the community of light-independent producers will develop our understanding of not only subsurface ecology, but environmental science/engineering, astrobiology, and the evolution of early life and ecosystems.
Microbial fuel cells are a technology that converts chemical energy to electricity by using electron transfer metabolisms (Du et al., 2007). While research has concentrated on the conversion of organic substrates to electricity, the application of light-independent producers is also expected to produce carbon compounds from CO2 in a renewable manner. However, because of low product yields and productivity, the practical application of light-independent producers requires improved efficiency under industrial conditions (Claassens et al., 2016). For some microbial fuel cells that use organic substrates, higher power generation in co-cultures, as opposed to individual strains, has been reported (Logan et al., 2019). While more realistic modeling is needed, our approach will be useful in understanding productivity in co-cultures by linking population dynamics with the availability of chemical energy. This application will also be useful in understanding the different efficiencies of wastewater treatment by light-independent producers in pure and co-cultures (e.g., Parshina et al., 2005).
Theoretical, experimental, and field investigations have established the physical and chemical limits of microbial life under extreme conditions. In the field of astrobiology, the potential survival and existence of life on other planets has been debated based on these limitations. The activity of microbes, including light-independent producers, helps in the search for life on extraterrestrial planets and in planetary protection against microbial contamination from and to Earth in the course of space missions. Although microbial life can be limited by multiple factors (e.g., the availability of water, gravity, temperature, pressure, and the intensity of UV/ionizing radiation) (Rummel et al., 2014; Moissl-Eichinger et al., 2016), the presence of the potential energy-source reaction is one important factor that restricts microbial activity. The limits on microbial growth are often measured based on single-culture experiments, but, as our results suggest, the presence of a mutualistic counterpart may expand limits on microbial growth. This suggests that the limits on microbial growth require an understanding of the effects of biological interactions, in addition to abiotic factors.
Although the energy source for the earliest life is still under debate, both phylogenetic and biochemical evidence suggest that the earliest life forms were chemoautotrophs (Pace, 1997; Lane et al., 2010; Judson, 2017), which may have used hydrogen and carbon dioxide as geochemical sources (Weiss et al., 2016). The early Earth was deficient in oxygen and organic matter previous to the evolution of oxygenic photosynthetic organisms, which limited the energy-source reactions for the light-independent producers listed in Table 1. One interesting question deals with how microbial metabolic diversity evolved and was constructed in the earliest ecosystem under extreme energy limitations. If the earliest life forms depended on chemical energy, the most likely place of origin would be the hydrothermal vent system, which creates an interface between thermal fluids with electron-abundant substances and the relatively electron-deficient substances in the bottom water. Another interesting question is how life could have abandoned this attractive energy source to expand the ecosystems. Our results suggest that the evolution of a mutualistic metabolic counterpart may have enabled life under extreme energy limitations to be freed from energetic constraints and invade other systems (Seto and Iwasa, 2019b, 2020). Especially for microbes harnessing reactions with low −ΔrG° and those that do not use either organic substrates or oxygen, the ARP may play an important role in potential growth, survival and community structure.
Author Contributions
Both authors contributed to the writing of the manuscript.
Funding
This work was supported by JSPS Grant-in-Aid for Scientific Research (C) [Grant Number 19K06853] awarded to MS.
Conflict of Interest
The authors declare that the research was conducted in the absence of any commercial or financial relationships that could be construed as a potential conflict of interest.
Acknowledgments
We thank the following people for their helpful comments: K. Kadowaki, K. Koba, M. Kondoh, Y. Tachiki, and K. Uriu. We also thank NM and SDV for their comments.
