- 1Facultad de Ciencias, Instituto de Ciencias Ambientales y Evolutivas, Universidad Austral de Chile, Valdivia, Chile
- 2Departamento de Ciencias, Facultad de Artes Liberales, Universidad Adolfo Ibáñez, Santiago, Chile
- 3Instituto Milenio de Socio-Ecología Costera “SECOS”, Santiago, Chile
Environmental variability in coastal oceans associated with upwelling dynamics probably is one of the most pervasive forces affecting the physiological performance of marine life. As the environmental temperature is the abiotic factor with major incidence in the physiology and ecology of marine ectotherms, the abrupt temperature changes in upwelling systems could generate important variations in these organisms’ functional processes. The relationship between ambient temperature and physiological performance can be described through a thermal performance curve (TPC). The parameters of this curve usually show geographic variation usually is in accordance with the predictions of the climate variability hypothesis (CVH), which states that organisms inhabiting more variable environments should have broader ranges of environmental tolerance in order to cope with the fluctuating environmental conditions they experience. Here we study the effect generated by the environmental variability in an active upwelling zone on the physiological performance of the marine ectotherm Achanthopleura echinata. In particular, we compared the parameters of the TPC and the metabolic rate of two populations of A. echinata, one found in high semi-permanent upwelling (Talcaruca), while the other is situated in an adjacent area with seasonal upwelling (Los Molles) and therefore more stable environmental conditions. Our results show that: (1) oxygen consumption increases with body size and this effect is more significant in individuals from the Talcaruca population, (2) optimal temperature, thermal breadth, upper critical limit and maximum performance were higher in the population located in the area of high environmental heterogeneity and (3) individuals from Talcaruca showed greater variance in optimal temperature, thermal breadth, upper critical limit but not in maximum performance. Although it is clear that a variable environment affects the thermal physiology of organisms, expanding their tolerance ranges and generating energy costs in the performance of individuals, it is relevant to note that upwelling systems are multifactorial phenomena where the rise of water masses modifies not only temperature, but also decreases O2, pH, and increases pCO2 which in turn could modify metabolism and TPC.
Introduction
Environmental variability in coastal oceans associated with upwelling dynamics probably is one of the most pervasive forces affecting the physiological performance of marine life (Gaitán-Espitia et al., 2017; Doney et al., 2020). Coastal upwelling originates from large-scale processes that couple atmospheric and oceanographic features (Hill et al., 1998) and its waters are characterized by a high primary productivity, cold temperatures, low pH, low O2 and high CO2 concentrations (Kapsenberg and Hofmann, 2016; Ramajo et al., 2020). Therefore, upwelling systems are characterized to be highly variable and unpredictable for intertidal and sub-tidal ectotherms in both, short time and large spatial scales, in several environmental conditions such as temperature, pH and oxygen availability (Torres et al., 2011; Aravena et al., 2014; Vargas et al., 2017; Ramajo et al., 2020; Lardies et al., 2021). These variations in the physicochemical characteristics of water have a profound impact in the physiological capacities of marine fauna (Duarte et al., 2018; Ramajo et al., 2020). This is particularly true for the broad daily (see Osores et al., 2018) and seasonal variations in temperature, which can be as extensive as their cold/heat tolerance limits (Helmuth et al., 2006; Somero, 2010). Furthermore, as the environmental temperature is the abiotic factor with major incidence in the physiology and ecology of marine ectotherms (Pörtner, 2001), the abrupt temperature changes in upwelling systems could generate important variations in these organisms’ functional process (e.g., growth, reproduction, physiology) (Lardies et al., 2011; Barria et al., 2014, 2018; Broitman et al., 2021).
The relationship between ambient temperature and physiological performance can be described through a thermal performance curve (TPC) (Huey et al., 2001). This curve is usually characterized by four parameters: the minimum and maximum critical temperatures (CTmin; CTmax), which are, respectively, the minimum and maximum environmental temperatures at which performance is zero; and the optimal temperature (Topt), which is the temperature at which the performance is maximized (Pmax) (Barria and Bacigalupe, 2017). The TPC has been used to describe mechanistically the thermal sensitivity among populations of ectothermic species (Kingsolver et al., 2004; Barria et al., 2018; Broitman et al., 2018). In addition, it is well known that the parameters of the TPC usually show geographic variation depending on the local environment and genetic background of populations (Gilchrist, 1996; Kingsolver et al., 2004; Gaitán-Espitia et al., 2014, 2017; Barria et al., 2018; Broitman et al., 2021). This geographic variation usually is in accordance with the predictions of the climate variability hypothesis (CVH), which states that organisms inhabiting more variable environments (i.e., high altitudes or latitudes) should have broader ranges of environmental performance in order to cope with the fluctuating environmental conditions they experience (e.g., Gaitán-Espitia et al., 2017). However, living in highly variable environments is not without costs (Van Buskirk and Steiner, 2009; Murren et al., 2015). The metabolic rate of an organism is linked to its energy use and as such reflects the energetic cost of adaptation to a particular thermal environment (Gillooly et al., 2001; Lardies et al., 2011). In this context, and under the basis of CVH, it would be expected that populations living in places exposed to abrupt temperature fluctuations show higher energy costs (Barria and Bacigalupe, 2017; Broitman et al., 2018; Lardies et al., 2021).
The north-central coast of Chile (20–35°S) along the western edge of South America exhibits typical structures of an upwelling system (Rahn et al., 2011). The climate is dominated by the Pacific-South anticyclone that favors the semi-arid zones and the prevalence of south winds during most of the year, promoting the upwelling of cold waters along the coast, which are equally rich in nutrients, supersaturated in CO2, low in O2, and pH (Ramajo et al., 2020; Lardies et al., 2021). In particular, Punta Lengua de Vaca (30°S; front Talcaruca) is recognized as one of the most active upwelling centers of the region (Figueroa and Moffat, 2000; Torres et al., 2011; Rahn et al., 2015), which undoubtedly generates an extreme scenario for the organisms inhabiting there. These different patterns of upwelling produce strong differences in the variability of sea surface temperature, total alkalinity, carbonate and the partial pressure of CO2 among other factors (see Table 1). Hence, here we study the effect generated by the environmental variability in an active upwelling zone on the physiological performance of the marine ectotherm Achanthopleura echinata (Barnes, 1824). This polyplacophore is distributed from Punta Filomena (North of Perú, 9°16′S) to Punta Parra (South of Chile, 36°40′S) (Tobar-Villa and Ibáñez, 2013) and is one of the most common mollusks of the coastal benthic fauna. Despite this, there are extremely few studies on this species (Camus et al., 2012). Therefore, we compared the parameters of the TPC and the metabolic rate of two populations of Achanthopleura echinata, one found in high semi-permanent upwelling (Talcaruca), while the other is situated in an adjacent area with seasonal upwelling and therefore more stable environmental conditions (Los Molles, 32°24′S).
