- Department of Computer Science, Stanford University, Palo Alto, CA, United States
Cancer prevention is superior to cancer treatment—indeed, understanding and controlling cancer risk is a key question in the fields of applied ecology and evolutionary oncology. Ecological cancer risk models offer the dual benefit of being generalizable across cancer types, and unveiling common mechanisms underlying cancer development and spread. Understanding the biological mechanisms of cancer risk may also guide the design of interventions to prevent cancer. Ecological considerations are central to many of these mechanisms; as one example, the ecologically-based hypothesis of metabolic cancer suppression posits that restricted vascular supply of limiting resources to somatic tissues normally suppresses the evolution of somatic cells toward cancer. Here we present a critical review of published evidence relevant to this hypothesis, and we conclude that there is substantial evidence that cancer risk does increase with an abnormal excess of limiting cell resources, including both dietary macronutrients as well as certain micronutrients.
1. Introduction
Cancer is the result of an evolutionary process. This is a process fueled by mutation and shaped by ecology (Reynolds et al., 2020), making the assessment and reduction of cancer risk an important problem in the fields of applied ecology and evolution. Consequently, a evolutionary approach to cancer modeling can improve models for individualized risk assessment, which are currently in need of improvement (Louro et al., 2019). Moreover, forming a theoretical account of the ecological mechanisms of cancer risk can guide the design of evolutionarily-enlightened interventions to prevent cancer.
The difference between indolent and aggressive cancers may sometimes lie not within the tumor itself, but rather in the tissue micro-environment where the tumor is growing. The ecology of the tissue microenvironment is central to understanding and intercepting cancer risk in two ways: Firstly, while mutations are stochastic and unpredictable, ecological effects on evolutionary trajectories are deterministic and predictable (Lenski, 2017; Barrick et al., 2020)—especially in cases of convergent evolution, such as the cellular evolution of cancer (Fortunato et al., 2017). Secondly, unlike mutation, tissue ecology is a modifiable source of cancer risk; understanding it can help us to not only predict the risk of cancer, but also take proactive steps to reduce it.
In this review, we focus on resource supply and limitations in the tumor microenvironment. Based on systems biology simulation models, it was proposed that in the somatic ecology of tissues, an abnormal excess of limiting energetic resources may increase cancer risk in the affected tissues (Wu et al., 2019). Indeed, a consortium of scientists identified responses to energetic resource limitations as one of two primary ways to classify neoplasms (Maley et al., 2017). Here, we broaden the scope of this ecological idea to include not only energetic resources, but any resources that can be limiting for cell proliferation. We undertake a critical review of published empirical evidence relevant to testing this broader hypothesis.
2. Mechanism
A foundational principle of ecology is Liebig's Law, which states that growth is controlled not by the total amount of all resources available, but by the scarcest (limiting) resource type (Egli and Zinn, 2003; Shapiro et al., 2018). For a given population, a resource type is limiting if an increase in its availability increases growth. Both in classical species ecology, and in somatic cell ecology, there is only one limiting resource type per population, but this is may vary over time, or between tissues. The single resource that is likely to be limiting for most somatic cells at most times is energy for biosynthesis, growth, and division. This is, however, not a universal rule; other limiting resources may include micronutrients, growth signals, oxygen, and many others. The relation between cancer development and resource oversupply is an extension of Leibig's law; cancer cells are treated as organisms whose growth is unlocked by resource surplus.
Heretofore, in considering cancer risk, much attention has been focused on the driver mutations in somatic cells that are believed to trigger oncogenesis. However, it has become clear that such mutations often do not drive oncogenesis, but instead remain safely “parked” in normal tissues (Tomasetti, 2019; Nam et al., 2020; Solary and Laplane, 2020). These observations support the notion that cancer prevention can be viewed as an attempt to change the selective pressures within tissues to prevent or delay cancer (Maley et al., 2011). It is still unknown what selective pressures might direct driver mutations toward malignancy; this is a fundamental open question in the science of cancer prevention. One potential explanation is the hypothesis of metabolic cancer suppression, which is based on known epidemiology of cancer risk. According to this hypothesis, restricted vascular supply of resources to somatic tissues normally limits resources critical to cell proliferation, and thereby suppresses cellular evolution toward cancer, even in the presence of driver mutations (Figure 1). Under this framework, the importance of driver mutations is attributable only to their impact on cellular fitness in the context of the tumoral microenvironment. This accords with an earlier mathematical model (Beerenwinkel et al., 2007), which suggested that the waiting time to cancer depends strongly on the selective advantage (s) conferred by oncogenic driver mutations, with the average waiting time proportional to .
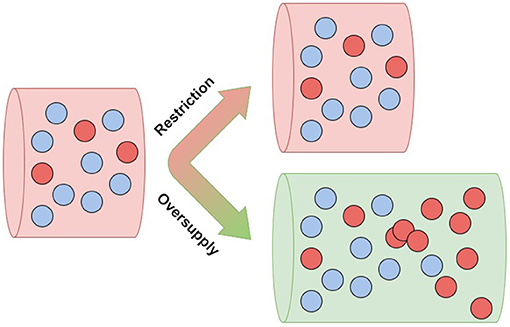
Figure 1. Resource oversupply unlocks clonal expansion—aggressive clones (red) flourish in an abundant environment (green).