Footnotes
References
Bae, W., and Rittmann, B. E. (1996). A structured model of dual-limitation kinetics. Biotechnol. Bioeng. 49, 683–689. doi: 10.1002/(SICI)1097-0290(19960320)49:6<683::AID-BIT10<3.0.CO;2-7
Balser, T. C., and Firestone, M. K. (2005). Linking microbial community composition and soil processes in a California annual grassland and mixed-conifer forest. Biogeochemistry 73, 395–415. doi: 10.1007/s10533-004-0372-y
Bar-On, Y. M., Phillips, R., and Milo, R. (2018). The biomass distribution on Earth. Proc. Natl. Acad. Sci. U.S.A. 115, 6506–6511. doi: 10.1073/pnas.1711842115
Bell, T., Newman, J. A., Silverman, B. W., Turner, S. L., and Lilley, A. K. (2005). The contribution of species richness and composition to bacterial services. Nature 436, 1157–1160. doi: 10.1038/nature03891
Berg, I. A. (2011). Ecological aspects of the distribution of different autotrophic CO2 fixation pathways. Appl. Environ. Microbiol. 77, 1925–1936. doi: 10.1128/AEM.02473-10
Berg, I. A., Kockelkorn, D., Ramos-Vera, W. H., Say, R. F., Zarzycki, J., Hügler, M., et al. (2010). Autotrophic carbon fixation in archaea. Nat. Rev. Microbiol. 8, 447–460. doi: 10.1038/nrmicro2365
Biddle, J. F., Lipp, J. S., Lever, M. A., Lloyd, K. G., Sørensen, K. B., Anderson, R., et al. (2006). Heterotrophic Archaea dominate sedimentary subsurface ecosystems off Peru. Proc. Natl. Acad. Sci. U.S.A. 103, 3846–3851. doi: 10.1073/pnas.0600035103
Burgin, A. J., Yang, W. H., Hamilton, S. K., and Silver, W. L. (2011). Beyond carbon and nitrogen: how the microbial energy economy couples elemental cycles in diverse ecosystems. Front. Ecol. Environ. 9, 44–52. doi: 10.1890/090227
Carstensen, A., Herdean, A., Schmidt, S. B., Sharma, A., Spetea, C., Pribil, M., et al. (2018). The impacts of phosphorus deficiency on the photosynthetic electron transport chain. Plant Physiol. 177, 271–284. doi: 10.1104/pp.17.01624
Ciobanu, M. C., Burgaud, G., Dufresne, A., Breuker, A., Rédou, V., Ben Maamar, S., et al. (2014). Microorganisms persist at record depths in the subseafloor of the Canterbury Basin. ISME J. 8, 1370–1380. doi: 10.1038/ismej.2013.250
Claassens, N. J., Sousa, D. Z., Dos Santos, V. A. P. M., De Vos, W. M., and Van Der Oost, J. (2016). Harnessing the power of microbial autotrophy. Nat. Rev. Microbiol. 14, 692–706. doi: 10.1038/nrmicro.2016.130
Colman, D. R., Poudel, S., Stamps, B. W., Boyd, E. S., and Spear, J. R. (2017). The deep, hot biosphere: twenty-five years of retrospection. Proc. Natl. Acad. Sci. U.S.A. 114, 6895–6903. doi: 10.1073/pnas.1701266114
Dimroth, P. (1987). Sodium ion transport decarboxylases and other aspects of sodium ion cycling in bacteria. Microbiol. Rev. 51, 320–340. doi: 10.1128/mmbr.51.3.320-340.1987
Druschel, G. K., Emerson, D., Sutka, R., Suchecki, P., and Luther, G. W. (2008). Low-oxygen and chemical kinetic constraints on the geochemical niche of neutrophilic iron(II) oxidizing microorganisms. Geochim. Cosmochim. Acta 72, 3358–3370. doi: 10.1016/j.gca.2008.04.035
Du, Z., Li, H., and Gu, T. (2007). A state of the art review on microbial fuel cells: a promising technology for wastewater treatment and bioenergy. Biotechnol. Adv. 25, 464–482. doi: 10.1016/j.biotechadv.2007.05.004
Eiler, A. (2006). Evidence for the ubiquity of mixotrophic bacteria in the upper ocean: implications and consequences. Appl. Environ. Microbiol. 72, 7431–7437. doi: 10.1128/AEM.01559-06
Emerson, D., and Revsbech, N. P. (1994). Investigation of an iron-oxidizing microbial mat community located near Aarhus, Denmark: field studies. Appl. Environ. Microbiol. 60, 4022–4031.
