- Invasive Species and Pollinator Health Research Unit, USDA-ARS, Davis, CA, United States
As social insects, honey bees (Apis mellifera) rely on the coordinated performance of various behaviors to ensure that the needs of the colony are met. One of the most critical of these behaviors is the feeding and care of egg laying honey bee queens by non-fecund female worker attendants. These behaviors are crucial to honey bee reproduction and are known to be elicited by the queen’s pheromone blend. The degree to which workers respond to this blend can vary depending on their physiological status, but little is known regarding the impacts of developmental exposure to agrochemicals on this behavior. This work investigated how exposing workers during larval development to chronic sublethal doses of insect growth disruptors affected their development time, weight, longevity, and queen pheromone responsiveness as adult worker honey bees. Exposure to the juvenile hormone analog pyriproxyfen consistently shortened the duration of pupation, and pyriproxyfen and diflubenzuron inconsistently reduced the survivorship of adult bees. Finally, pyriproxyfen and methoxyfenozide treated bees were found to be less responsive to queen pheromone relative to other treatment groups. Here, we describe these results and discuss their possible physiological underpinnings as well as their potential impacts on honey bee reproduction and colony performance.
Introduction
Managed honey bees (Apis mellifera) are crucial agricultural pollinators that improve food security for growing global populations (Southwick and Southwick, 1992; Aizen et al., 2008; Calderone, 2012), and the transportation of large numbers of pollinators into agricultural fields is simplified by the social nature of honey bees, where thousands of worker bees live together in a self-contained unit (Winston, 1987). This communal living arrangement relies upon a complex social structure wherein tasks such as reproduction, rearing offspring, and gathering food are delegated by caste and by age (Hölldobler and Wilson, 2009). Like most social insects, honey bee workers exhibit temporal polyethism, or age related division of labor (Johnson, 2010). In a productive colony, older workers leave the hive to forage, while younger workers remain in the hive and perform nursing and housekeeping tasks (Seeley, 1982). Of the many tasks typically performed by younger workers, one of the most critical is tending to the queen (Allen, 1960).
Inside a functional colony, young worker bees feed and care for a single egg-laying honey bee queen, enabling her to produce fertilized eggs that develop into the next generation of workers (Oster and Wilson, 1978). The behavior of worker bees attending to the queen, commonly referred to as queen retinue behavior, is reliant on the responsiveness of workers to the queen’s nine-component pheromone blend (Robinson, 1985; Slessor et al., 1988; Kaminski et al., 1990; Keeling et al., 2003). Attraction to queen pheromone (QP) can be influenced by numerous parameters including the worker’s physical health (Walton et al., 2018), queen health and physiology (Kocher et al., 2009; Rangel et al., 2016; Walsh et al., 2020), worker reproductive potential (Galbraith et al., 2015), and worker age (Allen, 1960; Pham-Delègue et al., 1993). Workers that are less responsive to QP may perform other tasks such as foraging (Pham-Delègue et al., 1993). These divisions of labor create a strong codependence between hive members, and the continued functioning of a colony is reliant on the balanced performance of these behaviors (Oster and Wilson, 1978). However, various stressors can shift the optimal balance of these divisions, resulting in a disruption to the normal processes required to sustain colony activities like reproduction (Perry et al., 2015; Booton et al., 2017; Bordier et al., 2017), which may eventually lead to colony loss.
Since 2007, beekeepers have been reporting high annual colony losses (vanEngelsdorp et al., 2007, 2008; Kulhanek et al., 2017) caused primarily by stress due to parasitic infestations (Neumann and Carreck, 2010; Steinhauer et al., 2021), pathogenic infections (Berthoud et al., 2010), poor nutrition (Leonhardt and Blüthgen, 2012; Donkersley et al., 2014), and exposure to pesticides (Sanchez-Bayo and Goka, 2014; Dively et al., 2015). These ubiquitous and interacting stressors have been shown to negatively affect various aspects of honey bee health including larval and pupal development (Wu et al., 2011; Chen et al., 2016), longevity (Wu et al., 2011; DeGrandi-Hoffman and Chen, 2015), immune function (Nazzi and Pennacchio, 2018; Harwood and Dolezal, 2020), and memory (van Dame et al., 1995; Siviter et al., 2018). Perhaps one of the more troubling and cryptic effects of these stressors relate to disruptions in the performance of important honey bee social behaviors and alterations to the expected pattern of temporal polyethism (Tasei, 2001; Thompson et al., 2007; Fine and Corby-Harris, 2021).
Changes in honey bee worker behavior and colony dynamics, which can be difficult to diagnose in field colonies (Henry et al., 2015), are known to occur in response to both abiotic and biotic stressors such as parasites (Downey et al., 2000), pathogens (Goblirsch et al., 2013; Natsopoulou et al., 2016), pesticides (Robinson, 1985; Tasei, 2001; Thompson et al., 2007), and poor nutrition (Free, 1961; Schulz et al., 1998; Mattila and Otis, 2006). Larval nutrition, which directly affects caste determination (Haydak, 1970; Leimar et al., 2012; Slater et al., 2020), and worker responsiveness to QP (Walton et al., 2018) is of particular importance in regulating temporal polyethism, and agrochemical exposure is well known to affect honey bee behavior (Robinson, 1985; Ciarlo et al., 2012; Liao et al., 2017; Colin et al., 2019). As managed pollinators, honey bees frequently encounter agrochemicals when they are applied in their foraging range or administered directly into hives to control parasite infestations (Mullin et al., 2010; Traynor et al., 2016, 2021a). Inside the colony, honey bee larvae may be exposed to agrochemical residues through the wax of their cell (Mullin et al., 2010) and potentially through diet, though the extent to which the latter occurs is debated (Böhme et al., 2018). Therefore, it is important to investigate and understand the potential effects of developmental exposure to agrochemicals on adult bees.
One of the most ubiquitous classes of agrochemicals honey bees can encounter are insect growth disruptors (IGDs) (Traynor et al., 2016; Fine and Corby-Harris, 2021), which are commonly applied in blooming almond orchards (CalDPR, 2019; Wade et al., 2019). IGDs are agrochemical pesticides that target pathways associated with insect growth and development (Pener and Dhadialla, 2012), though they are also known to impact numerous aspects of adult insect behavior including oviposition (Smagghe and Degheele, 1994; Hamaidia and Soltani, 2021), mating (Thompson et al., 2005), memory and learning (Abramson et al., 2004), and behavioral maturation (Jaycox et al., 1974; Robinson, 1985). Because IGDs exhibit low lethality to adult honey bees (Tasei, 2001), they are often applied to blooming crops where bees forage, such as almonds (Mullin et al., 2016; Wade et al., 2019; CalPIP Home - California Pesticide Information Portal). Foragers that encounter IGDs and other pesticides can return to the colony with contaminated resources (Mullin et al., 2010; Traynor et al., 2016, 2021a), resulting in a systemic exposure scenario in which all colony members including the queen and developing larvae may be at risk (Traynor et al., 2021a,b). Because of the known effects of IGDs on insect development, much consideration is given to the lethal effects of IGDs on immature pollinators (Tasei, 2001; Chen et al., 2016; Milchreit et al., 2016), and growers may attempt to select IGDs that exhibit less toxicity to honey bee brood when making an application while bees are foraging. There has been significant progress made in understanding how IGD exposure affects adult worker health and behavior (Robinson, 1985; Abramson et al., 2004; Fisher et al., 2018), however, the sublethal effects of developmental exposure to IGDs are seldom explored. Given the importance of the pathways targeted by IGDs, it is possible that adult bees exposed during development to IGDs may exhibit altered behavioral patterns as adults (Fourrier et al., 2015).
In this work, we explored the effects of sublethal doses of IGDs during honey bee development as described by Moriarty (1969). Metrics examined included the survival of immature bees, the longevity of adult bees, and their queen pheromone responsiveness. We focus on three IGDs known to be applied in flowering almond orchards while bees are foraging: the juvenile hormone (JH) analog pyriproxyfen, the ecdysone agonist methoxyfenozide, and the chitin synthesis inhibitor diflubenzuron (CalPIP Home - California Pesticide Information Portal). Here, we examine how larval exposure to these chemicals affects adult bee responsiveness to queen pheromone and discuss implications for the continued health and performance of an affected colony.
Materials and Methods
Honey Bees
First instar honey bee larvae were obtained from Apis mellifera colonies maintained according to standard commercial methods at the Harry H. Laidlaw Jr. Honey Bee Research Facility at the University of California at Davis. To best account for differences in susceptibility to IGDs due to genetic background (Crailsheim et al., 2013), three colonies were used per experimental replicate with 1 colony replaced between replicates 1 and 2 due to an accidental queen death. In total, 4 colonies, herein referred to as Colonies 1–4, with queens of Caucasian descent were used. All colonies were apparently healthy with no obvious evidence of disease visible upon inspection.
Larval Rearing
Larvae were reared according to an established protocol (Schmehl et al., 2016) following first instar eclosion and fed an artificial, royal jelly based diet. Briefly, queens from 3 colonies were caged for 24 h for each experimental replicate, and first instar larvae were grafted into queen cups (day 0) set into 48 well plates approximately 72–84 h later. Subsequently, larvae were housed inside a modified desiccator within a HerathermTM incubator (Thermo Fisher Scientific, Waltham, MA, United States) maintained at 34.5°C. Relative humidity inside the desiccator was maintained at 95% using a saturated aqueous solution of potassium sulfate. Larvae were reared on artificial diet composed of royal jelly, sugars, yeast extract, and water at different concentrations depending on larval age for 5 days after grafting and transferred to new 48 well plates lined in Kim wipes prior to pupation only after all treatment diet had been consumed. While pupating, larvae were maintained in a separate desiccator within the same incubator with relative humidity maintained at 75% using a saturated aqueous solution of sodium chloride. Because contact with the meconium is suspected to cause mortality in developing larvae (Crailsheim et al., 2013), the larvae were removed from the experiment if they were observed to have defecated prior to the transfer.
For each colony, 144 larvae were grafted onto four 48 well plates. Each of the 4 plates were randomly assigned to receive 1 of 4 dietary treatments on days 2–5 of larval development for a total of 3 plates per treatment for each biological replicate. The experiment was repeated twice (Replicates 1 and 2) during the month of June. In the second replicate, a single colony used in Replicate 1 was replaced with a new colony due to an accidental queen death.
Diet Preparation and IGD Exposure
All treatments were administered through 140 μL royal jelly based diet at chronic dosages scaled to generate sublethal effects. Methoxyfenozide was administered at 16.1 μg/mL of diet (Wade et al., 2019), diflubenzuron at 0.1674 μg/mL (Dai et al., 2018), and pyriproxyfen at 0.164 μg/mL (Fourrier et al., 2015). To prepare the diet, stock solutions were prepared by dissolving IGDs in a 1:1 acetone/methanol solution to accommodate solubility differences between diflubenzuron and methoxyfenozide and stored at −20°C when not in use. See Schmehl et al. (2016) for the recommended volumes of diet used per day. For each treatment, 0.05 mL of stock solution was added to 9.95 mL of diet and vortexed for 30 s for a final solvent concentration of 0.5%. An equivalent volume of pure solvent was added to the control diet. All dietary treatments were prepared fresh daily and warmed in an incubator set to 34.5°C for 30 min prior to their administration. The treatment period began on the second day after grafting and concluded after the final day of feeding, prior to the transfer of larvae to the pupation plates.
