- 1College of Forestry/Wuzhishan National Long-Term Forest Ecosystem Monitoring Research Station, Hainan University, Haikou, China
- 2Key Laboratory of Genetics and Germplasm Innovation of Tropical Special Forest Trees and Ornamental Plants (Hainan University), Ministry of Education, College of Forestry, Hainan University, Haikou, China
- 3Key Laboratory of Vegetation Restoration and Management of Degraded Ecosystem, South China Botanical Garden, Chinese Academy of Sciences, Guangzhou, China
Deforestation continues to be extensive in the tropics, resulting in reduced soil water content. Reforestation is an effective way to recover soil water content, but the recovery depends on the type of reforestation efforts that are implemented. Monoculture of fast-growing species is a common reforestation strategy, because it is an effective means of preventing landslides resulting from the frequent typhoons and heavy rains in the tropics and easy to implement. To quantify whether monoculture plantings can help recover soil water content, we initiated a reforestation project within a 0.2 km2 area of an extremely degraded tropical monsoon forest. We hypothesized that much higher transpiration rate of fast-growing tree species would deplete soil water more than the dominant slow-growing species in the adjacent secondary tropical rain forest during both wet and dry seasons, thereby resulting in much lower soil water content. To test this hypothesis, we compared transpiration rates and key functional traits that can distinguish transpiration rates between fast-growing and dominant slow-growing species in both wet and dry seasons. We also quantified whether soil water content around these species differed. We found that fast-growing species had transpiration rate and transpiration-related trait values that were 5–10 times greater than the dominant slow-growing species in both seasons. We also found that soil water content around dominant slow-growing species was 1.5–3 times greater than for fast-growing species in both seasons. Therefore, reforestation based on monoculture plantings of fast-growing species seems difficult to effectively recover the soil water content. We also provide a simple method for guiding the use of reforestation efforts to recover soil water content in extremely degraded tropical rain forests. We expect that this simple method can be an effective means to restore extremely degraded tropical rain forests in other parts of the world.
Introduction
Forests provide sustainable and high quality freshwater across the globe (Vose, 2019). Forests store significant amounts of water in soils, and are thus a key regulator of the global freshwater supply (Evaristo and McDonnell, 2019). Compared to agricultural and grassland ecosystems, forests have extensive and deep root systems and the soil is characterized by a thick leaf litter layers, which allow them to retain high levels of soil water content (Neary et al., 2009). Together, these properties of forests result in high soil infiltration rates and low soil surface runoff (Neary et al., 2009). Of the precipitation infiltrating in the soil, a fraction is taken up by roots and transpired, while the rest is stored in deep soil layers to provide freshwater to human society via subsurface runoff (Jackson, 2006). As a result, soil water content stored in forests is vital for providing sustainable freshwater to human society (Chapin et al., 2011).
Past and current human disturbances (e.g., ore mining, and plantation of commercial trees) have resulted in high rates of deforestation and ecosystem degradation across the world, which have, in turn, resulted in a major threat to the global supply of freshwater (Sahin and Hall, 2004; Foley et al., 2011; Lambin and Meyfroidt, 2011; Vörösmarty et al., 2015). It is expected that deforestation in the tropics will continue to accelerate in the near future (Laurance et al., 2014), and a large amount of the water stored in forest soils may thus be lost as a result. Indeed, many studies have found that extensive deforestation in the tropics has already led to large-scale losses of freshwater in these systems (Castello and Macedo, 2016; Fugère et al., 2016; Luke et al., 2017; Gebrehiwot et al., 2019; Mapulanga and Naito, 2019). It is thus urgent to initiate and maintain reforestation projects aimed at recovering soil water content and increasing freshwater supply to human society (Chapin et al., 2011).
A serious challenge in managing soil water loss after degradation in tropics is that frequent typhoons and heavy rains can result in landslides that remove the soil layer (Guidicini and Iwasa, 1977; Chang et al., 2008; Yumul et al., 2012; Acosta et al., 2016; Villamayor et al., 2016). Such landslide events may make reforestation of large areas extremely difficult. To alleviate these problems, monocultures of fast-growing tree species with high survival rates perform better than slower-growing late-successional tropical tree species (Stokes et al., 2009; Walker et al., 2009; Lohbeck et al., 2013; Pang et al., 2018). As a result, monocultures of fast-growing tree species that are easy to cultivate are used extensively in reforestation projects across the tropics (Lu et al., 2017).
Although it is widely believed that reforestation will recover the soil water content (Bruijnzeel, 2004), only the reforestation in Flores, eastern Indonesia is proven to have successfully restored soil water content, as mentioned by Carson (1989) and Nooteboom (1987). Moreover, whether reforestation can recover the soil water content depends on time and spatial scales (Bruijnzeel, 2004). As a result, it is expected that reforestation based on monocultures of fast-growing species may be not able to recover soil water content in highly degraded tropical forest. Nevertheless, nearly no study has evaluated the influence of large-scale reforestation projects on soil water content recovery in humid tropical regions (Solange et al., 2017).
Plant functional traits including morphological and physiological traits across species can directly capturing critical dimensions of the vulnerability and response to seasonal drought across species and ecosystems (Maherali et al., 2004; Engelbrecht et al., 2007; Anderegg, 2014), comparing the differences in functional traits between fast-growing tree species used for reforestation and dominant slow-growing tree species in the secondary tropical rain forest can reveal whether and why reforestation with fast-growing tree species can recover soil water content in highly degraded tropical rain forest. It has been found that fast-growing tree species usually have higher transpiration rates than the dominant slower growing tree species in secondary tropical rain forests (Tardieu and Parent, 2017), which often leads to higher rates of soil water uptake for fast-growing species (Krishnaswamy et al., 2013). These higher transpiration rates are expected to result in lower soil water content in monoculture stands of fast-growing tree species (Evaristo and McDonnell, 2019). Thus, we hypothesized that during the wet season, fast-growing tree species used for reforestation will have higher transpiration rates than dominant slower-growing species in the secondary tropical rain forest. As a result, we expect soil water content around fast-growing species to be much lower than for dominant slow-growing species (Figure 1). In the dry season, we expect that dominant slow-growing species will exhibit suppressed transpiration rates which make soil water content around slow-growing species decline only slightly during the dry season. In contrast, for fast-growing tree species, high transpiration rates are required to meet their very high growth rates, which is impossible when precipitation is limited. As a result, fast-growing tree species must absorb large amounts of water from soils formed because of stored precipitation in the wet season in the soil layers. In such cases, we expect soil water content around fast-growing species to decline precipitously during the dry season.