In computer simulations, cancer driver mutations quickly caused cancer in microenvironments that were oversupplied with limiting cell resources, but had little effect in tissues with normally restricted supplies of those resources (Wu et al., 2019). These ecological effects on cancer risk are consistent with our general understanding of how ecology shapes evolution. Several authors have noted that both in classical species ecology and in the tissue ecology of somatic cells, it is only populations in resource-rich environments that typically evolve the rapid-proliferation life histories that rely on rapid resource consumption (Alfarouk et al., 2013; Ducasse et al., 2015). Therefore, according to the hypothesis of metabolic cancer suppression, even cell resources that are healthy and essential in normal quantities can become carcinogenic in excess.
The eco-evolutionary effects of resource availability are difficult to observe in healthy tissue that is evolving toward cancer, but have been observed in cancer itself. Cancer cells that could gain a fitness advantage by exploiting a given resource typically evolve to do so only when that resource is available in their local microenvironment. For example, in breast cancer, elevated expression of estrogen receptor can increase cell fitness and proliferation, but only in the presence of adequate estrogen, which is supplied through vascular delivery. Cell phenotyping has shown that within a breast tumor, there is a strong correlation between cells' access to vascular delivery, and with their evolved expression of estrogen receptor (Lloyd et al., 2014). Such local effects on the evolution of cancer cells, as described above, suggest that even when a limiting resource is at normal levels in blood, vascular abnormalities creating excess local blood flow might increase cancer risk in the locally affected tissues. There are published observations suggesting that local vascular abnormalities increasing blood flow do, in fact, increase localized cancer risk in humans (Feinmesser et al., 1997; Lapidoth et al., 2006; Blatt et al., 2019). However, such local tissue effects are unlikely to be generally important to cancer prevention and control. In contrast, systemic excess of resources that are often limiting for cell proliferation affect more tissues and organs, and do so in many more people. Such systemic excess is likely to be important to cancer prevention and control, and it will be the focus of this review.
3. Limiting Resources
Although there are few empirical studies investigating the effect of general limiting resources on cancer development, there is a rich body of literature investigating the carcinogenic effect of specific resources which are likely to be limiting (Table 1). We consider these studies here, not to offer a comprehensive consideration of all of the possible limiting resources within the tumor microenvironment, but instead to illustrate the myriad ways in which resource limitations may arise and be subverted.
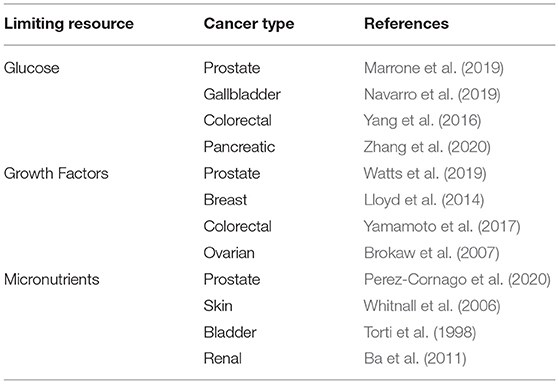
Table 1. Some examples of evidence supporting the importance of limiting resources in cancer control.
3.1. Glucose
The single class of resources that is likely to be limiting for most somatic cells at most times is energy; used for biosynthesis, growth, and division. This energy is supplied primarily by blood glucose, the supply of which is tightly regulated in normal physiology. In accordance with Liebig's law, researchers hypothesize that glucose oversupply may be associated with cancer risk.
In support of this hypothesis, it has been found that while hyperglycemia and diabetes were not significantly associated with total prostate cancer incidence, glycemia values above the normal range were associated with increased risk of lethal prostate cancer, and with prostate cancer mortality (Marrone et al., 2019). Similarly, a study of the aggressiveness of gallbladder cancer using circulating glucose-to-lymphocyte ratio (GLR) as an indicator of glucose availability, found that preoperative GLR was an independent predictor of survival (Navarro et al., 2019). A similar effect of blood glucose on cancer aggressiveness was reported for colorectal cancer (Yang et al., 2016), and also for pancreatic cancer (Zhang et al., 2020).
These consistent effects of glucose level on cancer risk are understandable through an eco-evolutionary perspective of carcinogenesis as the Darwinian evolution of somatic cells into cancer cells. Abnormally elevated glucose in the cell microenvironment alters the selective forces on cells in two ways: Firstly, excess glucose directly selects for cells with abnormally accelerated growth and proliferation that disproportionately benefit from this energetic windfall. Secondly, this hyperglycemic microenvironment allows neoplastic cells to gain a further fitness advantage over normal cells by generating energy through anaerobic glycolysis. While less efficient in glucose use than aerobic cellular respiration, this glycolytic pathway is an allelopathic strategy—it generates an acidic and toxic microenvironment that is more toxic to competing normal cells than to the cancer cells themselves (Gillies et al., 2008). In doing so, this destructive cell phenotype removes both competitive and physical barriers, thereby accelerating clonal expansion. These considerations, supported by empirical evidence and mathematical modeling, led some to conclude that elevated glucose consumption is a necessary cell phenotype for the formation of metastatic cancers (Gillies et al., 2008).
