Do Microbiota in the Soil Affect Embryonic Development and Immunocompetence in Hatchling Reptiles?
- Department of Biological Sciences, Macquarie University, Sydney, NSW, Australia
Reptile eggs develop in intimate association with microbiota in the soil, raising the possibility that embryogenesis may be affected by shifts in soil microbiota caused by anthropogenic disturbance, translocation of eggs for conservation purposes, or laboratory incubation in sterile media. To test this idea we incubated eggs of keelback snakes (Tropidonophis mairii, Colubridae) in untreated versus autoclaved soil, and injected lipopolysaccharide (LPS) into the egg to induce an immune response in the embryo. Neither treatment modified hatching success, water uptake, incubation period, or white-blood-cell profiles, but both treatments affected hatchling size. Eggs incubated on autoclaved soil produced smaller hatchlings than did eggs on untreated soil, suggesting that heat and/or pressure treatment decrease the soil’s suitability for incubation. Injection of LPS reduced hatchling size, suggesting that the presence of pathogen cues disrupts embryogenesis, possibly by initiating immune reactions unassociated with white-blood-cell profiles. Smaller neonates had higher ratios of heterophils to leucocytes, consistent with higher stress in smaller snakes, or body-size effects on investment into different types of immune cells. Microbiota in the incubation medium thus can affect viability-relevant phenotypic traits of hatchling reptiles. We need further studies to explore the complex mechanisms and impacts of environmental conditions on reptilian embryogenesis.
Introduction
The embryos of most species of oviparous reptiles spend the majority of their total developmental period (typically, the latter two-thirds) outside the mother’s body, in external nests (e.g., Shine, 1983). As a result, embryos of many species are exposed to a wide range in both the average values and temporal variance of abiotic factors such as temperature and moisture (e.g., Singh et al., 2020). Experimental studies confirm that even minor variation in such factors can significantly modify fitness-relevant traits such as incubation duration, and the phenotypic traits (morphological, physiological, and behavioral) of hatchlings (e.g., Georges et al., 2005; Amiel and Shine, 2012; Bodensteiner et al., 2015). Importantly, embryos in external nests are also exposed to biotic challenges including predation and infection by pathogens (Moreira and Barata, 2005), potentially inducing adaptive responses (e.g., immediate hatching of eggs induced by physical disturbance: Doody, 2011).
In the current article, we explore a neglected aspect of embryo-environment interactions in reptiles: the possibility that microbiota (bacteria, viruses, fungi, etc.) in the soil within a nest may penetrate the eggshell and affect the embryo. Although we are not aware of any studies that have looked for such penetration, it is feasible on physical grounds because soil microbiota are diverse and abundant (e.g., Wolmarans and Swart, 2014), pores within the squamate eggshell are large enough to permit ingress of micro-organisms (Packard and DeMarco, 1991), and eggs take up substantial water from the incubation substrate during development (thereby providing a straightforward means of entry for microbiota: Warner et al., 2011).
Eggs might also be exposed to potentially harmful microbiota even before oviposition. Pathogens could be present as the eggs pass through the oviduct (e.g., vertical transmission of Salmonella) and through the cloaca (fecal contamination) (D’Alba and Shawkey, 2015; Zając et al., 2021). Hydric and thermal conditions inside nests also may expose eggs to high concentrations of saprophytic microbes (e.g., Löwenborg et al., 2011).
The most critical factor thus may not be whether or not microbiota can find their way into developing eggs (surely, they can) but the effects of such exposure on embryos. Studies on later life stages (free-living reptiles) document many effects of infection, including immune responses and stress reactions (e.g., Wellehan and Johnson, 2005; Jacobson, 2007). By analogy, embryos might exhibit such responses as well (as they do in other vertebrates: e.g., Ygberg and Nilsson, 2012). The selective benefit of responding to microbial invasion might include adjusting immune-system development to better match the pathogens likely to be encountered after hatching (Grindstaff et al., 2006). Plausibly, natural selection might fine-tune embryonic responses to microbial exposure either directly, or via facultative manipulation of maternal allocation of resources to the egg (e.g., transfer of immune compounds such as antibodies) in response to the mother’s own history of encounters with pathogens.
The potential impacts of soil microbiota on reptilian embryogenesis are relevant to anthropogenic disturbances to the nest environment. For example, human activities such as agriculture and pollution greatly modify microbial communities within the soil (Wolmarans and Swart, 2014), humans affect nest-site selection of reptiles by destroying traditional nest-sites and/or creating new options (Francis et al., 2019; Doherty et al., 2020), and conservation-focused management frequently involves incubation of eggs in places other than where they were laid (e.g., Jarvie et al., 2014; Mitchell et al., 2016) or in substrates that contain very different microbiota than are found in natural nest-sites. In the extreme, captive breeding of oviparous reptiles often includes incubation of eggs in artificial media such as vermiculite, which may be virtually sterile (Köhler, 2005; Tefera and Vidal, 2009). In humans, artificial elimination of contact with the vaginal microbiota in surgically delivered (cesarean) babies can have deleterious long-term effects on infant health (Dominguez-Bello et al., 2016).
As a first step toward addressing this gap in knowledge, we conducted experimental incubation trials with the eggs of an oviparous tropical snake. In the first experiment, we incubated eggs in soil that either contained or did not contain the natural microbiota (i.e., was or was not autoclaved prior to use). Because any null result from such a study may be due to lack of penetration of the shell by local micro-organisms, we then ran another study in which we directly introduced lipopolysaccharide (LPS, a component of bacterial cell walls) into the egg to stimulate an immune response by the embryo. This second experiment allowed us to assess the effects of chemically signaling the presence of pathogenic microbiota without introducing any confounding deleterious affects (such as propagation) that would arise from actually introducing harmful bacteria. To quantify the effects of these manipulations, we measured aspects of developmental rate (incubation period), hatchling morphology, and circulating immune cells (leucocytes). Because the ratio of certain leucocytes (heterophils: lymphocytes; H:L) provides an index of stress (Davis et al., 2008), we looked specifically at levels of those cell types. Specifically, we predicted that the presence of microbiota (or their chemical cues) could impose developmental costs on embryos that would be manifested in altered morphological or immunological measures.
Materials and Methods
Study Site
Fogg Dam (12.56°S, 13130°E) is located 50 km southeast of the city of Darwin in Australia’s Northern Territory. The 1,500-m long man-made wall of the dam impounds a 250-ha body of water. The area experiences a wet–dry tropical climate, with high temperatures all year (mean daily maximum >30°C every month) but with rainfall primarily restricted to a 6-month (November–April) “wet season” (Brown and Shine, 2006a,b).
Study Species
Keelbacks (Tropidonophis mairii, Figure 1) are non-venomous natricine colubrid snakes (a group sometimes elevated to a separate family, Natricidae). Keelbacks are found in freshwater habitats across northern Australia (Cogger, 2015). At Fogg Dam, maximum body size (snout-vent length; SVL) of females is 82.2 cm and maximum body size of males is 67.7 cm. Males and females both grow rapidly and mature in <12 months (Brown et al., 2017).
At our study site, keelbacks are active year-round with a diet consisting largely of amphibians (Brown and Shine, 2002). Female keelbacks produce one or two clutches of eggs each year during the dry season (April–October; Brown and Shine, 2002). When females are ready to oviposit, they move from riparian habitats to the higher, well-drained soil of the dam wall to nest (Brown and Shine, 2005b). Eggs typically are laid around 10–20 cm beneath the soil surface, and nests are often communal (Brown and Shine, 2005b,2009). Female keelbacks exhibit natal philopatry, returning to the site at which their mother oviposited when it is time to lay their own eggs (Brown and Shine, 2007a). Incubation requires approximately 8 weeks, and the phenotypic traits of hatchlings are influenced by the thermal and hydric conditions that they experience during this period (Webb et al., 2001; Brown and Shine, 2004).