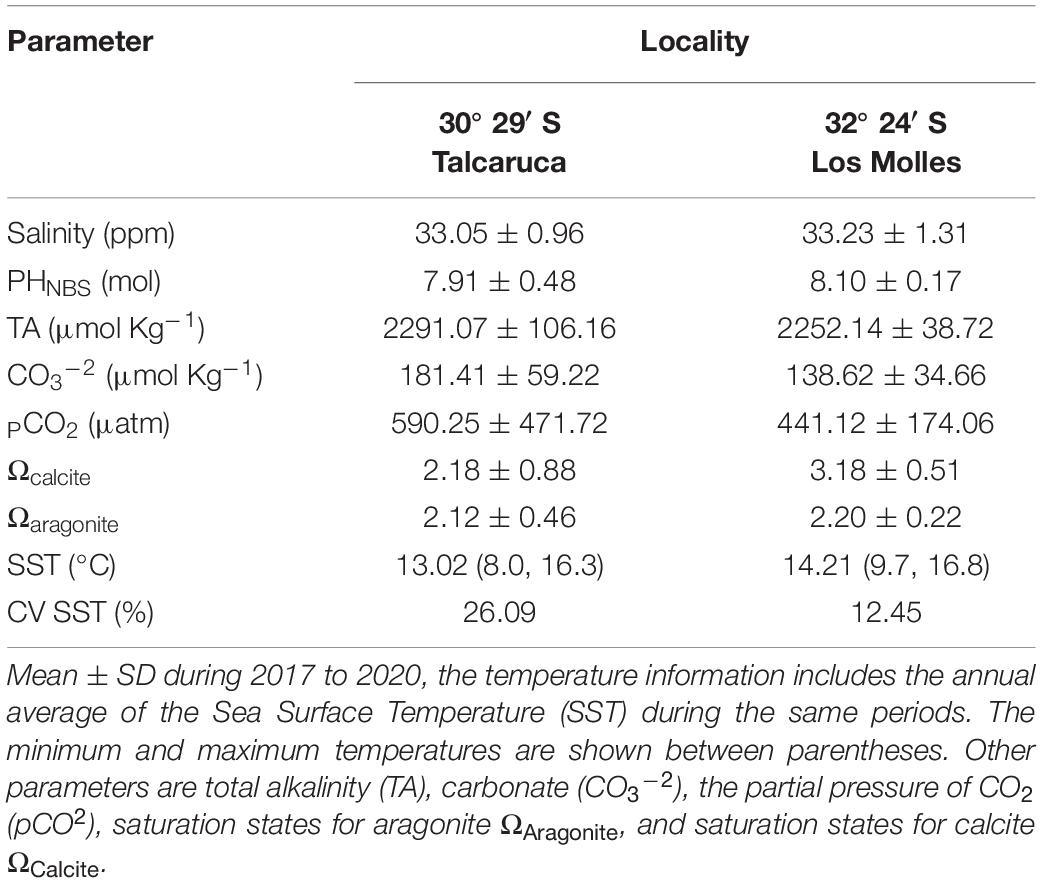
Table 1. Summary of salinity and seawater carbonate chemistry variables measured in two study locations.
Materials and Methods
Study Sites
Fifty-two individuals of Acanthopleura echinata were randomly collected by hand during the austral spring in low intertidal rocky shores from Talcaruca (30°29′S, 71°41′W; N = 32) and Los Molles (32°24′S, 71°50′W; N = 20). Animals were transported during 2–3 days in refrigerated containers to the laboratory at Adolfo Ibañez University in Santiago. These sites are characterized by contrasting variability in upwelling dynamics and in the physical-chemical characteristics of their coastal waters (Table 1). While Talcaruca demarcates the southern border of the Peruvian province (Rivadeneira and Fernández, 2005; Barria et al., 2014), and is characterized by a strong, continuous upwelling, supersatured with dissolved CO2 and low pH levels in near-shore areas (Torres et al., 2011) Los Molles (32°S) is exposed to seasonal, fluctuating upwelling events (see Vargas et al., 2017; Broitman et al., 2018) in the transitional zone. Ocean temperature, salinity, and seawater carbonate system parameters were measured directly in the intertidal zone (see below and Table 1). For the temperature estimation, submersible temperature data loggers (HOBOTM, Onset Computer Corp., MA, United States) were installed, housed inside PVC pipes embedded in concrete blocks, and deployed at –1 m below the surface (see Broitman et al., 2018 for details). The loggers recorded the sea surface temperature data every 30 min, which was downloaded on a monthly schedule; see details Lardies et al. (2021). Data for local temperature climatology (see Table 1) are long-term daily averages, and in both sites have more than 2 years of high frequency data. Using the same methodology and frequency described above, we anchored conductivity dataloggers (HOBO U24-001 Conductivity Data LoggerTM, ONSET Computer Corp., MA, United States) to determine salinity in the intertidal zone (∼0.5 m below the seawater surface at the mid-intertidal). The pH in the field was measured biweekly for 2019 and 2020 using a Metrohm 713 Meter (MetrohmTM) connected to a combined electrode (Metrohm model 6.0219.100) previously calibrated with the Metrohm pH 4 (6.2307.200), pH 7 (6.2307.210), and pH 9 (6.2307.220) standard buffers. The pH values are reported on the NBS scale. Furthermore, seawater samples were taken with a biweekly frequency (for 2019 and 2020) to measure Total Alkalinity (TA). Given this information, we proceed to estimate carbonate system parameters (see Ramajo et al., 2020 for details of estimation of carbonate parameters) in both localities. Samples for Total Alkalinity (TA) were poisoned using HgCl2 50 μL, and the bottles (PyrexTM, Corning) were sealed using parafilm. Then, TA was determined using the open-cell titration method (Dickson et al., 2007) using an automatic alkalinity titrator (Model AS-ALK2, Apollo SciTech) equipped with a combination pH electrode (8102BNUWP, Thermo Fisher Scientific) and temperature probe (Star ATC, Thermo Fisher Scientific) connected to a pH meter (Orion Star A211, Thermo Fisher Scientific). All samples were analyzed at 25°C (± 0.1°C), and the temperature was regulated using a water bath (Lab Companion CW-05G). Accuracy was controlled using certified reference material (CRM, supplied by A. Dickson, University California San Diego), and the TA repeatability was 2–3 μmol kg–1 on average.
At each study site stones covered Ulva spp., corallines and barnacles were collected and transported to the lab as food for the sampled individuals (see Camus et al., 2012). As A. echinata inhabits the low intertidal (Otaiza and Santelices, 1985) it is not exposed to air for most part of the day, thus animals from both localities were acclimated for 2 weeks at water temperature of 14 ± 1°C (SunSun®) without tidal regime. Nevertheless, aquariums (dimensions 80 × 40 × 40 cm) were covered with just 2/3 of their height in water and rocks to provide individuals the opportunity to be exposed to air if they wanted to. Photoperiod was maintained at 14:L–10:D (i.e., spring condition), pH at 8.1 ± 0.1 and salinity at 33 ± 1 PSU, controlled by a pH-metere (Metrohm model 826) and a salinometer, respectively. Food was provided ad libitum and sea water was constantly aerated (InstantOcean®). Each individual was marked with a numerical and colored bee mark (i.e., beeworks©) on the II plate. Before experiments, each individual was measured (from the anterior to the posterior mantle girdle) using a digital caliper Litz and weighted with an analytical balance (Shimazu, model AUX220).