Mortality and Eclosion Monitoring
On day 2, prior to the treatment period, larvae that did not survive the grafting procedure were removed from the experiment. From this point until transfer to pupation plates, larval mortality was monitored daily. Dead larvae were identified by black coloration, a deflated appearance, or lack of spiracle movement (Crailsheim et al., 2013). Mortality was recorded and dead larvae were removed.
Because of previously observed mortality caused by excessive handling during pupation (Fine, unpublished), larvae were left undisturbed after transfer to pupation plates until day 9, when pupation status and survivorship were noted for each larva. Thereafter, immature bees were monitored daily for pupation, and mortality checks resumed. Dead pupae were identified by black or brown coloration or obvious lack of development. Upon discovery, failed pupations, dead pupae, and dead pre-pupa were noted and removed from their wells, which were cleaned with 10% bleach solution on a cotton swab.
Date of pupation was recorded for each bee, and beginning on day 15, each plate of pupae was checked twice daily for adults.
Adult Care
Pharate Bee Caging
Eclosing adults were weighed to the nearest tenth of a milligram using a Mettler Toledo ML104T scale (Columbus, OH, United States) and transferred to modified cup cages (Evans et al., 2009) assembled from 16oz clear plastic cups glued onto egg laying plates (ELP), keeping bees from different colonies and treatments separated. ELPs, which are described by Fine et al. (2018), consist of artificial plastic comb designed for the collection of fertilized eggs from a mated honey bee queen. In a colony, pharate adults do not exit their cells until their cuticle has sufficiently hardened (Elias-Neto et al., 2009). Bees reared using standard in vitro methods typically eclose in 48 or 24 well plates (Crailsheim et al., 2013; Schmehl et al., 2016), and in this work, they were transferred to cup cages immediately after they were observed to have eclosed as pharate adults. This novel in vitro handling practice presents pharate bees with an opportunity to move into ELP cells and complete their sclerotization process in a more natural, physically protected place.
Each cage had a 2 cm diameter hole covered with mesh on the side of the cup for ventilation and another 1 cm hole on the top through which eclosing bees and diet were added. While in use, the top hole was covered with a piece of laboratory tape. Prior to use, 2 mL of 30% (w/w) aqueous sucrose solution and 1 mL deionized water were added to the cells of the ELP and diet was replenished daily. Because an adult bee’s behavioral development and responsiveness to queen pheromone is influenced by early exposure to queen pheromone (Robinson et al., 1998; Hoover et al., 2003; Grozinger and Robinson, 2007; Vergoz et al., 2009), we added 1 queen equivalent (Qeq) of artificial 9 component QP blend, administered as 1/10th of a TempQueen (INTKO Supply, Vancouver, Canada) lure fixed to a safety pin, to all cup cages.
Pharate Bee Microbial Inoculation
Newly eclosed adult bees in a colony acquire their gut microbiome from natural comb and interactions with nurse bees, but in vitro reared bees do not have an opportunity to be exposed to the microbial communities that typically inhabit their digestive tract (Zheng et al., 2018). In this work, newly eclosed, in vitro reared bees were given a microbial inoculum prepared from the digestive tracts of nurse bees as described by Powell et al. (2014) while in cup cages. Nurse bees were collected from the callow bees’ hives of origin and anesthetized by chilling at 4°C in a refrigerator for 15 min. The nurses were then dissected alive on a petri dish floating in an ice bath to reduce movement while preserving gut flora. Immediately after dissection, the entire gut of a single nurse from crop to rectum was homogenized with a pestle and added to 250 μL of 50% sucrose solution (Kwong et al., 2017). The microbial solutions of four nurse bees from a single hive were mixed and placed into one cell of each of the cup cages corresponding to the nurse bees’ hive of origin in addition to the 30% sucrose solution prior to adding newly eclosed bees. The microbes were ingested and assumed to spread through the population through trophallaxis (Powell et al., 2014).
Cup cages were placed in an incubator maintained at 34 ± 0.5°C. Humidity was maintained at 75 ± 10% RH using a saturated aqueous sodium chloride solution placed on the bottom shelf of the incubator. These conditions were selected to mimic those of a healthy honey bee colony (Winston, 1987). Bees were maintained in cup cages for 12–72 h depending on the timing of eclosion, and mortality was noted daily.
Callow Bee Caging
On day 19 after larval grafting, approximately 1–3 days after adult eclosion, bees were transferred from cup cages to petri dish cages derived from Shpigler and Robinson (2015). Alterations to this design included two 1.3 cm diameter holes in the edge of the petri dish for 2 mL feeder tubes, with an additional 0.6 cm diameter hole centered between them. Petri dish cages were assembled with a wax foundation base and stood on edge with feeder holes facing up using wooden stands (see Figure 1). A ball of pollen supplement made with 1:1 w/w 50% sucrose solution and MegaBee pollen supplement powder weighing roughly 1.5 g was added to the bottom of each dish, and a maximum of 10 bees were added from the cup cages to each dish. Bees were kept in separate cages according to treatment and colony of origin. One Qeq of artificial QP secured onto a safety pin and tied to a length of fishing line was dangled into the cage through a feeder hole and taped so that the safety pin rested in the center of the dish. Two feeders made from 2 mL microcentrifuge tubes, one containing a 50% sucrose solution and one deionized water, were added to the dish and the smaller hole between them was taped closed.
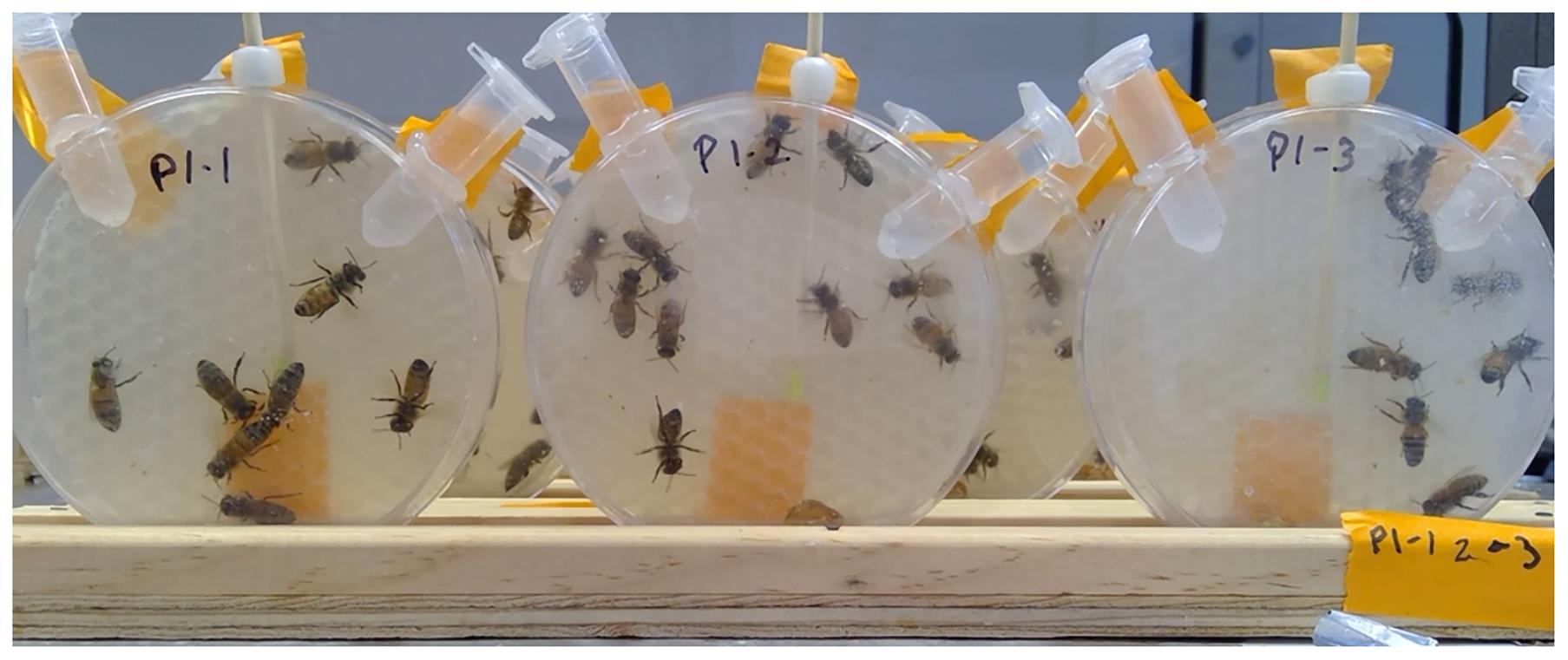
Figure 1. Petri dish cages with Apis mellifera workers approximately 8 days after adult eclosion inside heated PCR cabinet. Workers were exposed during larval development to IGDs. This image was taken prior to recording worker responses to queen pheromone. Coded labels correspond to treatment and hive.
Petri dish cages were kept inside an incubator maintained at 34 ± 0.5°C and 75 ± 10% RH. Bees remained in this incubator until they were approximately 7–9 days old, which corresponds to the period when worker bees are most responsive to queen pheromone (Kaminski et al., 1990). During this time, mortality was recorded daily and bees were fed ad libitum. Details on the minimum number of bees used for behavioral assessments are given in the “Statistical Analysis” section.
Queen Pheromone Response Behavior
Synthetic QP is widely used as a substitute for a mated queen in packages and temporarily queenless colonies prior to the introduction of a new queen (Naumann et al., 1990). For this reason, a nine component synthetic QP blend, which is comprised of the same components as natural QP (Keeling et al., 2003), was used in this experiment in leu of QP extracted from a sacrificed queen. QP lures for observations were made by fixing 1 Qeq of a TempQueen plastic strip to a 25 cm long wooden dowel and wrapping a piece of parafilm around the dowel 5.5 cm from the QP. The parafilm skirt ensured the QP would sit in the center of the petri dish cage during observations (Figure 1). To increase the novelty of the stimulus and encourage bees to respond to QP, safety pins with QP were removed from the petri dish cages 24 h before beginning observations.
On day 25 after grafting, when bees were between 7 and 9 days old, petri dish cages on stands were placed in a PCR workstation (AirClean 600 PCR Workstation, AirClean Systems) with a space heater (Fan-Forced Utility Heater, Soleil, Geneva Industrial Group) to maintain a temperature of roughly 32°C. The bees were permitted to acclimate to the new environment for 30 min before observations began.
