- 1Department of Anthropology, Dartmouth College, Hanover, NH, United States
- 2Ecology, Evolution, Environment, and Society, Dartmouth College, Hanover, NH, United States
- 3Department of Biology, Boston University, Boston, MA, United States
- 4Graduate Program in Neuroscience, Boston University, Boston, MA, United States
- 5Department of Anthropology, Boston University, Boston, MA, United States
- 6Department of Evolution, Ecology, and Organismal Biology, The Ohio State University, Columbus, OH, United States
- 7Department of Anatomy and Cell Biology, Oklahoma State University Center for Health Sciences, Tulsa, OK, United States
Human brain size nearly quadrupled in the six million years since Homo last shared a common ancestor with chimpanzees, but human brains are thought to have decreased in volume since the end of the last Ice Age. The timing and reason for this decrease is enigmatic. Here we use change-point analysis to estimate the timing of changes in the rate of hominin brain evolution. We find that hominin brains experienced positive rate changes at 2.1 and 1.5 million years ago, coincident with the early evolution of Homo and technological innovations evident in the archeological record. But we also find that human brain size reduction was surprisingly recent, occurring in the last 3,000 years. Our dating does not support hypotheses concerning brain size reduction as a by-product of body size reduction, a result of a shift to an agricultural diet, or a consequence of self-domestication. We suggest our analysis supports the hypothesis that the recent decrease in brain size may instead result from the externalization of knowledge and advantages of group-level decision-making due in part to the advent of social systems of distributed cognition and the storage and sharing of information. Humans live in social groups in which multiple brains contribute to the emergence of collective intelligence. Although difficult to study in the deep history of Homo, the impacts of group size, social organization, collective intelligence and other potential selective forces on brain evolution can be elucidated using ants as models. The remarkable ecological diversity of ants and their species richness encompasses forms convergent in aspects of human sociality, including large group size, agrarian life histories, division of labor, and collective cognition. Ants provide a wide range of social systems to generate and test hypotheses concerning brain size enlargement or reduction and aid in interpreting patterns of brain evolution identified in humans. Although humans and ants represent very different routes in social and cognitive evolution, the insights ants offer can broadly inform us of the selective forces that influence brain size.
We live in a community of knowledge. Everything we do depends on knowledge that is both inside our head as well as out in the world and in other people’s heads.
Steven Slomen1, author of Knowledge Illusion: Why We Never Think Alone
The key to the origin of the human condition is not to be found in our species exclusively, because the story did not start and end with humanity.
E. O. Wilson, The Social Conquest of Earth
Only humans and social insects can build and manage large-scale societies according to complex economic decision rules.
Introduction
Understanding the causes and consequences of brain evolution in humans—particularly the role of social life—is significant to understanding the nature of humanity. Across diverse clades, sociality is hypothesized to drive brain size and structure. In primates, greater cognitive challenges associated with forming bonded social groups in large societies, among other influences (DeCasien et al., 2017; González-Forero and Gardner, 2018; DeCasien and Higham, 2019), appear to have selected for increased brain size (Dunbar, 1998; Dunbar and Shultz, 2007, 2017; Meguerditchian et al., 2021). A broad phylogenetic perspective can be of significant value in exploring the evolution of nervous systems (Striedter et al., 2014; Keifer and Summers, 2016; Shigeno, 2017). Although significantly different in sociality, computation and decision-making in humans and social insects are accomplished by physical neuroarchitectures (“solid brains”) as well as “liquid brains” formed by interactions of group members that create collective intelligence (Couzin, 2009; Pagán, 2019; Piñero and Solé, 2019; Reséndiz-Benhumea et al., 2021). Across diverse species that vary in social organization, cognitive demands on individuals may be lower in societies in which group decision-making is more efficacious than individual decision-making (Surowiecki, 2004; Sumpter, 2006; Krause et al., 2010; Woolley et al., 2010; Sasaki and Pratt, 2018; Bak-Coleman et al., 2021). Superorganismic decentralized “brain” networks characterize humans and ants, the premier social insect. Collective intelligence may reduce brain size in both clades (Bailey and Geary, 2009; Feinerman and Traniello, 2016). Therefore, the size of groups and society-level intelligence may affect behavioral performance and cognitive loads and increase or reduce brain size, depending on context.
Over the course of hominin evolution, encephalization has been dynamic (e.g., Miller et al., 2019). Australopithecus cranial capacities were on average 20% larger than those of the late Miocene hominins Sahelanthropus and Ardipithecus or modern chimpanzees, despite having chimpanzee-sized bodies (Wolpoff, 1999; Cartmill and Smith, 2009; DeSilva, 2011). These ∼450 cc brains remained roughly unchanged in size from 3.5 to 2.0 million years ago even though late australopiths (e.g., Paranthropus) underwent extensive diversification. With the evolution of Homo, brains began to expand but gross neural organization may have remained primitive (Ponce de León et al., 2021). Additionally, brain expansion was not universal in fossil Homo as evidenced by the small-brained Middle and Late Pleistocene hominins Homo naledi (Berger et al., 2015; Montgomery, 2018) and Homo floresiensis (Brown et al., 2004; Figure 1). Although an almost fourfold increase in brain volume during the last 2 million years is a hallmark in human evolution, it remains unappreciated—but well-documented—that both absolute and relative brain size have decreased since the end of the Pleistocene (Schwidetzky, 1976; Wiercinski, 1979; Beals et al., 1984; Henneberg, 1988; Henneberg and Steyn, 1993; Ruff et al., 1997; Bailey and Geary, 2009; Hawks, 2011; Bednarik, 2014; Liu et al., 2014; Bruner and Gleeson, 2019). The precise timing of this decrease in brain size, however, is unclear. Some have placed its origin in the late Pleistocene ∼35 kyr (Ruff et al., 1997) and others in the more recent Holocene ∼10 kyr (Henneberg, 1988; Hawks, 2011).
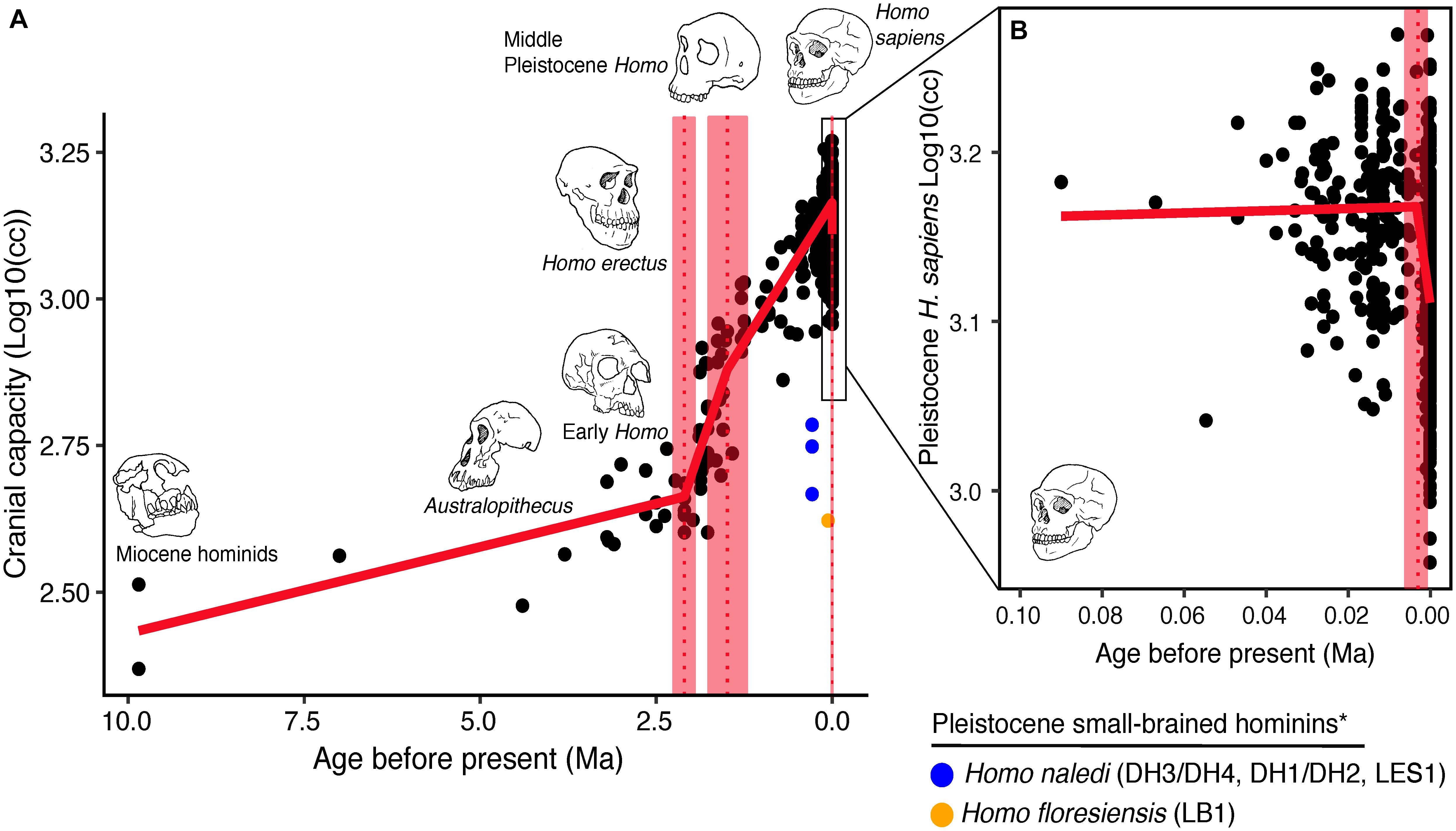
Figure 1. Trends in hominin brain evolution. (A) Cranial capacity in fossil apes (Miocene hominids) and hominins over the last 10 million years. Brain size remained relatively steady throughout the late Miocene and Pliocene, increasing only slightly in Australopithecus compared with earlier hominins. However, by 2 million years ago, there was a dramatic increase in the rate of growth, coinciding with fossil evidence for the earliest members of genus Homo. This change point is illustrated by a red vertical dotted line (95% CI shown as thick pink vertical line). A second change-point is detected at ∼1.5 million years ago and the rate of brain size increase remains steady through the Pleistocene and the evolution of Homo sapiens. If the small-brained Middle and Late Pleistocene hominins H. naledi (blue dots) and H. floresiensis (orange dot) are included, these two change points merge into a single, overlapping encephalization event between 1.97 and 2.21 million years ago. (B) During the last 100,000 years, brain size has remained steady in H. sapiens until a rapid and dramatic change point only 3,000 years ago decreased Holocene human brain size at a rate fifty times greater than the previous increases in Pleistocene brain volume. Each black dot represents an individual fossil skull or osteological specimen.
