- 1WasserCluster Lunz - Biologische Station, Inter-University Center for Aquatic Ecosystem Research, Lunz am See, Austria
- 2Department for Biomedical Research, Donau-Universität Krems, Krems an der Donau, Austria
- 3Department of Geological Science, University of Saskatchewan, Saskatoon, SK, Canada
- 4Department of Biology, University of Western Ontario, London, ON, Canada
- 5Centro de Investigaciones en Geografía Ambiental, Universidad Nacional Autónoma de México, Morelia, Mexico
- 6Environment and Climate Change Canada, Saskatoon, SK, Canada
Introduction: Among long-distance migratory insects, the monarch butterfly (Danaus plexippus) is one of the most iconic, whose journey is fueled by nectar from flowering plants along the migratory route which may involve up to 3,500 km. Understanding how and where monarchs obtain their dietary resources to fuel migratory flight and ensure overwintering stores would provide new insights into the migratory strategy of this species and subsequently help focus conservation efforts.
Methods: This pilot study was designed as a first attempt to assess the composition, dynamics, and isotopic (δ2H, δ13C) composition of essential and non-essential fatty acids (FA) acquired or manufactured de novo from larval host milkweed (Asclepias spp.) by monarch butterflies and from adult emergence to overwintering.
Results: Data from controlled laboratory isotopic tracer tests suggested that adult monarchs convert their dietary energy mainly into 16:0 and 18:1 fatty acids and store them as neutral lipids in their abdomen. FA isotopic composition reflects not only dietary sources but also subsequent isotopic fractionation from metabolism. On the other hand, δ2H values of essential omega-3 fatty acid alpha-linolenic acid (ALA) correlated with δ2HWing, as markers of an individual’s geographic origin and indicated the importance of larval diet. Additionally, in wild-type females, high isotopic fractionation in δ13CALA between neutral and polar lipids might indicate increased bioconversion activity during gravidity. Finally, δ2HLIN showed positive H isotope fractionation from larval dietary sources, indicating that catabolic processes were involved in their manufacture. Furthermore, δ2HLIN showed a negative correlation with δ2HWing values, which could potentially be useful when investigating individual life-history traits, such as migratory catabolic efforts or periods of fasting.
Discussion: This interpretation was supported by significant larger variations in δ2HLIN and δ2HLIN overwintering monarchs compared to other FA. Altogether, our results provide the first evidence that the H isotopic analysis of individual fatty acids in migrating and overwintering monarchs can be used to infer the nutritional history of individuals including the provenance of nectaring sites used to fuel key life history events.
Introduction
Migratory animals have evolved numerous morphometric, physiological, and behavioral adaptations to successfully achieve seasonal habitat movements, often involving impressive transcontinental passages (Dingle, 2014; Hobson et al., 2019). Independent of biome, vertebrate, and invertebrate adult migrants using terrestrial and aquatic habitats fuel their migratory journeys using energy-dense lipids, mainly triacyclglycerols (storage lipids), which are acquired directly from diet or are synthesized de novo, for example, from carbohydrates (McWilliams et al., 2004). Fats are typically accumulated and stored to fuel the entire migratory episode, or are obtained at stopover refueling sites. The dietary quality for animals at pre-migratory staging sites and along stopovers used during migration, along with the ability of animals to convert food into fuel, are crucial factors influencing the overall success of their migration cycle (Lennox et al., 2016).
Among long-distance migratory insects, the monarch butterfly (Danaus plexippus) is the most iconic. The eastern population of this species in North America undergoes a continental-scale fall migration of up to 3,500 km to overwinter in high altitude (3,000–4,000 m.a.s.l.) colonies in the oyamel (Abies religiosa) forests of the transvolcanic belt of central Mexico (Slayback et al., 2007). After reaching the overwintering colony, monarchs fast and rely on their accumulated fats to sustain them from December to March. Only successful gravid females begin re-migration north in the springtime reaching as far as Texas where they oviposit on milkweed (Asclepia sp.) plants. Successive generations continue northward, making use of newly available milkweed until the late summer cohort of individuals is produced, many of which migrate south to Mexico. The monarch’s fall migratory journey is fueled by nectar (Brown and Chippendale, 1974; Brower et al., 2006) obtained from flowering plants along the migratory route (Beall, 1948; Gibo and McCurdy, 1993; Brower et al., 2006). It has been proposed that nectaring resources in Texas provide the bulk of the lipids required to sustain both overwintering and subsequent reproduction the following spring (Brower, 1985). However, in a year of record drought in Texas (Brower et al., 2015) found that butterflies collected there contained lipid contents well below normal, while butterflies collected from overwintering sites had higher contents. They hypothesized that there should be other areas in Mexico where they could have access to abundant nectar. Recently, Hobson et al. (2020) provided hydrogen isotopic evidence contrasting total lipid δ2H and wing δ2H values that suggested migrant monarchs acquire overwintering storage lipids much closer to their winter roost sites than expected, rather than gradual by accumulation along the migratory route. Understanding where and how monarchs obtain the dietary fat resources to fuel migratory flight and to ensure overwintering stores would provide new insights into the migratory strategy of this species and subsequently inform conservation strategies (Brower et al., 2006).
Besides non-essential saturated and monounsaturated fatty acids composing the majority of energy storage in lipids, attention has turned to polyunsaturated fatty acids (PUFA) in animal nutritional ecology, and especially omega-3 polyunsaturated fatty acids (n-3 PUFA), such as the essential alpha-linolenic acid (18:3n-3, ALA), as well as the conditionally indispensable eicosapentaenoic acid (20:5n-3, EPA) and docosahexaenoic acid (22:6n-3, DHA), which are physiologically crucial but of limited dietary availability in nature (reviewed by Twining et al., 2016). Animals vary in their ability to convert essential ALA into EPA and DHA but they cannot synthesize ALA de novo. Currently, our understanding of animal lipid biochemistry and physiology is biased to marine food webs (Baird, 2022), with far less information available for freshwater, terrestrial, or insect food webs (but see Twining et al., 2016; Génier et al., 2021; Parmar et al., 2022). Little attention has been given to understanding the interactions between the physiological demands of long-distance migration (e.g., for insects, birds), spatial origin of lipids and subsequent storage, utilization, and conservation and dynamics of PUFA and the essential precursors such as linoleic acid (18:2n-6, LIN) and ALA.
Increasingly, efforts are underway to gain a better understanding of the spatio-temporal environmental conditions experienced by migrating monarchs (and other animals) during migration with respect to understanding population demographic trends and conservation (Saunders et al., 2019; Taylor et al., 2019). The findings of Hobson et al. (2020) were based on H isotopic measurements of “total” lipids in migrant monarchs, which for adult monarchs predominantly reflects the abundant non-essential oleic (18:1n-9) and palmitic acids (16:0) (Cenedella, 1971). Here we explore the idea that fatty acid profiles combined with compound-specific fatty acid isotopic (δ13C and δ2H) analyses of storage lipids could provide new information on energy provenance because lipids are composed of carbon and hydrogen atoms derived from spatially-distributed plant nectars [see West et al. (2008, 2010) regarding plant-based H and C isoscape patterns]. We hypothesized that the essential PUFA (i.e., ALA and LIN) would be derived exclusively from the larval milkweed host plant diet, because no notable amounts are taken up by the nectar feeding adults, and these PUFA should retain the isotopic composition of their geographical provenance (O’Brien et al., 2000; but see Levin et al., 2017), whereas non-essential fatty acids, such as palmitic acid (16:0), stearic acid (18:0), or oleic acid (18:1n-9) would be synthesized from dietary sources like nectar taken up along the migratory route, and simultaneously being used during flight. However, carbon and hydrogen isotope discrimination of stored fatty acids through mobilization kinetics (e.g., burning of fat) and by other biochemical processes are possible and could complicate isotopic-based inferences of these fatty acid spatial origins (Soto et al., 2013). Regardless, the measurement of the stable isotopic composition of stored lipids could be a powerful new method to infer origins of nectaring sites of migratory insects and potentially (re)fueling sites for migratory birds, other insects, and vertebrates (Hobson et al., 2020).
