- Guangzhou Key Laboratory of Subtropical Biodiversity and Biomonitoring, Guangdong Provincial Key Laboratory of Biotechnology for Plant Development, School of Life Sciences, South China Normal University, Guangzhou, China
Mikania micrantha is one of the most notorious invasive weeds in south China, especially in orchard habitats. Based on the principle of niche competition, screening plants with strong competitiveness and managing vacant niches through natural alternative methods (replacement control) were expected to achieve sustainable ecological management of invasive species. To this end, two legumes, Desmodium heterocarpon and Senna tora, were selected to conduct field competition experiments with M. micrantha to investigate the interspecific competitiveness of these two legumes and M. micrantha from the aspects of adaptability to low light and response to drought stress. We found that the relative interaction indexes of D. heterocarpon and S. tora to M. micrantha were both negative and the competitive inhibition of S. tora on M. micrantha was higher than that of D. heterocarpon. Compared with M. micrantha, D. heterocarpon and S. tora have higher photosynthetic efficiency and lower dark respiration efficiency under low-light conditions, thus maintaining positive plant carbon balance capacity in the low-light understory and becoming more shade-tolerant. Besides, the water stress experiment found that M. micrantha had the lowest tolerance to drought stress, followed by S. tora, and D. heterocarpon was the most drought tolerant. These results showed that D. heterocarpon and S. tora can effectively prevent and control M. micrantha, mainly due to their higher competitiveness, shade tolerance, and drought tolerance. The control effect of D. heterocarpon is better than that of S. tora which is an alien species. Therefore, we believed that the replacement control of the invasive weed M. micrantha by D. heterocarpon is expected to be a sustainable ecological management strategy for M. micrantha biocontrol in the dryland orchard habitat. These findings provide a theoretical basis for the selection of species for alternative control in the future and provide new ideas for solving the problem of repeated regeneration in the existing M. micrantha control process.
1. Introduction
Due to the rapid development of economic globalization and international transportation, more and more species have been introduced, intentionally or unintentionally, into new environments previously isolated by natural barriers (Chen et al., 2016; Courchamp et al., 2017). These alien species are often highly adaptable and able to survive, reproduce, and spread in new environments, resulting in severe alien species invasions (Theoharides and Dukes, 2007). Biological invasions pose an enormous threat to ecosystems and public health by replacing native species, reducing biodiversity, altering community structure, and impairing ecosystem function (Slingenberg et al., 2009), resulting in huge economic losses (Pimentel et al., 2001). Therefore, how to effectively control invasive alien species, especially the control of alien weeds, is a hot spot in the field of invasive ecology research.
Mikania micrantha (mile-a-minute weed) is native to tropical regions of Central and South America and the Caribbean and is a fast-growing perennial vine belonging to the Asteraceae family (Choudhury et al., 2016). Due to natural and anthropogenic factors, M. micrantha is widely distributed in tropical and subtropical regions of Asia and the Pacific, and is one of the 100 worst invasive alien species announced by the International Union for Conservation of Nature (IUCN; Day et al., 2016). M. micrantha germinates early in the growing season, has a fast growth rate (up to 20 cm per day), and can reproduce vegetatively by forming roots and branches at each node, resulting in new individuals (Kaur et al., 2012). Therefore, if not controlled, it may rapidly spread to new habitats, covering the forest or plantation canopy (Day et al., 2012), causing the covered plants to suffocate and die due to lack of sunlight, so M. micrantha is also known as “plant killer” (Zhang et al., 2004). The rapid spread of M. micrantha not only destabilized the ecosystems in which it invaded, but also caused great economic losses (Day et al., 2016). For example, due to the invasion of M. micrantha every year in Neilingding Island in Shenzhen, China, the average annual economic loss amounts to CNY 4.5–10.13 million for the whole island (Zhong et al., 2004). Most notably, M. micrantha is responsible for the reduction of the production in many orchards by climbing up the orchard canopy and blocking the sunlight (Macanawai et al., 2012; Shen et al., 2015). It is thus essential to seek an effective method for controlling M. micrantha in orchards.
The serious invasion of M. micrantha in tropical and subtropical regions has attracted more and more attention around the world. In order to remove M. micrantha from the invasion area, different control methods have been developed, mainly divided into physical control, chemical control, and biological control (Li M. et al., 2012, 2015). Physical control, usually harvesting the above-ground part of the M. micrantha, can only control the invasion in a short term and does not completely eradicate M. micrantha, but may increase the potential threat of further spread (Swamy and Ramakrishnan, 1987). Herbicide is the main method of chemical control to remove M. micrantha. However, it can lead to drug resistance and chemical contamination of the local environment (Islam et al., 2018; Mohanty and Jena, 2019). At present, the most successful method for M. micrantha is biological control. It has been found that the rust fungus Puccinia spegazzinii and the butterfly Actinote anteas (natural enemy insect of M. micrantha) have good pathogenicity and encroach on M. micrantha, and can control M. micrantha to a certain extent (Barreto and Evans, 1995; Li et al., 2004; Day et al., 2013). Previous studies found that the parasitic field dodder Cuscuta campestris parasitized M. micrantha, which could cause M. micrantha to die (Li F. L. et al., 2012). However, due to the safety and limitations of biological control, there is no precedent for biological control of M. micrantha in orchards. The growth of fruit trees and the formation of orchard habitats have their unique characteristics. With the fruit trees bearing from young age to mature, the stand space appeared obvious differentiation, which was manifested as distinct differences in plant composition, spatial distance, and microclimate between canopy and understory. However, the long-term periodic reclamation and weeding operations of understory make understory vegetation dwarf, sparse, or even lose, and the ecological niche is finally vacant, resulting in ecosystem loopholes, which leads to the invasion of notorious weeds led by M. micrantha. If we choose native or valuable species with high competitiveness to cover the orchard ground based on replacement control technology, the growth of M. micrantha could be suppressed or its establishment can be prevented.
The current control methods for M. micrantha cannot solve the problem of regeneration and proliferation of M. micrantha, the main reason is that the management of the vacant ecological niche after the control is neglected. If we can use the principle of niche competition, select plants with strong competitiveness, and fill vacant niches progressively through natural succession, it will be possible to achieve ecological sustainability in the management of invasive species, which can be regarded as replacement control (Piemeisel and Carsner, 1951; Piemeisel, 1954; Li et al., 2015; Shen et al., 2015, 2016). Compared with chemical or mechanical control methods, replacement control is generally considered more economical, safe, eco-friendly, and sustainable. Regarding the replacement control of M. micrantha, the reported replacement plants are mainly herbs, shrubs, and fast-growing trees, such as grass species tall fescue Festuca arundinacea (Xu et al., 2011), Mosla chinensis (Song et al., 2020), perennial Italian ryegrass Lolium multiflorum (Xu et al., 2011), annual wormwood Artemisia annua (Xu et al., 2011) and sweet potato Ipomoea batatas (Shen et al., 2016), leguminous shrub Stylosanthes guianensis (Song et al., 2020), southeast Asian pioneer tree Macaranga tanarius (Li M. et al., 2012), and kesseru plant Heteropanax fragrans (Li M. et al., 2012), etc. Among them, the replacement effect of sweet potato was better, and field experiments have been done. The results showed that when sweet potato was mixed with M. micrantha, it can accumulate more biomass and absorb more soil nutrients, so it has a stronger competitive advantage (Shen et al., 2015), and can inhibit the reproductive ability of M. micrantha (Shen et al., 2016). However, the reported replacement plants and M. micrantha belong to different life forms and occupy different ecological niches. Although sweet potatoes can disperse by crawling, they cannot climb upward. According to the limiting similarity theory, species with similar niches tend to be more competitive, so whether they have a similar niche to invasive species is an important determinant for replacement plants to resist invasive species (Price and Partel, 2013; Kimball et al., 2014). Different species with the same ecological niche strive to suppress each other in order to compete for limited resources and space, which directly leads to interspecific competition (MacArthur and Levins, 1964). Replacement control research has recently focused on screening valuable or native species for competitiveness, mechanisms of competition, and native ecosystem restoration effects. Therefore, screening native species in similar ecological niches to control invasive species is beneficial to the sustainable ecological management of invasive species.
The principle of replacement control is to use the mutual competition between plant species to control harmful weeds, especially invasive weeds, with one or more plants with competitive advantage. Plant competition plays an important role in shaping individual plant morphology, physiology, and life history and in determining plant community structure and succession (Grace, 2012), thus plant competition is one of the areas that ecologists focus on. The maximum growth rate theory proposed by Grime (1979) and the minimum resource requirement theory by Tilman (1980) are two classic competition theories. Grime’s theory is based on the life strategies and traits of plants, and believes that the species with the greatest resource capture potential will be the competition winner (Grime, 1977, 1979). Tilman’s theory proposes a numerical model of plant individual populations with resource capture rates as a function of plant traits, which believes that the species with the smallest resource requirements will be the winner of the competition (Tilman, 1988). In recent years, many studies have carried out researches on the competition between indigenous plants and forages and invasive alien plants, and found that some indigenous plants and forages have a strong competitive effect on invasive alien plants, which can better control the growth, development, and spread of alien plants (Scasta et al., 2015). Our preliminary work found that using native legumes can be a better option for the replacement control of invasive weeds (Li et al., 2015). Therefore, in order to elucidate which theory can explain such phenomenon, we selected a variety of plants for trial to screen replacement plants in the orchard, and finally screened out two forage legumes with the potential to control M. micrantha, and explored the ecological processes and mechanisms of replacement control of M. micrantha under low-light and drought-stressed treatments in two legumes to provide a theoretical basis for the selection of replacement species in the future. The results obtained in this study are of great significance for ensuring ecological security and promoting sustainable prevention and control of invasive species and ecological restoration.
2. Materials and methods
2.1. Screening of replacement plants in the field
We selected an orchard with severe invasion of M. micrantha to carry out the replacement control experiment of M. micrantha. The orchard was located at Huaguo village in the northeast of Xinxu Town, Huiyang District, Huizhou City, Guangdong Province (22°52′24”N, 114°21′21″E, at the altitude of 93 meters), with a total area of 24 square kilometers and a subtropical monsoon climate. The village has sufficient water resources, and the fruit trees mainly include bananas and wampees. Due to its proximity to Shenzhen, most of the orchards in the village were invaded by M. micrantha. In June 2016, clean tillage was carried out in an orchard of Huaguo Village with mowing of natural weed flora in case of weeds emerged. We also applied herbicide with a hand pump sprayer backpack to make the plot weed-free. After the clean tillage was completed, we established 16 plots, including 3 plots of Desmodium heterocarpon monoculture, 3 plots of Senna tora monoculture, 4 plots of Spermacoce latifolia monoculture, 3 plots of Ageratum conyzoides monoculture, and 3 plots of M. micrantha monoculture. Each plot was 3 m × 3 m. After the experimental plot was constructed, photographs were taken once a month to record the control effects of the four test plants on M. micrantha.
2.2. Interspecific competition experiment in the field
The experimental materials were D. heterocarpon, S. tora, and M. micrantha, and the seeds were collected from the replacement control experimental site in Huaguo village, Huizhou city from November to December 2016. The experimental design was as follows: M. micrantha, D. heterocarpon, and S. tora were planted individually; D. heterocarpon and S. tora were planted with M. micrantha together with a ratio of 1:1, respectively. There were five treatments in total, and each plot was 1.5 m × 1.5 m. Three months after transplanting, the coverage and plant height of D. heterocarpon, S. tora and M. micrantha was measured. The number of leaves per plant was counted, and the area of leaves with medium size was measured with a leaf planimeter (LI-3100, LI-COR, USA). At the end of the experiment, the medium-sized plants in each treatment were harvested. The shoots and roots were separated from each plant and dried to constant weight for at least 72 h at 60°C and then weighed to get the total biomass of shoots and roots.
Relative interaction index (RII) was calculated as: RII = (Bw-Bo)/(Bw + Bo), where Bw was the observed biomass of the target plant when growing with other species and Bo was the biomass of the target plant when growing individually. A negative value indicates that other species compete with the target plant and therefore inhibit the growth of target plants. A positive value indicates that other species promote the growth of the target plant.
2.3. Low-light treatment of Desmodium heterocarpon, Senna tora, and Mikania micrantha
2.3.1. Plant materials and seedling cultivation
The seeds of M. micrantha, D. heterocarpon, and S. tora were collected from the replacement control experimental field and germinated in Petri dishes. After germination, the seedlings were transferred to a float tray with nutrition soil and we watered them to keep the soil moist. After about 30 days of growth, healthy seedlings were selected and transplanted into a pot (plastic flowerpot 13 cm in diameter and 12 cm in height filled with mixed soil up to 2/3 of the height of the pot to ensure that the soil weight of each pot was basically the same) in the greenhouse on the top floor of the School of Life Science, South China Normal University. The mixed soil is composed of garden soil, which was collected from the topsoil of the Botanical Garden of South China Normal University, and nutrition soil in a ratio of 1:1.
