Biological responses to change in Antarctic sea ice habitats
- 1Institute for Marine and Antarctic Studies, University of Tasmania, Hobart, TAS, Australia
- 2Australian Antarctic Program Partnership, Institute for Marine and Antarctic Studies, University of Tasmania, Hobart, TAS, Australia
- 3Centre for Marine Socioecology, University of Tasmania, Hobart, TAS, Australia
- 4Australian Antarctic Division, Department of Climate Change, Energy, the Environment and Water, Kingston, TAS, Australia
- 5The Australian Centre for Excellence in Antarctic Science, University of Tasmania, Hobart, TAS, Australia
- 6Institute for the Study of the Anthropic Impacts and the Sustainability of the Marine Environment (IAS), National Research Council of Italy, Genoa, Italy
- 7Department of Chemical, Biological, Pharmaceutical and Environmental Sciences, University of Messina, Messina, Italy
- 8Stazione Zoologica Anton Dohrn, Napoli, Italy
- 9British Antarctic Survey, Natural Environment Research Council, Cambridge, United Kingdom
- 10GEOMAR Helmholtz Center for Ocean Research Kiel, Kiel, Germany
- 11Channel and North Sea Fisheries Research Unit, IFREMER, Boulogne-sur-Mer, France
- 12UFR 918 Terre Environnement et Biodiversité, Sorbonne Université, Paris, France
- 13National Institute of Polar Research, Research Organization of Information and Systems, Tachikawa, Japan
- 14Department of Polar Science, The Graduate University for Advanced Studies, SOKENDAI, Tachikawa, Japan
- 15Department of Ocean Sciences, Tokyo University of Marine Science and Technology, Minato, Japan
- 16School of Biological Sciences, Victoria University of Wellington, Wellington, New Zealand
- 17Department of Earth, Ocean and Atmospheric Sciences and the Institute for the Oceans and Fisheries, University of British Columbia, Vancouver, BC, Canada
- 18Alfred Wegener Institute Helmholtz Centre for Polar and Marine Research, Bremerhaven, Germany
- 19Department of Mathematics, University of Utah, Salt Lake City, UT, United States
- 20School of Biological Sciences, University of Utah, Salt Lake City, UT, United States
- 21Australian Bureau of Meteorology, Hobart, TAS, Australia
- 22CSIRO Oceans and Atmosphere, Hobart, TAS, Australia
Sea ice is a key habitat in the high latitude Southern Ocean and is predicted to change in its extent, thickness and duration in coming decades. The sea-ice cover is instrumental in mediating ocean–atmosphere exchanges and provides an important substrate for organisms from microbes and algae to predators. Antarctic krill, Euphausia superba, is reliant on sea ice during key phases of its life cycle, particularly during the larval stages, for food and refuge from their predators, while other small grazers, including copepods and amphipods, either live in the brine channel system or find food and shelter at the ice-water interface and in gaps between rafted ice blocks. Fish, such as the Antarctic silverfish Pleuragramma antarcticum, use platelet ice (loosely-formed frazil crystals) as an essential hatching and nursery ground. In this paper, we apply the framework of the Marine Ecosystem Assessment for the Southern Ocean (MEASO) to review current knowledge about relationships between sea ice and associated primary production and secondary consumers, their status and the drivers of sea-ice change in this ocean. We then use qualitative network modelling to explore possible responses of lower trophic level sea-ice biota to different perturbations, including warming air and ocean temperatures, increased storminess and reduced annual sea-ice duration. This modelling shows that pelagic algae, copepods, krill and fish are likely to decrease in response to warming temperatures and reduced sea-ice duration, while salp populations will likely increase under conditions of reduced sea-ice duration and increased number of days of >0°C. Differences in responses to these pressures between the five MEASO sectors were also explored. Greater impacts of environmental pressures on ice-related biota occurring presently were found for the West and East Pacific sectors (notably the Ross Sea and western Antarctic Peninsula), with likely flow-on effects to the wider ecosystem. All sectors are expected to be impacted over coming decades. Finally, we highlight priorities for future sea ice biological research to address knowledge gaps in this field.
1. Introduction
Sea ice is a major structuring component in Antarctic marine ecosystems and a key driver of Southern Ocean biogeochemical cycles (Thomas, 2017; Henley et al., 2020). It forms a physical and chemically-active barrier on the surface of the ocean, and strongly affects fluxes of gas (e.g., climate-active gases such as carbon dioxide and various sulphur compounds, e.g., dimethyl sulphide) and energy across the atmosphere–ocean interface (Tison et al., 2017). Sea ice also forms a key habitat for ice algae (Arrigo, 2017); it is highly reflective (particularly when snow covered) and reduces light levels under the ice to 0.1%–1% of incoming surface radiation (Arndt et al., 2017), resulting in strong control on Southern Ocean phytoplankton production and phenology (Henley et al., 2020; Pinkerton et al., 2021). Sea-ice cover also decreases wind-driven mixing of the surface ocean, and its seasonal melt can induce stratification in the upper layers of the water column that is conducive for pelagic phytoplankton blooms during ice retreat in spring (Saba et al., 2014; Sabu et al., 2014; Kauko et al., 2021). Antarctic sea ice extent is sensitive to both atmospheric and oceanic forcing; however, regional-scale atmospheric circulation changes are thought to be the dominant driver of recent trends, i.e., a slight increasing overall trend in sea-ice extent since 1979 comprising different regional and seasonal contributions (Hobbs et al., 2016; Turner et al., 2022).
The life cycles of many Antarctic species are attuned to the seasonal cycle of sea-ice advance and retreat. For example, penguins and seals use sea ice as a resting and breeding platform; their sea-ice requirements are complex and include icescape variables such as landfast ice extent and duration, pack-ice concentration and seasonality and local snow cover thickness and surface roughness (e.g., Massom and Stammerjohn, 2010; Bestley et al., 2020). Sea ice also provides a substrate and habitat for ice-associated (sympagic) communities consisting of bacteria, micro-algae, heterotrophic protists and small metazoans including copepods, flatworms, gastropods and ctenophores (Bluhm et al., 2017). Larger organisms living in the water column below can also use sea ice as a refuge from predators and/or as feeding grounds (Figure 1).
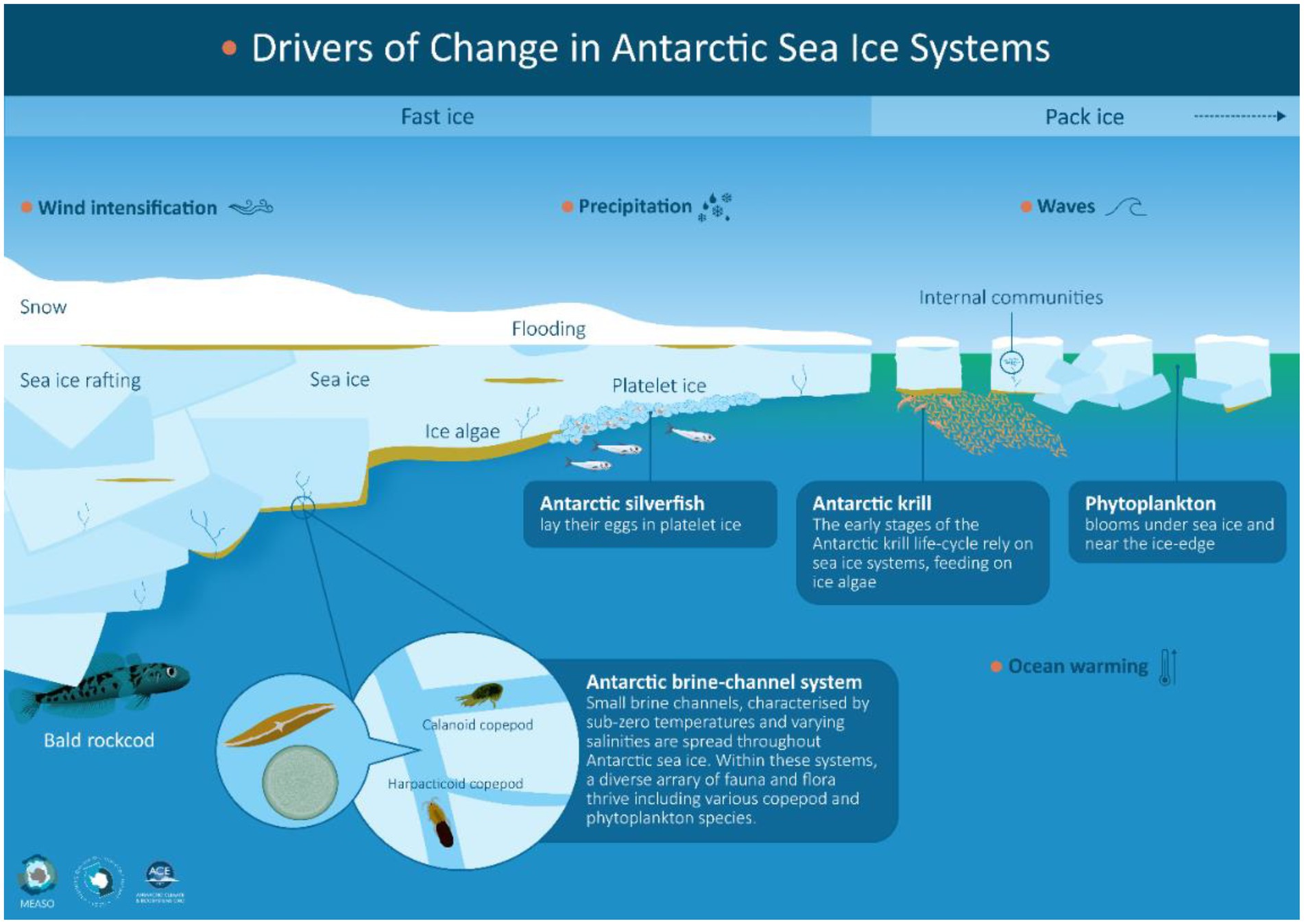
Figure 1. Sea ice habitat showing transition from inshore (stationary) landfast ice to offshore (mobile) pack ice. The diagram features key ice-associated biota and the drivers of change that are used as perturbations in the qualitative network analysis (see Table 1B).
Ecosystem services provided by sea ice and their associated communities include biogeochemical cycling, food web maintenance, primary productivity and climate regulation, along with benefits for science, tourism and recreation, food security and fisheries (Grant et al., 2013, 2021; Cavanagh et al., 2021; Murphy et al., 2021; Steiner et al., 2021). At present, our understanding of how global environmental change will affect the biota associated with Antarctic sea ice is limited (IPCC, 2019, 2021). Here we apply the Marine Ecosystem Assessment of the Southern Ocean (MEASO) framework to synthesise the present state of knowledge of key lower trophic level biota that have strong connections with sea ice, either by occupying the sympagic habitat or by living in seasonally ice-covered waters. We then build a qualitative network model to show the interactions between these physical, chemical and biological components of the Southern Ocean ecosystem and explore how cascading feedback effects might affect the system under the influence of future global environmental change.
2. Sea ice
The annual cycle of Antarctic sea ice coverage represents the largest seasonal physical change on the surface of the Earth, varying between ~3.1 million km2 in February and 18.5 million km2 in September (Eayrs et al., 2019; Parkinson, 2019). For ecosystems, a crucial distinction is between extensive mobile pack ice and stationary coastal landfast ice, because they provide different habitats for different species (e.g., Massom et al., 2009; Massom and Stammerjohn, 2010; Meiners et al., 2018; Labrousse et al., 2019). Around the Antarctic coast, landfast ice distribution is largely governed by coastal configuration and bathymetric depth (Fraser et al., 2012, 2020). Landfast ice can be either seasonally-recurring or perennial (persist through one or more summers), and the limit of its offshore coverage is generally determined by the location of icebergs grounded on seafloor shoals at a depth of ~400 m below sea level (Giles et al., 2008). The ice spanning from the coast to these pinning points can reach widths up to 300 km in certain areas, but is generally less extensive (Fraser et al., 2020). Although the spatial distribution and variability of Antarctic landfast ice are well-characterised (Fraser et al., 2021), detailed understanding of the drivers of its annual variation is currently lacking, though it is clear that it is affected by both oceanic and atmospheric forcing (Massom et al., 2009; Fraser, 2011; Aoki, 2017; Arndt et al., 2020). At some locations offshore from ice shelves, the shoaling of supercooled water originating from ice shelf basal melting, with its temperature below the freezing point, results in the formation of free-floating ice crystals that may form along the ice shelf gradient; these are known as platelet crystals, which accumulate and grow locally beneath fast ice (Foldvik and Kvinge, 1974; Langhorne et al., 2015). As shown in Figure 1, platelet ice represents an important sub-habitat for some species. A comprehensive review of platelet ice and its role in the Antarctic ecosystem is provided by Hoppmann et al. (2020).
By comparison, pack-ice comprises a matrix of floes of varying size, age, concentration and thickness that are in constant motion in response to ocean currents and winds, which may cause complex rafting or dispersal of ice floes. In winter, the maximum width of Antarctica’s pack-ice zone varies from a few hundred kilometres (e.g., off East Antarctica from 120°E to 135°E) to >2,000 km in the deep poleward embayment of the Weddell Sea. Antarctica’s pack-ice zone is the dominant part of the sea-ice system that undergoes annual advance and retreat. Although Antarctic pack-ice thickness is a major unknown (IPCC, 2019), largely due to difficulties in making in situ measurements, it is thought to be typically 0.3–2.0 m thick (Worby et al., 2008), although larger thicknesses (of ~10 m) can occur through ice deformation where floes collide (Massom et al., 2006). Perennial ice is largely confined to the southwestern Weddell, Amundsen and eastern Ross seas (Parkinson, 2019).
The key attributes of Antarctic sea ice include areal coverage/extent, the thickness distributions of the ice and its snow cover, rates and patterns of advance and retreat and resultant duration, and the associated variability (Massom and Stammerjohn, 2010). These attributes result from the complex interplay of both thermodynamic processes (freeze/formation and melt) and dynamic processes (movement and deformation of the ice by winds, ocean currents, swell and waves; Weeks, 2010). Although this combination of processes and the frequent passage of storms creates a highly heterogeneous mixture of different ice types, thickness, age and degree of deformation at small scales, overall the Antarctic sea-ice zone is characterised by distinctive large-scale zones (Massom and Stammerjohn, 2010). Moving from north to south, these are: (1) the marginal ice zone or MIZ (outer pack strongly influenced by the high-energy Southern Ocean and ocean waves); (2) the mid pack-ice zone, which is buffered by the MIZ and generally characterised by larger floes and thicker snow cover, but still influenced by storms; and (3) the coastal zone, where sea-ice conditions are strongly influenced by the Antarctic Coastal Current, coastal configuration and the distribution of icebergs; this is also the realm of landfast ice. Recurrent coastal polynyas (i.e., persistent areas of open water bounded by sea ice) are of particular importance as areas of both high sea-ice formation rates (during freezing months) and enhanced primary production in spring–summer (Barber and Massom, 2007). Around coastal Antarctica, polynyas generally form in the lee of blocking features such as coastal promontories and areas of landfast ice, where they are also maintained by strong katabatic winds blowing seawards from the ice-sheet interior (Nihashi and Ohshima, 2015).
At the microscale (millimetres), sea ice is made up of a matrix of ice crystals separated by small inclusions (pockets) of high-salinity brine (Petrich and Eicken, 2017), the characteristics of which are constantly modified by the interaction of physical, biological and chemical processes that vary in space and time. Regarding the ice crystals themselves, Antarctic sea ice comprises a mix of both granular (or “frazil” ice), which forms under turbulent conditions, and elongated columnar ice, which grows downwards under calmer conditions (Weeks and Ackley, 1986). Near peripheral ice shelves, platelet crystals form a porous layer beneath the sea ice called the sub-ice platelet layer which has a high liquid fraction (~75%, e.g., Wongpan et al., 2015). The columnar ice acts as an advancing interface, filling in the “interplateletary” space of the layer, and is where incorporated platelet ice is formed (e.g., Hoppmann et al., 2020).
Snow is a crucially-important part of the Antarctic sea-ice system, for both landfast and pack ice (Massom et al., 2001). It accumulates as an insulative and high-albedo blanket on the ice surface, to substantially modify the optical, thermodynamic and physico-chemical properties and evolution of the underlying ice (Sturm and Massom, 2017). Notably, and depending on its thickness and properties, which themselves change over space and time (Massom et al., 2001), snow exerts strong influence on the intensity and spectral properties of light available for algal growth both within and under the ice (Wongpan et al., 2018). It can also depress the ice surface below sea level (Massom et al., 2001), leading to surface flooding and creation of an “infiltration community” (Fritsen et al., 1994; Kattner et al., 2004; Arrigo, 2017).
2.1. Variability in sea ice in Marine Ecosystem Assessment for the Southern Ocean sectors
Satellite passive microwave time series data obtained since 1978, when the records began, show a slight increasing trend in overall circumpolar Antarctic sea-ice extent (Parkinson, 2019). This overall extent masks contrasting sectoral contributions [i.e., loss in the Amundsen-Bellingshausen seas sector and gain elsewhere (Parkinson, 2019)], as well as widely-varying patterns of change and variability in sea-ice seasonality (i.e., the timings of annual advance and retreat and resultant duration of coverage Massom et al., 2013; Stammerjohn and Maksym, 2017). Moreover, the last decade has been characterised by extreme variability in overall Antarctic sea-ice extents, with record maxima in 2012–2014 (Reid and Massom, 2015) being followed by a strong negative anomaly beginning in 2015/2016 (e.g., Turner et al., 2017; Meehl et al., 2019), and, most recently, the lowest extent on record in 2021/2022 (Reid et al., 2022; Turner et al., 2022).
The MEASO framework divides Antarctica into five sectors, namely the Atlantic, Central Indian, East Indian, West Pacific and East Pacific sectors (Figure 2; Table 1). The Antarctic Peninsula, bordering the East Pacific and Atlantic sectors, is the best studied region biologically, with the western Antarctic Peninsula (wAP) currently the region of greatest environmental change, even when accounting for the cooling trend in the 1990s (Bozkurt et al., 2021). Landfast ice represents between 4.0% (during winter) and 12.8% (during late summer) of total sea ice area, with the largest area found in the central and east Indian sectors, and only small areas in the remaining three sectors (Fraser et al., 2021). While circum-Antarctic sea-ice extent showed no significant changes based on satellite data from 1979 to 2018 (IPCC, 2019, 2021), the regional response is divergent, with the East Pacific sector (wAP, including Bellingshausen and Amundsen Seas) showing ice loss, while West Pacific (Ross Sea) and Atlantic (Weddell Sea) sectors gained sea ice and the Indian sectors show no clear trend. There is also significant seasonal variability in the trends (Table 1), such that some seasons are associated with decreases in the ice while others remain static; only the western Ross Sea shows a trend that is statistically significant during all seasons (Holland, 2014). The IPCC (2021) ranks meridional winds as an important driver of sea-ice extent with high confidence. Antarctic sea ice is changing at a rate that is much lower than observed for Arctic sea ice, which is likely due to Southern Ocean circulation driving surface heat from anthropogenic warming deeper into the water column. While the decrease in overall Antarctic sea-ice coverage is believed to be too modest to be separated from natural variability (high confidence; IPCC, 2021), it is probable that if anthropogenic greenhouse gas emissions continue at the current rate, the loss of seasonal sea ice within the next 50 years could be as high as 40% (Rintoul et al., 2018).