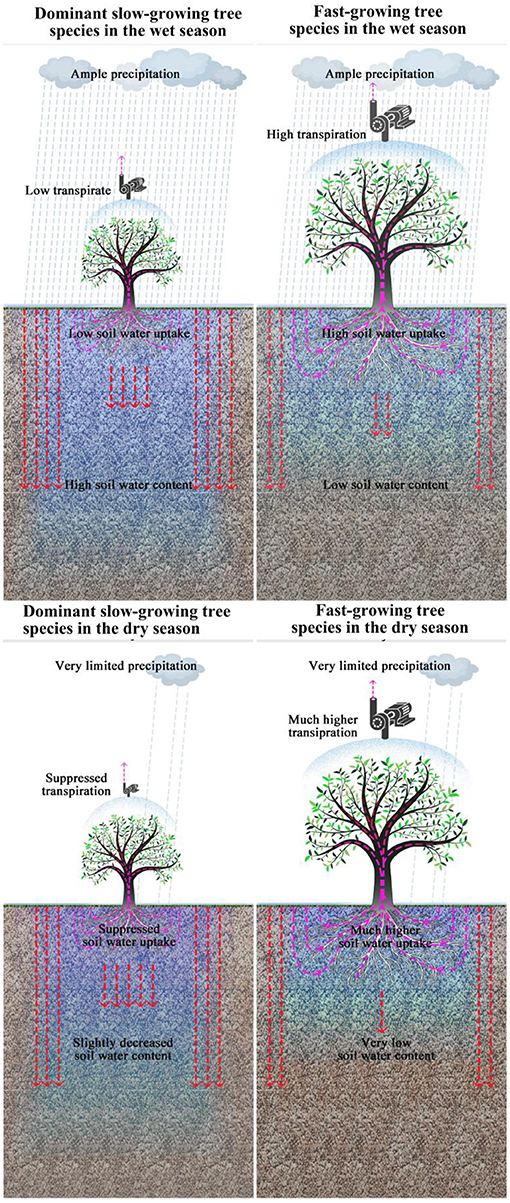
Figure 1. Hypothesized differences in transpiration, soil water uptake, and soil water content between fast-growing tree species used for reforestation in the 0.2 km2 extremely degraded tropical rain forest and dominant slow-growing species in the adjacent secondary tropical rain forest in wet and dry season respectively.
To test our hypothesis, we initiated a large-scale reforestation project used to restore a 0.2 km2 area of extremely degraded tropical rain forest in Sanya City, Hainan, China in 2016. Outside of this degraded area is secondary tropical rain forest. To prevent landslides, we planted monocultures of eight fast-growing tree species separately. This project thus provides a suitable system in which to test whether monocultures of fast-growing species can help recover soil water content compared with that in the secondary tropical rain forest. Specifically, we compared differences in transpiration rates between the eight fast-growing tree species used for reforestation and eight dominant slow-growing tree species sampled from the secondary tropical rain forest. Separate comparisons were made in both the wet and dry seasons. We also determined whether water content differed between soils surrounding fast-growing vs. slow-growing tree species in both seasons. Tree transpiration rates can be determined by using a suite of functional traits associated with plant transpiration, including photosynthesis rate, stomatal conductance, leaf hydraulic conductivity, and drought stress tolerance (Tuzet et al., 2003; Miyashita et al., 2005; Fisher et al., 2007; Maherali et al., 2008; Santos et al., 2018). Therefore, we also compared these functional traits between fast-growing species used for reforestation and dominant slow-growing species in the secondary tropical rain forest in both wet and dry seasons to reveal which factors regulate transpiration for these two type of trees across wet and dry season.
Materials and Methods
Study Site
Our study site is located on Baopoling mountain (BPL), which is a limestone mountain in Sanya City, Hainan, China (109°51′01′′E, 18°31′99′′N). The area has a tropical monsoon oceanic climate with a mean annual temperature of ~28°C. The average annual precipitation on the island is 1,500 mm, ~91% of which occurs from June to October (Luo et al., 2020). Vegetation of the BPL is described as species-rich tropical rain broad-leaf forest (Luo et al., 2020). Due to 20 years of limestone mining by the cement industry, a 0.2 km2 area of the BPL has now become extremely degraded, consisting of bare rocky soils where minimal plant life is found. Areas of the BPL outside of the degraded site are composed of secondary tropical rain forest (see Figure 2A). Here we utilized a nearly natural reforestation method to perform the reforestation to restored the extremely degraded tropical rainforest and detail reforestation processes are described in detail as below. In May 2016, reforestation was firstly enacted by using the slope and the deep soil layer for the secondary tropical forest as a reference to reconstruct slope and soil layers (Figures 2B,C). Then separately refiling the same soils from the secondary tropical monsoon forest to monoculture seedlings [3 m height and 2 cm diameter at breast height (DBH)] of eight fast-growing species, which included Terminalia neotaliala, Bombax malabarica, Cleistanthus sumatranus, Ficus microcarpa, Muntingia colabura, Acacia mangium, Leucaena glauca, and Bougainvillea spectabilis (Figures 2C–E). Seedlings from these eight fast-growing species can be purchased commercially, and are known to be fast-growing and have high survival rates within the study region. Thus, these eight fast-growing species should have high potential to prevent landslide resulting from frequent typhoon and heavy rain. The eight fast-growing species was separately monocultured from the top to the bottom of BPL (Figure 2D) and planting density for each of the eight species was all kept at 80 stems per hectare. The restoration project was finished at end of year 2016. In 2019, thirty plots, each of 20 × 20 m2 (an area of 400 m2 for each plot) that were at least 100–300 m apart from one another, were randomly sampled across the adjacent secondary forest. Within each plot, all freestanding trees with diameter of ≥ 1 cm at breast height (DBH) were measured and identified to species. We finally found 80 tree species in the secondary forest and we select the 8 tree species (200–300 stems per hectare) (Bridelia tomentosa, Radermachera frondosa, Lepisanthes rubiginosa, Rhaphiolepis indica, Pterospermum heterophyllum, Fissistigma oldhamii, Psychotria rubra, and Cudrania cochinchinensis) as our candidate dominant slow-growing species.