In laboratory studies, female keelbacks actively selected moist rather than dry substrates for oviposition; this nest-site choice resulted in larger hatchlings with a higher probability of surviving their first year of life in the field (Brown and Shine, 2004). Moisture levels in the incubation medium also affect immune function of hatchlings. Eggs incubated on moist substrate had higher lymphocyte counts, a trait that was linked to increased probability of survival in the field (Brown and Shine, 2018).
Collection of Eggs
We hand-captured female keelbacks at night on the wall of Fogg Dam during May 2015 and March–April 2017. Snakes were returned to the lab and kept overnight in cloth bags. The following day they were weighed, measured (snout-vent length, SVL), individually marked by scale-clipping, and palpated for the presence of shelled eggs. Gravid females were held in captivity in 12-L plastic cages lined with newspaper and equipped with a nest box containing damp vermiculite and a small water dish. For the present study, we used clutches from five females in 2015 and from four females in 2017. After laying, females were returned to their original capture location and released. At the conclusion of experimental studies (below), hatchlings were released at the mother’s site of capture.
Experiment #1: Effect of Incubation Substrate Disinfection on Hatchling Snakes
In 2015 we used a split-clutch design to incubate 68 eggs from five clutches on either natural soil substrate, or soil substrate that had been autoclaved to kill microbes and other potential egg pathogens. We collected soil from the site where the gravid female keelbacks had been captured. In a location typical of keelback nesting habitat (Brown and Shine, 2005b), we collected 2 L of damp soil from 10 to 20 cm below the surface. We placed 40 g of the damp soil into 100-mL plastic cups, and autoclaved half of those cups at 131°C and 0.22 Bar for 4 min.
Within 24 h of oviposition, eggs were measured and weighed. Forty-eight hours after oviposition, eggs within each clutch were paired by mass as closely as possible and one member of each mass-matched pair was assigned to the “Dirty” soil treatment and the other to the “Clean” soil treatment. We covered the cups with lids and placed them into an insulated coolbox at ambient room temperature (25°C). Eggs were re-weighed after 2–3 weeks and again after 5–6 weeks.
Experiment #2: Effect of Lipopolysaccharide Injection on Hatchling Snakes
In 2017, this experiment was conducted on four clutches consisting of 46 eggs. Within 24 h of oviposition, eggs were measured and weighed. Each clutch was then placed in a plastic bag containing 20 g of vermiculite and 5 g water (i.e., 25% water by weight). This level of substrate moisture causes slight water loss in keelback eggs (Brown and Shine, 2006a), generating slightly desiccated eggs that facilitated injection of 0.03 mL of fluid into each one. Bags containing each clutch were placed in an insulated coolbox and incubated at room temperature (25°C).
After 24 days, eggs were reweighed and half the eggs in each clutch were injected with 0.03 mL of a 2 mg/mL solution of lipopolysaccharide (LPS, Sigma L2630) dissolved in sterile phosphate buffered saline (PBS), under sterile conditions. We were unable to find any published reports in which LPS doses were administered to reptile embryos. Thus we chose a dosage rate (approximately 0.02 mg/g) capable of inducing behavioral changes in adult reptiles (e.g., Deen and Hutchison, 2001; do Amaral et al., 2002). This dosage of LPS also elicited significant changes in white blood cell (WBC) differential counts (specifically an increase in H:L ratios) in adult rattlesnakes (Sistrurus miliarius) (Lind et al., 2020). Because LPS is a component of bacterial cell walls, its injection into an organism typically elicits an immune response because the animal’s immune system recognizes LPS as indicative of bacterial infection (e.g., Bonneaud et al., 2003; de Figueiredo et al., 2021). As controls, the other half of the eggs in each clutch were injected with 30 μL of sterile phosphate buffered saline (PBS), which is not expected to induce any physiological response (e.g., Bonneaud et al., 2003). To inject each egg, the apical tip was swabbed with 70% ethanol and a 50-μL micro-injector used to administer either LPS or PBS into the yolk, taking care to avoid the embryo. Following injections, eggs were individually placed in covered 100-mL cups containing 3 g vermiculite and 9 g water (i.e., 300% water by weight) and placed back into the insulated coolbox to complete incubation. Eggs were reweighed after 5–6 weeks.
Scoring of Phenotypic Traits of Hatchlings (Experiments #1 and #2)
As hatching dates approached, we checked eggs daily for pipping. Within 25 h of emerging, each hatchling was checked for sex (based on tail shape and eversion of hemipenes: Brown and Shine, 2002), measured for SVL, head length and tail length, and weighed. We excised the terminal 1 mm from each snake’s tail with a sterile scalpel blade, and smeared the droplet of blood onto a clean glass slide. We fixed blood smears in methanol, stained them with modified Wright’s stain and fitted them with cover slips. We examined slides under 1,000× magnification until 100 white blood cells (WBCs) were encountered and identified as either basophil, heterophil, monocyte, lymphocyte, or azurophil. Eosinophils are rare or absent in snakes (Sykes and Klaphake, 2008) and were not observed on any slides during the present study.
Analysis of Data
We divided heterophil counts by lymphocyte count for each snake to calculate the H:L ratio. We log-transformed WBC differential counts and H:L ratios data prior to analysis to better meet assumptions of multiple regression. Because sex and size of hatchling keelbacks can affect their WBC profiles (Brown and Shine, 2018), we included these factors as independent variables in models assessing the effects of treatment. We also included Maternal ID as a random effect in these models, to recognize relatedness among clutch-mates. Residuals from all analyses were inspected to verify that all model assumptions were met. Analyses were conducted using JMP 14 (SAS Institute Inc., Cary, NC, United States).
Results
Experiment #1: Effect of Incubation Substrate Disinfection on Hatchling Snakes
Mean mass of eggs did not differ significantly between Clean and Dirty treatments at the beginning of the experiment (2.80 g vs. 2.81 g; F1,66 = 0.01, P = 0.91), nor after 2–3 weeks (3.57 g vs. 3.57 g; F1,66 = 0.001, P = 0.97) or after 5–6 weeks (4.50 g vs. 4.53 g; F1,66 = 0.07, P = 0.79) of incubation (Figure 2).