Oxygen Consumption
The metabolic rate was measured as oxygen consumption using fiber optic oxygen sensors (Precision Sensing, GmbH, Germany) connected to a PreSens Oxy-4 mini® respirometer. Each individual was put inside a glass respirometry chamber of 1 L connected to a sensor and filled up with UV filtered water. All individuals were fasted 24 h at maintenance conditions before the O2 consumption was measured. Temperature was stabilized using a recirculating water bath (BOYU, Model L075). Sensors were previously calibrated with saturated Na2SO3 solution and aerated sea water, to estimate 0 and 100% oxygen, respectively (see Gaitán-Espitia et al., 2014). Sensors measured the oxygen in the water every 15 s for a period of approximately 60 min to obtain several estimates. The first 5 and last 5 min of each measurement were discarded to avoid possible disturbances associated to stress of the animals. The mean value of those 50 min in between was considered as the O2 consumption rate of each individual. The same respirometry chambers were used as controls, but without chitons inside, under the same experimental conditions (the control never had a reduction of the oxygen concentration higher than 3% of measurements).
Thermal Performance Curves
Thermal effects on physiological performance were estimated for 18 individuals from Los Molles and 15 from Talcaruca using an electric heart sensor positioned VII on the plate (heart location) connected to an Oscilloscope (TiePie engineering, model HS4; see Gaitán-Espitia et al., 2017 for methodology) to estimate heart rate (HR, cardiac activity). Animals were immobilized with adhesive tape on a wet plate and introduced into a thermorregulated chamber (JeioTech, model RW-2025). Electric impulses were registered as an electrocardiogram by the program Multi Channel Oscilloscope v1.31.2.0 (TiePie engineering). Measurements of HR were made to each organism at fourteen temperatures: 0, 3, 6, 9, 12, 14, 17, 20, 23, 26, 29, 32, 35, and 37°C. These temperatures are supported by prior pilot experiments on the TPC in A. echinata. All individuals were first tested at the maintenance temperature (i.e., 14°C). After a short acclimation of 30 min to remove any potential effect caused by manipulation, the heart rate was recorded for 10 min, after which the temperature was set to 12°C. After 20 min of adjustment, the heart rate was recorded for 10 min, after which the temperature was decreased to the next value (i.e., 9°C). This procedure was repeated until rate was recorded at 6, 3, and 0°C. After a 2-day recovery period for all individuals, a similar protocol was used to measure HR from 14 to 37°C.
Statistical Analyses
We used a simple linear model to evaluate the effects of population and wet weight on metabolic rate. Both numeric variables were log10 transformed to comply with the assumptions of a normal distribution. Regarding the TPC, for each locality we obtained a mean value and SD at each environmental temperature, which were then used to create 100 null TPC distributions (see Rezende and Bozinovic, 2019 for details). From each run of bootstrapping we obtained the maximum performance (Pmax), the temperature at which performance is maximized (Topt), the upper critical limit (CTmax) and the temperature range in which performance remains above 80% of its maximum (Breath80). We then used a simple linear model to compare the means of these parameters between localities. Nineteen of those 200 null distributions failed to converge (N = 9 in Talcaruca, and N = 10 in Los Molles) which gives a final sample size for analyzing the TPC parameters of 181. Furthermore, the estimation of CTmax was not possible in 15 of those 181 null distributions (N = 12 in Talcaruca and 3 in Los Molles) while the estimation of Tbreadth was not possible for one null distribution in Talcaruca, all of which affected the final error degrees of freedom. All variables were log10 transformed to meet normality assumptions. Finally, we used a Fisher test to compare the variances of the parameters of the TPC between localities. All analyses were carried out in R version 3.6.3 (R Core Team, 2020).
Results
Salinity in both coastal localities was similar, with values around 33.10 ppm. Instead, ocean temperature showed differences with a colder annual media of 13.02°C, an annual maximum of 16.30°C, and a minimum of 8.00°C in Talcaruca (see Table 1). On the other hand, Los Molles has a hotter annual media of 14.21°C, with an annual maximum of 16.8°C and a minimum of 9.70°C. Interestingly, the coefficient of variation of sea surface temperature was higher in Talcaruca with 26.1% compared with only 12.4% in Los Molles (see Table 1). Seawater carbonate chemistry parameters varied notably among studied localities (Table 1). The highest and more variable levels of pCO2 were observed in Talcaruca (590.2 ± 471.7 μmol kg–1), while a pCO2 of 441.1 ± 174.0 (μmol Kg–1) was observed in Los Molles. Los Molles exhibited higher pH values but more minor variation (8.10 ± 0.17) than Talcaruca site, which evidenced a higher variability (7.91 ± 0.48). The saturation states for calcite (Ωcalcite) and aragonite (Ωaragonite) were lower in Talcaruca, with some events of undersaturation, compared with Los Molles (Table 1). TA (Total Alkalinity) presented similar values in both sites (see Table 1).
Although the metabolic rate was positively associated with body mass in both populations, the rate of increase in metabolic rate per unit of change in mass was higher in Talcaruca [Interaction log10(Body mass) × Locality: F(1,47) = 4.408, P = 0.041] (Figure 1). This suggest that the overall metabolic costs increase with body mass in both localities, but those costs are higher for bigger individuals in Talcaruca. Overall, the TPC was taller and wider in the population near the upwelling system (Figure 2). That is, Talcaruca showed higher Pmax [F(1,178) = 9.611, P = 0.002], Topt [F(1,178) = 26.19, P < 0.001], CTmax [F(1,163) = 42.074, P < 0.001] and Tbreadth [F(1,177) = 11.438, P < 0.001] (Figure 2). Additionally, individuals from Talcaruca showed a higher variance in Topt [F(89,89) = 0.400, P < 0.001], CTmax [F(86,77) = 0.594, P = 0.019] and Tbreadth [F(89,88) = 0.486, P < 0.001] but not in Pmax [F(89,89) = 0.866, P = 0.499] (Figure 2).