Here we investigate historical patterns of human brain evolution to date major inflection points of changes in size to attempt to identify selective factors in the environment that may have prevailed during times of significant change in brain size. Because the deep history of neural tissue and its organization is difficult to explore, we use ants to model the broad impacts of social selection on brain size evident in nature to gain insights into the possible selective forces that influenced patterns of human brain evolution. Although phylogenetically remote from humans, ants provide examples of brain evolution that may help identify selective factors and offer neuroarchitectural details to complement the metric of brain size. These advantages may compensate for some of the limitations of behavioral and neurobiological research on extinct hominin forms.
Ants are eusocial insects characterized by reproductive division of labor, cooperative brood care, and overlap of generations. They are exemplars of social life that encompass extant species basal in social structure and highly complex species, enabling comparative studies. Ants are eusocial, and humans have been characterized as eusocial (Foster and Ratnieks, 2005; Boomsma and Franks, 2006; Betzig, 2014; D’Ettorre, 2017b), both forming large, complex, kin-oriented societies, including those with agricultural practices and full-time division of labor. In humans, however, division of labor is not associated with a loss of reproductive potential. Ultrasocial species (Campbell, 1983; Gowdy and Krall, 2013, 2016) produce their own food crops and include some ants (and termites) and humans. Sociobiological parallels can be leveraged to understand the general role of advanced social life in brain evolution. While divergent in key aspects of social organization, humans and ants exhibit important convergences, for example, in the ability to act collectively. And although their brains are structurally and functionally different, our understanding of brain size scaling and structural allometries in ants provide opportunities to address general questions of brain evolution—including size reduction — in humans.
Materials and Methods
To understand temporal patterns of human brain evolution, we applied a change-point analysis to identify the timing of inflection points in hominin brain evolution using our brain size dataset of 985 dated log10 transformed estimates of hominin cranial capacities (cc) compiled from the literature (Supplementary File 1). The dataset represents brain evolution over the last 10 million years of hominid and hominin evolution and includes Rudapithecus (N = 2), Sahelanthropus (N = 1), Ardipithecus (N = 1), Australopithecus (including Paranthropus) (N = 29), Early Pleistocene Homo (N = 37), Middle Pleistocene Homo (N = 60), Late Pleistocene Homo (N = 156), and Holocene H. sapiens (N = 699). We only used published cranial volumes (cc or mL) and not cadaver-derived weights (g) since cranial volume and brain weight are not equivalent (e.g., Tobias, 1970). Analysis was limited to individuals that are estimated to have been at least 10 years old and thus had exceeded the age at which modern H. sapiens achieves adult brain volume (Coqueugniot and Hublin, 2012).
Changepoints were determined using the packages changepoint (Killick and Eckley, 2014) and segmented (Muggeo, 2008) in R Studio (Version 1.2. 5019). Changepoint was first used to provide approximate prior estimates for changes in the mean log10(cc) across the time series using the “BinSeg” method. These prior estimates were then used to fit a piecewise generalized linear model to the data with the segmented package (Muggeo, 2008), which provided estimates of 1) the locations of changepoints (or breakpoints) in the slope of the time series; and 2) the slopes of the lines around each changepoint, which we interpreted as approximate rates of evolutionary change. Because the phylogenetic relationship of the different hominin species remains contentious and because there is genetic evidence for interbreeding between many Late Pleistocene hominin populations (e.g., Gokcumen, 2020), we included all hominin specimens in the change point analysis. However, we ran two separate models: one including the small-brained Middle and Late Pleistocene fossils from H. naledi and H. floresiensis and one excluding them. We mainly describe the results for when these small brained species were excluded (N = 981), but the timing of the decrease in hominin brain size in the Holocene was negligible between these two models.
Results
The best piecewise GLM model fit explained approximately 79% of the variance (adj r2 = 0.79) in log10(cc) values and identified three changepoints (Table 1). The first was detected at 2.10 ± 0.07 Ma, coincident with fossil evidence near the first known occurrence of Homo erectus (Herries et al., 2020). At 2.10 Ma, the rate of evolution increased sharply from 0.03 ± 0.01 log10(cc)/Ma to 0.35 ± 0.05 log10(cc)/Ma. A second changepoint occurred at 1.49 ± 0.14 Ma when the rate of evolution slowed to 0.19 ± 0.01 log10 (cc)/Ma. A steady increase in brain size—independent of body size—followed and lasted through the Pleistocene (Ruff et al., 1997; Lee and Wolpoff, 2003; Rightmire, 2004; Hawks, 2011). We identified a third changepoint at 0.003 ± 0.001 Ma at a rate of −17.16 ± 6.69 log10(cc)/Ma. This rate is 50 times greater than the renowned increase in human brain size. Our data suggest that this reduction may have been more recent—3,000 years ago—than previously suggested. We interpret our result conservatively, and caution that any findings about brain size changes throughout human evolution are contingent on the resolution of the available dataset (e.g., VanSickle et al., 2020). Indeed, the inclusion of smaller-brained Pleistocene hominins (H. naledi and H. floresiensis) reduced model fit slightly (adj r2 = 0.74) and had the effect of widening the 95% CIs of the first two changepoints such that they overlapped between the wider time interval of 2.2–1.7 Ma (Table 2). Yet the timing of the third Holocene changepoint in this model was unaffected by the inclusion of these specimens, although its 95%CI also widened slightly (Table 2).
Discussion
Brain Size Increase and Reduction in Humans
The changepoints we identified document trends in brain size but do not reveal underlying causes and mechanisms of encephalization. The expensive tissue hypothesis (Aiello and Wheeler, 1995) posits that a trade-off in allocated resources from one expensive tissue (the brain) to another (the gut) was made possible by shifts to a higher quality diet in Homo, enabled in part by enhanced technological skills (Lepre et al., 2011), exploitation of diverse resources (Braun et al., 2010), and the invention of cooked foods through controlled fire (Wrangham, 2009; Herculano-Houzel, 2016). In addition to evidence that energetic constraints on encephalization may have been released by dietary shifts in early Homo, others have posited that brain expansion in early Homo may have been driven by the need for enhanced social intelligence (Dunbar, 1998; Dunbar and Shultz, 2017). With growing complexity in social life, perhaps associated with increased group size, brain expansion occurred. Resource sharing within groups and allocare may have provided the energy surplus needed to support the increased energetic cost of a larger brain (Isler and van Schaik, 2012). These hypotheses are not mutually exclusive: a combination of social challenges and ecological pressures and increased dietary quality and breadth drove Pleistocene brain expansion in our ancestors, who were living in increasingly larger groups and likely benefitted from enhanced group-level cognitive abilities and greater cultural intelligence (van Schaik et al., 2012), which could in turn accelerate brain expansion via a feedback loop (Markov and Markov, 2020).