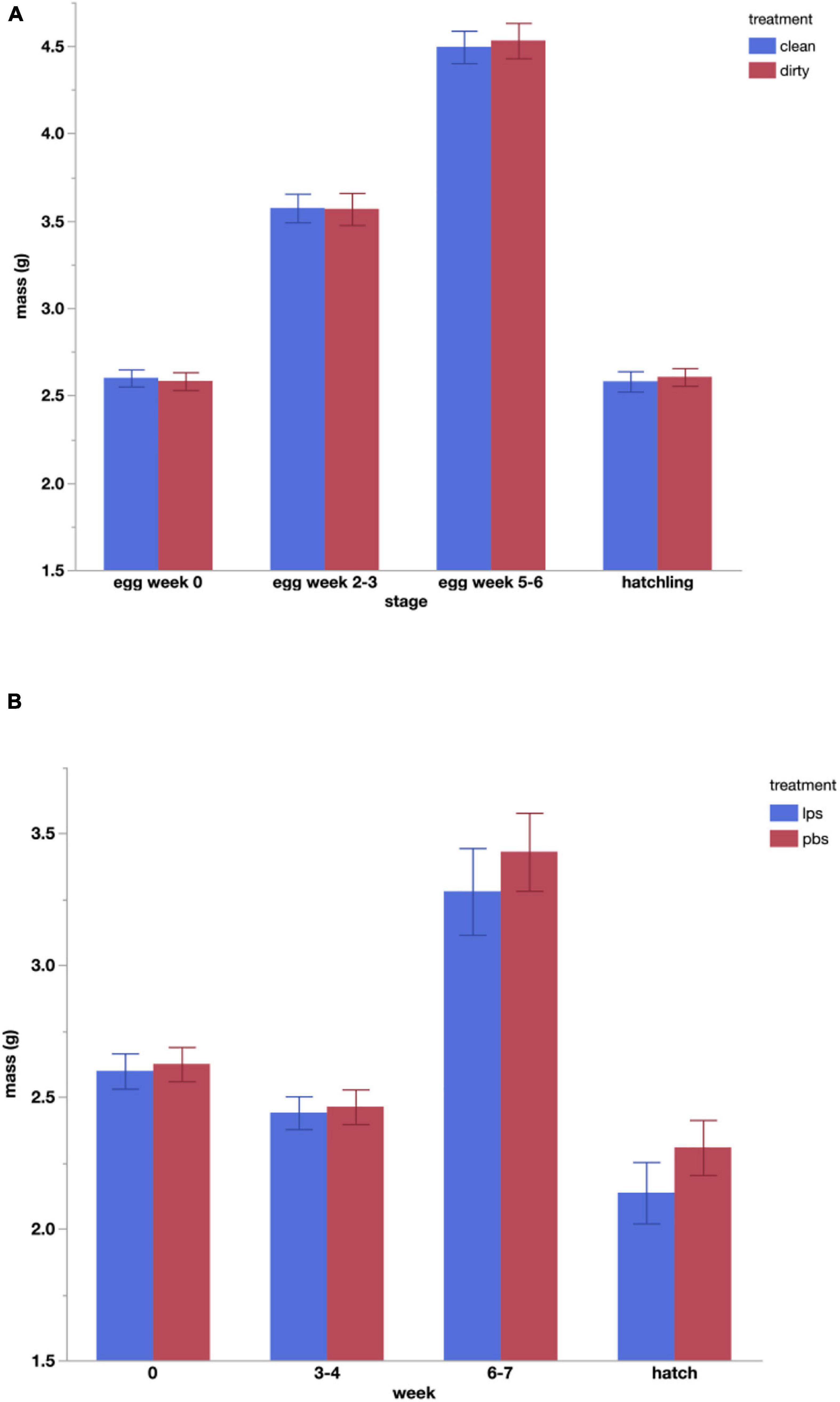
Figure 2. Changes in mean (±standard error) mass of eggs over the course of incubation and resultant hatchling mass of keelback snakes (Tropidonophis mairii) for the two experiments. (A) Eggs incubated on untreated soil from a natural nest site (Dirty) vs. soil from the same site that had been autoclaved (Clean). (B) Eggs injected with 30 μL lipopolysaccharide solution after week 4 (LPS) vs. eggs injected with 30 μL phosphate buffered saline (PBS) after week 4.
Hatching success was 94% (31 of 33 eggs) for eggs incubated on the Clean substrate and 100% for those on the Dirty substrate (35 of 35 eggs) and did not differ significantly between treatments (χ2 = 2.96, 1 df, P = 0.09). The mean duration of the incubation period also did not differ significantly between treatments (39.8 days vs. 39.8 days; F1,66 = 0.001, P = 0.99). However, hatchling snakes from the Dirty incubation treatment were longer than those from the Clean treatment and had longer heads (Table 1).
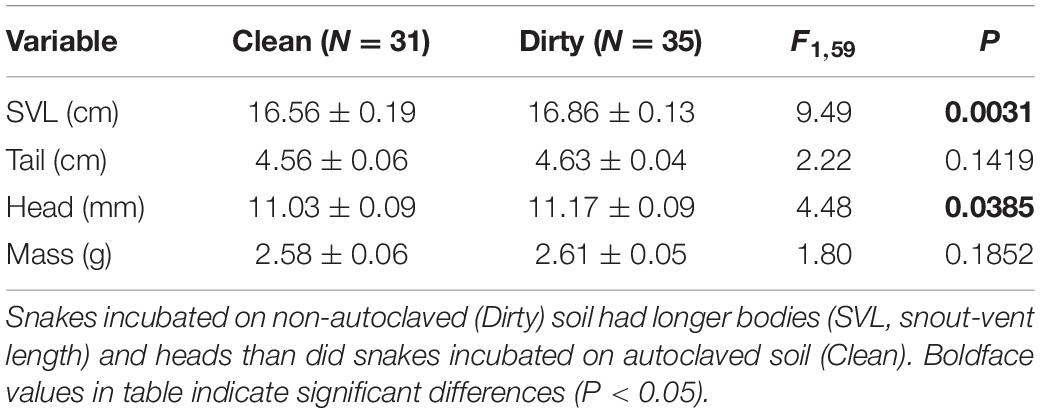
Table 1. Effect of autoclaving soil (the incubation substrate) on hatchling phenotype of keelback snakes (Tropidonophis mairii) in Experiment #1.
Autoclaving the incubation substrate had no significant effect on any WBC measures (Table 2). However, several measures were affected by hatchling body size. Basophils, heterophils and H:L ratios decreased with SVL (Table 2 and Figures 3, 4) whereas lymphocytes increased with SVL (Table 2 and Figure 3). Monocyte counts were higher in females than in males.
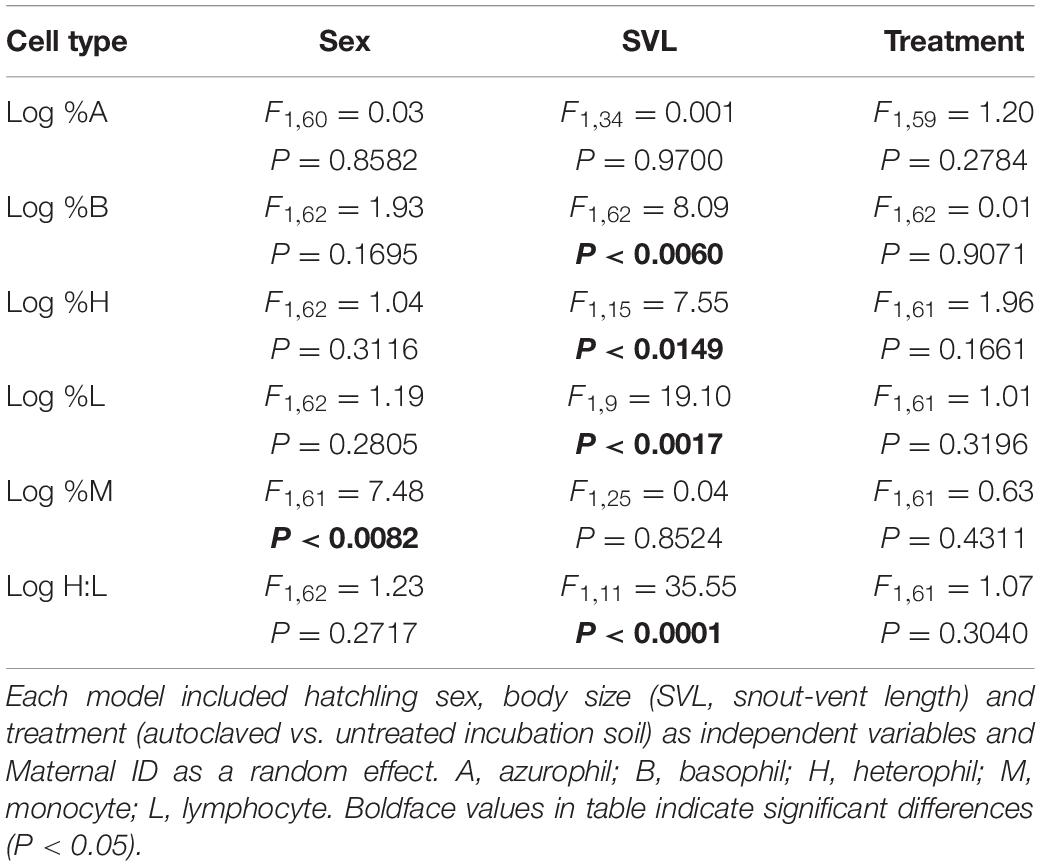
Table 2. Effects of mixed model analyses on the factors affecting white blood cell counts of hatchling keelback snakes (Tropidonophis mairii) in Experiment #1.