Erwin, D. H. (2008). Macroevolution of ecosystem engineering, niche construction and diversity. Trends Ecol. Evol. 23, 304–310. doi: 10.1016/j.tree.2008.01.013
Falkowski, P. G., Fenchel, T., and Delong, E. F. (2008). The microbial engines that drive Earth’s biogeochemical cycles. Science 320, 1034–1039. doi: 10.1126/science.1153213
Flemming, H. C., and Wuertz, S. (2019). Bacteria and archaea on Earth and their abundance in biofilms. Nat. Rev. Microbiol. 17, 247–260. doi: 10.1038/s41579-019-0158-9
Glombitza, C., Adhikari, R. R., Riedinger, N., Gilhooly, W. P., Hinrichs, K. U., and Inagaki, F. (2016). Microbial sulfate reduction potential in coal-bearing sediments down to ~2.5 km below the seafloor off Shimokita Peninsula, Japan. Front. Microbiol. 7:1576. doi: 10.3389/fmicb.2016.01576
Gold, T. (1992). The deep, hot biosphere. Proc. Natl. Acad. Sci. U.S.A. 89, 6045–6049. doi: 10.1073/pnas.89.13.6045
Hector, A., Schmid, B., Beierkuhnlein, C., Caldeira, M. C., Diemer, M., Dimitrakopoulos, P. G., et al. (1999). Plant diversity and productivity experiments in European grasslands. Science 286, 1123–1127. doi: 10.1126/science.286.5442.1123
Hoehler, T. M., and Jørgensen, B. B. (2013). Microbial life under extreme energy limitation. Nat. Rev. Microbiol. 11, 83–94.
Hubalek, V., Wu, X., Eiler, A., Buck, M., Heim, C., Dopson, M., et al. (2016). Connectivity to the surface determines diversity patterns in subsurface aquifers of the Fennoscandian shield. ISME J. 10, 2447–2458. doi: 10.1038/ismej.2016.36
Hügler, M., and Sievert, S. M. (2011). Beyond the Calvin cycle: autotrophic carbon fixation in the ocean. Annu. Rev. Mar. Sci. 3, 261–289. doi: 10.1146/annurev-marine-120709-142712
Inagaki, F., Hinrichs, K. U., Kubo, Y., Bowles, M. W., Heuer, V. B., Hong, W. L., et al. (2015). Exploring deep microbial life in coal-bearing sediment down to ∼2.5 km below the ocean floor. Science 349, 420–424. doi: 10.1126/science.aaa6882
Jin, Q., and Bethke, C. M. (2003). A new rate law describing microbial respiration. Appl. Environ. Microbial. 69, 2340–2348. doi: 10.1128/AEM.69.4.2340
Jin, Q., and Bethke, C. M. (2007). The thermodynamics and kinetics of microbial metabolism. Am. J. Soc. 307, 643–677.