Before each recording, a QP lure was inserted through the small center hole of each petri dish cage. The lure was positioned in the cage with the TempQueen strip facing toward the lid, visible to the camera, with the back of the dowel pressed into the wax foundation for stability. Once the lures were in place, 5 min video recordings were taken for 3 petri dish cages at a time. After the recording, the lures were removed and placed in the next set of 3 cages before beginning a new recording. This process was repeated until the bees in all petri dish cages had been recorded, rotating the placement of the cages in the PCR Workstation. The cycle was then repeated two more times for a total of three 5 min videos for each petri dish cage.
The queen pheromone responsiveness of workers was assessed using a modified point sampling approach described by Rangel et al. (2016). This assessment involved counting the number of bees antennating, licking, or otherwise contacting the QP strip during 10 s intervals with 20 s between observations. For each video recording, the number of contacts observed during the entire 5 min period was summed and divided by the number of bees present in the dish.
Statistical Analysis
Kaplan Meier Survival Analysis was used to evaluate differences in immature and adult survivorship among treatment groups. Although adult bees were maintained for 6–9 days prior to the behavioral assay, the majority were 8 days old at the conclusion of the experiment. Because bees eclosing at different time points were mixed as adults, all bees were assigned an age of 2 days at the time of transfer to petri dish cages.
Separate linear mixed effects models (LMM) were constructed to assess treatment dependent differences in pupal development time and weight at adult eclosion with replicate and source colony treated as random effects. Significance of predictors was evaluated using Wald’s tests. Post hoc comparisons between treatments were made using Tukey’s HSD tests. Generalized linear models (GLM) with a binomial distribution were used for each replicate to evaluate the proportion of adult bees eclosing from each treatment group with obvious deformities for each replicate. Random effects were not incorporated in this analysis due to issues with model singularity relating to the homogeneity of the data.
Spearman’s rank correlation coefficient was used to assess the relationship between the number of bees in a petri dish cage and worker QP response. A significant correlation between the number of bees in a dish and the number of QP responses per bee was observed when petri dish cages containing less than 3 bees were included in the analysis (Replicate 1: Rs = 0.0554, p = 0.0440; Replicate 2: Rs = 0.0805, p = 0.0059), therefore, only cages containing 3–10 bees were used to assess worker QP response (Replicate 1: Rs = 0.0118, p = 0.6773; Replicate 2: Rs = −0.0308, p = 0.3186). Using the censored worker QP response data, a generalized linear mixed effects model (GLMM) was constructed with QP response per bee as the response variable and treatment group as a predictor. Replicate and source colony were treated as random effects and a Poisson distribution was specified. Prior to analysis, QP response data were transformed to integers using the following equation and rounding to the nearest whole number: (x + 1) × 102. Significance of predictors was evaluated using Wald’s tests. Post hoc comparisons between treatments were made using Tukey’s HSD tests.
For this work, all statistical analysis was performed in R Studio 1.2.5003 (Boston, MA, United States). Figures were prepared using R Studio, JMP Pro 15 and Photoshop CC 2019 (Adobe Inc., San Jose, CA, United States).
Results
Development: First Instar to Adult Eclosion
Survivorship to Adult Eclosion
There was no difference in larval survivorship among treatment groups in Replicates 1 or 2 (Replicate 1: χ2 = 0.4, df = 3, p = 1.00; Replicate 2: χ2 = 3.5, df = 3, p = 0.3). Overall, average survivorship was 76.30 ± 0.51% for Replicate 1 and 73.43 ± 2.25% for Replicate 2.
Development Time
The duration of pupation was significantly affected by developmental exposure to pyriproxyfen (T = −8.986, df = 751.3, p ≤ 0.0001) and diflubenzuron (T = 2.047, df = 752.7, p = 0.0410; Figure 2), though post hoc pairwise comparisons between diflubenzuron and all treatment groups indicated no significant differences. Conversely, post hoc pairwise comparisons revealed that pyriproxyfen treated bees experienced significantly shortened pupation periods relative to all other treatments. See Table 1 for a summary of post hoc Tukey HSD tests.
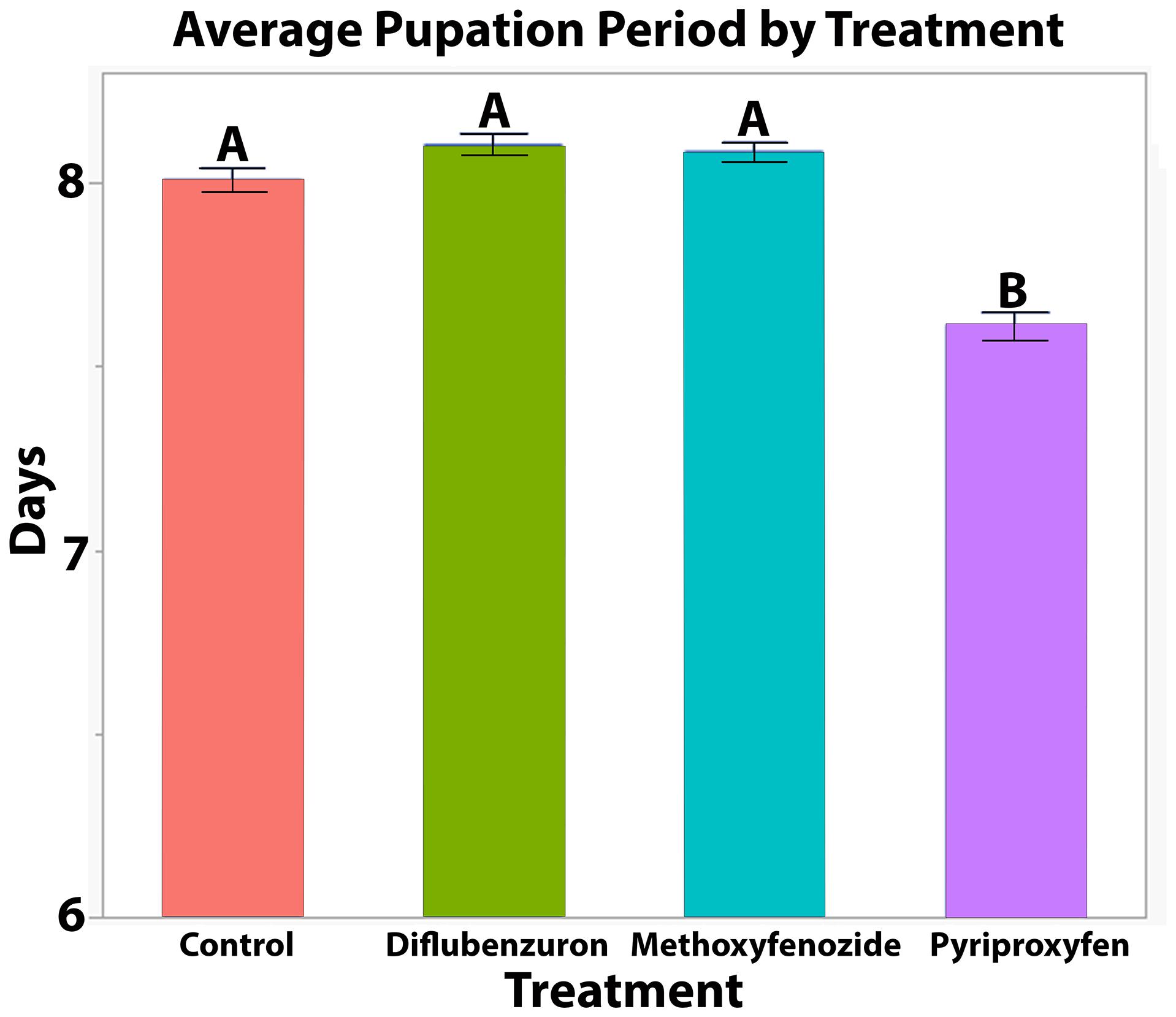
Figure 2. Average ± S.E. time from first observation of successful pupal eclosion to successful adult eclosion of Apis mellifera workers following developmental exposure to IGDs. Significance indicated by letters (LMM, Tukey HSD, See Table 1).
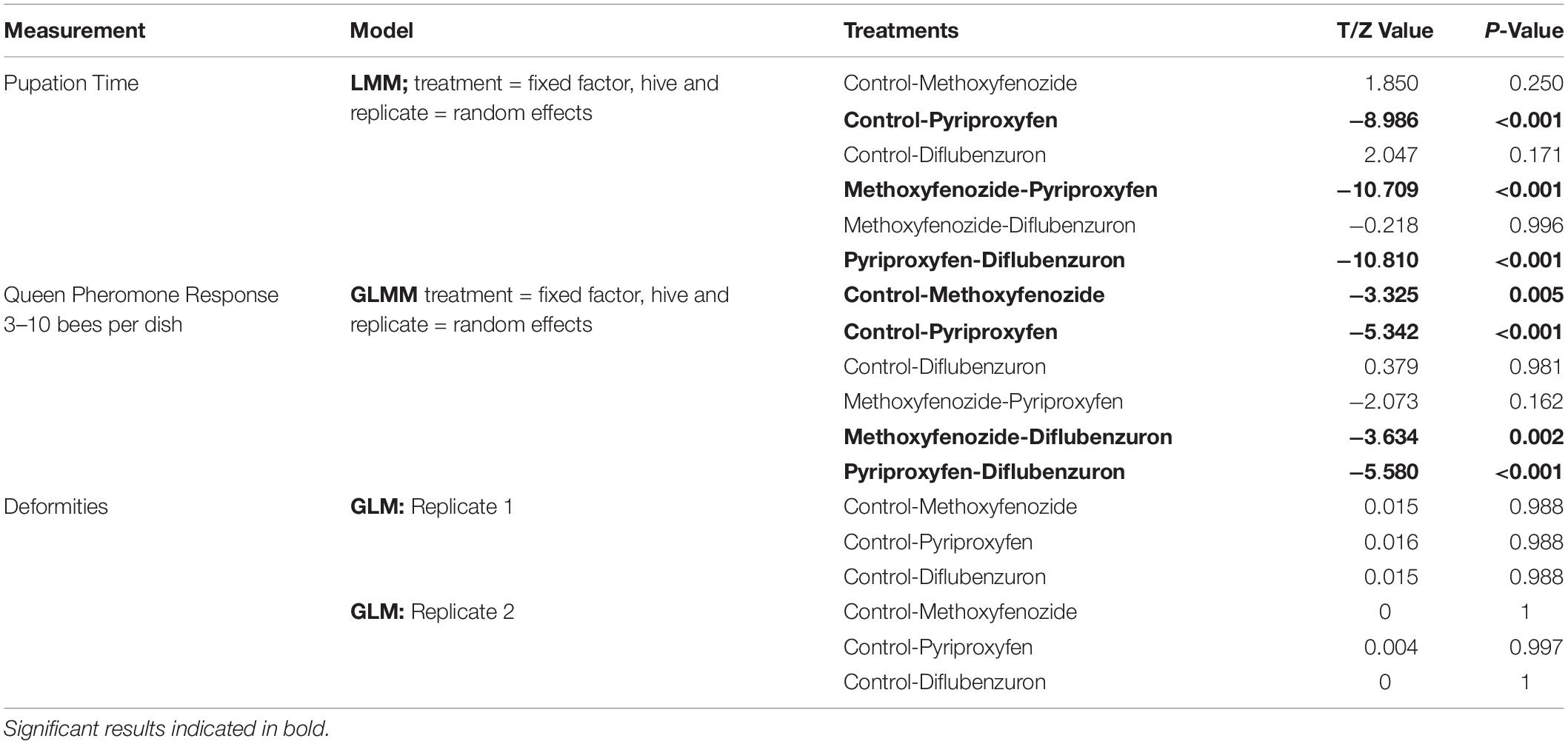
Table 1. Results of statistical analysis of pupation time, QP response per bee and rate of deformities in eclosing Apis mellifera adults following developmental exposure to IGDs.
