- 1Institute of Evolution, Centre for Ecological Research, Budapest, Hungary
- 2MTA-ELTE Theoretical Biology and Evolutionary Ecology Research Group, Budapest, Hungary
- 3Parmenides Foundation, Centre for the Conceptual Foundation of Science, Pullach im Isartal, Germany
- 4International Institute for Applied Systems Analysis (IIASA), Laxenburg, Austria
- 5Centre for Social Sciences, Budapest, Hungary
Metabolic cooperation is widespread, and it seems to be a ubiquitous and easily evolvable interaction in the microbial domain. Mutual metabolic cooperation, like syntrophy, is thought to have a crucial role in stabilizing interactions and communities, for example biofilms. Furthermore, cooperation is expected to feed back positively to the community under higher-level selection. In certain cases, cooperation can lead to a transition in individuality, when freely reproducing, unrelated entities (genes, microbes, etc.) irreversibly integrate to form a new evolutionary unit. The textbook example is endosymbiosis, prevalent among eukaryotes but virtually lacking among prokaryotes. Concerning the ubiquity of syntrophic microbial communities, it is intriguing why evolution has not lead to more transitions in individuality in the microbial domain. We set out to distinguish syntrophy-specific aspects of major transitions, to investigate why a transition in individuality within a syntrophic pair or community is so rare. We review the field of metabolic communities to identify potential evolutionary trajectories that may lead to a transition. Community properties, like joint metabolic capacity, functional profile, guild composition, assembly and interaction patterns are important concepts that may not only persist stably but according to thought-provoking theories, may provide the heritable information at a higher level of selection. We explore these ideas, relating to concepts of multilevel selection and of informational replication, to assess their relevance in the debate whether microbial communities may inherit community-level information or not.
Introduction
Metabolic-mediated interactions and cooperation are both extremely common and widespread among microbes. However, they do not necessarily and always come hand in hand. A metabolic interaction may not be cooperative (e.g., inhibition (Netzker et al., 2020)) and cooperation may not be metabolite-mediated (e.g., host providing services like transportation to non-motile partners (Fröstl and Overmann, 1998; Bronstein, 2015)). Unsurprisingly, metabolite-mediated cooperation dominates microbial interactions, especially among prokaryotes, and in communities such as biofilms. While such complex, multi-species microbial communities are prevalent in nature, dripping with leaked metabolites and extensive cooperation, they rarely (if ever) show higher level organization.
Chemical products (nutrients, amino acids (Mee et al., 2014), siderophores (Cordero et al., 2012), enzymes (Gore et al., 2009), antibiotic degrading factors (Yurtsev et al., 2013), electrons (Stams et al., 2006), signal molecules (Antonova and Hammer, 2011), etc.) are secreted actively or leaked inadvertently by microbes into the intercellular medium, often serving the benefit of the producer and other community members as publicly available goods (West et al., 2007b; Cohen et al., 2012; Kallus et al., 2017; D’Souza et al., 2018; Smith and Schuster, 2019; Evans et al., 2020; Fritts et al., 2021) both in natural and artificial environments (Shou et al., 2007; Cavaliere et al., 2017; McCarty and Ledesma-Amaro, 2019). Public goods, however, generate conflicts between microbes (Tarnita, 2017). Those exploiting the goods increase their benefit, usually at the expense of producers, that may lead to ecological or evolutionary instability (West et al., 2007b). Nevertheless, metabolically cooperating pairwise symbioses and complex communities are both widespread and stable in the microbial world (Pande and Kost, 2017).
A biological individual can stably reproduce because of its replicating genes and epigenetic development. Individuals are of prime importance of biology, but are not the sole subjects of selection and evolution. Traditionally, it was believed that selection happens mostly at the lower level (those of genes) and becomes rare at higher levels (individuals, populations, species) (Lewontin, 1970). However, according to the general approach ((Hull, 1980; Dawkins, 1982; Maynard Smith, 1987), etc.), the same selection process acts on any entity that can multiply, inherit properties and, occasionally, variations (called units of evolution (Maynard Smith, 1987)), be those naked genes, cells, transient groups or populations. Consequently, selection may act at multiple levels concurrently, of genes, cells, populations (Okasha, 2005). And it is only a quantitative matter how much information at a given level can be stably inherited from generation to generation. Transition theory (Maynard Smith and Szathmáry, 1995; Szathmáry and Maynard Smith, 1995) posits that units can traverse these levels. For example, independent cells may form transient associations to increase their overall success, and, ultimately, waive their individual replication for the greater good of the group. When such entities are irreversibly coupled so that none can replicate without the other, a bona fide new unit of evolution emerges and a transition in individuality is made (Estrela et al., 2016).