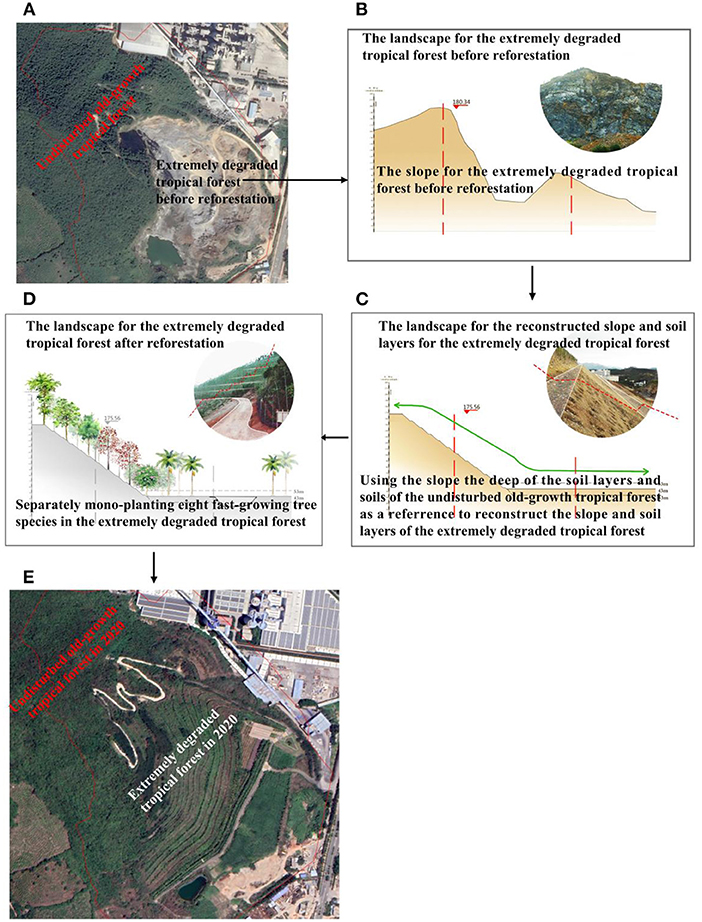
Figure 2. Baopoling mountain (BPL), the surrounding landscape and detailed procedures regarding the reforestation project in the 0.2 km2 extremely degraded tropical monsoon forest. (A) BPL and the surrounding landscape including the 0.2 km2 area of extremely degraded tropical monsoon forest and the adjacent secondary tropical monsoon forests. (B) the original landscape and slope of the 0.2 km2 extremely degraded tropical monsoon forest; (C) using the slope, the deep of soil layers as a reference to reconstructing slope and soil layers, and to refill soils from the secondary tropical forests to form planting area in the extremely degraded tropical monsoon forest; (D) separately mono-planting eight fast-growing tropical tree species; (E) the landscape of secondary tropical rain forest and the 0.2 km2 extremely degraded tropical rain forest after reforestation efforts in 2020.
Sampling
In the peak of wet season (August) in 2019, we sampled 20 fully expanded, healthy leaves from the same five independent individuals for each of the eight fast-growing species and eight dominant slow-growing species found in the surrounding secondary region. Resampling was performed again in the dry season (February) in 2020. We selected trees that had a DBH that was comparable to the mean DBH of the species (Table 1). Leaf samples were used to measure five hydraulic traits, including transpiration rate (TR; μmol m−2 s−1), maximum photosynthesis rate (Aarea; μmol m−2 s−1), stomatal conductance (SC; mmol m−2 s−1), leaf hydraulic conductivity (LHC; mmol m−2 s−1 MPa−1), and leaf turgor loss point (TLP; Mpa).
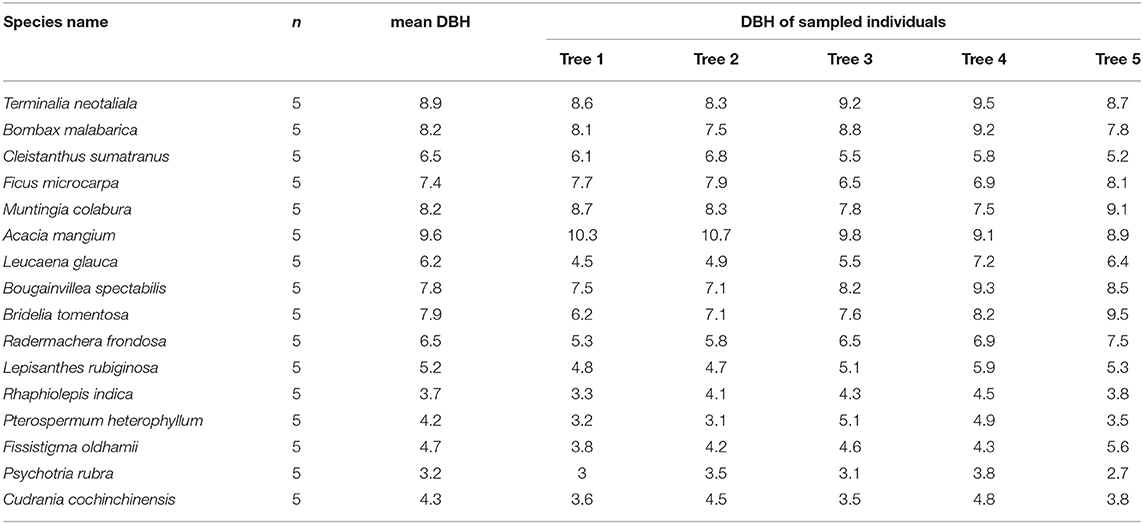
Table 1. The mean diameter at the breast height DBH (in cm) of the eight non-native species (Terminalia neotaliala, Bombax malabarica, Cleistanthus sumatranus, Ficus microcarpa, Muntingia colabura, Acacia mangium, Leucaena glauca, and Bougainvillea spectabilis) and the eight dominant native species (Bridelia tomentosa, Radermachera frondosa, Lepisanthes rubiginosa, Rhaphiolepis indica, Pterospermum heterophyllum, Fissistigma oldhamii, Psychotria rubra, and Cudrania cochinchinensis) and the DBH values of the sampled individuals (n) of each study species.