Jørgensen, B. B., and Boetius, A. (2007). Feast and famine – microbial life in the deep-sea bed. Nat. Rev. Microbiol. 5, 770–781. doi: 10.1038/nrmicro1745
Judson, O. P. (2017). The energy expansions of evolution. Nat. Ecol. Evol. 1:0138. doi: 10.1038/s41559-017-0138
Karlsson, P. M., Herdean, A., Adolfsson, L., Beebo, A., Nziengui, H., Irigoyen, S., et al. (2015). The Arabidopsis thylakoid transporter PHT4;1 influences phosphate availability for ATP synthesis and plant growth. Plant J. 84, 99–110. doi: 10.1111/tpj.12962
Kimura, S., Bryan, C. G., Hallberg, K. B., and Johnson, D. B. (2011). Biodiversity and geochemistry of an extremely acidic, low-temperature subterranean environment sustained by chemolithotrophy. Environ. Microbiol. 13, 2092–2104. doi: 10.1111/j.1462-2920.2011.02434.x
Kirby, C. S., Thomas, H. M., Southam, G., and Donald, R. (1999). Relative contributions of abiotic and biological factors in Fe(II) oxidation in mine drainage. Appl. Geochem. 14, 511–530. doi: 10.1016/S0883-2927(98)00071-7
Kolber, Z. S., Plumley, F. G., Lang, A. S., Beatty, J. T., Blankenship, R. E., VanDover, C. L., et al. (2001). Contribution of aerobic photoheterotrophic bacteria to the carbon cycle in the ocean. Science 292, 2492–2495. doi: 10.1126/science.1059707
Lacey, D. T., and Lawson, F. (1970). Kinetics of the liquid−phase oxidation of acid ferrous sulfate by the bacterium Thiobacillus ferrooxidens. Biotechnol. Bioeng. 12, 29–50. doi: 10.1002/bit.260120104
Laland, K., Matthews, B., and Feldman, M. W. (2016). An introduction to niche construction theory. Evol. Ecol. 30, 191–202. doi: 10.1007/s10682-016-9821-z
Lane, N., Allen, J. F., and Martin, W. (2010). How did LUCA make a living? Chemiosmosis in the origin of life. Bioessays 32, 271–280. doi: 10.1002/bies.200900131
LaRowe, D. E., Dale, A. W., Amend, J. P., and Van Cappellen, P. (2012). Thermodynamic limitations on microbially catalyzed reaction rates. Geochim. Cosmochim. Acta 90, 96–109.
Lau, M. C. Y., Kieft, T. L., Kuloyo, O., Linage-Alvarez, B., Van Heerden, E., Lindsay, M. R., et al. (2016). An oligotrophic deep-subsurface community dependent on syntrophy is dominated by sulfur-driven autotrophic denitrifiers. Proc. Natl. Acad. Sci. U.S.A. 113, E7929–E7936. doi: 10.1073/pnas.1612244113
Lau, M. P., Niederdorfer, R., Sepulveda-Jauregui, A., and Hupfer, M. (2018). Synthesizing redox biogeochemistry at aquatic interfaces. Limnologica 68, 59–70. doi: 10.1016/j.limno.2017.08.001
Litchman, E. (2000). Growth rates of phytoplankton under fluctuating light. Freshw. Biol. 44, 223–235. doi: 10.1046/j.1365-2427.2000.00559.x
Litchman, E., and Klausmeier, C. A. (2001). Competition of phytoplankton under fluctuating light. Am. Nat. 157, 170–187.
Liu, D., Zhu, W., Wang, X., Pan, Y., Wang, C., Xi, D., et al. (2017). Abiotic versus biotic controls on soil nitrogen cycling in drylands along a 3200 km transect. Biogeosciences 14, 989–1001. doi: 10.5194/bg-14-989-2017
Logan, B. E., Rossi, R., Ragab, A., and Saikaly, P. E. (2019). Electroactive microorganisms in bioelectrochemical systems. Nat. Rev. Microbiol. 17, 307–319. doi: 10.1038/s41579-019-0173-x
Lovley, D. R. (2006). Microbial fuel cells: novel microbial physiologies and engineering approaches. Curr. Opin. Biotechnol. 17, 327–332. doi: 10.1016/j.copbio.2006.04.006
Lovley, D. R. (2008). The microbe electric: conversion of organic matter to electricity. Curr. Opin. Biotechnol. 19, 564–571. doi: 10.1016/j.copbio.2008.10.005
Magnabosco, C., Lin, L. H., Dong, H., Bomberg, M., Ghiorse, W., Stan-Lotter, H., et al. (2018). The biomass and biodiversity of the continental subsurface. Nat. Geosci. 11, 707–717. doi: 10.1038/s41561-018-0221-6
Majzlan, J., Navrotsky, A., and Schwertmann, U. (2004). Thermodynamics of iron oxides: Part III. Enthalpies of formation and stability of ferrihydrite (∼Fe(OH)3), schwertmannite (∼FeO(OH)3/4(SO4)1/8), and ε-Fe2O3. Geochim. Cosmochim. Acta 68, 1049–1059. doi: 10.1016/S0016-7037(03)00371-5
Martin, W., Baross, J., Kelley, D., and Russell, M. J. (2008). Hydrothermal vents and the origin of life. Nat. Rev. Microbiol. 6, 805–814. doi: 10.1038/nrmicro1991
Matthies, D., Bräuer, I., Maibom, W., and Tscharntke, T. (2004). Population size and the risk of local extinction: empirical evidence from rare plants. Oikos 105, 481–488. doi: 10.1111/j.0030-1299.2004.12800.x
McCollom, T. M. (2007). Geochemical constraints on sources of metabolic energy for chemolithoautotrophy in ultramafic-hosted deep-sea hydrothermal systems. Astrobiology 7, 933–950.