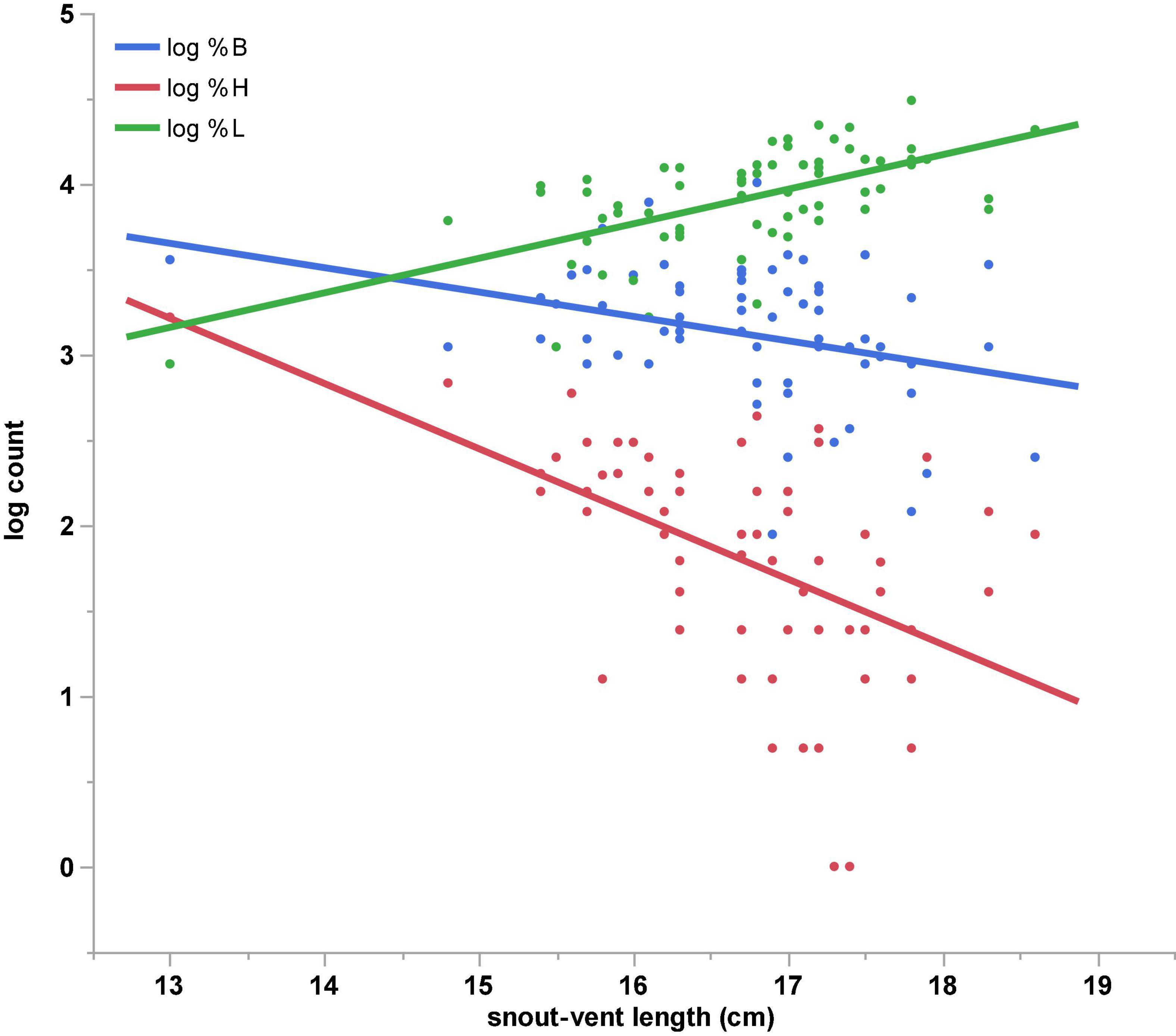
Figure 3. Significant effects of hatchling body size of keelback snakes (Tropidonophis mairii) on white-blood-cell measures found in Experiment #1. Basophil and heterophil counts decreased with hatchling body size (SVL, snout-vent length) whereas lymphocyte counts increased with snout-vent length (SVL).
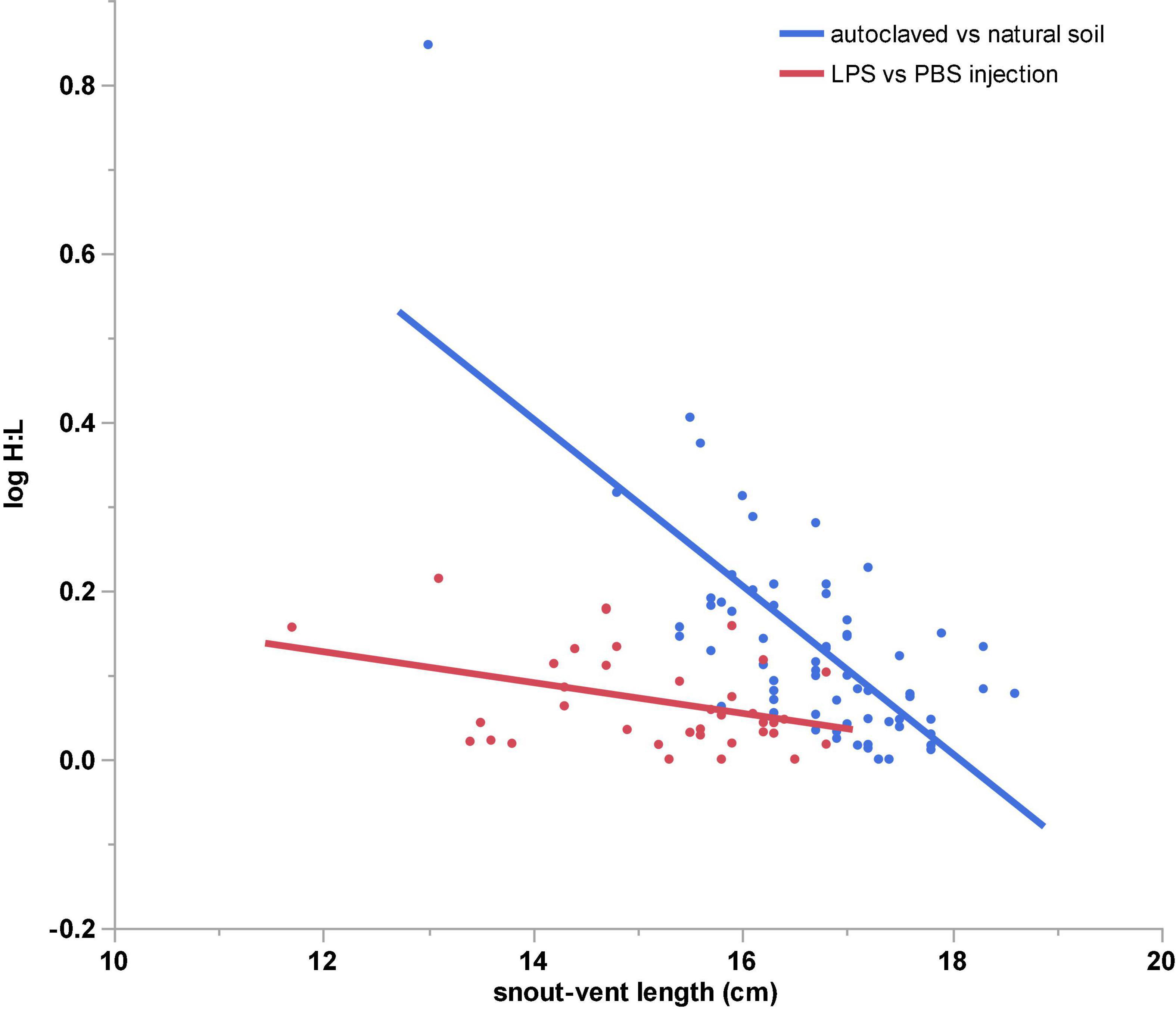
Figure 4. Effects of hatchling body size (SVL, snout-vent length) of keelback snakes (Tropidonophis mairii) on heterophil:lymphocyte (H:L) ratios. H:L ratio decreased significantly with snout-vent length (SVL) in both experiments.