External Morphology
No effect of treatment on the rate of deformities was observed (see Table 1). Wing deformation was the only deformity observed in eclosing bees in this study, and overall, rates of this deformity remained low among all replicates (Replicate 1 = 3.39 ± 1.27%, Replicate 2 = 0.52 ± 0.52%).
Treatment had no effect on the weight of eclosing bees (Diflubenzuron: T = 1.777, df = 745.9, p = 0.0760; Methoxyfenozide: T = −0.747, df = 744.3, p = 0.4551, Pyriproxyfen: T = 0.553, df = 744.6, p = 0.5807).
Adult Survivorship and Behavior
Adult Survival
Over the course of the 8 days following the final day of adult eclosion, the survivorship of adult bees in Replicate 1 was negatively affected by developmental exposure to IGD laced diet (χ2 = 38.5, df = 3, p ≤ 0.0001; Figure 3). Bees that had been developmentally exposed to pyriproxyfen exhibited the lowest rates of survival relative to all treatments (Control: p ≤ 0.0001; Methoxyfenozide: p ≤ 0.0001; Diflubenzuron: p = 0.0054), and bees developmentally exposed to diflubenzuron had lower survival relative to control (p = 0.0054). In Replicate 2, there was no effect of treatment on adult survivorship observed (χ2 = 3.9, df = 3, p = 0.3).
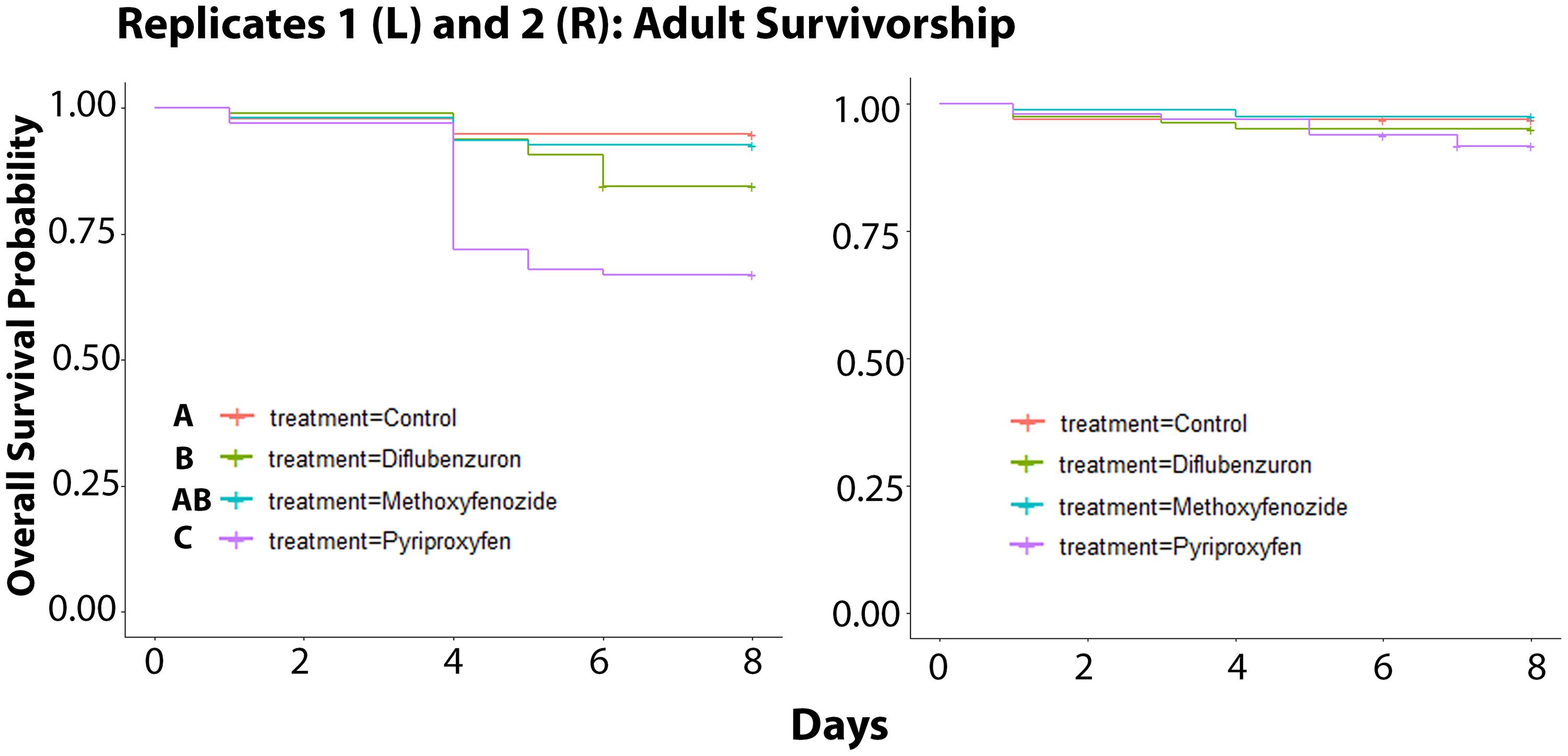
Figure 3. Survivorship of adult Apis mellifera workers following developmental exposure to IGDs for Replicates 1 (Kaplan Meier Survival Analysis, χ2 = 38.5, df = 3, p ≤ 0.0001) and 2 (χ2 = 3.9, df = 3, p = 0.3). Significance indicated by letters.
Queen Pheromone Response
Developmental exposure to methoxyfenozide and pyriproxyfen had significant effects on the QP responses of worker bees (Pyriproxyfen: Z-value = −5.342, p ≤ 0.0001; Methoxyfenozide: Z-value = −3.325, p = 0.0009; Figure 4). Post hoc pairwise testing between treatments showed that pyriproxyfen and methoxyfenozide treated bees responded significantly less to queen pheromone relative to all other treatments except for each other. See Table 1 for post hoc test statistics and p-values.
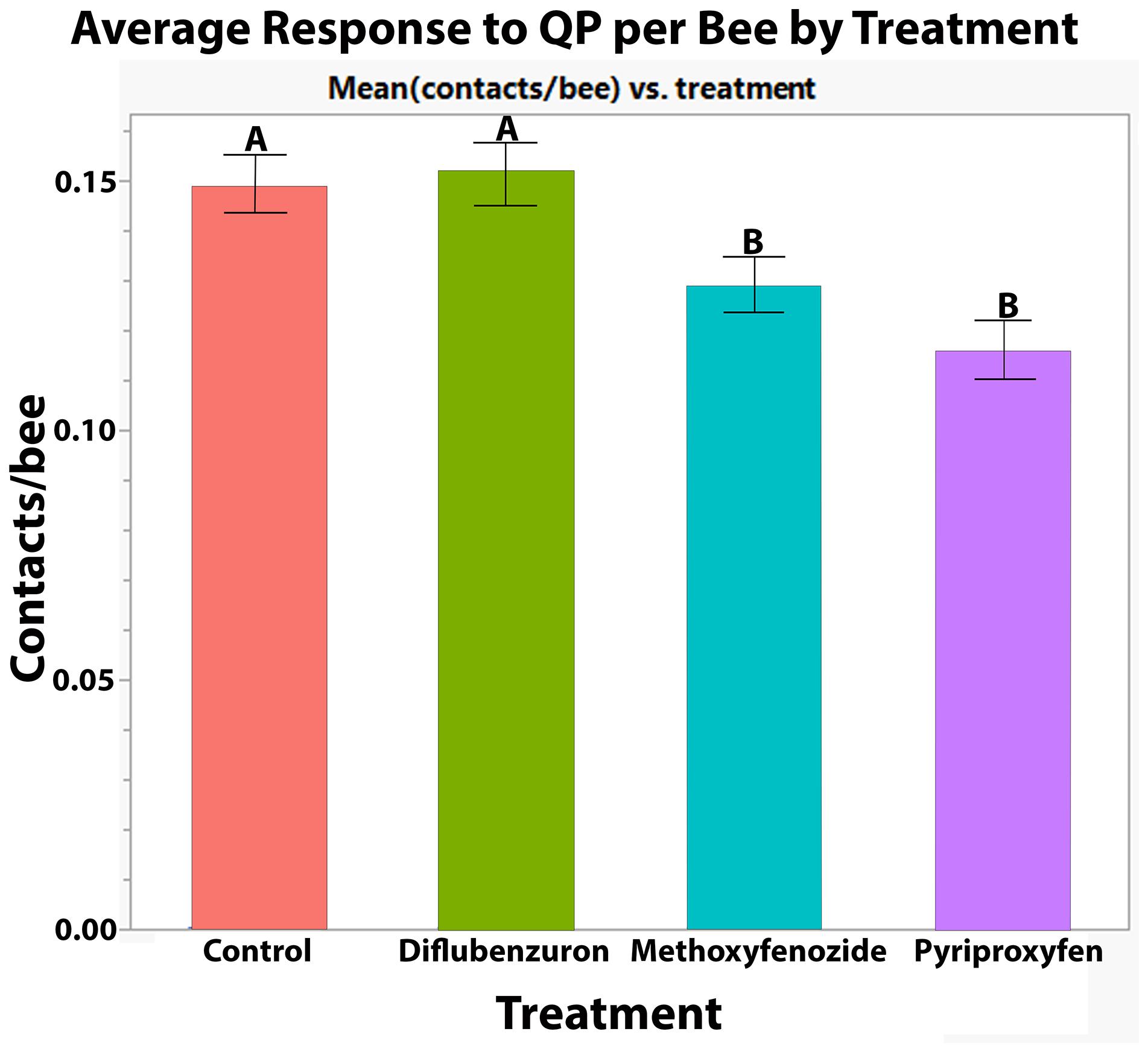
Figure 4. Average ± S.E. number of contacts with QP lure per bee made by Apis mellifera workers in petri dish cages following developmental exposure to IGDs. Significance indicated by letters (GLMM, Tukey HSD).