3.2. Inorganic Micronutrients
In addition to oxidizable energy substrates such as glucose, several inorganic trace nutrients, such as iron and phosphate, are also potentially limiting for cell proliferation, and are also over-consumed by rapidly dividing cells. According to Liebig's law, in those patients and tissues in which energy is the limiting resource, these trace nutrients are not limiting, thus their availability will not greatly affect cancer risk. However, in those patients with elevated blood glucose, cell proliferation is unlikely to be limited by energy availability, and so may be limited instead by crucial inorganic trace nutrients. In areas experiencing the current epidemic of obesity and metabolic syndrome, this situation may be common.
Iron is critical to cell proliferation because of its key roles in energy production and the biosynthesis of DNA and RNA. Like glucose, iron homeostasis is highly regulated, and its dysregulation has been associated with carcinogenesis (Weinberg, 1996; Torti and Torti, 2013). Both infections and neoplasms consist of rapidly dividing pathogenic cells, and host physiology defends against both infections and neoplasms by sequestering the iron required for rapid cell proliferation (Weinberg, 1984). Hosts respond to both infections and neoplasms by lowering their plasma iron levels; consequently, we expect that iron supplements enhance both microbial and neoplastic cell growth. Long-established animal experiments have demonstrated that experimental oversupply of iron by tissue injection can induce cancer at the injection site (Richmond, 1959). This is despite the fact the fact that physiological levels of iron are neither mutagenic nor carcinogenic (Weinberg, 1984). Substantial evidence from multiple studies suggests that abnormal iron excess is closely associated with tumorigenesis in multiple types of human cancer (Chen et al., 2019). In humans, excess tissue accumulation of iron due to food additives and iron supplements may be contributing to increased risk of cancer generally (Davoodi et al., 2016).
A second inorganic cell nutrient implicated in oncogenesis is phosphate. Evidence suggests that excess cellular phosphate, associated with dysregulated phosphate metabolism, acts as a growth-promoter in various human and experimental models of tumors (Brown and Razzaque, 2018). In a prospective study of multiple serum biomarkers potentially indicating risk of prostate cancer in a large cohort of men, phosphate was the only biomarker significantly positively correlated with prostate cancer during long term follow-up (Perez-Cornago et al., 2020). In an example of cancer niche construction, the phosphate proclivities of tumors are reflected in their tendency to accumulate more phosphorus in their microenvironment compared to normal tissue. In mouse models of spontaneous cancer, in vivo measurements of several aspects of the chemical tumor microenvironment revealed that the greatest difference of tumor tissue compared to normal tissue was not the well-established chemical differences in oxygen and pH, but rather, differences in extracellular concentration of inorganic phosphate (Bobko et al., 2017). Levels of inorganic phosphate (Pi) were more than two-fold higher in tumor microenvironments vs. normal tissue. Moreover, Pi concentration was the only parameter that allowed for discrimination between non-metastatic and highly metastatic tumors (Bobko et al., 2017).
3.3. Growth Factors
In addition to the nutrients discussed above, other candidates for resources limiting to cell proliferation include various endogenous signals. Similarly to nutrients, endogenous signaling molecules are received only through vascular delivery, and are often are taken up faster by hyperproliferating cells.
For example, insulin-like growth factors (IGFs) have been implicated in the etiology of several cancers. Epidemiological evidence implicates IGFs in risk for prostate, breast, colorectal cancer, and possibly thyroid cancer—IGF1 is consistently positively associated with an increased risk of these cancers (Watts et al., 2019). Estrogen is another endogenous signaling molecule that can be a limiting proliferation resource for some female reproductive cancers, in particular estrogen receptor positive (ER+) breast cancer. Within an ER+ tumor, estrogen dependence and uptake may vary from 1 to 100% of cells. It has been hypothesized that expression of estrogen receptors is positively selected only if estrogen is present in the microenvironment, and further, that tumor regions with higher blood flow would contain larger numbers of ER+ cells than areas of low blood flow (Lloyd et al., 2014). By examining the spatial distribution within tumors of ER+ and ER- cells relative to blood vessel area, the authors found a strong positive correlation between vascular area and ER expression. The authors concluded that ER expression, specifically by well-vascularized tumor cells, resulted from different selection pressures in well-vascularized regions. These results suggest that an abnormal excess of circulating estrogen could also lead to abnormal cell proliferation and to increased cancer risk.