Experiment #2: Effect of Injecting Lipopolysaccharide on Hatchling Snakes
There was no significant difference in the initial mass of eggs that were assigned to each treatment group (means for LPS 2.60 g vs. Control 2.63 g; F1,44 = 0.08, P = 0.78). After 24 days incubation on 25% vermiculite, eggs lost an average of 0.16 g, but mean mass did not differ significantly between groups (LPS 2.44 g vs. Control 2.46 g; F1,44 = 0.06, P = 0.81). This mass loss allowed us to inoculate the eggs with 30 μL of LPS or PBS. After a further 3 weeks’ incubation in individual cups with 300% vermiculite, mean mass was again similar between treatment groups (LPS 3.28 g vs. Control 3.43 g; F1,39 = 0.46, P = 0.50) (Figure 2).
Hatching success of eggs was 96% (21 of 22 eggs) in the LPS treatment and 92% (22 of 24 eggs) in the Control treatment and did not differ significantly between the groups (χ2 = 0.27, 1 df, P = 0.60). The mean length of the incubation period also did not differ between LPS and Control treatments (44.6 days vs. 44.7 days; F1,43 = 0.001, P = 0.95). However, hatchlings from the Control treatment had longer bodies, tails and head than did those from the LPS treatment (Table 3).
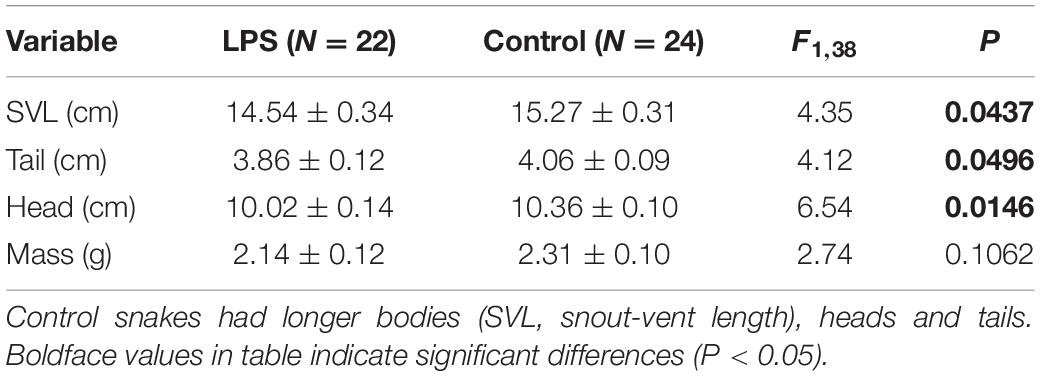
Table 3. Effect of inoculating the eggs of keelback snakes (Tropidonophis mairii) with lipopolysaccharide (LPS) or phosphate buffered saline (Control) on phenotypes of hatchlings in Experiment #2.
Lipopolysaccharide treatment had no significant effect on any WBC counts, nor did sex or body size (Table 4). However, H:L ratio decreased with body size (Table 4 and Figure 4) and was lower in females than in males (Table 4).
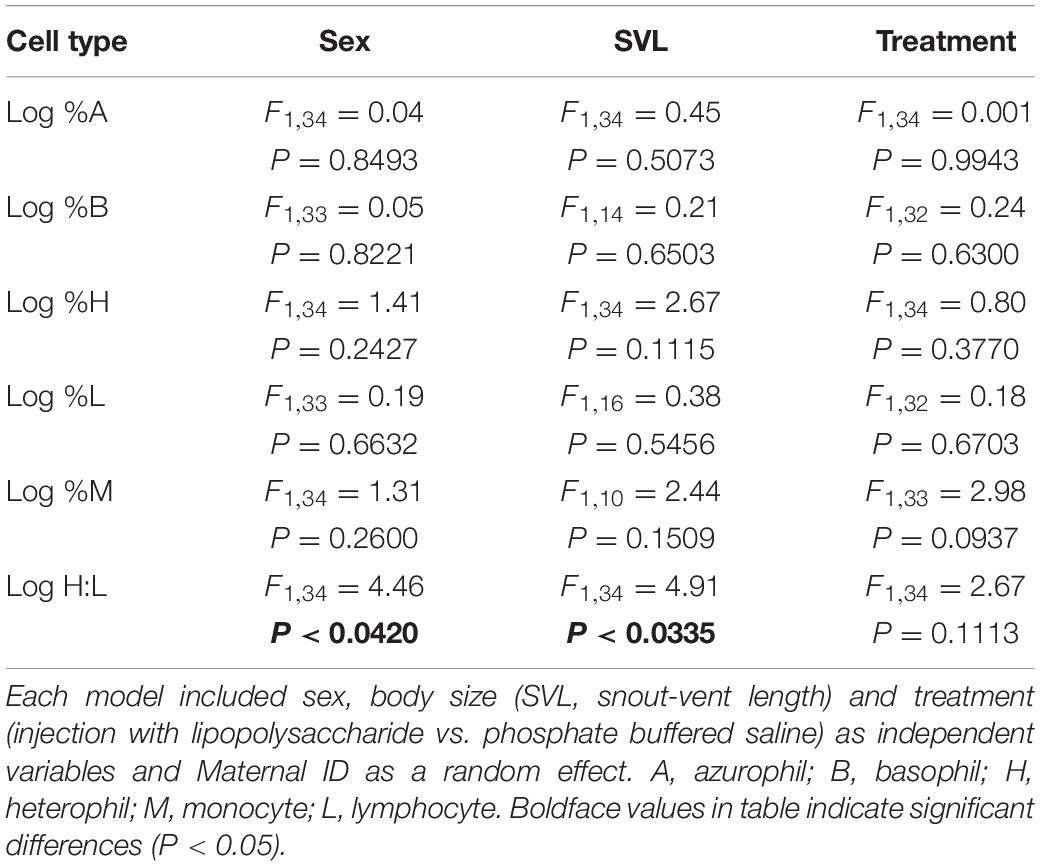
Table 4. Effects of mixed model analyses on the factors affecting white blood cell counts of hatchling keelback snakes (Tropidonophis mairii) in Experiment #2.