McCollom, T. M., and Amend, J. P. (2005). A thermodynamic assessment of energy requirements for biomass synthesis by chemolithoautotrophic micro-organisms in oxic and anoxic environments. Geobiology 3, 135–144. doi: 10.1111/j.1472-4669.2005.00045.x
McCollom, T. M., and Shock, E. L. (1997). Geochemical constraints on chemolithoautotrophic metabolism by microorganisms in seafloor hydrothermal systems. Geochim. Cosmochim. Acta 61, 4375–4391.
Melton, E. D., Swanner, E. D., Behrens, S., Schmidt, C., and Kappler, A. (2014). The interplay of microbially mediated and abiotic reactions in the biogeochemical Fe cycle. Nat. Rev. Microbiol. 12, 797–808. doi: 10.1038/nrmicro3347
Mitchell, P. (1961). Coupling of phosphorylation to electron and hydrogen transfer by a chemi-osmotic type of mechanism. Nature 191, 144–148. doi: 10.1038/191144a0
Mobarry, B. K., Wagner, M., Urbain, V., Rittmann, B. E., and Stahl, D. A. (1996). Phylogenetic probes for analyzing abundance and spatial organization of nitrifying bacteria. Appl. Environ. Microbiol. 62, 2156–2162.
Moissl-Eichinger, C., Cockell, C., and Rettberg, P. (2016). Venturing into new realms? Microorganisms in space. FEMS Microbiol. Rev. 40, 722–737. doi: 10.1093/femsre/fuw015
Morono, Y., Terada, T., Nishizawa, M., Ito, M., Hillion, F., Takahata, N., et al. (2011). Carbon and nitrogen assimilation in deep subseafloor microbial cells. Proc. Natl. Acad. Sci. U.S.A. 108, 18295–18300. doi: 10.1073/pnas.1107763108
Müller, V., and Hess, V. (2017). The minimum biological energy quantum. Front. Microbiol. 8:2019. doi: 10.3389/fmicb.2017.02019
Nordstrom, D. K. (1985). “The rate of ferrous iron oxidation in a stream receiving acid mine effluent,” in Selected Papers in the Hydrologic Sciences, US Geological Survey Water-Supply Paper 2270, ed. S. Subitzky (CA, United States: University of California, San Diego), 113–119.