Measurement of Maximum Photosynthesis Rate, Stomatal Conductance, and Transpiration Rate
We used a Li-6400 portable photosynthesis system (Li-6400, Li-Cor, Lincoln, Nebraska, USA) to measure maximum photosynthesis rate, transpiration rate, and stomatal conductance for the 20 fully expanded and sun-exposed leaves collected from five mature individuals between 9:00 and 11:00 on sunny days. We set photosynthetic photon flux density at 1,500 μmol m−2 s−1 to ensure that light-saturated photosynthetic rates were measured for all forest species. We maintained ambient CO2 and air temperature at 390 μmol mol−1 and 28°C, respectively. Before data were recorded, we exposed leaves to the above conditions for about 5 min to allow photosynthetic parameters to stabilize.
Measurement of Leaf Hydraulic Conductivity and Leaf Turgor Loss Point
We collected leaf-bearing branches from five individuals of each forest species where the basal ends of the branches were immersed in distilled water and re-cut to measure leaf hydraulic conductivity and leaf turgor loss point. We rehydrated all branch samples until leaf water potential was greater than −0.05 MPa. Then 20 fully expanded and healthy leaves were selected to measure leaf hydraulic conductivity using rehydration technique following Brodribb and Holbrook (2003). We also selected another 20 fully expanded and health leaves to determine leaf turgor loss point using the pressure-volume relationship analysis program developed by Schulte and Hinckley (1985).
Measurement of Soil Water Content in Both Wet and Dry Seasons
We first randomly selected three individuals for each of the eight fast-growing and eight dominant forest species in the secondary forest. Then we collected one soil sample at a depth of 0–100 cm in soils around each focal individual every day in the peak of wet (August) in 2019 and dry seasons (February) in 2020, respectively to measure gravimetric soil water content (g kg−1) in both sites in both wet and dry seasons, respectively.
Statistical Analyses
We used a Wilcoxon signed-rank test to detect differences in functional traits values between the dominant slow-growing and fast-growing species. Separate comparisons were also made within the dry and wet seasons. We also utilized Wilcoxon signed-rank test to compare soil water content in soils surrounding the fast-growing and slow-growing species. As above, comparisons were made in both wet and dry seasons.
Results
The restoration project was finished in 2016, and till now typhoons and heavy rains during the wet season have not resulted in destructive landslides (Figure 1E). DBH for these eight fast-growing forest species are 2.2 times higher than the dominant slow-growing forest species in the secondary tropical rain forest in 2020 (Table 1). For dominant slow-growing species, all five traits were lower during the dry season than in the wet season (Figure 3, p < 0.001 based on Wilcoxon signed-rank tests). In contrast, only transpiration rate and leaf hydraulic conductivity were different for fast-growing species (Figure 3, p < 0.001). During the dry season, functional traits values of fast-growing species were 1.5 to 10 times higher than those of dominant slow-growing species (Figure 4, p < 0.001). And during the wet season, most functional traits values for fast-growing species were 3 to 10 times higher than for dominant slow-growing species. However, we did not observe any difference in leaf turgor loss point between fast-growing and dominant slow-growing species (Figure 4, p > 0.05).
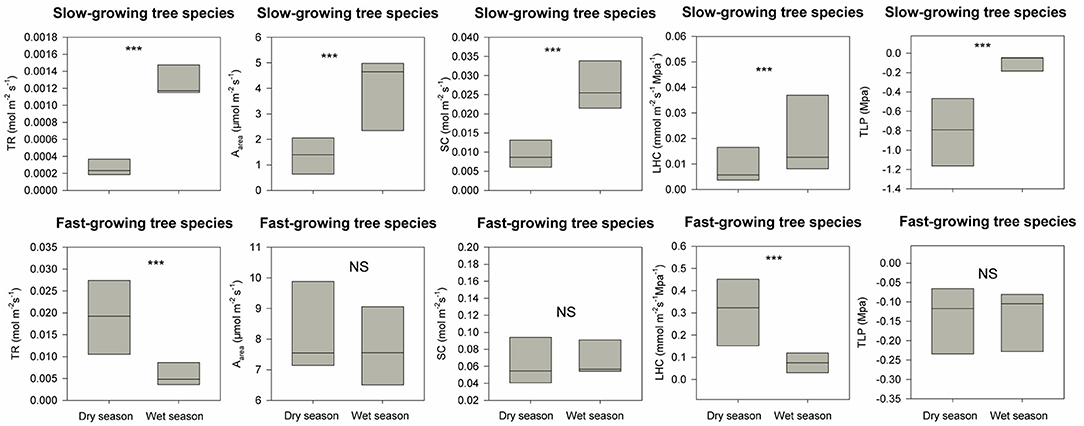
Figure 3. Differences in five hydraulic traits (i.e., transpiration rate, TR; maximum photosynthesis rate, Aarea; stomatal conductance, SC; leaf hydraulic conductivity, LHC; leaf turgor loss point, TLP) between the wet and dry season for both the fast-growing species used for reforestation and the dominant slow-growing species in the adjacent secondary tropical rain forest. *** indicates P < 0.05 and NS (short for non-significant) indicates P > 0.05 based on Wilcoxon signed-rank tests.