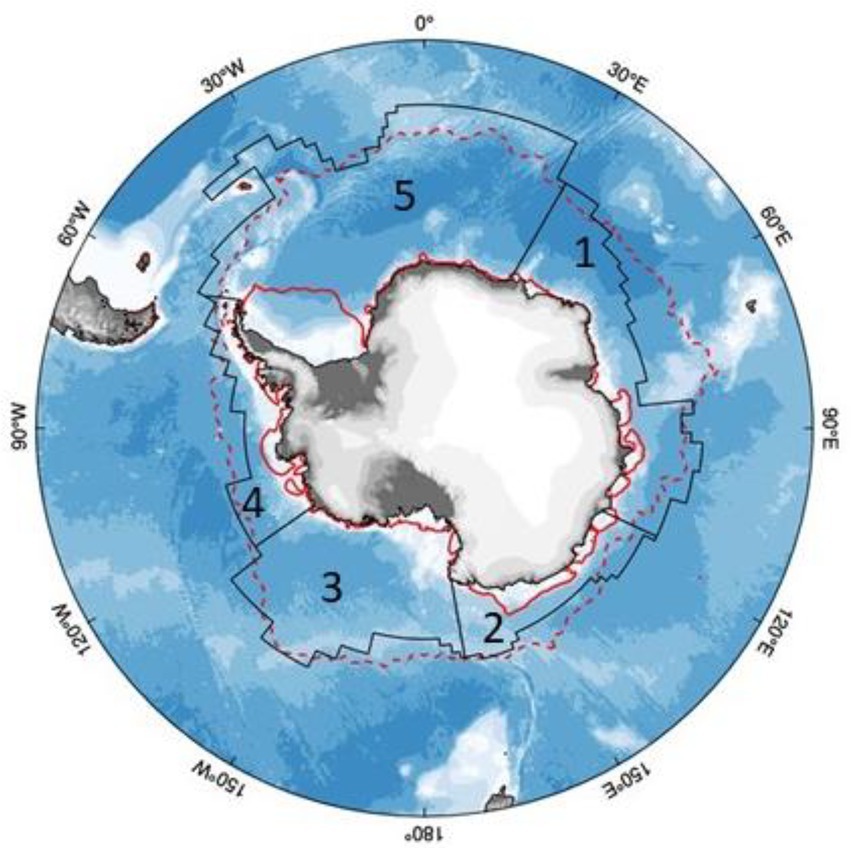
Figure 2. Map showing the five MEASO sectors, with average minimum (solid red line) and maximum (dotted red line) sea-ice extent highlighted. Sectors are numbered 1 (Central Indian), 2 (East Indian), 3 (West Pacific), 4 (East Pacific) and 5 (Atlantic).
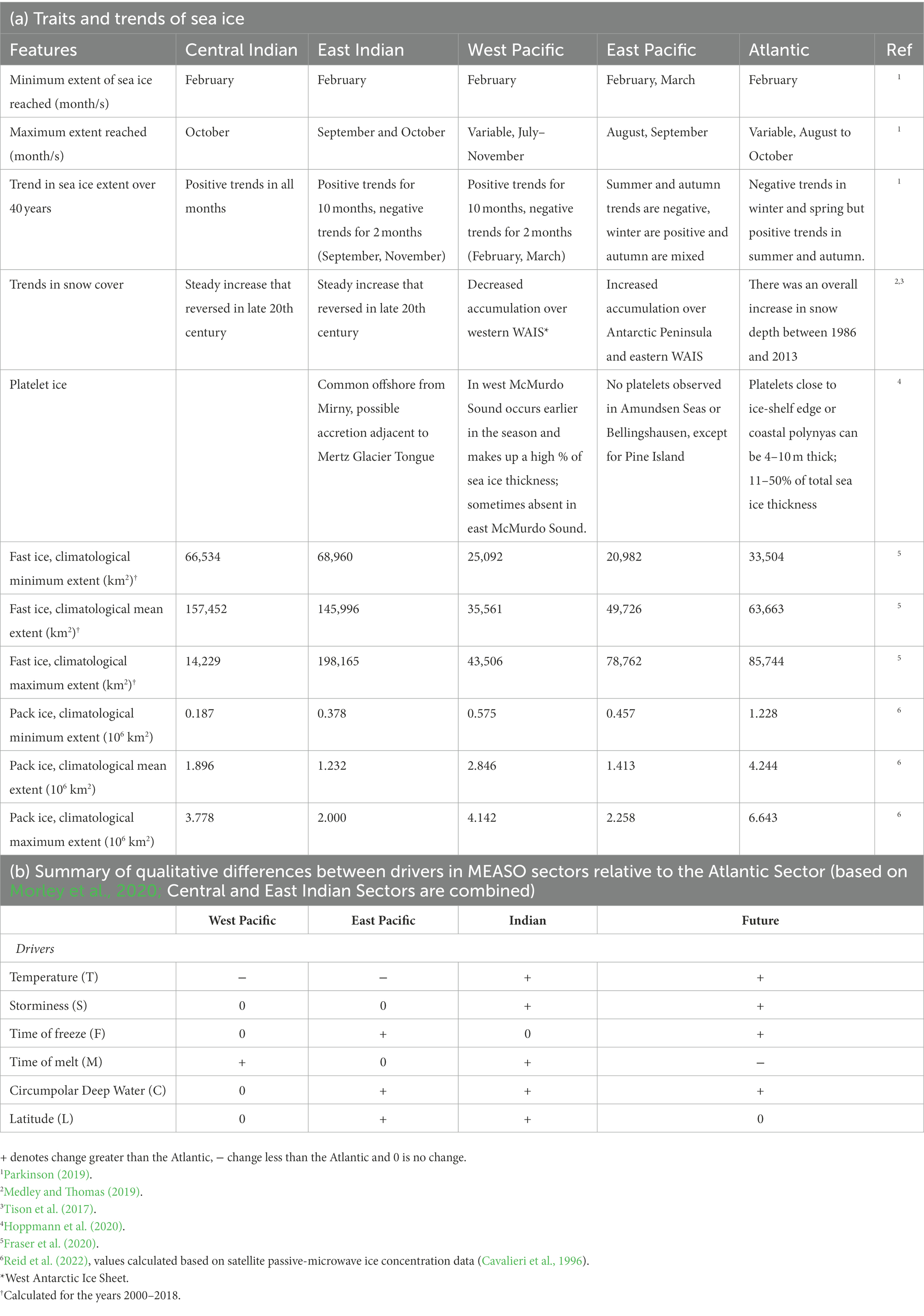
Table 1. Summary of the variation in physical factors of Antarctic sea ice between MEASO sectors (see Figure 2).
3. State of knowledge of the sea ice associated biota
Biogeographical patterns for Antarctic sea-ice biota are largely based on patchy sampling efforts in both time and space. However, there are considerable data available on the distribution, population structure and ecology of Antarctic krill (Euphausia superba), as a result of its importance as key species in the food web (Johnston et al., 2022), and in supporting an international fishery managed by the Convention for the Conservation of Antarctic Marine Living Resources (CCAMLR). The SCAR (Scientific Committee on Antarctic Research) Biogeographic Atlas of the Southern Ocean (De Broyer et al., 2014) summarised knowledge of distributions of many key species, including those that associate with sea ice (Duhamel et al., 2014; Swadling, 2014). Much of these data are geographically patchy and insufficient in duration for evaluating the current status of ice-associated species. In many cases chlorophyll a concentration is the only biological parameter recorded from ice cores and there are limited winter biological data available for most ice-covered regions (Meiners et al., 2012). Sampling of landfast ice habitats is biased in favour of locations close to Antarctic research stations (Meiners et al., 2018), while for the pack ice there is a strong seasonal emphasis on sampling during spring–summer marine research cruises, particularly around the Antarctic Peninsula and the Weddell Sea (Meiners et al., 2012). This paper focusses on the lower trophic levels that are associated with sea ice, including algae, zooplankton and fish. Further analyses in relation to the MEASO can be found in Pinkerton et al. (2021) for phytoplankton and Johnston et al. (2022) for zooplankton. Pelagic marine predators are discussed in Bestley et al. (2020).
3.1. Ice algae
The biomass of ice-associated communities is generally dominated by autotrophs, in particular pennate diatoms, but also flagellated algal species (e.g., van Leeuwe et al., 2018). Ice-algal communities form distinct communities in bottom, interior and surface layers of sea ice floes (Meiners et al., 2012, 2018). Spatial and temporal distributions of ice-algal communities, and their productivity, are strongly controlled by environmental processes, e.g., light availability and nutrients. Surface communities are promoted by snow loading resulting in surface flooding by seawater and brine, which creates surface-slush and gap layers that form habitat (Fritsen et al., 1994; Kattner et al., 2004; Ackley et al., 2008). In thicker pack ice, interior communities are likely to emerge following the rafting and ridging of ice floes, which are two processes that significantly contribute to the dynamical thickening of Antarctic sea ice (Worby et al., 2008). Furthermore, scavenging of phytoplankton during ice formation (and particularly frazil-ice formation Garrison et al., 1989; DeJong et al., 2018) and subsequent growth of incorporated algae may explain the occurrence of interior communities in undeformed sea ice (Arrigo et al., 2010). Bottom ice-algal communities thrive in the lowermost porous parts of sea ice floes characterised by favorable brine salinities and high nutrient availability. Surface and interior sea-ice algal communities are a characteristic feature of Antarctic pack ice (Meiners et al., 2012), while bottom algal communities generally dominate integrated ice algal biomass in Antarctic pack ice (Meiners et al., 2012). Extremely high ice algal biomass concentrations (with values >1,000 mg chlorophyll a m−3 of melted ice) have been reported for Antarctic fast ice containing platelet ice (Arrigo et al., 1995; Günther and Dieckmann, 1999), which forms from super-cooled Ice Shelf Water at the base of sea ice adjacent to ice shelves (see Section 2; Langhorne et al., 2015; Hoppmann et al., 2020). This sub-ice platelet layer under landfast ice provides a large surface area that serves as an important algal micro-habitat and a critical hatching and nursery ground for Antarctic silverfish, Pleuragramma antarcticum (e.g., Vacchi et al., 2004, 2012; Ghigliotti et al., 2017).
Ice algae contribute substantially to the regional annual primary production of Southern Ocean ice-covered areas (Legendre et al., 1992; McMinn et al., 2010; Pinkerton et al., 2021). The ice algal communities are strongly affected by light availability and by the thermodynamic sea ice regime and its phase-equilibrium that regulates percolation and convection of sea ice brines, thereby controlling vertical material transport within the sea ice and across the ice-water interface (Saenz and Arrigo, 2014). In general, there is an increase in the relative contribution of ice-algal production to overall production with increasing sea-ice cover duration. Model outputs suggest that ice algae contribute about 10%–20% to the overall sea-ice zone production of the Southern Ocean (Saenz and Arrigo, 2014). The ice algal production generally continues through the autumn and becomes re-invigorated in spring when light conditions for water column production is low, extending the productive season and reducing seasonal oscillations in food supply for pelagic and benthic food webs (Swadling et al., 2000, 2004; Kohlbach et al., 2018; Wing et al., 2018). Dominant taxa in pack ice include the diatoms Fragilariopsis curta, Fragilariopsis cylindrus, Nitzschia stellata and Pseudonitzschia tugiduloides while F. curta, Entomeneis kjellmanii and N. stellata (Scott et al., 1994; Takahashi et al., 2022) and Berkeleya adeliensis (Ryan et al., 2006) are more characteristic of fast ice. Surface communities tend to be dominated by flagellated taxa such as the dinoflagellate Polarella glacialis (Thompson et al., 2006).
3.2. Copepods (Arthropoda)
There are four common species of copepods that inhabit the brine channel system of sea ice, namely the calanoids Stephos longipes and Paralabidocera antarctica and the harpacticoids Drescheriella glacialis and Harpacticus furcatus. These copepods are small in size, measuring no more than 600 μm in width, and can number in the thousands of individuals per square metre of ice (Swadling et al., 1997, 2000; Kramer et al., 2011; Bluhm et al., 2017). All of these species have circum-Antarctic distributions, although P. antarctica is abundant along the East Antarctic coast (Tanimura et al., 1984; Swadling et al., 2000; Loots et al., 2009), while S. longipes is prevalent in the Weddell, Amundsen, Bellingshausen and Ross seas (Schnack Schiel et al., 1995, 1998; Guglielmo et al., 2007). Copepods are important in coastal sea-ice ecosystems as prey items for fish (e.g., Trematomus borchgrevinki, Hoshiai et al., 1989), which in turn are food for other marine predators (Johnston et al., 2022). Both P. antarctica and S. longipes have also been found in summer platelet ice (Günther et al., 1999) in the Drescher Inlet (72°50′S, 19°02′W) in the Weddell Sea. Kiko et al. (2008a) also found high abundances of S. longipes in the infiltration layer and Kiko (2010) argues that the acquisition of antifreeze properties via horizontal gene transfer might be an adaptation specifically to the occurrence of extremely low temperatures in this habitat. The four common ice-associated copepods use sea ice as a nursery ground, although the extent of their reliance on ice varies seasonally. Paralabidocera antarctica show synchronised development, with an unusually long overwintering phase as the naupliar stages that live within the brine channel system; when they reach late copepodid and adult stages in spring, they leave the sea ice to feed and reproduce at the under-ice surface (Tanimura et al., 1996; Swadling et al., 2004). Stephos longipes occupies the sea ice predominantly as nauplii (Schnack Schiel et al., 1995; Costanzo et al., 2002) then relocates to the water column, with some individuals remaining in contact with the ice (Guglielmo et al., 2007) and others moving into deeper waters (Kurbjeweit et al., 1993). Drescheriella glacialis carry their eggs in paired sacs and show overlapping generations throughout the year. This species inhabits the interstices of the ice, feeding on ice algae and other particulate organic matter (Schmidt et al., 2003). These ice-associated species are also known to appear in the water column as sea ice is melting, highlighting the inter-relationships between sea ice and the pelagic systems (Makabe et al., 2022).
At the ice-water interface, a greater diversity of copepod species uses the ice as feeding grounds and/or for refuge. Frequently-recorded species include the calanoids Ctenocalanus citer, Calanus propinquus and Microcalanus pygmaeus, while cyclopoid copepods include Oithona similis, Oncaea curvata and Pseudocyclopina belgicae. Calanus propinquus is a biomass dominant species in the Southern Ocean; a proportion of the population remains in surface waters during winter where the individuals stay trophically active (Burghart et al., 1999), with stable isotope analysis suggesting this species could change its feeding mode to include sea-ice algae (Jia et al., 2016). Calanus propinquus also showed nocturnal feeding activity during winter and a modest vertical migration by descending into deeper layers during daytime (Hunt et al., 2014). Pseudocyclopina belgicae is the only cyclopoid copepod that might have a strong association with sea ice during its reproductive cycle, possibly overwintering in the ice as late-stage copepodites (Waghorn and Knox, 1988; Menshenina and Melnikov, 1995). Note that while copepods represent considerable diversity and biomass in ice-associated habitats, other groups including pteropods (Thibodeau et al., 2019) and amphipods (Rakusa Suszczewski, 1972; Richardson and Whitaker, 1979; Krapp et al., 2008) can be important in the under-ice environment. Moreover, ciliata, foraminifera, platyhelminthes, and rarer species such as the nudibranch Tergipes antarcticus and the anemone Edwardsiella andrillae (Daly et al., 2013) and even ctenophores are found inhabiting the brine channel system and/or the infiltration layer (e.g., Kiko et al., 2008a,b; Kramer et al., 2011 and references therein). These taxa are not addressed in this review.
3.3. Euphausiids (Arthropoda)
Antarctic krill, Euphausia superba, are a major link between primary producers and higher trophic levels, and use seasonal sea ice as habitat and as a potential source of their winter food supply (Quetin and Ross, 2009). Survival through the first winter is a critical time in the life cycle of krill (Kohlbach et al., 2018; Murphy et al., 2018; Johnston et al., 2022). Larval krill rely on habitat complexity (e.g., rafting, ridging) in sea ice for refuge from predators (Daly, 2004; Meyer, 2012; Meyer et al., 2017, 2020) and as a site of reduced competition for resources (David et al., 2021). Recruitment is driven largely by winter survival of krill larval stages, which is the period of growth, when they are very susceptible to climate change (Flores et al., 2012; Meyer et al., 2020). Under poor feeding conditions, larval krill cannot grow and moult to their next stage of development (Ross and Quetin, 1991). Larval krill enter their first winter with no lipid reserve and, unlike adult krill, they need to feed continually to meet their energetic needs (Kohlbach et al., 2018; Thorpe et al., 2019). Without food they die within days (O’Brien et al., 2011). Furcilia larvae are capable of scraping ice algal films from the ice surface, especially from greenish sea ice (Hamner et al., 1989). However, as the larvae are not well-equipped to actively harvest sea ice-entrained algal cells in the winter when algal films on the ice surface are not well developed, they rely on the cells being released into the water column by physical processes including ice melting (Meyer et al., 2017). In the autumn, algae are entrained into sea ice as it grows, and these algae serve as potential food for larval krill (Kohlbach et al., 2018). Krill can exploit additional food sources in addition to sea ice algae, such as microzooplankton or detrital material within sea ice (Schmidt et al., 2014; Virtue et al., 2016). There is a strong correlation between sea ice extent and krill populations, which varies regionally (Massom et al., 2006; Atkinson et al., 2019), while the timings of annual sea-ice advance and retreat are another important factor (Quetin et al., 2007). In the Antarctic Peninsula region, krill recruitment is directly correlated with winter sea ice extent (Loeb and Siegel 1995; Johnston et al., 2022). Warming and ice loss has led krill populations in the Southwest Atlantic sector to contract southward. Numerical densities have declined sharply and the population has become more concentrated towards the Antarctic shelves (Atkinson et al., 2019). However, how representative these findings are for the entire Southern Ocean remains unknown and needs to be investigated.
Crystal krill, Euphausia crystallorophias, has a circumpolar distribution and is a smaller counterpart of Antarctic krill, replacing it in neritic environments (Harrington and Thomas, 1987; Thomas and Green, 1988; Ainley et al., 2004; Johnston et al., 2022). Highest crystal krill densities are generally associated with ice shelves and coastal polynyas (Boysen Ennen et al., 1991; Pakhomov and Perissinotto, 1996; La et al., 2015; Davis et al., 2017). The crystal krill synchronise the its reproduction with coastal sea ice conditions including the appearance and persistence of polynyas. Spawning is initiated under fast ice but peaks during coastal polynya breakout (Pakhomov and Perissinotto, 1996). Eggs of crystal krill are neutrally buoyant, which allows them to concentrate in the upper layers, while hatched larvae are often associated with sea ice (Pakhomov and Perissinotto, 1996; Daly and Zimmerman, 2004; Wiebe et al., 2011). Crystal krill has a narrow thermal habitat restricted to coldest, coastal waters and thus can be vulnerable to climate change (Guglielmo et al., 2009; Papot et al., 2016). Changes in sea ice “dynamics”, potential pelagic ecosystem modifications and shift of the Antarctic krill into the coastal environments under climate change may rebalance interactions and increase competition between two species, although with unknown outcomes at this point (Smith et al., 2014; Papot et al., 2016). Nevertheless, coastal seas with large continental shelves may provide crystal krill with refugia pockets, limiting its range.