We designed this pilot study in a first attempt to assess the composition, trophic dynamics (i.e., trophic discrimination factors), and isotopic (δ2H and δ13C) structure of essential and non-essential fatty acids acquired or manufactured de novo from larval hostplant milkweed by monarch butterflies from adult emergence stage to overwintering. We used controlled captive experiments to raise monarchs from eggs on swamp (A. incarnata) and common (A. syriaca) milkweed hostplants to the eclosed adult butterfly. This experiment allowed us to examine stable isotopic relationships between the natal host plant and larval dietary fatty acids and those in newly emerged adults. With later diet-switching of newly eclosed adults to an isotopically spiked (δ2H) nectar we were able to infer which FAs were produced de novo and which were obtained directly via the larvae from the larval milkweed hostplant.
Using analyses of polar and neutral lipids, the compositional and isotopic structure of fatty acids derived from the head and thorax (i.e., functional compartments) were compared to those in the abdominal fat body (i.e., storage compartment) of the captive-raised monarchs. We examined the lipid composition and isotopic (δ13C and δ2H) structure of a small sample (n = 20) of wild monarchs obtained from an overwintering colony in Mexico in February 2022, and correlated wing δ2H measurements (e.g., proxy for migratory distance) to individual fatty acids to determine whether δ2H values of larval-derived essential fatty acids also provided information on spatial origins of individuals (Wassenaar and Hobson, 1998; Flockhart et al., 2013). The isotopic structure (δ13C and δ2H) of non-essential fatty acids, which were presumably derived from southern nectaring sites close to the winter colonies (Hobson et al., 2020) were examined to evaluate their potential for spatial inferences of where monarchs acquired the key storage lipids required to sustain overwintering.
Materials and methods
Captive rearing and tracer experiments
In a first experiment, swamp milkweed was grown in planter pots in a greenhouse and randomly assigned to two groups (10 pots per treatment). One group was watered for 4 weeks using tap water (∼δ2H-54‰) and the other on water spiked with 99% deuterated water (Sigma-Aldrich) to achieve a watering δ2H value of ∼+350‰. Milkweed leaves from these spiked and tap water groups were thereafter used to raise monarchs from eggs through the larval stage and pupa to the eclosed adult monarch. Eclosed adult monarchs (n = 8 per treatment) were sampled immediately after emergence without any subsequent nectaring.
In a second experiment, monarchs were raised on a single batch of wild-sourced common milkweed from Manitoulin Island, ON, Canada. Similar to those raised in captivity on the swamp milkweed, we sampled eclosed adults without any nectaring. A second group of the emerged adults were kept for seven additional days and fed a 2H spiked nectar consisting of a 3:1 water:sugar solution using spiked dilution water (δ2H = ∼+350‰).
Adult monarchs were euthanized and stored in a −80°C freezer and freeze-dried before conducting isotopic analyses. Subsamples of milkweed leaves representing (equally) all treatments were freeze-dried and powdered for subsequent stable isotope and lipid analyses.
Overwintering monarchs (Mexico)
A small sampling of 20 (10 M and 10 F) overwintering monarchs were collected live in late January 2022, at the Sierra Chincua wintering colony in Michoacan, Mexico. These wild monarch samples were transported cold on the collection day to the UNAM-Campus Morelia where they were euthanized and immediately freeze dried. These monarchs were stored whole at −20°C before being transported to the laboratory for lipid composition and stable isotopic analysis.
Monarch wing H isotopic analyses
Samples (0.35 ± 0.02 mg) of monarch wing chitin were weighed into 3.5 mm × 5 mm silver capsules, placed in a Uni-Prep autosampler (Eurovector, Milan, Italy, Wassenaar et al., 2015) and subsequently analyzed in a Eurovector 3000 Elemental Analyzer, coupled to a Thermo Delta V Plus isotope ratio mass spectrometer (Bremen, Germany) in continuous flow mode at the University of Western Ontario. After the samples were loaded, the Uniprep autosampler was heated to 60°C and evacuated and subsequently flushed with dry helium to remove adsorbed atmospheric moisture from the samples. Two USGS keratin standards, CBS: Caribou Hoof Standard and KHS: Kudu Horn Standard were included every ten samples. Samples were combusted at 1,350°C using a glassy carbon reactor. Values of δ2H of non-exchangeable hydrogen were derived using the comparative equilibration approach of Wassenaar and Hobson (2003) and calibrated to Vienna Standard Mean Ocean Water (VSMOW) using CBS (±1.9‰ 1 SD, n = 18, accepted δ2H = −197.0‰) and KHS (±1.6‰, n = 17, accepted δ2H = −54.1‰). Overall (within-run) measurement error for CBS and KHS δ2H was <∼2‰.
For the laboratory experiments, the δ2H analyses of water were done using a DLT-100 laser spectrometer (ABB/Los Gatos Research, Mountain View, CA, USA) using laboratory standards INV1 δ2H = −217.7‰ and ROD3 δ2H = −3.9‰, respectively, to normalize delta values to the VSMOW-SLAP scale. Accuracy as determined by replicate analyses of samples and reference waters was <±1 and 0.1‰, respectively.
Fatty acid quantification and stable isotope analysis
Lipids of the selected monarch body tissues and milkweed hostplants were extracted as in Heissenberger et al. (2010) and the lipid content was determined gravimetrically. Briefly, freeze-dried monarch and milkweed samples were homogenized and mixed with chloroform:methanol (2:1 vol/vol) and after sonication, vortexed and centrifuged three times to remove non-lipid material. Solvent-extracted lipids were evaporated to a final volume of 1.5 mL under N2 flow. Lipids were then separated into neutral lipids (NL), free-fatty acids (FF), and polar lipids (PL) using solid phase extraction (SPE; Bond Elut LRC–SI, Agilent Technologies, Santa Clara, CA, USA). The SPE columns were conditioned using hexane and loaded using 0.5 mL of the sample extract. NL were eluded using Chloroform:2-propanol (2:1 v/v), followed by elution of the FF using 2% acetic acid in diethyl ether and PL using methanol (Kaluzny et al., 1985). For fatty acid methyl ester (FAME) formation, lipid samples were incubated with a sulfuric acid:methanol mixture (1:100 vol/vol) for 16 h at 50°C, following the addition of KHCO3 and hexane. Samples were shaken, vortexed, and centrifuged and the upper organic layers collected, pooled, and concentrated under N2 gas flow.
All δ13C and δ2H analyses of FA were performed following the analytical methodology described previously (Pilecky et al., 2021a). Briefly, a Thermo Trace 1310 GC (ThermoFisher Scientific, Waltham, MA, USA) was connected via a ConFlo IV combustion or reduction interface (ThermoFisher Scientific) to an Isotope Ratio Mass Spectrometer (IRMS, DELTA V Advantage, ThermoFisher Scientific). FAMEs were separated using a VF-WAXms 30 m column, 0.32 mm ID, film thickness 1 μm (Agilent) and then for δ13C analysis oxidized to CO2 gas in a combustion reactor, filled with Ni, Pt, and Cu wires, at a temperature of 1,000°C, or for δ2H analysis reduced to H2 gas by passing through a high thermal conversion (HTC) ceramic reactor at 1,420°C.