That hypothesis has been supported by two unintended observational “experiments” on patterns of reproductive cycling in women. The first natural phenomenon involving abnormally increased estrogen exposure in women (relative to ancestral conditions) resulted from the introduction of agriculture and birth control. In the ancestral environment that humans are adapted to, women undergo relatively few ovulatory cycles with their accompanying surges of endogenous estrogen. In contrast, contemporary American women start cycling younger and cycle more often, resulting in about triple the lifetime ovulatory cycles as were typical of pre-agricultural women. Based on a theoretical model, this translates into a risk of breast cancer by age 60 that is about 100 times as high as that of pre-agricultural women, and this model prediction is consistent with the available epidemiological data (Eaton et al., 1994). The second phenomenon resulted from the introduction of hormone replacement therapy (HRT) to treat the symptoms of menopause. Multiple observational studies have showed an increased risk of breast cancer with multi-year use of HRT (Franceschini et al., 2020). These authors argue that this increased cancer risk has resulted not only from abnormally extended exposure to estrogen, but also from use of some older drugs with excessively high levels of estrogens. The combined weight of this evidence suggests that, as in the case of the nutrients discussed above, it may not be correct to consider estrogen to be carcinogenic per se, but rather to consider an abnormal excess of estrogen exposure to be carcinogenic.
4. Discussion
In conclusion, a large body of evidence indicates that cancer risk does increase with an abnormal excess of limiting cell resources, including both exogenous dietary factors, and endogenous signaling molecules. Among dietary factors, this effect is most widely investigated in energetic macronutrients, which can upset the normal energy balance and create hyperglycemia, but the supporting evidence is also strong for at least two limiting micronutrients: iron and phosphate. The idea that normal and necessary nutrients could sometimes have a carcinogenic effect may seem paradoxical, but it should not be too surprising—when pathology is plotted as a function of various physiological measurements or dietary inputs, the result is often a U-shaped response curve, with pathology increasing as physiology becomes abnormally extreme in either direction.
Ideally, a systematic review of an association between resource oversupply and cancer outcomes should compare the number of studies that reported a positive association vs. those that found a negative or no association (Pati and Lorusso, 2018). While we have, to our best knowledge, included an unbiased sample of all relevant literature, few empirical studies have been designed specifically to test the hypothesis we examine. Instead, much of the available evidence is observational, or was incidental to the focus of the reported study. We found no published negative reports regarding association between dietary excess and cancer outcomes, but this could reflect either a limited literature, or a reporting bias toward positive association. We are optimistic that if the hypothesis addressed here gains plausibility, there will soon be more empirical research directly targeted at investigating resource oversupply.
4.1. Exogenous and Endogenous Resources
It is difficult to disentangle the role of endogenous signals from that of exogenous resources, because levels of the two are often highly correlated. Limiting resources are closely regulated physiologically, so that extreme levels of intake will cause a rapid increase in regulatory signaling molecules.
However, there is a fundamental difference between the constraints on carcinogenesis resulting from limited signaling molecules compared to that of limited exogenous nutritional resources—the latter has a more fundamental and robust causal effect. Indeed, the evolution of independence from endogenous signals and factors that would normally limit proliferation is considered a canonical hallmark of cancer (Hanahan and Weinberg, 2000). Therefore, restricted levels of endogenous signals can only transiently impede the evolution of cancer. In contrast, conservation of energy dictates that cells cannot evolve away from dependence on energy and materials for growth and proliferation. Thus, restricted levels of exogenous limiting resources offer a more robust and important obstacle to somatic cell evolution toward cancer. As an example of this difference between the risks from exogenous resources vs. endogenous signals, normal somatic cells do not proliferate in the absence of insulin or of insulin-like growth factor 1 (IGF1). However, evolving cancer cells can evade this dependence, often through a single mutation. Tumors with this property have been described as resistant to caloric restriction, but in fact, animal experiments have only established their resistance to low serum levels of insulin and IGF1, and not to low levels of glucose itself (Kalaany and Sabatini, 2009). The problem of disentangling the effects of endogenous signals vs. exogenous resources is especially acute for glucose. Hyperglycemia, with its comorbidities metabolic syndrome and obesity, increases cancer risk not only through oversupply of glucose to tissues, but also through abnormal levels of IGF1, leptin, adiponectin, steroid hormones, insulin, and sirtuins (Hursting and Berger, 2010). As a mechanism of cancer risk, and as an opportunity for cancer prevention, serum glucose may prove to be the most important of these multiple intercorrelated risk factors.
4.2. Aggressive and Indolent Cancers
A key difficulty in cancer screening is the onerous diagnostic task of distinguishing between indolent cancers and clinically aggressive cases that demand immediate treatment (Srivastava et al., 2016). This problem is especially pressing for prostate cancer, which simultaneously proffers many cases of aggressive and dangerous disease, as well as many cases of over-diagnosed and over-treated tumors (Da Huang et al., 2020). Efforts at molecular characterization have not yet found mutations or other cell-intrinsic markers of cancer aggressiveness, and detailed consideration of tissue ecology may help. Indeed, it has been shown that the aggressiveness of prostate cancer is associated with circulating levels of several limiting cell resources, including glucose (Marrone et al., 2019), iron (Choi et al., 2008), and phosphate (Bobko et al., 2017). Given that resource oversupply may act as the “enabler” of carcinogenic mutations and clonal expansion, monitoring and analysis of the tumor microenvironment offers a promising method by which to identify clones which are likely to be aggressive.