The cause of brain reduction in the Holocene is also unclear. One possibility is that it is associated with a corresponding decrease in body mass (Henneberg, 1988; Ruff et al., 1997). The early expansion of the brain in Homo has been explained as an increase in absolute body size (Ruff et al., 1997; Wood and Collard, 1999; McHenry and Coffing, 2000; Lee and Wolpoff, 2003) though Grabowski (2016) later employed quantitative genetics to suggest that selection had favored brain enlargement and body size increase was a by-product. Hawks (2011) contends that the absolute change in brain size from the Pleistocene to the Holocene is greater than expected based on changes in body mass in the same time period, emphasizing that given the correlation between human brain and body size (Holloway, 1980), the observed 5 kg decrease in body size in the Holocene would account for only a 22 mL decrease in brain volume (Hawks, 2011). However, the actual reduction is more than 5x greater, suggesting that body size alone cannot entirely explain the decrease in brain volume. We find here that body size reduction may have preceded brain size reduction by several millennia. Ruff et al. (1997) notes that the decrease in body size in late Pleistocene humans began around 50 kyr, whereas brain reduction appears to been a Holocene phenomenon.
Yet there may be other non-allometric explanations for the reduction in human brain size at the Pleistocene-Holocene transition due to energetic, nutritional (Wiercinski, 1979), and/or developmental constraints (Hawks, 2011). The brain size reduction in Holocene humans parallels that of domesticated animals, suggesting that humans have self-domesticated by deliberately removing highly aggressive individuals from breeding populations, leading to a reduction in intra-population (but not inter-population) aggression (Leach, 2003; Hare and Tomasello, 2005; Wrangham, 2018, 2019; Bruner and Gleeson, 2019; Hare and Woods, 2020). Brain reduction in this case would be a by-product (or cause) of docility, a phenomenon documented recently in domesticated cattle (Balcarcel et al., 2021). Groves (1999) suggests that domesticated dogs have sufficiently co-evolved with humans to have symbiotically become our external senses, thereby decreasing our reliance on brain centers that process sensory information. However, our finding here—that brain size reduction occurred in just within last 3,000 years—is temporally inconsistent with these prior explanations. Human self-domestication is argued to have occurred at the onset of species ∼300,000 years ago (Wrangham, 2019) or coincident with the evolution of what some have called “behavioral modernity” ∼80,000 years ago (Hare and Woods, 2020). Recent fossil and genetic evidence indicate that dogs were domesticated > 20,000 years ago (Perri et al., 2021).
Human and Ant Sociobiology and Evolutionary Neurobiology: Insights Into Brain Elaboration and Reduction
Human social life has long been analyzed in reference to the social organization of ants. Imms (1946) review of Haskins (1939) Of Ants and Men described ants as “predominant” among the “very few living creatures whose social development at all parallels our own.” He emphasized the value of having “a nearly complete series of evolutionary forms among living ants, numerous ‘missing links’ and ‘living fossils”’ and a history “much more complete and much better preserved than that of man.” Comparisons made to identify commonalities in patterns of sociality between these diverse clades continue to be made today (Boomsma and Franks, 2006; Wilson, 2012; Crespi, 2014; D’Ettorre, 2017a,b; Friedman and Søvik, 2021; Gowdy, 2021). The benefits of ant models for the study of human social evolution noted by Imms are made more compelling by an additional 80 + years of new species discovery (roughly 5,000–6,000 circa 1,940 to > 14,000 today), an expanded phylogeny (6 to ∼20 subfamilies), and an eruption of integrative sociobiological and neurobiological research. Ants exhibit striking variation in colony size and demography, and individual and colony-level cognition. Diversity in social phenotypes creates the potential to provide general insights into human social structure and brain evolution.
Acknowledging the limitations of analogies, human and ant comparisons may reveal patterns in nature that broadly suggest social and ecological selective forces relevant to brain evolution. We recognize that the uniqueness of human cognition (Laland and Seed, 2021) cannot be overemphasized, and neurobiological parallels with ants are as constrained as sociobiological comparisons, if not more limited. Although ant and human brains have the common function of processing environmental information to adaptively respond to social signals and cues, they are structured very differently. The size, neuron number, and synaptic connectivity of an ant brain is a minute fraction of that of a human brain (Herculano-Houzel, 2016; Godfrey et al., 2021). However, the computational power of an ant brain is remarkable for its size and miniaturization does not appear to constrain behavioral performance and/or higher-order processing (Muscedere et al., 2014), social learning, or consciousness (Avarguès-Weber and Giurfa, 2013; Barron and Klein, 2016; Perry et al., 2017; Lihoreau et al., 2019; Perry and Chittka, 2019; Elek et al., 2020; D’Ettorre et al., 2021). Social organization in ants may “require relatively simple and computationally inexpensive forms of cognition” (Lihoreau et al., 2012), some extrinsic to brain operations, that could mitigate the need for advanced processing capability. Behavior in ants typically involves task routines and kinesthetic performance rather than sophisticated cognition. Unlike the human brain (Noonan et al., 2018), ant brains appear to lack executive function and the ability to mentalize, among other circuitry specialized for human social performance.
Relative needs for social information processing are strikingly different in ants and humans. Olfaction is dominant in ants and involves a relatively small number of chemicals to guide individual actions and organize colony-level behavior. Vision appears to have very limited social functions and mainly functions in navigation during foraging (Hölldobler and Wilson, 1990). The anatomy of ant brains thus largely reflects investment in brain centers such as the antennal lobes and optic lobes responsible for processing olfactory and visual stimuli, respectively. These inputs are mainly integrated in the mushroom bodies, brain compartments specialized in higher-order processing, learning, and memory, and to a lesser extent in the central complex. The mushroom bodies, deeply homologous to the vertebrate cortex (Tomer et al., 2010), have long been consider to be the neuroanatomical seat of intelligence in ants and other insects (Strausfeld et al., 1998), but the nature of ant intelligence is only very loosely comparable to that of humans. Mushroom body elaboration also preceded the evolution of eusociality (Farris and Schulmeister, 2011).
Ants have nevertheless emerged as important models for understanding the role of sociality (Ilies et al., 2015; Kamhi et al., 2016, 2019; Godfrey and Gronenberg, 2019) and behavioral performance and cognition (Muratore and Traniello, 2020; Muratore et al., 2021) in brain evolution. Analyses of sociality and brain size scaling (e.g., Godfrey and Gronenberg, 2019; Muratore and Traniello, 2020) and information processing through social interaction (e.g., Davidson et al., 2016) can contribute to broadly understanding how social biology may have influenced general aspects of human brain evolution. The allometric scaling of functionally specialized brain centers in ants allows the adaptiveness of brain mosaics to be explored (e.g., Muratore et al., 2021) and the metabolic costs of neural tissues that are highly significant to brain evolution (Aiello and Wheeler, 1995) can be recorded in individual ant brains (Coto and Traniello, 2021). Studies of the influences of diet, which is considered significant in primate (and human) brain evolution (Wrangham, 2009; DeCasien et al., 2017), are facilitated by the diverse feeding ecologies of ants. Ant nutritional socioecology spans highly specialized as well as generalist predators that vary in colony size and degree of complexity of social organization, allowing the relative roles of diet and social complexity to be separated because specialist and generalist species that each form small and large colonies (Azorsa et al., in preparation). Ants also have culture in the form of tool use (Zhou et al., 2020 and references therein), and although they do not process food by cooking, they may preserve prey (Maschwitz et al., 1979).
The application of theories of vertebrate brain evolution to social insects has been debated (Lihoreau et al., 2012; Farris, 2016) and studies describe variable relationships between sociality, brain and brain compartment size, body size, and group size in eusocial insects (Farris and Schulmeister, 2011; Seid et al., 2011; Riveros et al., 2012). Workers of ant species characterized by larger colony size have larger brains, suggesting greater social interaction selects for increased brain size (Wehner et al., 2007). Similarly, comparisons of socially basic and social complex ant species suggest larger colony size and collective actions have selected for larger brains and mushroom bodies (Kamhi et al., 2016). In contrast, in monomorphic fungus-growing ants, which have agrarian habits, larger colony size is associated with decreased brain size (Riveros et al., 2012). This latter comparison among agricultural ant species provides insight into how increased group size and sociality in human populations may have reduced brain size (Bailey and Geary, 2009) due to a high level of emergent complexity.
Worker behavior undergoes age-related development in ants. Like human brains, ant worker brains exhibit age-related synaptic remodeling, suggesting synaptic pruning in association with an increasingly diverse, flexible, and efficient behavioral repertoire (Seid et al., 2005; Seid and Traniello, 2006; Muscedere et al., 2009). Age and behavioral development can be associated with increased brain volume (Muscedere and Traniello, 2012) and declines in the density of mushroom body microglomeruli (Gordon and Traniello, 2018)—“microprocessor”-like synaptic structures that underlie plasticity in sensory processing capability and behavior (Groh and Rössler, 2011; Groh et al., 2014). Elements of human brain development are thus mirrored in ant brain ontogeny and social behavior. In mole rats, eusocial mammals convergent in social structure with eusocial insects, there is no clear association between social system and relative brain size: eusocial species do not have smaller or larger brains than social or solitary species (Kverková et al., 2018). Although there is an apparent trend between social system and forebrain neuron number, this is likely an artifact of the extreme metabolic adaptations to subterranean life found in H. glaber (Kverková et al., 2018; Browe et al., 2020). Ants appear to provide more useful models than eusocial mammals.