Lipid samples were reference scale-normalized using three-point calibration with FAME stable isotope (Me-C20:0) reference materials (USGS70: δ13C = –30.53‰, δ2H = –183.9‰, USGS71: δ13C = –10.5‰, δ2H = –4.9‰, and USGS72: δ13C = –1.54‰, δ2H = +348.3‰). FA δ13C and δ2H values (δIFA) were corrected for methylation according to the formula (Pilecky et al., 2021a):
Where δIFAME are the δ2H or δ13C values of the measured FAME and δIMeOH the δ2H or δ13C values of the methanol used during methylation and n equals the total number of H-/C-atoms of the FAME molecule. Values for δ13C are referenced to Vienna PeeDee Belemite (VPDB).
Values for δ2H are standardized against Vienna Standard Mean Ocean Water (VSMOW).
Data analysis
All data and graphical analyses were performed in R (Version 4.1.0) using the packages rstatix, ggplot2, and ggpubr. Data were presented as mean ± standard deviation including propagated errors wherever applicable. A student’s t-test, or one-way ANOVA with Tukey post-hoc test was used for two or multiple group comparison, respectively. The Pearson method was used for linear correlation analysis. Z-scores for individual FA relative abundance (% of total FAME of a sample) were calculated by normalization to the global study mean and standard deviation within this study.
Results
Fatty acid profiles of milkweed, wild, and captive monarchs
Milkweed host plant leaves had an average lipid content of 120.5 ± 12.3 μg mg–1 of dry weight. The most abundant FA in milkweed leaves were palmitic acid 16:0 (11.3 ± 6.6 μg mg–1) and ALA (10.3 ± 5.8 μg mg–1), followed by LIN (2.7 ± 1.5 μg mg–1), 18:1n-9 (2.2 ± 0.9 μg mg–1), 18:0 (1.6 ± 0.9 μg mg–1), and 16:1n-7 (0.7 ± 0.6 μg mg–1). Furthermore, only traces of 16:1n-9, GLA, 18:1n-7, and 14:0 could be identified.
For monarchs, the lipid content was dependent on the sample type (ANOVA, F4,31 = 19.8, p < 0.001). The lipid content of overwintering wild monarchs was approximately double for females (628 ± 208 μg mg–1) compared to males (313 ± 136 μg mg–1, Tukey, p < 0.001). Adults eclosed under controlled laboratory conditions and without any subsequent nectaring began life with an average lipid content of 136 μg mg–1 ± 52, and the abdomen had twice the lipid content (214 ± 54 μg mg–1) of the head/thorax tissue (94 ± 10 μg mg–1).
Neutral lipids (NL) were positively correlated with total lipids (R2 = 0.5, p < 0.001). The marked increase in the NL fraction of the wild individuals was due to the accumulation of 16:0 and 18:1n-9. The phospholipid (PL) mass fraction was slightly higher in the captive monarch adults (13.8 ± 3.2 μg mg–1) compared to the overwintering individuals (female: 9.7 ± 4.6 μg mg–1, male: 10.6 ± 3.7 μg mg–1), with head and thorax tissue particularly rich in PL (21.2 ± 9.7 μg mg–1).
Mass fractions of LIN (t-test, p < 0.001), ALA (t-test, p < 0.001) and EPA (t-test, p < 0.001) were significantly higher in the captive monarchs compared to wild overwintering individuals. The EPA content did not differ (paired t-test, p = 0.75) between NL and PL, however, higher mass fractions were found in the newly emerged adults (∼0.4 μg mg–1) compared to wild overwintering monarchs (∼0.1 μg mg–1), as well as having higher mass fractions in the head/thorax tissue (∼0.4 μg mg–1) compared to the abdomen (∼0.1 μg mg–1). Other PUFA detected in trace amounts were gamma-linoleic acid (GLA, 18:3n-6), 20:3n-3, and 20:4n-6, however, no SDA or PUFA with >20 carbon atoms were found (Table 1 and Figure 1). In total, six fatty acids were found to be abundant enough to allow for compound-specific stable isotope analysis: 16:0, 16:1 (sum of all isomers), 18:0, 18:1 (sum of all isomers), LIN and ALA. The C and H isotopic composition of EPA could only be measured in the PL fraction.
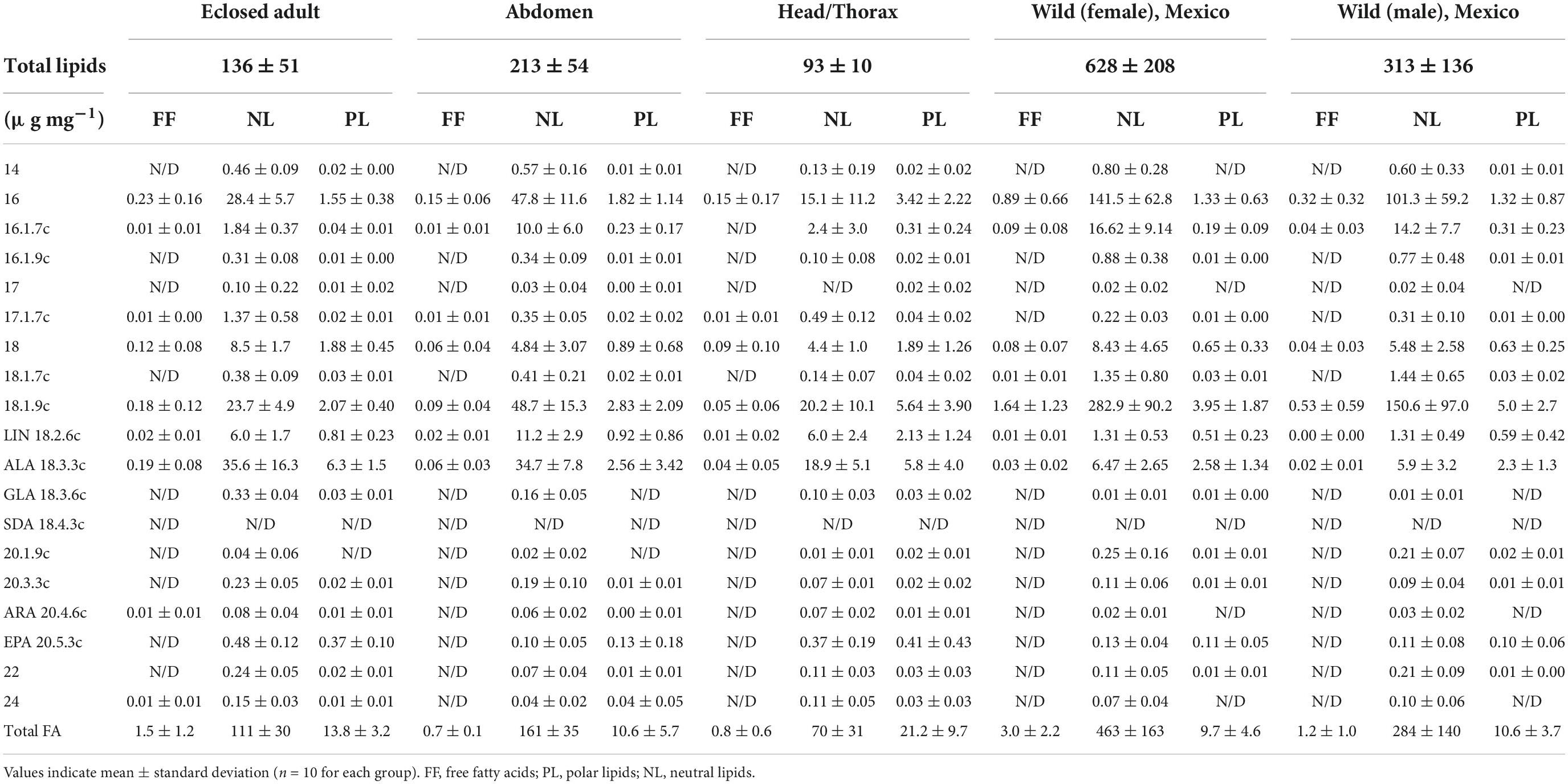
Table 1. Fatty acid mass fractions (μg mg–1) in individual lipid classes for monarchs raised in captivity and over-wintering in Mexico.