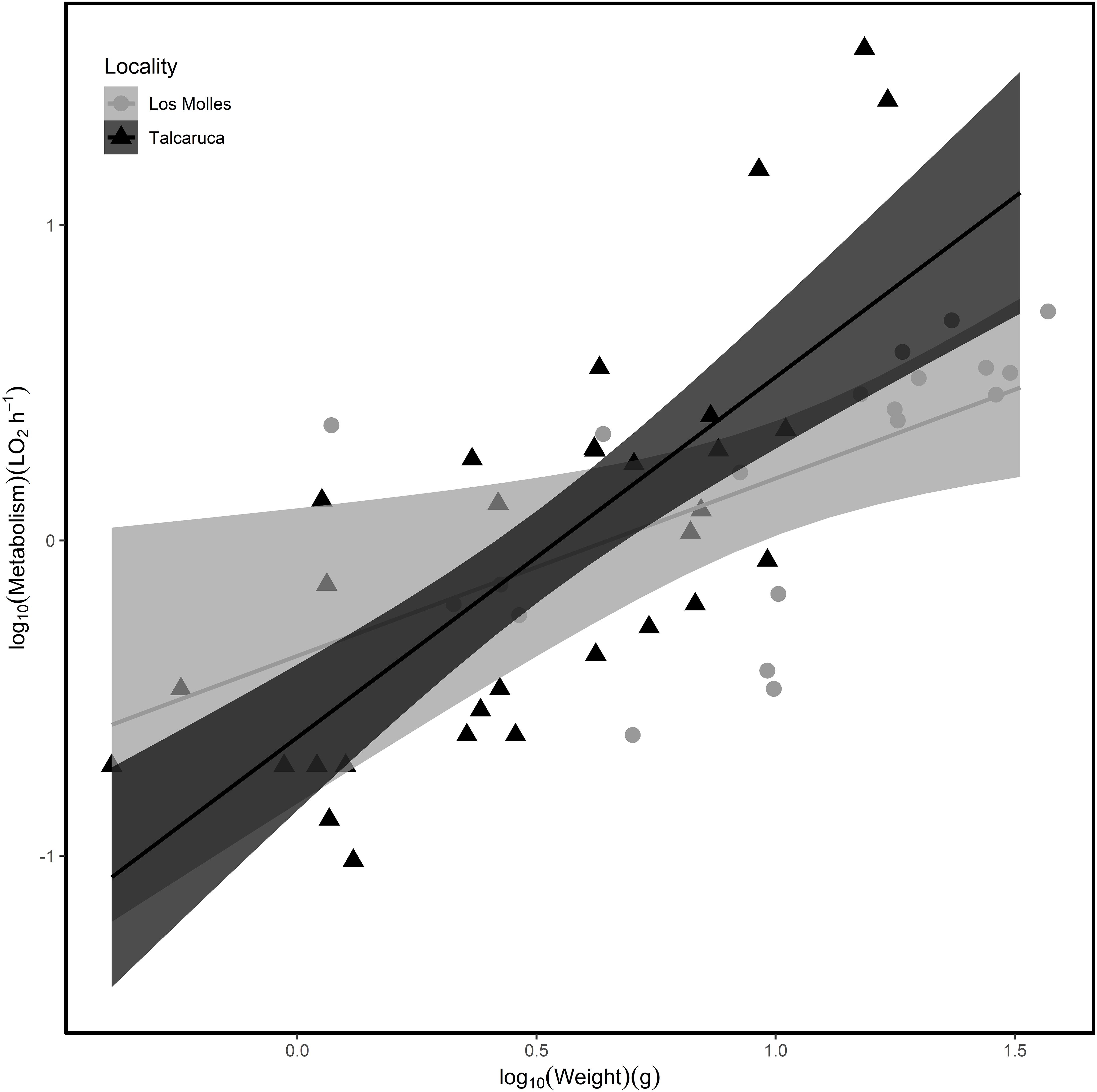
Figure 1. Relationship between weight and metabolism in each population of Acanthopleura echinata. (Circle/black = Los Molles; Triangle/gray = Talcaruca). The shaded area represents 95% confidence intervals. The parameters of the relationship for each locality in a log10–log10 scale are: (i) Talcaruca = –0.624 (intercept) and 1.143 (slope) and (ii) Los Molles = –0.366 (intercept) and 0.523 (slope).
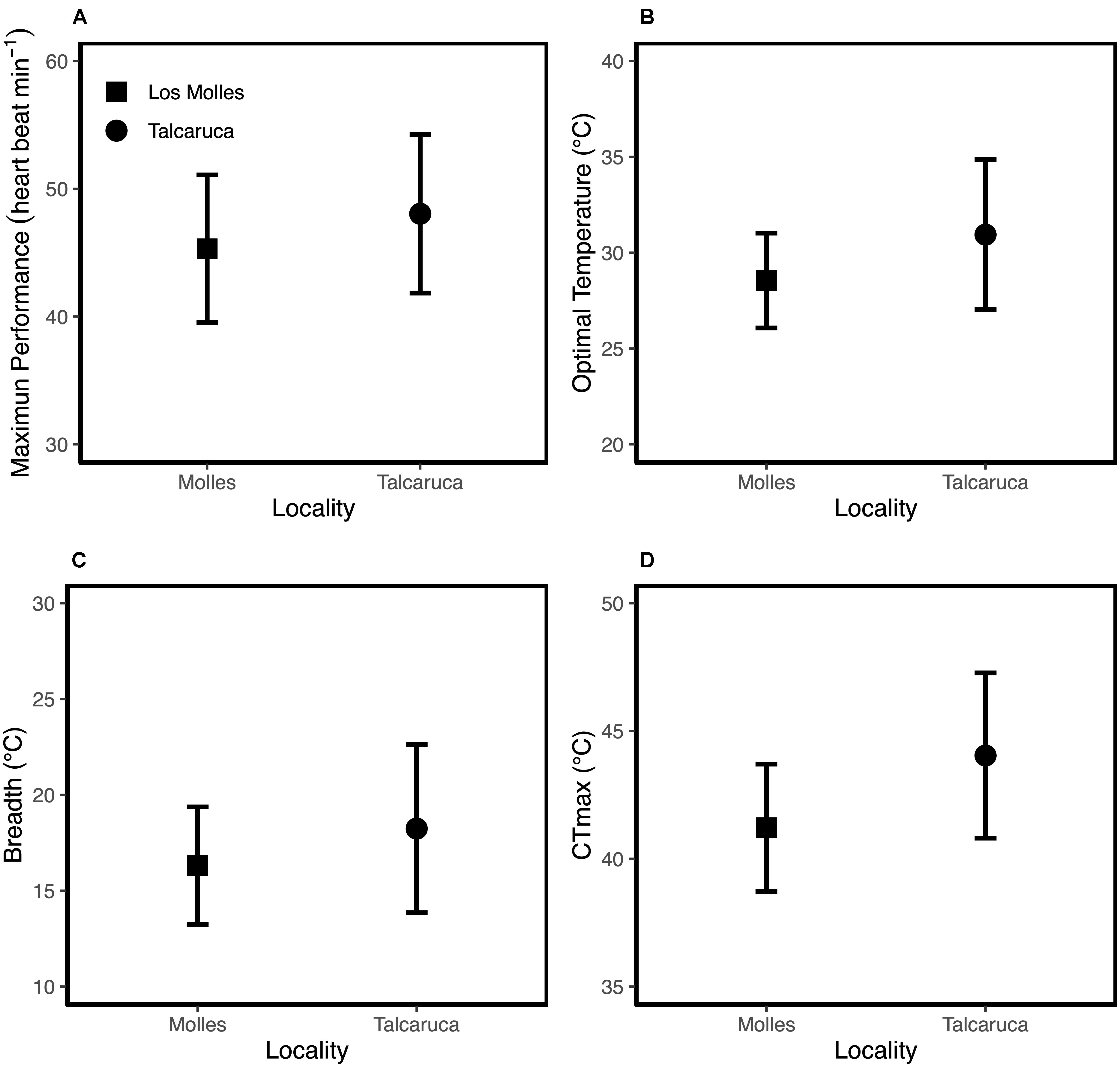
Figure 2. Mean values ± SD for Maximum performance (A); optimal temperature (B); thermal breadth (C) and critical maximum temperature (D).