Division of Labor and Brain Investment Patterns
Division of labor is a core social trait in humans and ants that potentially influences brain size evolution by unequally distributing behavioral performance needs and cognitive loads across group members. Greater social complexity in ants is correlated with large group size and division of labor among polyphenic workers (Anderson and McShea, 2001; Kappeler, 2019). Division of labor by morphologically differentiated and behaviorally specialized workers (physical castes) is an attribute of a relatively small number of ant species. Analyses of brain and behavior in these ants, especially those featuring extraordinary polymorphisms and high degrees of behavioral specialization, can provide important information on how distributed cognition impacts the size and scaling of brain size and structure in relation to body size. For example, mushroom bodies are disproportionally large in the brains of media (mid-size) workers of the fungus-growing ant Atta cephalotes. The diverse and behaviorally challenging task repertoire of these workers encompasses leaf-harvesting, and their social role and neuroanatomy differs from that of smaller and larger workers that perform other tasks (Muratore et al., 2021). Using this ant as a model to understand how agriculture has influenced brain evolution may help identify social conditions, life histories, and ecological factors favoring either an increase or decrease in brain size. It can also help identify how brain centers responsible for higher-order processing have evolved in response to either narrowly circumscribed or pluripotent and flexible task repertoires. In humans, such changes may have occurred during the transition from hunter-gatherer to agrarian habits. Ants provide a window through which such evolutionary processes may have occurred, and neuroanatomical outcomes.
Other strongly polymorphic ants offer additional insights into the role of division of labor in the evolution of brain size and compartmental scaling (Muscedere and Traniello, 2012; Ilies et al., 2015; Gordon et al., 2017, 2019). Ecologically different species may have distinct neural phenotypes that vary in the size of sensory input compartments and the mushroom bodies, among other brain centers (Muscedere and Traniello, 2012). Mushroom body size correlates positively with task plasticity, supporting the notion that greater demands on behavioral performance are reflected in greater investment in higher-order processing tissues. Task specialization may also affect brain size and compartmental scaling: larger colonies may show a higher level of task specialization among workers, reflected in relatively larger mushroom bodies (Amador-Vargas et al., 2015). The larger picture is that brain differentiation can occur in association with division of labor in the absence of striking changes in body size, illustrating how selection may have operated in humans as social groups increased in size.
Collective Intelligence and Neuroenergetic Costs
Ponce de León et al. (2008) and Hawks (2011) suggested that reduction of human brain size—without any evidence for intellectual diminishment—implies selection for brain efficiency. In ants, there is evidence of selection for metabolic efficiency in the brain. Kamhi et al. (2016) used cytochrome oxidase (COX) activity, a proxy for neuron metabolism, to contrast brain evolution and social evolution in the weaver ant Oecophylla smaragdina and the garden ant Formica subsericea, two sister clades whose workers are equivalent in body size but differ strongly in social organization and collective intelligence. Increased social complexity in weaver ants—reflected in division of labor by worker physical castes, large colony size, and remarkable decentralized group action including cooperative nest building—was associated with larger mushroom bodies, implying greater needs for higher-order information processing. Weaver ant worker mushroom bodies have reduced COX activity. Therefore, increased brain size in socially complex species may be associated with reduced brain operation costs, contrasting with the assumption that increased brain size increases metabolic costs (Aiello and Wheeler, 1995; Isler and van Schaik, 2009).
Social Selection and Brain Evolution
Reduction in brain size may not compromise cognitive performance if intelligence is an attribute of the society rather than the individual. Galton (1907) first described that the accuracy of decision making by human groups could exceed that of any individual group member. This concept of collective intelligence has since been elaborated in studies ranging from insects to humans (Woolley et al., 2010; Ward et al., 2011; Sasaki and Pratt, 2018; Almaatouq et al., 2020). If brain production, maintenance, and operation costs are metabolically significant (Aiello and Wheeler, 1995; Leonard et al., 2003; Isler and van Shaik, 2006; Chittka and Niven, 2009; Navarrete et al., 2011; Kuzawa et al., 2014; Pontzer et al., 2016; Herculano-Houzel, 2017), then collective intelligence may reduce demands for neural tissue to support individual cognitive capabilities. Using a multidisciplinary modeling approach, Reséndiz-Benhumea et al. (2021) indeed demonstrated that agents with relatively small brains can through social interaction achieve a level of behavioral performance comparable to those of larger-brained but solitary agents. Further testing of this idea, however, will require a better understanding of whether Holocene human brains reduced isometrically or if specific regions reduced in size.
We suggest that group cognition lowered the demands for neural architechtures required to support some aspects of individual intelligence and decision making (Bailey and Geary, 2009). This effect may have become even more pronounced with the advent of writing ca. 5000 years ago (Schmandt-Besserat, 2010), which falls within the estimated 95% CI for the pronounced reduction in Holocene human brain size (Figure 1). During human history, social groups became larger, social interactions more frequent, social networks more complex, and tracking relationships more demanding (Bailey and Geary, 2009; Foley and Gamble, 2009). A rise in sociocultural complexity was not due to particular individuals becoming more intelligent and culturally skilled, but because of the emergence of collective intelligence resulting from a growing population of interconnected humans and interacting human groups. As group size increases, interactions with a dynamic and exceedingly complex social landscapes result in increased demands on the brain (Bickart et al., 2011; Kanai et al., 2012). However, because of the metabolic demands of the brain (Pontzer et al., 2016), there may be limits to feedback loops between social network size and brain structure. If group decision-making generated adaptive group responses exceeding the cognitive accuracy and speed of individual decisions and had a fitness consequence, then human brain size may have decreased as a consequence of metabolic cost savings.
Population size expanded dramatically with the advent of agriculture, beginning ∼10 kyr and grew exponentially from an estimated five million to over 100 million by 3000 years ago (Goldewijk et al., 2011). This increase in population coincided with deterioration in individual health (Armelagos et al., 1991; Milner, 2019) and increases in infection rate (Eshed et al., 2010), pathogenic load (Page et al., 2016), and virulence (Menneret et al., 2010). It remains possible, then, that the high energetic cost of a heightened immune response (Wells and Stock, 2020), might have been a factor in Holocene brain reduction. In fact, Crabtree (2013a; 2013b) proposed this immunity-for-intelligence trade-off in his controversial “Idiocracy Hypothesis,” though this idea has been criticized on the basis of flawed assumptions (Kalinka et al., 2013; Mitchell, 2013).
Gowdy and Krall (2013) draw parallels between the ultrasocial human superorganism, complete with division of labor and “economic organization around surplus” that arose in the Holocene and the sociobiology of agricultural eusocial insects, including some ants and termites. Brain size reduction occurred in traditional hunter-gatherer human populations (Wiercinski, 1979) that never adopted sedentary agricultural practices, but have complex social networks (Apicella et al., 2012). Foster and Ratnieks (2005) suggest that the presence of post-reproductive female helpers (grandmothers) universally present in human societies is sufficient to characterize humans as a “new eusocial vertebrate,” offering additional support for the value of broad comparisons across unrelated taxa.
Conclusion
We suggest that patterns of human brain evolution were influenced by collective intelligence, a convergent characteristic of diverse group-living animals (Surowiecki, 2004; Sumpter, 2006; Woolley et al., 2010; Morand-Ferron and Quinn, 2011; Reid et al., 2015; Biro et al., 2016; Bates and Gupta, 2017; Sasaki and Biro, 2017). The precise role of societal information flow, distribution, and transfer as emergent group properties that may affect brain evolution and neural functioning is not well understood (e.g., Weaverdyck and Parkinson, 2018). Large brains may not be required to generate complex behavior, and brain mosaicism and circuitry—rather than overall size—may be important (Healy and Rowe, 2007, 2013; Chittka and Niven, 2009; Avarguès-Weber et al., 2018; Logan et al., 2018; Godfrey and Gronenberg, 2019). Computational models (e.g., Feinerman and Traniello, 2016; Reséndiz-Benhumea et al., 2021) and patterns in some ant clades (Muscedere and Traniello, 2012; Riveros et al., 2012; Muratore et al., 2021) suggest that group-level cognition may select for reduced brain size and/or adaptive brain size variation. Moreover, complex systems theory predicts that greater social complexity derives from individual simplicity (Delgado and Solé, 1997), although the neurobiological and behavioral meaning of “simplicity” is unclear. Complexity in eusocial insect colony organization may involve selection for either smaller, neurally differentiated worker brains (Lihoreau et al., 2012; Riveros et al., 2012; O’Donnell et al., 2015; Feinerman and Traniello, 2016) or larger brains (Wehner et al., 2007) able to metabolically offset increased production and operation costs (Kamhi et al., 2016). It seems unlikely that a “theory of theories” will appear to universally and meaningfully explain the multiple roles sociality can play across taxa as evolutionarily as divergent as humans and ants. We advocate for an ecumenical and open-minded approach that integrates theories of social, sociocultural, ecological, and mosaic and metabolic brain evolution to create an awareness of the breadth of the natural landscape of possibilities that can encompass both brain size increase and reduction. Human/ant comparisons have heuristic value and can offer a conceptual compass to guide future research.