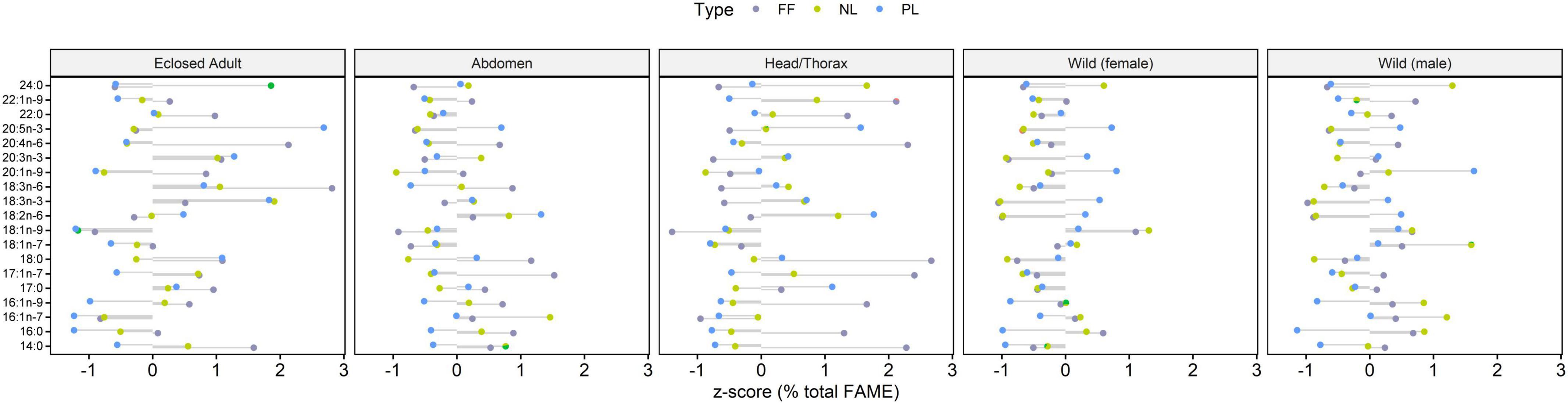
Figure 1. Z-scores (for all samples in this study) of relative fatty acid abundance (% total FAME) for each sample and corresponding mass fractions (μg mg–1 dw). Wild monarchs, especially females, contained higher lipid fractions than males or monarchs raised in the lab, in which the abdomen had ca. twice the lipid content compared to the head/thorax region. Polar lipids (PL) content was comparable for all monarchs but particularly concentrated in head/thorax. Neutral lipids (NL) correlated with lipid content, mainly driven by the increase in 16:0 and 18:1n-9. Notably, EPA was concentrated in PL, while 18:1 and 24 were enriched in the NL. Relative EPA contents in the PL were lower in freshly emerged monarchs for both head/thorax and abdomen compared to those migrant individuals overwintering in Mexico. Relative fatty acid content of LIN and ALA was comparable between NL and PL.
Isotope fractionation in fatty acids of freshly eclosed adults compared to larval diet
The captive-raised milkweed and monarchs treated with 2H enriched (∼+350‰) water resulted in a ∼50–100‰ increase in the δ2H values of the fatty acids of milkweed and correspondingly of the fatty acids of monarch larvae, as reflected in the eclosed non-nectaring adults feeding on them (Figure 2A). Non-essential FA were significantly lower in δ2H values in the newly eclosed adults compared to larval milkweed diet (Δδ2H: 16:0: −79.7 ± 23.8‰; 16:1: −193.2 ± 31.5‰; 18:0: −72.5 ± 20.2‰; 18:1: −251.0 ± 26.8‰; all t-test, p < 0.001), while LIN was significantly enriched (Δδ2H +54.8 ± 12.4‰; t-test, p < 0.001). ALA showed a slight but insignificant positive trend (Δδ2H 7.8 ± 18.2‰; t-test, p = 0.665). There was no significant difference in the δ2H values between fatty acids of NL and PL of eclosed adults, except for 16:0, which were slightly enriched in 2H in PL compared to NL (Δδ2H −241.9 ± 5.9 vs. −267.8 ± 11.2‰, t-test, p = 0.007). The δ2H values of EPA could only be measured in PL and were approximately 40‰ higher compared to ALA when feeding on leaves from milkweed raised on tap water (Δδ2H −111.1 ± 7.7 vs. −149.7 ± 3.2‰) and ca. 60‰ enriched in individuals feeding on 2H-enriched leaves (Δδ2H −46.3 ± 0.8 vs. −111.8 ± 0.8‰) (Figure 2A).
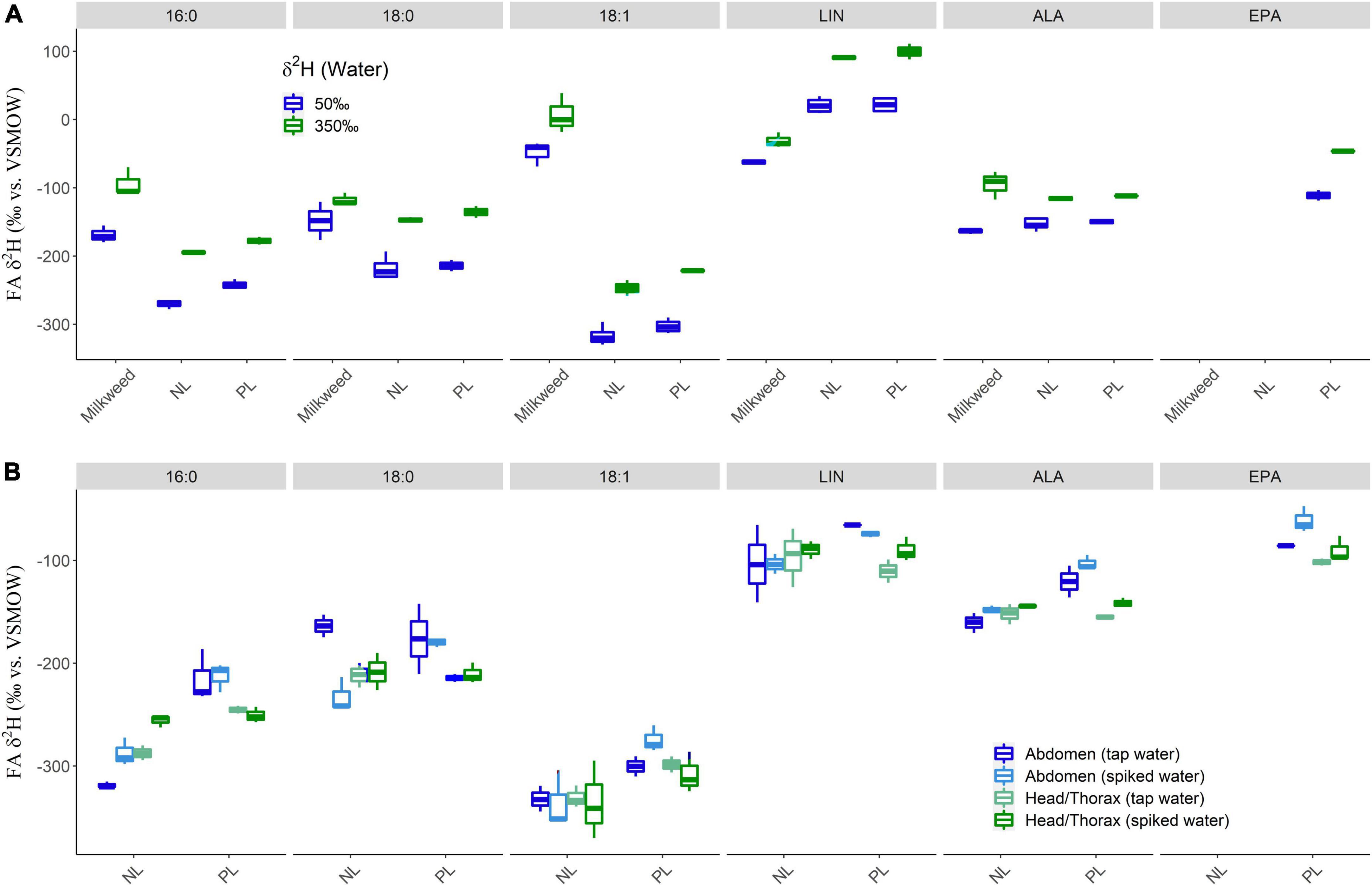
Figure 2. Hydrogen isotopic fractionation between diet and fatty acids (FA) of (A) eclosed monarchs after feeding on milkweed grown under two different water treatments at larval stage (experiment 1) and (B) adult monarchs feeding on nectar with or without spiked D2O (experiment 2). (A) While the effect of 2H spiked water was universally seen, the saturated and monounsaturated fatty acids were significantly lighter in larvae compared to diet, while LIN was significantly enriched. Alpha-linoleic acid (ALA) closely reflected the diet. There were no differences in δ2H values between neutral lipids (NL) and polar lipids (PL). δ2HEPA could only be determined in PL and was enriched compared to the corresponding ALA values. (B) 16:0 and 18:1 in PL were isotopically enriched compared to NL and δ2H16:0 values of NL were lower in the abdomen, compared to head/thorax tissue, while the opposite held for PL. In addition, 16:0 was the only FA showing significant integration of deuterium, although slight isotopic effects could also be observed for ALA and EPA. LIN, ALA, and EPA in PL of the abdomen were isotopically enriched compared to NL or both PL and NL of head/thorax tissue.