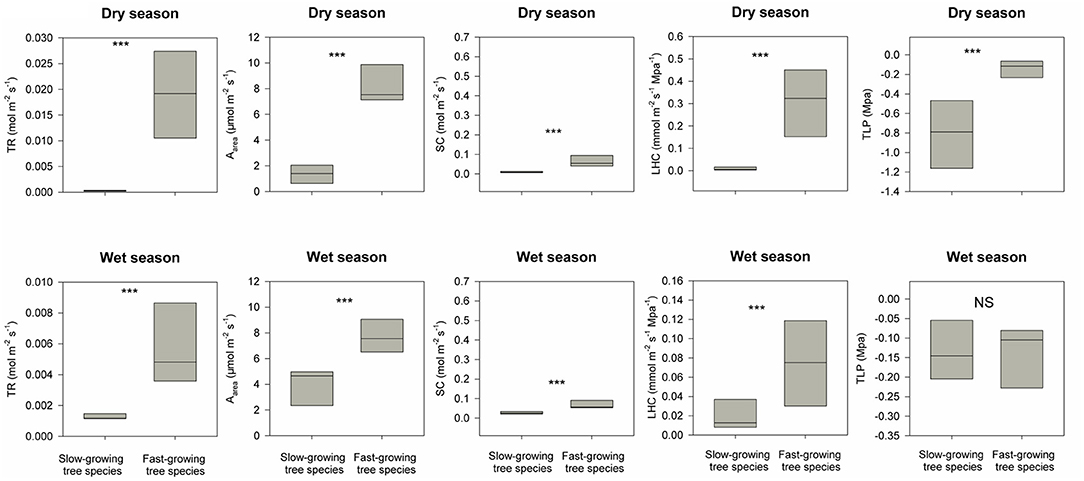
Figure 4. Differences in distributions of five hydraulic traits (i.e., transpiration rate, TR; maximum photosynthesis rate, Aarea; stomatal conductance, SC; leaf hydraulic conductivity, LHC; leaf turgor loss point, TLP) between the fast-growing species used for reforestation and dominant slow-growing species in the adjacent secondary tropical rain forest in dry and wet seasons respectively. *** indicates P < 0.05 and NS (short for non-significant) indicates P > 0.05 based on Wilcoxon signed-rank tests.
Soil water content around both the fast-growing and dominant slow-growing species decrease significantly from wet to dry seasons, with an 8% decrease in dominant slow-growing species, but a 190% decrease in fast-growing species (Figures 5A,B). That is, soil water content around the dominant slow-growing species remained almost constant across the seasons, whereas soil water content surrounding the fast-growing species decline dramatically from the wet to dry seasons. Soil water content in soils around the dominant slow-growing species in the secondary tropical rainforest ecosystem was as much as 1.5–3 times higher than in soils around the fast-growing species in the restoration area in the both wet and dry seasons (Figures 5C,D). Moreover, soil water content surrounding the dominant slow-growing species in the dry season was still 1.3 times higher than that for the fast-growing species in the wet season (Figure 5E).
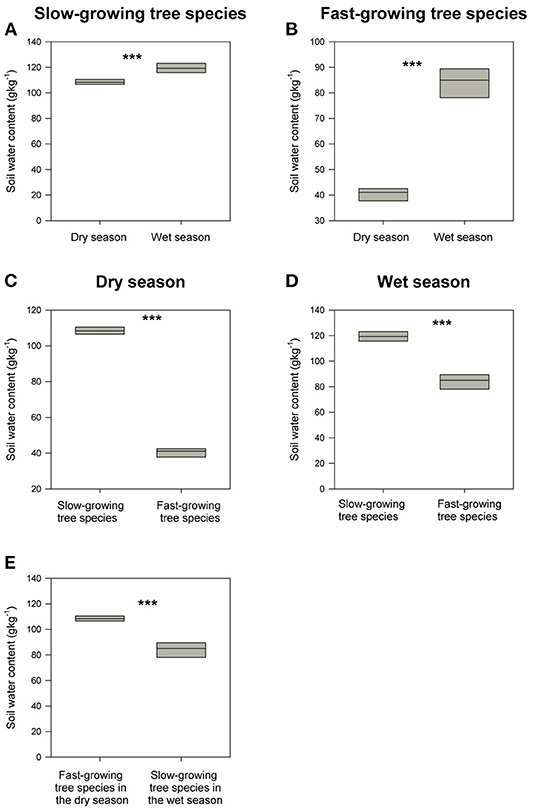
Figure 5. (A,B) demonstrates the differences in soil water content between wet and dry season for slow-growing tree species and fast-growing tree species respectively. (C,D) shows the differences in soil water content between slow-growing tree species and fast-growing tree species in wet and dry season individually. (E) describes the differences in soil water content between fast-growing tree species in the dry season and slow-growing tree species in the wet season. ***indicates P < 0.05 and NS (short for non-significant) indicates P > 0.05 based on Wilcoxon signed-rank tests.
Discussion
Our study provides convincing evidence that transpiration rates of the fast-growing species used for reforestation are much higher than that of the dominant slow-growing species in the secondary tropical rain forest, in both wet and dry seasons. This results in more uptake of water from the soil by the fast-growing species than by the slow-growing species, ultimately lower soil water content around the fast-growing species than that around the dominant slow-growing species, particularly during the dry season.
We found DBH for these eight fast-growing forest species are 2.2 times higher than the dominant slow-growing forest species in secondary tropical rain forest, indicating our selected fast-growing species can indeed grow much faster than the dominant forest species in the adjacent secondary tropical rainforest. We also found typhoons and heavy rains during the wet season had not resulted in destructive landslides on the reforestation project which was implemented by separately mono-planting these eight fast-growing forest species. These results demonstrated that mono-planting fast-growing species can indeed be effective for alleviating the potential landslide when performing reforestation in tropics due to frequent typhoon and heavy rain. (Stokes et al., 2009; Walker et al., 2009; Pang et al., 2018).
During the wet season, the fast-growing species had a much higher photosynthesis rate and stomatal conductivity than the dominant slow-growing species. Higher photosynthesis rates and stomatal conductance usually require higher leaf hydraulic conductivity to maintain high rates of transpiration (Tuzet et al., 2003; Miyashita et al., 2005; Maherali et al., 2008). Indeed, we found that the fast-growing species had much higher leaf hydraulic conductivity and transpiration rates than those of the dominant slow-growing species during the wet season. This suggests that the fast-growing species have much higher photosynthetic water demands and transpiration rates than the dominant slow-growing tree species. However, we observed no significant differences in leaf turgor loss point between them. Leaf turgor loss point reflects leaf water supply, with low leaf turgor loss point when plant leaf water supply is not enough (Bartlett et al., 2012). This indicated that leaf water supply was likely adequate for both types of trees. This is not surprising, as 90% precipitation occur in the wet season (Luo et al., 2020), which should meet the water demand of the trees. Nevertheless, higher plant transpiration rates will result in higher soil water uptake, which will lead to overall lower soil water content (Krishnaswamy et al., 2013; Peña-Arancibia et al., 2019). Indeed, we found that mean soil water content around the dominant slow-growing species was 1.5 times higher than in soils surrounding the fast-growing species. Thus, fast-growing species' much higher transpiration rates can indeed result in much lower soil water content, although precipitation is not limited.