Discussion
In both experiments, incubation treatments generated significant differences in hatchling body size. Although much of the variation among clutches in hatchling size is driven by heritable (genetic) factors in this species (Brown and Shine, 2007b), considerable variation also is induced by the conditions under which eggs are incubated. For example, incubation on dry substrates substantially reduces offspring size in keelbacks (Shine and Brown, 2002; Brown and Shine, 2005a) as in many other species of squamate reptiles (Bodensteiner et al., 2015). Longer incubation also can allow squamate embryos to develop more fully, affecting body size at the time of hatching (Shine and Olsson, 2003). These complicating factors cannot explain the effect of treatment on offspring size in the present study, however, because neither rates of water uptake nor incubation periods were affected by our experimental treatments (above). Thus, exposure to microbiota (Experiment #1) or to cues associated with bacterial infection (Experiment #2) invoked morphological changes in offspring that were independent of egg size, incubation period or moisture balance. Our data thus add yet another variable to the long list of external factors that are known to affect developmental trajectories in embryonic reptiles [e.g., see reviews by Noble et al. (2018) and While et al. (2018)].
In Experiment #1, autoclaving the soil used for incubation resulted in a small but statistically significant reduction in hatchling head and body length but did not affect body mass (Table 1). The mechanism behind this shift in hatchling morphology is unknown. Soil is difficult to sterilize (Nowak and Wronkowska, 1987) so our single bout of autoclaving might only have resulted in a temporary decrease or alteration of the microbiotic community. Further, the high temperature (131°C) and pressure (2.2 Bar) associated with autoclaving might also have altered physical or chemical (e.g., pH) characteristics of the soil. Changes in the substrate characteristics such as these could have caused subtle developmental shifts in embryos.
In contrast, WBC profiles of hatchling snakes were not significantly affected by exposure to the immune challenges that were manipulated in the present study. The lack of a significant impact of microbiota presence on hatchling immune function in Experiment #1 could be attributed to the eggshell blocking signals regarding external bacterial levels from reaching the embryo. If this were the case, then studies on other systems (different focal species, different soil microbiota, etc.) might generate different results. To test that possibility, our second experiment inoculated pathogen signals directly into the egg, bypassing any shielding provided by the shell. Again, however, we saw no significant immune response in WBC counts of hatchlings.
That lack of response in Experiment #2 is surprising, because administration of LPS into an animal typically initiates a strong immune reaction (Lind et al., 2020; de Figueiredo et al., 2021). By exposing the individual to a component of bacterial cell walls, injection of LPS signals the presence of a pathogen, and thereby triggers a range of immunological and behavioral responses. In particular, WBC profiles often change in response to LPS injection, as specific cell types are recruited to fight the apparent challenge (Keller et al., 2006; Bowen et al., 2009). Why, then, did we find no difference in WBC profiles between treatment and control hatchlings in either of our experiments?
One possible explanation is that both egg yolk and albumin contain compounds with immune functions. Because reptile eggs are in close contact with soil microbiota, and typically without parental care (i.e., cleaning) of incubating eggs, shells and egg contents may have been under strong selection to develop antimicrobial capability (Gayen et al., 1977; Araki et al., 1998; Zimmerman et al., 2010; van Hoek, 2014). Compounds with antimicrobial abilities (e.g., lysozyme, defensin, ovotransferrin) have been isolated from reptile eggs and others likely remain to be identified (van Hoek, 2014). Furthermore, embryos may be chemically protected by specific maternally provisioned immune compounds (such as antibodies) based on the mother’s previous exposure to pathogens or her general health (Hasselquist et al., 2012). Such maternal effects on offspring immunocompetence have been documented in reptiles, but identifying the precise mechanisms through which such effects operate remains a nascent field (Uller et al., 2006; Itonaga et al., 2011; Brown and Shine, 2016). LPS may possibly be destroyed or altered by these compounds before reaching the embryo, thereby preventing any immune response involving WBC populations.
It remains possible that other components of the immune system were activated, without altering WBC profiles. Reptiles in general and snakes in particular are known to possess several extracellular immune mechanisms (Zimmerman et al., 2010). For example, natural antibodies and complement provide rapid non-specific protection against general common pathogens (Madsen et al., 2007; Brusch et al., 2019, 2020). Notably, exposing embryos to LPS produced significantly smaller offspring, consistent with energy allocation trade-offs. The nature of these trade-offs, and the specific components involved, remain to be identified.
Although our experimental treatments did not affect the WBC profiles of hatchling snakes, we did identify strong correlates of WBC variation among individuals. Notably, both the sex and body size of a hatchling snake affected its WBC profile, consistent with our previous research in keelbacks. Two earlier studies reported a negative correlation between H:L ratio and SVL in this species (Brown and Shine, 2016, 2018). This congruence among disparate studies suggest that the association is a consistent feature of keelback biology. The H:L ratio is often described as a stress leukogram because the number of heterophils relative to lymphocytes increases after exposure to known stressors (Davis et al., 2008; Davis and Maney, 2018). However, constitutive differences in H:L ratios among hatchlings might also reflect different allocation to “cheap” vs. “expensive” immune mechanisms (Brown and Shine, 2016).
If H:L ratio does indeed represent a stress response, our results suggest that the treatments we imposed in the present study were not stressful. The lack of stress response following injection of bacterial-derived compound (LPS) directly into the egg suggests that either the embryo’s immune system is not yet sufficiently well-developed to respond to such a challenge; or that as-yet-unknown defense mechanisms protect a growing embryo either by shielding it from these hostile incursions, or by buffering the effects of infective agents by changes other than those revealed by WBC counts.
Lastly, we address the implications of our results for anthropogenic disruptions to microbiota in the soil. As noted in the Introduction to this article, humans may modify the incubation-associated microbiota either inadvertently (e.g., through pollution) or intentionally (e.g., by translocating eggs to new nest sites, or by incubating eggs in sterile media). Our results suggest that (contrary to our a priori hypotheses), such manipulations do not affect immune-system function in hatchlings; but do affect hatchling body sizes, which in turn are strongly linked to immune-function parameters. In keelbacks (as is likely the case in many reptile populations), hatchling size is under strong directional selection in the field (Brown and Shine, 2004); and thus, managers need to evaluate the impacts of their manipulations on such traits in order to maximize the viability of hatchlings produced either in the field or the laboratory.
Data Availability Statement
The raw data supporting the conclusions of this article will be made available by the authors, without undue reservation.
Ethics Statement
The animal study was reviewed and approved by The University of Sydney Animal Ethics Committee.
Author Contributions
GB devised the study, gathered data, and conducted statistical analyses. Both authors drafted the manuscript.
Funding
This research received funding from the Australian Research Council (FT120100095 to GB).
Conflict of Interest
The authors declare that the research was conducted in the absence of any commercial or financial relationships that could be construed as a potential conflict of interest.
Publisher’s Note
All claims expressed in this article are solely those of the authors and do not necessarily represent those of their affiliated organizations, or those of the publisher, the editors and the reviewers. Any product that may be evaluated in this article, or claim that may be made by its manufacturer, is not guaranteed or endorsed by the publisher.
Acknowledgments
We thank the Northern Territory Land Corporation for providing facilities. AN and GC provided comments that improved the manuscript. The project was conducted under approvals issued by the Parks and Wildlife Commission of the Northern Territory (approval 47830) and The University of Sydney Animal Ethics Committee (approval # 2013/6010), and we adhered to all appropriate animal-welfare guidelines.