Integration of deuterium into fatty acids in adults feeding on enriched nectar
Significant integration of the 2H tracer into the fatty acids of individuals feeding on 2H2O enriched nectar was observed in 16:0 of NL (Δδ2H abdomen: 31.1 ± 13.8‰; t-test, p = 0.017; head/thorax: 31.7 ± 9.3‰; t-test, p = 0.004) but not for PL. Non-significant trends were observed in 18:1 in PL of the abdomen (Δδ2H 25.7 ± 18.7‰; t-test, p = 0.12) as well as ALA and EPA of all tissues and lipid classes (Δδ2H ∼+10‰). A negative trend was observed in 18:0 of NL of abdominal tissue (spiked – tap water Δδ2H −69.2 ± 22.9‰; t-test, p = 0.019; Figure 2B).
A significant positive H isotopic fractionation between the head/thorax and abdominal tissue was found in 16:0 of NL (Δδ2H 31.5 ± 11.2‰; t-test, p = 0.016), while a corresponding negative isotope fractionation was observed for 16:0 of PL (Δδ2H −34.1 ± 20.9‰; t-test, p = 0.003). Furthermore, in PL δ2HLIN (Δδ2H −30.4 ± 14.0‰; t-test, p = 0.017), δ2HALA (Δδ2H −36.6 ± 15.2‰; t-test, p = 0.002), and δ2HEPA (Δδ2H −22.4 ± 11.1‰; t-test, p = 0.018) values were lower in the head/thorax than in the abdomen.
Significant inter-class H isotopic fractionation between NL and PL was observed in 16:0 in abdominal tissue (Δδ2H 89.1 ± 22.4‰; t-test, p < 0.001), but also in head/thorax tissue (Δδ2H 23.6 ± 9.3‰; t-test, p = 0.027) as well as to a lesser extend in 18:1 (Δδ2H abdominal: 46.8 ± 24.7‰; t-test, p = 0.002; head/thorax: 29.7 ± 28.9‰; t-test, p = 0.05). Finally, δ2HALA values were lower in NL than PL in abdominal tissue (Δδ2H 42.6 ± 15.9‰; t-test, p < 0.001; Figure 2B).
Compound-specific isotope analyses (CSIA) of fatty acids in overwintering monarchs
Compared to monarchs raised in captivity in the laboratory, the samples of overwintering monarchs from Mexico had lower δ2H16:0 (−328 ± 222 vs. −250 ± 15‰), δ2H16:1 (−362 ± 25 vs. −310 ± 46‰) and δ2H18:1 (−354 ± 11 vs. −307 ± 17‰), but higher δ2H18:0 (−133 ± 46 vs. −203 ± 28‰) and δ2HALA values (−101 ± 27 vs. −135 ± 25‰). As observed in the captive study, the δ2H16:0 values were lower in NL than PL, whereby the H isotopic fractionation was slightly higher in male than female monarchs (Δδ2H: 39.6 ± 11.6 vs. 27.9 ± 8.1‰), but in addition theΔδ2H18:0 values were higher in NL than in PL (Δδ2H: female: −79.9 ± 34.3‰; male: −89.1 ± 53.4‰). The standard deviation of H isotopic fractionation between NL and PL in all 20 monarchs was lower in δ2H16:0 (11.3‰), δ2H18:1 (7.7‰), and δ2HALA (9.0‰) than in δ2H18:0 (47.5‰) and δ2HLIN (56.2‰). The δ2HALA values of both lipid classes strongly correlated with wing chitin δ2H values (Pearson, NL: R2 = 0.78; PL: R2 = 0.64). No significant correlation with wing chitin was observed for δ2H values of any other FA (Figure 3A).