4.3. Caloric Restriction and Dietary Regimens
Consistent with the hypothesis that excess blood glucose drives oncogenesis, a recent review concluded that the internal tissue environment determines whether cancers progress to advanced disease, and that glucose availability is an important component of this somatic ecology (Holly et al., 2019). Furthermore, a meta-analysis comparing people with and without diabetes found that diabetes was associated with substantial premature death from several types of cancer, independent of other major risk factors (Collaboration, 2011). These included cancers of the liver, pancreas, ovary, colorectum, lung, bladder, and breast. A prospective study also found blood glucose to predict risk of breast cancer (Muti et al., 2002). This work suggests that controlling blood glucose levels by managing caloric intake and increasing physical activity is likely to confer protective benefits for many types of cancer in economically developed areas (Giovannucci, 1999). Indeed, caloric restriction dietary regimens, which restrict energy intake to minimal survival levels, have strong cancer preventive effects in animal models. Observational data reflect a similar effect in humans. Across species, calorie restriction appears to be the most potent, broadly acting dietary regimen for suppressing carcinogenesis (Hursting et al., 2010).
4.4. Aging
It is not immediately clear why cancer incidence increases sharply with age, given the evidence (reviewed in section 1), that while cancer driver mutations do accumulate with age, they are not enough alone to initiate cancer. An ecological factor that may explain this trend is that hyperglycemia increases with age, increasing positive selection for mutated neoplastic cells that can use excess glucose for rapid proliferation (Golubev and Anisimov, 2019). Similarly, age-related metabolic shifts which remove resource restrictions have been linked to epithelial cancers (Holly et al., 2013). In general, the process of aging appears to loosen the tightly orchestrated biological controls on tissue nutrition and resource supply, thereby creating intermittent excess.
4.5. Micronutrients
Oversupply of limiting cell resources does appear to be an important biological mechanism of cancer risk. This is well-established for energetic macronutrients—the case for micronutrients is less well-established, but also holds potential. The highly-processed foods that now constitute the majority of calories consumed in developed nations not only cause excessive energy intake and tend to increase average daily blood glucose levels (Hall et al., 2019), but also contain additives that can create excessive intake of the limiting micronutrients iron and phosphate. Of all the micronutrients, phosphate may be the most promising candidate for managing cancer risk. Dietary phosphorus deficiency is uncommon and usually observed only in rare inherited disorders, or near-total starvation. There would be little health risk in reducing dietary intake, especially by avoiding the extra phosphate that is an additive in many processed foods and beverages (Erem and Razzaque, 2018). Excess phosphate is implicated in risk of both lung cancer (Jin et al., 2009), and prostate cancer (Perez-Cornago et al., 2020), and high dietary phosphorus intake is associated with all-cause mortality (Chang et al., 2014).
4.6. Prevention and Intervention
An excess of limiting cell resources offers excellent opportunities for management of individual cancer risk, because in many cases it may be measured non-invasively. For example, inexpensive wearable monitors of serum glucose are widely available. Similarly, measurement of urinary phosphate is routinely used to manage kidney disease, and standard test kits might be repurposed for managing cancer risk. In addition to addressing cancer risk, evaluating tissue ecology may also be helpful in the difficult diagnostic task of distinguishing between indolent cancers and clinically aggressive cases that demand immediate treatment (Srivastava et al., 2016). As this association between excess resources and increased cancer risk appears to be robust across cancer subtypes and stages, we expect that ecologically-informed risk management will be an important element of cancer prevention research, and ultimately, of better patient outcomes.
Author Contributions
The author confirms being the sole contributor of this work and has approved it for publication.
Conflict of Interest
The author declares that the research was conducted in the absence of any commercial or financial relationships that could be construed as a potential conflict of interest.
Acknowledgments
The author gives special thanks to John W. Pepper, whose ideas and feedback greatly contributed to this work.