Pace, N. R. (1997). A molecular view of microbial diversity and the biosphere. Science 276, 734–740. doi: 10.1126/science.276.5313.734
Parkes, R. J., Cragg, B. A., Bale, S. J., Getlifff, J. M., Goodman, K., Rochelle, P. A., et al. (1994). Deep bacterial biosphere in Pacific Ocean sediments. Nature 371, 410–413. doi: 10.1038/371410a0
Parshina, S. N., Kijlstra, S., Henstra, A. M., Sipma, J., Plugge, C. M., and Stams, A. J. M. (2005). Carbon monoxide conversion by thermophilic sulfate-reducing bacteria in pure culture and in co-culture with Carboxydothermus hydrogenoformans. Appl. Microbiol. Biotechnol. 68, 390–396. doi: 10.1007/s00253-004-1878-x
Philippot, L., Spor, A., Hénault, C., Bru, D., Bizouard, F., Jones, C. M., et al. (2013). Loss in microbial diversity affects nitrogen cycling in soil. ISME J. 7, 1609–1619. doi: 10.1038/ismej.2013.34
Roden, E. E., Sobolev, D., Glazer, B., and Luther, G. W. (2004). Potential for microscale bacterial Fe redox cycling at the aerobic-anaerobic interface. Geomicrobiol. J. 21, 379–391. doi: 10.1080/01490450490485872
Rummel, J. D., Beaty, D. W., Jones, M. a., Bakermans, C., Barlow, N. G., Boston, P. J., et al. (2014). A new analysis of Mars “Special Regions”: findings of the second MEPAG special regions science analysis group (SR-SAG2). Astrobiology 14, 887–968. doi: 10.1089/ast.2014.1227
Russell, J. B. (2007). The energy spilling reactions of bacteria and other organisms. J. Mol. Microbiol. Biotechnol. 13, 1–11. doi: 10.1159/000103591
Sattley, W. M., and Madigan, M. T. (2006). Isolation, characterization, and ecology of cold-active, chemolithotrophic, sulfur-oxidizing bacteria from perennially ice-covered Lake Fryxell, Antarctica. Appl. Environ. Microbiol. 72, 5562–5568. doi: 10.1128/AEM.00702-06
Seto, M., and Iwasa, Y. (2019a). Population dynamics of chemotrophs in anaerobic conditions where the metabolic energy acquisition per redox reaction is limited. J. Theor. Biol. 467, 164–173. doi: 10.1016/j.jtbi.2019.01.037
Seto, M., and Iwasa, Y. (2019b). The fitness of chemotrophs increases when their catabolic by-products are consumed by other species. Ecol. Lett. 22, 1994–2005. doi: 10.1111/ele.13397
Seto, M., and Iwasa, Y. (2020). Microbial material cycling, energetic constraints and ecosystem expansion in subsurface ecosystems. Proc. R. Soc. B Biol. Sci. 287:20200610. doi: 10.1098/rspb.2020.0610
Silvertown, J. (2004). Plant coexistence and the niche. Trends Ecol. Evol. 19, 605–611. doi: 10.1016/j.tree.2004.09.003
Six, C., Finkel, Z. V., Irwin, A. J., and Campbell, D. A. (2007). Light variability illuminates niche-partitioning among marine picocyanobacteria. PLoS One 12:e1341. doi: 10.1371/journal.pone.0001341
Sobolev, D., and Roden, E. E. (2002). Evidence for rapid microscale bacterial redox cycling of iron in circumneutral environments. Antonie Van Leeuwenhoek 81, 587–597. doi: 10.1023/A:1020569908536
Sorokin, D. Y., and Kuenen, J. G. (2005). Chemolithotrophic haloalkaliphiles from soda lakes. FEMS Microbiol. Ecol. 52, 287–295. doi: 10.1016/j.femsec.2005.02.012
Sterck, F., Markesteijn, L., Schieving, F., and Poorter, L. (2011). Functional traits determine trade-offs and niches in a tropical forest community. Proc. Natl. Acad. Sci. U.S.A. 108, 20627–20632. doi: 10.1073/pnas.1106950108
Stevens, T. (1997). Lithoautotrophy in the subsurface. FEMS Microbiol. Rev. 20, 327–337. doi: 10.1016/S0168-6445(97)00015-6
Takizawa, K., Kanazawa, A., and Kramer, D. M. (2008). Depletion of stromal Pi induces high “energy-dependent” antenna exciton quenching (qE) by decreasing proton conductivity at CF O-CF1 ATP synthase. Plant Cell Environ. 31, 235–243. doi: 10.1111/j.1365-3040.2007.01753.x
Tanikawa, W., Tadai, O., Morono, Y., Hinrichs, K. U., and Inagaki, F. (2018). Geophysical constraints on microbial biomass in subseafloor sediments and coal seams down to 2.5 km off Shimokita Peninsula, Japan. Prog. Earth Planet. Sci. 5:58. doi: 10.1186/s40645-018-0217-2
Thébault, E., and Loreau, M. (2003). Food-web constraints on biodiversity-ecosystem functioning relationships. Proc. Natl. Acad. Sci. U.S.A. 100, 14949–14954. doi: 10.1073/pnas.2434847100
Thrash, J. C., and Coates, J. D. (2008). Review: direct and indirect electrical stimulation of microbial metabolism. Environ. Sci. Technol. 42, 3921–3931. doi: 10.1021/es702668w
Tilman, D. (1982). Resource Competition and Community Structure. Princeton, NJ: Princeton University Press.