According to transitions theory, cooperation may be selected for at a higher level and could stabilize higher level evolutionary units, integrating individually reproducing cells (Szathmáry, 2015). Syntrophic microbial partnerships may strengthen to form obligate symbiosis. For example, most archaea live in obligate dependence of their syntrophic partners (Pande and Kost, 2017). But the interesting question is: can metabolic cooperation itself lead to a major transition in individuality (Maynard Smith and Szathmáry, 1995; Szathmáry and Maynard Smith, 1995)? In such a transition, previously free-living individuals evolve toward strong dependence so that they can only reproduce as part of an integrated collective (Michod and Nedelcu, 2003; West et al., 2015). Transitions theory is predominantly interested in how conflicting interests and cooperation may yield multiple levels of selection (Okasha, 2005) and collectives as new evolutionary units. For example, how microbes overcome their selfish interests to form a mutually dependent cooperative collective?
While evolution toward a transition increases the degree of symbiotic organismality (i.e., integration), the transition, ultimately, is characterized by strict vertical transmission and mutual dependence of partners (West et al., 2015; Estrela et al., 2016). The threshold separating these two phases is not well defined, and we usually are only aware of cases that have already concluded. Before such a transition, interspecies interactions are known to be context-dependent, shifting freely between parasitic, commensal or mutualistic (Bronstein, 1994; Chamberlain et al., 2014; Chomicki et al., 2020) as partners adapt to each other and to changes of the environment. The outcome depends on the net costs and benefits of association (Estrela et al., 2016). It is thus not clear how and why a metabolic partnership may stabilize under higher-level selection. We set out to understand whether microbial communities possess (have ever possessed or may acquire) the necessary properties to qualify as units of selection. We review the evolutionary trajectories of metabolically coupled (cross-feeding) microbial communities where selection may favor reciprocal cooperation (mutualism) and possibly entail dependence and integration. We ask whether and how such communities may become collectives, i.e., units of evolution and make a transition in individuality. We explore these trajectories from the general points of view of replicator theory and multilevel selection. This may help identifying microbial interactions that entail multilevel selection and may help recognizing how far particular communities have advanced on the path toward an irreversible transition in individuality, and ultimately provide predictions of evolutionary transitions in microbial systems.
Cooperation and Mutualism
Cooperation, in general, refers to an ecological interaction between any two individuals, conspecifics or not, where at least one enjoys a benefit (often cooperation is reserved for intra-species interactions, and mutualism for inter-species interactions). Cooperation, formally described, is a higher-order interaction among replicating entities (Szathmáry, 2013), for example species. It is an interaction where one species exerts a positive effect on the reproduction rate of another species. In other words, species A aids the reproduction of species B. In chemistry language, this is called cross-catalysis, and means that the overall growth of species A depends on the density of species B. This definition so far does not assume anything about costs payed and benefits received by A, or that there is any reward or reciprocation from B. Cooperation is an “action that is beneficial to the recipient regardless of its effect on the donor” (Sachs et al., 2004), that is, a cooperative act may or may not be costly (see later). Nevertheless, in the evolutionary context, paying to help other organisms will be selected against, unless there is also benefit for the cooperator, perhaps averaged over a longer timespan, multiple contexts, or kin (West et al., 2006). This condition ensures that trophic interactions, such as microbial predation (where the prey also benefits the predator in a density-dependent manner), are not categorized as cooperation.