2.3.2. Low-light treatment establishment
Three light conditions (light 100%, light 42%, and light 17%) were established. After 2 weeks of adaptive cultivation, the transplanted seedlings were shaded under three light conditions. There were 8 replicates per treatment for M. micrantha, D. heterocarpon, and S. tora (72 pots in total). All pots were positioned randomly and the experiment lasted for 60 days. At the end of the experiment, the plants in each treatment were harvested. The above-ground and below-ground parts were separated from each plant and dried to a constant weight for at least 72 h at 60°C and then weighed. The total biomass was the sum of above-ground and below-ground parts.
2.3.3. Measurements of chlorophyll fluorescence parameters
Chlorophyll fluorescence parameters were determined on a sunny day with a portable PAM-2100 fluorometer (Waltz, Germany) once a week. All fluorescence measurements were started after an additional 20 min dark adaptation. Minimal fluorescence (F0) and maximal fluorescence (Fm) were recorded and the maximal photochemical efficiency (Fv/Fm) is calculated as: Fv/Fm = (Fm-F0)/Fm. After the plant leaves were adapted to the light intensity of 200 mol m−2 s−1, steady-state fluorescence yield (Fs), maximal fluorescence yield (Fm′), electron transfer rate (ETR), and quantum yield of photosystem II (Yield) were recorded once per week.
2.3.4. Measurements of the light response curve
The light response curve was determined on a clear sunny day with a Li-6,400 portable photosynthesis analyzer (LiCor Inc., United States). The measurement time was 9:00–11:00 a.m. and 3:00–5:00 p.m. The chamber flow rate was adjusted to 500 μmol s−1. Before measuring the curve, the light intensity of 1,600 μmol m−2 s−1 was set to induce the leaves to reach a stable state. The light intensity gradient was set to 1,600, 1,400, 1,200, 1,000, 800, 600, 400, 200, 100, 40, 20, and 0 μmol m−2 s−1. After reaching the maximum stabilization time, we recorded the parameters of the instrument at each light intensity and measured continuously for 2 days until all data were obtained. Next, we took the light intensity as the x-axis and the net photosynthetic rate as the y-axis to draw a scatter plot of the light response curve. According to the method of Feng et al. (2004), we fitted the light response curve equation to calculate the maximum net photosynthetic rate (Pmax), light compensation point (LCP), light saturation point (LSP), dark respiration rate (Rd), and apparent quantum yield (AQY). AQY was represented by the initial slope of the light response curve when the light intensity was less than 200 mol m−2 s−1 and was fitted by the standard form of linear regression.
2.4. Drought-stressed treatment of Desmodium heterocarpon, Senna tora, and Mikania micrantha
After 1 week of adaptive cultivation of the seedlings, drought stress experiment was carried out. All experimental groups were subjected to drought treatment after fully absorbing water. The method of drought stress was carried out by natural drought without water. The control group was normally watered. The experimental group was treated with drought stress for 10 days and rehydrated for 3 days from the 11th day. There were 12 replicates per treatment for M. micrantha, D. heterocarpon, and S. tora (108 pots in total). All pots were placed randomly. At the end of the experiment, the plants in each treatment were harvested. The above-ground and below-ground parts were separated from each plant and dried to a constant weight for at least 72 h at 60°C and then weighed. The total biomass was the sum of above-ground and below-ground parts.
2.4.1. Measurements of gas exchange parameters in the drought stress experiment
Gas exchange parameters were determined using the Li-6,400 portable photosynthesis analyzer (LiCor Inc., United States) at 9:00 a.m. and were completed within 2 h on a sunny day. The photosynthetic photon flux density (PPFD) of the natural light was set to 1,000 μmol m−2 s−1 under daily ambient temperatures varied from 28 to 30°C. CO2 concentration inside the leaf chamber was maintained at 380 cm3 m−3 through the CO2-controlling system of the Li-6,400 attached to a portable CO2 cylinder. The PPFD of 1,000 μmol m−2 s−1 on the cuvette surface was provided by an LED source. Before taking measurements, the leaves were equilibrated under artificial light conditions in the leaf chamber for at least 10 min. Net photosynthetic rate (Pn), intercellular CO2 concentration (Ci), stomatal conductance (Gs), and transpiration rate (Tr) were recorded.
2.4.2. Measurements of proline content in the drought stress experiment
Proline was extracted by sulfosalicylic acid and the proline content was determined by ninhydrin colorimetry. 0.1 g fresh weight leaves of each plant in the control group (well-watered treatment) and the experiment group on the 10th day of drought treatment and the 3rd day of rehydration were placed in a centrifuge tube, respectively. 2.5 ml 3% sulfosalicylic acid solution was added to each tube and then all tubes were treated in the boiling water bath for 10 min. After filtering and cooling, 1 ml filtrate was absorbed into another centrifuge tube and then 1 ml glacial acetic acid and 1 ml 2.5% acid ninhydrin reagent were added. The mixture was heated in the boiling water bath for 30 min and turned red. After the mixture was cooled, 2 ml toluene was added. After 30 s of oscillation, the supernatant was transferred into a 10 ml centrifuge tube and centrifuged at 3000 rpm for 5 min. The red proline toluene supernatant was absorbed into a colorimetric cup. The toluene solution was used as blank control and the absorbance value was determined at A520. The standard curve of proline was prepared and the content of proline was calculated according to the regression equation of the standard curve.
2.4.3. Measurements of soluble sugar content in the drought stress experiment
The soluble sugar content was determined by thiobarbituric acid method. 0.1 g plant leaves were placed in a pre-cooled mortar and 1 ml pre-cooled 10% acetic acid (TCA) was added. After the leaves were grounded on ice homogeneously, the homogenate was transferred to the centrifuge tube, and then the mortar was washed with 1 ml 10% TCA. The washing liquid was also transferred to the centrifuge tube and centrifuged at the speed of 4,000 rpm for 10 min. 1 ml supernatant (1 ml sterile water in blank control) and 1 ml 0.6% thiobarbituric acid solution (TBA) were added into a new centrifuge tube and thoroughly mixed. The mixture was heated in a boiling water bath for 15 min and centrifuged at the speed of 4,000 rpm for 10 min after cooling down at room temperature. The supernatant of the mixture was taken to determine the absorbance value at A450.
2.5. Statistics analysis
We used one-way ANOVA analysis and Duncan’s test (p < 0.05) to test for differences between treatments using SPSS 18.0 (SPSS Inc., United States). SigmaPlot 12.0 and Origin 8.5 software were used for data visualization.
3. Results
3.1. The preliminary selection of replacement plants in the field
One month after clean tillage (Figure 1A), the seedlings of S. tora successfully established with a survival rate of over 95% (Figure 1B). The seedlings of D. heterocarpon successfully established with a survival rate of over 90% (Figure 1C). A large number of S. latifolia and a few M. micrantha individuals regenerated in the quadrats gap (Figure 1D). A few seedlings of A. conyzoides but a large number of M. micrantha individuals regenerated in the quadrats (Figure 1E). Four months after clean tillage (Figure 1G), S. tora entered into reproductive growth, some of the leaves aged and turned yellow, and lots of pods grew. Few M. micrantha was found in the plot, but weeds appeared in the lower layer (Figure 1H). D. heterocarpon also entered into reproductive growth, bearing a lot of pods, but the leaves remain green, with a small amount of M. micrantha (Figure 1I). When S. latifolia entered the aging stage, its stem lodging occurred and leaves withered and turned yellow, and a large amount of M. micrantha occupied the quadrats (Figure 1J). A. conyzoides entered the aging stage, while M. micrantha grew vigorously, occupying most of the quadrats (Figure 1K). Therefore, we did not involve S. latifolia and A. conyzoides in the following experiments. We observed that compared with the natural growth of M. micrantha (Figure 1F, L), D. heterocarpon and S. tora had obvious replacement control effect on M. micrantha, which can inhibit the growth and regeneration of M. micrantha in the orchards. Next, we used these two plants as replacement plants to study the interspecific competition effects between two legumes and M. micrantha in the field.
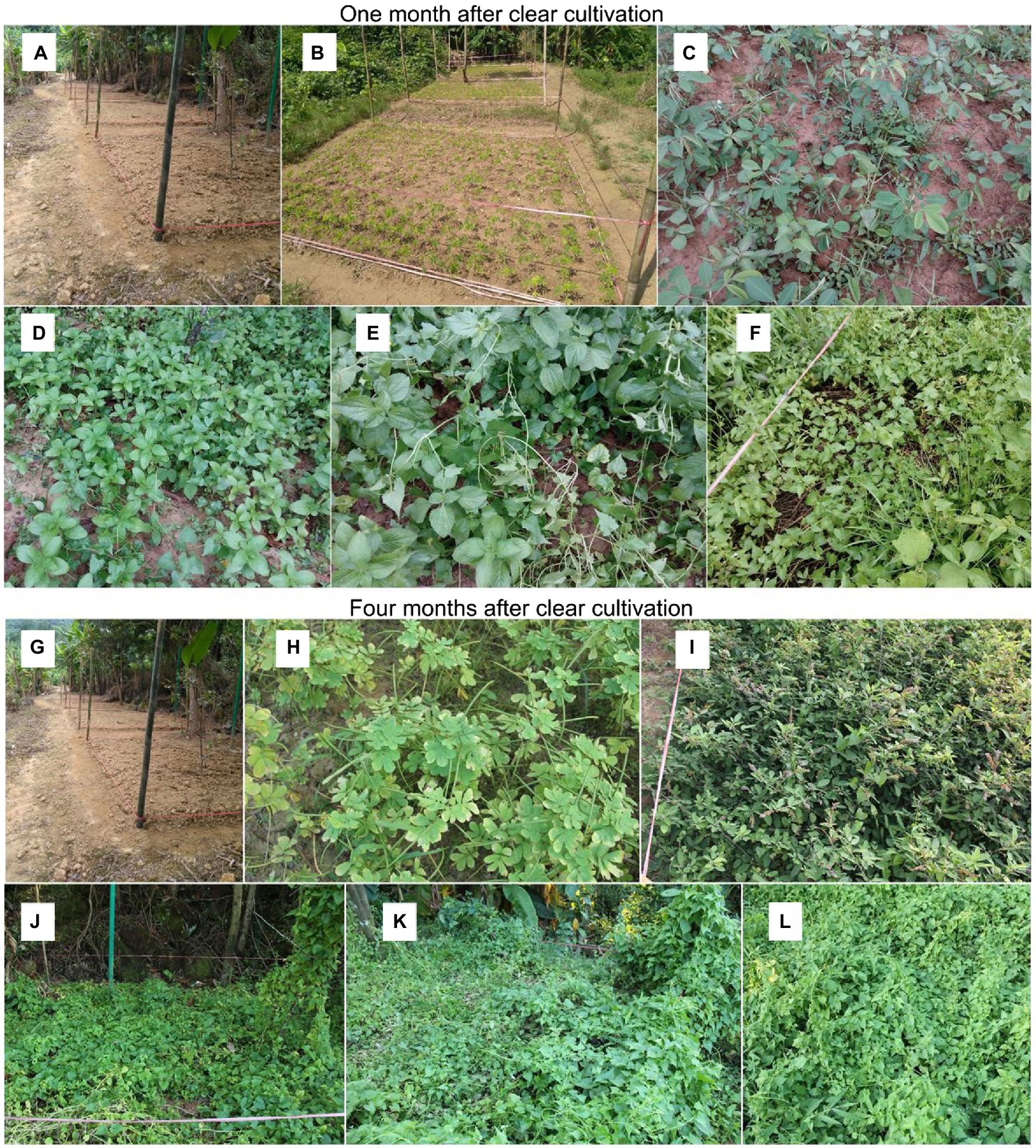
Figure 1. Growth status of four replacement plants after one and four months of clean tillage, respectively. (A, G) The original status after clean tillage; (B, H) S. tora; (C, I) D. heterocarpon; (D, J) S. latifolia; (E, K) A. conyzoides; (F, L) M. micrantha naturally regenerated as the control.