Data Availability Statement
The original contributions presented in the study are included in the article/Supplementary Material, further inquiries can be directed to the corresponding author/s.
Author Contributions
JD and JT conceived the study and wrote the manuscript. JD accumulated the hominin brain dataset. LF ran the change-point analysis and produced Figure 1. JD and JT edited the manuscript, with contributions from AC and LF. All authors edited the manuscript.
Funding
This work was supported by the National Science Foundation grants IOS 1354291 and IOS 1953393 to JT. LF also acknowledges support from a National Science Foundation Graduate Research Fellowship.
Conflict of Interest
The authors declare that the research was conducted in the absence of any commercial or financial relationships that could be construed as a potential conflict of interest.
Publisher’s Note
All claims expressed in this article are solely those of the authors and do not necessarily represent those of their affiliated organizations, or those of the publisher, the editors and the reviewers. Any product that may be evaluated in this article, or claim that may be made by its manufacturer, is not guaranteed or endorsed by the publisher.
Acknowledgments
We gratefully acknowledge the comments of reviewers in revising the manuscript.
Supplementary Material
The Supplementary Material for this article can be found online at: https://www.frontiersin.org/articles/10.3389/fevo.2021.742639/full#supplementary-material
Footnotes
- ^ From Big Picture Science podcast, Collective “Knowledge.” May 17, 2021.
References
Aiello, L. C., and Wheeler, P. (1995). The expensive-tissue hypothesis: the brain and the digestive system in human and primate evolution. Curr. Anthro. 36, 199–221. doi: 10.1086/204350
Almaatouq, A., Noriega-Campero, A., Alotaibi, A., Krafft, P. M., Moussaid, M., and Pentland, A. (2020). Adaptive social networks promote the wisdom of crowds. Proc. Natl. Acad. Sci. U S A. 117, 11379–11386. doi: 10.1073/pnas.1917687117
Amador-Vargas, S., Gronenberg, W., Wcislo, W. T., and Mueller, U. (2015). Specialization and group size: brain and behavioural correlates of colony size in ants lacking morphological castes. Proc. R. Soc. B 282:20142502. doi: 10.1098/rspb.2014.2502
Anderson, C., and McShea, D. W. (2001). Individual versus social complexity, with particular reference to ant colonies. Biol. Rev. 76, 211–237. doi: 10.1017/s1464793101005656
Apicella, C. L., Marlowe, F. W., Fowler, J. H., and Christakis, N. A. (2012). Social networks and cooperation in hunter-gatherers. Nature 481, 497–501. doi: 10.1038/nature10736
Armelagos, G. J., Goodman, A. H., and Jacobs, K. H. (1991). The origins of agriculture: population growth during a period of declining health. Popul. Environ. 13, 9–22. doi: 10.1007/bf01256568
Avarguès-Weber, A., d’Amaro, D., Metzler, M., Finke, V., Baracchi, D., and Dyer, A. G. (2018). Does holistic processing require a large brain? insights from honeybees and wasps in fine visual recognition tasks. Front. Psychol. 9:1313. doi: 10.3389/fpsyg.2018.01313
Avarguès-Weber, A., and Giurfa, M. (2013). Conceptual learning by miniature brains. Proc. R. Soc. B: Biol. Sci. 280:20131907. doi: 10.1098/rspb.2013.1907
Bailey, D. H., and Geary, D. C. (2009). Hominid brain evolution. testing climatic, ecological, and social competition models. Hum. Nat. 20, 67–79. doi: 10.1007/s12110-008-9054-0
Bak-Coleman, J. B., Alfano, M., Barfuss, W., Bergstrom, C. T., Centeno, M. A., Couzin, I. D., et al. (2021). Stewardship of global collective behavior. Proc. Natl. Acad. Sci. U.S.A. 118:e2025764118. doi: 10.1073/pnas.2025764118
Balcarcel, A. M., Veitschegger, K., Clauss, M., and Sánchez-Villagra, M. R. (2021). Intensive human contact correlates with smaller brains: differential brain size reduction in cattle types. Proc. R. Soc. Lond. B 288:20210813. doi: 10.1098/rspb.2021.0813
Barron, A. B., and Klein, C. (2016). What insects can tell us about the origins of consciousness. Proc. Natl. Acad. Sci. U.S.A. 113, 4900–4908. doi: 10.1073/pnas.1520084113
Bates, T. C., and Gupta, S. (2017). Smart groups of smart people: evidence for IQ as the origin of collective intelligence in the performance of human groups. Intelligence 60, 46–56. doi: 10.1016/j.intell.2016.11.004
Beals, K. L., Smith, C. L., and Dodd, S. M. (1984). Brain size, cranial morphology, climate and time machines. Curr. Anthropol. 25, 301–330. doi: 10.1086/203138
Bednarik, R. G. (2014). Doing with less: hominin brain atrophy. Homo J. Comp. Hum. Biol. 65, 433–449. doi: 10.1016/j.jchb.2014.06.001
Berger, L. R., Hawks, J., de Ruiter, D. J., Churchill, S. E., Schmid, P., Delezene, L., et al. (2015). Homo naledi, a new species of the genus Homo from the Dinaledi chamber. South Africa. eLife 4:e09560.
Betzig, L. (2014). Eusociality: from the first foragers to the first states. introduction to the special issue. Hum. Nat. 25, 1–5. doi: 10.1007/s12110-013-9187-7
Bickart, K. C., Wright, C. I., Dautoff, R. J., Dickerson, B. C., and Barrett, L. F. (2011). Amygdala volume and social network size in humans. Nat. Neurosci. 14, 163–164. doi: 10.1038/nn.2724
Biro, D., Sasaki, T., and Portugal, S. J. (2016). Bringing a time-depth perspective to collective animal behaviour. Trends Ecol. Evol. 31, 550–562. doi: 10.1016/j.tree.2016.03.018
Boomsma, J. J., and Franks, N. R. (2006). Social insects: from selfish genes to self organisation and beyond. Trends Ecol. Evol. 21, 303–308. doi: 10.1016/j.tree.2006.04.001
Braun, D. R., Harris, J. W., Levin, N. E., McCoy, J. T., Herries, A. I., Bamford, M. K., et al. (2010). Early hominin diet included diverse terrestrial and aquatic animals 1.95 Ma in East Turkana Kenya. Proc. Natl. Acad. Sci. U.S.A. 107, 10002–10007. doi: 10.1073/pnas.1002181107
Browe, B. M., Vice, E. N., and Park, T. J. (2020). Naked mole-rats: blind, naked, and feeling no pain. Anat. Rec. 303, 77–88. doi: 10.1002/ar.23996
Brown, P., Sutikna, T., Morwood, M. J., Soejono, R. P., Saptomo, E. W., and Due, R. A. (2004). A new small-bodied hominin from the Late Pleistocene of Flores, Indonesia. Nature 431, 1055–1061. doi: 10.1038/nature02999
Bruner, E., and Gleeson, B. T. (2019). Body cognition and self-domestication in human evolution. Front. Psychol. 10:1111. doi: 10.3389/fpsyg.2019.01111
Campbell, D. T. (1983). “The two distinct routes beyond kin selection to ultrasociality: implications for the humanities and social sciences,” in The Nature of Prosocial Development: Theories and Strategies, ed. D. L. Bridgeman (Academic press), 11–41.
Chittka, L., and Niven, J. (2009). Are bigger brains better? Curr. Biol. 19, R995–R1008. doi: 10.1016/j.cub.2009.08.023
Coqueugniot, H., and Hublin, J. J. (2012). Age-related changes of digital endocranial volume during human ontogeny: results from an osteological reference collection. Am. J. Phys. Anthropol. 147, 312–318. doi: 10.1002/ajpa.21655
Coto, Z. N., and Traniello, J. F. A. (2021). Brain size, metabolism, and social evolution. Front. Physiol. 12:612865. doi: 10.3389/fphys.2021.612865
Couzin, I. D. (2009). Collective cognition in animal groups. Trends Cogn. Sci. 13, 36–43. doi: 10.1016/j.tics.2008.10.002
Crabtree, G. R. (2013a). Our fragile intellect. Part I. Trends Genet. 29, 1–3. doi: 10.1016/j.tig.2012.10.002
Crabtree, G. R. (2013b). Our fragile intellect. Part II. Trends Genet. 29, 3–5. doi: 10.1016/j.tig.2012.10.003
Davidson, J. D., Arauco-Aliaga, R. P., Crow, S., Gordon, D. M., and Goldman, M. S. (2016). Effect of interactions between harvester ants on forager decisions. Front. Ecol. Evol. 4:115. doi: 10.3389/fevo.2016.00115
DeCasien, A. R., and Higham, J. P. (2019). Primate mosaic brain evolution reflects selection on sensory and cognitive specialization. Nat. Ecol. Evol. 3, 1483–1493. doi: 10.1038/s41559-019-0969-0
DeCasien, A. R., Williams, S. A., and Higham, J. P. (2017). Primate brain size is predicted by diet but not sociality. Nat. Ecol. Evol. 1:0122.