References
Amiel, J. J., and Shine, R. (2012). Hotter nests produce smarter young lizards. Biol. Lett. 8, 372–374. doi: 10.1098/rsbl.2011.1161
Araki, T., Yamamoto, T., and Torikata, T. (1998). Reptile lysozyme: the complete amino acid sequence of soft-shelled turtle lysozyme and its activity. Biosci. Biotechnol. Biochem. 62, 316–324. doi: 10.1271/bbb.62.316
Bodensteiner, B. L., Mitchell, T. S., Strickland, J. T., and Janzen, F. J. (2015). Hydric conditions during incubation influence phenotypes of neonatal reptiles in the field. Funct. Ecol. 29, 710–717. doi: 10.1111/1365-2435.12382
Bonneaud, C., Mazuc, J., Gonzalez, G., Haussy, C., Chastel, O., Faivre, B., et al. (2003). Assessing the cost of mounting an immune response. Am. Nat. 161, 367–379. doi: 10.1086/346134
Bowen, O. T., Dienglewicz, R. L., Wideman, R. F., and Erf, G. F. (2009). Altered monocyte and macrophage numbers in blood and organs of chickens injected i.v. with lipopolysaccharide. Vet. Immunol. Immunopathol. 131, 200–210. doi: 10.1016/j.vetimm.2009.04.010
Brown, G. P., and Shine, R. (2002). Reproductive ecology of a tropical natricine snake, Tropidonophis mairii (Colubridae). J. Zool. 258, 63–72. doi: 10.1017/S0952836902001218
Brown, G. P., and Shine, R. (2004). Maternal nest-site choice and offspring fitness in a tropical snake (Tropidonophis mairii, Colubridae). Ecology 85, 1627–1634. doi: 10.1890/03-0107
Brown, G. P., and Shine, R. (2005b). Nesting snakes (Tropidonophis mairii, Colubridae) selectively oviposit in sites that provide evidence of previous successful hatching. Can. J. Zool. 83, 1134–1137. doi: 10.1139/Z05-115
Brown, G. P., and Shine, R. (2005a). Do changing moisture levels during incubation influence phenotypic traits of hatchling snakes (Tropidonophis mairii, Colubridae)? Physiol. Biochem. Zool. 78, 524–530. doi: 10.1086/430231
Brown, G. P., and Shine, R. (2006a). Effects of nest temperature and moisture on phenotypic traits of hatchling snakes (Tropidonophis mairii, Colubridae) from tropical Australia. Biol. J. Linn. Soc. 89, 159–168. doi: 10.1111/j.1095-8312.2006.00669.x
Brown, G. P., and Shine, R. (2006b). Why do most tropical animals reproduce seasonally? Testing hypotheses on an Australian snake. Ecology 87, 133–143. doi: 10.1890/04-1882
Brown, G. P., and Shine, R. (2007a). Like mother, like daughter: inheritance of nest-site location in snakes. Biol. Lett. 3, 131–133. doi: 10.1098/rsbl.2006.0605
Brown, G. P., and Shine, R. (2007b). Repeatability and heritability of reproductive traits in free-ranging snakes. J. Evol. Biol. 20, 588–596. doi: 10.1111/j.1420-9101.2006.01256.x
Brown, G. P., and Shine, R. (2009). Beyond size–number trade-offs: clutch size as a maternal effect. Philos. Trans. R. Soc. B 364, 1097–1106. doi: 10.1098/rstb.2008.0247
Brown, G. P., and Shine, R. (2016). Maternal body size influences offspring immune configuration in an oviparous snake. R. Soc. Open Sci. 3:160041. doi: 10.1098/rsos.160041
Brown, G. P., and Shine, R. (2018). Immune configuration in hatchling snakes is affected by incubation moisture, and is linked to subsequent growth and survival in the field. J. Exp. Zool. A 329, 222–229. doi: 10.1002/jez.2154
Brown, G. P., Madsen, T. R., and Shine, R. (2017). Resource availability and sexual size dimorphism: differential effects of prey abundance on the growth rates of tropical snakes. Funct. Ecol. 31, 1592–1599. doi: 10.1111/1365-2435.12877
Brusch, G. A., Heulin, B., and DeNardo, D. F. (2019). Dehydration during egg production alters egg composition and yolk immune function. Comp. Biochem. Physiol. A 227, 68–74. doi: 10.1016/j.cbpa.2018.10.006
Brusch, G. A., Walman, R. M., Mills, A. M., Masuda, G., Byeon, A., DeNardo, D. F., et al. (2020). Dehydration enhances cellular and humoral immunity in a mesic snake community. J. Exp. Zool. 333, 306–315. doi: 10.1002/jez.2358
D’Alba, L., and Shawkey, M. D. (2015). Mechanisms of antimicrobial defense in avian eggs. J. Ornithol. 156, 399–408.
Davis, A. K., and Maney, D. L. (2018). The use of glucocorticoid hormones or leucocyte profiles to measure stress in vertebrates: what’s the difference? Methods Ecol. Evol. 9, 1556–1568. doi: 10.1111/2041-210X.13020
Davis, A. K., Maney, D. L., and Maerz, J. C. (2008). The use of leukocyte profiles to measure stress in vertebrates: a review for ecologists. Funct. Ecol. 22, 760–772. doi: 10.1111/j.1365-2435.2008.01467.x
de Figueiredo, A. C., Titon, S. C., Titon, B. Jr., Vasconcelos-Teixeira, R., Barsotti, A. M., and Gomes, F. R. (2021). Systemic hormonal and immune regulation induced by intraperitoneal LPS injection in bullfrogs (Lithobates catesbeianus). Comp. Biochem. Physiol. A 253:110872. doi: 10.1016/j.cbpa.2020.110872
Deen, C. M., and Hutchison, V. H. (2001). Effects of lipopolysaccharide and acclimation temperature on induced behavioral fever in juvenile Iguana iguana. J. Therm. Biol. 26, 55–63.
do Amaral, J. P. S., Marvin, G. A., and Hutchison, V. H. (2002). The influence of bacterial lipopolysaccharide on the thermoregulation of the box turtle Terrapene carolina. Physiol. Biochem. Zool. 75, 273–282.
Doherty, T. S., Balouch, S., Bell, K., Burns, T. J., Feldman, A., Fist, C., et al. (2020). Reptile responses to anthropogenic habitat modification: a global meta-analysis. Glob. Ecol. Biogeogr. 29, 1265–1279. doi: 10.1111/geb.13091
Dominguez-Bello, M. G., De Jesus-Laboy, K. M., Shen, N., Cox, L. M., Amir, A., Gonzalez, A., et al. (2016). Partial restoration of the microbiota of cesarean-born infants via vaginal microbial transfer. Nat. Med. 22:250. doi: 10.1038/nm.4039
Doody, J. S. (2011). Environmentally cued hatching in reptiles. Integr. Comp. Biol. 51, 49–61. doi: 10.1093/icb/icr043
Francis, E. A., Moldowan, P. D., Greischar, M. A., and Rollinson, N. (2019). Anthropogenic nest sites provide warmer incubation environments than natural nest sites in a population of oviparous reptiles near their northern range limit. Oecologia 190, 511–522. doi: 10.1007/s00442-019-04383-3
Gayen, S. K., Som, S., Sinha, N. K., and Sen, A. (1977). Lysozyme in egg whites of tortoises and turtle: purification and properties of egg white lysozyme of Trionyx gangeticus cuvier. Arch. Biochem. Biophys. 183, 432–442. doi: 10.1016/0003-9861(77)90378-2
Georges, A., Beggs, K., Young, J. E., and Doody, J. S. (2005). Modelling development of reptile embryos under fluctuating temperature regimes. Physiol. Biochem. Zool. 78, 18–30. doi: 10.1086/425200
Grindstaff, J. L., Hasselquist, D., Nilsson, J. Å, Sandell, M., Smith, H. G., and Stjernman, M. (2006). Transgenerational priming of immunity: maternal exposure to a bacterial antigen enhances offspring humoral immunity. Proc. R. Soc. B 273, 2551–2557. doi: 10.1098/rspb.2006.3608
Hasselquist, D., Tobler, M., and Nilsson, J. Å (2012). Maternal Modulation Of Offspring Immune Function In Vertebrates. Oxford: Oxford University Press, 165–224.