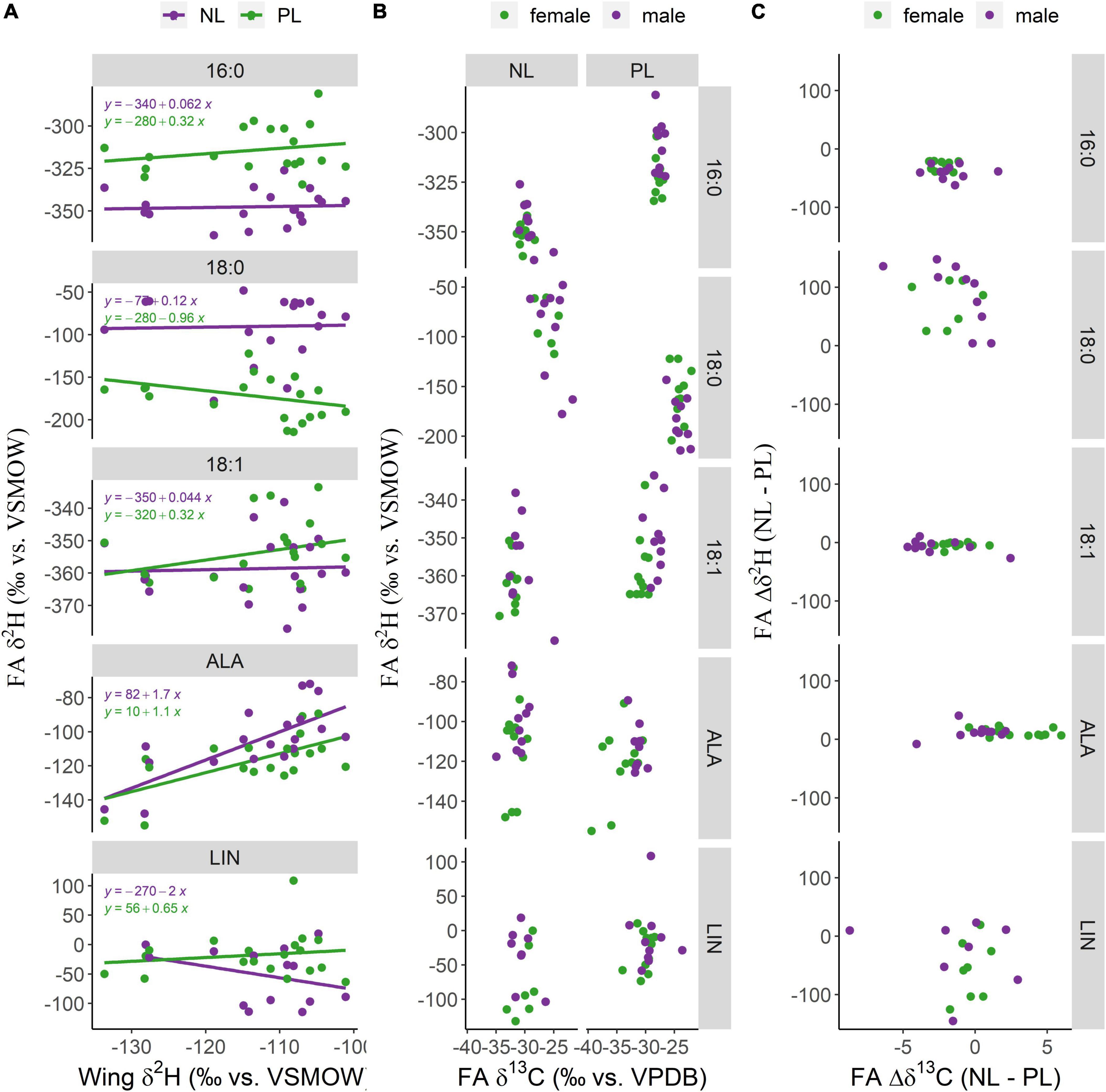
Figure 3. Compound specific isotope analysis fatty acids (FA) for 20 monarchs at their overwintering habitat in Mexico. (A) In contrast to all other FA, δ2HALA correlated with wing δ2H suggesting strict conservation of this FA from their natal provenance. (B) δ2H and δ13C fatty acid specific biplots of 10 female and 10 male monarchs from the overwintering site in Mexico. Distinct groups in an isotopic biplot, indicating different nectaring provenance, could not be identified in this small dataset. (C) The individual isotopic fractionation between neutral lipids (NL) and polar lipids (PL).
Regarding δ13C, all saturated and monounsaturated FA had slightly lower values in NL compared to PL (δ13C 16:0 −2.1 ± 1.2‰; 18:0 −1.5 ± 2.0‰; 18:1 −2.0 ± 1.9‰). About half of the female monarchs had up to 6‰ higher δ13CALA values in NL than in PL, while no carbon isotopic fractionation in ALA between lipid classes was observed in males (Figures 3B,C).
Discussion
This is the first study investigating fatty acids in monarchs (or any migratory insect) using compound-specific δ2H and δ13C and controlled tracer test analysis. Data from controlled laboratory isotope tracer tests and from overwintering individuals suggested that adult monarchs convert their dietary energy mainly into 16:0 and 18:1 FA and store them as NL in their abdomen. Accordingly, the H and C isotopic values of the non-essential saturated and monounsaturated fatty acids should not correlate with the δ2H of monarch wings, a proxy of the natal origin of each individual (Wassenaar and Hobson, 1998; Flockhart et al., 2013), but are instead expected to correlate to the spatial origins of their nectar sources. On the other hand, the δ2H values of the essential omega-3 PUFA ALA were correlated with the δ2HWing, underscoring the physiological importance of ALA because it is retained by the migratory individual throughout its entire life cycle. Finally, δ2HLIN showed a 2H enrichment compared to the larval milkweed diet, indicating the predominance of catabolic processes. In fact, we found considerable variations in the isotopic fractionation of δ2HLIN and δ13CLIN between NL and PL of overwintering monarchs. The absolute Δδ2HLIN values of NL revealed a negative correlation with δ2H values of wing chitin which could potentially be useful when investigating individual life-history traits, such as past catabolic events or periods of fasting. Taken altogether, our results provide evidence that H and C isotopic analyses of individual fatty acids in migrating and overwintering monarchs can be used to gain new insights into the nutritional history of individuals including provenance of nectaring sites used to fuel key life history events.
Monarch larvae feeding on 2H-labeled milkweed leaves accordingly integrated the isotope tracer into all FA. The δ2H values of saturated and monounsaturated FA were significantly lower in the larvae than the hostplant milkweed, indicating predominant anabolic processes, that is, ongoing fatty acid synthesis. Conversely, δ2HALA closely reflected the milkweed hostplant diet, and those of LIN were significantly higher, indicating a predominance of catabolic processes. We were able to detect low contents of EPA, whose δ2H values were slightly higher compared to ALA (∼40‰), but even higher when feeding on the 2H-labeled leaves (∼60‰). This could be explained by the integration of the liquid water hydrogen/deuterium atoms into the FA molecule during the conversion process of ALA to EPA (Pilecky et al., 2022), which monarchs must perform because terrestrial plants do not contain any PUFA with more than 18 C atoms (Twining et al., 2016). Bioconversion is likely initiated by an elongation of ALA to 20:3n-3, followed by double desaturation to EPA as has been found in other hexapods (Strandberg et al., 2020) and because we did not detect any traces of stearidonic acid (18:4n-3) in monarchs in this study. Integration of deuterium atoms into EPA and into ALA of PL of head/thorax tissue also occurred for adults feeding on a sugar solution with 2H-enriched water but without any FA, which revealed that bioconversion of ALA to EPA is not only performed in monarch larvae, but also in adults, and that EPA in monarchs is potentially subjected to retro-conversion to ALA in head/thorax tissue. Still, the monarch larval stage appeared to be the only possibility for monarch adults to obtain this essential fatty acid. A recent study by Pocius et al. (2022) found a correlation between larval diet, i.e., milkweed species, and the free-flight energetics of monarch adults, hence it would be of great interest to establish whether the species of milkweed host plant (and its distribution) impacts the EPA quantity in freshly eclosed monarch adults. In many animal species, EPA increases the efficacy of neuronal tissue (e.g., providing higher visual acuity, memory capacity, or spatial navigation) and promotes reproduction, and is therefore particularly biosynthesized by females for maternal provision to offspring (Pilecky et al., 2021b). In our small pilot dataset of 20 wild overwintering monarchs, we found that about half of females showed a high carbon isotopic fractionation in δ13CALA between NL and PL, which suggest increased bioconversion activity during gravidity, although our sample size is too small to solidly support this idea. Unfortunately, determining δ2HEPA and δ13CEPA is analytically challenging in adult monarch lipid extracts due to the extremely low ratio of these EPA to total FAME, particularly in NL and abdominal tissue. As a result, we were unable to reliably measure δ2HEPA and δ13CEPA in the wild overwintering monarchs. Further methodological improvements will be required for studying EPA bioconversion rates in the wild monarch populations.