3.4. Salps (Chordata)
Salps are gelatinous organisms that are found over a wide area of the Southern Ocean and are most numerous north and south of the Antarctic Polar Front (Foxton, 1966; Pakhomov et al., 2002; Johnston et al., 2022). Salps exhibit a complex life cycle and reproduction consisting of alternating generations of sexual (colonial forms or blastozoids) and asexual (single forms or oozoids) forms that differ morphologically (Foxton, 1966; Bone and Trueman, 1983). Salps can pump water through their internal cavity using their muscles, to create water currents that they use for propulsion, breathing, and feeding (Bone et al., 2000). Salps are indiscriminate filter feeders and ingest a wide range of particles, from 1 μm (bacteria) to several mm (small crustaceans; Sutherland et al., 2010) in size and, based on lipids and molecular observations, flagellates are a large component of their diet (von Harbou et al., 2011; Metfies et al., 2014). It has been documented that at high chlorophyll a concentrations (>>1 mg m−3) salps can suffer clogging of the feeding mesh and are rarely found in regions of dense phytoplankton blooms (Perissinotto and Pakhomov, 1998). Observations of the faecal pellets of salps suggest that some of these larger particles are passed through the digestive tract relatively intact and are therefore lost to epipelagic food webs as the heavy pellets sink rapidly out of the euphotic zone (Cabanes et al., 2017; Iversen et al., 2017; Pauli et al., 2021a). They appear to flourish in warmer waters in the Southern Ocean and in regions of low to moderate chlorophyll a concentration (Loeb et al., 1997; Pakhomov et al., 2002; Pillai et al., 2018). In the sea ice zones of the Southern Ocean, information about salps is limited but it is noted that in the Antarctic Peninsula region, where temperatures are warming much faster than the global average, they replace krill on a regular basis and this appears to be contingent on sea ice conditions (Pakhomov et al., 2002; Atkinson et al., 2004). Over the last century, salps expanded southward, which increased their spatial overlap with the Antarctic krill distribution range (Atkinson et al., 2004). However, salp populations in the high Antarctic are not permanently established (Pakhomov et al., 2011). Although salps have been observed under the seasonal sea ice (Perissinotto and Pakhomov, 1998; Pakhomov et al., 2018), krill are not yet replaced by salps because the species occupy different water masses and salps do not respond directly to sea ice conditions.
3.5. Fish (Chordata)
Sea ice in the Southern Ocean is an important habitat for a variety of notothenioid fish species, traditionally defined as “cryopelagic” species (Andriashev, 1987). Trematomus borchgrevinki is the best known ice-associated fish, with adaptations for cryopelagic life including camouflage against the background colour of the ice owing to silvery reflective layers beneath the skin and in the eyes, and protection from freezing provided by the high quantity of antifreeze glycoproteins in their body fluids (Eastman and DeVries, 1985). Other notothenioid species, which also contain antifreeze glycoproteins and associate with sea ice, include Trematomus amphitreta, Trematomus (=Pagothenia) brachysoma and Trematomus (=Cryothenia) peninsulae (Duhamel et al., 2014). Cryopelagic fishes often stay inactive, in an upside-down position, with their ventral side against the ice, and move erratically over short distances, possibly using the underside of the sea ice as a resting structure to reduce energetic requirements (Gon and Heemstra, 1990; Kock, 1992; Gutt, 2002). Large adult specimens of T. borchgrevinki swim slowly, in the normal position, in close vicinity to the sea ice, and hide between crevices (Eastman, 1993; Gutt, 2002), using the sea ice as a protective structure to avoid predation.
Although not included in the list of cryopelagic species, there are species whose distribution is influenced by the presence/absence of sea-ice cover; e.g. Trematomus newnesi occupy a cryopelagic niche as juveniles (Duhamel et al., 2014). Although living in swarms and being largely planktivorous, T. newnesi feeds on sea ice-associated fauna (Casaux et al., 1990; La Mesa et al., 2000). Trematomus eulepidotus occurs in the shallow coastal waters of Terra Nova Bay early in the summer, when the area is covered by sea ice, while moving into deep waters later in the season and after the sea-ice melting, thus suggesting an advantageous use of the sea-ice cover as shelter by this species (Vacchi, personal observation).
Some other fish species are linked to the sea ice during a crucial phase of their life, notably their embryonic development. Dragonfish Gymnodraco acuticeps eggs have been found in nests in shallow waters amongst anchor ice (submerged fast ice that is attached to the bottom), and ready-to-hatch eggs of T. borchgrevinki have been collected within a crevice on the side of an iceberg (Cziko et al., 2006). The most striking example is provided by the Antarctic silverfish Pleuragramma antarcticum (a commonly cited synonym is P. antarctica), whose developing eggs have been found dispersed in the platelet ice layer under the solid fast ice at Terra Nova Bay (Ross Sea), where they form the only known to-date silverfish hatching area (Ghigliotti et al., 2017). Survival of embryos and early larvae in that icy environment is possibly due to a suite of morphological adaptations (Cziko et al., 2006; Bottaro et al., 2009). Furthermore, the platelet-ice layer with its tridimensional crystal lattice structure provides protection from large predators (Guidetti et al., 2015).
4. Qualitative network modelling
In the following sections, we use qualitative network modelling (based on Melbourne-Thomas et al., 2012) to investigate potential synergistic, antagonistic and cascading effects on the ecosystem, given long-term change in the physical and chemical nature of the sea ice system. Details of the rationale behind qualitative network modelling are presented in Appendix. In short, we applied the network diagraph approach of Melbourne-Thomas et al. (2012) to construct a qualitative network model where plausible interactions (edges) between the components (nodes) are either positive, neutral or negative, but with no quantitative magnitude of change. Such a network can be used to synthesise information on individual relationships between nodes to better capture the chains of interactions (cascades), compounding effects (synergisms, antagonisms) and positive and negative feedback loops. Many simulations (up to 10,000) can then determine probabilities of nodes increasing, decreasing or not changing when one or more of the physical attributes of the system are permanently changed (positively or negatively), in a process known as a press perturbation (Melbourne-Thomas et al., 2012). We undertook several press perturbations using the R package QPress (Melbourne-Thomas et al., 2012). In describing the outcomes, we focus on those impacted nodes where the directional change has a probability greater than or equal to 0.6, but report all probabilities.
The interactions between the different physical and biological components investigated are shown in Figure 3. The habitat variables that were considered in the model are precipitation (falling as rain or snow, depending on temperature), snow cover thickness, habitat in the sea ice (complexity, e.g., ridging and rafting, total ice volume, a function of area, duration, and thickness, and brine volume), platelet ice, nutrients in the ice and pelagic and light (in the autumn, winter and spring seasons; Table 2). Biotic variables that were included are ice algae and seasonal phytoplankton, copepods and Antarctic krill (with larvae of both groups as a separate node), salps, fish (myctophids, Pleuragramma antarcticum) and ecosystem metabolism in the ice and pelagial (Table 2). The nodes and edges are simplified from a more complex system structure. Nodes may represent many attributes that interact with other nodes in the same way. Most edges are self-explanatory and represent the links between nodes. Some edges represent the outcome of complex interactions rather than a full chain of events in order to avoid unwanted feedbacks in the qualitative model. For example, an increase in the proportion of ice that is brine will give rise to a decrease in nutrients released from the ice in spring. This is because the increase in brine increases the availability of particulate nutrients in the ice to algal production, which therefore reduces the particulate nutrients in the ice released in the spring melt. A different combination of edges would make this difficult to represent. Similarly, we have not represented the full complexity of the brine system food web, which in some regions contains many small meiofaunal species with numerous trophic interactions.
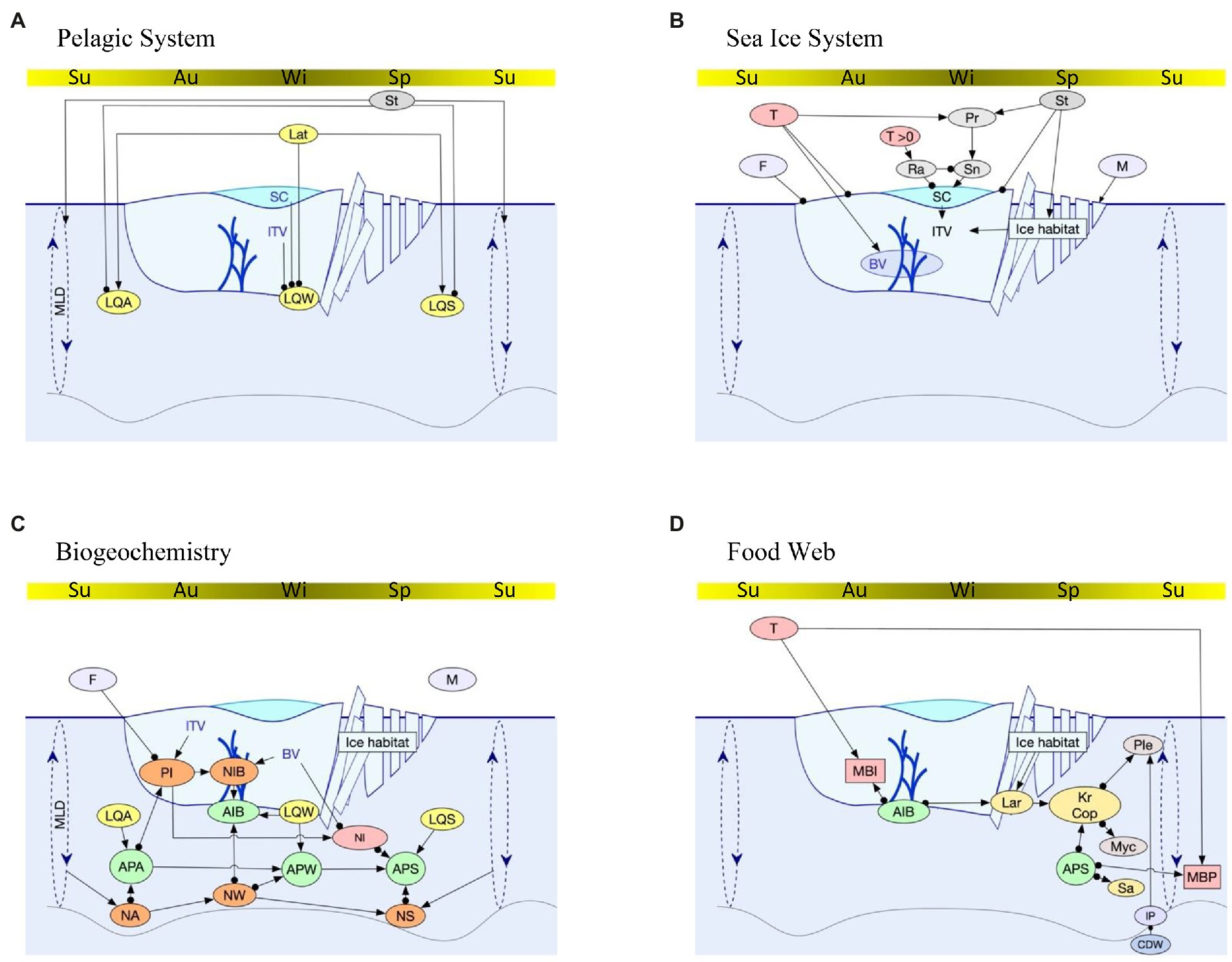
Figure 3. Nodes and linkages (edges) used in the qualitative network model. Each panel represents a part of the overall sea ice system. Top bar on a panel represents annual seasonal sea ice cycle from summer, autumn, winter, spring, summer. The remainder of a panel from top to bottom represents atmosphere, sea ice and pelagic realm with the bottom line indicating the bottom of the mixed layer. Cumulative symbols are as follows (Explanations in Table 2): (A) Pelagic system: Lat = Latitude; SC = Snow cover; ITV = Ice total volume; MLD = Mixed layer depth; LQA/LQW/LQS = Photosynthetically active radiation in autumn, winter, spring. (B) Sea ice system: T = Temperature; T > 0 is air temperatures >0°C. St = Storms; Pr = Precipitation; Ra = Rain; Sn = snow; F = Freezing; M = Melting; BV = Brine volume. (C) Biogeochemistry: PI = Particulates taken up into ice; NIB = Nutrients in sea ice brine; AIB = Algae at lower sea ice boundary; APA/APW/APS = algae in pelagic waters in autumn, winter, spring; NI = Nutrients from melting sea ice; NA/NW/NS = Nutrients in pelagic water in autumn, winter, spring. (D) Food web: MBI/MBP = Biological metabolism in sea ice, pelagic; CDW = Circumpolar Deep Water; IP = Platelet Ice; Lar = Larvae; Kr = Antarctic Krill; Cop = Copepods; Ple = Pleuragramma; Myc = Myctophids; Sa = Salps.
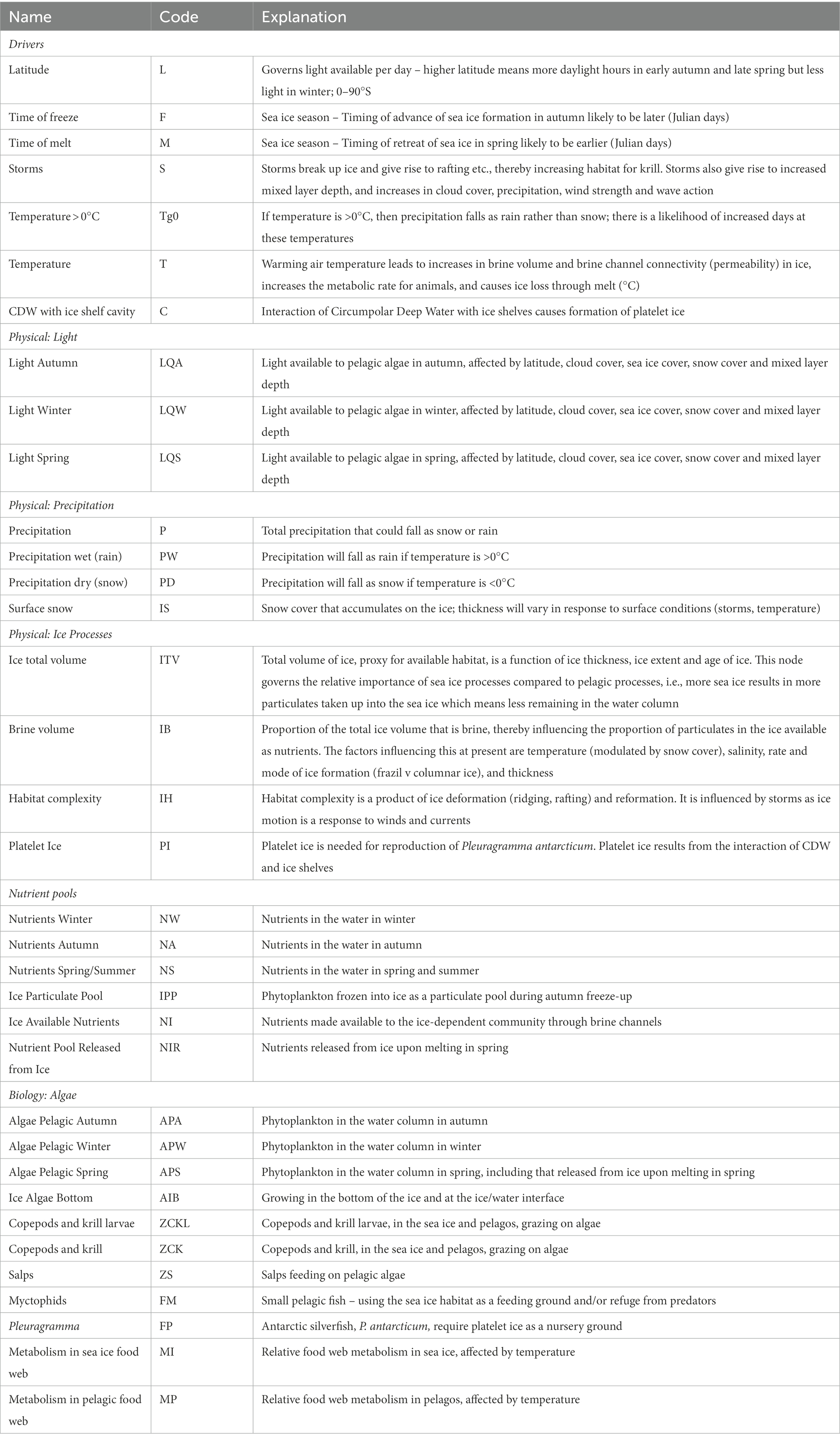
Table 2. Description of the nodes used in the qualitative network model, as displayed in Figure 3.
5. Response of sea ice and biota to spatial and temporal shifts in sea ice habitats
The primary drivers of sea ice habitats that are considered in this paper are ocean temperature, air temperatures changing the type of precipitation, storminess, change in the length of sea ice season, the potential effects of Circumpolar Deep Water, and the latitude in which the system is located. We use the qualitative network model to understand how changes in all of these drivers may impact the sea ice physical and biological systems. First, to assist our understanding of the roles of different drivers, we examined the effects of changes in individual drivers according to common hypotheses. Second, we applied known differences in the drivers around Antarctica to investigate the likely differences in sea ice systems between MEASO sectors. Last, we examined the consequences of future change in the drivers for Antarctic sea ice systems.
5.1. Responses of sea ice systems to change in individual drivers
The following hypotheses were tested with the qualitative network model, with the results presented in Table 3:
1. Increasing temperature leads to an increase in the relative cost of sympagic and pelagic metabolism and a decrease in biomass;
2. Increasing air temperatures (number of days when T > 0°C) will result in decreased habitat and increasing light, and therefore pelagic primary productivity will increase;
3. Storminess will increase habitat complexity, which will lead to increases in sympagic biota;
4. Later freezing will lead to less habitat space available for primary producers and ice algae biomass will decrease;
5. Earlier melting of sea ice will lead to decreased abundance of ice-associated species;
6. Increasing Circumpolar Deep Water (CDW) will result in less platelet ice and therefore the fish Pleuragramma antarcticum will decrease in abundance and biomass;
7. Higher latitude ecosystems are subjected to less light from late autumn. Increased latitude means shorter days in winter and longer days in summer, shifting primary production away from winter to the advantage of the pelagic system.