3.2. Interspecific competition between two legumes and Mikania micrantha in the field
When M. micrantha was planted with S. tora or D. heterocarpon, its coverage was significantly decreased compared with that of M. micrantha monoculture, which was 77% or 73.9%, respectively. Compared to D. heterocarpon monoculture, the coverage of D. heterocarpon decreased significantly by 17.3% when it was planted with M. micrantha, while the coverage of S. tora was not significantly different between monoculture and polyculture with M. micrantha (Figure 2A). Due to the competition of S. tora and D. heterocarpon, the leaf area of M. micrantha per plant decreased significantly by 30.9 and 36.3%, respectively. On the contrary, the leaf area of S. tora and D. heterocarpon per plant increased significantly by 7.1 and 25%, respectively, when they were planted with M. micrantha, compared to the monoculture (Figure 2B). The total biomass of M. micrantha decreased by 23.5 and 58.3%, respectively, when M. micrantha was planted with S. tora and D. heterocarpon, compared to the monoculture. There was no significant difference between the total biomass of D. heterocarpon when it was planted with M. micrantha and the monoculture, so as S. tora (Figure 2C). The RII can indicate the interaction between plants. A negative value indicates competition and a positive value indicates facilitation. The RII of M. micrantha in the presence of D. heterocarpon and S. tora were both negative (RIIMm (Dh) = −0.42, RIIMm(St) = −0.13, respectively), indicating that two replacement species competed well with M. micrantha, and that the strength of the negative effect of D. heterocarpon on M. micrantha was larger than that of S. tora on M. micrantha (Figure 2D). On the other hand, the effect of M. micrantha on D. heterocarpon was negative [RIIDh(Mm) = −0.03], close to zero, while the effect of M. micrantha on S. tora was positive [RIISt(Mm) = 0.12, Figure 2D], indicating that M. micrantha could promote the growth of S. tora.
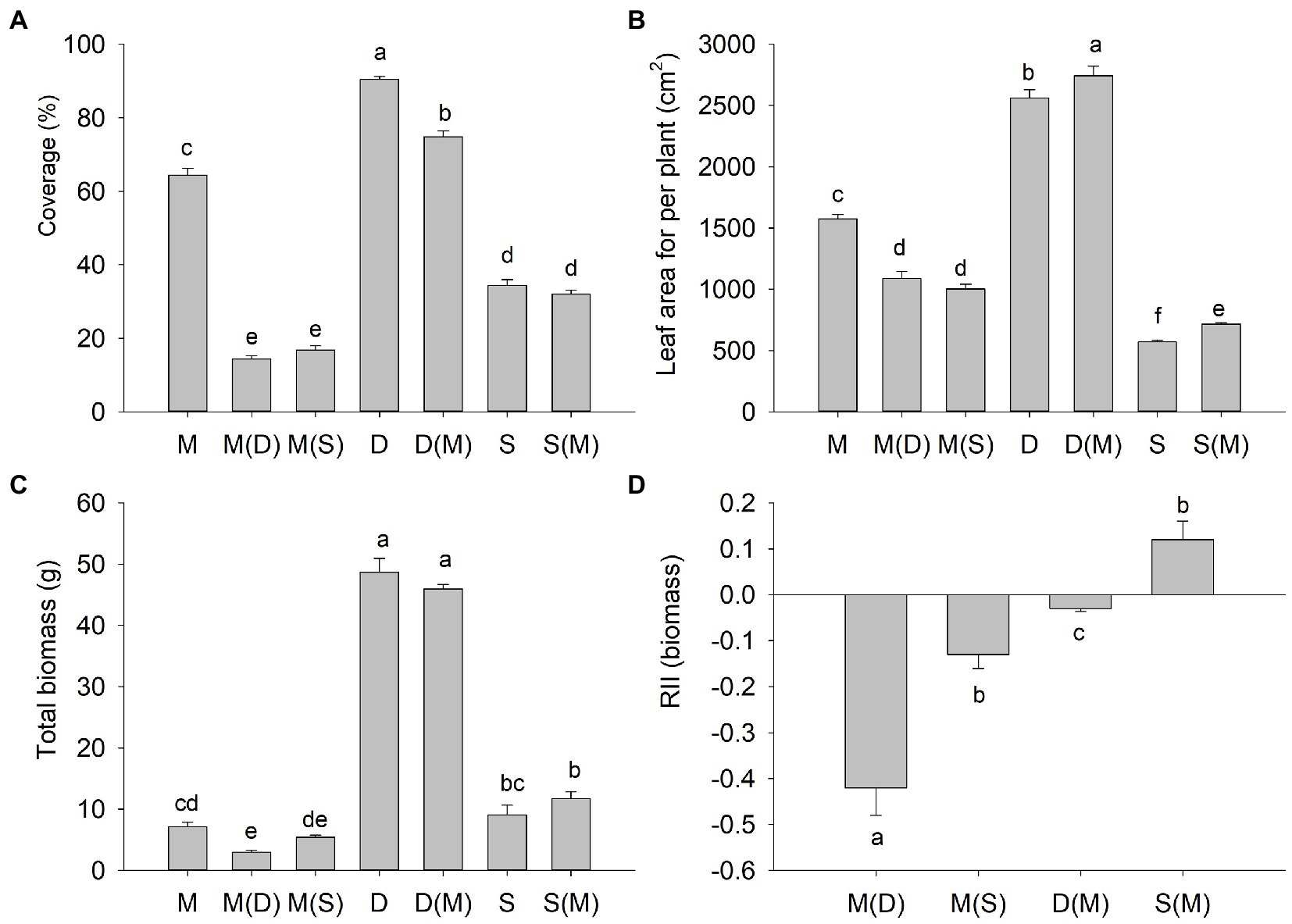
Figure 2. Changes of the plant coverage (A), leaf area (B), total biomass (C), and relative interaction index (D) of M. micrantha and the replacement plants under different interspecific competition experiments (average ± SE, n = 4). M(D): M. micrantha when grown under competition with D. heterocarpon; M(S): M. micrantha when grown under competition with S. tora; D(M): D. heterocarpon when grown under competition with M. micrantha; S(M): S. tora when grown under competition with M. micrantha. Different letters above bar plots indicate significant differences (Duncan test, p < 0.05).
3.3. The adaptability of two replacement forage legumes and Mikania micrantha to low-light stress
Under light 100%, M. micrantha had more branches and leaves, but the leaves turned yellow. As the light intensity decreased, the number of branches and leaves of M. micrantha decreased significantly and the greenness of the leaves deepened. M. micrantha was the smallest and grew the worst under light 17% (Figure 3A). Under light 100% and light 42%, D. heterocarpon had more branches and leaves. The number of branches and leaves decreased under light 17%. As the light intensity decreased, the greenness of D. heterocarpon leaves deepened (Figure 3B). Under light 100%, S. tora grew low and stout with yellowish leaves and branches; with the decrease in light intensity, the plants became thinner and taller, and the greenness of leaves deepened. S. tora was the highest under light 42% (Figure 3C).
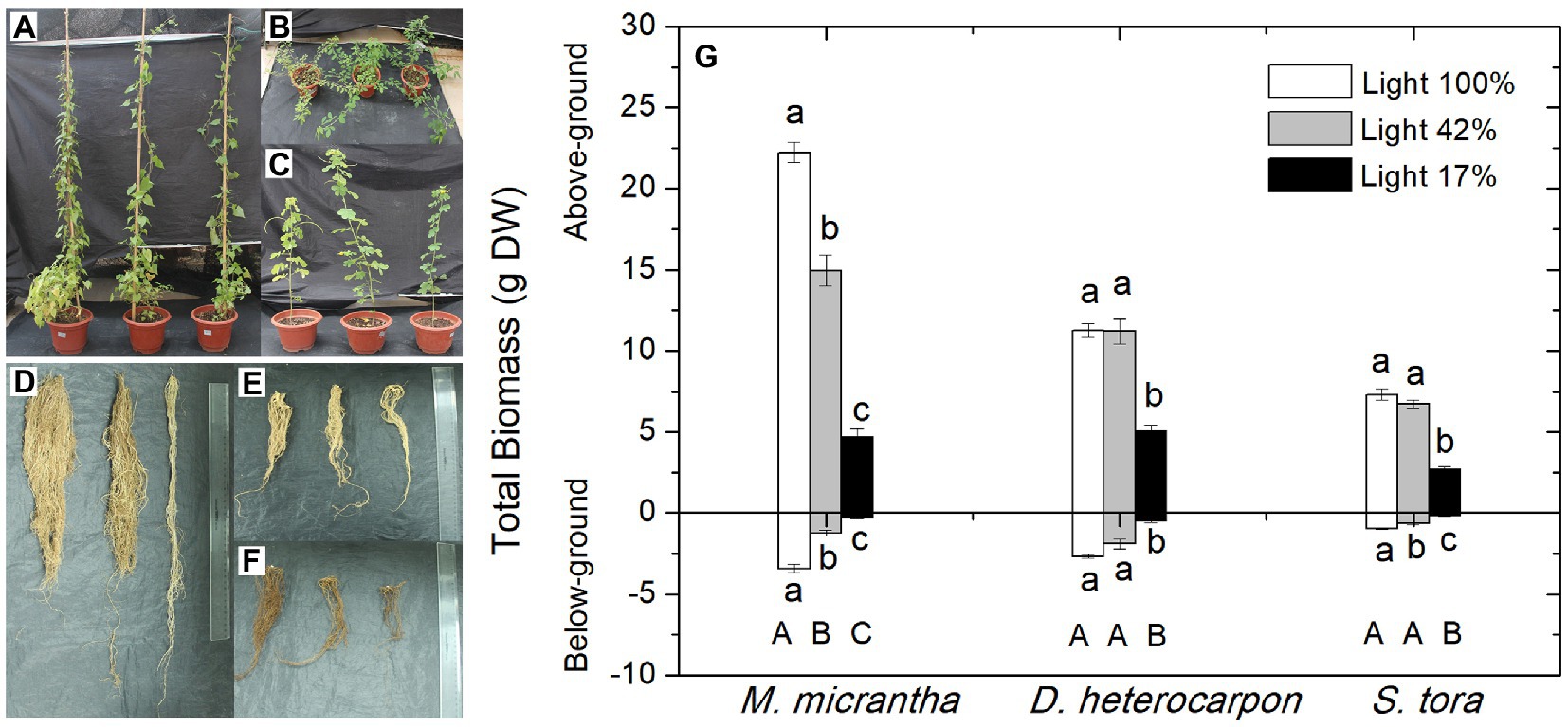
Figure 3. Changes of shoot phenotype, root phenotype and biomass of two replacement forage legumes and M. micrantha under different light conditions. (A, D) Represent M. micrantha; (B, E) Represent D. heterocarpon; (C, F) represent S. tora. The treatments of each plant from left to right were light 100%, light 42% and light 17%, respectively. (G) The average values ± standard errors of five replicates were shown. The lowercase letters above bar plots represent the difference in the above-ground biomass of each plant under different light treatments. The lowercase below bar plots represent the difference in the below-ground biomass of each plant, and the capital letters below bar plots represent the difference in the total biomass (Duncan test, p < 0.05).
From the perspective of root phenotype changes, M. micrantha had the most developed roots and the largest number of roots under light 100%. With the decrease in light intensity, the number of roots decreased significantly. Under light 17%, the growth of roots was greatly affected and the number of roots was the least (Figure 3D). Under light 100%, the roots of D. heterocarpon were the most developed. With the decrease in light intensity, the number of roots gradually decreased, and the number of roots was the least under light 17% (Figure 3E). Under light 100%, the number of S. tora roots was the largest, and with the decrease in light intensity, the number of roots decreased dramatically. Under light 17%, the growth of S. tora roots was greatly inhibited and the number was the least (Figure 3F).
Compared with light 100%, the above-ground biomass of M. micrantha decreased significantly by 32.8 and 78.8% under light 42% and light 17%, respectively (Figure 3G). The above-ground biomass of D. heterocarpon and S. tora decreased by 55 and 63.6% under light 17% compared with that under light 100%. The root biomass of M. micrantha decreased significantly by 63 and 91.3% under light 42% and light 17%, respectively, compared with that under light 100%. The root biomass of D. heterocarpon significantly decreased by 81% under light 17% compared with light 100%, while there was no significant difference between the root biomass of D. heterocarpon under light 42% and light 100%. The root biomass of S. tora was significantly decreased by 31.3 and 79.7% under 42% and light 17% compared with light 100%, respectively. Under light 42% and light 17%, the total biomass of M. micrantha decreased significantly by 38.5 and 86.5%, respectively. The total biomass of D. heterocarpon decreased significantly by 60.2% under light 17% compared with light 100%and the total biomass of S. tora decreased by 66.1% under light 17% compared with light 100%. It can be seen that under the low-light treatment the biomass of M. micrantha decreased the most, followed by S. tora, and the biomass of D. heterocarpon was the smallest, and it was also the least affected by low light.