If the benefit is reciprocated, the interaction is reciprocal cooperation, or mutualism (Bronstein, 2015). Cooperation can be nutrient mediated (e.g., exchanging metabolic components) or service type (e.g., transportational or protective) (Bronstein, 2015). Such reciprocity may not be apparent when looking at, e.g., metabolic interactions, as it often looks like exploitation (Sørensen et al., 2019). This is because benefits may realize in different forms and at different timescales, from nutritional and transportation to protection benefit [for more details see Zachar and Boza (2020)]. Symbiosis is when partners of different species live in a physical contact or integration in most or all of their life cycles for a prolonged time allowing evolutionary adaptations to take place. Symbiosis is not necessarily mediated by metabolites, nor is necessarily mutually beneficial (Bronstein, 2015). A special case of symbiosis, involving metabolic interactions is called cross-feeding and syntrophy.
How Microbes Cooperate: Syntrophy and Biofilms
Most of cooperation in the microbial world is dominantly mediated by metabolites (Fritts et al., 2021). Metabolite exchange by itself, however, does not induce cooperation: a metabolic interaction may or may not provide direct or indirect benefit for one or more party, producer included. If there is benefit for at least one partner, the metabolic interaction entails an (often implicit) catalytic aid (Figure 1D). Moreover, the benefit for the recipient depends on the product produced by the donor, which can be safely assumed to correlate with the density of the donor.
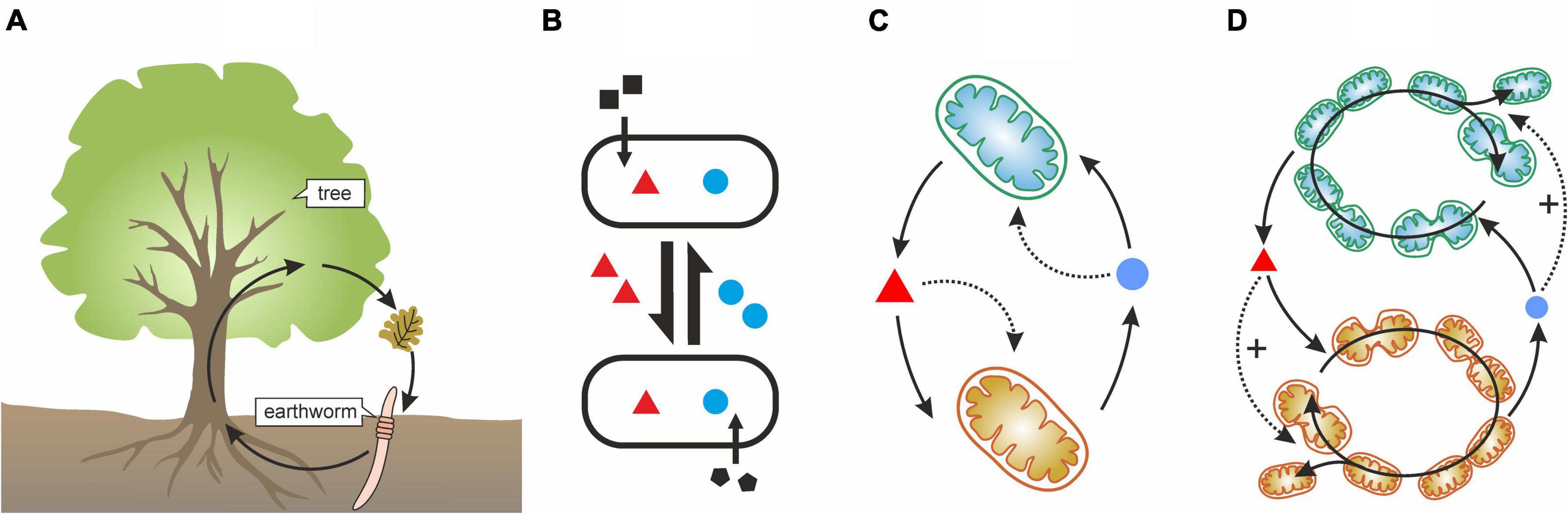
Figure 1. Different representations of ecological interactions. (A) An ecological hypercycle between two organisms [from Maynard Smith and Szathmáry (1995), p.58]. This example is deliberately confusing: without further specification, arrows could represent purely trophic interactions, cooperation, or both. It is also not immediately apparent if there is any auto or cross-catalysis going on. Clearly, a better representation is required to give a full account of the relevant interactions. (B) Bidirectional cooperative cross-feeding with reciprocal exchange of a costly metabolite (red triangle, blue disk) that benefits both partners, as depicted in D’Souza et al. (2018). Note, that neither the cost, nor the benefit (cooperative act) is obvious without additional information. As a matter of fact, arrows could simply indicate the transformation of matter without any cooperative aid. (C) A more accurate representation of a syntrophic interaction: normal arrows indicate production and consumption, dotted arrows indicate cooperative benefit (catalytic aid). (D) A more precise representation of syntrophy with reproduction made explicit: benefit is only cooperative if it enhances the growth of the receiver, that is, it feeds back positively on its reproduction.