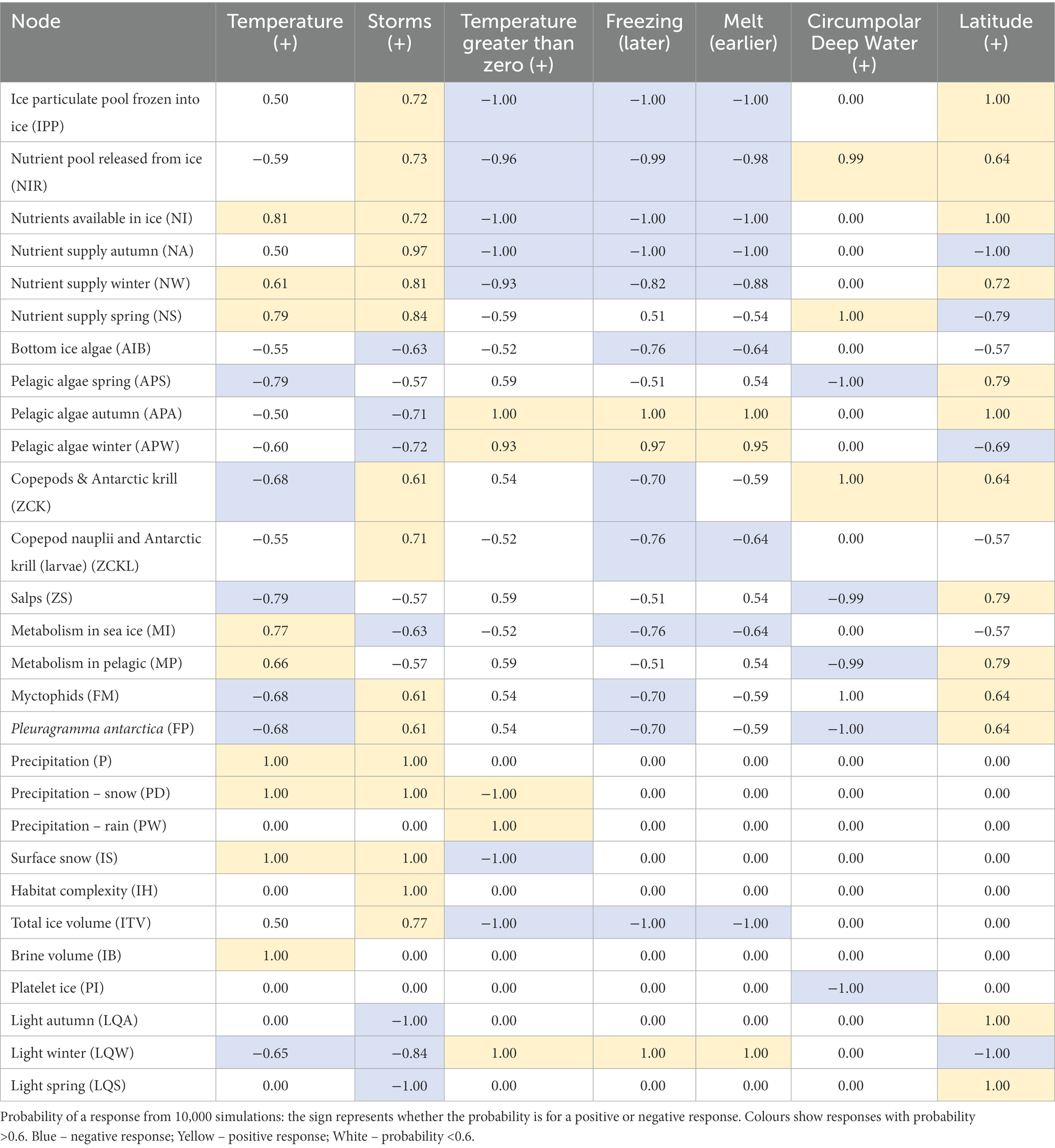
Table 3. Assessment of the impacts of key physical drivers of change on the magnitude of food web components associated with sea ice, based on the Qualitative Network Modelling.
5.1.1. Change in physical habitat
Increasing temperature would contribute to a decrease in total ice volume over a season, although the decrease would be most likely if atmospheric temperature was >0°C due to an increase in precipitation as rain. The proportion of the ice as brine would also increase. Temperature of sea ice directly affects porosity and permeability, chemical composition of the pore microstructure and salinity. At −5°C, the ice fraction of brine represents 35%, and the salinity of the brine is around 87 (Golden et al., 1998). At −30°C, the brine fraction falls to below 8%, reducing the habitat available for microorganisms, while the salinity of brine increases (Ewert and Deming, 2013), consequently restricting the habitat space to only species that are tolerant to high salinities. Based on the relationships between temperature and brine volume, and given warming air temperatures (Tg0 increasing), brine volume, and therefore available habitat, will increase on scales <10 mm. However, warming will also increase melting, leading to reduced duration and thickness of the ice cover on the larger scale.
Increased storminess can be described as increased wind speed, higher rates of precipitation (under blizzard conditions) and increased strength and duration of waves. These changes will have complex and possibly contradictory implications for the Southern Ocean sea ice system. Increased windiness will increase wave energy, possibly resulting in deeper ocean mixing while also bringing warmer waters to the surface; this could then increase the loss of ice through basal melt. Increased storminess could also enhance deformation of the ice, leading to greater habitat complexity (IH) and ice total volume (ITV, depending on coincident change in ice areal coverage – also affected by changes in wind direction). Notably, habitat complexity was directly related to storminess in our model. Yet the influences on sea ice-dependent species may be more complex than just the relationship with habitat complexity. Many of the responses relate to changes in extent and duration of sea ice, the timing of the sea ice season relative to the spring and autumn blooms, and the relative importance of pelagic algae and ice algae in the productivity of species.
5.1.2. Responses of the biological system
i. Increasing temperature. Warming produced positive responses in relative metabolism for both sympagic and pelagic communities, while negative or neutral responses in the algae, copepods, krill, salps and fish suggest that their overall numbers would either decrease (negative) or remain the same (neutral). An increase in temperature is likely to result in more resources going towards metabolism rather than growth of individuals and reproduction. Thus an increase in temperature means an increase in the cost of metabolism relative to the production of biomass, resulting in a reduction in biomass.
ii. Increase in the number of days >0°C. Increases in the number of days that are above 0°C produced opposing responses in the two habitats, whereby sea ice metabolism decreased and pelagic metabolism increased. As expected, pelagic algae increased due to the reduced ice cover and an increase in light. Ice algae decreased, most likely due to a reduction of the available habitat. There were increases in krill, copepods, salps and fish, though the larvae of krill and copepods decreased, indicating the contrasting influences of the pelagic and ice habitats, respectively. Larval and juvenile krill are highly ice-dependent, and consequently any perturbations in sea ice deformation, thickness and extent (species-specific sea ice “quality”) will directly affect population dynamics (Melbourne-Thomas et al., 2016). Nevertheless, decreased survival of larvae may be offset by increased biomass accumulation by adults. The qualitative model is unable to differentiate between the relative importance of adult productivity and larval/juvenile survival to the overall dynamics of the populations. How increases in adult survival may balance out concurrent decreases in juvenile recruitment depends on the strength of the responses, and cannot be determined from a qualitative network model. Thus the effects on the total population size are unknown.
iii. Storminess. Increased storminess led to decreased light, increased ice habitat and ice volume and concomitant decreases in ice algae and phytoplankton. Algae require a minimum day length to initiate growth so increased cloud cover, particularly in early spring, could decrease the growing season. In contrast, storminess could enhance deep mixing, bringing warmer, nutrient-rich, iron-rich water to the surface, resulting in favourable conditions for algal growth; overall, the model results suggests that light is the primary factor influencing algae in the sea ice and water column. Krill and copepods benefit from the increased habitat that can result from greater storminess, e.g., greater habitat area provides a refuge for krill (Meyer et al., 2017, 2020). The increases in prey could be beneficial for ice-associated fish that are feeding on crustaceans.
iv. Later freezing and v. earlier melting. The model simulations show negative responses from krill, copepods and fish and a decrease in larvae of krill and copepods, largely from the reduced volume of sea ice. Lower numbers of crustacean larvae could be a phenological response to reduced annual duration of the ice cover, preventing the ice-associated species from completing their life cycles; e.g. early retreat of sea ice leads to poor recruitment of krill in warm years (Loeb and Santora, 2015). Salps on the other hand responded positively to reduced sea ice coverage, which is to be expected based on observations that salps thrive under conditions of reduced ice cover (Quetin et al., 2007; Loeb and Santora, 2015). At the same time, lower duration of sea ice coverage could lead to increases in phytoplankton in spring as the amount of light reaching the surface layers is increased and sets off the open-ocean bloom earlier in the year.
vi. Increasing Circumpolar Deep Water (CDW). Increased CDW resulted in a negative response in platelet ice and the fish P. antarcticum. Upwelled CDW near ice shelves leads to basal melting, and, consequently, platelet ice production is reduced (as per Hoppmann et al., 2020). As P. antarcticum requires platelet ice for early life stages (eggs and larvae; Guglielmo et al., 1998; Vacchi et al., 2004, 2012; Ghigliotti et al., 2017), reduction in this ice type leads to poor recruitment of the fish. Evidence from the Antarctic Peninsula (La Mesa et al., 2015; Corso et al., 2022) has shown that numbers of P. antarcticum have decreased substantially in line with reductions in sea ice. Consequent effects include increases in copepods and krill and their predators, myctophid fish, while salps decrease.
vii. Increased Latitude (L). Increased latitude means shorter days in winter and longer days in summer, shifting primary production away from winter to the advantage of the pelagic system.
5.2. Expectations for differences between Marine Ecosystem Assessment for the Southern Ocean sectors
Using an assessment of drivers in each sector, following Morley et al. (2020), we examined how the response of biota associated with the sea ice system might vary between the five MEASO sectors (Table 4). We compare how the different sectors might vary relative to the Atlantic Sector because of that sector having a mid-range sea-surface temperature regime in the Antarctic Zone compared to the other sectors.
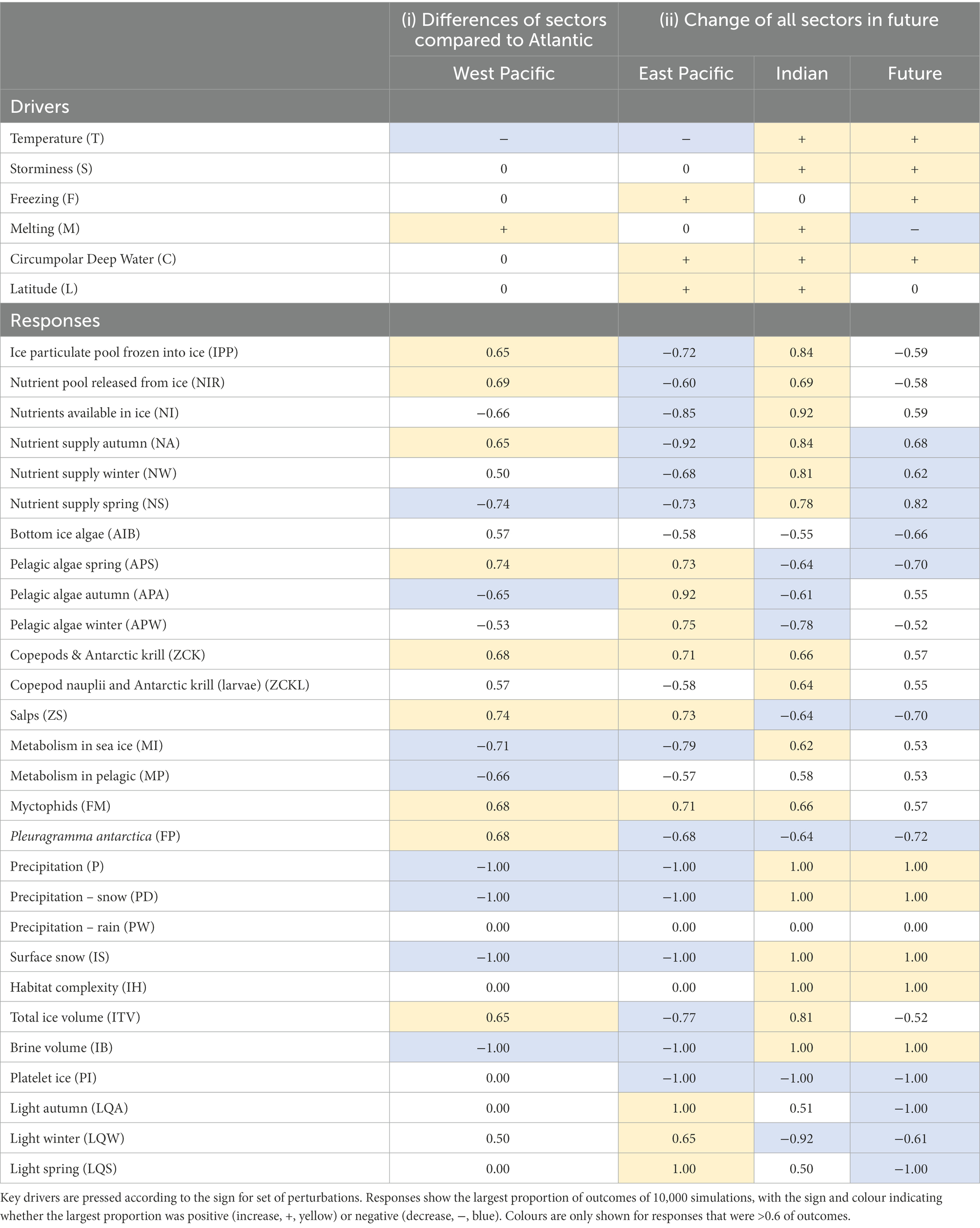
Table 4. Responses of attributes of the sea ice system from qualitative press perturbations of (i) differences in key drivers between MEASO sectors (see Figure 2) relative to the Atlantic Sector, and (ii) changes in the key drivers in all sectors into the future.
Table 1 outlines differences in sectors based on available literature. If the global temperature warms by the expected 1.5°C by 2030, it is likely that the Antarctic Peninsula, particularly the northern region, will experience up to 130 days above 0°C (Siegert et al., 2019). Ocean-air interactions will lead to increased ocean turbulence resulting in shoaling of CDW. Increased heating in shallow waters is contributing to the basal melting of ice shelves and, consequently, a reduction in the formation of platelet ice (Hoppmann et al., 2020). Positive CDW incursions yield decreased platelet ice in the East Pacific and Indian sectors compared to the Atlantic, giving an expectation that Pleuragramma antarticum would be less in number in those sectors than the Atlantic. In contrast, while CDW incursions are not expected to be different in the Atlantic sector and the West Pacific (Ross Sea), changes to P. antarcticum are expected to be larger in the Ross Sea due to other factors such as difference in productivity, through less metabolic demand in the Ross Sea and greater spring algal growth (Table 4). Silverfish comprise up to 90% of the mid-water fish biomass in the Ross Sea (Smith et al., 2007) and are prey for Weddell Seals, whales, Adélie penguins, emperor penguins and other seabirds (Smith et al., 2007). Sampling for P. antarcticum along the western Antarctic Peninusula (wAP) has shown that they are severely reduced in number around the central wAP, a region where they were historically abundant (La Mesa et al., 2015). This was linked by the authors to catastrophic loss of spawning habitat as a result of sea ice loss. The loss of silverfish as a prey item in the central wAP is likely leading to marine predators in the region exhibiting dietary switching, e.g., to myctophids (Moline et al., 2008), for which there is an expectation in our results that myctophids will be more abundant in the East Pacific.
The perturbation responses for the sea ice system in the Pacific sectors are consistent with observations. Sea ice extent and duration are currently increasing in the Ross Sea and decreasing in the Amundsen Sea (Comiso et al., 2011; Stammerjohn et al., 2012; Parkinson, 2019). In the Ross Sea, colder sea surface temperatue (SST) and/or more southerly winds lead to a northward extensions of the productive marginal ice zone (MIZ), highlighted in Table 4 by an increase in pelagic algae in the East Pacfic sector in spring, though no change occurred in the bottom ice algae. Algae contained in the ice are released by earlier melting, then mixed in the upper layers of the water column to be consumed by meso- and microzooplankton grazing (Hecq et al., 2000). Copepods and krill also increased, while their larvae decreased, perhaps indicating that earlier melting meant less habitat space available for the young stages, reinforcing the importance of adult productivity compared to recruitment driving this population. A reduction in light penetration through the ice may limit sea ice algal biomass, with consequences for krill and other zooplankton. Krill collected under less deformed, thinner ice with less snow cover had higher lipid levels as a result of a higher biomass of sea ice biota (Virtue et al., 2016). Models also indicate that Antarctic marginal ice zones are important areas of carbon export driven by krill grazing and faecal pellet production (Belcher et al., 2019). Phytoplankton blooms form at retreating ice-edges due to a combination of melt-water stratification, and release of micronutrients (e.g., iron) and algae from the melting sea ice (Lancelot et al., 1993; Lannuzel et al., 2016). Ice-edges serve as hotspots for pelagic food-web interactions by providing critical food for pelagic herbivores (Schmidt et al., 2018), which, in turn, are preyed on by higher trophic levels.
Antarctic krill, copepods and their larvae showed mixed responses to the environmental perturbations (Table 4). The Southwest Atlantic (20oW–80oW), which holds >50% of the circumpolar krill stock (Atkinson et al., 2019) has warmed rapidly over the last decades. Their distribution has contracted southward by ~440 km over the past 90 years and become centred more strongly over Antarctic continental shelves (Atkinson et al., 2019). Krill further showed an increase of ~75% in body mass, indicating the presence of more adults and less juveniles in the population and, consequently, a reduction in recruitment. No directional trends in krill population and sea ice have been established outside of the Atlantic sector (Flores et al., 2012).
Our perturbation experiments give an expectation that Antarctic krill would have better conditions in the other sectors in relation to the sea ice zone. These perturbations do not account for total productivity and the size of the area in which that occurs. Nevertheless Antarctic krill populations in other sectors may be relatively stable at present (Yang et al., 2020). With more stable sea ice coverage, no significant evidence for decline in krill stocks in the Indian sector was found (Nicol et al., 2000; Atkinson et al., 2017). The Ross Sea represents a mosaic of functionally different marine subsystems. The environmental changes in the Ross Sea could benefit diatoms and change krill distributions in future, although krill were not considered able to invade much of the continental shelf owing to the requirement for larval development at great depth (Smith et al., 2014). In Terra Nova Bay, large amounts of biopolymeric carbon accumulated in the bottom layer of the ice column concomitantly with the early spring increase in sympagic algal biomass. Such organic material, mostly accounted for by autotrophic biomass, was characterised by high food quality and was rapidly exported to the sea bottom during sea ice melting (Pusceddu et al., 2000). The Amundsen Sea was considered to be a potential new habitat supporting successful krill spawning in future due to delayed sea ice formation and smaller ice concentration which could benefit phytoplankton blooms and facilitate krill recruitment (Piñones and Fedorov, 2016). These models suggest that other regions besides the Atlantic sector could be potential krill biomass hotspots in future.
5.3. Expectations for future change
The fate of Antarctic sea ice under a range of greenhouse gas emissions scenarios to 2,100 has been investigated under the auspices of the IPCC’s various assessment reports, underpinned by the Coupled Model Intercomparison Project (CMIPs). However, confidence in long-term projection of Antarctic sea ice extent is currently low due to the inability of many global climate models (GCMs) to accurately reproduce large-scale patterns observed by satellite, i.e., either the seasonal cycle or multi-decadal trends (Turner et al., 2013). Moreover, model estimates of changes in Antarctic sea ice thickness (and hence volume) are of even lower confidence (IPCC, 2021). This is due to a lack (until recently) of reliable large-scale snow and ice freeboard estimates required for accurate computation of the thickness of both sea ice (Paul et al., 2018; Fons and Kurtz, 2019; Kacimi and Kwok, 2020) and its snow cover (Webster et al., 2018). Nevertheless, the former CMIP5 models project a range of decreases in Antarctic sea ice extent of between 8% and 67% for the 21st Century, depending on month of trend and emissions scenario (Collins et al., 2013). Most models predict nearly ice-free summertime (minimum extent) conditions by 2,100 under the highest emissions scenario, known as RCP 8.5 (Collins et al., 2013). There are currently no projections for coastal fast ice, since this ice type is currently not represented in any GCM.
Application of the environmental perturbations to future conditions produced only negative and neutral responses for krill and copepods, including their larvae (Table 4). Changes in the extent and area of the sea ice habitat have not been uniform or unidirectional, so the implications for sea ice-associated organisms are not clear. The magnitude and timing of the spring ice-associated algal bloom has changed over the last three decades and this change is directly related to sea-ice extent and duration of the previous winter (Stammerjohn et al., 2008, 2015). Changes in the timing of annual sea ice advance and retreat will impact the capacity for ice-associated microbes to initiate bloom events in spring. For example, ice algae are a food source for larval Antarctic krill during late winter and early spring. Other studies have highlighted the role of complex under- and intra-ice habitats in providing shelter for larval krill (Frazer et al., 2002; Massom et al., 2006; Meyer et al., 2017) and the importance of sea-ice drift patterns in transporting krill larvae to areas in which they can thrive in spring (Meyer et al., 2017). Strong relationships have also been established between sea-ice conditions (e.g., duration/persistence), their influence on ice algal carbon productivity and export, and subsequently, on coastal benthic community characteristics (e.g., Clark et al., 2017; Cummings et al., 2018). Few copepod species appear to be obligate ice dwellers. The harpacticoid copepod Drescheriella glacialis seems to be very ice-dependent due to its poor swimming capabilities, and Stephos longipes has acquired antifreeze protection (Kiko, 2010), which also indicates a high level of adaptation to the ice environment. It is possible that copepods can continue to thrive under scenarios of thinner or less frequent ice cover, as plasticity in their diets and life cycles might be the key to their continual success (e.g., Swadling et al., 2004). Understanding the relative importance of factors that drive organisms to colonise sea ice (e.g., response to predation versus reproductive needs) would enhance our ability to predict their responses to environmental change.