During the dry season, it is likely that leaf water supply was insufficient for both fast-growing and dominant slow-growing species. However, we found that dominant slow-growing species had a much lower leaf turgor loss point during the dry season, while for fast-growing species it did not differ between seasons. This suggests that fast-growing species likely get adequate leaf water supply, while dominant slow-growing species do not. This was surprising, given that photosynthesis rate and stomatal conductivity was very high for fast-growing species during the dry season. This should make leaf water supply for fast-growing species not be fulfilled by the very limited precipitation in the dry season. Thus, fast-growing species must have a high leaf hydraulic conductivity, resulting in a high transpiration rate to meet their invariably high photosynthetic water demand. Indeed, we found that the fast-growing species had much higher leaf hydraulic conductivity and transpiration rates than in the wet season. As a result, the fast-growing species absorbed higher amounts of soil water than the dominant slow-growing species. Actually, we found that soil water content around fast-growing species during the dry season were only half of the amount observed during the wet season. Moreover, soil water content around the dominant slow-growing species during the dry season was also much higher than the fast-growing species. These results demonstrate that the high photosynthetic water demand of the fast-growing species cannot be fulfilled during the dry season without uptaking large amounts of water stored in soils. As a result, we expect that widespread plantings of fast-growing species will lead to long-term depletion of water in forest ecosystems.
A lower leaf turgor loss point also causes suppressed photosynthetic water demand, which results in suppressed leaf hydraulic conductivity and transpiration rates (Santos et al., 2018). Indeed, we found that dominant slow-growing species had much higher rates of leaf hydraulic conductivity and transpiration during the wet season than in the dry season. It is possible that dominant slow-growing species' suppressed transpiration rates can be sufficiently met by the limited water supply. Thus, dominant slow-growing species only requires to absorb a small amount of soil water content formed because of stored precipitation in the wet season in the soil layers. Indeed, we found that soil water content in soils around dominant slow-growing species decreased merely 8% in the dry season compared with the wet season. These results indicate that dominant slow-growing species' suppressed transpiration rates by lowering leaf turgor loss point can be largely met by the limited precipitation and thus they only absorb a small amount of soil water content formed in the wet season, thereby maintaining relatively high soil water content in the dry season.
Together, our results suggest that using monocultures of fast-growing tree species for large scale reforestation projects is difficult to recover the soil water content. Because of dominant species' lower transpiration rates, mixtures of fast-growing and dominant slow-growing species may be useful. Indeed, many studies from other sites have found that planting mixtures of the dominant slow-growing plant species and fast-growing species can enhance soil water content capacity (Sprenger et al., 2013; Amazonas et al., 2018; Jonsson et al., 2019). We expect that at our site, mixing dominant slow-growing species with the fast-growing monocultures would be useful for recovering soil water content. Still, initial plantings of fast-growing species remains important for preventing adverse effects from landslides and heavy rains (Stokes et al., 2009; Walker et al., 2009; Pang et al., 2018).
Based on all findings, we recommend a three-step method for recovering soil water content of extremely degraded tropical forest ecosystems via reforestation. First, using the slope, the deep of soil layers of the secondary tropical forest as a reference to reconstruct slope and soil layers. Then refiling the same soils from the secondary tropical monsoon forest to plant fast-growing tree species to minimize impacts from landslides and other soil disturbance events. Third, dominant slow-growing tree species from the adjacent secondary area should be planted within the fast-growing species stands to increase soil water content. We expect that this simple three-steps method can be an effective means of restoring extremely degraded tropical forests in other parts of the world.
Conclusion
Here we clearly demonstrate that our nearly nature reforestation method can help quickly and successfully perform reforestation to restore extremely degraded tropical rainforest. However, native forest species should be further mixed to effectively recover the soil water content. We also provide a simple method for guiding the use of reforestation efforts to recover soil water content in extremely degraded tropical rain forests.
Data Availability Statement
The original contributions presented in the study are included in the article/supplementary material, further inquiries can be directed to the corresponding author/s.
Author Contributions
TL, HZ, and CW: designed research, analyzed data, and wrote the paper. KJ, ZT, and QH: performed research. All authors contributed to the article and approved the submitted version.
Funding
This work was funded by Scientific research project of ecological restoration of Baopoling mountain in Sanya and start-up fund from Hainan University (KYQD (ZR) 1863 and KYQD (ZR) 1876).
Conflict of Interest
The authors declare that the research was conducted in the absence of any commercial or financial relationships that could be construed as a potential conflict of interest.
References
Acosta, L. A., Eugenio, E. A., Macandog, P. B. M., Macandog, D. B. M., Lin, E. K. H., Abucay, E. R., et al. (2016). Loss and damage from typhoon-induced floods and landslides in the Philippines: community Perceptions on climate impacts and adaptation options. Int. J. Glob. Warm. 9, 33–65. doi: 10.1504/ijgw.2016.074307
Amazonas, N. T., Forrester, D. I., Oliveira, R. S., and Brancalion, P. H. S. (2018). Combining Eucalyptus wood production with the recovery of native tree diversity in mixed plantings: implications for water use and availability. For. Ecol. Manage. 418, 34–40. doi: 10.1016/j.foreco.2017.12.006
Anderegg, W. R. (2014). Spatial and temporal variation in plant hydraulic traits and their relevance for climate change impacts on vegetation. New Phytol. 205, 1008–1014. doi: 10.1111/nph.12907
Bartlett, M. K., Scoffoni, C., and Sack, L. (2012). The determinants of leaf turgor loss point and prediction of drought tolerance of species and biomes: a global meta-analysis. Ecol. Lett. 15, 393–405. doi: 10.1111/j.1461-0248.2012.01751.x
Brodribb, T. J., and Holbrook, N. M. (2003). Stomatal closure during leaf dehydration, correlation with other leaf physiological traits. Plant Physiol. 132, 2166–2173. doi: 10.1104/pp.103.023879
Bruijnzeel, L. A. (2004). Hydrological functions of tropical forests: not seeing the soil for the trees? Agric. Ecosyst. Environ. 104, 185–228. doi: 10.1016/j.agee.2004.01.015
Carson, B. (1989). Soil Conservation Strategies for Upland Areas of Indonesia. Occasional Paper No. 9. Honolulu, HI: East–West Center, Environment and Policy Institute, 120.