Delgado, J., and Solé, R. V. (1997). Collective-induced computation. Phys. Rev. E 55:2338. doi: 10.1103/physreve.55.2338
DeSilva, J. M. (2011). A shift toward birthing relatively large infants early in human evolution. Proc. Natl. Acad. Sci. U.S.A. 108, 1022–1027. doi: 10.1073/pnas.1003865108
D’Ettorre, P. (2017b). “Invasive eusocieties: commonalities between ants and humans,” in Human Dispersal and Species Movement: from Prehistory to the Present, eds N. Boivin, R. Crassard, and M. Petraglia (Cambridge: Cambridge University Press).
D’Ettorre, P. (2017a). “Distributed agency in ants,” in Distributed Agency, eds N. J. Enfield and P. Kockelman (Oxford, NY: Oxford University Press).
D’Ettorre, P., Meunier, P., Simonelli, P., and Call, J. (2021). Quantitative cognition in carpenter ants. Behav. Ecol. Sociobiol. 75:86.
Dunbar, R. I. M., and Shultz, S. (2017). Why are there so many explanations for primate brain evolution? Proc. R. Soc. Lond. B 372:20160244. doi: 10.1098/rstb.2016.0244
Elek, M. I., Roelandt, G., and D’Ettore, P. (2020). A small number of workers with specific personality traits perform tool use in ants. eLife 9:e61298. doi: 10.7554/eLife.61298
Eshed, V., Gopher, A., Pinhasi, R., and Hershkovitz, I. (2010). Paleopathology and the origin of agriculture in the Levant. Am. J. Phys. Anthropol. 143, 121–133. doi: 10.1002/ajpa.21301
Farris, S. M. (2016). Insect societies and the social brain. Curr. Opin. Insect. Sci. 15, 1–8. doi: 10.1016/j.cois.2016.01.010
Farris, S. M., and Schulmeister, S. (2011). Parasitoidism, not sociality, is associated with the evolution of elaborate mushroom bodies in the brains of hymenopteran insects. Proc. R. Soc. Lond. B 278, 940–951. doi: 10.1098/rspb.2010.2161
Feinerman, O., and Traniello, J. F. A. (2016). Social complexity, diet, and brain evolution: modeling the effects of colony size, worker size, brain size, and foraging behavior on colony fitness in ants. Behav. Ecol. Sociobiol. 69, 1–12.
Foley, R., and Gamble, C. (2009). The ecology of social transitions in human evolution. Philos. Trans. R. Soc. B Biol. Sci. 364, 3267–3279. doi: 10.1098/rstb.2009.0136
Foster, K. R., and Ratnieks, F. L. (2005). A new eusocial vertebrate? Trends Ecol. Evol. 20, 363–364. doi: 10.1016/j.tree.2005.05.005
Friedman, D. A., and Søvik, E. (2021). The ant colony as a test for scientific theories of consciousness. Synthese 198, 1457–1480. doi: 10.1007/s11229-019-02130-y
Godfrey, R. K., and Gronenberg, W. (2019). Brain evolution in social insects: advocating for the comparative approach. J. Comp. Physiol. A 205, 13–32. doi: 10.1007/s00359-019-01315-7
Godfrey, R. K., Swartzlander, M., and Gronenberg, W. (2021). Allometric analysis of brain cell number in Hymenoptera suggests ant brains diverge from general trends. Proc. R. Soc. B 288:20210199. doi: 10.1098/rspb.2021.0199
Gokcumen, O. (2020). Archaic hominin introgression into modern human genomes. Am. J. Phys. Anthropol. 171, 60–73. doi: 10.1002/ajpa.23951
Goldewijk, K. K., Beusen, A., Van Drecht, G., and De Vos, M. (2011). The HYDE 3.1 spatially explicit database of human-induced global land-use change over the past 12,000 years. Global Ecol. Biogeography 20, 73–86. doi: 10.1111/j.1466-8238.2010.00587.x
González-Forero, M., and Gardner, A. (2018). Inference of ecological and social drivers of human brain-size evolution. Nature 557, 554–557. doi: 10.1038/s41586-018-0127-x
Gordon, D. G., Ilieş, I., and Traniello, J. F. A. (2017). Behavior, brain, and morphology in a complex insect society: trait integration and social evolution in the exceptionally polymorphic ant Pheidole rhea. Behav. Ecol. Sociobiol. 71, 1–13. doi: 10.1007/s00265-017-2396-z
Gordon, D. G., and Traniello, J. F. A. (2018). Synaptic organization and division of labor in the exceptionally polymorphic ant Pheidole rhea. Neurosci. Lett. 676, 46–50. doi: 10.1371/journal.pone.0213618
Gordon, D. G., Zelaya, A., Arganda-Carreras, I., Arganda, S., and Traniello, J. F. A. (2019). Division of labor and brain evolution in insect societies: neurobiology of extreme specialization in the turtle ant Cephalotes varians. PLoS One 14:e0213618.
Gowdy, J., and Krall, L. (2013). The ultrasocial origin of the Anthropocene. Ecol. Econ. 95, 137–147. doi: 10.1016/j.ecolecon.2013.08.006
Gowdy, J., and Krall, L. (2016). The economic origins of ultrasociality. Behav. Brain Sci. 36, 563–595. doi: 10.1017/S0140525X1500059X
Grabowski, M. (2016). Bigger brains led to bigger bodies? the correlated evolution of human brain and body size. Curr. Anthro. 57, 174–185. doi: 10.1086/685655
Groh, C., Kelber, C., Grübel, K., and Rössler, W. (2014). Density of mushroom body synaptic complexes limits intraspecies brain miniaturization in highly polymorphic leaf-cutting ant workers. Proc. R. Soc. B 281:20140432. doi: 10.1098/rspb.2014.0432
Groh, C., and Rössler, W. (2011). Comparison of microglomerular structures in the mushroom body calyx of neopteran insects. Arthr. Struc.Devel. 40, 358–367. doi: 10.1016/j.asd.2010.12.002
Groves, C. P. (1999). The advantages and disadvantages of being domesticated. Perspect. Hum. Biol. 4, 1–12.
Hare, B., and Tomasello, M. (2005). The emotional reactivity hypothesis and cognitive evolution. Trends Cogn. Sci. 9, 464–465. doi: 10.1016/j.tics.2005.08.010
Hare, B., and Woods, V. (2020). Survival of the Friendliest: Understanding our Origins and Rediscovering our Common Humanity. New York, NY: Random House.
Hawks, J. (2011). Selection for smaller brains in Holocene human evolution. arXiv [preprint]. Available online at: https://arxiv.org/abs/1102.5604#:~:text=Background%3A%20Human%20populations%20during%20the,linear%20measurements%20of%20the%20cranium (accessed 2020).
Healy, S. D., and Rowe, C. (2007). A critique of comparative studies of brain size. Proc. R. Soc. B. 274, 453–464. doi: 10.1098/rspb.2006.3748
Healy, S. D., and Rowe, C. (2013). Costs and benefits of evolving a larger brain: doubts over the evidence that large brains lead to better cognition. Anim. Behav. 86, e1–e3. doi: 10.1016/j.anbehav.2013.05.017
Henneberg, M., and Steyn, M. (1993). Trends in cranial capacity and cranial index in subsaharan Africa during the Holocene. Am. J. Human Biol. 5, 473–479. doi: 10.1002/ajhb.1310050411
Herculano-Houzel, S. (2016). The Human Advantage: How Our Brains Became Remarkable. Cambridge, MA: MIT Press.