References
Alfarouk, K. O., Ibrahim, M. E., Gatenby, R. A., and Brown, J. S. (2013). Riparian ecosystems in human cancers. Evol. Appl. 6, 46–53. doi: 10.1111/eva.12015
Ba, Q., Hao, M., Huang, H., Hou, J., Ge, S., Zhang, Z., et al. (2011). Iron deprivation suppresses hepatocellular carcinoma growth in experimental studies. Clin. Cancer Res. 17, 7625–7633. doi: 10.1158/1078-0432.CCR-10-3099
Barrick, J. E., Deatherage, D. E., and Lenski, R. E. (2020). “A test of the repeatability of measurements of relative fitness in the long-term evolution experiment with Escherichia coli,” in Evolution in Action: Past, Present and Future (Springer), 77–89. doi: 10.1007/978-3-030-39831-6_8. Available online at: https://link.springer.com/chapter/10.1007%2F978-3-030-39831-6_8
Beerenwinkel, N., Antal, T., Dingli, D., Traulsen, A., Kinzler, K. W., Velculescu, V. E., et al. (2007). Genetic progression and the waiting time to cancer. PLoS Comput. Biol. 3:e225. doi: 10.1371/journal.pcbi.0030225
Blatt, J., Finger, M., Price, V., Crary, S. E., Pandya, A., and Adams, D. M. (2019). Cancer risk in klippel-trenaunay syndrome. Lymphat. Res. Biol. 17, 630–636. doi: 10.1089/lrb.2018.0049
Bobko, A. A., Eubank, T. D., Driesschaert, B., Dhimitruka, I., Evans, J., Mohammad, R., et al. (2017). Interstitial inorganic phosphate as a tumor microenvironment marker for tumor progression. Sci. Rep. 7:41233. doi: 10.1038/srep41233
Brokaw, J., Katsaros, D., Wiley, A., Lu, L., Su, D., Sochirca, O., et al. (2007). IGF-I in epithelial ovarian cancer and its role in disease progression. Growth Fact. 25, 346–354. doi: 10.1080/08977190701838402
Brown, R. B., and Razzaque, M. S. (2018). Phosphate toxicity and tumorigenesis. Biochim. Biophys. Acta Rev. Cancer 1869, 303–309. doi: 10.1016/j.bbcan.2018.04.007
Chang, A. R., Lazo, M., Appel, L. J., Gutierrez, O. M., and Grams, M. E. (2014). High dietary phosphorus intake is associated with all-cause mortality: results from Nhanes III. Am. J. Clin. Nutr. 99, 320–327. doi: 10.3945/ajcn.113.073148
Chen, Y., Fan, Z., Yang, Y., and Gu, C. (2019). Iron metabolism and its contribution to cancer. Int. J. Oncol. 54, 1143–1154. doi: 10.3892/ijo.2019.4720
Choi, J.-Y., Neuhouser, M. L., Barnett, M. J., Hong, C.-C., Kristal, A. R., Thornquist, M. D., et al. (2008). Iron intake, oxidative stress-related genes (MnSOD and MPO) and prostate cancer risk in caret cohort. Carcinogenesis 29, 964–970. doi: 10.1093/carcin/bgn056
Collaboration, E. R. F. (2011). Diabetes mellitus, fasting glucose, and risk of cause-specific death. N. Engl. J. Med. 364, 829–841. doi: 10.1056/NEJMoa1008862
Da Huang, Y.-S. W., Ye, D.-W., Qi, J., Liu, F., Helfand, B. T., Zheng, S. L., et al. (2020). Prostate volume does not provide additional predictive value to prostate health index for prostate cancer or clinically significant prostate cancer: results from a multicenter study in china. Asian J. Androl. 22:539. doi: 10.4103/aja.aja_136_19
Davoodi, S. H., Jamshidi-Naeini, Y., Esmaeili, S., Sohrabvandi, S., and Mortazavian, A. M. (2016). The dual nature of iron in relation to cancer: a review. Iran. J. Cancer Prevent. 9:e5494. doi: 10.17795/ijcp-5494
Ducasse, H., Arnal, A., Vittecoq, M., Daoust, S. P., Ujvari, B., Jacqueline, C., et al. (2015). Cancer: An emergent property of disturbed resource-rich environments? Ecology meets personalized medicine. Evol. Appl. 8, 527–540. doi: 10.1111/eva.12232
Eaton, S. B., Pike, M. C., Short, R. V., Lee, N. C., Trussell, J., Hatcher, R. A., et al. (1994). Women's reproductive cancers in evolutionary context. Q. Rev. Biol. 69, 353–367. doi: 10.1086/418650
Egli, T., and Zinn, M. (2003). The concept of multiple-nutrient-limited growth of microorganisms and its application in biotechnological processes. Biotechnol. Adv. 22, 35–43. doi: 10.1016/j.biotechadv.2003.08.006
Erem, S., and Razzaque, M. S. (2018). Dietary phosphate toxicity: an emerging global health concern. Histochem. Cell Biol. 150, 711–719. doi: 10.1007/s00418-018-1711-8
Feinmesser, M., Taube, E., Badani, E., and Kristt, D. (1997). Basal cell carcinomas arising over arteriovenous malformations: some speculations on the theme. Am. J. Dermatopathol. 19, 575–579. doi: 10.1097/00000372-199712000-00004