Salps responded positively to increased storminess and the number of days >0°C and negatively to a positive press perturbation of CDW (Table 4). There appears to be considerable spatial segregation between krill and salp populations (Nicol et al., 2000; Atkinson et al., 2004), although a comparative study of their feeding behaviour showed similar diets, which might enhance the competition between both species (Pauli et al., 2021b). Currently it is assumed that temperature, sea ice and chlorophyll a drive large-scale population segregation between the two species, at least in the Indian sector (Nicol et al., 2000). Observations from the wAP also indicate that E. superba and S. thompsoni rarely, if ever, co-exist in high abundances in that region (Pakhomov et al., 2002; Steinberg et al., 2015) and instead oscillate between years of salp dominance and years of krill dominance (Ross et al., 2014). However, the rapid reproduction capacity of salps under favorable conditions could change current ecosystem structure and thus alter the behaviour and distribution of E. superba. The direct effect of increased S. thompsoni presence on E. superba populations, however, is unknown.
6. Discussion
The sea ice environment is pivotal for the Southern Ocean ecosystem and is likely to change (Meredith et al., 2019) over the 21st Century. There is high confidence that changes in meridional wind stress will have a strong effect on sea ice variability (IPCC, 2021), and Southern Ocean westerlies are expected to intensify. Understanding how sea ice will respond to environmental change is key to predicting the effects on the biota that rely on sea ice for all or part of their life cycles. Some species are already adapted to the ephemeral nature of sea ice, so it is the magnitude and rate of change to this habitat that might be key determinants of how well species fare in the future. For these species, developing a means of weighting CMIP models for their ability to represent the Southern Ocean sea ice system is an important step to making informative predictions about the future of sympagic and pelagic biota. Climate variations may influence the duration of sea ice by disrupting the timing of formation and retreat and thus primary productivity, with consequences for entire food webs (Seibel and Dierssen, 2003; McCormack et al., 2021; Murphy et al., 2021). One example is differences between phytoplankton assemblages, with blooms of Phaeocystis antarctica often dominating in the Ross Sea vs. diatoms occurring in the WAP (Ducklow et al., 2007; Smith et al., 2007; Mangoni et al., 2017; Schofield et al., 2017; Pinkerton et al., 2021).
Our qualitative network model showed realistic expectations for differences between sectors. For that reason, it is a useful tool for exploring different future scenarios for the ecosystems in different sectors, given that changes in the physical system are likely to vary in more nuanced ways than the future scenario we present here. A key lesson from our results is that different taxa did not respond in a consistent direction (positive or negative) across all environmental perturbations, highlighting the complexities of networks of interactions between the physical and biological variables. Increasing storminess produced positive responses in the pelagic nutrient pools, possibly due to deeper ocean mixing bringing nutrient-rich waters to the surface. At the same time, warming temperatures might lead to increased stratification trapping nutrients at depth. If temperature is the more important driver, then we might expect to observe decreases in biomass of phytoplankton and grazers.
Species that require the presence of sea ice are more-or-less certain to fare badly under reduced ice scenarios, e.g., the dependency of the silverfish P. antarcticum on platelet ice during its early development. Kramer et al. (2011) also argued that the perennial sea ice of the Weddell Sea is colonised by highly adapted species that probably rely on year-round sea ice (e.g., D. glacialis and T. antarcticus), and therefore a change to a seasonal ice cover might be detrimental for these species. Also S. longipes might be adversely impacted by sea ice loss, as it seems to rely on sea ice as a nursery ground. In this case, the seasonal timing of sea ice formation and melting might also be important. Other species, such as Paralabidocera antarctica, appear to be facultative rather obligate inhabitants of sea ice (Arndt and Swadling, 2006) and might be able to adjust their life history strategies to meet their needs in a reduced sea-ice world.
Of course, it is most likely that species will have variable responses to changing sea-ice conditions and will depend upon how much their populations as a whole depend on a sea-ice phase in their overall dynamics, coupled with the potential for non-linear responses by various taxa (Constable et al., 2014). However, for benthic organisms it is already predicted that there will be “more losers than winners” (Griffiths et al., 2017). It is these complex adjustments in biology and ecology that will pose the biggest challenges to attempts to manage the Southern Ocean ecosystem (Atkinson et al., 2019).
Temperature affects sea ice structure, freeze/melt and extent and its carrying capacity for biota, expressed as biomass, at the same time as impacting metabolism in a system. The qualitative network model showed metabolism increased while primary producers and grazers decreased with increasing temperatures. However, as the model is purely qualitative, it is not possible to scale metabolism according to the biomass of each component and to obtain a measurable response from a change in temperature. Carrying capacity is determined by complex interactions between habitat complexity (e.g., deformation processes), the volume of brine available for colonisation and the total volume of the ice. Earlier we discussed how increased ice complexity can improve outcomes for larval krill (Frazer et al., 2002; Meyer et al., 2017) by providing them with refuge from predators and competitors. This underlines the importance of better defining the relationships between Antarctic sea ice physical properties and processes and biological and ecological processes, as they relate to optimal “habitat quality” for key species, including at different life stages (e.g., Quetin et al., 2007). As stated by Janssen et al. (2021), this is critical for robust projections of future states of the Southern Ocean ecosystem. A critical step will be to evolve the qualitative model into a quantitative model where the effects of quantitative change in the drivers can be better explored.
Understanding the ways that biota will respond between and within sectors is also a high priority. This research should be undertaken at a multi-national level, with sampling strategies designed to facilitate our understanding of poorly-studied regions along with those where we have better information (e.g., the Southern Ocean Observing System 2021–2025 Science and Implementation Plan, Newman et al., 2022). There is patchy data coverage between sectors and the key taxonomic groups have been sampled on varying timescales. We know that sea ice is not changing uni-directionally, with some regions (primarily the southwestern Atlantic and eastern Pacific) showing rapid change while other regions (Ross, Indian) are showing some increases or not changing substantially – although there was an unexpected switch to high variability in 2012 (Parkinson, 2019). Additionally, as fast ice contains high biomass of true ice inhabitants (e.g., the algae and small grazers), being able to predict whether it will increase, decrease or stay the same is important and is an area needing attention.
Understanding and quantifying these changes will require rigorous scientific data collection drawn from species and ecosystems that are sensitive to multiple stressors of climate change, using novel field and laboratory technologies and methodologies to fill knowledge gaps in our understanding of the fundamental biology and ecology of many Southern Ocean species. This should ideally involve long-term cross-disciplinary monitoring of the coupled interactive sea ice-ocean–atmosphere-biology-biogeochemistry system in key regions, i.e., along the lines of the long-running Palmer Long-Term Ecological Research program (Ducklow et al., 2013) – noting that Antarctica’s sea ice habitat is also affected by ice sheet and ice shelf melt and icebergs (Massom and Stammerjohn, 2010).
7. Key messages for policy makers
Key message 1 Sea ice in the Southern Ocean is fundamentally important to the lower trophic levels of this ecosystem, including for primary productivity, krill and fish. This has major implicatons for marine predators, including marine mammals, penguins and other bird species, that collectively support different parts of the Antarctic marine food webs (very high confidence).
Key message 2 The sea-ice-system, including fast ice and the marginal ice zone, is threatened by both atmospheric and ocean warming as well as strengthening oceanic winds as a result of climate change, but the timing and magnitude of these impacts is uncertain (high confidence).
Key message 3 Reduction in global greenshouse gas emissions is the only mitigation approach as sea ice is too expansive to be manipulated by direct human intervention (statement of fact).
Key message 4 While reduced sea-ice extent may impact the range of sea-ice dependent species examined here, such as Antarctic krill, the increased complexity of habitat may be a positive local benefit to these species (medium confidence).
Key message 5 Whole-of-system research on the role of sea ice in Antarctic ecosystems needs to be extended beyond the Antarctic Peninsula and Weddell Sea to establish a circum-Antarctic understanding of the coupled physical-chemical-biological sea ice system.
Key message 6 Predicting how perturbations in sea-ice extent and duration will impact Antarctic krill populations and in turn the Southern Ocean ecosystem requires both regionally-focussed and multidisciplinary experimental approaches, together with coupled physical and biological models of the sea-ice system embedded within Earth System models.
Data availability statement
The original contributions presented in the study are included in the article/supplementary material, further inquiries can be directed to the corresponding author.
Author contributions
KS and AC conceived the study. AF, RAM, MB, LGh, AG, LGu, NJ, SK, FK, RK, PK, RM, AMa, AMc, MM, EP, IP, JR, PR, KR, MV, PV, CW, PW, and SW wrote sections and/or provided comprehensive comments. All authors contributed to the article and approved the submitted version.
Funding
This project received grant funding from the Australian Government’s as part of the Antarctic Science Collaboration Initiative program. KS, AF, SK, RAM, PR, CW, and PW are supported by the Institute for Marine and Antarctic Studies. RAM, SK, and SW are supported by the Australian Antarctic Division. RAM, FK, AMa, and PV are supported by the Australian Research Council Special Research Initiative Australian Centre for Excellence in Antarctic Science (Project Number SR200100008). IP is supported by the PoF IV program “Changing Earth – Sustaining our Future” Topic 6.3 of the of the German Helmholtz Association. RK acknowledges funding by the Heisenberg program of the German Science Foundation under Project Number 469175784.
Acknowledgments
We thank Stacey McCormack for Figure 1. Klaus Meiners provided valuable comments. Sea ice data were accessed courtesy of the US National Snow and Ice Data Centre. Additional incident light data were obtained from the International Satellite Cloud Climatology Project (ISCCP).
Conflict of interest
AC is a Topic Editor of this MEASO Special Issue.
The remaining authors declare that the research was conducted in the absence of any commercial or financial relationships that could be construed as a potential conflict of interest.
Publisher’s note
All claims expressed in this article are solely those of the authors and do not necessarily represent those of their affiliated organizations, or those of the publisher, the editors and the reviewers. Any product that may be evaluated in this article, or claim that may be made by its manufacturer, is not guaranteed or endorsed by the publisher.
References
Ackley, S. F., Lewis, M. J., Fritsen, C. H., and Xie, H. (2008). Internal melting in Antarctic Sea ice: development of “gap layers”. Geophys. Res. Lett. 35:L11503. doi: 10.1029/2008GL033644
Ainley, D. G., Ribic, C. A., Ballard, G., Heath, S., Gaffney, I., Karl, B. J., et al. (2004). Geographic structure of Adélie penguin populations: overlap in colony-specific foraging areas. Ecol. Monogr. 74, 159–178. doi: 10.1890/02–4073
Andriashev, A. P. (1987). “A general review of the Antarctic bottom fish fauna” in Proceedings of the Fifth Congress of European Ichthyologists, Stockholm, 1985. eds. S. O. Kullander and B. Fernholm (Stockholm, Sweden: Swedish Museum of Natural History), 357–372.
Aoki, S. (2017). Breakup of land-fast sea ice in Lützow-Holm Bay, East Antarctica, and its teleconnection to tropical Pacific Sea surface temperatures. Geophys. Res. Lett. 44, 3219–3227. doi: 10.1002/2017GL072835
Arndt, S., Hoppmann, M., Schmithüsen, H., Fraser, A. D., and Nicolaus, M. (2020). Seasonal and interannual variability of landfast sea ice in Atka Bay, Weddell Sea, Antarctica. Cryosphere 14, 2775–2793. doi: 10.5194/tc–14–2775–2020
Arndt, S., Meiners, K. M., Ricker, R., Krumpen, T., Katlein, C., and Nicolaus, M. (2017). Influence of snow depth and surface flooding on light transmission through Antarctic pack ice. J. Geophys. Res. Oceans 122, 2108–2119. doi: 10.1002/2016JC012325
Arndt, C. E., and Swadling, K. M. (2006). Crustacea in Arctic and Antarctic Sea ice: distribution, diet and life history strategies. Adv. Mar. Biol. 51, 197–315. doi: 10.1016/S0065–2881(06)51004–1
Arrigo, K. R. (2017). “Sea ice as a habitat for primary producers,” in Sea Ice. 3rd Edn. ed. D. N. Thomas (Oxford, UK: Wiley-Blackwell), 352–369.
Arrigo, K. R., Dieckmann, G., Gosselin, M., Robinson, D. H., Fritsen, C. H., and Sullivan, C. W. (1995). High resolution study of the platelet ice ecosystem in McMurdo Sound, Antarctica: biomass, nutrient, and production profiles within a dense microalgal bloom. Mar. Ecol. Prog. Ser. 127, 255–268. doi: 10.3354/meps127255
Arrigo, K. R., Mock, T., and Lizotte, M. P. (2010). “Primary producers and sea ice” in Sea Ice. eds. D. N. Thomas and G. S. Dieckmann. 2nd ed (Oxford, UK: Wiley-Blackwell), 283–326.
Atkinson, A., Hill, S. L., Pakhomov, E. A., Siegel, V., Anadon, R., Chiba, S., et al. (2017). KRILLBASE: a circumpolar database of Antarctic krill and salp numerical densities, 1926–2016. Earth Syst. Sci. Data 9, 193–210. doi: 10.5194/essd–9–193–2017
Atkinson, A., Hill, S. L., Pakhomov, E. A., Siegel, V., Reiss, C. S., Loeb, V. J., et al. (2019). Krill (Euphausia superba) distribution contracts southward during rapid regional warming. Nat. Clim. Chang. 9, 142–147. doi: 10.1038/s41558–018–0370–z
Atkinson, A., Siegel, V., Pakhomov, E., and Rothery, P. (2004). Long-term decline in krill stock and increase in salps within the Southern Ocean. Nature 432, 100–103. doi: 10.1038/nature02996
Barber, D. G., and Massom, R. A. (2007). “The role of sea ice in Arctic and Antarctic polynyas” in Polynyas. eds. W. Smith and D. G. Barber (Amsterdam: Windows to the World, Elsevier), 1–43.
Belcher, A., Henson, S. A., Manno, C., Hill, S. L., Atkinson, A., Thorpe, S. E., et al. (2019). Krill faecal pellets drive hidden pulses of particulate organic carbon in the marginal ice zone. Nat. Commun. 10:889. doi: 10.1038/s41467–019–08847–1
Bender, E. A., Case, T. J., and Gilpin, M. E. (1984). Perturbation experiments in community ecology: theory and practice. Ecology 65, 1–13. doi: 10.2307/1939452
Bestley, S., Ropert-Coudert, Y., Bengtson Nash, S., Brooks, C. M., Cotté, C., Dewar, M., et al. (2020). Marine ecosystem assessment for the Southern Ocean: birdsand marine mammals in a changing climate. Front. Ecol. Evol. 8:566936.. doi: 10.3389/fevo.2020.566936
Bluhm, B. A., Swadling, K. M., and Gradinger, R. (2017). “Sea ice as a habitat for macrograzers” in Sea Ice. 3rd Edn. ed. D. N. Thomas. (Oxford, UK: Wiley–Blackwell), 394–414.
Bone, Q., Carre, C., and Ryan, K. P. (2000). The endostyle and the feeding filter in salps (Tunicata). J. Mar. Biol. Ass. UK 80, 523–534. doi: 10.1017/S0025315400002228
Bone, Q., and Trueman, E. R. (1983). Jet propulsion in salps (Tunicata: Thaliacea). J. Zool. Lond. 201, 481–506. doi: 10.1111/j.1469–7998.1983.tb05071
Bottaro, M., Oliveri, D., Ghigliotti, L., Pisano, E., Ferrando, S., and Vacchi, M. (2009). Born among the ice: first morphological observations on two developmental stages of the Antarctic silverfish Pleuragramma antarcticum, a key species of the Southern Ocean. Reviews Fish. Biol. Fish. 19, 249–259. doi: 10.1007/s11160–009–9106–5
Boysen Ennen, E., Hagen, W., Hubold, G., and Piatkowski, U. (1991). Zooplankton biomass in the ice–covered Weddell Sea. Antarctica. Mar. Biol. 111, 227–235. doi: 10.1007/bf01319704
Bozkurt, D., Bromwich, D. H., Carrasco, J., and Rondanelli, R. (2021). Temperature and precipitation projections for the Antarctic peninsula over the next two decades: contrasting global and regional climate model simulations. Clim. Dyn. 56, 3853–3874. doi: 10.1007/s00382–021–05667–2
Burghart, S., Hopkins, T., Vargo, G. A., and Torres, J. J. (1999). Effects of a rapidly receding ice edge on the abundance, age structure and feeding of three dominant calanoid copepods in the Weddell Sea, Antarctica. Polar Biol. 22, 279–288. doi: 10.1007/s003000050421
Cabanes, D. J. E., Norman, L., Santos Echeandia, J., Iversen, M. H., Trimborn, S., Laglera, L. M., et al. (2017). First evaluation of the role of salp fecal pellets on iron biogeochemistry. Front. Mar. Sci. 3:289. doi: 10.3389/FMARS.2016.00289
Casaux, R. J., Mazzotta, A. S., and Barrera-Oro, E. R. (1990). Seasonal aspects of the biology and diet of nearshore nototheniid fish at potter cove, South Shetland Islands, Antarctica. Polar Biol. 11, 63–72. doi: 10.1007/BF00236523
Cavalieri, D. J., Parkinson, C. L., Gloersen, P., and Zwally, H. J. (1996). Sea Ice Concentrations from Nimbus-7 SMMR and DMSP SSM/I-SSMIS Passive Microwave Data, Version 1. Boulder, CO. NASA National Snow and Ice Data Center Distributed Active Archive Center.