Discussion
Exposure to agrochemicals has been identified as a major contributing factor in honey bee colony losses (Goulson et al., 2015), yet the effects of sublethal agrochemical exposure during honey bee development are still not fully understood. For social insects, the performance of altruistic behaviors that contribute to the overall productivity of the colony rather than the individual is necessary to sustain the structure of the superorganism (Hamilton, 1963; Oster and Wilson, 1978; Ratnieks and Wenseleers, 2008; Ratnieks and Helanterä, 2009; Shorter and Rueppell, 2012), and any shifts in these behaviors may be deleterious to the colony unit (Perry et al., 2015). The results of this work demonstrate that developmental exposure to IGDs can influence adult survival and the performance of a social behavior that is necessary to sustain queen productivity and colony expansion (Allen, 1960).
Residues of methoxyfenozide have been reported in the pollen stores of colonies at concentrations ranging from 5.7–1820.0 ppb in 2.2% of samples (Rennich et al., 2013, 2014), and diflubenzuron has been measured at 84.3–252.0 ppb in 0.6% of samples (Rennich et al., 2013, 2014). Pyriproxyfen has been found at 1.5–277.0 ppb in 0.9% of samples (Rennich et al., 2013, 2014), though a more recent survey reported a lower range of 1–13.6 ppb in 0.76% of samples (Traynor et al., 2021a). In comparison, the doses used in our study correspond to 16100 ppb (g/mL) methoxyfenozide, 167.4 ppb diflubenzuron, and 164 ppb pyriproxyfen. Therefore, the concentrations used here are high, but such concentrations of pyriproxyfen and diflubenzuron can be found within honey bee colonies. The highest reported concentrations of methoxyfenozide, however, are nearly ten-fold lower than the concentration used here, making it unlikely that larvae would be directly exposed to the levels used in this work.
For many compounds, much lowered concentrations have been observed in larval queen diet following colony exposure (Böhme et al., 2018), and developing workers only receive a small amount of pollen in their diet in the latter days of larval development (Haydak, 1970), suggesting that larvae are at much lower exposure risk through diet relative to adults. It is not known how prevalent IGD residues are in royal jelly, but numerous studies have shown profound impacts on larvae following colony exposure, suggesting that IGDs do translocate to larval diet at appreciable concentrations, albeit when colonies are directly exposed to heavily contaminated food sources [as reviewed in Fine and Corby-Harris (2021)]. Furthermore, beekeepers frequently report heavy brood loss following almond pollination, where IGDs, along with other agrochemicals, are often applied directly to blooming crops (Pollinator Stewardship Council, 2014). Another source of IGD exposure is contaminated wax (Mullin et al., 2010), though this exposure scenario may affect larvae differently than through direct ingestion. Ultimately, more work is needed to evaluate the concentrations of IGDs in larval diet, but this work demonstrates that IGDs, particularly those that mimic insect hormones, can affect the behavior of adult bees exposed during development.
In this work, exposure to the JH analog, pyriproxyfen, and the ecdysteroid agonist, methoxyfenozide, resulted in significantly fewer observed responses to artificial QP. Unlike diflubenzuron, which acts to inhibit chitin synthesis in developing bees (Grosscurt, 1978), hormone mimics act on the insect endocrine system to interfere with developmental processes (Williams, 1967; Hoffmann and Lorenz, 1998; Jindra and Bittova, 2020). In adult honey bees, rising JH triggers the transition from in hive tasks like nursing and queen care to riskier tasks like foraging and guarding (Robinson, 1985). Ecdysteroids are involved in oocyte maturation and embryogenesis (Bloch et al., 2002), and caged feeding studies have demonstrated that they can affect hypopharyngeal gland development (Corby-Harris et al., 2016, 2019). However, in developing bees, JH and ecdysteroids have different functions. Primarily, JH and the major ecdysteroid in honey bees, makisterone A (Feldlaufer et al., 1986) act in concert to regulate the timing of molting and development (Weir, 1970). Dramatic changes in the titers of these hormones can disrupt this process completely by delaying or accelerating molting too drastically for the insect to recover (Dhadialla et al., 1998), but natural variation in these titers, particularly in JH, can affect caste determination.
During larval development, increases in the hemolymph titers of JH cause female larvae to develop as queens rather than workers (Bloch et al., 2002). Relative to workers, queens experience shortened pupation periods and greatly increased reproductive potential as adults (Winston, 1987). In this work, pyriproxyfen treated bees consistently experienced shorter pupation periods compared to other treatments, which is suggestive of a queen intermediate state. Queen intermediates or intercaste honey bees, are understood to be physiological and behavioral hybrids between queens and workers (Beetsma, 1979) and are less likely to participate in typical worker bee tasks (Hillesheim et al., 1989; Mattila et al., 2012). Shortened development time in response to pyriproxyfen has been previously demonstrated (Bitondi et al., 1998; Elekonich et al., 2003; Fourrier et al., 2015), and Fourrier et al. (2015) found that developmental pyriproxyfen exposure resulted in decreased performance of social behaviors in adult bees. Reproductive potential is known to negatively influence QP responsiveness (Galbraith et al., 2015), which may be the cause of the reduced QP response observed after exposure to pyriproxyfen.
While the effects of sublethal methoxyfenozide exposure during development on adult bees are less well established, it has been shown that colony level exposure can result in decreased thermoregulation (Meikle et al., 2019). Here, we demonstrated that the underpinning of queen retinue behavior, QP responsiveness, can also be affected by methoxyfenozide exposure. More work is needed to explore why methoxyfenozide treatment resulted in lower responsiveness to QP, but these results suggest that, like JH, ecdysteroids and their agonists may affect the physiology and brain development of honey bees during larval development.
Beyond the reduced QP responsiveness, reductions in adult longevity were particularly pronounced for pyriproxyfen treated bees in Replicate 1, which experienced more than 25% higher mortality relative to the control group prior to the behavioral assay. Similarly, diflubenzuron treated bees experienced reduced adult survival relative to control bees. Although the exact cause of the mortality reported in Replicate 1 cannot be determined from this work, it is possible that pyriproxyfen and diflubenzuron treated bees were more vulnerable to a stressor introduced through an uncontrolled variable in this experiment. Due to a queen loss event, a new colony was introduced in the second experimental replicate. Response to stressors like pesticide exposure is known to have a heritable component (Rinkevich et al., 2015; Milone and Tarpy, 2021), though striking differences in sensitivity within the same stock are unlikely. Another possibility is that the variation was related to differences in the adult microbiome of the inoculated worker bees. The honey bee microbiome is known to significantly impact honey bee health (Zheng et al., 2017; Raymann and Moran, 2018; Vernier et al., 2020; Retschnig et al., 2021). The microbial inoculations in this work were performed to more realistically mimic colony conditions, though this practice may have exposed our bees to pathogens which are known to affect honey bee health and behaviors in unpredictable ways (Goblirsch et al., 2013; Gómez-Moracho et al., 2017; Geffre et al., 2020). Furthermore, combined stressors like pathogens and agrochemicals are known to synergize (Doublet et al., 2015; Fine et al., 2017; O’Neal et al., 2018). Therefore, while any negative effects of the microbial inoculations used in this work are purely speculative, we suggest that it may benefit future work to use a standardized microbial inoculum.
This work demonstrates that pyriproxyfen and methoxyfenozide affect the performance of a social behavior intrinsic to colony reproduction and longevity while inducing no obvious abnormalities in brood or newly eclosed adults. Stress induced changes in honey bee behavior are known to negatively affect colony dynamics and accelerate colony failure (Thompson et al., 2007; Perry et al., 2015). Until recently, the majority of studies have focused on behavioral effects of stress during insect adulthood, though developmental conditions are also known to influence physiological and behavioral characteristics of adult insects (Mousseau and Dingle, 1991; Rossiter, 1991; Fox, 1993). Like all other insects, honey bee development is hormonally regulated, and changes to larval and pupal hormone balance can result in mortality or altered physiological and behavioral phenotypes (Tunaz and Uygun, 2004; Fourrier et al., 2015; Jindra and Bittova, 2020).
This work did not investigate the reproductive potential or physiology of pyriproxyfen and methoxyfenozide treated bees, and thus, the underlying cause of the decreased responsiveness to QP cannot be definitively determined. However, it can be inferred from models examining the influence of precocious foraging on colony reproduction that if a high enough proportion of bees are unresponsive to a true, fertilized queen, colony populations would quickly dwindle due to the low number of new workers produced (Thompson et al., 2007; Perry et al., 2015). Given the importance of honey bee pollinators to global food production, it is imperative to understand how IGDs and other stressors may influence the development of immature bees and how these changes may contribute to the success or failure of colony units.
Data Availability Statement
Upon request, the raw data supporting the conclusions of this article will be made available by the authors, without undue reservation.
Author Contributions
EL contributed to the experimental design, implementation, and reporting of the results with guidance from JF. JF performed statistical analysis. SC assisted in performing relevant research and in the implementation of the experiment. EL and JF wrote the final manuscript. All authors edited the manuscript.
Funding
This research was supported by USDA project 2030-21000-053-00-D.
Conflict of Interest
The authors declare that the research was conducted in the absence of any commercial or financial relationships that could be construed as a potential conflict of interest.
Publisher’s Note
All claims expressed in this article are solely those of the authors and do not necessarily represent those of their affiliated organizations, or those of the publisher, the editors and the reviewers. Any product that may be evaluated in this article, or claim that may be made by its manufacturer, is not guaranteed or endorsed by the publisher.
Acknowledgments
We thank Dr. Arian Avalos and Professor Adam Dolezal for their helpful reviews of this manuscript. We also thank Dr. Elina Lastro Niño and Bernardo Niño for assisting with the apicultural aspects of this work. We wish to thank Dr. Hagai Shpigler for his advice regarding the design of the queen retinue response assay. Mention of trade names or commercial products in this publication is solely for the purpose of providing specific information and does not imply recommendation or endorsement by the U.S. Department of Agriculture. USDA is an equal opportunity provider and employer.