Cross-feeding (or syntrophy) is a special case of microbial metabolic cooperation, relying on (accidentally or intentionally) externalized (by-) products. Historically, cross-feeding referred to microbial interactions involving transfer of molecules entailing enhanced growth of participant species (D’Souza et al., 2018). Often, definitions focus on the transfer of compounds (Morris et al., 2013), on enhanced growth in the presence of partners (Fritts et al., 2021), on mutualistic nutrient exchange (Searcy, 2002), on obligate dependence, without which none can grow (Libby et al., 2019), or on the possibility of enabling new resources and niches (Schink, 2002; Stams and Plugge, 2009; Libby et al., 2019). Others simply define syntrophy phenomenologically as an interaction involving leaked products that increase the carrying capacity (or growth) of one or more species1 (Jimenez and Scheuring, 2021). There is a rather diverse range of definitions in the literature (see D’Souza et al., 2018; Smith et al., 2019), rendering cross-feeding and syntrophy umbrella terms. Although different types of metabolic interactions rely on different mechanisms that imply different dynamics (see later), the literature in general fails to properly formalize, blurring fundamental differences between them. For the sake of simplicity, we will use syntrophy to denote a metabolite-mediated interaction that is beneficial at least to one party.
Syntrophy can form between phenotypically different or spatially displaced individuals of the same species (Liu et al., 2015), or between different species (Shou et al., 2007; Hillesland and Stahl, 2010; Goldford et al., 2018). The significance of syntrophy in ecosystem functioning and evolution is clear for three different reasons. First, syntrophy seems to be widespread, ubiquitous, and easily evolvable. As it turns out, microbes, especially archaea (Castelle et al., 2015), depend heavily on products of other prokaryotes due to syntrophic interactions, being potentially responsible for the unculturability of archaea (Pande and Kost, 2017) and bacteria (Staley and Konopka, 1985). The ubiquity of microbial auxotrophies (D’Souza et al., 2018) and metabolic cooperation indicate that it is easy to encounter complementary metabolisms among microbes, even without prior co-evolutionary history. Second, metabolic community, and especially biofilm, design and stability became one of the leading research areas of artificial microbiology and biotechnology (Hays et al., 2015; Libby et al., 2019; McCarty and Ledesma-Amaro, 2019). Third, our increasing understanding of the frequent metabolic dependencies of archaea on microbial partners (Pande and Kost, 2017) and the fact that there is extensive gene transfer between microbial community members (Gogarten et al., 2002; Madsen et al., 2012) have shifted mitochondrial origin hypotheses from early phagocytosis to metabolic syntrophy (Zachar and Szathmáry, 2017; López-García and Moreira, 2020). According to these, mitochondrial endosymbiosis has emerged from mutually beneficial syntrophy of an archaeon and a bacterium that may have evolved as part of a community rather than as separately living organisms (Martin et al., 2015; Spang et al., 2019; Imachi et al., 2020; López-García and Moreira, 2020). As such, syntrophy may have been the starting point of one of the most intriguing major evolutionary transitions, the emergence of eukaryotes.
According to Dennis Searcy, there are two major patterns of syntrophy, flow-through and recycle, and only the latter is capable of accumulating nutrients (Searcy, 2002). They depend on different mechanisms that likely entail different dynamics.