Castello, L., and Macedo, M. N. (2016). Large-scale degradation of Amazonian freshwater ecosystems. Glob. Change Biol. 22, 990–1007. doi: 10.1111/gcb.13173
Chang, K. T., Chiang, S. H., and Lei, F. (2008). Analysing the relationship between typhoon-triggered landslides and critical rainfall conditions. Earth Surf. Proc. Land 33, 1261–1271. doi: 10.1002/esp.1611
Chapin, F. S. III., Matson, P. A., and Vitousek, P. (2011). Principles of Terrestrial Ecosystem Ecology. New York, NY: Springer. doi: 10.1007/b97397
Engelbrecht, B. M., Comita, L. S., Condit, R., Kursar, T. A., Tyree, M. T., Turner, B. L., et al. (2007). Drought sensitivity shapes species distribution patterns in tropical forests. Nature 447, 80–82. doi: 10.1038/nature05747
Evaristo, J., and McDonnell, J. J. (2019). Global analysis of streamflow response to forest management. Nature 570, 455–461. doi: 10.1038/s41586-019-1306-0
Fisher, R. A., Williams, M., Da Costa, A. L., Malhi, Y., Da Costa, R. F., Almeida, S., et al. (2007). The response of an Eastern Amazonian rain forest to drought stress: results and modelling analyses from a throughfall exclusion experiment. Glob. Change Biol. 13, 2361–2378. doi: 10.1111/j.1365-2486.2007.01417.x
Foley, J. A., Ramankutty, N., Brauman, K. A., Cassidy, E. S., Gerber, J. S., Johnston, M., et al. (2011). Solutions for a cultivated planet. Nature 478, 337–342. doi: 10.1038/nature10452
Fugère, V., Nyboer, E. A., Bleecker, J. C., and Chapman, L. J. (2016). Impacts of forest loss on inland waters: identifying critical research zones based on deforestation rates, aquatic ecosystem services, and past research effort. Biol. Conserv. 201, 277–283. doi: 10.1016/j.biocon.2016.07.012
Gebrehiwot, S. G., Ellison, D., Bewket, W., Seleshi, Y., Inogwabini, B.-I., and Bishop, K. (2019). The Nile Basin waters and the West African rainforest: rethinking the boundaries. WIREs Water 6:e1317. doi: 10.1002/wat2.1317
Guidicini, G., and Iwasa, O. Y. (1977). Tentative correlation between rainfall and landslides in a humid tropical environment. B. Eng. Geol. Environ. 16, 13–20. doi: 10.1007/BF02591434
Jackson, C. R. (2006). “Wetland hydrology,” in Ecology of Freshwater and Estuarine Wetlands, eds D. P. Batzer and R. Sharitz (Berkeley, CA: University of California Press), 43–81.
Jonsson, M., Bengtsson, J., Gamfeldt, L., Moen, J., and Snäll, T. (2019). Levels of forest ecosystem services depend on specific mixtures of commercial tree species. Nat. Plants 5, 141–147. doi: 10.1038/s41477-018-0346-z
Krishnaswamy, J., Bonell, M., Venkatesh, B., Purandara, B., Rakesh, K. N., Lele, S., et al. (2013). The groundwater recharge response and hydrologic services of tropical humid forest ecosystems to use and reforestation: support for the “infiltration-evapotranspiration trade-off hypothesis”. J. Hydrol. 498, 191–209. doi: 10.1016/j.jhydrol.2013.06.034
Lambin, E. F., and Meyfroidt, P. (2011). Global land use change, economic globalization, and the looming land scarcity. Proc. Natl. Acad. Sci. U.S.A. 108, 3465–3472. doi: 10.1073/pnas.1100480108
Laurance, W. F., Sayer, J., and Cassman, K. G. (2014). Agricultural expansion and its impacts on tropical nature. Trends. Ecol. Evol. 29, 107–116. doi: 10.1016/j.tree.2013.12.001
Lohbeck, M., Poorter, L., Lebrija-Trejos, E., Martinez-Ramos, M., Meave, J. A., Paz, H., et al. (2013). Successional changes in functional composition contrast for dry and wet tropical forest. Ecology 94, 1211–1216. doi: 10.1890/12-1850.1
Lu, Y., Ranjitkar, S., Harrison, R. D., Xu, J., Ou, X., Ma, X., et al. (2017). Selection of native tree species for subtropical forest restoration in Southwest China. PLoS ONE 12:e0170418. doi: 10.1371/journal.pone.0170418
Luke, S. H., Barclay, H., Bidin, K., Chey, V. K., Ewers, R. M., Foster, W. A., et al. (2017). The effects of catchment and riparian forest quality on stream environmental conditions across a tropical rainforest and oil palm landscape in Malaysian Borneo. Ecohydrology 10:e1827. doi: 10.1002/eco.1827
Luo, J. H., Cui, J., Pandey, S. P., Jiang, K., Tan, Z. Y., He, Q. F., et al. (2020). Seasonally distinctive growth and drought stress functional traits enable Leucaena Leucocephala to successfully invade a Chinese tropical forest. Trop. Conserv. Sci. 13, 1–7. doi: 10.1177/1940082920949176
Maherali, H., Pockman, W. T., and Jackson, R. B. (2004). Adaptive variation in the vulnerability of woody plants to xylem cavitation. Ecology 85, 2184–2199. doi: 10.1890/02-0538
Maherali, H., Sherrard, M. E., Clifford, M. H., and Latta, R. G. (2008). Leaf hydraulic conductivity and photosynthesis are genetically correlated in an annual grass. New Phytol. 180, 240–247. doi: 10.1111/j.1469-8137.2008.02548.x
Mapulanga, A. M., and Naito, H. (2019). Effect of deforestation on access to clean drinking water. Proc. Natl. Acad. Sci. U.S.A. 116:8249. doi: 10.1073/pnas.1814970116
Miyashita, K., Tanakamaru, S., Maitani, T., and Kimura, K. (2005). Recovery responses of photosynthesis, transpiration, and stomatal conductance in kidney bean following drought stress. Environ. Exp. Bot. 53, 205–214. doi: 10.1016/j.envexpbot.2004.03.015
Neary, D. G., Ice, G. G., and Jackson, C. R. (2009). Linkages between forest soils and water quality and quantity. For. Ecol. Manage. 258, 2269–2281. doi: 10.1016/j.foreco.2009.05.027
Nooteboom, H. P. (1987). Further views on environmental impacts of (de)forestation in the humid tropics. Wallaceana 47, 10–11.