From the perspective of photosynthetic parameters, Pmax of M. micrantha significantly decreased by 23.4 and 37.8% respectively, under light 42% and light 17%, compared with light 100% (Table 1). There was no significant difference in Pmax between D. heterocarpon and S. tora under three light conditions, indicating that D. heterocarpon and S. tora have higher photosynthetic efficiency under medium-light and low-light conditions. Under the conditions of light 100% and light 17%, the LSPs of M. micrantha and S. tora were greater than those of D. heterocarpon, indicating that D. heterocarpon has stronger photosynthetic utilization ability under low-light conditions. The LCPs of M. micrantha and D. heterocarpon both decreased with the decrease in light intensity, and the LCP of D. heterocarpon was lower than that of M. micrantha and S. tora under light 42% and light 17%, indicating that D. heterocarpon was more tolerant to low-light condition. There was no significant difference in the AQY of D. heterocarpon and S. tora under different light conditions, while the AQY of M. micrantha significantly decreased by 22.4 and 28.2% under light 42% and light 17%, respectively, compared with light 100%, which indicated that the two forage legumes were more efficient for low light. The Rd. of M. micrantha under light 42% and light 17% was not significantly different from that under light 100%. Under light 42% and light 17%, the Rd. of D. heterocarpon decreased significantly by 29.9 and 38.8% compared with light 100%, respectively, and that of S. tora decreased significantly by 32.7% under light 17% compared with light 100%, which indicated that under low-light conditions, D. heterocarpon and S. tora can adapt to the low-light environment by reducing their own energy consumption.
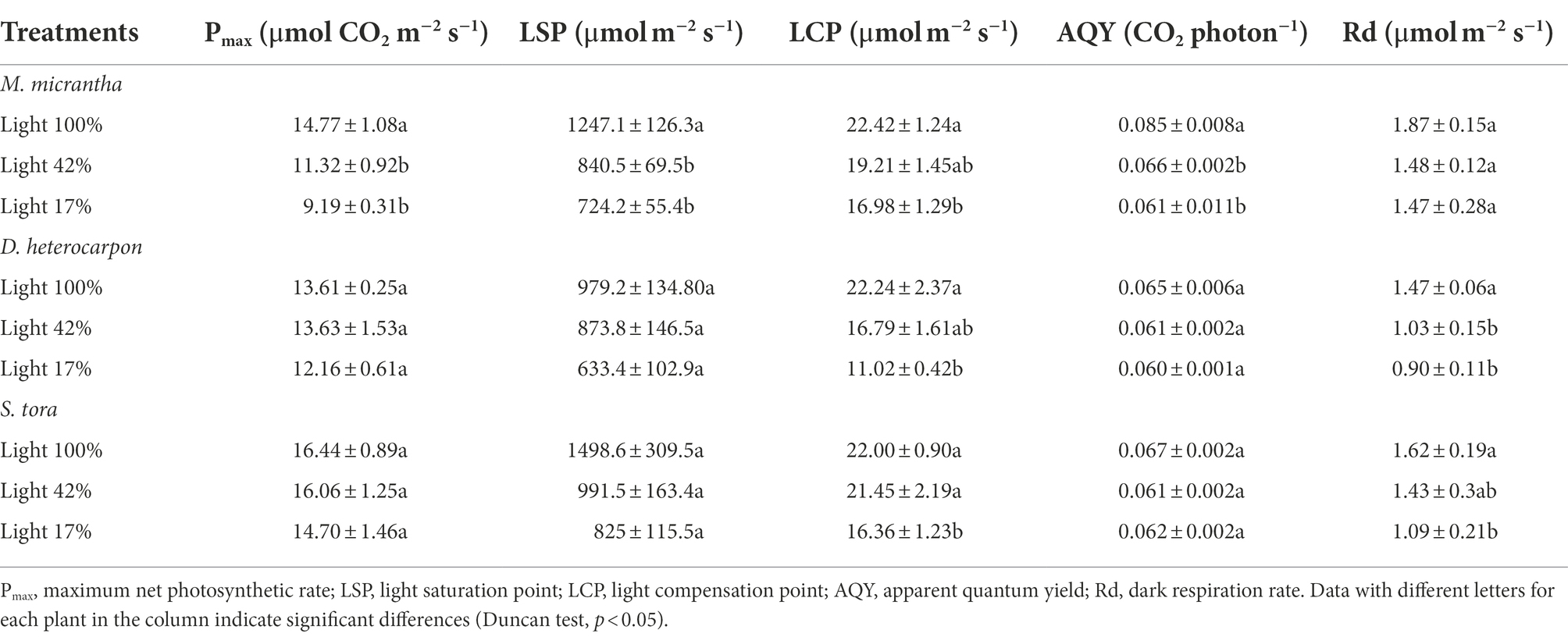
Table 1. Changes of photosynthetic parameters under different light conditions (average ± SE, n = 4).
With the increase of treatment time, the Fv/Fm of M. micrantha continued to decrease under the three light conditions (Figure 4A), while that of D. heterocarpon was not significant under different light conditions and showed an upward trend (Figure 4B). Similar to M. micrantha, the Fv/Fm of S. tora also continued to decrease under three light conditions (Figure 4C). Under light 100%, the ETR and Yield of M. micrantha remained stable, showed a downward trend under light 42% and light 17%, and decreased dramatically under light 17% (Figures 4D, G). The ETR and Yield of D. heterocarpon showed an upward trend under all light conditions (Figures 4E, H), showing good adaptability to the low-light environment. The ETR and Yield of S. tora increased gradually with the increase of treatment time, and there was little difference among different treatments (Figures 4F, I). After light 42% and light 17% treatment for 49 days, the photochemical quenching (qp) of M. micrantha decreased significantly compared with light 100% (Figure 4J), while that of D. heterocarpon and S. tora both increased with the increase of treatment time (Figures 4K, L).
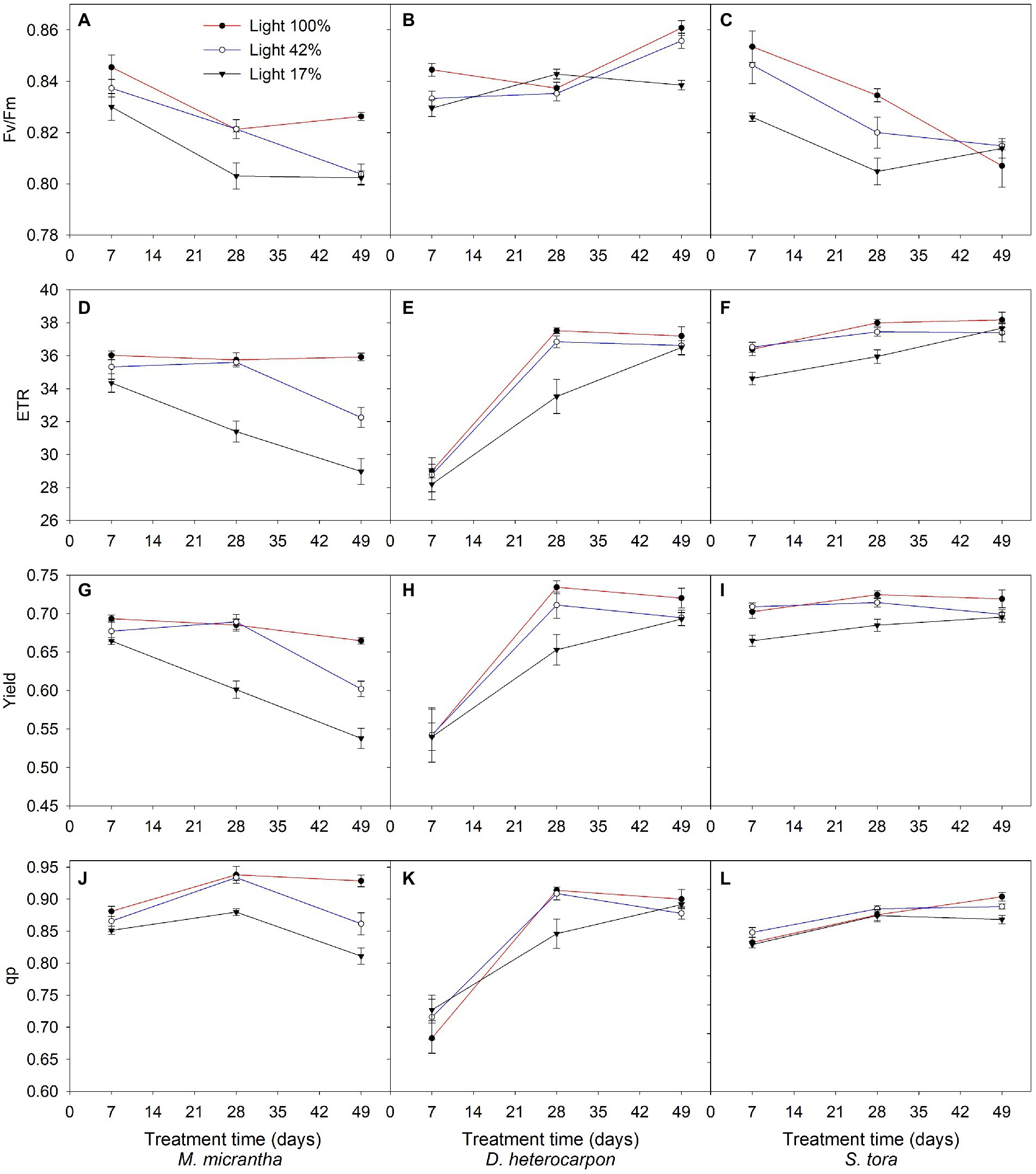
Figure 4. Changes of chlorophyll fluorescence parameters of two replacement forage legumes and M. micrantha under different light treatments (average ± SE, n = 3). (A, D, G, J) M. micrantha; (B, E, H, K) D. heterocarpon; (C, F, I, L) S. tora.
3.4. The response of two replacement forage legumes and Mikania micrantha to drought stress
After 7 days of drought treatment, the leaves of M. micrantha began to wilt and the leaves of S. tora wilted on the 10th day, while the leaves of D. heterocarpon did not change significantly (Figure 5). After 10 days of drought treatment, the phenotypes of two replacement forage legumes and M. micrantha showed obvious differences. The leaves of D. heterocarpon were still green without visible changes, while the leaves of M. micrantha shrunk due to water loss, and the stem tips were dry and withered. The leaves of S. tora also lost water and wilted, and the leaves were closed, but the degree of wilting was significantly lower than that of M. micrantha. From the observation of phenotype, the tolerance to drought stress of D. heterocarpon was higher than that of S. tora and M. micrantha, among which M. micrantha has the deepest degree of wilting. After rehydration, the leaves of M. micrantha were still wilted, the wilting symptoms of S. tora leaves disappeared, and the leaves of D. heterocarpon did not change significantly and remained green.
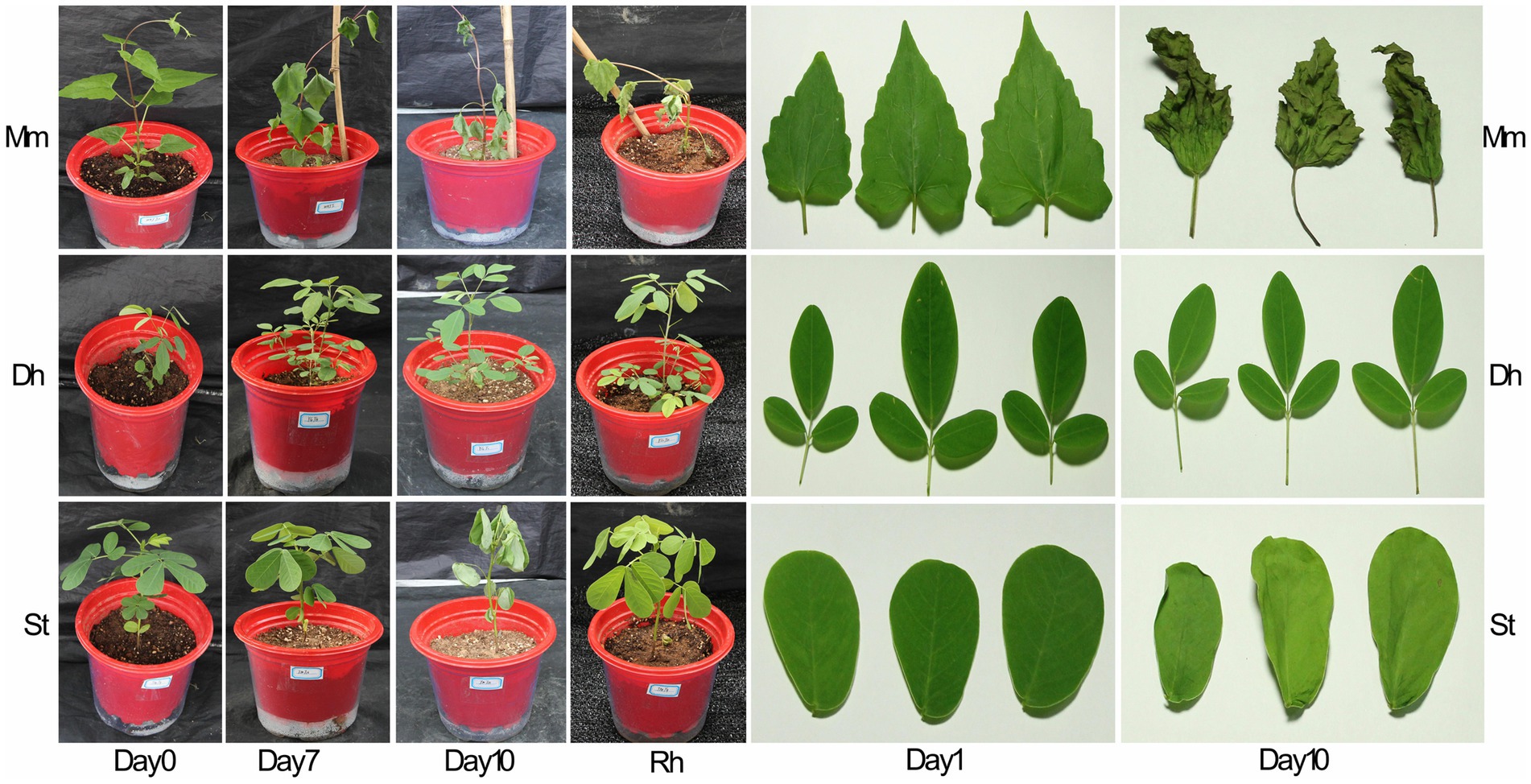
Figure 5. Changes of plant phenotype and leaf phenotype of two replacement forage legumes and M. micrantha under drought stress. Mm: M. micrantha; Dh: D. heterocarpon; St: S. tora; Rh: rehydration.