Discussion
Ambient temperature plays a fundamental role on the performance and fitness of ectotherm organisms (Angilletta, 2009; Sunday et al., 2011), and therefore, knowing the physiological capacities of populations would allow us to better understand the implications of temperature changes in the current scenario of global warming (Calosi et al., 2008; Sunday et al., 2012; Gunderson and Stillman, 2015; Ramajo et al., 2020). In the present study we found that: (1) oxygen consumption increases with body size and that this effect is greater in individuals from the Talcaruca population, (2) Topt, Tbr, CTmax, and Pmax are higher in the population located in the area of high environmental heterogeneity (i.e., semi-permanent upwelling) and (3) individuals from Talcaruca have greater variance in Topt, Tbr, CTmax, but not in Pmax.
The pattern of energy use of an organism is reflected in its energy expenditure, and the metabolic rate is the most common way to measure it (Seibel and Drazen, 2007; Lardies et al., 2011, 2021). In this context, our results show that individuals with larger body sizes have higher energy expenditures, which would impact the reproduction, feeding and growth, among others consequences (Lardies and Bozinovic, 2008; Lardies et al., 2011; Ramajo et al., 2020; Broitman et al., 2021). Furthermore, the increase in energy expenditure with body size is more pronounced in the Talcaruca population, which suggests that exposure to a fluctuating environment has important implications on the performance of individuals (Bozinovic et al., 2016; Barria et al., 2018; Lardies et al., 2021). In particular, this higher cost associated with body size in Talcaruca could be related to the need for constant shell production, since the water masses that rise during upwelling have lower pH and high pCO2, which causes the permanent dissolution of this structure in mollusks (see Table 1; Duarte et al., 2014; Broitman et al., 2018; Osores et al., 2018; Lardies et al., 2021). Furthermore, carbonate dissolution increases at low temperatures (Morse et al., 2007; Lagos et al., 2016) and therefore, plate production and mineralization are more difficult to perform in upwelling zones (see Broitman et al., 2018; Ramajo et al., 2020).
Intertidal ectotherms are subject to significant body temperature variations in temporal and spatial scales due to exposure to tides (Helmuth et al., 2006) and temperatures during emersion periods at low tides can exceed the thermal limits of marine invertebrates (Harley, 2008). For example, signals of heating days have been evidenced along the coast of Chile in Talcaruca location (Gaitán-Espitia et al., 2014), however, the thermal stress in intertidal ectotherm is more related to elevated water temperature than aerial temperature (Seabra et al., 2016). Furthermore, we found chitons during low tides events under macroalgae canopy and not in sun-exposed microhabitats (see Otaiza and Santelices, 1985). In general, this thermal refugia are positively correlated with air temperature and solar radiation on marine invertebrates and where selection of these cool microhabitats produces a low respiratory performance (see Monaco et al., 2015). For example, a recent study in the chiton Cryptochiton stelleri indicates that its large size and reduced mobility force it to select lower temperatures to avoid overheating in those favorable thermal habitats (McIntire and Bourdeau, 2020). The previous may be the case of Acantopleura echinata due to their larger size (see Ibáñez et al., 2021), which can also be a poor thermoregulator, since therefore, future scenarios of increased temperature will expose chitons to more stressful temperature and can have a detrimental effect on organismal performance (see Miller, 2013) especially in highly variable thermal environments as Talcaruca location. In general, intertidal invertebrates live very close to their thermal tolerance (Denny et al., 2011; Gaitán-Espitia et al., 2017; Barria et al., 2018), but preference of A. echinata for cool microhabitats during low tide suggest that survival probability regarding to body temperatures could be high even in this variable environment.
Additionally, our results show that individuals living in more variable environments (i.e., Talcaruca) not only have greater thermal amplitudes (Tbr) and are capable of tolerating higher temperatures (CTmax) compared to individuals from more constant environments (i.e., Los Molles), but they also present greater variance in these traits (see Barria et al., 2018). These results are in agreement with the predictions of the CVH, which indicates that populations inhabiting environments with fluctuating temperatures require wider tolerance limits (Angilletta, 2009; Gaitán-Espitia et al., 2017; Broitman et al., 2021; Lardies et al., 2021). However this increase in thermal tolerance is not related with latitude in our chitons populations (see Bozinovic et al., 2011). Similarly, the higher Pmax in the population with semi-permanent upwelling would indicate that changing and variable environments require greater cardiac activity, which at the same time needs higher energy expenditures. In this context, a higher cardiac output would allow for a greater flow of hemolymph and the distribution of heat between the center and the surface of the body (Grigg and Seebacher, 1999) which could permit a more adequate thermoregulation to cope with environmental variations (Angilletta, 2009; Kodirov, 2011). We found higher heart rate under high temperatures probably occur during low tide (i.e., increase of aerial temperature) and this increase allows a rapid transport of oxygen to all tissues of chitons which elevated concurrently the metabolic rate of individuals. Finally, our results show that Topt of the chitons was larger in the population located in Talcaruca than in Los Molles and also showed a larger variance. Although this might be counterintuitive at first, since the population of Talcaruca is exposed to colder average temperatures, these differences in Topt might be explained by the fact that usually, Topt and Tbr are positively associated (Barria et al., 2018; Broitman et al., 2018). In particular, individuals in the permanent upwelling zone experience more extreme cold seawater surface temperature (SST) as well as hot SST and air temperatures in comparison to individuals from Los Molles, which could influence the increase of this trait (see Lardies et al., 2011). In addition, these results might be explained by the known negative relationship between Topt and latitude (Gaitán-Espitia et al., 2014; Barria et al., 2018; Broitman et al., 2018).
According to our results, it is clear that a variable environment affects the thermal physiology of organisms, expanding their tolerance ranges and generating energy costs in the performance of individuals. However, it is relevant to note that upwelling systems are phenomena where the rise of water masses modifies not only temperature, but also decreases O2, pH, and increases pCO2 (Torres and Ampuero, 2009; Schneider et al., 2017; Ramajo et al., 2020; Lardies et al., 2021). Together, these factors can influence the energy expenditure of mollusks, since, according to the literature, the differences in metabolic rates can be related to oxygen limitations in the environment (Frederich and Pörtner, 2000) and/or with its aerobic capacity as an adaptation to environmental heterogeneity (Clarke and Fraser, 2004; Watson et al., 2014; Pörtner and Gutt, 2016). Therefore, exposure to variable environments in oxygen concentration and pCO2 could also increase metabolic costs for maintaining homeostasis and thus, to compensate for energy expenditure, chitons could reduce shell formation (i.e., reduction in calcification rate) in environments with abrupt variations in pH (see Lannig et al., 2010; Lagos et al., 2016; Osores et al., 2017).
The vulnerability of organisms to variations in the temperature of the environment will depend on exposure, resilience and plasticity to adapt to environmental change (Huey et al., 2012; Donelson et al., 2019). Together, our observational and experimental results suggest that environmental conditions around large upwelling centers (i.e., high environmental heterogeneity) display higher levels of phenotypic variability (i.e., plasticity) in local population performance of adult individuals, and may represent a genetic buffer for the effects of ocean warming. Therefore, it is necessary to understand the thermal performance of marine organisms, considering the consequences of climate change, where the increase in ocean temperature could cause an increase in the frequency and intensity of upwelling systems on the Pacific Coast (Sydeman et al., 2014; Broitman et al., 2021) generating repercussions on the distribution, performance and fitness of the coastal populations and communities.