The large isotopic fractionation in δ2H16:0 between PL and NL in abdominal tissue revealed a high rate of fatty acid synthesis and thereby underscores its importance for dietary energy conversion from carbohydrates to storage lipids. Unfortunately, our overwintering monarch sample size was too small to fully validate the suitability of compound-specific non-essential stable isotope analysis for linking wintering individual’s FA to different food sources spatially, and we were unable to identify any subgroups by dual (C + H) isotope plots of any FA to potentially differentiate nectaring locations. However, data from the controlled experiments showed that nectar sources were clearly reflected in the δ2H16:0 values and may be integrated into δ2H18:0 and δ2H18:1 over a longer period of time, encouraging further studies investigating the suitability of this FA as food provenance and energy source marker.
Linoleic acid was the only fatty acid whose δ2H values were significantly enriched compared to diet and showed the largest variation in δ2H values in the 20 wild overwintering monarchs from Mexico, both in their absolute values but particularly in the isotopic fractionation between NL and PL. Furthermore, the δ2HLIN of NL showed a negative correlation with wing chitin δ2H values (e.g., natal origin). We found low mass fractions of arachidonic acid (20:4n-6, ARA) mainly in the NL and almost none in PL, which suggested that ARA was a by-product of fatty acid bioconversion of the non-selective (between n-3 and n-6) bioconversion, rather than having an ARA-demanding physiological function. LIN is taken up together with ALA from milkweed leaves at the larval stage, whereas adults have no significant access to both PUFA. Omega-3 PUFA are vital for functioning of the neuronal system including vision and navigation and are thus particularly retained rather than catabolized (Pilecky et al., 2021b), while no similar biological function for LIN is known. We therefore postulate that LIN is accumulated at the larval stage up to eclosure of the adult. From that time on, LIN is partially used for energy production, particularly when running low on non-essential fatty acids. This is likely the cause of the observed increase in δ2HLIN values in all samples, because fatty acids with lighter isotopic composition are mobilized faster, rendering the residual unused pool with heavier isotopic composition. It could be speculated that δ2HLIN values are an energetics marker for individual life-history of migrant monarchs, but it remains to be tested whether directly or indirectly, for example by correlation of isotopic fractionation between LINNL and LINPL with spatiotemporal parameters such as total flight time or migratory distance and/or the number of over-wintering fasting days.
Conclusion
The controlled laboratory and field results of this pilot study encourage further investigation on the application of compound-specific 2H and 13C analysis investigations of the migration routes and nectaring energy sources of monarchs and for other migratory Lepidoptera or insects. Further studies using appropriately larger population samples sizes are warranted to better understand the physiological processes altering isotopic values of saturated and mono-unsaturated FA in addition to isotopic values of food sources. Larger datasets are also needed to correct for isotopic “noise” introduced by each individuals’ life-history (i.e., catabolism), which may blur the energy source signal from diet. Within this context, we suggest δ2HLIN could potentially be useful as life-history marker, and our results establish an encouraging basis for future studies with flight-time/distance and/or fasting periods. Finally, our study reveals the power of compound-specific isotope analyses (CSIA) for a detailed investigation of the migration routes and fuel sources of insects and other migrating species.
Data availability statement
The original contributions presented in this study are included in the article, further inquiries can be directed to the corresponding author.
Author contributions
KH and JM designed the study and provided financial support. MP, LW, and KH performed the data analysis and led wrote the manuscript. KH, JM, and MR collected the samples. LW, KH, MK, JM, and MR assisted with manuscript editing. All authors critically assessed the manuscript and approved the submitted version.
Funding
This work was supported by discovery grant to KH from the Natural Sciences and Engineering Council (NSERC) of Canada (grant 2017-04430). The open access fee was covered by Donau-Universität Krems. Funding was provided in part by a grant from Environment and Climate Change Canada to JM.
Conflict of interest
The authors declare that the research was conducted in the absence of any commercial or financial relationships that could be construed as a potential conflict of interest.
Publisher’s note
All claims expressed in this article are solely those of the authors and do not necessarily represent those of their affiliated organizations, or those of the publisher, the editors and the reviewers. Any product that may be evaluated in this article, or claim that may be made by its manufacturer, is not guaranteed or endorsed by the publisher.
References
Baird, P. (2022). Diatoms and fatty acid production in arctic and estuarine ecosystems—a reassessment of marine food webs, with a focus on the timing of shorebird migration. Mar. Ecol. Prog. Ser. 688, 173–196. doi: 10.3354/meps14025
Beall, G. (1948). The fat content of a butterfly, Danaus Plexippus Linn., As affected by migration. Ecology 29, 80–94. doi: 10.2307/1930346
Brower, L. P. (1985). “New perspectives on the migration biology of the monarch butterfly, Danaus plexippus L,” in Contributions in marine science, Vol. 27, (Austin: The University of Texas Libraries), 748–786.
Brower, L. P., Fink, L. S., and Walford, P. (2006). Fueling the fall migration of the monarch butterfly. Integr. Comp. Biol. 46, 1123–1142. doi: 10.1093/icb/icl029
Brower, L., Fink, L., Kiphart, R., Pocius, V., Zubieta, R., and Ramírez, M. (2015). “The effect of the 2010-2011 drought on the lipid content of monarch butterflies (Danaus plexippus L., Danainae) migrating through Texas to their overwintering sites in Mexico,” in Monarchs in a changing world: Biology and conservation of an iconic butterfly, eds K. S. Obserhauser, K. R. Nail, and S. Altizer (Ithaca, NY: Cornell University Press), 117–129.