Under drought stress, the total biomass of M. micrantha and S. tora decreased significantly by 65 and 14.9%, respectively, while D. heterocarpon was not affected by drought stress (Figure 6A). The root biomass of M. micrantha was significantly reduced under drought stress, while neither D. heterocarpon nor S. tora was affected by drought stress (Figure 6C). Under the drought treatment, the plant height and root length of M. micrantha decreased significantly by 38.7 and 32%, respectively, compared with the well-watered group (Figures 6B, D). There were no significant differences in plant height and root length between the well-watered group and drought stress group for D. heterocarpon and S. tora (Figures 6B, D).
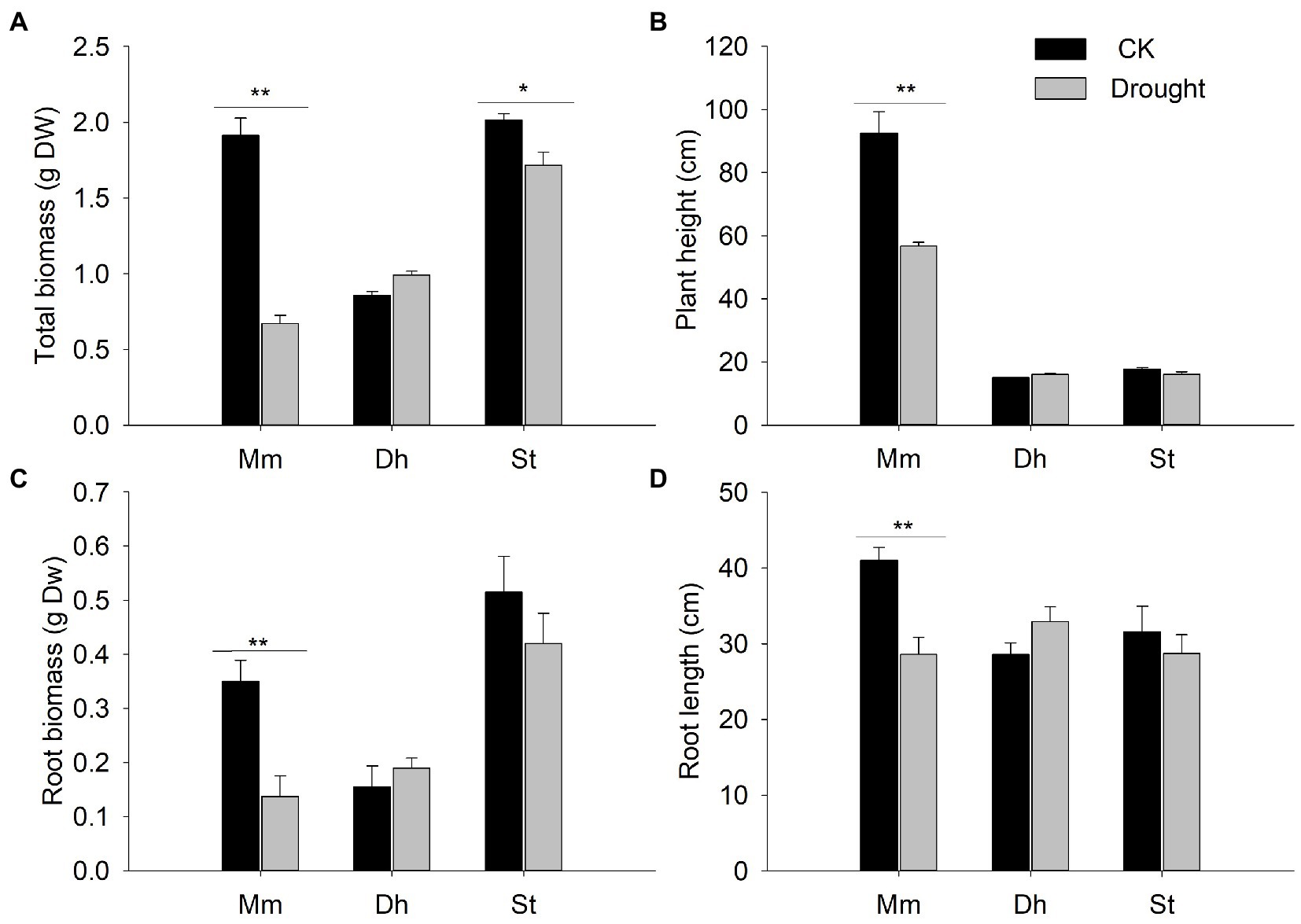
Figure 6. Changes of (A) total biomass, (B) plant height, (C) root biomass, and (D) root length of two replacement forage legumes and M. micrantha under drought stress (average ± SE, n = 4). Different letters above bar plots indicate significant differences analyzed using ANOVA followed by Dunnett’s test. Significance levels: ** p < 0.01; * p < 0.05.
Drought stress had a significant effect on the Pn of M. micrantha and S. tora. With the increase of drought treatment time, Pn of M. micrantha and S. tora showed a continuous downward trend, and M. micrantha had the largest decrease (96.71%), followed by S. tora, with a decrease of 92.4%, while the Pn of D. heterocarpon remained at a relatively high level after 10 days of drought treatment, only decreased by 2.4%. After rehydration, the Pn of M. micrantha remained at a low level and could not be recovered, and that of S. tora recovered to a level comparable to the well-watered group, while that of D. heterocarpon increased slightly (Figure 7A). The Gs and Tr of M. micrantha and S. tora showed similar changing patterns, both of which continued to decline with the increase of drought treatment time. After rehydration, the Gs and Tr of M. micrantha could not recover to a level comparable to the well-watered group, while that of S. tora could basically recover (Figures 7B, D). During the drought treatment, Gs, Ci, and Tr of D. heterocarpon remained at a stable level with little change (Figures 7B–D).
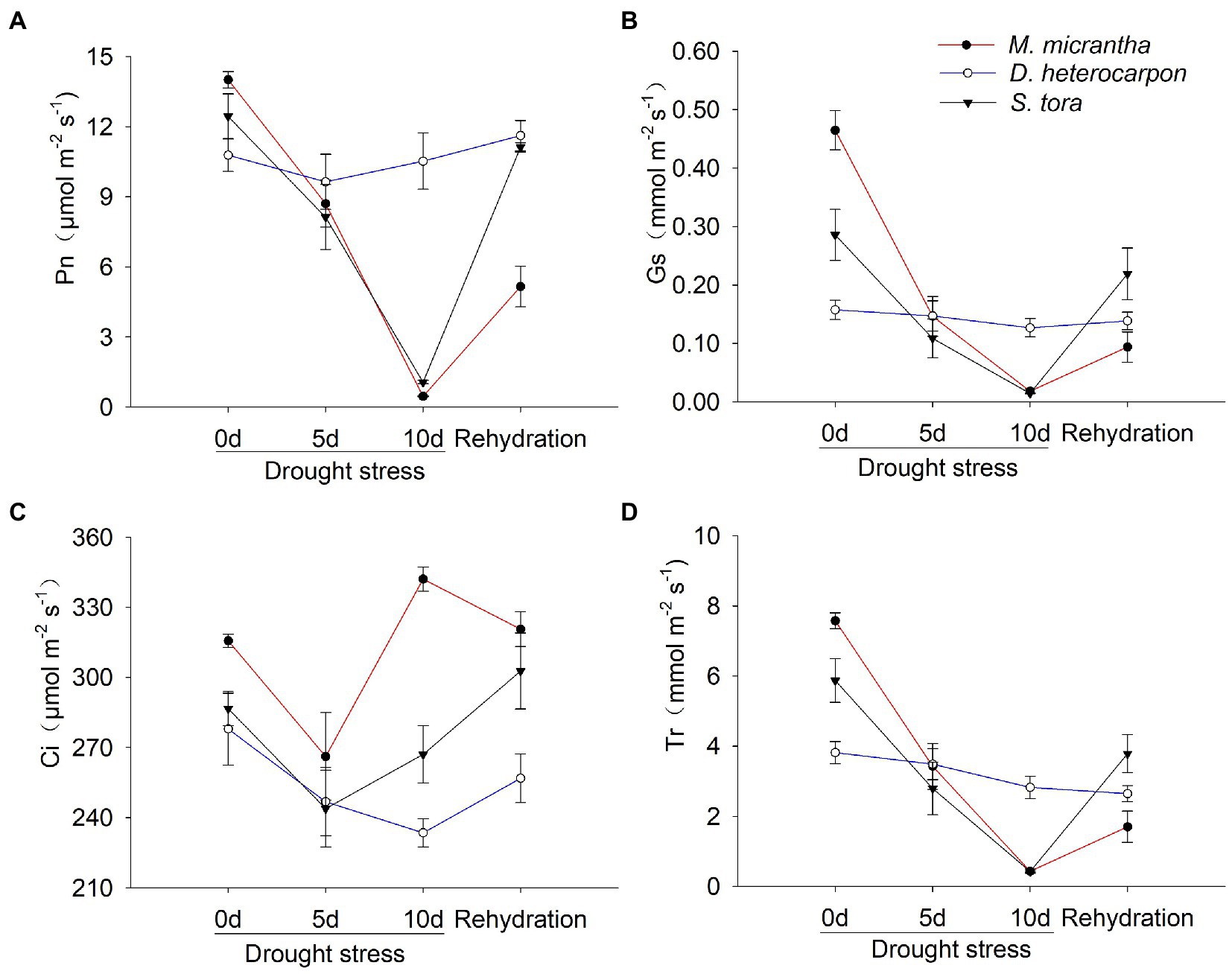
Figure 7. Changes of (A) net photosynthetic rate, (B) intercellular CO2 concentration, (C) stomatal conductance and (D) transpiration rate of two replacement forage legumes and M. micrantha under drought stress and after rehydration (average ± SE, n = 4).
The Fv/Fm of M. micrantha and S. tora showed a downward trend under drought treatment, which could not be recovered after rehydration, but continued to decline, while that of D. heterocarpon did not change and slightly increased after rehydration (Figure 8A). As drought treatment continued, ETR of M. micrantha decreased by 16%, and that of S. tora decreased by 26.6%, while ETR of D. heterocarpon increased by 6.5% (Figure 8B). After rehydration, the ETR of M. micrantha was unable to recover, while that of S. tora was partially recovered (Figure 8B). On the 10th day of drought treatment, the Yield of M. micrantha and S. tora decreased by 30.6 and 21.9%, respectively. After rehydration, the Yield of S. tora partially recovered, while that of M. micrantha could not recover to a level comparable to the well-watered group (Figure 8C). During the drought treatment, the Yield of D. heterocarpon was at a high level (Figure 8C). With the increase of drought treatment time, the qP of M. micrantha and S. tora decreased by 11.6 and 20.7%, respectively, on the 10th day and could be recovered to a level comparable to the untreated group after rehydration, while that of D. heterocarpon remained basically unchanged under all conditions (Figure 8D).