References
Abramson, C. I., Squire, J., Sheridan, A., and Mulder, P. G. (2004). The effect of insecticides considered harmless to honey bees (Apis mellifera): proboscis conditioning studies by using the insect growth regulators tebufenozide and diflubenzuron. Environ. Entomol. 33, 378–388. doi: 10.1603/0046-225X-33.2.378
Aizen, M. A., Garibaldi, L. A., Cunningham, S. A., and Klein, A. M. (2008). Long-Term global trends in crop yield and production reveal no current pollination shortage but increasing pollinator dependency. Curr. Biol. 18, 1572–1575. doi: 10.1016/j.cub.2008.08.066
Allen, M. D. (1960). The honeybee queen and her attendants. Anim. Behav. 8, 201–208. doi: 10.1016/0003-3472(60)90028-2
Beetsma, J. (1979). The process of queen-worker differentiation in the honeybee. Bee World 60, 24–39. doi: 10.1080/0005772X.1979.11097727
Berthoud, H., Imdorf, A., Haueter, M., Radloff, S., and Neumann, P. (2010). Virus infections and winter losses of honey bee colonies (Apis mellifera). J. Apic. Res. 49, 60–65. doi: 10.3896/IBRA.1.49.1.08
Bitondi, M. M. G., Mora, I. M., Simões, Z. L. P., and Figueiredo, V. L. C. (1998). The Apis mellifera pupal melanization program is affected by treatment with a juvenile hormone analogue. J. Insect Physiol. 44, 499–507. doi: 10.1016/s0022-1910(97)00113-3
Bloch, G., Wheeler, D. E., and Robinson, G. E. (2002). “40 - endocrine influences on the organization of insect societies,” in Hormones, Brain and Behavior, eds D. W. Pfaff, A. P. Arnold, S. E. Fahrbach, A. M. Etgen, and R. T. Rubin (San Diego, CA: Academic Press), 195–235.
Böhme, F., Bischoff, G., Zebitz, C. P. W., Rosenkranz, P., and Wallner, K. (2018). From field to food—will pesticide-contaminated pollen diet lead to a contamination of royal jelly? Apidologie 49, 112–119. doi: 10.1007/s13592-017-0533-3
Booton, R. D., Iwasa, Y., Marshall, J. A. R., and Childs, D. Z. (2017). Stress-mediated Allee effects can cause the sudden collapse of honey bee colonies. J. Theor. Biol. 420, 213–219. doi: 10.1016/j.jtbi.2017.03.009
Bordier, C., Suchail, S., Pioz, M., Devaud, J. M., Collet, C., Charreton, M., et al. (2017). Stress response in honeybees is associated with changes in task-related physiology and energetic metabolism. J. Insect Physiol. 98, 47–54. doi: 10.1016/j.jinsphys.2016.11.013
Calderone, N. W. (2012). Insect pollinated crops, insect Pollinators and US agriculture: trend analysis of aggregate data for the period 1992–2009. PLoS One 7:e37235. doi: 10.1371/journal.pone.0037235
CalDPR (2019). CalPIP Home - California Pesticide Information Portal. CalPIP. Available online at: https://calpip.cdpr.ca.gov/main.cfm (accessed February 11, 2020).
Chen, Y.-W., Wu, P.-S., Yang, E.-C., Nai, Y.-S., and Huang, Z. Y. (2016). The impact of pyriproxyfen on the development of honey bee (Apis mellifera L.) colony in field. J. Asia-Pac. Entomol. 19, 589–594. doi: 10.1016/j.aspen.2016.06.005
Ciarlo, T. J., Mullin, C. A., Frazier, J. L., and Schmehl, D. R. (2012). Learning impairment in honey bees caused by agricultural spray adjuvants. PLoS One 7:e40848. doi: 10.1371/journal.pone.0040848
Colin, T., Meikle, W. G., Wu, X., and Barron, A. B. (2019). Traces of a neonicotinoid induce precocious foraging and reduce foraging performance in honey bees. Environ. Sci. Technol. 53, 8252–8261. doi: 10.1021/acs.est.9b02452
Corby-Harris, V., Meador, C. A. D., Snyder, L. A., Schwan, M. R., Maes, P., Jones, B. M., et al. (2016). Transcriptional, translational, and physiological signatures of undernourished honey bees (Apis mellifera) suggest a role for hormonal factors in hypopharyngeal gland degradation. J. Insect Physiol. 85, 65–75. doi: 10.1016/j.jinsphys.2015.11.016
Corby-Harris, V., Snyder, L., and Meador, C. (2019). Fat body lipolysis connects poor nutrition to hypopharyngeal gland degradation in Apis mellifera. J. Insect Physiol. 116, 1–9. doi: 10.1016/j.jinsphys.2019.04.001
Crailsheim, K., Brodschneider, R., Aupinel, P., Behrens, D., Genersch, E., Vollmann, J., et al. (2013). Standard methods for artificial rearing of Apis mellifera larvae. J. Apic. Res. 52, 1–16. doi: 10.3896/IBRA.1.52.1.05
Dai, P., Jack, C. J., Mortensen, A. N., Bloomquist, J. R., and Ellis, J. D. (2018). The impacts of chlorothalonil and diflubenzuron on Apis mellifera L. larvae reared in vitro. Ecotoxicol. Environ. Saf. 164, 283–288. doi: 10.1016/j.ecoenv.2018.08.039
DeGrandi-Hoffman, G., and Chen, Y. (2015). Nutrition, immunity and viral infections in honey bees. Curr. Opin. Insect Sci. 10, 170–176. doi: 10.1016/j.cois.2015.05.007
Dhadialla, T. S., Carlson, G. R., and Le, D. P. (1998). New insecticides with ecdysteroidal and juvenile hormone activity. Annu. Rev. Entomol. 43, 545–569. doi: 10.1146/annurev.ento.43.1.545
Dively, G. P., Embrey, M. S., Kamel, A., Hawthorne, D. J., and Pettis, J. S. (2015). Assessment of chronic sublethal effects of imidacloprid on honey bee colony health. PLoS One 10:e0118748. doi: 10.1371/journal.pone.0118748
Donkersley, P., Rhodes, G., Pickup, R. W., Jones, K. C., and Wilson, K. (2014). Honeybee nutrition is linked to landscape composition. Ecol. Evol. 4, 4195–4206. doi: 10.1002/ece3.1293
Doublet, V., Labarussias, M., Miranda, J. R., Moritz, R. F. A., and Paxton, R. J. (2015). Bees under stress: sublethal doses of a neonicotinoid pesticide and pathogens interact to elevate honey bee mortality across the life cycle. Environ. Microbiol. 17, 969–983. doi: 10.1111/1462-2920.12426
Downey, D., Higo, T. T., and Winston, M. L. (2000). Single and dual parasitic mite infestations on the honey bee, Apis mellifera L. Insectes Sociaux 47, 171–176. doi: 10.1007/PL00001697
Elekonich, M. M., Jez, K., Ross, A. J., and Robinson, G. E. (2003). Larval juvenile hormone treatment affects pre-adult development, but not adult age at onset of foraging in worker honey bees (Apis mellifera). J. Insect Physiol. 49, 359–366. doi: 10.1016/S0022-1910(03)00020-9
Elias-Neto, M., Soares, M. P. M., and Bitondi, M. M. G. (2009). Changes in integument structure during the imaginal molt of the honey bee. Apidologie 40, 29–39. doi: 10.1051/apido:2008064
Evans, J. D., Chen, Y. P., Prisco, G., Pettis, J., and Williams, V. (2009). Bee cups: single-use cages for honey bee experiments. J. Apic. Res. 48, 300–302. doi: 10.1080/00218839.2009.11101548
Feldlaufer, M. F., Svoboda, J. A., and Herbert, E. W. (1986). Makisterone A and 24-methylenecholesterol from the ovaries of the honey bee,Apis mellifera L. Experientia 42, 200–201. doi: 10.1007/BF01952468
Fine, J. D., and Corby-Harris, V. (2021). Beyond brood: the potential impacts of insect growth disruptors on the long-term health and performance of honey bee colonies. Apidologie 52, 580–595. doi: 10.1007/s13592-021-00845-x
Fine, J. D., Cox-Foster, D. L., and Mullin, C. A. (2017). An inert pesticide adjuvant synergizes viral pathogenicity and mortality in honey bee larvae. Sci. Rep. 7:40499. doi: 10.1038/srep40499
Fine, J. D., Shpigler, H. Y., Ray, A. M., Beach, N. J., Sankey, A. L., Cash-Ahmed, A., et al. (2018). Quantifying the effects of pollen nutrition on honey bee queen egg laying with a new laboratory system. PLoS One 13:e0203444. doi: 10.1371/journal.pone.0203444
Fisher, A., Colman, C., Hoffmann, C., Fritz, B., and Rangel, J. (2018). The effects of the insect growth regulators methoxyfenozide and pyriproxyfen and the acaricide bifenazate on honey bee (Hymenoptera: apidae) forager survival. J. Econ. Entomol. 111, 510–516. doi: 10.1093/jee/tox347
Fourrier, J., Deschamps, M., Droin, L., Alaux, C., Fortini, D., Beslay, D., et al. (2015). Larval exposure to the juvenile hormone analog pyriproxyfen disrupts acceptance of and social behavior performance in adult honeybees. PLoS One 10:e0132985. doi: 10.1371/journal.pone.0132985
Fox, C. W. (1993). The influence of maternal age and mating frequency on egg size and offspring performance in Callosobruchus maculatus (Coleoptera: bruchidae). Oecologia 96, 139–146. doi: 10.1007/BF00318042
Free, J. B. (1961). Hypopharyngeal gland development and division of labour in honey-bee (apis Mellifera L.) colonies. Proc. R. Entomol. Soc. Lond. Ser. Gen. Entomol. 36, 5–8. doi: 10.1111/j.1365-3032.1961.tb00253.x
Galbraith, D. A., Wang, Y., Amdam, G. V., Page, R. E., and Grozinger, C. M. (2015). Reproductive physiology mediates honey bee (Apis mellifera) worker responses to social cues. Behav. Ecol. Sociobiol. 69, 1511–1518. doi: 10.1007/s00265-015-1963-4
Geffre, A. C., Gernat, T., Harwood, G. P., Jones, B. M., Morselli Gysi, D., Hamilton, A. R., et al. (2020). Honey bee virus causes context-dependent changes in host social behavior. Proc. Natl. Acad. Sci. U.S.A. 117, 10406–10413. doi: 10.1073/pnas.2002268117
Goblirsch, M., Huang, Z. Y., and Spivak, M. (2013). Physiological and behavioral changes in honey bees (Apis mellifera) induced by nosema ceranae infection. PLoS One 8:e58165. doi: 10.1371/journal.pone.0058165
Gómez-Moracho, T., Heeb, P., and Lihoreau, M. (2017). Effects of parasites and pathogens on bee cognition. Ecol. Entomol. 42, 51–64. doi: 10.1111/een.12434
Goulson, D., Nicholls, E., Botías, C., and Rotheray, E. L. (2015). Bee declines driven by combined stress from parasites, pesticides, and lack of flowers. Science 347:1255957. doi: 10.1126/science.1255957
Grosscurt, A. C. (1978). Diflubenzuron: some aspects of its ovicidal and larvicidal mode of action and an evaluation of its practical possibilities. Pestic. Sci. 9, 373–386. doi: 10.1002/ps.2780090502
Grozinger, C. M., and Robinson, G. E. (2007). Endocrine modulation of a pheromone-responsive gene in the honey bee brain. J. Comp. Physiol. A 193, 461–470. doi: 10.1007/s00359-006-0202-x
Hamaidia, K., and Soltani, N. (2021). Methoxyfenozide, a molting hormone agonist, affects autogeny capacity, oviposition, fecundity, and fertility in culex pipiens (Diptera: culicidae). J. Med. Entomol. 58, 1004–1011. doi: 10.1093/jme/tjaa260
Hamilton, W. D. (1963). The evolution of altruistic behavior. Am. Nat. 97, 354–356. doi: 10.1086/497114
Harwood, G. P., and Dolezal, A. G. (2020). Pesticide–Virus interactions in honey bees: challenges and opportunities for understanding drivers of bee declines. Viruses 12:566. doi: 10.3390/v12050566
Haydak, M. H. (1970). Honey bee nutrition. Annu. Rev. Entomol. 15, 143–156. doi: 10.1146/annurev.en.15.010170.001043
Henry, M., Cerrutti, N., Aupinel, P., Decourtye, A., Gayrard, M., Odoux, J.-F., et al. (2015). Reconciling laboratory and field assessments of neonicotinoid toxicity to honeybees. Proc. R. Soc. B Biol. Sci. 282:20152110. doi: 10.1098/rspb.2015.2110
Hillesheim, E., Koeniger, N., and Moritz, R. F. A. (1989). Colony performance in honeybees (Apis mellifera capensis Esch.) depends on the proportion of subordinate and dominant workers. Behav. Ecol. Sociobiol. 24, 291–296. doi: 10.1007/BF00290905
Hoffmann, K. H., and Lorenz, M. W. (1998). Recent advances in hormones in insect pest control. Phytoparasitica 26, 323–330. doi: 10.1007/BF02981447
Hölldobler, B., and Wilson, E. O. (2009). The Superorganism: The Beauty, Elegance, and Strangeness of Insect Societies. New York, NY: W. W. Norton & Company.