Data Availability Statement
The raw data supporting the conclusions of this article will be made available by the authors, without undue reservation.
Author Contributions
ML acquired funding for the study and carried out experiments. ML and LB conceived the idea and designed the study. All authors analyzed the data, drafted and commented on the manuscript.
Funding
This work was funded by the ANID FONDECYT No. 1190444. ML appreciate the support given by the Programa Investigacioìn Asociativa (ANID ACT 172037). Further, support by the ANID – Millennium Science Initiative Program – Code ICN2019_015 to ML was greatly appreciated.
Conflict of Interest
The authors declare that the research was conducted in the absence of any commercial or financial relationships that could be construed as a potential conflict of interest.
Publisher’s Note
All claims expressed in this article are solely those of the authors and do not necessarily represent those of their affiliated organizations, or those of the publisher, the editors and the reviewers. Any product that may be evaluated in this article, or claim that may be made by its manufacturer, is not guaranteed or endorsed by the publisher.
Acknowledgments
We would like to thank Carolina Fernaìndez for field and laboratory assistance and Nelson Lagos for providing equipment for alkalinity measurements. We would also like to thank Laura Ramajo and Roberto García-Huidobro for their help with carbonate system parameter estimations.
References
Angilletta, M. J. (2009). Thermal Adaptation: A Theoretical and Empirical Synthesis. Oxford: Oxford University Press.
Aravena, G., Broitman, B., and Stenseth, N. C. (2014). Twelve years of change in coastal upwelling along the central-northern coast of Chile: spatially heterogeneous responses to climatic variability. PLoS One 9:e90276. doi: 10.1371/journal.pone.0090276
Barria, A. M., and Bacigalupe, L. D. (2017). Intraspecific geographic variation in thermal limits and acclimatory capacity in a wide distributed endemic frog. J. Therm. Biol. 69, 254–260. doi: 10.1016/j.jtherbio.2017.08.010
Barria, A. M., Bacigalupe, L. D., Lagos, N. A., and Lardies, M. A. (2018). Thermal physiological traits and plasticity of metabolism are sensitive to biogeographic breaks in a rock-pool marine shrimp. J. Exp. Biol. 221, 1–10. doi: 10.1242/jeb.181008
Barria, A. M., Lardies, M. A., Beckerman, A. P., and Bacigalupe, L. D. (2014). Latitude or biogeographic breaks? Determinants of phenotypic (co)variation in fitness-related traits in Betaeus truncatus along the Chilean coast. Mar. Biol. 161, 111–118. doi: 10.1007/s00227-013-2319-0
Bozinovic, F., Bastías, D. A., Boher, F., Clavijo-Baquet, S., Estay, S. A., and Angilletta, M. J. (2011). The mean and variance of environmental temperature interact to determine physiological tolerance and fitness. Physiol. Biochem. Zool. 84, 543–552. doi: 10.1086/662551
Bozinovic, F., Sabat, P., Rezende, E. L., and Canals, M. (2016). Temperature variability and thermal performance in ectotherms: acclimation, behaviour, and experimental considerations. Evol. Ecol. Res. 17, 111–124.
Broitman, B. R., Aguilera, M. A., Lagos, N. A., and Lardies, M. A. (2018). Phenotypic plasticity at the edge: contrasting population-level responses at the overlap of the leading and rear edges of the geographical distribution of two Scurria limpets. J. Biogeogr. 45, 2314–2325. doi: 10.1111/jbi.13406
Broitman, B. R., Lagos, N. A., Opitz, T., Figueroa, D., Maldonado, K., Ricote, N., et al. (2021). Phenotypic plasticity is not a cline: thermal physiology of an intertidal barnacle over 20° of latitude. J. Anim. Ecol. 90, 1961–1972. doi: 10.1111/1365-2656.13514
Calosi, P., Bilton, D. T., and Spicer, J. I. (2008). Thermal tolerance, acclimatory capacity and vulnerability to global climate change. Biol. Lett. 4, 99–102. doi: 10.1098/rsbl.2007.0408
Camus, P. A., Navarrete, A. H., Sanhueza, ÁG., and Felipe Opazo, L. (2012). Trophic ecology of the chiton Acanthopleura echinata on Chilean rocky shores. Rev. Chilena Historia Natural 85, 123–135. doi: 10.4067/S0716-078X2012000100010
Clarke, A., and Fraser, K. P. P. (2004). Why does metabolism scale with temperature? Funct. Ecol. 18, 243–251. doi: 10.1111/j.0269-8463.2004.00841.x
Denny, M. W., Dowd, W. W., Bilir, L., and Mach, K. J. (2011). Spreading the risk: small-scale body temperature variation among intertidal organisms and its implications for species persistence. J. Exp. Mar. Biol. Ecol. 400, 175–190.
Dickson, A. G., Sabine, C. L., and Christian, J. R. (2007). Guide to best practices for ocean CO2 measurements. PICES Special Publ. 3:191.
Donelson, J. M., Sunday, J. M., Figueira, W. F., Gaitán-Espitia, J. D., Hobday, A. J., Johnson, C. R., et al. (2019). Understanding interactions between plasticity, adaptation and range shifts in response to marine environmental change. Philos. Trans. R. Soc. B Biol. Sci. 374:20180186. doi: 10.1098/rstb.2018.0186
Doney, S. C., Busch, D. S., Cooley, S. R., and Kroeker, K. J. (2020). The impacts of ocean acidification on marine ecosystems and reliant human communities. Annu. Rev. Environ. Resour. 45, 83–112. doi: 10.1146/annurev-environ-012320-083019
Duarte, C., Navarro, J. M., Acuña, K., Torres, R., Manríquez, P. H., Lardies, M. A., et al. (2014). Combined effects of temperature and ocean acidification on the juvenile individuals of the mussel Mytilus chilensis. J. Sea Res. 85, 308–314. doi: 10.1016/j.seares.2013.06.002
Duarte, C., Navarro, J. M., Quijón, P. A., Loncon, D., Torres, R., Manríquez, P. H., et al. (2018). The energetic physiology of juvenile mussels, Mytilus chilensis (Hupe): the prevalent role of salinity under current and predicted pCO2 scenarios. Environ. Pollut. 242, 156–163. doi: 10.1016/j.envpol.2018.06.053
Figueroa, D., and Moffat, C. (2000). On the influence of topography in the induction of coastal upwelling along the Chilean coast. Geophys. Res. Lett. 27, 3905–3908. doi: 10.1029/1999GL011302
Frederich, M., and Pörtner, H. O. (2000). Oxygen limitation of thermal tolerance defined by cardiac and ventilatory performance in spider crab, Maja squinado. Am. J. Physiol. Regul. Integr. Comp. Physiol. 279, 1531–1538. doi: 10.1152/ajpregu.2000.279.5.r1531
Gaitán-Espitia, J. D., Bacigalupe, L. D., Opitz, T., Lagos, N. A., Osores, S., and Lardies, M. A. (2017). Exploring physiological plasticity and local thermal adaptation in an intertidal crab along a latitudinal cline. J. Therm. Biol. 68, 14–20. doi: 10.1016/j.jtherbio.2017.02.011
Gaitán-Espitia, J. D., Bacigalupe, L. D., Opitz, T., Lagos, N. A., Timmermann, T., and Lardies, M. A. (2014). Geographic variation in thermal physiological performance of the intertidal crab Petrolisthes violaceus along a latitudinal gradient. J. Exp. Biol. 217, 4379–4386. doi: 10.1242/jeb.108217
Gilchrist, G. W. (1996). A quantitative genetic analysis of thermal sensitivity in the locomotor performance curve of Aphidius ervi. Evolution 50, 1560–1572. doi: 10.1111/j.1558-5646.1996.tb03928.x
Gillooly, J. F., Brown, J. H., West, G. B., Savage, V. M., and Charnov, E. L. (2001). Effects of size and temperature on metabolic rate. Science 293, 2248–2251. doi: 10.1126/science.1061967
Grigg, G. C., and Seebacher, F. (1999). Field test of a paradigm: hysteresis of heart rate in thermoregulation by a free-ranging lizard (Pogona barbata). Proc. Biol. Sci. 266, 1291–1297.