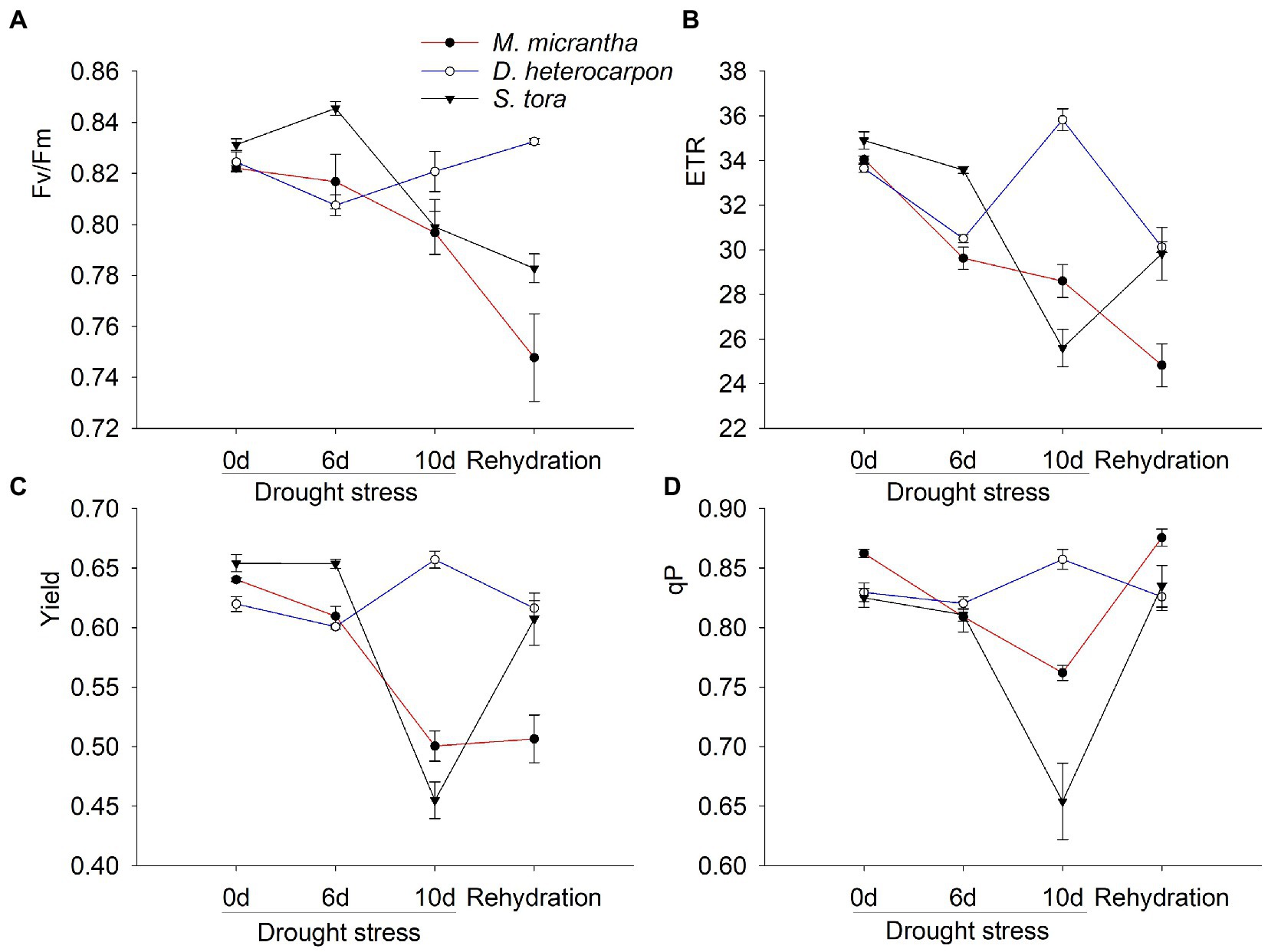
Figure 8. Changes of (A) maximal photochemical efficiency, (B) electron transfer rate, (C) quantum yield of photosystem II and (D) photochemical quenching of two replacement forage legumes and M. micrantha under drought-stressed treatment and after rehydration (average ± SE, n = 4).
Under the drought treatment, the proline content of M. micrantha, D. heterocarpon, and S. tora increased to varying degrees, and were significantly increased by 229.3, 0.96 and 47.7 times, respectively, compared with well-watered treatment (Figure 9A). After rehydration, the proline content of M. micrantha remained at a high level and both D. heterocarpon and S. tora recovered to a level comparable to the well-watered group (Figure 9A). Under drought treatment, the soluble sugar content of M. micrantha, D. heterocarpon, and S. tora increased significantly by 72.4, 28.9, and 31% respectively, compared with the well-watered treatment. The soluble sugar content of all three plants recovered to a level comparable to the well-watered group after rehydration (Figure 9B).
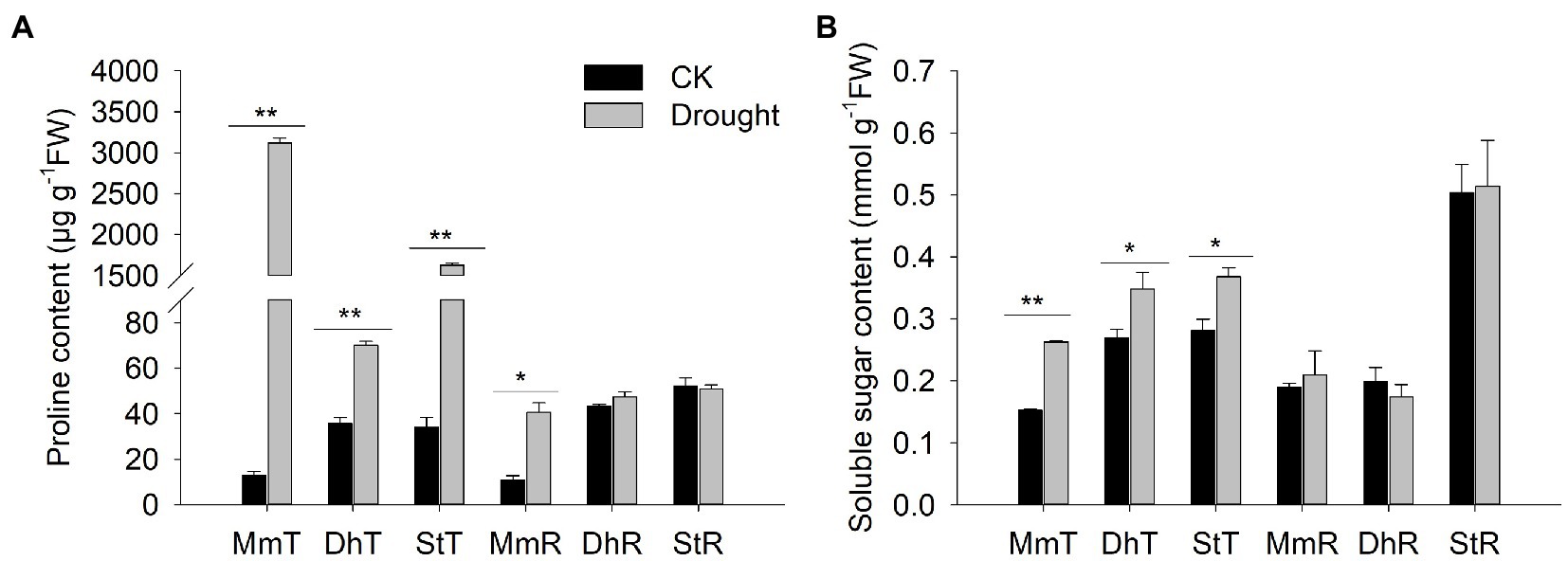
Figure 9. Changes of (A) proline and (B) soluble sugar content of two replacement forage legumes and M. micrantha under drought stress and rehydration (average ± SE, n = 3). Different letters above bar plots indicate significant differences analyzed using ANOVA followed by Dunnett’s test. Significance levels: ** p < 0.01; * p < 0.05.
4. Discussion
Much work so far has focused on the ecological mechanism of why biological invasion could be successful (Hengeveld, 1988; van der Putten et al., 2007; Rejmánek, 2014; Olenin et al., 2017). However, rather than understanding the mechanism of the invasion, it might be more important to apply the mechanism to the actual restoration and management of invaded sites. We provided experimental evidence that replacement control of invasive plants, through selecting and cultivating suitable native plants, is effective. In the field experiment, we selected 4 replacement plants that commonly appear in orchard habitats (i.e., D. heterocarpon, S. tora, S. latifolia, and A. conyzoides) and screened out two potentially effective plants among these four plants, D. heterocarpon and S. tora, for further experiments to investigate the potential in controlling M. micrantha. One of the most important reasons for the successful replacement of invasive plants by replacement plants is the interspecific competitive advantage of replacement plants (Gaudent and Keddy, 1988; Keddy et al., 2002). Species with greater competitiveness can inhibit the growth of invasive plants and replace them, thereby enabling sustainable restoration of damaged soil ecosystems (Chen et al., 2017). We investigated the effect of interspecific competition on the replacement effect of two forage legumes, D. heterocarpon and S. tora in mixed cultivation with M. micrantha. Both D. heterocarpon and S. tora significantly reduced the coverage of M. micrantha and suppress the growth of M. micrantha. At the same time, the total leaf area per plant and the total biomass of M. micrantha were also significantly reduced when mixed cultivation, indicating that D. heterocarpon and S. tora had great potential in controlling the expansion of M. micrantha. RII is one of the important indicators to measure the competition strength of plants (Ulrich and Perkins, 2014). RII of M. micrantha grown in the presence of D. heterocarpon [RIIMm(Dh)] was −0.42, while that of M. micrantha grown in the presence of S. tora [RIIMm(St)] was −0.13, indicating that these two species have a more competitive advantage compared to the alien species. In addition, we observed that RIIMm(Dh) was significantly smaller than RIIMm(St), indicating that D. heterocarpon was more effective in controlling M. micrantha than S. tora. The question we were trying to answer in this study is why D. heterocarpon and S. tora are so effective in suppressing the expansion of M. micrantha in the field experiment. The field competition experiment was carried out in an orchard habitat with low soil moisture content and some degree of light blocking. Therefore, we simulated low-light and drought-stressed environmental conditions and compared the growth and photosynthetic characteristics of these two plants with M. micrantha in order to study the physiological and ecological mechanism of the success replacement controlling effect.
M. micrantha is a tropical heliophytic plant (Han et al., 2017; Sheam et al., 2020), and the biomass of M. micrantha decreased significantly with the decrease in light intensity after low-light treatment. On one hand, the AQY of the M. micrantha significantly decreased under low-light condition, illustrating that the amount of the light-harvesting pigment–protein complexes which absorbs sunlight and transforms light energy into chemical energy might be reduced, resulting in a decrease of photosynthetic rate and a consequent weakening of the ability to utilize weak light (Richardson and Berlyn, 2002). On the other hand, the dark respiration rate of M. micrantha was maintained at a high level, while the photosynthetic rate decreased, leading to a proportional reduction in biomass accumulation. However, under low-light treatment, the dark respiration rate of D. heterocarpon and S. tora were significantly decreased, indicating that the replacement plants were able to adapt to the low-light environment by reducing energy consumption and maintaining a higher photosynthetic rate (Gyimah and Nakao, 2007; Martínez Pastur et al., 2007). Fv/Fm reflects the maximum light energy conversion efficiency of PSII, which tends to decrease significantly when plants are stressed (Guo and Tan, 2014). ETR reflects the activity of PSII and is directly related to plant photosynthetic rate (Koblížek et al., 2001; Burda, 2007; Yamori et al., 2008), and Yield is also positively correlated with the activity of PSII (Krall and Edwards, 1992). Fv/Fm, ETR, Yield, and qp of the replacement plants under low-light treatment did not change significantly compared with those values under light 100%, while those of M. micrantha decreased to different degrees after low-light treatment. Therefore, D. heterocarpon and S. tora show good adaptability and strong shade tolerance under low-light environment so that they can quickly grow and cover the soil surface to rob most of the light.