Fortunato, A., Boddy, A., Mallo, D., Aktipis, A., Maley, C. C., and Pepper, J. W. (2017). Natural selection in cancer biology: from molecular snowflakes to trait hallmarks. Cold Spring Harb. Perspect. Med. 7:a029652. doi: 10.1101/cshperspect.a029652
Franceschini, G., Lello, S., and Masetti, R. (2020). Hormone replacement therapy: revisiting the risk of breast cancer. Eur. J. Cancer Prevent. 29, 303–305. doi: 10.1097/CEJ.0000000000000548
Gillies, R. J., Robey, I., and Gatenby, R. A. (2008). Causes and consequences of increased glucose metabolism of cancers. J. Nucl. Med. 49:24S. doi: 10.2967/jnumed.107.047258
Giovannucci, E. (1999). “Nutritional factors in human cancers,” in Advances in Nutrition and Cancer 2 (Boston, MA: Springer), 29–42. doi: 10.1007/978-1-4757-3230-6_3. Available online at: https://link.springer.com/chapter/10.1007%2F978-1-4757-3230-6_3
Golubev, A. G., and Anisimov, V. N. (2019). Aging and cancer: is glucose a mediator between them? Oncotarget 10:6758. doi: 10.18632/oncotarget.27344
Hall, K. D., Ayuketah, A., Brychta, R., Cai, H., Cassimatis, T., Chen, K. Y., et al. (2019). Ultra-processed diets cause excess calorie intake and weight gain: an inpatient randomized controlled trial of ad libitum food intake. Cell Metab. 30, 67–77. doi: 10.1016/j.cmet.2019.05.008
Hanahan, D., and Weinberg, R. A. (2000). The hallmarks of cancer. Cell 100, 57–70. doi: 10.1016/S0092-8674(00)81683-9
Holly, J. M., Biernacka, K., and Perks, C. M. (2019). Systemic metabolism, its regulators, and cancer: past mistakes and future potential. Front. Endocrinol. 10:65. doi: 10.3389/fendo.2019.00065
Holly, J. M., Zeng, L., and Perks, C. M. (2013). Epithelial cancers in the post-genomic era: should we reconsider our lifestyle? Cancer Metast. Rev. 32, 673–705. doi: 10.1007/s10555-013-9445-5
Hursting, S. D., and Berger, N. A. (2010). Energy balance, host-related factors, and cancer progression. J. Clin. Oncol. 28:4058. doi: 10.1200/JCO.2010.27.9935
Hursting, S. D., Smith, S. M., Lashinger, L. M., Harvey, A. E., and Perkins, S. N. (2010). Calories and carcinogenesis: lessons learned from 30 years of calorie restriction research. Carcinogenesis 31, 83–89. doi: 10.1093/carcin/bgp280
Jin, H., Xu, C.-X., Lim, H.-T., Park, S.-J., Shin, J.-Y., Chung, Y.-S., et al. (2009). High dietary inorganic phosphate increases lung tumorigenesis and alters AKT signaling. Am. J. Respir. Crit. Care Med. 179, 59–68. doi: 10.1164/rccm.200802-306OC
Kalaany, N. Y., and Sabatini, D. M. (2009). Tumours with pi3k activation are resistant to dietary restriction. Nature 458, 725–731. doi: 10.1038/nature07782
Lapidoth, M., Ad-El, D., David, M., Alcalay, J., and Azaria, R. (2006). Basal cell carcinoma arising over facial port wine stain: a single-centre experience. J. Eur. Acad. Dermatol. Venereol. 20, 1066–1069. doi: 10.1111/j.1468-3083.2006.01701.x
Lenski, R. E. (2017). Convergence and divergence in a long-term experiment with bacteria. Am. Nat. 190, S57–S68. doi: 10.1086/691209
Lloyd, M. C., Alfarouk, K. O., Verduzco, D., Bui, M. M., Gillies, R. J., Ibrahim, M. E., et al. (2014). Vascular measurements correlate with estrogen receptor status. BMC Cancer 14:279. doi: 10.1186/1471-2407-14-279
Louro, J., Posso, M., Boon, M. H., Román, M., Domingo, L., Castells, X., et al. (2019). A systematic review and quality assessment of individualised breast cancer risk prediction models. Br. J. Cancer 121, 76–85. doi: 10.1038/s41416-019-0476-8
Maley, C. C., Aktipis, A., Graham, T. A., Sottoriva, A., Boddy, A. M., Janiszewska, M., et al. (2017). Classifying the evolutionary and ecological features of neoplasms. Nat. Rev. Cancer 17, 605–619. doi: 10.1038/nrc.2017.69
Maley, C. C., Szabo, E., and Reid, B. J. (2011). “Somatic evolution in neoplastic progression and cancer prevention,” in Pre-Invasive Disease: Pathogenesis and Clinical Management (Springer), 111–127. doi: 10.1007/978-1-4419-6694-0_7
Marrone, M. T., Selvin, E., Barber, J. R., Platz, E. A., and Joshu, C. E. (2019). Hyperglycemia, classified with multiple biomarkers simultaneously in men without diabetes, and risk of fatal prostate cancer. Cancer Prevent. Res. 12, 103–112. doi: 10.1158/1940-6207.CAPR-18-0216
Muti, P., Quattrin, T., Grant, B. J., Krogh, V., Micheli, A., Schünemann, H. J., et al. (2002). Fasting glucose is a risk factor for breast cancer: a prospective study. Cancer Epidemiol. Prevent. Biomark. 11, 1361–1368.