Flow-through or waste-removal: The product can accumulate and self-inhibit the producer, while the partner cannot grow without the product. Removing the waste helps both the receiver and the producer to grow. The benefit depends on the rates of production and removal. For example, H2 production in methanogenic communities and by endosymbionts (Fenchel and Finlay, 1991; Embley and Finlay, 1994; Lengeler et al., 1999; Fenchel, 2006; Nowack and Melkonian, 2010; Madigan et al., 2014), or proteobacterial ectosymbionts improving growth of aerobic protists by removing toxic photosynthesis waste (Hünken et al., 2008). A special case is the situation where “organisms combine their metabolic capabilities to catabolize a substrate that cannot be catabolized by either one of them alone” (Stams and Plugge, 2009). For example, methane formation and oxidation by syntrophic communities of archaea and bacteria where reducing equivalents are transferred in an interspecies electron transfer (Stams and Plugge, 2009). In obligately syntrophic communities, for the degradation of specific organic compounds and growth, both the archaeal and bacterial partners are essential, no one can degrade it alone. Regarding the origin of mitochondria, hydrogen hypothesis (Sousa et al., 2016) and organic acid syntrophy (John and Whatley, 1975; Searcy et al., 1978; Margulis, 1981) fits here.
Nutrient recycling: If a resource is limited in the environment, neither species can grow unless one can recycle it back to its initial state becoming available for consumption again. Species therefore depend on each other. For example, sulfur cycling between reducer heterotroph Sulfurospirillum and oxidizer photosynthetic Chlorobium (Wolfe and Pfennig, 1977), anaerobic ciliate Strombidium purpureum and endosymbiotic PNSB (Fenchel and Bernard, 1993a,b). In the mitochondriogenesis context, sulfide hypothesis (Searcy, 1992, 2014) and syntrophy hypothesis (López-García and Moreira, 2020) belong here. This type is especially important, if the cycled product is required only in catalytic amounts (such as enzymes) and is quickly regenerated. The benefit in such an interaction depends more on the rate of regeneration than on the rate of production, the former being presumably faster.
We must emphasize an important point here. Many interpretations and illustrations of the syntrophy literature is insufficient or downright misleading, suggesting that metabolite exchange automatically entails cooperation. However, this is not necessarily true. The conversion of metabolites and the cooperative benefit of these interactions cannot be represented with a single type of arrow in a simple interaction graph (Figure 1B). Both metabolite transformation and catalytic interactions (benefit) must be explicitly and differently represented to account for microbial cooperation (Figures 1C,D). The classical illustration of a tree and a worm forming a cooperative cycle of Figure 1A is misleading as it simultaneously depicts trophic and cooperative interactions between partners, without differentiating between them explicitly.
Cooperation, such as producing a shared metabolite, is a costly act that benefits the recipient, hence the fitness of the actors is decreased while the recipient’s is increased. It is easy to see that without additional mechanisms strategies that do not produce but enjoy the benefits (free-riders or cheaters) are expected to reproduce faster and overtake the population (West et al., 2006, 2007a; Nadell et al., 2009; Tarnita, 2017; Smith and Schuster, 2019). Consequently, extracellular materials may act as public goods, generating social conflicts between microbes (Tarnita, 2017). There is a subtle difference between public (or shared (Cavaliere et al., 2017)) and common goods. Public goods are non-rivalrous (one using it does not make it less valuable for others) while common resources are rivalrous (one using it diminishes its accessibility or value for others) (Kollock, 1998; Dionisio and Gordo, 2006). For example, the extracellular biofilm matrix or extracellular enzymes can be characterized as public goods, while extracellular nutrients or leaky macromolecules as common goods. From a game-theoretic point of view, cooperators contribute to the production of the public good (or refrain overconsuming the common resource), while defectors do not contribute to public good (or overexploit the commons). As a result, defector dominance results in the degradation of the collective resource (Smith and Schuster, 2019). For example, the presence of cheater strains in P. aeruginosa reduced the thickness and density and the population growth of the biofilm, as such strains do not contribute in extracellular factor production (public good), but enjoy the benefits (Popat et al., 2012). Analogously, a “wasteful” strain with a high rate of ATP production displaces a “prudent” strain with a high yield of ATP production when competing for an extracellular common resource, leading to a tragedy of the commons (MacLean, 2008). For more information on the challenges posed by free-riders in syntrophic communities (endosymbioses included), the Reader is referred to Zachar and Boza (2020), especially to section “Issues of syntrophic consortia.”