Hoover, S. E. R., Keeling, C. I., Winston, M. L., and Slessor, K. N. (2003). The effect of queen pheromones on worker honey bee ovary development. Naturwissenschaften 90, 477–480. doi: 10.1007/s00114-003-0462-z
Jaycox, E. R., Skowronek, W., and Guynn, G. (1974). Behavioral changes in worker honey bees (Apis mellifera) induced by injections of a juvenile hormone mimic. Ann. Entomol. Soc. Am. 67, 529–534. doi: 10.1093/aesa/67.4.529
Jindra, M., and Bittova, L. (2020). The juvenile hormone receptor as a target of juvenoid “insect growth regulators.”. Arch. Insect Biochem. Physiol. 103:e21615. doi: 10.1002/arch.21615
Johnson, B. R. (2010). Division of labor in honeybees: form, function, and proximate mechanisms. Behav. Ecol. Sociobiol. 64, 305–316. doi: 10.1007/s00265-009-0874-7
Kaminski, L.-A., Slessor, K. N., Winston, M. L., Hay, N. W., and Borden, J. H. (1990). Honeybee response to queen mandibular pheromone in laboratory bioassays. J. Chem. Ecol. 16, 841–850. doi: 10.1007/BF01016494
Keeling, C. I., Slessor, K. N., Higo, H. A., and Winston, M. L. (2003). New components of the honey bee (Apis mellifera L.) queen retinue pheromone. Proc. Natl. Acad. Sci. U.S.A. 100, 4486–4491. doi: 10.1073/pnas.0836984100
Kocher, S. D., Richard, F.-J., Tarpy, D. R., and Grozinger, C. M. (2009). Queen reproductive state modulates pheromone production and queen-worker interactions in honeybees. Behav. Ecol. 20, 1007–1014. doi: 10.1093/beheco/arp090
Kulhanek, K., Steinhauer, N., Rennich, K., Caron, D. M., Sagili, R. R., Pettis, J. S., et al. (2017). A national survey of managed honey bee 2015–2016 annual colony losses in the USA. J. Apic. Res. 56, 328–340. doi: 10.1080/00218839.2017.1344496
Kwong, W. K., Mancenido, A. L., and Moran, N. A. (2017). Immune system stimulation by the native gut microbiota of honey bees. R. Soc. Open Sci. 4:170003. doi: 10.1098/rsos.170003
Leimar, O., Hartfelder, K., Laubichler, M. D., and Page, R. E. (2012). Development and evolution of caste dimorphism in honeybees – a modeling approach. Ecol. Evol. 2, 3098–3109. doi: 10.1002/ece3.414
Leonhardt, S. D., and Blüthgen, N. (2012). The same, but different: pollen foraging in honeybee and bumblebee colonies. Apidologie 43, 449–464. doi: 10.1007/s13592-011-0112-y
Liao, L.-H., Wu, W.-Y., and Berenbaum, M. R. (2017). Behavioral responses of honey bees (Apis mellifera) to natural and synthetic xenobiotics in food. Sci. Rep. 7, 1–8. doi: 10.1038/s41598-017-15066-5
Mattila, H. R., and Otis, G. W. (2006). Influence of pollen diet in spring on development of honey bee (Hymenoptera: apidae) colonies. J. Econ. Entomol. 99, 604–613. doi: 10.1093/jee/99.3.604
Mattila, H. R., Reeve, H. K., and Smith, M. L. (2012). Promiscuous honey bee queens increase colony productivity by suppressing worker selfishness. Curr. Biol. 22, 2027–2031. doi: 10.1016/j.cub.2012.08.021
Meikle, W. G., Corby-Harris, V., Carroll, M. J., Weiss, M., Snyder, L. A., Meador, C. A. D., et al. (2019). Exposure to sublethal concentrations of methoxyfenozide disrupts honey bee colony activity and thermoregulation. PLoS One 14:e0204635. doi: 10.1371/journal.pone.0204635
Milchreit, K., Ruhnke, H., Wegener, J., and Bienefeld, K. (2016). Effects of an insect growth regulator and a solvent on honeybee (Apis mellifera L.) brood development and queen viability. Ecotoxicology 25, 530–537. doi: 10.1007/s10646-016-1611-4
Milone, J. P., and Tarpy, D. R. (2021). Effects of developmental exposure to pesticides in wax and pollen on honey bee (Apis mellifera) queen reproductive phenotypes. Sci. Rep. 11:1020. doi: 10.1038/s41598-020-80446-3
Moriarty, F. (1969). The sublethal effects of synthetic insecticides on insects. Biol. Rev. 44, 321–356. doi: 10.1111/j.1469-185X.1969.tb01214.x
Mousseau, T. A., and Dingle, H. (1991). Maternal effects in insect life histories. Annu. Rev. Entomol. 36, 511–534. doi: 10.1146/annurev.en.36.010191.002455
Mullin, C. A., Fine, J. D., Reynolds, R. D., and Frazier, M. T. (2016). Toxicological risks of agrochemical spray adjuvants: organosilicone surfactants may not be safe. Front. Public Health 4:92. doi: 10.3389/fpubh.2016.00092
Mullin, C. A., Frazier, M., Frazier, J. L., Ashcraft, S., Simonds, R., vanEngelsdorp, D., et al. (2010). High levels of miticides and agrochemicals in north american apiaries: implications for honey bee health. PLoS One 5:e9754. doi: 10.1371/journal.pone.0009754
Natsopoulou, M. E., McMahon, D. P., and Paxton, R. J. (2016). Parasites modulate within-colony activity and accelerate the temporal polyethism schedule of a social insect, the honey bee. Behav. Ecol. Sociobiol. 70, 1019–1031. doi: 10.1007/s00265-015-2019-5
Naumann, K., Winston, M. L., Wyborn, M. H., and Slessor, K. N. (1990). Effects of synthetic, honey bee (Hymenoptera: apidae) queen mandibular-gland pheromone on workers in packages. J. Econ. Entomol. 83, 1271–1275. doi: 10.1093/jee/83.4.1271
Nazzi, F., and Pennacchio, F. (2018). Honey bee antiviral immune barriers as affected by multiple stress factors: a novel paradigm to interpret colony health decline and collapse. Viruses 10:159. doi: 10.3390/v10040159
Neumann, P., and Carreck, N. L. (2010). Honey bee colony losses. J. Apic. Res. 49, 1–6. doi: 10.3896/IBRA.1.49.1.01
O’Neal, S. T., Anderson, T. D., and Wu-Smart, J. Y. (2018). Interactions between pesticides and pathogen susceptibility in honey bees. Curr. Opin. Insect Sci. 26, 57–62. doi: 10.1016/j.cois.2018.01.006
Oster, G. F., and Wilson, E. O. (1978). Caste and Ecology in the Social Insects. New Jersey, NJ: Princeton University Press.
Pener, M. P., and Dhadialla, T. S. (2012). “Chapter one - an overview of insect growth disruptors; applied aspects,” in Advances in Insect Physiology Insect Growth Disruptors ed T. S. Dhadialla (Cambridge, MA: Academic Press), 1–162.
Perry, C. J., Søvik, E., Myerscough, M. R., and Barron, A. B. (2015). Rapid behavioral maturation accelerates failure of stressed honey bee colonies. Proc. Natl. Acad. Sci. U.S.A. 112, 3427–3432. doi: 10.1073/pnas.1422089112
Pham-Delègue, M. H., Trouiller, J., Caillaud, C. M., Roger, B., and Masson, C. (1993). Effect of queen pheromone on worker bees of different ages: behavioural and electrophysiological responses. Apidologie 24, 267–281. doi: 10.1051/apido:19930307
Pollinator Stewardship Council (2014). CATCH THE BUZZ: Huge Bee Kill In Almonds. Bee Cult. Available online at: https://www.beeculture.com/catch-the-buzz-huge-bee-kill-in-almonds/ (accessed April 14, 2020).
Powell, J. E., Martinson, V. G., Urban-Mead, K., and Moran, N. A. (2014). Routes of acquisition of the gut microbiota of the honey bee apis mellifera. Appl. Environ. Microbiol. 80, 7378–7387. doi: 10.1128/AEM.01861-14
Rangel, J., Böröczky, K., Schal, C., and Tarpy, D. R. (2016). Honey bee (Apis mellifera) queen reproductive potential affects queen mandibular gland pheromone composition and worker retinue response. PLoS One 11:e0156027. doi: 10.1371/journal.pone.0156027
Ratnieks, F. L. W., and Helanterä, H. (2009). The evolution of extreme altruism and inequality in insect societies. Philos. Trans. R. Soc. B Biol. Sci. 364, 3169–3179. doi: 10.1098/rstb.2009.0129
Ratnieks, F. L. W., and Wenseleers, T. (2008). Altruism in insect societies and beyond: voluntary or enforced? Trends Ecol. Evol. 23, 45–52. doi: 10.1016/j.tree.2007.09.013
Raymann, K., and Moran, N. A. (2018). The role of the gut microbiome in health and disease of adult honey bee workers. Curr. Opin. Insect Sci. 26, 97–104. doi: 10.1016/j.cois.2018.02.012
Rennich, K., Kunkel, G., Abban, S., Bozarth, R., Eversole, H., Evans, J., et al. (2013). 2012-2013 National Honey Bee Pests and Diseases Survey Report. Animal and Plant Health Inspection Service. Available online at: https://ushoneybeehealthsurvey.info/wp-content/uploads/sites/3/2020/04/2012-13-End-of-year-final-report.pdf (accessed May 12, 2021).