Herculano-Houzel, S. (2017). Numbers of neurons as biological correlates of cognitive capability. Curr. Opin. Behav. Sci. 16, 1–7. doi: 10.1016/j.cobeha.2017.02.004
Herries, A. I., Martin, J. M., Leece, A. B., Adams, J. W., Boschian, G., Joannes-Boyau, R., et al. (2020). Contemporaneity of Australopithecus, Paranthropus, and early Homo erectus in South Africa. Science 368:eaaw7293. doi: 10.1126/science.aaw7293
Holloway, R. L. (1980). Within-species brain-body weight variability: a reexamination of the Danish data and other primate species. Am. J. Phys. Anthropol. 53, 109–121. doi: 10.1002/ajpa.1330530115
Ilies, I., Muscedere, M. L., and Traniello, J. F. A. (2015). Neuroanatomical and morphological trait clusters in the ant genus Pheidole: evidence for modularity and integration in brain structure. Brain Behav. Evol. 85, 63–76. doi: 10.1159/000370100
Isler, K., and van Schaik, C. P. (2009). The expensive brain: a framework for explaining evolutionary changes in brain size. J. Hum. Evol. 57, 392–400. doi: 10.1016/j.jhevol.2009.04.009
Isler, K., and van Schaik, C. P. (2012). Allomaternal care, life history and brain size evolution in mammals. J. Hum. Evol. 63, 52–63. doi: 10.1016/j.jhevol.2012.03.009
Isler, K., and van Shaik, C. P. (2006). Metabolic costs of brain size evolution. Biol. Lett. 2, 557–560. doi: 10.1098/rsbl.2006.0538
Kalinka, A. T., Kelava, I., and Lewitus, E. (2013). Our robust intellect. Trends Genet. 29, 125–127. doi: 10.1016/j.tig.2013.01.008
Kamhi, J. F., Gronenberg, W. G., Robson, S. K. A., and Traniello, J. F. A. (2016). Social complexity influences brain production and operation costs in ants. Proc. R. Soc. B 283:20161949. doi: 10.1098/rspb.2016.1949
Kamhi, J. F., Ilieş, I., and Traniello, J. F. A. (2019). Social complexity and brain evolution: comparative analysis of modularity and integration in formicine ant brain organization. Brain, Behav. Evol. 93, 4–18. doi: 10.1159/000497267
Kanai, R., Bahrami, B., Roylance, R., and Rees, G. (2012). Online social network size is reflected in human brain structure. Proc. R. Soc. B: Biol. Sci. 279, 1327–1334. doi: 10.1098/rspb.2011.1959
Keifer, J., and Summers, C. H. (2016). Putting the “Biology” back into “Neurobiology”: the strength of diversity in animal model systems for neuroscience research. Front. Syst. Neurosci. 10:69. doi: 10.3389/fnsys.2016.00069
Killick, R., and Eckley, I. A. (2014). changepoint: an R package for changepoint analysis. J. Statistical Software 58, 1–19.
Krause, J., Ruxton, G. D., and Krause, S. (2010). Swarm intelligence in animals and humans. Trends Ecol. Evol. 25, 28–34. doi: 10.1016/j.tree.2009.06.016
Kuzawa, C. W., Chugani, H. T., Grossman, L. I., et al. (2014). Metabolic costs and evolutionary implications of human brain development. Proc. Natl. Acad. Sci. U.S.A. 111, 13010–13015. doi: 10.1073/pnas.1323099111
Kverková, K., Olkowicz, S., Pavelkova, Z., et al. (2018). Sociality does not drive the evolution of large brains in eusocial African mole rats. Sci. Rep. 8:9203. doi: 10.1038/s41598-018-26062-8
Laland, K., and Seed, A. (2021). Understanding human cognitive uniqueness. Ann. Rev. Psychol. 72, 689–716. doi: 10.1146/annurev-psych-062220-051256
Leach, H. (2003). Human domestication reconsidered. Curr. Anthropol. 44, 349–368. doi: 10.1086/368119
Lee, S. H., and Wolpoff, M. H. (2003). The pattern of evolution in Pleistocene human brain size. Paleobiology 29, 186–196. doi: 10.1017/s0094837300018054
Leonard, W. R., Robertson, M. L., Snodgrass, J. J., and Kuzawa, C. W. (2003). Metabolic correlates of hominid brain evolution. Comp. Biochem. Physiol. Part A: Mol. Int. Physiol. 136, 5–15. doi: 10.1016/s1095-6433(03)00132-6
Lepre, C. J., Roche, H., Kent, D. V., Harmand, S., Quinn, R. L., Brugal, J. P., et al. (2011). An earlier origin for the Acheulian. Nature 477, 82–85. doi: 10.1038/nature10372
Lihoreau, M., Dubois, T., Gomez-Moracho, T., and Kraus, S. (2019). Putting the ecology back into insect cognition research. Adv. In Insect Phys. 57, 1–25. doi: 10.1016/bs.aiip.2019.08.002
Lihoreau, M., Latty, T., and Chittka, L. (2012). An exploration of the social brain hypothesis in insects. Front. Physiol. 3:442. doi: 10.3389/fphys.2012.00442
Liu, C., Tang, Y., Ge, H., Wang, F., Sun, H., Meng, H., et al. (2014). Increasing breadth of the frontal lobe but decreasing height of the human brain between two Chinese samples from a Neolithic site and from living humans. Am. J. Phys. Anthropol. 154, 94–103. doi: 10.1002/ajpa.22476
Logan, C. J., Avin, S., Boogert, N., Buskell, A., Cross, F. R., Currie, A., et al. (2018). Beyond brain size: uncovering the neural correlates of behavioral and cognitive specialization. Comp. Cog. Behav. Rev. 13, 55–89. doi: 10.3819/CCBR.2018.130008
Markov, A. V., and Markov, M. A. (2020). Runaway brain-culture coevolution as a reason for larger brains: exploring the “cultural drive” hypothesis by computer modeling. Ecol. Evol. 10, 6059–6077. doi: 10.1002/ece3.6350
Maschwitz, U., Hahn, M., and Schönegge, P. (1979). Paralysis of prey in ponerine ants. Naturwiss 66, 213–214. doi: 10.1007/bf00366035
McHenry, H. M., and Coffing, K. (2000). Australopithecus to Homo: transformations in body and mind. Ann. Rev. Anthropol. 29, 125–146.
Meguerditchian, A., Marie, D., Margiotoudi, K., Roth, M., Nazarian, B., Anton, J. L., et al. (2021). Baboons (Papio anubis) living in larger social groups have bigger brains. Evol. Hum. Behav. 42, 30–34. doi: 10.1016/j.evolhumbehav.2020.06.010
Menneret, A., Nilsen, F., Ebert, D., and Skorping, A. (2010). Intensive farming: evolutionary implications for parasites and pathogens. Evol. Biol. 37, 59–67. doi: 10.1007/s11692-010-9089-0
Miller, I. F., Barton, R. F., and Nunn, C. L. (2019). Quantitative uniqueness of human brain evolution revealed through phylogenetic comparative analysis. eLife 8:e41250.
Milner, G. R. (2019). Early agriculture’s toll on human health. Proc. Natl. Acad. Sci. U.S.A. 28, 13721–13723. doi: 10.1073/pnas.1908960116
Mitchell, K. J. (2013). Genetic entropy and the human intellect. Trends Genet. 29, 59–60. doi: 10.1016/j.tig.2012.11.010
Morand-Ferron, J., and Quinn, J. L. (2011). Larger groups of passerines are more efficient problem solvers in the wild. Proc. Natl. Acad. Sci. U.S.A. 108, 15898–15903. doi: 10.1073/pnas.1111560108
Muggeo, V. M. R. (2008). segmented: an R package to fit regression models with broken-line relationships. R News 8, 20–25.