Brown, J. J., and Chippendale, G. M. (1974). Migration of the monarch butterfly, Danaus plexippus: Energy sources. J. Insect Physiol. 20, 1117–1130. doi: 10.1016/0022-1910(74)90218-2
Cenedella, R. J. (1971). The lipids of the female monarch butterfly, Danaus plexippus, during fall migration. Insect Biochem. 1, 244–247. doi: 10.1016/0020-1790(71)90077-1
Flockhart, D. T. T., Wassenaar, L. I., Martin, T. G., Hobson, K. A., Wunder, M. B., and Norris, D. R. (2013). Tracking multi-generational colonization of the breeding grounds by monarch butterflies in eastern North America. Proc. Biol. Sci. 280:20131087. doi: 10.1098/rspb.2013.1087
Génier, C. S. V., Guglielmo, C. G., Mitchell, G. W., Falconer, M., and Hobson, K. A. (2021). Nutritional consequences of breeding away from riparian habitats in Bank Swallows: New evidence from multiple endogenous markers. Conserv. Physiol. 9:coaa140. doi: 10.1093/conphys/coaa140
Gibo, D. L., and McCurdy, J. A. (1993). Lipid accumulation by migrating monarch butterflies (Danaus plexippus L.). Can. J. Zool. 71, 76–82. doi: 10.1139/z93-012
Heissenberger, M., Watzke, J., and Kainz, M. J. (2010). Effect of nutrition on fatty acid profiles of riverine, lacustrine, and aquaculture-raised salmonids of pre-alpine habitats. Hydrobiologia 650, 243–254. doi: 10.1007/s10750-010-0266-z
Hobson, K. A., García-Rubio, O. R., Carrera-Treviño, R., Anparasan, L., Kardynal, K. J., McNeil, J. N., et al. (2020). Isotopic (δ2H) analysis of stored lipids in migratory and overwintering monarch butterflies (Danaus plexippus): Evidence for Southern critical late-stage nectaring sites? Front. Ecol. Evol. 8:572140. doi: 10.3389/fevo.2020.572140
Hobson, K. A., Norris, D. R., Kardynal, K. J., and Yohannes, E. (2019). “Chapter 1 - animal migration: A context for using new techniques and approaches,” in Tracking animal migration with stable isotopes (Second Edition), eds K. A. Hobson and L. I. Wassenaar (Cambridge: Academic Press), 1–23. doi: 10.1016/B978-0-12-814723-8.00001-5
Kaluzny, M. A., Duncan, L. A., Merritt, M. V., and Epps, D. E. (1985). Rapid separation of lipid classes in high yield and purity using bonded phase columns. J. Lipid Res. 26, 135–140. doi: 10.1016/S0022-2275(20)34412-6
Lennox, R. J., Chapman, J. M., Souliere, C. M., Tudorache, C., Wikelski, M., Metcalfe, J. D., et al. (2016). Conservation physiology of animal migration. Conserv. Physiol. 4:cov072. doi: 10.1093/conphys/cov072
Levin, E., McCue, M. D., and Davidowitz, G. (2017). More than just sugar: Allocation of nectar amino acids and fatty acids in a Lepidopteran. Proc. Biol. Sci. 284:20162126. doi: 10.1098/rspb.2016.2126
McWilliams, S. R., Guglielmo, C., Pierce, B., and Klaassen, M. (2004). Flying, fasting, and feeding in birds during migration: A nutritional and physiological ecology perspective. J. Avian Biol. 35, 377–393. doi: 10.1111/j.0908-8857.2004.03378.x
O’Brien, D. M., Schrag, D. P., and del Rio, C. M. (2000). Allocation to reproduction in a Hawkmoth: A quantitative analysis using stable carbon isotopes. Ecology 81, 2822–2831. doi: 10.1890/0012-96582000081[2822:ATRIAH]2.0.CO;2
Parmar, T. P., Kindinger, A. L., Mathieu-Resuge, M., Twining, C. W., Shipley, J. R., Kainz, M. J., et al. (2022). Fatty acid composition differs between emergent aquatic and terrestrial insects—A detailed single system approach. Front. Ecol. Evol. 10:952292. doi: 10.3389/fevo.2022.952292
Pilecky, M., Kämmer, S. K., Mathieu-Resuge, M., Wassenaar, L. I., Taipale, S. J., Martin-Creuzburg, D., et al. (2022). Hydrogen isotopes (δ2H) of polyunsaturated fatty acids track bioconversion by zooplankton. Funct. Ecol. 36, 538–549. doi: 10.1111/1365-2435.13981
Pilecky, M., Winter, K., Wassenaar, L. I., and Kainz, M. J. (2021a). Compound-specific stable hydrogen isotope (δ2H) analyses of fatty acids: A new method and perspectives for trophic and movement ecology. Rapid Commun. Mass Spectrom. 35:e9135. doi: 10.1002/rcm.9135
Pilecky, M., Závorka, L., Arts, M. T., and Kainz, M. J. (2021b). Omega-3 PUFA profoundly affect neural, physiological, and behavioural competences – implications for systemic changes in trophic interactions. Biol. Rev. 96, 2127–2145. doi: 10.1111/brv.12747
Pocius, V. M., Cibotti, S., Ray, S., Ankoma-Darko, O., McCartney, N. B., Schilder, R. J., et al. (2022). Impacts of larval host plant species on dispersal traits and free-flight energetics of adult butterflies. Commun. Biol. 5:469. doi: 10.1038/s42003-022-03396-8
Saunders, S. P., Ries, L., Neupane, N., Ramírez, M. I., García-Serrano, E., Rendón-Salinas, E., et al. (2019). Multiscale seasonal factors drive the size of winter monarch colonies. Proc. Natl. Acad. Sci. U.S.A. 116, 8609–8614. doi: 10.1073/pnas.1805114116
Slayback, D., Brower, L., Ramírez, M., and Fink, L. (2007). Establishing the presence and absence of overwintering colonies of the monarch butterfly in Mexico by the use of small aircraft. Am. Entomol. 53, 28–40. doi: 10.1093/ae/53.1.28
Soto, D. X., Hobson, K. A., and Wassenaar, L. I. (2013). The influence of metabolic effects on stable hydrogen isotopes in tissues of aquatic organisms. Isotopes Environ. Health Stud. 49, 305–311. doi: 10.1080/10256016.2013.820727
Strandberg, U., Vesterinen, J., Ilo, T., Akkanen, J., Melanen, M., and Kankaala, P. (2020). Fatty acid metabolism and modifications in Chironomus riparius. Philos. Trans. R. Soc. B Biol. Sci. 375:20190643. doi: 10.1098/rstb.2019.0643
Taylor, O. R., Lovett, J. P., Gibo, D. L., Weiser, E. L., Thogmartin, W. E., Semmens, D. J., et al. (2019). Is the timing, pace, and success of the monarch migration associated with sun angle? Front. Ecol. Evol. 7:442. doi: 10.3389/fevo.2019.00442
Twining, C. W., Brenna, J. T., Hairston, N. G., and Flecker, A. S. (2016). Highly unsaturated fatty acids in nature: What we know and what we need to learn. Oikos 125, 749–760. doi: 10.1111/oik.02910
Wassenaar, L. I., and Hobson, K. A. (1998). Natal origins of migratory monarch butterflies at wintering colonies in Mexico: New isotopic evidence. Proc. Natl. Acad. Sci. U.S.A. 95, 15436–15439. doi: 10.1073/pnas.95.26.15436
Wassenaar, L. I., and Hobson, K. A. (2003). Comparative equilibration and online technique for determination of non-exchangeable hydrogen of keratins for use in animal migration studies. Isotopes Environ. Health Stud. 39, 211–217. doi: 10.1080/1025601031000096781
Wassenaar, L. I., Hobson, K. A., and Sisti, L. (2015). An online temperature-controlled vacuum-equilibration preparation system for the measurement of δ2H values of non-exchangeable-H and of δ18O values in organic materials by isotope-ratio mass spectrometry. Rapid Commun. Mass Spectrom. 29, 397–407. doi: 10.1002/rcm.7118
West, J. B., Sobek, A., and Ehleringer, J. R. (2008). A simplified GIS approach to modeling global leaf water isoscapes. PLoS One 3:e2447. doi: 10.1371/journal.pone.0002447
Keywords: lipids, migration, habitat conservation, stable isotopes, fatty acids, deuterium, 13C
Citation: Pilecky M, Wassenaar LI, Kainz MJ, Anparasan L, Ramirez MI, McNeil JN and Hobson KA (2022) Isotopic (δ 2H and δ 13C) tracing the provenance and fate of individual fatty acids fueling migrating animals: A case study of the monarch butterfly (Danaus plexippus). Front. Ecol. Evol. 10:1051782. doi: 10.3389/fevo.2022.1051782
Received: 23 September 2022; Accepted: 14 November 2022;
Published: 25 November 2022.
Edited by:
Todd Jason McWhorter, University of Adelaide, AustraliaReviewed by:
Louie Yang, University of California, Davis, United StatesDavid Nelson, University of Maryland, College Park, United States
Copyright © 2022 Pilecky, Wassenaar, Kainz, Anparasan, Ramirez, McNeil and Hobson. This is an open-access article distributed under the terms of the Creative Commons Attribution License (CC BY). The use, distribution or reproduction in other forums is permitted, provided the original author(s) and the copyright owner(s) are credited and that the original publication in this journal is cited, in accordance with accepted academic practice. No use, distribution or reproduction is permitted which does not comply with these terms.
*Correspondence: Matthias Pilecky, bWF0dGhpYXMucGlsZWNreUBkb25hdS11bmkuYWMuYXQ=