Moisture is an important limiting factor during plant growth and development (Davies et al., 1990; Ghaderi and Siosemardeh, 2011). Soil moisture is a key factor in M. micrantha seed germination and population expansion, and determines the growth and distribution of M. micrantha (Yue et al., 2019). After drought treatment, we found that the biomass of M. micrantha decreased by 65% and the plant height and root also decreased by 38.7 and 32%, respectively, compared to the well-watered group, while the replacement plants outperformed M. micrantha in all aspects of plant growth parameters. In addition, under drought-stressed condition, phenotype change of M. micrantha was the most serious, and the water loss was severe, so that M. micrantha needed to absorb more water from the soil for supplementation, resulting in serious soil water loss and soil compaction, which was difficult to restore soil nutrient supply and return to original soil status (Demchik and Sharpe, 2000; Ober and Parry, 2011; Kilpeläinen et al., 2017). A large number of studies have shown that photosynthesis is sensitive to water stress, and the photosynthetic rate decreases with the increase of water stress (Boyer, 1970). We observed that M. micrantha was irreversibly damaged after 10 days of drought treatment, and all photosynthetic indexes of M. micrantha failed to recover after rehydration. Chlorophyll fluorescence parameters can detect the effects of drought stress on plant photosynthesis (Bi et al., 2008; Su et al., 2015). Chlorophyll fluorescence parameters of M. micrantha revealed that drought stress caused irreversible damage to the PSII of M. micrantha. However, both the photosynthetic index and chlorophyll fluorescence parameters of S. tora recovered after rehydration, indicating that S. tora had strong self-repair potential in drought stress and showed resistance to drought stress (Georgieva et al., 2006). The performance of D. heterocarpon was better than that of S. tora, and its various indicators remained stable during drought stress, reflecting excellent adaptability and tolerance to drought stress. Among two replacement forage legumes and M. micrantha, D. heterocarpon was the most drought-resistant, while M. micrantha was the least drought-resistant and its growth was susceptible to water limitation. In addition, in response to drought stress, M. micrantha accumulated a large amount of proline, and after rehydration, the proline concentration remained high, indicating that M. micrantha is often difficult to recover after severe damage (Waldren and Teare, 1974; Patel and Vora, 1985; Carvalho et al., 2019). Nevertheless, the proline content of D. heterocarpon and S. tora recovered to a level comparable to the well-watered group after rehydration. The replacement plants show excellent adaptation to drought stress, suggesting that the use of D. heterocarpon and S. tora to control the spread of M. micrantha in dryland orchards has good application prospects.
Theoretical studies of plant interspecific competition have been the focus of research in the field of ecology, and the long-standing debate on explaining interspecific competition is between Philip Grime and David Tilman (Grime, 1977; Tilman, 1980). A series of previous studies tend to support one side (Jabot and Pottier, 2012; DeMalach et al., 2016). In our study, we found that the replacement plants can obtain sufficient nutrients to sustain themselves and grow rapidly in low-level resource environments, accumulate more above-ground and underground biomass, and more mineral nutrition, water, and sunlight, to eventually win in the competition against invasive plants, eliminate them, and achieve the ecological restoration of the invaded sites. The invasive plants often covered the soil surface quickly through rapid growth and excluded the native plants, which severely disrupted ecological balance and threatening biodiversity (Pyšek and Richardson, 2010; Oduor, 2013). When the resource was sufficient, the plant height and above-ground biomass of M. micrantha were significantly higher than those of D. heterocarpon and S. tora, indicating that these two legumes are not better than M. micrantha in terms of growth rate during the same time period. The key to the legume’s victory over M. micrantha in the field was not its rapid growth, which was not in line with Grime’s theory. However, with the reduction of light resources and the lack of water, the difference between the plant heights of D. heterocarpon, S. tora, and M. micrantha has narrowed, while the above-ground biomass of D. heterocarpon has successfully surpassed M. micrantha under low light and water shortage. The above-ground biomass of S. tora also surpassed that of M. micrantha under drought stress. It can be seen that low light and drought were the environmental constraints that can significantly reduce the above-ground biomass of M. micrantha. When the light and water resources were limited, the two legumes have higher resource utilization and smaller resource requirements. The limited resources can be fully utilized, thereby accumulating more above-ground biomass and ultimately winning the competition. This is consistent with the idea that “resource availability can affect competition” proposed by Carlyle et al. (2010). Therefore, we propose that the reason why D. heterocarpon and S. tora are more competitive than M. micrantha may be that they have smaller resource requirements and can survive in the absence of light and water resources, which is in line with Tilman’s theory of minimum resource requirements.
Because of the rapid growth and expansion of invasive plants, environmental conditions and resources are particularly important to them and also tend to be limiting conditions. The findings of this study mean that in the future, people can control one or more resources that restrict the rapid growth of invasive plants by analyzing the environmental resources of the invaded site, and screening out the native plants that can grow rapidly and cover the soil surface under stressed conditions and eventually the invasive plants can be replaced without leaving other ecological problems. The two-phase resource dynamics hypothesis states that resources appear as pulses rather than continuously, including two stages, one is pulse periods when the available resources are high and most plants can grow and accumulate resources; the other is interpulse periods when the available resources are too low for most plants to absorb, and most plants die due to insufficient resources (Goldberg and Novoplansky, 1997). Our experimental environment is the understory habitat of the orchard, the trees have a canopy effect on light, and soil moisture content is relatively low, which means that light resources and water resources are always in interpulse periods. Therefore, we infer that in the pulse period, the resources can meet the growth of the invasive plant M. micrantha, and once transferred to the interpulse period, the replacement plants can survive and grow well, and M. micrantha was eventually eliminated because it was at a disadvantage in the competition for resources (Goldberg et al., 2017).
5. Conclusion
In this study, we selected two plants with practical application value for replacement control, which is a potential method to prevent invasive weeds M. micrantha from regeneration. D. heterocarpon and S. tora have strong competitive advantage over M. micrantha, mainly due to their strong shade tolerance and drought tolerance. However, considering that S. tora is an alien species, it is hoped that the sustainable ecological management of M. micrantha in the dryland orchard will be achieved by using D. heterocarpon, a native legume, as a competitive substitute for M. micrantha. Our study revealed the physiological and ecological mechanism of using replacement plants to control biological invasion, and provide a new method to solve the problem of alien invasive weeds. Based on Tilman’s theory of minimum resource requirements, native plants with higher resource utilization and smaller resource requirements (e.g., shade tolerance and drought tolerance) are potential species for controlling M. micrantha in dryland orchard habitats.
Data availability statement
The raw data supporting the conclusions of this article will be made available by the authors, without undue reservation.
Author contributions
WL designed the study. PJ, JW, HL, Z-hW, and FL performed the experiments and analyzed the data. PJ and WL wrote the first draft of the manuscript and contributed substantially to revisions. All authors contributed to the article and approved the submitted version.
Funding
This study was financially supported by the National Natural Science Foundation of China (no. 32172430), the Natural Science Foundation of Guangdong Province of China (no. 2022A1515011942), and the Guangdong Basic and Applied Basic Research Foundation (no. 2021B1515120039).
Conflict of interest
The authors declare that the research was conducted in the absence of any commercial or financial relationships that could be construed as a potential conflict of interest.
Publisher’s note
All claims expressed in this article are solely those of the authors and do not necessarily represent those of their affiliated organizations, or those of the publisher, the editors and the reviewers. Any product that may be evaluated in this article, or claim that may be made by its manufacturer, is not guaranteed or endorsed by the publisher.
References
Barreto, R. W., and Evans, H. C. (1995). The mycobiota of the weed Mikania micrantha in southern Brazil with particular reference to fungal pathogens for biological control. Mycol. Prog. 99, 343–352. doi: 10.1016/S0953-7562(09)80911-8
Bi, J. J., Liu, J. D., Ye, B. X., and Xie, L. J. (2008). Effects of drought stress on photosynthesis and chlorophyll fluorescence of the summer maize leaf. Meteorol. Environ. Sci. 31, 10–15. doi: 10.16765/j.cnki.1673-7148.2008.01.018
Boyer, J. S. (1970). Differing sensitivity of photosynthesis to low leaf water potentials in corn and soybean. Plant Physiol. 46, 236–239. doi: 10.1104/pp.46.2.236
Burda, K. (2007). Dynamics of electron transfer in photosystem II. Cell Biochem. Biophys. 47, 271–284. doi: 10.1007/s12013-007-0011-z
Carlyle, C. N., Fraser, L. H., and Turkington, R. (2010). Using three pairs of competitive indices to test for changes in plant competition under different resource and disturbance levels. J. Veg. Sci. 21, 1025–1034. doi: 10.1111/j.1654-1103.2010.01207.x
Carvalho, M., Castro, I., Moutinho-Pereira, J., Correia, C., Egea-Cortines, M., Matos, M., et al. (2019). Evaluating stress responses in cowpea under drought stress. J. Plant Physiol. 241:153001. doi: 10.1016/j.jplph.2019.153001
Chen, C., Huang, D., Wang, Q., Wu, J., and Wang, K. (2016). Invasions by alien plant species of the agro-pastoral ecotone in northern China: species-specific and environmental determinants. J. Nat. Conserv. 34, 133–144. doi: 10.1016/j.jnc.2016.10.004
Chen, B. M., Liao, H. X., Chen, W. B., Wei, H. J., and Peng, S. L. (2017). Role of allelopathy in plant invasion and control of invasive plants. Allelop. J. 41, 155–166. doi: 10.26651/2017-41-2-1092
Choudhury, M. R., Deb, P., Singha, H., Chakdar, B., and Medhi, M. (2016). Predicting the probable distribution and threat of invasive Mimosa diplotricha Suavalle and Mikania micrantha Kunth in a protected tropical grassland. Ecol. Eng. 97, 23–31. doi: 10.1016/j.ecoleng.2016.07.018
Courchamp, F., Fournier, A., Bellard, C., Bertelsmeier, C., Bonnaud, E., Jeschke, J. M., et al. (2017). Invasion biology: specific problems and possible solutions. Trends Ecol. Evol. 32, 13–22. doi: 10.1016/j.tree.2016.11.001
Davies, W. J., Mansfield, T. A., and Hetherington, A. M. (1990). Sensing of soil water status and the regulation of plant growth and development. Plant Cell Environ. 13, 709–719. doi: 10.1111/j.1365-3040.1990.tb01085.x
Day, M. D., Clements, D. R., Gile, C., Senaratne, W. K. A. D., Shen, S., Weston, L. A., et al. (2016). Biology and impacts of pacific islands invasive species. 13. Mikania micrantha Kunth (Asteraceae). Pac. Sci. 70, 257–285. doi: 10.2984/70.3.1
Day, M. D., Kawi, A. P., and Ellison, C. A. (2013). Assessing the potential of the rust fungus Puccinia spegazzinii as a classical biological control agent for the invasive weed Mikania micrantha in Papua New Guinea. Biol. Control 67, 253–261. doi: 10.1016/j.biocontrol.2013.08.007
Day, M. D., Kawi, A., Kurika, K., Dewhurst, C. F., Waisale, S., Saul-Maora, J., et al. (2012). Mikania micrantha Kunth (Asteraceae) (mile–a–minute): its distribution and physical and socioeconomic impacts in Papua New Guinea. Pac. Sci. 66, 213–223. doi: 10.2984/66.2.8
DeMalach, N., Zaady, E., Weiner, J., and Kadmon, R. (2016). Size asymmetry of resource competition and the structure of plant communities. J. Ecol. 104, 899–910. doi: 10.1111/1365-2745.12557
Demchik, M. C., and Sharpe, W. E. (2000). The effect of soil nutrition, soil acidity and drought on northern red oak (Quercus rubra L.) growth and nutrition on Pennsylvania sites with high and low red oak mortality. For. Ecol. Manag. 136, 199–207. doi: 10.1016/S0378-1127(99)00307-2
Feng, Y. L., Cao, K. F., and Zhang, J. L. (2004). Photosynthetic characteristics, dark respiration, and leaf mass per unit area in seedlings of four tropical tree species grown under three irradiances. Photosynthetica 42, 431–437. doi: 10.1023/B:PHOT.0000046163.83729.e5
Gaudent, C. L., and Keddy, P. A. (1988). Comparative approach to predicting competitive ability from plant traits. Nature 334, 242–243. doi: 10.1038/334242a0
Georgieva, K., Szigeti, Z., Sarvari, E., Gaspar, L., Maslenkova, L., Peeva, V., et al. (2006). Photosynthetic activity of homoiochlorophyllous desiccation tolerant plant Haberlea rhodopensis during dehydration and rehydration. Planta 225, 955–964. doi: 10.1007/s00425-006-0396-8
Ghaderi, N., and Siosemardeh, A. (2011). Response to drought stress of two strawberry cultivars (cv. Kurdistan and Selva). Hortic. Environ. Biotechnol. 52, 6–12. doi: 10.1007/s13580-011-0019-6
Goldberg, D. E., Martina, J. P., Elgersma, K. J., and Currie, W. S. (2017). Plant size and competitive dynamics along nutrient gradients. Am. Nat. 190, 229–243. doi: 10.1086/692438
Goldberg, D., and Novoplansky, A. (1997). On the relative importance of competition in unproductive environments. J. Ecol. 85, 409–418. doi: 10.2307/2960565
Grime, J. P. (1977). Evidence for the existence of three primary strategies in plants and its relevance to ecological and evolutionary theory. Am. Nat. 111, 1169–1194. doi: 10.1086/283244
Guo, Y., and Tan, J. (2014). Recent advances in the application of chlorophylla fluorescence from photosystem II. Photochem. Photobiol. 91, 1–14. doi: 10.1111/php.12362
Gyimah, R., and Nakao, T. (2007). Early growth and photosynthetic responses to light in seedlings of three tropical species differing in successional strategies. New For (Dordr). 33, 217–236. doi: 10.1007/s11056-006-9028-1
Han, S., Li, Z., Xu, Q., and Zhang, L. (2017). “Mile-a-minute weed Mikania micrantha Kunth,” in Biological invasions and its Management in China. Vol. 2 eds. F. Wan, M. Jiang, and A. Zhan (Singapore: Springer), 131–141.