The most widespread of structured microbial communities are biofilms, in which microbes from all domains establish diverse social interactions (West et al., 2007b). Biofilm formation appears to be an ancient prokaryotic feature (Hall-Stoodley et al., 2004). Biofilms can appear on any surface, from hydrothermal vents and freshwater bodies through soil and leaves to the internals of multicellular hosts. They develop by aggregation exhibiting both temporal and structural succession and differentiation (Hall-Stoodley et al., 2004). As a result, recruitment of species, differential gene expression and development of phenotypes are ordered (Stoodley et al., 2002). Biofilms always produce an extracellular matrix, secreted by all or some members, and serves as the medium for various metabolic and facilitating processes. The matrix sticks cells together, providing protection against external hazards (e.g., grazing (Seiler et al., 2017)) but also forms an internal network to facilitate water flow and the exchange of resources like public goods (Stoodley et al., 2002). In multispecies biofilms, multispecies spore formation is not expected, thus they disperse by coordinated degradation of the matrix (Davies, 2011) or by fragmentation via single cells or clusters (Stoodley et al., 2001; Rumbaugh and Sauer, 2020). This imposes a serious bottleneck resulting in new colonies being subject to a severe founder effect (see later). Due to the structure of biofilms, interactions are localized and neighborhoods are stable for a prolonged time. In biofilms, but also in other forms of microbial communities, metabolic cross-feeding (sensu (D’Souza et al., 2018)) and syntrophic microbial partnerships are ubiquitous and diverse (Morris et al., 2013; Pande and Kost, 2017). Since most prokaryotic species cannot be cultured without partners (Staley and Konopka, 1985; Pande and Kost, 2017; Imachi et al., 2020), metabolic complementarity is expected to be common and stable in nature. Furthermore, since syntrophy is a form of cooperation, it is expected to stabilize larger communities too.
How Mutualism May Evolve in Microbial Communities
In general, ecosystems evolve toward increased productivity and higher metabolic efficiency, leading to decreased productivity/biomass ratio and tighter nutrient cycles (Odum, 1969; Loreau, 1998). Both within-cycle and between-cycle (in terms of material cycles) competition constitute selective forces that drive this process (Loreau, 1998). One would expect that natural selection, after sufficiently long time, eliminates inefficient types and maximizes energy fitness by optimizing the growth, reproduction, and survival rates, and the efficiency of energy production (Burger et al., 2021). There are, however, a few limiting factors and trade-offs (de Lorenzo et al., 2014; Cavaliere et al., 2017; Burger et al., 2021). First, the lack of sufficient genetic variation caused by the continual selection that reduces heritable variation and hence gradually slows down the evolution of improvements. Second, ecological compensation and limits to growth. As evolution increases efficiency considerably, such a population will start to grow faster and counteracting environmental (ecological) limitations, such as the carrying capacity or the increased appeal as a resource of a population with high biomass for predators, will become stronger impeding the initial growth advantage. Third, continual coevolution and the Red Queen mechanism. Since the overall biomass energy delivered by the net primary production is the ultimate limiting resource for life in the biosphere, there is a continual selection on all species to increase their shares (Burger et al., 2021). Due to the continuous ecological and evolutionary dynamics of species, and often also changing environmental conditions, evolving a best strategy in a certain biotic and abiotic environment can only give temporary advantage. The complex relationship between ecological interactions and coevolution of species leads to fluctuating levels of inefficiency at the species level, but higher efficiency at the ecosystem level (Burger et al., 2021). Such a scenery, however, allows for the enhancement of energy uptake by means of inter-species cooperative metabolic interactions.
Segregating conflicting or complimentary metabolic pathways into separate compartments (such as protocells and cells) and securing the increasingly efficient flow of metabolites between these compartments (i.e., syntrophy), either through a medium or through special structures (Mori et al., 2016; Fritts et al., 2021), offers a very powerful way to increase metabolic productivity (van Gestel et al., 2015; West and Cooper, 2016; Tsoi et al., 2018). Such pathway modularization, a community-level division of labor, can emerge under various conditions (de Lorenzo et al., 2014; Cooper and West, 2018), and relies on social interactions between partners.