Cavanagh, R. D., Melbourne-Thomas, J., Grant, S. M., Barnes, D. K. A., Hughes, K. A., Halfter, S., et al. (2021). Future risk for Southern Ocean ecosystem services under climate change. Front. Mar. Sci. 7:615214. doi: 10.3389/fmars.2020.615214
Clark, G. F., Stark, J. S., Palmer, A. S., Riddle, M. J., and Johnston, E. L. (2017). The roles of sea-ice, light and sedimentation in structuring shallow Antarctic benthic communities. PLoS One 12:e0168391. doi: 10.1371/journal.pone.0168391
Collins, M., Knutti, R., Arblaster, J., Dufresne, J.-L., Fichefet, T., Friedlingstein, P., et al. (2013). “Long-term climate change: projections, commitments and irreversibility” in Climate Change 2013: The Physical Science Basis. Contribution of Working Group I to the Fifth Assessment Report of the Intergovernmental Panel on Climate Change. eds. T. F. Stocker, D. Qin, G.-K. Plattner, M. Tignor, S. K. Allen, and J. Boschung, et al. (Cambridge, UK and New York, NY: Cambridge University Press)
Comiso, J. C., Kwok, R., Martin, S., and Gordon, A. L. (2011). Variability and trends in sea ice extent and ice production in the Ross Sea. J. Geophys. Res. 116:C04021. doi: 10.1029/2010JC006391
Constable, A. J., Melbourne Thomas, J., Corney, S. P., Arrigo, K., Barbraud, C., Barnes, D., et al. (2014). Climate change and Southern Ocean ecosystems I: how changes in physical habitats directly affect marine biota. Glob. Change Biol. 20, 3004–3025. doi: 10.1111/gcb.12623
Corso, A. D., Steinberg, D. K., Stammerjohn, S. E., and Hilton, E. J. (2022). Climate drives long-term change in Antarctic silverfish along the western Antarctic peninsula. Commun. Biol. 5:104. doi: 10.1038/s42003–022–03042–3
Costanzo, G., Zagami, G., Crescenti, N., and Granata, A. (2002). Naupliar development of Stephos longipes (Copepoda: Calanoida) from the annual sea ice of Terra Nova Bay, Antarctica. J. Crust. Biol. 22, 855–860. doi: 10.1163/20021975–99990297
Cummings, V. J., Hewitt, J. E., Thrush, S. F., Marriott, P. M., Halliday, N. J., and Norkko, A. M. (2018). Linking Ross Sea coastal benthic ecosystems to environmental conditions: documenting baselines in a changing world. Front. Mar. Sci. 5:232. doi: 10.3389/fmars.2018.00232
Cziko, P. A., Evans, C. W., Cheng, C. H. C., and DeVries, A. L. (2006). Freezing resistance of antifreeze-deficient larval Antarctic fish. J. Exp. Biol. 209, 407–420. doi: 10.1242/jeb.02008
Daly, K. (2004). Overwintering growth and development of larval Euphausia superba: an interannual comparison under varying environmental conditions west of the Antarctic Peninsula. Deep-Sea Res. Part II 51, 2139–2168. doi: 10.1016/j.dsr2.2004.07.010
Daly, M., Rack, F., and Zook, R. (2013). Edwardsiella andrillae, a new species of sea anemone from Antarctic ice. PLoS One 8:e83476. doi: 10.1371/journal.pone.0083476
Daly, K. L., and Zimmerman, J. J. (2004). Comparisons of morphology and neritic distributions of Euphausia crystallorophias and Euphausia superba furcilia during autumn and winter west of the Antarctic peninsula. Polar Biol. 28, 72–81. doi: 10.1007/s00300–004–0660–y
Dambacher, J. M., Li, H. W., and Rossignol, P. A. (2002). Relevance of community structure in assessing indeterminacy of ecological predictions. Ecology 83, 1372–1385. doi: 10.2307/3071950
Dambacher, J. M., Li, H. W., and Rossignol, P. A. (2003). Qualitative predictions in model ecosystems. Ecol. Model. 161, 79–93. doi: 10.1016/S0304–3800(02)00295–8
Dambacher, J. M., and Ramos-Jiliberto, R. (2007). Understanding and predicting effects of modified interactions through a qualitative analysis of community structure. Q. Rev. Biol. 82, 227–250. doi: 10.1016/S0304–3800(02)00295–8
David, C. L., Schaafsma, F. L., van Franeker, J. A., Pakhomov, E. A., Hunt, B. P. V., Lange, B. A., et al. (2021). Sea-ice habitat minimizes grazing impact and predation risk for larval Antarctic krill. Polar Biol. 44, 1175–1193. doi: 10.1007/s00300–021–02868–7
Davis, L. B., Hofmann, E. E., Klinck, J. M., Piñones, A., and Dinniman, M. S. (2017). Distributions of krill and Antarctic silverfish and correlations with environmental variables in the western Ross Sea. Antarctica. Mar. Ecol. Prog. Ser. 584, 45–65. doi: 10.3354/meps12347
De Broyer, C., Koubbi, P., Griffiths, H. J., Raymond, B., Udekem Acoz, C. D., Van de Putte, A. P., et al. (eds.) (2014). Biogeographic Atlas of the Southern Ocean. Scientific Committee on Antarctic Research, Cambridge, XII 498.
DeJong, H. B., Dunbar, R. B., and Lyons, E. A. (2018). Late summer frazil ice–associated algal blooms around Antarctica. Geophys. Res. Lett. 45, 826–833. doi: 10.1002/2017GL075472
Ducklow, H. W., Baker, K., Martinson, D. G., Quetin, L. B., Ross, R. M., Smith, R. C., et al. (2007). Marine pelagic ecosystems: the West Antarctic peninsula. Philos. Trans. R. Soc. Lond. Ser. B Biol. Sci. 362, 67–94. doi: 10.1098/rstb.2006.1955
Ducklow, H., Fraser, W. R., Meredith, M. P., Stammerjohn, S. E., Doney, S. C., Martinson, D. G., et al. (2013). West Antarctic peninsula: an ice–dependent coastal marine ecosystem in transition. Oceanography 26, 190–203. doi: 10.5670/oceanog.2013.62
Duhamel, G., Hulley, P. A., Causse, R., Koubbi, P., Vacchi, M., Pruvost, S., et al. (2014). Part 7. Biogeographic patterns of fish. In C. BroyerDe, P. Koubbi, H. J. Griffiths, B. Raymond, and C. D. Udekem Acoz, et al. Biogeographic atlas of the Southern Ocean. Scientific Committee on Antarctic Research, Cambridge, 327–362.
Eastman, J. T. (1993). Antarctic Fish Biology: Evolution in a Unique Environment. San Diego: Academic Press, xiii 322.
Eastman, J. T., and DeVries, A. L. (1985). Adaptations for cryopelagic life in the Antarctic notothenioid fish Pagothenia borchgrevinki. Polar Biol. 4, 45–52. doi: 10.1007/BF00286816
Eayrs, C., Holland, D. M., Francis, D., Wagner, T. J. W., Kumar, R., and Li, X. (2019). Understanding the seasonal cycle of Antarctic Sea ice extent in the context of longer-term variability. Rev. Geophys. 57, 1037–1064. doi: 10.1029/2018RG000631
Ewert, M., and Deming, J. W. (2013). Sea ice microorganisms: environmental constraints and extracellular responses. Biology 2, 603–628. doi: 10.3390/biology2020603
Flores, H., Atkinson, A., Kawaguchi, S., Krafft, B., Milinevsky, G., Nicol, S., et al. (2012). Impact of climate change on Antarctic krill. Mar. Ecol. Prog. Ser. 458, 1–19. doi: 10.3354/meps09831
Foldvik, A., and Kvinge, T. (1974). Conditional instability of sea water at the freezing point. Deep-Sea Res. Oceanogr. Abstr. 21, 169–174. doi: 10.1016/0011–7471(74)90056–4
Fons, S. W., and Kurtz, N. T. (2019). Retrieval of snow freeboard of Antarctic Sea ice using waveform fitting of CryoSat–2 returns. Cryosphere 13, 861–878. doi: 10.5194/tc–13–861–2019
Foxton, P. (1966). The distribution and life history of Salpa Thompsoni Foxton with observation on a related species, Salpa gerlachei Foxton. Discovery Rep. 21, 1–116.
Fraser, A. D. (2011). East Antarctic Landfast Sea-Ice Distribution and Variability. Ph D. thesis. Hobart, Tasmania: Institute for Marine and Antarctic Studies, University of Tasmania.
Fraser, A. D., Massom, R. A., Handcock, M. S., Reid, P., Ohshima, K. I., Raphael, M. N., et al. (2021). Eighteen-year record of circum-Antarctic landfast–sea-ice distribution allows detailed baseline characterisation and reveals trends and variability. Cryosphere 15, 5061–5077. doi: 10.5194/tc–15–5061–2021
Fraser, A. D., Massom, R. A., Michael, K. J., Galton-Fenzi, B. K., and Lieser, J. L. (2012). East Antarctic landfast sea ice distribution and variability. J. Clim. 25, 1137–1156. doi: 10.1175/JCLI–D–10–05032.1
Fraser, A. D., Massom, R. A., Ohshima, K. I., Willmes, S., Kappes, P. J., Cartwright, J., et al. (2020). High-resolution mapping of circum-Antarctic landfast sea ice distribution, 2000–2018. Earth Syst. Sci. Data Discuss. doi: 10.5194/essd–12–2987–2020
Frazer, T. K., Quetin, L. B., and Ross, R. M. (2002). Abundance, sizes and developmental stages of larval krill, Euphausia superba, during winter in ice-covered seas west of the Antarctic peninsula. J. Plankton Res. 24, 1067–1077. doi: 10.1093/plankt/24.10.1067
Fritsen, C. H., Lytle, V. I., Ackley, S. F., and Sullivan, C. W. (1994). Autumn bloom of Antarctic pack-ice algae. Science 266, 782–784. doi: 10.1126/science.266.5186.782
Garrison, D., Close, A., and Reimnitz, E. (1989). Algae concentrated by frazil ice: evidence from laboratory experiments and field measurements. Ant. Sci. 1, 313–316. doi: 10.1017/S0954102089000477
Ghigliotti, L., Herasymchuk, V. V., Kock, K. H., and Vacchi, M. (2017). “Reproductive strategies of the Antarctic silverfish: known knowns, known unknowns and unknown unknowns” in The Antarctic Silverfish: A Keystone Species in a Changing Ecosystem. eds. M. Vacchi, E. Pisano, and L. Ghigliotti, vol. 3 (Cham: Springer International Publishing), 173–192.
Giles, A. B., Massom, R. A., and Lytle, V. I. (2008). Fast-ice distribution in East Antarctica during 1997 and 1999 determined using RADARSAT data. J. Geophys. Res. 113:C02S14. doi: 10.1029/2007JC004139
Golden, K. M., Ackley, S. F., and Lytle, V. I. (1998). The percolation phase transition in sea ice. Science 18, 2238–2241. doi: 10.1126/science.282.5397.2238
Gon, O., and Heemstra, P. C. (1990). Fishes of the Southern Ocean. Grahamstown: J. L. B. Smith Institute of Ichthyology.
Grant, S. M., Hill, S. L., Trathan, P. N., and Murphy, E. J. (2013). Ecosystem services of the Southern Ocean: trade-offs in decision-making. Antarct. Sci. 25, 603–617. doi: 10.1017/S0954102013000308
Grant, S. M., Waller, C. L., Morley, S. A., Barnes, D. K. A., Brasier, M. J., Double, M. C., et al. (2021). Local drivers of change in Southern Ocean ecosystems: human activities and policy implications. Front. Ecol. Evol. 9:365. doi: 10.3389/fevo.2021.624518
Griffiths, H., Meijers, A., and Bracegirdle, T. (2017). More losers than winners in a century of future Southern Ocean seafloor warming. Nature Clim. Change 7, 749–754. doi: 10.1038/nclimate3377
Guglielmo, L., Donato, P., Zagami, G., and Granata, A. (2009). Spatio-temporal distribution and abundance of Euphausia crystallorophias in Terra Nova Bay (Ross Sea, Antarctica) during austral summer. Polar Biol. 32, 347–367. doi: 10.1007/s00300–008–0546–5
Guglielmo, L., Granata, A., and Greco, S. (1998). Distribution and abundance of postlarval and juvenile Pleuragramma antarcticum (Pisces, Nototheniidae) off Terra Nova Bay (Ross Sea, Antarctica). Polar Biol. 19, 37–51. doi: 10.1007/s003000050214
Guglielmo, L., Zagami, G., Saggiomo, V., Catalano, G., and Granata, A. (2007). Copepods in spring annual sea ice at Terra Nova Bay (Ross Sea, Antarctica). Polar Biol. 30, 747–758. doi: 10.1007/s00300–006–0234–2
Guidetti, P., Ghigliotti, L., and Vacchi, M. (2015). Insights into spatial distribution patterns of early stages of the Antarctic silverfish, Pleuragramma antarctica, in the platelet ice of Terra Nova Bay, Antarctica. Polar Biol. 38, 333–342. doi: 10.1007/s00300–014–1589–4
Günther, S., and Dieckmann, G. S. (1999). Seasonal development of algal biomass in snow-covered fast ice and the underlying platelet layer in the Weddell Sea, Antarctica. Antarct. Sci. 11, 305–315. doi: 10.1017/S0954102099000395
Günther, S., George, K. H., and Gleitz, M. (1999). High sympagic metazoan abundance in platelet layers at Drescher inlet, Weddell Sea, Antarctica. Polar Biol. 22, 82–89. doi: 10.1007/s003000050393
Gutt, J. (2002). The Antarctic ice shelf: an extreme habitat for notothenioid fish. Polar Biol. 25, 320–322. doi: 10.1007/s00300–001–0352–9
Hamner, W. M., Hamner, P. P., Obst, B. S., and Carleton, J. H. (1989). Field observations on the ontogeny of schooling of Euphausia superba furciliae and its relationship to ice in Antarctic waters. Limnol. Oceanogr. 34. doi: 10.4319/lo.1989.34.2.0451
Harrington, S. A., and Thomas, P. G. (1987). Observations on spawning by Euphausia crystallorophias form waters adjacent to Enderby Land (East Antarctica) and speculation on the early ontogenetic ecology of neritic euphausiids. Polar Biol. 7, 93–95. doi: 10.1007/BF00570446
Hecq, J. H., Guglielmo, L., Goffart, A., Catalano, G., and Goosse, H. (2000). “A modelling approach to the Ross Sea plankton ecosystem” in Ross Sea Ecology. eds. F. M. Faranda, L. Guglielmo, and A. Ianora (Berlin, Heidelberg: Springer), 395–411.
Henley, S. F., Cavan, E. L., Fawcett, S. E., Kerr, R., Monteiro, T., Sherrell, R. M., et al. (2020). Changing biogeochemistry of the Southern Ocean and its ecosystem implications. Front. Mar. Sci. 7:581. doi: 10.3389/fmars.2020.00581
Hobbs, W., Massom, R., Stammerjohn, S., Reid, P., Williams, G., and Meier, W. (2016). A review of recent changes in Southern Ocean sea ice, their drivers and forcings. Glob. Planet. Change 143, 228–250. doi: 10.1016/j.gloplacha.2016.06.008
Holland, P. R. (2014). The seasonality of Antarctic Sea ice trends. Geophys. Res.Lett. 41, 4230–4237. doi: 10.1002/2014GL060172
Hoppmann, M., Richter, M., Smith, I., Jendersie, S., Langhorne, P., Thomas, D., et al. (2020). Platelet ice, the Southern Ocean's hidden ice: a review. Ann. Glaciol. 61, 341–368. doi: 10.1017/aog.2020.54
Hoshiai, T., Tanimura, A., Fukuchi, M., and Watanabe, K. (1989). Feeding by the nototheniid fish, Pagothenia borchgrevinki on the ice–associated copepod, Paralabidocera Antarctica. Proc. NIPR Symp. Polar Biol. 2, 61–64.
Hunt, B. P. V., Pakhomov, E. A., Teschke, M., King, R., Cantzler, H., Halbach, L., et al. (2014). Multi–net 24 hour stations. Rep. Polar Mar. Res. (Berichte zur Polar- und Meeresforschung). 674, 43–48.
IPCC (2019). IPCC Special Report on the Ocean and Cryosphere in a Changing Climate. eds. H.-O. Pörtner, D. C. Roberts, V. Masson-Delmotte, P. Zhai, M. Tignor, E. Poloczanska et al. (NY, USA: Cambridge University Press, Cambridge, UK and New York), 77.
IPCC (2021). Climate change 2021: the physical science basis. Contribution of working group 1 to the sixth assessment report of the intergovernmental panel on climate change. eds. M. Delmotte, V. P. Zhai, A. Pirani, S. L. Connors, C. Péan, and S. Berger, et al. (Cambridge, UK and New York, NY: Cambridge University Press).