Gunderson, A. R., and Stillman, J. H. (2015). Plasticity in thermal tolerance has limited potential to buffer ectotherms from global warming. Proc. R. Soc. B Biol. Sci. 282:20150401. doi: 10.1098/rspb.2015.0401
Harley, C. D. G. (2008). Tidal dynamics, topographic orientation, and temperature-mediated mass mortalities on rocky shores. Mar. Ecol. Prog. Ser. 371, 37–46. doi: 10.3354/meps07711
Helmuth, B., Mieszkowska, N., Moore, P., and Hawkins, S. J. (2006). Living on the edge of two changing worlds: forecasting the responses of rocky intertidal ecosystems to climate change. Annu. Rev. Ecol. Evol. Syst. 37, 373–404. doi: 10.1146/annurev.ecolsys.37.091305.110149
Hill, A., Hickey, B., Shillington, F., Strub, T., Brink, K., Barton, E., et al. (1998). “Chapter 2- Eastern ocean boundaries coastal segment,” in The Sea: The Global Coastal Ocean, Regional Studies and Synthesis, Vol. 11, eds A. R. Robinson and K. H. Brink (New York, NY: John Wiley and Sons, Inc.), 29–67.
Huey, R. B., David, B., and Url, S. (2001). The University of Chicago Temperature. Mography Ectotherm Fitness 158, 204–210.
Huey, R. B., Kearney, M. R., Krockenberger, A., Holtum, J. A. M., Jess, M., and Williams, S. E. (2012). Predicting organismal vulnerability to climate warming: roles of behaviour, physiology and adaptation. Philos. Trans. R. Soc. B Biol. Sci. 367, 1665–1679. doi: 10.1098/rstb.2012.0005
Ibáñez, C. M., Carter, M. J., Aguilera, M. A., Pardo-Gandarillas, M. C., and Rezende, E. L. (2021). Body size variation in polyplacophoran molluscs: geographical clines and community structure along the south-eastern Pacific. Global Ecol. Biogeogr. 30, 1781–1795. doi: 10.1111/geb.13341
Kapsenberg, L., and Hofmann, G. E. (2016). Ocean pH time-series and drivers of variability along the northern Channel Islands, California, USA. Limnol. Oceanogr. 61, 953–968. doi: 10.1002/lno.10264
Kingsolver, J. G., Izem, R., and Ragland, G. J. (2004). Plasticity of size and growth in fluctuating thermal environments: comparing reaction norms and performance curves. Integr. Comp. Biol. 44, 450–460. doi: 10.1093/icb/44.6.450
Kodirov, S. A. (2011). The neuronal control of cardiac functions in Molluscs. Comp. Biochem. Physiol. A Mol. Integr. Physiol. 160, 102–116. doi: 10.1016/j.cbpa.2011.06.014
Lagos, N. A., Benítez, S., Duarte, C., Lardies, M. A., Broitman, B. R., Tapia, C., et al. (2016). Effects of temperature and ocean acidification on shell characteristics of Argopecten purpuratus: implications for scallop aquaculture in an upwelling-influenced area. Aquacul. Environ. Interactions 8, 357–370. doi: 10.3354/AEI00183
Lannig, G., Eilers, S., Pörtner, H. O., Sokolova, I. M., and Bock, C. (2010). Impact of ocean acidification on energy metabolism of oyster, Crassostrea gigas - Changes in metabolic pathways and thermal response. Mar. Drugs 8, 2318–2339. doi: 10.3390/md8082318
Lardies, M. A., and Bozinovic, F. (2008). Genetic variation for plasticity in physiological and life-history traits among populations of an invasive species, the terrestrial isopod Porcellio laevis. Evol. Ecol. Res. 10, 747–762.
Lardies, M. A., Caballero, P., Duarte, C., and Poupin, M. J. (2021). Geographical variation in phenotypic plasticity of intertidal sister Limpet’s species under ocean acidification scenarios. Front. Mar. Sci. 8:647087. doi: 10.3389/fmars.2021.647087
Lardies, M. A., Munoz, J. L., Paschke, K. A., and Bozinovic, F. (2011). Latitudinal variation in the aerial/aquatic ratio of oxygen consumption of a supratidal high rocky-shore crab. Mar. Ecol. 32, 42–51. doi: 10.1111/j.1439-0485.2010.00408.x
McIntire, L. C., and Bourdeau, P. E. (2020). World’s largest chiton (Cryptochiton stelleri) is an inefficient thermoregulator. Mar. Ecol. Prog. Ser. 652, 63–76. doi: 10.3354/meps13477
Miller, L. P. (2013). The effect of water temperature on drilling and ingestion rates of the dogwhelk Nucella lapillus feeding on Mytilus edulis mussels in the laboratory. Mar. Biol. 160, 1489–1496. doi: 10.1007/s00227-013-2202-z
Monaco, C. J., Wethey, D. S., Gulledge, S., and Helmuth, B. (2015). Shore-level size gradients and thermal refuge use in the predatory sea star Pisaster ochraceus: the role of environmental stressors. Mar. Ecol. Prog. Ser. 539, 191–205.
Morse, J. W., Arvidson, R. S., and Lu, A. (2007). Calcium carbonate formation and dissolution. Chem. Rev. 979, 342–381. doi: 10.1021/cr050358j
Murren, C. J., Auld, J. R., Callahan, H., Ghalambor, C. K., Handelsman, C. A., Heskel, M. A., et al. (2015). Constraints on the evolution of phenotypic plasticity: limits and costs of phenotype and plasticity. Heredity 115, 293–301. doi: 10.1038/hdy.2015.8Osores
Osores, S. J. A., Lagos, N. A., San Marnn, V., Manríquez, P. H., Vargas, C. A., Torres, R., et al. (2017). Plasticity and inter-population variability in physiological and life-history traits of the mussel Mytilus chilensis: a reciprocal transplant experiment. J. Exp. Mar. Biol. Ecol. 490, 1–12.