Muratore, I. B., Fandozzi, E. M., and Traniello, J. F. A. (2021). Behavioral performance requirements for division of labor influence adaptive brain mosaicism in a socially complex ant. BioRxiv [preprint] doi: 10.1101/2021.07.03.450997
Muratore, I. B., and Traniello, J. F. A. (2020). Fungus-growing ants as models for the integrative analysis of cognition and brain evolution. Front. Behav. Neurosci. 14:599234. doi: 10.3389/fnbeh.2020.599234
Muscedere, M. L., Gronenberg, W., Moreau, C. M., and Traniello, J. F. A. (2014). Investment in higher-order central processing regions is not constrained by brain size in social insects. Proc. R. Soc. B. 281:20140217. doi: 10.1098/rspb.2014.0217
Muscedere, M. L., and Traniello, J. F. A. (2012). Division of labor in the hyperdiverse ant genus Pheidole is associated with distinct patterns of worker brain organization. PLoS One 7:e31618. doi: 10.1371/journal.pone.0031618
Muscedere, M. L., Willey, T. A., and Traniello, J. F. A. (2009). Age and task efficiency in the ant Pheidole dentata: young minor workers are not specialist nurses. Anim. Behav. 77, 911–918. doi: 10.1016/j.anbehav.2008.12.018
Navarrete, A., Van Schaik, C. P., and Isler, K. (2011). Energetics and the evolution of human brain size. Nature 480, 91–93. doi: 10.1038/nature10629
Noonan, M. P., Mars, R. B., Sallet, J., Dunbar, R. I. M., and Fellows, L. K. (2018). The structural and functional brain networks that support human social networks. Behav. Brain Res. 355, 12–23. doi: 10.1016/j.bbr.2018.02.019
O’Donnell, S., Bulova, S. J., DeLeon, S., Khodak, P., Miller, S., and Sulger, E. (2015). Distributed cognition and social brains: reductions in mushroom body investment accompanied the origins of sociality in wasps (Hymenoptera: Vespidae). Proc. R. Soc. B. 282:20150791. doi: 10.1098/rspb.2015.0791
Pagán, O. R. (2019). The brain: a concept in flux. Philos. Trans. R. Soc. B 374:20180383. doi: 10.1098/rstb.2018.0383
Page, A. E., Viguier, S., Dyble, M., Smith, D., Chaudhary, N., Salali, G. D., et al. (2016). Reproductive trade-offs in extant hunter-gatherers suggest adaptive mechanism for the Neolithic expansion. Proc. Natl. Acad. Sci. U.S.A. 113, 4694–4699. doi: 10.1073/pnas.1524031113
Perri, A. R., Feuerborn, T. R., Frantz, L. A., Larson, G., Malhi, R. S., Meltzer, D. J., et al. (2021). Dog domestication and the dual dispersal of people and dogs into the Americas. Proc. Natl. Acad. Sci. U.S.A. 118:e2010083118. doi: 10.1073/pnas.2010083118
Perry, C. J., Barron, A. B., and Chittka, L. (2017). The frontiers of insect cognition. Curr. Opin. Behav. Sci. 16, 111–118. doi: 10.1016/j.cobeha.2017.05.011
Perry, C. J., and Chittka, L. (2019). How foresight might support the behavioral flexibility of arthropods. Curr. Opin. Neurobiol. 54, 171–177. doi: 10.1016/j.conb.2018.10.014
Piñero, J., and Solé, R. (2019). Statistical physics of liquid brains. Philos. Trans. R. Soc. B 374:20180376. doi: 10.1098/rstb.2018.0376
Ponce de León, M. S., Bienvenu, T., Marom, A., Engel, S., Tafforeau, P., et al. (2021). The primitive brain of early Homo. Science 372, 165–171. doi: 10.1126/science.aaz0032
Ponce de León, M. S., Golovanova, L., Doronichev, V., Romanova, G., Akazawa, T., et al. (2008). Neanderthal brain size at birth provides insights into the evolution of human life history. Proc. Natl. Acad. Sci. U.S.A. 105, 13764–13768. doi: 10.1073/pnas.0803917105
Pontzer, H., Brown, M. H., Raichlen, D. A., Dunsworth, H., et al. (2016). Metabolic acceleration and the evolution of human brain size and life history. Nature 533, 390–392. doi: 10.1038/nature17654
Reid, C. R., Lutz, M. J., Powell, S., Kao, A. B., Couzin, I. D., and Garnier, S. (2015). Army ants dynamically adjust living bridges in response to a cost–benefit trade-off. Proc. Natl. Acad. Sci. U.S.A. 112, 15113–15118. doi: 10.1073/pnas.1512241112
Reséndiz-Benhumea, G. M., Sangati, E., Sangati, F., Keshmiri, S., and Froese, T. (2021). Shrunken social brains? a minimal model of the role of social interaction in neural complexity. Front. Neurorobot. 15:634085. doi: 10.3389/fnbot.2021.634085
Rightmire, G. P. (2004). Brain size and encephalization in early to mid-pleistocene homo. Am. J. Phys. Anthropol. 124, 109–123. doi: 10.1002/ajpa.10346
Riveros, A. J., Seid, M. A., and Wcislo, W. T. (2012). Evolution of brain size in class-based societies of fungus-growing ants (Attini). Anim. Behav. 83, 1043–1049. doi: 10.1016/j.anbehav.2012.01.032
Ruff, C. B., Trinkaus, E., and Holliday, T. W. (1997). Body mass and encephalization in Pleistocene Homo. Nature 387, 173–176. doi: 10.1038/387173a0
Sasaki, T., and Biro, D. (2017). Cumulative culture can emerge from collective intelligence in animal groups. Nat. Comm. 8:15049.
Sasaki, T., and Pratt, S. C. (2018). The psychology of superorganisms: collective decision making by insect societies. Ann. Rev. Entomol. 63, 259–275. doi: 10.1146/annurev-ento-020117-043249
Schwidetzky, I. (1976). Postpleistocene evolution of the brain? Am. J. Phys. Anthropol. 45, 605–611. doi: 10.1002/ajpa.1330450327
Seid, M. A., Castillo, A., and Wcislo, W. (2011). The allometry of brain miniaturization in ants. Brain Behav. Evol. 77, 5–13. doi: 10.1159/000322530
Seid, M. A., Harris, K. M., and Traniello, J. F. A. (2005). Age-related changes in the number and structure of synapses in the lip region of the mushroom bodies in the ant Pheidole dentata. J. Comp. Neurol. 488, 269–277. doi: 10.1002/cne.20545
Seid, M. A., and Traniello, J. F. A. (2006). Age-related repertoire expansion and division of labor in Pheidole dentata (Hymenoptera: Formicidae): a new perspective on temporal polyethism and behavioral plasticity in ants. Behav. Ecol. Sociobiol. 60, 631–644. doi: 10.1007/s00265-006-0207-z
Shigeno, S. (2017). “Brain evolution by design: from neural origin to cognitive architecture,” in Diversity and Commonality in Animals, eds S. Shigeno, Y. Murakami, and T. Nomura (Tokyo: Springer), doi: 10.1007/978-4-431-56469-0_19
Strausfeld, N. J., Hansen, L., Li, Y., Gomez, R. S., and Ito, K. (1998). Evolution, discovery, and interpretations of arthropod mushroom bodies. Learn. Memory 5, 11–37.
Striedter, G. F., Belgard, T. G., Chen, C., et al. (2014). NSF workshop report: discovering general principles of nervous system organization by comparing brain maps across species. J. Comp. Neurol. 522, 1445–1453. doi: 10.1002/cne.23568
Tobias, P. V. (1970). Brain-size, grey matter and race—fact or fiction? Am. J. Phys. Anthropol. 32, 3–25. doi: 10.1002/ajpa.1330320103
Tomer, R., Denes, A. S., Tessmar-Raible, K., and Arendt, D. (2010). Profiling by image registration reveals common origin of annelid mushroom bodies and vertebrate pallium. Cell 142, 800–809. doi: 10.1016/j.cell.2010.07.043
van Schaik, C. P., Isler, K., and Burkart, J. M. (2012). Explaining brain size variation: from social to cultural brain. Trends Cogn. Sci. 16, 277–284. doi: 10.1016/j.tics.2012.04.004
VanSickle, C., Cofran, Z., and Hunt, D. (2020). Did Neandertals have large brains? factors affecting endocranial volume comparisons. Am. J. Phys. Anthropol. 173, 768–775. doi: 10.1002/ajpa.24124
Ward, A. J., Herbert-Read, J. E., Sumpter, D. J., and Krause, J. (2011). Fast and accurate decisions through collective vigilance in fish shoals. Proc. Natl. Acad. Sci. U.S.A. 108, 2312–2315. doi: 10.1073/pnas.1007102108
Weaverdyck, M. E., and Parkinson, C. (2018). The neural representation of social networks. Curr. Opin. Psych. 24, 58–66.
Wehner, R., Fukushi, T., and Isler, K. (2007). On being small: brain allometry in ants. Brain Behav. Evol. 69, 220–228. doi: 10.1159/000097057
Wells, J. C. K., and Stock, J. T. (2020). Life history transitions at the origins of agriculture: a model for understanding how niche construction impacts human growth, demography and health. Front. Endocrinol. 11:325. doi: 10.3389/fendo.2020.00325
Wiercinski, A. (1979). Has the brain size decreased since the upper paleolithic period? Bull. Mem. Soc. Anthropol. Paris 6, 419–427. doi: 10.3406/bmsap.1979.1979
Wolpoff, M. H. (1999). Paleoanthropology. New York, NY: McGraw-Hill Humanities, Social Sciences & World Languages.
Wood, B., and Collard, M. (1999). The human genus. Science 284, 65–71. doi: 10.1126/science.284.5411.65
Woolley, A. W., Chabris, C. F., Pentland, A., Hashmi, N., and Malone, T. W. (2010). Evidence for a collective intelligence factor in the performance of human groups. Science 330, 686–688. doi: 10.1126/science.1193147
Wrangham, R. (2019). The Goodness Paradox: The Strange Relationship between Virtue and Violence in Human Evolution. New York, NY: Vintage.
Wrangham, R. W. (2018). Two types of aggression in human evolution. Proc. Natl. Acad. Sci. U.S.A. 115, 245–253. doi: 10.1073/pnas.1713611115
Keywords: brain, evolution, ant, hominin, Holocene
Citation: DeSilva JM, Traniello JFA, Claxton AG and Fannin LD (2021) When and Why Did Human Brains Decrease in Size? A New Change-Point Analysis and Insights From Brain Evolution in Ants. Front. Ecol. Evol. 9:742639. doi: 10.3389/fevo.2021.742639
Received: 16 July 2021; Accepted: 23 September 2021;
Published: 22 October 2021.
Edited by:
Mathieu Lihoreau, Centre National de la Recherche Scientifique (CNRS), FranceReviewed by:
Robin Dunbar, University of Oxford, United KingdomKazuki Tsuji, University of the Ryukyus, Japan
Copyright © 2021 DeSilva, Traniello, Claxton and Fannin. This is an open-access article distributed under the terms of the Creative Commons Attribution License (CC BY). The use, distribution or reproduction in other forums is permitted, provided the original author(s) and the copyright owner(s) are credited and that the original publication in this journal is cited, in accordance with accepted academic practice. No use, distribution or reproduction is permitted which does not comply with these terms.
*Correspondence: Jeremy M. DeSilva, amVyZW15Lm0uZGVzaWx2YUBkYXJ0bW91dGguZWR1
†These authors have contributed equally to this work