Iversen, M. H., Pakhomov, E. A., Hunt, B. P. V., van der Jagt, H., Wolf-Gladrow, D., and Klaas, C. (2017). Sinkers or floaters? Contribution from salp pellets to the export flux during a large bloom event in the Southern Ocean. Deep-Sea Res. II 138, 116–125. doi: 10.1016/j.dsr2.2016.12.004
Janssen, A. R., Badke, R., Bransome, N. C., Bricher, P., Cavanagh, R., and de Bruin, T. (2021). Report: 1st Southern Ocean Regional Workshop, United Nations Decade of Ocean Science for Sustainable Development. Available at: usercontent.one/wp/www.sodecade.org/wp-content/uploads/2021/07/UN-Ocean-Decade-Southern-Ocean-workshop-report-May20_1589187161.pdf
Jia, Z., Swadling, K. M., Meiners, K. M., Kawaguchi, S., and Virtue, P. (2016). The zooplankton food web under East Antarctic pack ice – a stable isotope study. Deep-Sea Res. II 131, 189–202. doi: 10.1016/j.dsr2.2015.10.010
Johnston, N. M., Murphy, E. J., Atkinson, A., Constable, A. J., Cotté, C., Cox, M., et al. (2022). Status, change, and futures of zooplankton in the Southern Ocean. Front. Ecol. Evol. 9:624692. doi: 10.3389/fevo.2021.624692
Kacimi, S., and Kwok, R. (2020). The Antarctic Sea ice cover from ICESat-2 and CryoSat-2: freeboard, snow depth and ice thickness. Cryosphere Discuss. 14, 4453–4474. doi: 10.5194/tc–14–4453–2020
Kattner, G., Thomas, D. N., Haas, C., Kennedy, H., and Dieckmann, G. (2004). Surface ice and gap layers in Antarctic Sea ice: highly productive habitats. Mar. Ecol. Prog. Ser. 277, 1–12. doi: 10.3354/meps277001
Kauko, H. M., Hattermann, T., Ryan-Keogh, T., Singh, A., de Steur, L., Fransson, A., et al. (2021). Phenology and environmental control of phytoplankton blooms in the Kong Håkon VII Hav in the Southern Ocean. Front. Mar. Sci. 8:623856. doi: 10.3389/fmars.2021.623856
Kiko, R. (2010). Acquisition of freeze protection in a sea-ice crustacean through horizontal gene transfer? Polar Biol. 33, 543–556. doi: 10.1007/s00300–009–0732–0
Kiko, R., Kramer, M., Spindler, M., and Wägele, H. (2008b). Tergipes antarcticus (Gastropoda, Nudibranchia): distribution, life cycle, morphology, anatomy and adaptation of the first mollusc known to live in Antarctic Sea ice. Polar Biol. 31, 1383–1395. doi: 10.1007/s00300–008–0478–0
Kiko, R., Michels, J., Mizdalski, E., Schnack Schiel, S. B., and Werner, I. (2008a). Living conditions, abundance and composition of the metazoan fauna in surface and sub-ice layers in pack ice of the western Weddell Sea during late spring. Deep-Sea Res. II 55, 1000–1014. doi: 10.1016/j.dsr2.2007.12.012
Kohlbach, D., Graeve, M., Lange, B. A., David, C., Schaafsma, F. L., van Franeker, J. A., et al. (2018). Dependency of Antarctic zooplankton species on ice algae-produced carbon suggests a sea ice-driven pelagic ecosystem during winter. Glob. Chang. Biol. 24, 4667–4681. doi: 10.1111/gcb.14392
Kramer, M., Swadling, K. M., Meiners, K. M., Kiko, R., Scheltz, A., Nicolaus, M., et al. (2011). Antarctic sympagic meiofauna in winter: comparing diversity, abundance and biomass between perennially and seasonally ice-covered regions. Deep Sea Res. II 58, 1062–1074. doi: 10.1016/j.dsr2.2010.10.029
Krapp, R. H., Berge, J., Flores, H., Gulliksen, B., and Werner, I. (2008). Sympagic occurrence of Eusirid and Lysianassoid amphipods under Antarctic pack ice. Deep Sea Res. II 55, 1015–1023. doi: 10.1016/j.dsr2.2007.12.018
Kurbjeweit, F., Gradinger, R., and Weissenberger, J. (1993). The life cycle of Stephos longipes – an example for cryopelagic coupling in the Weddell Sea (Antarctica). Mar. Ecol. Prog. Ser. 98, 255–262. doi: 10.3354/meps098255
La, H. S., Lee, H., Fielding, S., Kang, D., Ha, H. K., Atkinson, A., et al. (2015). High density of ice krill (Euphausia crystallorophias) in the Amundsen Sea coastal polynya, Antarctica. Deep Sea Res. I 95, 75–84. doi: 10.1016/j.dsr.2014.09.002
La Mesa, M., Riginella, E., Mazzoldi, C., and Ashford, J. (2015). Reproductive resilience of ice-dependent Antarctic silverfish in a rapidly changing system along the western Antarctic peninsula. Mar. Ecol. 36, 2235–2245. doi: 10.1111/maec.12140
La Mesa, M., Vacchi, M., and Zunini Sertorio, T. (2000). Feeding plasticity of Trematomus newnesi (Pisces, Nototheniidae) in Terra Nova Bay, Ross Sea, in relation to environmental conditions. Polar Biol. 23, 38–45. doi: 10.1007/s003000050006
Labrousse, S., Fraser, A. D., Sumner, M., Tamura, T., Pinaud, D., Wienecke, B., et al. (2019). Dynamic fine-scale sea icescape shapes adult emperor penguin foraging habitat in East Antarctica. Geophys. Res. Lett. 46, 11206–11218. doi: 10.1029/2019GL084347
Lancelot, C., Mathot, S., Veth, C., and de Baar, H. (1993). Factors controlling phytoplankton ice-edge blooms in the marginal ice–zone of the northwestern Weddell Sea during sea ice retreat 1988: field observations and mathematical modelling. Polar Biol. 13, 377–387. doi: 10.1007/BF01681979
Langhorne, P. J., Hughes, K. G., Gough, A. J., Smith, I. J., Williams, M. J. M., Robinson, N., et al. (2015). Observed platelet ice distributions in Antarctic Sea ice: an index for ocean-ice shelf heat flux. Geophys. Res. Lett. 42, 5442–5451. doi: 10.1002/2015GL064508
Lannuzel, D., Vancoppenolle, M., Van der Merwe, P., De Jong, J., Meiners, K. M., Grotti, M., et al. (2016). Iron in sea ice: review and new insights. Elem. Sci. Anth. 4:000130. doi: 10.12952/journal.elementa.000130
Legendre, L., Ackley, S. F., Dieckmann, G. S., Gulliksen, B., Horner, R., Hoshiai, T., et al. (1992). Ecology of sea ice biota. Polar Biol. 12, 429–444. doi: 10.1007/BF00243114
Levins, R. (1974). Discussion paper: the qualitative analysis of partially specified systems. Ann. N. Y. Acad. Sci. 231, 123–138. doi: 10.1111/j.1749–6632.1974.tb20562.x
Loeb, V., and Santora, J. L. (2015). Climate variability and spatiotemporal dynamics of five Southern Ocean krill species. Prog. Oceanogr. 134, 93–122. doi: 10.1016/j.pocean.2015.01.002
Loeb, B. A., and Siegel, H. M. (1995). Recruitment of Antarctic krill Euphausia superba and possible causes for its variability. Mar. Ecol. Prog. Ser. 123, 45–56. doi: 10.3354/meps123045
Loeb, V., Siegel, V., Holm Hansen, O., Hewitt, R., Fraser, W., Trivelpiece, W., et al. (1997). Effects of sea-ice extent and krill or salp dominance on the Antarctic food web. Nature 387, 897–900. doi: 10.1038/43174
Loots, C., Swadling, K. M., and Koubbi, P. (2009). Annual cycle of distribution of three ice-associated copepods along the coast near Dumont d’Urville, Terre Adélie (Antarctica). J. Mar. Syst. 78, 599–605. doi: 10.1016/j.jmarsys.2009.01.003
Makabe, R., Hasegawa, T., Sano, M., Kashiwase, H., and Moteki, M. (2022). Copepod assemblages in the water column and drifting sea-ice floes in the ice-edge region in the Indian Ocean sector of the Southern Ocean during the austral summer. Polar Biol. 45, 749–762. doi: 10.1007/s00300-022-03030-7
Mangoni, O., Saggiomo, V., Bolinesi, F., Margiotta, F., Budillon, G., Cotroneo, Y., et al. (2017). Phytoplankton blooms during austral summer in the Ross Sea, Antarctica: driving factors and trophic implications. PLoS One 12:e0176033. doi: 10.1371/journal.pone.0176033
Massom, R. A., Eicken, H., Haas, C., Jeffries, M. O., Drinkwater, M. R., Sturm, M., et al. (2001). Snow on Antarctic Sea ice. Rev. Geophys. 39, 413–445. doi: 10.1029/2000RG000085
Massom, R. A., Hill, K., Barbraud, C., Adams, N., Ancel, A., Emmerson, L., et al. (2009). Fast ice distribution in Adélie land, East Antarctica: interannual variability and implications for emperor penguins Aptenodytes forsteri. Mar. Ecol. Prog. Ser. 374, 243–257. doi: 10.3354/meps07734
Massom, R., Reid, P., Stammerjohn, S., Raymond, B., Fraser, A., and Ushio, S. (2013). Change and variability in East Antarctic Sea ice seasonality, 1979/80–2009/10. PLoS One 8:e64756. doi: 10.1371/journal.pone.0064756
Massom, R. A., and Stammerjohn, S. E. (2010). Antarctic Sea ice change and variability – physical and ecological implications. Pol. Sci. 4, 149–186. doi: 10.1016/j.polar.2010.05.001
Massom, R. A., Stammerjohn, S. E., Smith, R. C., Pook, M. J., Iannuzzi, R. A., Adams, N., et al. (2006). Extreme anomalous atmospheric circulation in the West Antarctic Peninsula region in austral spring and summer 2001/2, and its profound impact on sea ice and biota. J. Clim. 19, 3544–3571. doi: 10.1175/JCLI3805.1
May, R. M. (1973). Qualitative stability in model ecosystems. Ecology 54, 638–641. doi: 10.2307/1935352
McCormack, S. A., Melbourne-Thomas, J., Trebilco, R., Griffith, G., Hill, S. L., Hoover, C., et al. (2021). Southern Ocean food web modelling: progress, prognoses, and future priorities for research and policy makers. Front. Ecol. Evol. 9:626. doi: 10.3389/fevo.2021.624763
McMinn, A., Pankowskii, A., Ashworth, C., Bhagooli, R., Ralph, P., and Ryan, K. (2010). In situ net primary productivity and photosynthesis of Antarctic Sea ice algal, phytoplankton and benthic algal communities. Mar. Biol. 157, 1345–1356. doi: 10.1007/s00227–010–1414–8
Medley, B., and Thomas, E. R. (2019). Increased snowfall over the Antarctic ice sheet mitigated twentieth-century sea-level rise. Nat. Clim. Chang. 9, 34–39. doi: 10.1038/s41558–018–0356–x
Meehl, G. A., Arblaster, J. M., Chung, C. T. Y., Holland, M. M., DuVivier, A., Thompson, L., et al. (2019). Sustained Ocean changes contributed to sudden Antarctic Sea ice retreat in late 2016. Nat. Commun. 10:14. doi: 10.1038/s41467–018–07865–9
Meiners, K. M., Vancoppenolle, M., Carnat, G., Castellani, G., Delille, B., Delille, D., et al. (2018). Chlorophyll-a in Antarctic landfast sea ice: a first synthesis of historical ice core data. J. Geophys. Res. Oceans 123, 8444–8459. doi: 10.1029/2018JC014245
Meiners, K. M., Vancoppenolle, M., Thanassekos, S., Dieckmann, G. S., Thomas, D. N., Tison, J. L., et al. (2012). Chlorophyll a in Antarctic sea ice from historical ice core data. Geophys. Res. Lett. 39:L21602. doi: 10.1029/2012GL053478
Melbourne-Thomas, J., Constable, A., Wotherspoon, S., and Raymond, B. (2013). Testing paradigms of ecosystem change under climate warming in Antarctica. PLoS One 8:e55093. doi: 10.1371/journal.pone.0055093
Melbourne-Thomas, J., Corney, S. P., Trebilco, R., Meiners, K. M., Stevens, R. P., Kawaguchi, S., et al. (2016). Under ice habitats for Antarctic krill larvae: could less mean more under climate warming? Geophys. Res. Lett. 43, 10,322–10, 327. doi: 10.1002/2016GL070846
Melbourne-Thomas, J., Wotherspoon, S., Raymond, B., and Constable, A. (2012). Comprehensive evaluation of model uncertainty in qualitative network analyses. Ecol. Monogr. 82, 505–519. doi: 10.1890/12-0207.1
Menshenina, L. L., and Melnikov, I. A. (1995). Under–ice zooplankton of the western Weddell Sea. Proc. NIPR Symp. Polar Biol. 8, 126–138.
Meredith, M., Sommerkorn, M., Cassotta, S., Derksen, C., Ekaykin, A., Hollowed, A., et al. (2019). “Polar Regions” in IPCC Special Report on the Ocean and Cryosphere in a Changing Climate. eds. H. O. Pörtner, D. C. Roberts, V. Masson Delmotte, P. Zhai, M. Tignor, and E. Poloczanska, et al. (Cambridge, UK and New York, NY: Cambridge University Press), 203–320.
Metcalf, S. J., Pember, M. B., and Bellchambers, L. M. (2011). Identifying indicators of the effects of fishing using alternative models, uncertainty, and aggregation error. ICES J. Mar. Sci. 68, 1417–1425. doi: 10.1093/icesjms/fsr050
Metfies, K., Nicolaus, A., Von Harbou, L., Bathmann, U., and Peeken, I. (2014). Molecular analyses of gut contents: elucidating the feeding of co-occurring salps in the Lazarev Sea from a different perspective. Ant. Sci. 26, 545–553. doi: 10.1017/S0954102014000157
Meyer, B. (2012). The overwintering of Antarctic krill, Euphausia superba, from an ecophysiological perspective. Polar Biol. 35, 15–37. doi: 10.1007/s00300–011–1120–0
Meyer, B., Atkinson, A., Bernard, K., Brierley, A. S., Driscoll, R. M., Hill, S. L., et al. (2020). Successful ecosystem-based management of Antarctic krill should address uncertainties in krill recruitment, behaviour and ecological adaptation. Commun. Earth Environ. 1:28. doi: 10.1038/s43247-020-00026-1
Meyer, B., Freier, U., Grimm, V., Groeneveld, J., Hunt, B. P., Kerwath, S., et al. (2017). The winter pack-ice zone provides a sheltered but food-poor habitat for larval Antarctic krill. Nat. Ecol. Evol. 1, 1853–1861. doi: 10.1038/s41559–017–0368–3
Moline, M. A., Karnovsky, N. J., Brown, Z., Divoky, G. J., Frazer, T. K., Jacoby, C. A., et al. (2008). High latitude changes in ice dynamics and their impact on polar marine ecosystems. Ann. N. Y. Acad. Sci. 1134, 267–319. doi: 10.1196/annals.1439.010
Morley, S. A., Abele, D., Barnes, D. K. A., Cárdenas, C. A., Cotté, C., Gutt, J., et al. (2020). Global drivers on Southern Ocean ecosystems: changing physical environments and anthropogenic pressures in an earth system. Front. Mar. Sci. 7:547188. doi: 10.3389/fmars.2020.547188
Murphy, E., Johnston, N., Corney, S., and Reid, K. (2018). Integrating climate and ecosystem dynamics in the Southern Ocean (ICED) Programme: Report of the ICED-CCAMLR Projections Workshop, 5-7 Apr 2018. SC-CAMLRXXXVII/ BG/16,” in SC-CAMLR-XXXVII. (Hobart, TAS: CCAMLR).
Murphy, E. J., Johnston, N. M., Hofmann, E. E., Phillips, R. A., Jackson, J., Constable, A. J., et al. (2021). Global connectivity of Southern Ocean ecosystems. Front. Ecol. Evol. 9:624451. doi: 10.3389/fevo.2021.624451
Newman, L., Hancock, A. M., Hofmann, E., Williams, M. J. M., Henley, S. F., Moreau, S., et al. (2022). The Southern Ocean observing system 2021–2025 science and implementation plan. Zenodo doi: 10.5281/zenodo.6324359
Nicol, S., Pauly, T., Bindoff, N. L., Wright, S., Thiele, D., Hosie, G. W., et al. (2000). Ocean circulation off East Antarctica affects ecosystem structure and sea-ice extent. Nature 406, 504–507. doi: 10.1038/35020053
Nihashi, S., and Ohshima, K. I. (2015). Circumpolar mapping of Antarctic coastal polynyas and landfast sea ice: relationship and variability. J. Clim. 28, 3650–3670. doi: 10.1175/JCLI–D–14–00369.1
O’Brien, C., Virtue, P., Kawaguchi, S., and Nichols, P. D. (2011). Aspects of krill growth and condition during late winter-early spring off East Antarctica (110–130°E). Deep–Sea Res Part II 58, 1211–1221. doi: 10.1016/j.dsr2.2010.11.001
Pakhomov, E. A., Dubischar, C. D., Hunt, B. P. V., Strass, V., Cisewski, B., Siegel, V., et al. (2011). Biology and life cycles of pelagic tunicates in the Lazarev Sea, Southern Ocean. Deep Sea Res. II. 58, 1677–1689. doi: 10.1016/j.dsr2.2010.11.014
Pakhomov, E. A., Froneman, P. W., and Perissinotto, R. (2002). Salp/krill interactions in the Southern Ocean: spatial segregation and implications for the carbon flux. Deep Sea Res. 49, 1881–1907. doi: 10.1016/S0967–0645(02)00017–6
Pakhomov, E. A., Pakhomova, L. G., Iversen, M. H., Flintrop, C. M., Pauli, N.-C., and Konrad, C. (2018). Biology of the pelagic tunicate, Salpa thompsoni, in the western Atlantic sector of the Southern Ocean during March–May 2018. Rep. Polar Marine Res. (Berichte zur Polar– und Meeresforschung) 722, 28–33.
Pakhomov, E. A., and Perissinotto, R. (1996). Antarctic neritic krill Euphausia crystallorophias: Spatio–temporal distribution, growth and grazing rates. Deep Sea Res. I 43, 59–87. doi: 10.1016/0967-0637(95)00094-1
Papot, C., Cascella, K., Toullec, J.-Y., and Jollivet, D. (2016). Divergent ecological histories of two sister Antarctic krill species led to contrasted patterns of genetic diversity in their heat–shock protein (hsp70) arsenal. Ecol. Evol. 6, 1555–1575. doi: 10.1002/ece3.1989
Parkinson, C. (2019). A 40–y record reveals gradual Antarctic Sea ice increases followed by decreases at rates far exceeding the rates seen in the Arctic. Proc. Natl. Acad. Sci. U. S. A. 166, 14414–14423. doi: 10.1073/pnas.1906556116
Paul, S., Hendricks, S., Ricker, R., Kern, S., and Rinne, E. (2018). Empirical parametrization of Envisat freeboard retrieval of Arctic and Antarctic sea ice based on CryoSat-2: progress in the ESA Climate Change Initiative. The Cryosphere. 12, 2437–2460. doi: 10.5194/tc-12-2437-2018
Pauli, N.-C., Flintrop, C. M., Konrad, C., Pakhomov, E. A., Swoboda, S., Koch, F., et al. (2021b). Krill and salp faecal pellets contribute equally to the carbon flux at the Antarctic peninsula. Nature Comm. 12:7168. doi: 10.1038/s41467–021–27436–9
Pauli, N.-C., Metfies, K., Pakhomov, E. A., Neuhaus, S., Graeve, M., Wenta, P., et al. (2021a). Selective feeding in Southern Ocean key grazers-diet composition of krill and salps. Commun. Biol. 4:1061. doi: 10.1038/s42003-021-02581-5
Perissinotto, R., and Pakhomov, E. A. (1998). The trophic role of the tunicate Salpa thompsoni in the Antarctic marine ecosystem. J. Mar. Syst. 17, 361–374. doi: 10.1016/S0924–7963(98)00049–9
Petrich, C., and Eicken, H. (2017). “Overview of sea ice growth and properties,” in Sea Ice. 3rd Edn. ed. D. N. Thomas (Oxford, UK: Wiley-Blackwell).
Pillai, H., Anilkumar, N., Achuthankutty, C., Mendes, C., Sabu, P., Jayalakshmi, K., et al. (2018). Planktonic food web structure at SSTF and PF in the Indian sector of the Southern Ocean during austral summer 2011. Polar Res. 37:1495545. doi: 10.1080/17518369.2018.1495545
Pinkerton, M. H., Boyd, P. W., Deppeler, S., Hayward, A., Höfer, J., and Moreau, S. (2021). Evidence for the impact of climate change on primary producers in the Southern Ocean. Front. Ecol. Evol. 9:592027. doi: 10.3389/fevo.2021.592027
Piñones, A., and Fedorov, A. V. (2016). Projected changes of Antarctic krill habitat by the end of the 21st century. Geophys. Res. Lett. 43, 8580–8589. doi: 10.1002/2016GL069656
Puccia, C. J., and Levins, R. (1985). Qualitative Modeling of Complex Systems: An Introduction to Loop Analysis and Time Averaging. Cambridge: Harvard University Press.
Pusceddu, A., Dell'Anno, A., and Fabiano, M. (2000). Organic matter composition in coastal sediments at Terra Nova Bay (Ross Sea) during summer 1995. Polar Biol. 23, 288–293. doi: 10.1007/s003000050446
Quetin, L. B., Ross, R. M., Fritsen, C. H., and Vernet, M. (2007). Ecological responses of Antarctic krill to environmental variability: can we predict the future? Ant. Sci. 19, 253–266. doi: 10.1017/S0954102007000363
Quetin, L. B., and Ross, R. M. (2009). “Life under antarctic pack ice: a krill perspective,” in Smithsonian at the Poles: Contributions to International Polar Year Science. Smithsonian Institution Scholarly Press, 285–298.
Rakusa Suszczewski, S. (1972). The biology of Paramoera walkeri Stebbing (Amphipoda) and the Antarctic sub–fast ice community. Pol. Arch. Hydrobiol. 19, 11–36.
Raymond, B., McInnes, J., Dambacher, J. M., Way, S., and Bergstrom, D. M. (2011). Qualitative modelling of invasive species eradication on subantarctic Macquarie Island. J. Appl. Ecol. 48, 181–191. doi: 10.1111/j.1365–2664.2010.01916.x
Reid, P., and Massom, R. A. (2015). Successive Antarctic Sea ice extent records during 2012, 2013, and 2014 [in 'State of the climate in 2014′]. Bull. Amer. Meteor. Soc. 96, S163–S164.
Reid, P., Stammerjohn, S., Massom, R. A., Barreira, S., Scambos, T., and Lieser, J. L. (2022). (Antarctica) sea ice extent, concentration, and seasonality [in "State of the Climate in 2021"]. Bull. Amer. Meteor. Soc. 102, S336–S338. doi: 10.1175/BAMS-D-21-0081.1
Richardson, M. G., and Whitaker, T. M. (1979). An Antarctic fast–ice food chain: observations on the interaction of the amphipod Pontogeneia antarctica Chevreux with ice–associated micro–algae. Br. Antarct. Surv. Bull. 47, 107–115.
Rintoul, S. R., Chown, S. L., DeConto, R. M., England, M. H., Fricker, H. A., Masson-Delmotte, V., et al. (2018). Choosing the future of Antarctica. Nature 558, 233–241. doi: 10.1038/s41586–018–0173–4
Ross, R. M., and Quetin, L. B. (1991). Ecological physiology of larval euphausiids, Euphausia superba (Euphausiacea). Mem. Queensland Mus. 31, 321–333.