Nam, A. S., Chaligne, R., and Landau, D. A. (2020). Integrating genetic and non-genetic determinants of cancer evolution by single-cell multi-omics. Nat. Rev. Genet. 22, 3–18. doi: 10.1038/s41576-020-0265-5
Navarro, J., Kang, I., Hwang, H. K., Yoon, D. S., Lee, W. J., and Kang, C. M. (2019). Glucose to lymphocyte ratio as a prognostic marker in patients with resected PT2 gallbladder cancer. J. Surg. Res. 240, 17–29. doi: 10.1016/j.jss.2019.02.043
Pati, D., and Lorusso, L. N. (2018). How to write a systematic review of the literature. Health Environ. Res. Des. J. 11, 15–30. doi: 10.1177/1937586717747384
Perez-Cornago, A., Fensom, G. K., Andrews, C., Watts, E. L., Allen, N. E., Martin, R. M., et al. (2020). Examination of potential novel biochemical factors in relation to prostate cancer incidence and mortality in UK biobank. Br. J. Cancer 123, 1808–1817. doi: 10.1038/s41416-020-01081-3
Reynolds, B. A., Oli, M. W., and Oli, M. K. (2020). Eco-oncology: applying ecological principles to understand and manage cancer. Ecol. Evol. 10, 8538–8553. doi: 10.1002/ece3.6590
Richmond, H. (1959). Induction of sarcoma in the rat by iron-dextran complex. Br. Med. J. 1:947. doi: 10.1136/bmj.1.5127.947
Shapiro, B., Hoehler, T. M., and Jin, Q. (2018). Integrating genome-scale metabolic models into the prediction of microbial kinetics in natural environments. Geochim. Cosmochim. Acta 242, 102–122. doi: 10.1016/j.gca.2018.08.047
Solary, E., and Laplane, L. (2020). The role of host environment in cancer evolution. Evol. Appl. 13, 1756–1770. doi: 10.1111/eva.13039
Srivastava, S., Reid, B. J., Ghosh, S., and Kramer, B. S. (2016). Research needs for understanding the biology of overdiagnosis in cancer screening. J. Cell. Physiol. 231, 1870–1875. doi: 10.1002/jcp.25227
Tomasetti, C. (2019). Mutated clones are the new normal. Science 364, 938–939. doi: 10.1126/science.aax5525
Torti, S., Torti, F., Whitman, S., Brechbiel, M., Park, G., and Planalp, R. (1998). Tumor cell cytotoxicity of a novel metal chelator. Blood 92, 1384–1389. doi: 10.1182/blood.V92.4.1384.416k30_1384_1389
Torti, S. V., and Torti, F. M. (2013). Iron and cancer: more ore to be mined. Nat. Rev. Cancer 13, 342–355. doi: 10.1038/nrc3495
Watts, E. L., Perez-Cornago, A., Appleby, P. N., Albanes, D., Ardanaz, E., Black, A., et al. (2019). The associations of anthropometric, behavioural and sociodemographic factors with circulating concentrations of IGF-I, IGF-II, IGFBP-1, IGFBP-2 and IGFBP-3 in a pooled analysis of 16,024 men from 22 studies. Int. J. Cancer 145, 3244–3256. doi: 10.1002/ijc.32276
Weinberg, E. D. (1984). Iron withholding: a defense against infection and neoplasia. Physiol. Rev. 64, 65–102. doi: 10.1152/physrev.1984.64.1.65
Whitnall, M., Howard, J., Ponka, P., and Richardson, D. R. (2006). A class of iron chelators with a wide spectrum of potent antitumor activity that overcomes resistance to chemotherapeutics. Proc. Natl. Acad. Sci. U.S.A. 103, 14901–14906. doi: 10.1073/pnas.0604979103
Wu, D. J., Aktipis, A., and Pepper, J. W. (2019). Energy oversupply to tissues: a single mechanism possibly underlying multiple cancer risk factors. Evol. Med. Publ. health 2019, 9–16. doi: 10.1093/emph/eoz004
Yamamoto, N., Oshima, T., Yoshihara, K., Aoyama, T., Hayashi, T., Yamada, T., et al. (2017). Clinicopathological significance and impact on outcomes of the gene expression levels of IGF-1, IGF-2 and IGF-1R, IGFBP-3 in patients with colorectal cancer: overexpression of the IGFBP-3 gene is an effective predictor of outcomes in patients with colorectal cancer. Oncol. Lett. 13, 3958–3966. doi: 10.3892/ol.2017.5936
Yang, I.-P., Tsai, H.-L., Huang, C.-W., Lu, C.-Y., Miao, Z.-F., Chang, S.-F., et al. (2016). High blood sugar levels significantly impact the prognosis of colorectal cancer patients through down-regulation of microRNA-16 by targeting MYB and VEGFR2. Oncotarget 7:18837. doi: 10.18632/oncotarget.7719
Keywords: evolutionary oncology, cancer ecology, cancer prevention, resource oversupply, limiting resources
Citation: Wu DJ (2021) Oversupply of Limiting Cell Resources and the Evolution of Cancer Cells: A Review. Front. Ecol. Evol. 9:653622. doi: 10.3389/fevo.2021.653622
Received: 14 January 2021; Accepted: 08 April 2021;
Published: 05 May 2021.
Edited by:
Frederick R. Adler, The University of Utah, United StatesReviewed by:
Jeff M. P. Holly, University of Bristol, United KingdomFrancisco Garcia-Gonzalez, Estación Biológica de Dońana, Spain
Copyright © 2021 Wu. This is an open-access article distributed under the terms of the Creative Commons Attribution License (CC BY). The use, distribution or reproduction in other forums is permitted, provided the original author(s) and the copyright owner(s) are credited and that the original publication in this journal is cited, in accordance with accepted academic practice. No use, distribution or reproduction is permitted which does not comply with these terms.
*Correspondence: Daniel J. Wu, ZGFuand1QGNzLnN0YW5mb3JkLmVkdQ==