Tilman, D., Reich, P. B., Knops, J., Wedin, D., Mielke, T., and Lehman, C. (2001). Diversity and productivity in a long-term grassland experiment. Science 294, 843–845. doi: 10.1126/science.1060391
Torres, C. I., Marcus, A. K., Lee, H. S., Parameswaran, P., Krajmalnik-Brown, R., and Rittmann, B. E. (2010). A kinetic perspective on extracellular electron transfer by anode-respiring bacteria. FEMS Microbiol. Rev. 34, 3–17. doi: 10.1111/j.1574-6976.2009.00191.x
Trembath-Reichert, E., Morono, Y., Ijiri, A., Hoshino, T., Dawson, K. S., Inagaki, F., et al. (2017). Methyl-compound use and slow growth characterize microbial life in 2-km-deep subseafloor coal and shale beds. Proc. Natl. Acad. Sci. U.S.A. 114, E9206–E9215. doi: 10.1073/pnas.1707525114
Valentine, D. L. (2007). Adaptations to energy stress dictate the ecology and evolution of the Archaea. Nat. Rev. Microbiol. 5, 316–323. doi: 10.1038/nrmicro1619
Vazquez, A., Liu, J., Zhou, Y., and Oltvai, Z. N. (2010). Catabolic efficiency of aerobic glycolysis: the Warburg effect revisited. BMC Syst. Biol. 4:58. doi: 10.1186/1752-0509-4-58
Webster, G., Embley, T. M., Freitag, T. E., Smith, Z., and Prosser, J. I. (2005). Links between ammonia oxidizer species composition, functional diversity and nitrification kinetics in grassland soils. Environ. Microbiol. 7, 676–684. doi: 10.1111/j.1462-2920.2005.00740.x
Weiss, M. C., Sousa, F. L., Mrnjavac, N., Neukirchen, S., Roettger, M., Nelson-Sathi, S., et al. (2016). The physiology and habitat of the last universal common ancestor. Nat. Microbiol. 1:16116. doi: 10.1038/nmicrobiol.2016.116
Wertz, S., Degrange, V., Prosser, J. I., Poly, F., Commeaux, C., Freitag, T., et al. (2006). Maintenance of soil functioning following erosion of microbial diversity. Environ. Microbiol. 8, 2162–2169. doi: 10.1111/j.1462-2920.2006.01098.x
Wright, R. H. G., Lioutas, A., Le Dily, F., Soronellas, D., Pohl, A., Bonet, J., et al. (2016). ADP-ribose-derived nuclear ATP synthesis by NUDIX5 is required for chromatin remodeling. Science 352, 1221–1225. doi: 10.1126/science.aad9335
Keywords: microbial ecology, mutualism, mathematical models, abundant resource premiums, syntrophy, chemolithotrophy
Citation: Seto M and Iwasa Y (2020) How Thermodynamics Illuminates Population Interactions in Microbial Communities. Front. Ecol. Evol. 8:602809. doi: 10.3389/fevo.2020.602809
Received: 04 September 2020; Accepted: 10 November 2020;
Published: 30 November 2020.
Edited by:
John Maxwell Halley, University of Ioannina, GreeceReviewed by:
Nikolaos Monokrousos, International Hellenic University, GreeceStavros D. Veresoglou, Freie Universität Berlin, Germany
Copyright © 2020 Seto and Iwasa. This is an open-access article distributed under the terms of the Creative Commons Attribution License (CC BY). The use, distribution or reproduction in other forums is permitted, provided the original author(s) and the copyright owner(s) are credited and that the original publication in this journal is cited, in accordance with accepted academic practice. No use, distribution or reproduction is permitted which does not comply with these terms.
*Correspondence: Mayumi Seto, c2V0b0BpY3MubmFyYS13dS5hYy5qcA==