Itonaga, K., Jones, S. M., and Wapstra, E. (2011). Effects of variation in maternal carotenoid intake during gestation on offspring innate immune response in a matrotrophic viviparous reptile. Funct. Ecol. 25, 1318–1326.
Jacobson, E. R. (2007). “Bacterial diseases of reptiles,” in Infectious Diseases and Pathology of Reptiles, ed. E. R. Jacobsen (Boca Raton, FL: CRC Press), 475–540.
Jarvie, S., Besson, A. A., Seddon, P. J., and Cree, A. (2014). Assessing thermal suitability of translocation release sites for egg-laying reptiles with temperature-dependent sex determination: a case study with tuatara. Anim. Conserv. 17, 48–55. doi: 10.1111/acv.12152
Keller, J. M., Peden-Adams, M. M., and Aguirre, A. A. (2006). “Immunotoxicology and implications for reptilian health,” in Toxicology of Reptiles, eds S. C. Gardner and E. Oberdörster (Boca Raton, FL: CRC Press), 199–240.
Lind, C. M., Agugliaro, J., and Farrell, T. M. (2020). The metabolic response to an immune challenge in a viviparous snake, Sistrurus miliarius. J. Exp. Biol. 223, jeb225185.
Löwenborg, K., Shine, R., and Hagman, M. (2011). Fitness disadvantages to disrupted embryogenesis impose selection against suboptimal nest-site choice by female grass snakes, Natrix natrix (Colubridae). J. Evol. Biol. 24, 177–183. doi: 10.1111/j.1420-9101.2010.02153.x
Madsen, T., Ujvari, B., Nandakumar, K. S., Hasselquist, D., and Holmdahl, R. (2007). Do “infectious” prey select for high levels of natural antibodies in tropical pythons? Evol. Ecol. 21, 271–279. doi: 10.1007/s10682-006-9004-4
Mitchell, N. J., Rodriguez, N., Kuchling, G., Arnall, S. G., and Kearney, M. R. (2016). Reptile embryos and climate change: modelling limits of viability to inform translocation decisions. Biol. Conserv. 204, 134–147. doi: 10.1016/j.biocon.2016.04.004
Moreira, P. L., and Barata, M. (2005). Egg mortality and early embryo hatching caused by fungal infection of Iberian rock lizard (Lacerta monticola) clutches. Herpetol. J. 15, 265–272.
Noble, D. W., Stenhouse, V., and Schwanz, L. E. (2018). Developmental temperatures and phenotypic plasticity in reptiles: a systematic review and meta-analysis. Biol. Rev. 93, 72–97. doi: 10.1111/brv.12333
Nowak, A., and Wronkowska, H. (1987). On the efficiency of soil sterilization in autoclaves. Zentralbl. Mikrobiol. 142, 521–525. doi: 10.1016/S0232-4393(87)80062-8
Packard, M. J., and DeMarco, V. G. (1991). “Eggshell structure and formation in eggs of oviparous reptiles,” in Egg Incubation: Its Effects on Embryonic Development in Birds and Reptiles, ed. D. C. Deeming (Cambridge: Cambridge University Press), 53–69.
Shine, R. (1983). Reptilian reproductive modes: the oviparity-viviparity continuum. Herpetologica 39, 1–8.
Shine, R., and Brown, G. P. (2002). Effects of seasonally varying hydric conditions on hatchling phenotypes of keelback snakes (Tropidonophis mairii, Colubridae) from the Australian wet–dry tropics. Biol. J. Linn. Soc. 76, 339–347. doi: 10.1046/j.1095-8312.2002.00068.x
Shine, R., and Olsson, M. (2003). When to be born? Prolonged pregnancy or incubation enhances locomotor performance in neonatal lizards (Scincidae). J. Evol. Biol. 16, 823–832. doi: 10.1046/j.1420-9101.2003.00600.x
Singh, S. K., Das, D., and Rhen, T. (2020). Embryonic temperature programs phenotype in reptiles. Front. Physiol. 11:35. doi: 10.3389/fphys.2020.00035
Tefera, T., and Vidal, S. (2009). Effect of inoculation method and plant growth medium on endophytic colonization of sorghum by the entomopathogenic fungus Beauveria bassiana. BioControl 54, 663–669. doi: 10.1007/s10526-009-9216-y
Uller, T., Isaksson, C., and Olsson, M. (2006). Immune challenge reduces reproductive output and growth in a lizard. Funct. Ecol. 20, 873–879.
van Hoek, M. L. (2014). Antimicrobial peptides in reptiles. Pharmaceuticals 7, 723–753. doi: 10.3390/ph7060723
Warner, D. A., Moody, M. A., and Telemeco, R. S. (2011). Is water uptake by reptilian eggs regulated by physiological processes of embryos or a passive hydraulic response to developmental environments? Comp. Biochem. Physiol. A 160, 421–425. doi: 10.1016/j.cbpa.2011.07.013
Webb, J. K., Brown, G. P., and Shine, R. (2001). Body size, locomotor speed and antipredator behaviour in a tropical snake (Tropidonophis mairii, Colubridae): the influence of incubation environments and genetic factors. Funct. Ecol. 15, 561–568. doi: 10.1046/j.0269-8463.2001.00570.x
Wellehan, J. F., and Johnson, A. J. (2005). Reptile virology. Vet. Clinics Exotic Anim. Pract. 8, 27–52. doi: 10.1016/j.cvex.2004.09.006
While, G. M., Noble, D. W., Uller, T., Warner, D. A., Riley, J. L., Du, W. G., et al. (2018). Patterns of developmental plasticity in response to incubation temperature in reptiles. J. Exp. Zool. A 329, 162–176. doi: 10.1002/jez.2181
Wolmarans, K., and Swart, W. J. (2014). Influence of glyphosate, other herbicides and genetically modified herbicide-resistant crops on soil microbiota: a review. South Afr. J. Plant Soil 31, 177–186. doi: 10.1080/02571862.2014.960485
Ygberg, S., and Nilsson, A. (2012). The developing immune system – from foetus to toddler. Acta Paediatr. 101, 120–127. doi: 10.1111/j.1651-2227.2011.02494.x
Zając, M., Skarżyńska, M., Lalak, A., Kwit, R., Śmiałowska-Węglińska, A., Pasim, P., et al. (2021). Salmonella in captive reptiles and their environment—can we tame the dragon? Microorganisms 9:1012.
Keywords: lipopolysaccharide, LPS, leukogram, heterophil, immunocompetence, lymphocyte, Natricidae
Citation: Brown GP and Shine R (2022) Do Microbiota in the Soil Affect Embryonic Development and Immunocompetence in Hatchling Reptiles? Front. Ecol. Evol. 9:780456. doi: 10.3389/fevo.2021.780456
Received: 21 September 2021; Accepted: 14 December 2021;
Published: 05 January 2022.
Edited by:
Jeanine M. Refsnider, University of Toledo, United StatesReviewed by:
Gábor Árpád Czirják, Leibniz Institute for Zoo and Wildlife Research (LG), GermanyAlfredo G. Nicieza, University of Oviedo, Spain
Copyright © 2022 Brown and Shine. This is an open-access article distributed under the terms of the Creative Commons Attribution License (CC BY). The use, distribution or reproduction in other forums is permitted, provided the original author(s) and the copyright owner(s) are credited and that the original publication in this journal is cited, in accordance with accepted academic practice. No use, distribution or reproduction is permitted which does not comply with these terms.
*Correspondence: Gregory P. Brown, greg.brown@mq.edu.au