Rennich, K., Kunkel, G., Abban, S., Fahey, R., Eversole, H., Evans, J., et al. (2014). 2013-2014 National Honey Bee Pests and Diseases Survey Report. Animal and Plant Health Inspection Service. Available online at: https://ushoneybeehealthsurvey.info/wp-content/uploads/sites/3/2020/04/2013-2014-NHBS-Report.pdf (accessed May 12, 2021).
Retschnig, G., Rich, J., Crailsheim, K., Pfister, J., Perreten, V., and Neumann, P. (2021). You are what you eat: relative importance of diet, gut microbiota and nestmates for honey bee, Apis mellifera, worker health. Apidologie. 52, 632–646. doi: 10.1007/s13592-021-00851-z
Rinkevich, F. D., Margotta, J. W., Pittman, J. M., Danka, R. G., Tarver, M. R., Ottea, J. A., et al. (2015). Genetics, synergists, and age affect insecticide sensitivity of the honey bee, apis mellifera. PLoS One 10:e0139841. doi: 10.1371/journal.pone.0139841
Robinson, G. E. (1985). Effects of a juvenile hormone analogue on honey bee foraging behaviour and alarm pheromone production. J. Insect Physiol. 31, 277–282. doi: 10.1016/0022-1910(85)90003-4
Robinson, G. E., Winston, M. L., Huang, Z.-Y., and Pankiw, T. (1998). Queen mandibular gland pheromone influences worker honey bee (Apis mellifera L.) foraging ontogeny and juvenile hormone titers. J. Insect Physiol. 44, 685–692. doi: 10.1016/s0022-1910(98)00040-7
Rossiter, M. C. (1991). Environmentally-based maternal effects: a hidden force in insect population dynamics? Oecologia 87, 288–294. doi: 10.1007/BF00325268
Sanchez-Bayo, F., and Goka, K. (2014). Pesticide residues and bees – a risk assessment. PLoS One 9:e94482. doi: 10.1371/journal.pone.0094482
Schmehl, D. R., Tome, H. V. V., Mortensen, A. N., Ferreira Martins, G., and Ellis, J. D. (2016). Protocol for the in Vitro Rearing of Honey Bee (Apis mellifera L.) Workers. Available online at: https://www.tandfonline.com/doi/full/10.1080/00218839.2016.1203530 (accessed May 7, 2020).
Schulz, D. J., Huang, Z. Y., and Robinson, G. E. (1998). Effects of colony food shortage on behavioral development in honey bees. Behav. Ecol. Sociobiol. 42, 295–303. doi: 10.1007/s002650050442
Seeley, T. D. (1982). Adaptive significance of the age polyethism schedule in honeybee colonies. Behav. Ecol. Sociobiol. 11, 287–293. doi: 10.1007/BF00299306
Shorter, J. R., and Rueppell, O. (2012). A review on self-destructive defense behaviors in social insects. Insectes Sociaux 59, 1–10. doi: 10.1007/s00040-011-0210-x
Shpigler, H. Y., and Robinson, G. E. (2015). Laboratory assay of brood care for quantitative analyses of individual differences in honey bee (Apis mellifera) affiliative behavior. PLoS One 10:e0143183. doi: 10.1371/journal.pone.0143183
Siviter, H., Koricheva, J., Brown, M. J. F., and Leadbeater, E. (2018). Quantifying the impact of pesticides on learning and memory in bees. J. Appl. Ecol. 55, 2812–2821. doi: 10.1111/1365-2664.13193
Slater, G. P., Yocum, G. D., and Bowsher, J. H. (2020). Diet quantity influences caste determination in honeybees (Apis mellifera). Proc. R. Soc. B Biol. Sci. 287:20200614. doi: 10.1098/rspb.2020.0614
Slessor, K. N., Kaminski, L.-A., King, G. G. S., Borden, J. H., and Winston, M. L. (1988). Semiochemical basis of the retinue response to queen honey bees. Nature 332, 354–356. doi: 10.1038/332354a0
Smagghe, G., and Degheele, D. (1994). Action of the nonsteroidal ecdysteroid mimic RH 5849 on larval development and adult reproduction of insects of different orders. Invertebr. Reprod. Dev. 25, 227–236. doi: 10.1080/07924259.1994.9672389
Southwick, E. E., and Southwick, L. Jr. (1992). Estimating the economic value of honey bees (Hymenoptera: apidae) as agricultural pollinators in the United States. J. Econ. Entomol. 85, 621–633. doi: 10.1093/jee/85.3.621
Steinhauer, N., vanEngelsdorp, D., and Saegerman, C. (2021). Prioritizing changes in management practices associated with reduced winter honey bee colony losses for US beekeepers. Sci. Total Environ. 753:141629. doi: 10.1016/j.scitotenv.2020.141629
Tasei, J.-N. (2001). Effects of insect growth regulators on honey bees and non-Apis bees. A review. Apidologie 32, 527–545. doi: 10.1051/apido:2001102
Thompson, H. M., Wilkins, S., Battersby, A. H., Waite, R. J., and Wilkinson, D. (2005). The effects of four Insect Growth-Regulating (IGR) insecticides on honeybee (Apis mellifera L.) colony development, queen rearing and drone sperm production. Ecotoxicology 14, 757–769. doi: 10.1007/s10646-005-0024-6
Thompson, H. M., Wilkins, S., Battersby, A. H., Waite, R. J., and Wilkinson, D. (2007). Modelling long-term effects of IGRs on honey bee colonies. Pest Manag. Sci. 63, 1081–1084. doi: 10.1002/ps.1457
Traynor, K. S., Pettis, J. S., Tarpy, D. R., Mullin, C. A., Frazier, J. L., Frazier, M., et al. (2016). In-hive Pesticide Exposome: assessing risks to migratory honey bees from in-hive pesticide contamination in the Eastern United States. Sci. Rep. 6:33207. doi: 10.1038/srep33207
Traynor, K. S., Tosi, S., Rennich, K., Steinhauer, N., Forsgren, E., Rose, R., et al. (2021a). Pesticides in honey bee colonies: establishing a baseline for real world exposure over seven years in the USA. Environ. Pollut. 279:116566. doi: 10.1016/j.envpol.2021.116566
Traynor, K. S., vanEngelsdorp, D., and Lamas, Z. S. (2021b). Social disruption: sublethal pesticides in pollen lead to Apis mellifera queen events and brood loss. Ecotoxicol. Environ. Saf. 214:112105. doi: 10.1016/j.ecoenv.2021.112105
Tunaz, H., and Uygun, N. (2004). Insect growth regulators for insect. Turk. J. Agric. For. 28, 377–387.
van Dame, R., Meled, M., Colin, M.-E., and Belzunces, L. P. (1995). Alteration of the homing-flight in the honey bee Apis mellifera L. Exposed to sublethal dose of deltamethrin. Environ. Toxicol. Chem. 14, 855–860. doi: 10.1002/etc.5620140517
vanEngelsdorp, D., Hayes, J., Underwood, R. M., and Pettis, J. (2008). A survey of honey bee colony losses in the U.S., fall 2007 to spring 2008. PLoS One 3:e4071. doi: 10.1371/journal.pone.0004071
vanEngelsdorp, D., Underwood, R. M., Caron, D. M., and Hayes, J. (2007). An estimate of managed colony losses in the Winter of 2006-2007: a report commissioned by the apiary inspectors of America. Am. Bee J. 147, 599–603.
Vergoz, V., McQuillan, H. J., Geddes, L. H., Pullar, K., Nicholson, B. J., Paulin, M. G., et al. (2009). Peripheral Modulation of Worker bee Responses to Queen Mandibular Pheromone. - Abstract - Europe PMC. Available online at: https://europepmc.org/article/pmc/pmc2791564 (accessed May 4, 2021).
Vernier, C. L., Chin, I. M., Adu-Oppong, B., Krupp, J. J., Levine, J., Dantas, G., et al. (2020). The gut microbiome defines social group membership in honey bee colonies. Sci. Adv. 6:eabd3431. doi: 10.1126/sciadv.abd3431
Wade, A., Lin, C.-H., Kurkul, C., Regan, E., and Johnson, R. (2019). Combined toxicity of insecticides and fungicides applied to California almond orchards to honey bee larvae and adults. Insects 10:20. doi: 10.3390/insects10010020
Walsh, E., Sweet, S., Knap, A., Ing, N., and Rangel, J. (2020). Queen honey bee (Apis mellifera) pheromone and reproductive behavior are affected by pesticide exposure during development. Behav. Ecol. Sociobiol. 74:33. doi: 10.1007/s00265-020-2810-9
Walton, A., Dolezal, A. G., Bakken, M. A., and Toth, A. L. (2018). Hungry for the queen: honeybee nutritional environment affects worker pheromone response in a life stage-dependent manner. Funct. Ecol. 32, 2699–2706. doi: 10.1111/1365-2435.13222
Wu, J. Y., Anelli, C. M., and Sheppard, W. S. (2011). Sub-Lethal effects of pesticide residues in brood comb on worker honey bee (Apis mellifera) development and longevity. PLoS One 6:e14720. doi: 10.1371/journal.pone.0014720
Zheng, H., Powell, J. E., Steele, M. I., Dietrich, C., and Moran, N. A. (2017). Honeybee gut microbiota promotes host weight gain via bacterial metabolism and hormonal signaling. Proc. Natl. Acad. Sci. U.S.A. 114, 4775–4780. doi: 10.1073/pnas.1701819114
Keywords: reproduction, pesticide, sublethal effects, pollinator, honey bee, insect growth regulator, insect hormone mimic
Citation: Litsey EM, Chung S and Fine JD (2021) The Behavioral Toxicity of Insect Growth Disruptors on Apis mellifera Queen Care. Front. Ecol. Evol. 9:729208. doi: 10.3389/fevo.2021.729208
Received: 22 June 2021; Accepted: 31 August 2021;
Published: 07 October 2021.
Edited by:
Brad Metz, North Carolina State University, United StatesReviewed by:
Kirsten Shoshanna Traynor, Arizona State University, United StatesSelina Bruckner, Auburn University, United States
Geoff Williams, Auburn University, United States
Frank Rinkevich, Honey Bee Breeding, Genetics, and Physiology Research (USDA-ARS), United States
Copyright © 2021 Litsey, Chung and Fine. This is an open-access article distributed under the terms of the Creative Commons Attribution License (CC BY). The use, distribution or reproduction in other forums is permitted, provided the original author(s) and the copyright owner(s) are credited and that the original publication in this journal is cited, in accordance with accepted academic practice. No use, distribution or reproduction is permitted which does not comply with these terms.
*Correspondence: Julia D. Fine, SnVsaWEuZmluZUB1c2RhLmdvdg==