Osores, S. J. A., Ruz, G. A., Opitz, T., and Lardies, M. A. (2018). Discovering divergence in the thermal physiology of intertidal crabs along latitudinal gradients using an integrated approach with machine learning. J. Therm. Biol. 78, 140–150. doi: 10.1016/j.jtherbio.2018.09.016
Otaiza, R. D., and Santelices, B. (1985). Vertical distribution of chitons (Mollusca: Polyplacophora) in the rocky intertidal zone of central Chile. J. Exp. Mar. Biol. Ecol. 86, 229–240.
Pörtner, H. (2001). Climate change and temperature-dependent biogeography: oxygen limitation of thermal tolerance in animals. Naturwissenschaften 88, 137–146. doi: 10.1007/s001140100216
Pörtner, H. O., and Gutt, J. (2016). Impacts of climate variability and change on (marine) animals: physiological underpinnings and evolutionary consequences. Integr. Comp. Biol. 56, 31–44. doi: 10.1093/icb/icw019
R Core Team (2020). R: A Language and Environment for Statistical Computing. Vienna: R Foundation for Statistical Computing.
Rahn, D. A., Garreaud, R. D., and Rutllant, J. A. (2011). The low-level atmospheric circulation near tongoy bay-point lengua de vaca (Chilean Coast, 30°S). Monthly Weather Rev. 139, 3628–3647. doi: 10.1175/MWR-D-11-00059.1
Rahn, D. A., Rosenblüth, B., and Rutllant, J. A. (2015). Detecting subtle seasonal transitions of upwelling in North-Central Chile. J. Phys. Oceanogr. 45, 854–867. doi: 10.1175/JPO-D-14-0073.1
Ramajo, L., Valladares, M., Astudillo, O., Fernández, C., Rodríguez-Navarro, A. B., Watt-Arévalo, P., et al. (2020). Upwelling intensity modulates the fitness and physiological performance of coastal species: implications for the aquaculture of the scallop Argopecten purpuratus in the Humboldt Current System. Sci. Total Environ. 745:140949. doi: 10.1016/j.scitotenv.2020.140949
Rezende, E. L., and Bozinovic, F. (2019). Thermal performance across levels of biological organization. Philos. Trans. R. Soc. B Biol. Sci. 374:20180549. doi: 10.1098/rstb.2018.0549
Rivadeneira, M. M., and Fernández, M. (2005). Shifs in southern endpoints of distribution in rocky intertidal species along the south-eastern Pacific coast. J. Biogeogr. 32, 203–209. doi: 10.1111/j.1365-2699.2004.01133.x
Schneider, W., Donoso, D., Garcés-Vargas, J., and Escribano, R. (2017). Water-column cooling and sea surface salinity increase in the upwelling region off central-south Chile driven by a poleward displacement of the South Pacific High. Prog. Oceanogr. 151, 38–48. doi: 10.1016/j.pocean.2016.11.004
Seabra, R., Wethey, D. S., Santos, A. M., Gomes, F., and Lima, F. P. (2016). Equatorial range limits of an intertidal ectotherm are more linked to water than air temperature. Global Change Biol. 22, 3320–3331. doi: 10.1111/gcb.13321
Seibel, B. A., and Drazen, J. C. (2007). The rate of metabolism in marine animals: environmental constraints, ecological demands and energetic opportunities. Philos. Trans. R. Soc. B Biol. Sci. 362, 2061–2078. doi: 10.1098/rstb.2007.2101
Somero, G. N. (2010). The physiology of climate change: how potentials for acclimatization and genetic adaptation will determine “winners” and “losers.”. J. Exp. Biol. 213, 912–920. doi: 10.1242/jeb.037473
Sunday, J. M., Bates, A. E., and Dulvy, N. K. (2011). Global analysis of thermal tolerance and latitude in ectotherms. Proc. R. Soc. B Biol. Sci. 278, 1823–1830. doi: 10.1098/rspb.2010.1295
Sunday, J. M., Bates, A. E., and Dulvy, N. K. (2012). Thermal tolerance and the global redistribution of animals. Nat. Clim. Change 2, 686–690. doi: 10.1038/nclimate1539
Sydeman, W. J., García-Reyes, M., Schoeman, D. S., Rykaczewski, R. R., Thompson, S. A., Black, B. A., et al. (2014). Climate change and wind intensification in coastal upwelling ecosystems. Science 345, 77–80. doi: 10.1126/science.1251635
Tobar-Villa, C., and Ibáñez, C. (2013). Acanthopleura echinata (Barnes, 1824) (Barnes, 1824):antecedentes de la especie. Amici Molluscarum 21, 23–28.
Torres, R., and Ampuero, P. (2009). Strong CO2 outgassing from high nutrient low chlorophyll coastal waters off central Chile (30°S): the role of dissolved iron. Estuar. Coast. Shelf Sci. 83, 126–132. doi: 10.1016/j.ecss.2009.02.030
Torres, R., Pantoja, S., Harada, N., González, H. E., Daneri, G., Frangopulos, M., et al. (2011). Air-sea CO2 fluxes along the coast of Chile: from CO2 outgassing in central northern upwelling waters to CO2 uptake in southern Patagonian fjords. J. Geophys. Res. Oceans 116:C09006. doi: 10.1029/2010JC006344
Van Buskirk, J., and Steiner, U. K. (2009). The fitness costs of developmental canalization and plasticity. J. Evol. Biol. 22, 852–860. doi: 10.1111/j.1420-9101.2009.01685.x
Vargas, C. A., Lagos, N. A., Lardies, M. A., Duarte, C., Manríquez, P. H., Aguilera, V. M., et al. (2017). Species-specific responses to ocean acidification should account for local adaptation and adaptive plasticity. Nat. Ecol. Evol. 1, 1–7. doi: 10.1038/s41559-017-0084
Keywords: thermal sensitivity, heart rate, thermal performance curve, upwelling, geographic variation, environmental variability
Citation: Manríquez N, Bacigalupe LD and Lardies MA (2021) Variable Environments in an Upwelling System Trigger Differential Thermal Sensitivity in a Low Intertidal Chiton. Front. Ecol. Evol. 9:753486. doi: 10.3389/fevo.2021.753486
Received: 04 August 2021; Accepted: 13 September 2021;
Published: 04 October 2021.
Edited by:
Marco Fusi, Edinburgh Napier University, United KingdomReviewed by:
Rui Seabra, Centro de Investigacao em Biodiversidade e Recursos Geneticos (CIBIO-InBIO), PortugalSylvain Pincebourde, UMR 7261 Institut de Recherche sur la Biologie de l’Insecte (IRBI), France
Copyright © 2021 Manríquez, Bacigalupe and Lardies. This is an open-access article distributed under the terms of the Creative Commons Attribution License (CC BY). The use, distribution or reproduction in other forums is permitted, provided the original author(s) and the copyright owner(s) are credited and that the original publication in this journal is cited, in accordance with accepted academic practice. No use, distribution or reproduction is permitted which does not comply with these terms.
*Correspondence: Marco A. Lardies, bWFyY28ubGFyZGllc0B1YWkuY2w=