Hengeveld, R. (1988). Mechanisms of biological invasions. J. Biogeogr. 15, 819–828. doi: 10.2307/2845342
Islam, F., Wang, J., Farooq, M. A., Khan, M. S. S., Xu, L., Zhu, J., et al. (2018). Potential impact of the herbicide 2,4-dichlorophenoxyacetic acid on human and ecosystems. Environ. Int. 111, 332–351. doi: 10.1016/j.envint.2017.10.020
Jabot, F., and Pottier, J. (2012). A general modelling framework for resource-ratio and CSR theories of plant community dynamics. J. Ecol. 100, 1296–1302. doi: 10.1111/j.1365-2745.2012.02024.x
Kaur, R., Malhotra, S., and Inderjit, (2012). Effects of invasion of Mikania micrantha on germination of rice seedlings, plant richness, chemical properties and respiration of soil. Biol. Fertil. Soils 48, 481–488. doi: 10.1007/s00374-011-0645-2
Keddy, P., Nielsen, K., Weiher, E., and Lawson, R. (2002). Relative competitive performance of 63 species of terrestrial herbaceous plants. J. Veg. Sci. 13, 5–16. doi: 10.1111/j.1654-1103.2002.tb02018.x
Kilpeläinen, J., Barbero-López, A., Vestberg, M., Heiskanen, J., and Lehto, T. (2017). Does severe soil drought have after-effects on arbuscular and ectomycorrhizal root colonisation and plant nutrition? Plant Soil 418, 377–386. doi: 10.1007/s11104-017-3308-8
Kimball, S., Lulow, M. E., Mooney, K. A., and Sorenson, Q. M. (2014). Establishment and Management of Native Functional Groups in restoration. Restor. Ecol. 22, 81–88. doi: 10.1111/rec.12022
Koblížek, M., Kaftan, D., and Nedbal, L. (2001). On the relationship between the non-photochemical quenching of the chlorophyll fluorescence and the photosystem II light harvesting efficiency. A repetitive flash fluorescence induction study. Photosynth. Res. 68, 141–152. doi: 10.1023/A:1011830015167
Krall, J. P., and Edwards, G. E. (1992). Relationship between photosystem II activity and CO2 fixation in leaves. Physiol. Plant. 86, 180–187. doi: 10.1111/j.1399-3054.1992.tb01328.x
Li, Z., Han, S., Guo, M., Luo, L. W., Peng, T., et al. (2004). Biology and host specificity of Actinote anteas, a biocontrol agent for controlling Mikania micrantha. Chin. J. Biol. Control 20:170. doi: 10.16409/j.cnki.2095-039x.2004.03.006
Li, F. L., Li, M. G., Zan, Q. J., Guo, Q., Zhang, W. Y., Wu, Z., et al. (2012). Effects of the residues of Cuscuta campestris and Mikania micrantha on subsequent plant germination and early growth. J. Integr. Agric. 11, 1852–1860. doi: 10.1016/S2095-3119(12)60190-7
Li, M., Lu, E., Guo, Q., Zan, Q., Wei, P., Jiang, L., et al. (2012). Evaluation of the controlling methods and strategies for Mikania micrantha H. B. K. Acta Ecol. Sin. 32, 3240–3251. doi: 10.5846/STXB201104090460
Li, W., Luo, J., Tian, X., Soon Chow, W., Sun, Z., Zhang, T., et al. (2015). A new strategy for controlling invasive weeds: selecting valuable native plants to defeat them. Sci. Rep. 5:11004. doi: 10.1038/srep11004
Macanawai, A., Day, M., Tumaneng-Diete, T., and Adkins, S. (2012). Impact of Mikania micrantha on crop production systems in Viti Levu. Fiji. Pak. J. Weed Sci. Res. 18, 357–365.
MacArthur, R., and Levins, R. (1964). Competition, habitat selection, and character displacement in a patchy environment. Proc. Natl. Acad. Sci. U. S. A. 51, 1207–1210. doi: 10.1073/pnas.51.6.1207
Martínez Pastur, G., Lencinas, M. V., Peri, P. L., and Arena, M. (2007). Photosynthetic plasticity of Nothofagus pumilio seedlings to light intensity and soil moisture. For. Ecol. Manag. 243, 274–282. doi: 10.1016/j.foreco.2007.03.034
Mohanty, S. S., and Jena, H. M. (2019). A systemic assessment of the environmental impacts and remediation strategies for chloroacetanilide herbicides. J. Water Process. Eng. 31:100860. doi: 10.1016/j.jwpe.2019.100860
Ober, E., and Parry, M. A. J. (2011). The molecular and physiological basis of nutrient use efficiency in crops. New York: John Wiley & Sons.
Oduor, A. M. O. (2013). Evolutionary responses of native plant species to invasive plants: a review. New Phytol. 200, 986–992. doi: 10.1111/nph.12429
Olenin, S., Gollasch, S., Lehtiniemi, M., Sapota, M., and Zaiko, A. (2017). Biological oceanography of the Baltic Sea. Dordrecht: Springer.
Patel, J. A., and Vora, A. B. (1985). Free proline accumulation in drought-stressed plants. Plant Soil 84, 427–429. doi: 10.1007/BF02275480
Piemeisel, R. L. (1954). Replacement control: changes in vegetation in relation to control of pests and diseases. Bot. Re. 20, 1–32. doi: 10.2307/4353509
Piemeisel, R. L., and Carsner, E. (1951). Replacement control and biological control. Science 113, 14–15. doi: 10.1126/science.113.2923.14
Pimentel, D., McNair, S., Janecka, J., Wightman, J., Simmonds, C., O’Connell, C., et al. (2001). Economic and environmental threats of alien plant, animal, and microbe invasions. Agri. Ecosyst. Environ. 84, 1–20. doi: 10.1016/S0167-8809(00)00178-X
Price, J. N., and Partel, M. (2013). Can limiting similarity increase invasion resistance? A meta-analysis of experimental studies. Oikos 122, 649–656. doi: 10.1111/j.1600-0706.2012.00121.x
Pyšek, P., and Richardson, D. M. (2010). Invasive species, environmental change and management, and health. Annu. Rev. Environ. Resour. 35, 25–55. doi: 10.1146/annurev-environ-033009-095548
Rejmánek, M. (2014). Biological invasions and ecological hypotheses. Trends Ecol. Evol. 29:435. doi: 10.1016/j.tree.2014.06.003
Richardson, A. D., and Berlyn, G. P. (2002). Spectral reflectance and photosynthetic properties of Betula papyrifera (Betulaceae) leaves along an elevational gradient on Mt. Mansfield, Vermont, United States. Am. J. Bot. 89, 88–94. doi: 10.3732/ajb.89.1.88
Scasta, J. D., Engle, D. M., Fuhlendorf, S. D., Redfearn, D. D., and Bidwell, T. G. (2015). Meta-analysis of exotic forages as invasive plants in complex multi-functioning landscapes. Invas. Plant Sci. Mana. 8, 292–306. doi: 10.1614/IPSM-D-14-00076.1
Sheam, M., Haque, Z., and Nain, Z. (2020). Towards the antimicrobial, therapeutic and invasive properties of Mikania micrantha Knuth: a brief overview. J. Adv. Biotechnol. Exp. Ther. 3, 92–101. doi: 10.5455/jabet.2020.d112
Shen, S. C., Xu, G. F., Clements, D. R., Jin, G. M., Chen, A. D., Zhang, F. D., et al. (2015). Suppression of the invasive plant mile-a-minute (Mikania micrantha) by local crop sweet potato (Ipomoea batatas) by means of higher growth rate and competition for soil nutrients. BMC Ecol. 15, 1–10. doi: 10.5061/dryad.vb1qv
Shen, S. C., Xu, G. F., Clements, D. R., Jin, G. M., Chen, A. D., Zhang, F. D., et al. (2016). Suppression of reproductive characteristics of the invasive plant Mikania micrantha by sweet potato competition. BMC Ecol. 16, 30–39. doi: 10.1186/s12898-016-0085-9
Slingenberg, A., Braat, L., Windt, H. V., Rademaekers, K., Eichler, L., and Turner, K. (2009). Study on understanding the causes of biodiversity loss and the policy assessment framework. Available at: http://ec.europa.eu/environment/enveco/biodiversity/pdf/causes_biodiv_loss.pdf
Song, Z., Wang, Z. H., Fan, Z. W., Zhang, R. H., Zhang, G. L., and Fu, W. D. (2020). Screening and control effects of different alternative plants on Mikania micrantha. Chinese J. Agrometeorol. 41, 24–33. doi: 10.3969/j.issn.1000-6362.2020.01.003
Su, L. Y., Dai, Z. W., Li, S. H., and Xin, H. P. (2015). A novel system for evaluating drought–cold tolerance of grapevines using chlorophyll fluorescence. BMC Plant Biol. 15:82. doi: 10.1186/s12870-015-0459-8
Swamy, P. S., and Ramakrishnan, P. S. (1987). Contribution of Mikania micrantha during secondary succession following slash-and-burn agriculture (jhum) in north-East India I. biomass, litterfall and productivity. For. Ecol. Manag. 22, 229–237. doi: 10.1016/0378-1127(87)90107-1
Theoharides, K. A., and Dukes, J. S. (2007). Plant invasion across space and time: factors affecting nonindigenous species success during four stages of invasion. New Phytol. 176, 256–273. doi: 10.1111/j.1469-8137.2007.02207.x
Tilman, D. (1980). Resources: a graphical-mechanistic approach to competition and predation. Am. Nat. 116, 362–393. doi: 10.1086/679066
Tilman, D. (1988). Plant strategies and the dynamics and the structure of plant communities. Vol. 26. United States: Princeton University Press.
Ulrich, E., and Perkins, L. (2014). Bromus inermis and Elymus canadensis but not Poa pratensis demonstrate strong competitive effects and all benefit from priority. Plant Ecol. 215, 1269–1275. doi: 10.1007/s11258-014-0385-0
van der Putten, W. H., Klironomos, J. N., and Wardle, D. A. (2007). Microbial ecology of biological invasions. ISME J. 1, 28–37. doi: 10.1038/ismej.2007.9
Waldren, R. P., and Teare, I. D. (1974). Free proline accumulation in drought-stressed plants under laboratory conditions. Plant Soil 40, 689–692. doi: 10.1007/BF00010525
Xu, G. F., Zhang, F. D., Li, T. L., Shen, S. C., and Zhang, Y. H. (2011). Effects of 5 species and planting density on Mikania micrantha H. B. K. growth and competitive traits. Ecol. Environ. Sci. 20, 798–804. doi: 10.16258/j.cnki.1674-5906(2011)05-0798-07
Yamori, W., Noguchi, K., Kashino, Y., and Terashima, I. (2008). The role of electron transport in determining the temperature dependence of the photosynthetic rate in spinach leaves grown at contrasting temperatures. Plant Cell Physiol. 49, 583–591. doi: 10.1093/pcp/pcn030
Yue, M. F., Yu, H. X., Li, W. H., Yin, A. G., Cui, Y., and Tian, X. S. (2019). Flooding with shallow water promotes the invasiveness of Mikania micrantha. Ecol. Evol. 9, 9177–9184. doi: 10.1002/ece3.5465
Zhang, L. Y., Ye, W. H., Cao, H. L., and Feng, H. L. (2004). Mikania micrantha H. B. K. in China – an overview. Weed Res. 44, 42–49. doi: 10.1111/j.1365-3180.2003.00371.x
Keywords: plant invasion, Mikania micrantha, Desmodium heterocarpon, replacement control, resource competition
Citation: Jia P, Wang J, Liang H, Wu Z-h, Li F and Li W (2022) Replacement control of Mikania micrantha in orchards and its eco-physiological mechanism. Front. Ecol. Evol. 10:1095946. doi: 10.3389/fevo.2022.1095946
Edited by:
Xiang Liu, Lanzhou University, ChinaReviewed by:
Mu Liu, Lanzhou University, ChinaYunquan Wang, Zhejiang Normal University, China
Chen Zhu, Nanjing Agricultural University, China
Copyright © 2022 Jia, Wang, Liang, Wu, Li and Li. This is an open-access article distributed under the terms of the Creative Commons Attribution License (CC BY). The use, distribution or reproduction in other forums is permitted, provided the original author(s) and the copyright owner(s) are credited and that the original publication in this journal is cited, in accordance with accepted academic practice. No use, distribution or reproduction is permitted which does not comply with these terms.
*Correspondence: Weihua Li, d2hsaUBzY251LmVkdS5jbg==
†These authors have contributed equally to this work and share first authorship