Ross, R. M., Quetin, L. B., Newberger, T., Shaw, T. C., Jones, J. L., Oakes, S. A., et al. (2014). Trends, cycles, interannual variability for three pelagic species west of the Antarctic peninsula 1993–2008. Mar. Ecol. Prog. Ser. 515, 11–32. doi: 10.3354/meps10965
Ryan, K., Hegseth, E., Martin, A., Davy, S., O'Toole, R., Ralph, P., et al. (2006). Comparison of the microalgal community within fast ice at two sites along the Ross Sea coast. Antarctica. Ant. Sci. 18, 583–594. doi: 10.1017/S0954102006000629
Saba, G. K., Fraser, W. R., Saba, V. S., Iannuzzi, R. A., Coleman, K. E., Doney, S. C., et al. (2014). Winter and spring controls on the summer food web of the coastal West Antarctic peninsula. Nat. Commun. 5:4318. doi: 10.1038/ncomms5318
Sabu, P., Anilkumar, N., George, J. V., Chacko, R., Tripathy, S. C., and Achuthankutty, C. T. (2014). The influence of air-sea-ice interactions on an anomalous phytoplankton bloom in the Indian Ocean sector of the Antarctic zone of the Southern Ocean during the austral summer, 2011. Pol. Sci. 8, 370–384. doi: 10.1016/j.polar.2014.08.001
Saenz, B. T., and Arrigo, K. R. (2014). Annual primary production in Antarctic Sea ice during 2005–2006 from a sea ice state estimate. J. Geophys. Res. Oceans 119, 3645–3678. doi: 10.1002/2013JC009677+
Schmidt, K., Atkinson, A., McClelland, J. W., Montoya, J., Stübing, D., and Voss, M. (2003). Trophic relationships among Southern Ocean copepods and krill: some uses and limitations of a stable isotope approach. Limnol. Oceanogr. 48, 277–289. doi: 10.4319/lo.2003.48.1.0277
Schmidt, K., Atkinson, A., Pond, D. W., and Ireland, L. C. (2014). Feeding and overwintering of Antarctic krill across its major habitats: the role of sea ice cover, water depth, and phytoplankton abundance. Limnol. Oceanogr. 59, 17–36. doi: 10.4319/lo.2014.59.1.001717
Schmidt, K., Brown, T. A., Belt, S. T., Ireland, L. C., Taylor, K. W., Thorpe, S. E., et al. (2018). Do pelagic grazers benefit from sea ice? Insights from the Antarctic Sea ice proxy IPSO25. Biogeosciences 15, 1987–2006. doi: 10.5194/bg–15–1987–2018
Schnack Schiel, S. B., Thomas, D. N., Dahms, H. U., Haas, C., and Mizdalski, E. (1998). Copepods in Antarctic Sea ice. Antarct. Res. Ser. 73, 173–182. doi: 10.1029/AR073p0173
Schnack Schiel, S. B., Thomas, D. N., Dieckmann, G. S., Eicken, H., Gradinger, R., Spindler, M., et al. (1995). Life cycle strategy of the Antarctic calanoid copepod Stephos longipes. Prog. Oceanogr. 36, 45–75. doi: 10.1016/0079–6611(95)00014–3
Schofield, O., Saba, G., Coleman, K., Carvalho, F., Couto, N., Ducklow, H., et al. (2017). Decadal variability in coastal phytoplankton community composition in a changing West Antarctic peninsula. Deep–Sea Res. Part I 124, 42–54. doi: 10.1016/j.dsr.2017.04.014
Scott, P., McMinn, A., and Hosie, G. (1994). Physical parameters influencing diatom community structure in eastern Antarctic Sea ice. Polar Biol. 14, 507–517. doi: 10.1007/BF00238220
Seibel, B. A., and Dierssen, H. M. (2003). Cascading trophic impacts of reduced biomass in the Ross Sea Antarctica: just the tip of the iceberg? Biol. Bull. 205, 93–97. doi: 10.2307/1543229
Siegert, M., Atkinson, A., Banwell, A., Brandon, M., Convey, P., and Davies, B. (2019). The Antarctic peninsula under a 1.5 °C global warming scenario. Front. Environ. Sci. 7:102. doi: 10.3389/fenvs.2019.00102
Smith, W. O. Jr., Ainley, D. G., Arrigo, K. R., and Dinniman, M. S. (2014). The oceanography and ecology of the Ross Sea. Annu. Rev. Mar. Sci. 6, 469–487. doi: 10.1146/annurev-marine-010213-135114
Smith, W. O. Jr., Ainley, D. G., and Cattaneo Vietti, R. (2007). Trophic interactions within the Ross Sea continental shelf ecosystem. Phil. Trans. R. Soc. B 362, 95–11. doi: 10.1098/rstb.2006.1956
Smith, W. O. Jr., Dinniman, M. S., Hofmann, E. E., and Klinck, J. M. (2014). The effects of changing winds and temperatures on the oceanography of the Ross Sea in the 21st century. Geophys. Res. Lett. 41, 1624–1631. doi: 10.1002/2014GL059311
Stammerjohn, S., and Maksym, T. (2017). “Gaining (and losing) Antarctic Sea ice: variability, trends and mechanisms,” in Sea Ice. 3rd Edn. ed. D. N. Thomas (Oxford, UK: Wiley-Blackwell), 261–289.
Stammerjohn, S. E., Maksym, T., Massom, R. A., Lowry, K. E., Arrigo, K. R., Yuan, X., et al. (2015). Seasonal Sea ice changes in the Amundsen Sea, Antarctica, over the period of 1979–2014. Elementa Sci. Anthrop. 3:000055. doi: 10.12952/journal.elementa.000055
Stammerjohn, S. E., Martinson, D. G., Smith, R. C., Yuan, X., and Rind, D. (2008). Trends in Antarctic annual sea ice retreat and advance and their relation to El Niño–southern oscillation and southern annular mode variability. J. Geophys. Res. 108:C03S90. doi: 10.1029/2007JC004269
Stammerjohn, S. E., Massom, R., Rind, D., and Martinson, D. (2012). Regions of rapid sea ice change: an inter–hemispheric seasonal comparison. Geophys. Res. Lett. 39:L06501. doi: 10.1029/2012GL050874
Steinberg, D. K., Ruck, K. E., Gleiber, M. R., Garzio, L. M., Cope, J. S., Bernard, K. S., et al. (2015). Long–term (1993–2013) changes in macrozooplankton off the Western Antarctic peninsula. Deep–Sea Res. Part I 101, 54–70. doi: 10.1016/j.dsr.2015.02.009
Steiner, N. S., Bowman, J., Campbell, K., Chierici, M., Eronen Rasimus, E., Falardeau, M., et al. (2021). Climate change impacts on sea-ice ecosystems and associated ecosystem services. Elem. Sci. Anth. 9:1. doi: 10.1525/elementa.2021.00007
Sturm, M., and Massom, R. A. (2017). “Snow in the sea ice system: friend or foe?” in Sea Ice. ed. D. Thomas. 3rd ed (New York (USA) and Oxford (UK): Wiley–Blackwell), 65–109.
Sutherland, K. R., Madin, L. P., and Stocker, R. (2010). Filtration of submicrometer particles by pelagic tunicates. Proc. Natl. Acad. Sci. U. S. A. 107, 15129–15134. doi: 10.1073/pnas.1003599107
Swadling, K. M. (2014). “6.10 Sea-iceFauna,” in Biogeographic Atlas of the Southern Ocean. eds. C. De Broyer, P. Koubbi, H. J. Griffiths, B. Raymond and C. D Udekem Acozet (Cambridge: Scientific Committee on Antarctic Research), 321–325.
Swadling, K. M., Gibson, J. A. E., Ritz, D. A., and Nichols, P. D. (1997). Horizontal patchiness in sympagic organisms of the Antarctic fast ice. Antarct. Sci. 9, 399–406. doi: 10.1017/S0954102097000515
Swadling, K. M., McKinnon, A. D., Death, G., and Gibson, J. A. E. (2004). Life cycle plasticity and differential growth and development in marine and lacustrine populations of an Antarctic copepod. Limnol. Oceanogr. 49, 644–655. doi: 10.4319/lo.2004.49.3.0644
Swadling, K. M., McPhee, A. D., and McMinn, A. (2000). Spatial distribution of copepods in fast ice of eastern Antarctica. Polar Biosci. 13, 55–65.
Takahashi, K. D., Makabe, R., Takao, S., Kashiwase, H., and Moteki, M. (2022). Phytoplankton and ice-algal communities in the seasonal ice zone during January (Southern Ocean, Indian sector). J. Oceanogr. doi: 10.1007/s10872-022-00649-2
Tanimura, A., Fukuchi, M., and Ohtsuka, H. (1984). Occurrence and age composition of Paralabidocera Antarctica (Calanoida, Copepoda) under the fast ice near Syowa Station, Antarctica. NIPR Special Issue 32, 81–86.
Tanimura, A., Hoshiai, T., and Fukuchi, M. (1996). The life cycle strategy of the ice-associated copepod, Paralabidocera Antarctica (Calanoida, Copepoda), at Syowa Station, Antarctica. Antarct. Sci. 8, 257–266. doi: 10.1017/S0954102096000363
Thibodeau, P. S., Steinberg, D. K., Stammerjohn, S. E., and Hauri, C. (2019). Environmental controls on pteropod biogeography along the Western Antarctic Peninsula. Limnol. Oceanogr. 64, 240–256. doi: 10.1002/lno.11041
Thomas, P., and Green, K. (1988). Distribution of Euphausia crystallorophias within Prydz Bay and its importance to the inshore marine ecosystem. Polar Biol. 8, 327–331. doi: 10.1007/BF00442023
Thompson, P. G., McMinn, A., Kiessling, I., Watson, M., and Goldsworthy, P. M. (2006). Composition and succession of dinoflagellates and chrysophytes in the upper fast ice of Davis Station, East Antarctica. Polar Biol. 2, 337–345. doi: 10.1007/s00300-005-0060-y
Thorpe, S. E., Tarling, G. A., and Murphy, E. J. (2019). Circumpolar patterns in Antarctic krill larval recruitment: an environmentally driven model. Mar. Ecol. Prog. Ser. 613, 77–96. doi: 10.3354/meps12887
Tison, J.-L., Delille, B., and Papadimitriou, S. (2017). “Gases in sea ice” in Sea Ice. ed. D. N. Thomas. 3rd ed (Oxford, UK: Wiley-Blackwell), 433–471.
Turner, J., Bracegirdle, T. J., Phillips, T., Marshall, G. J., and Hosking, J. S. (2013). An initial assessment of Antarctic Sea ice extent in the CMIP5 models. J. Clim. 26, 1473–1484. doi: 10.1175/JCLI-D-12-00068.1
Turner, J., Holmes, C., Caton Harrison, T., Phillips, T., Jena, B., Reeves-Francois, T., et al. (2022). Record low Antarctic Sea ice cover in February 2022. Geophys. Res. Lett. 49:e2022GL098904. doi: 10.1029/2022GL098904
Turner, J., Phillips, T., Marshall, G. J., Hosking, J. S., Pope, J. O., Bracegirdle, T. J., et al. (2017). Unprecedented springtime retreat of Antarctic Sea ice in 2016. Geophys. Res. Lett. 44, 6868–6875. doi: 10.1002/2017GL073656
Vacchi, M., DeVries, A. L., Evans, C. W., Bottaro, M., Ghigliotti, L., Cutroneo, L., et al. (2012). A nursery area for the Antarctic silverfish Pleuragramma antarcticum at Terra Nova Bay (Ross Sea): first estimate of distribution and abundance of eggs and larvae under the seasonal sea–ice. Polar Biol. 35, 1573–1585. doi: 10.1007/s00300-012-1199-y
Vacchi, M., La Mesa, M., Dalù, M., and Macdonald, M. (2004). Early life stages in the life cycle of Antarctic silverfish, Pleuragramma antarcticum in Terra Nova Bay, Ross Sea. Antarct. Sci. 16, 299–305. doi: 10.1017/S0954102004002135
van Leeuwe, M., Tedesco, L., Arrigo, K. R., Assmy, P., Campbell, K., Meiners, K. M., et al. (2018). Microalgal community structure and primary production in Arctic and Antarctic Sea ice: a synthesis. Elem Sci. Anth. 6:4. doi: 10.1525/elementa.267
Virtue, P., Meyer, B., Freier, U., Nichols, P. D., Jia, Z., King, R., et al. (2016). Condition of larval (furcilia VI) and one year juvenile Euphausia superba during the winter–spring transition in East Antarctica. Deep-Sea Res. II 131, 182–188. doi: 10.1016/j.dsr2.2016.02.001
von Harbou, L., Dubischar, C. D., Pakhomov, E. A., Hunt, B. P. V., Hagen, W., and Bathmann, U. V. (2011). Salps in the Lazarev Sea, Southern Ocean: I. feeding dynamics. Mar. Biol. 158, 2009–2026. doi: 10.1007/s00227-011-1709-4
Waghorn, E. J., and Knox, G. A. (1988). Summer tide-crack zooplankton at White Island, McMurdo Sound, Antarctica. New Zeal. J. Mar. Fresh. 22, 577–582. doi: 10.1080/00288330.1988.9516327
Webster, M., Gerland, S., Holland, M., Hunke, E., Kwok, R., Lecomte, O., et al. (2018). Snow in the changing sea-ice systems. Nat. Clim. Chang. 8, 946–953. doi: 10.1038/s41558-018-0286-7
Weeks, W. F., and Ackley, S. F. (1986). “The growth, structure and properties of sea ice” in The Geophysics of Sea Ice. ed. N. Untersteiner (New York: Plenum Press), 9–164.
Wiebe, P. H., Ashjian, C. J., Lawson, G. L., Piñones, A., and Copley, N. J. (2011). Horizontal and vertical distribution of euphausiid species on the Western Antarctic peninsula US GLOBEC Southern Ocean study site. Deep-Sea Res. Part II 58, 1630–1651. doi: 10.1016/j.dsr2.2010.11.015
Wing, S. R., Leichter, J. J., Wing, L. C., Stokes, D., Genovese, S. J., McMullin, R. M., et al. (2018). Contribution of sea ice microbial production to Antarctic benthic communities is driven by sea ice dynamics and composition of functional guilds. Glob. Change Biol. 24, 3642–3653. doi: 10.1111/gcb.14291
Wongpan, P., Langhorne, P. J., Dempsey, D. E., Hahn-Woernle, L., and Sun, Z. (2015). Simulation of the crystal growth of platelet sea ice with diffusive heat and mass transfer. Ann. Glaciol. 56, 127–136. doi: 10.3189/2015AoG69A777
Wongpan, P., Meiners, K. M., Langhorne, P. J., Heil, P., Smith, I. J., Leonard, G. H., et al. (2018). Estimation of Antarctic land-fast sea ice algal biomass and snow thickness from under-ice radiance spectra in two contrasting areas. JGR Oceans 123, 1907–1923. doi: 10.1002/2017JC013711
Worby, A. P., Geiger, C. A., Paget, M. J., Van Woert, M. L., Ackley, S. F., and DeLiberty, T. L. (2008). Thickness distribution of Antarctic Sea ice. J. Geophys. Res. 113:C05S92. doi: 10.1029/2007JC004254
Yang, G., Atkinson, A., Hill, S. L., Guglielmo, L., Granata, A., and Li, C. (2020). Changing circumpolar distributions and isoscapes of Antarctic krill: indo–Pacific habitat refuges counter long-term degradation of the Atlantic sector. Limnol. Oceanogr. 66, 272–287. doi: 10.1002/lno.11603
Appendix: Qualitative network modelling
There is often considerable uncertainty surrounding the strength, functional forms, and even occasionally the direction of interactions within an ecological community. If we are confident in the type of interaction (e.g., predator–prey), but not the strength, we face parametric uncertainty. If we lack confidence in the nature of an interaction (e.g., whether a relationship is parasitic or mutualistic), then we face structural uncertainty. Qualitative network models were first developed to provide insights into community dynamics in the presence of parametric uncertainty. Early efforts to take a qualitative approach to existing community network theory harnessed the tools of graph theory and matrix analysis (Levins, 1974; Puccia and Levins, 1985). By shifting the focus away from precisely specified interaction terms and towards capturing the general community structure qualitatively, qualitative models can provide insights into the cumulative effects of simultaneous direct and indirect community interactions.
Qualitative network models may be understood most intuitively through the construction of a directed graph representing a community assumed to be at equilibrium. Graph nodes represent community members (either species or functional groups) and edges represent interactions between community members. An arrow at the terminus of an edge represents a positive interaction effect for that node, while a dot is typically used to indicate a negative interaction effect. The directed graph has a corresponding signed community matrix, A, where entry aij is either +, −, or 0, denoting a positive, negative, or neutral effect of the density of node j on the density of node i. Insights are gained by analysing this signed community matrix (see Puccia and Levins, 1985; Dambacher et al., 2002, 2003 for a more thorough treatment of these topics). If all eigenvalues of A are negative, then the community structure is stable (May, 1973). The effects of sustained changes to a model parameter (a so-called press perturbation) can be seen in the signed entries of −A−1 (Bender et al., 1984), or, alternatively, by considering the signs of the adjoint matrix, adj(−A) (Dambacher et al., 2002). This analysis suggests whether the density of each node will increase, decrease, or experience no change in response to a press perturbation in each of the other nodes.
Qualitative network models have been used to address a wide range of topics, such as identifying indicators of the effects of fishing (Metcalf et al., 2011), interpreting marine ecosystem changes in response to climate warming (Melbourne-Thomas et al., 2013), and exploring alternative strategies for invasive species eradication (Raymond et al., 2011). Analyses based on qualitative network models have been extended to incorporate modified interactions, in which the strength of an interaction between two species is modified by the density of a third species (Dambacher and Ramos-Jiliberto, 2007), as well as structural uncertainty (Raymond et al., 2011; Melbourne-Thomas et al., 2012).
Keywords: Southern Ocean, storms, warming, copepods, fish, primary production, krill, MEASO
Citation: Swadling KM, Constable AJ, Fraser AD, Massom RA, Borup MD, Ghigliotti L, Granata A, Guglielmo L, Johnston NM, Kawaguchi S, Kennedy F, Kiko R, Koubbi P, Makabe R, Martin A, McMinn A, Moteki M, Pakhomov EA, Peeken I, Reimer J, Reid P, Ryan KG, Vacchi M, Virtue P, Weldrick CK, Wongpan P and Wotherspoon SJ (2023) Biological responses to change in Antarctic sea ice habitats. Front. Ecol. Evol. 10:1073823. doi: 10.3389/fevo.2022.1073823
Edited by:
Christian Henri Nozais, Université du Québec à Rimouski, CanadaReviewed by:
Sarat Chandra Tripathy, National Centre for Polar and Ocean Research (NCPOR), IndiaConxita Avila, University of Barcelona, Spain
Copyright © 2023 Swadling, Constable, Fraser, Massom, Borup, Ghigliotti, Granata, Guglielmo, Johnston, Kawaguchi, Kennedy, Kiko, Koubbi, Makabe, Martin, McMinn, Moteki, Pakhomov, Peeken, Reimer, Reid, Ryan, Vacchi, Virtue, Weldrick, Wongpan and Wotherspoon. This is an open-access article distributed under the terms of the Creative Commons Attribution License (CC BY). The use, distribution or reproduction in other forums is permitted, provided the original author(s) and the copyright owner(s) are credited and that the original publication in this journal is cited, in accordance with accepted academic practice. No use, distribution or reproduction is permitted which does not comply with these terms.
*Correspondence: Kerrie M. Swadling, k.swadling@utas.edu.au