Pang, C. C., Ma, X. K.-K., Lo, J. P.-L., Hung, T. T.-H., and Hau, B. C.-H. (2018). Vegetation succession on landslides in Hong Kong: plant regeneration, survivorship and constraints to restoration. Glob. Ecol. Conserv. 15:e00428. doi: 10.1016/j.gecco.2018.e00428
Peña-Arancibia, J. L., Bruijnzeel, L. A., Mulligan, M., and van Dijk, A. I. J. M. (2019). Forests as ‘sponges’ and ‘pumps’: assessing the impact of deforestation on dry-season flows across the tropics. J. Hydrol. 574, 946–963. doi: 10.1016/j.jhydrol.2019.04.064
Sahin, V., and Hall, M. J. (2004). The effects of afforestation and deforestation on water yields. J. Hydrol. 178, 299–309. doi: 10.1016/0022-1694(95)02825-0
Santos, V. A. H. F.D., Ferreira, M. J., Rodrigues, J. V. F. C., Garcia, M. N., Ceron, J. V. B., Nelson, B. W., et al. (2018). Causes of reduced leaf-level photosynthesis during strong El Niño drought in a Central Amazon forest. Glob. Change Biol. 24, 4266–4279. doi: 10.1111/gcb.14293
Schulte, P., and Hinckley, T. (1985). A comparison of pressure-volume curve data analysis techniques. J. Exp. Bot. 36, 1590–1602. doi: 10.1093/jxb/36.10.1590
Solange, F., Ometto, B. M., Weiss, K. C. B., Palmer, M. A., and Silva, L. C. R. (2017). Impacts of forest restoration on water yield: a systematic review. PLoS ONE 12:e0183210. doi: 10.1371/journal.pone.0183210
Sprenger, M., Oelmann, Y., Weihermüller, L., Wolf, S., Wilcke, W., and Potvin, C. (2013). Tree species and diversity effects on soil water seepage in a tropical plantation. For. Ecol. Manage. 309, 76–86. doi: 10.1016/j.foreco.2013.03.022
Stokes, A., Atger, C., Bengough, A. G., Fourcaud, T., and Sidle, R. C. (2009). Desirable plant root traits for protecting natural and engineered slopes against landslides. Plant Soil 324, 1–30. doi: 10.1007/s11104-009-0159-y
Tardieu, F., and Parent, B. (2017). Predictable ‘meta-mechanisms’ emerge from feedbacks between transpiration and plant growth and cannot be simply deduced from short-term mechanisms. Plant Cell Environ. 40, 846–857. doi: 10.1111/pce.12822
Tuzet, A., Perrier, A., and Leuning, R. (2003). A coupled model of stomatal conductance, photosynthesis and transpiration. Plant Cell Environ. 26, 1097–1116. doi: 10.1046/j.1365-3040.2003.01035.x
Villamayor, B. M. R., Rollon, R. N., Samson, M. S., Albano, G. M. G., and Primavera, J. H. (2016). Impact of Haiyan on Philippine mangroves: implications to the fate of the widespread monospecific Rhizophora plantations against strong typhoons. Ocean Coast. Manage. 132, 1–14. doi: 10.1016/j.ocecoaman.2016.07.011
Vörösmarty, C. J., Hoekstra, A. Y., Bunn, S. E., Conway, D., and Gupta, J. (2015). Fresh water goes global. Science 349, 478. doi: 10.1126/science.aac6009
Vose, J. M. (2019). Forest and Water in the 21st Century: A Global Perspective. Washington, DC: Oxford University Press.
Walker, L. R., Velázquez, E., and Shiels, A. B. (2009). Applying lessons from ecological succession to the restoration of landslides. Plant Soil 324, 157–168. doi: 10.1007/s11104-008-9864-1
Yumul, J. G. P., Servando, N. T., Suerte, L. O., Magarzo, M. Y., Juguan, L. V. V., and Dimalanta, C. B. (2012). Tropical cyclone–southwest monsoon interaction and the 2008 floods and landslides in Panay island, central Philippines: meteorological and geological factors. Nat. Hazards 62, 827–840. doi: 10.1007/s11069-012-0109-5
Keywords: deforestation, fast-growing trees, freshwater scarcity, hydraulic response to seasonal drought, limited leaf water supply, soil water content
Citation: Liu T, Jiang K, Tan Z, He Q, Zhang H and Wang C (2021) A Method for Performing Reforestation to Effectively Recover Soil Water Content in Extremely Degraded Tropical Rain Forests. Front. Ecol. Evol. 9:643994. doi: 10.3389/fevo.2021.643994
Received: 19 December 2020; Accepted: 11 January 2021;
Published: 10 February 2021.
Edited by:
Wenxing Long, Hainan University, ChinaReviewed by:
Tiantian Yang, University of Oklahoma, United StatesXiang Liu, Lanzhou University, China
Copyright © 2021 Liu, Jiang, Tan, He, Zhang and Wang. This is an open-access article distributed under the terms of the Creative Commons Attribution License (CC BY). The use, distribution or reproduction in other forums is permitted, provided the original author(s) and the copyright owner(s) are credited and that the original publication in this journal is cited, in accordance with accepted academic practice. No use, distribution or reproduction is permitted which does not comply with these terms.
*Correspondence: Hui Zhang, NDQ2MDU2OTYyQHFxLmNvbQ==; Chen Wang, Y2hlbi53YW5nQHNjYmcuYWMuY24=
†These authors have contributed equally to this work