Consequently, the emergence of metabolic division of labor is driven by trade-offs in unicellular organisms, and can be selected for in several contexts (Goel et al., 2012; Großkopf and Soyer, 2016; Stump and Klausmeier, 2016; Dragoš et al., 2018; Louca et al., 2018). For example, a trade-off between metabolic efficiency in terms of rate and yield in ATP-producing pathways is hypothesized (Pfeiffer et al., 2001; Helling, 2002; Gudelj et al., 2007), which tradeoffs materialize because of the presence of alternative pathways in ATP production and the related thermodynamic principles (Pfeiffer and Schuster, 2005). Evolutionary game theory-based analysis reveals that if such alternative pathways are available, evolution should favor the fast but inefficient energy-production strategy feeding on a shared primary resource. Once such “wasteful” strategy outcompetes others and becomes dominant, their waste product also accumulates in the medium. In theory, the ratios of wasteful strategies and strategies feeding on their waste products can reach an equilibrium (Doebeli, 2002; Pfeiffer and Bonhoeffer, 2004; Pfeiffer and Schuster, 2005), in general favoring the emergence of stable cross-feeding interactions (Hansen et al., 2007; Germerodt et al., 2016; Gudelj et al., 2016; Cavaliere et al., 2017; Stump et al., 2018).
Besides the rate-yield type of conflicts, many other forms of conflicts, trade-offs, or complementarities can serve as the driver for the spatial and chemical segregation of cellular processes leading to bacterial polymorphism and cooperation (Doebeli, 2002; Gudelj et al., 2007, 2010; de Lorenzo et al., 2014; Meijer et al., 2020). The main cellular biochemical conflicts include competition for intracellular resources (ATP, synthesis machinery, cellular space, etc.), incompatibility and inhibitory conflicts, and enzyme-specificity conflicts (Gudelj et al., 2010; Johnson et al., 2012; de Lorenzo et al., 2014). While some conflicts can be resolved intracellularly (de Lorenzo et al., 2014), inter-cellular arrangements are often more effective (Johnson et al., 2012). Principally, the power of symbiosis lies in the fact that genetic and metabolic machinery from very distantly related organisms can be brought together (Maynard Smith, 1991; O’Malley, 2015). The new unit now can be treated as a dramatic mutational change allowing for a wider range of adaptations. Such an effect may be less pronounced, but still holds for microbial syntrophy and cross-feeding interactions as well.
There is ample evidence that syntrophy and metabolic complementation indeed evolves under various conditions (D’Souza and Kost, 2016; Großkopf et al., 2016; Mori et al., 2016; Van Hoek and Merks, 2017; Kallus et al., 2017; Meijer et al., 2020), and significant metabolic synergy can be found even between randomly paired partners without co-evolutionary history but with certain metabolic deficiencies (Wintermute and Silver, 2010). For example, securing physical proximity (ecological integration) induce evolutionary processes strengthening reciprocal fitness feedbacks resulting in a stable mutual cross-feeding between autotrophs (Harcombe et al., 2018; Preussger et al., 2020), although there is also a chance that the strains break free of the initial by-product based partnership (Preussger et al., 2020).
The most commonly known mechanism driving the evolution of bacterial syntrophy is provided by the Black Queen hypothesis, stating that leaky public (or shared) goods and selection for small genome sizes drive the evolution of mutual dependency between the members of a consortium (Morris et al., 2012). The genome size is decreased as cellular economization to ensure faster growth; often referred to as the streamlining hypothesis (Giovannoni et al., 2014; Martínez-Cano et al., 2015). At the same time, genomic reduction can often be understood as a community-dependent adaptive event: the lost functionality can be compensated by other members still possessing the necessary genes and functionality that benefits the whole community via a commonly available public good (Morris et al., 2012; Martínez-Cano et al., 2015). Such an adaptive gene loss can result in a diversity of, pair-wise or networked, mutualistic interactions and could potentially trigger the evolution of more specific and intimate mutualisms (Morris et al., 2012, 2013; Estrela et al., 2015, 2016).
Determining the fitness benefits arising from such dependencies and unraveling the reversibility of such relationships is particularly crucial. In evolutionary theory, we can distinguish proximate and ultimate mutualistic dependency (De Mazancourt et al., 2005; Zug and Hammerstein, 2014; Chomicki et al., 2020). Although both can be measured by removing the partner and by comparing the performances before and after removal, the picture appears to be more complex and fuzzy. Firstly, measuring the benefits of mutualisms, either in mono- or in co-cultures, and choosing the most evident measure, such as biomass, yield, growth rate, resistance against external perturbations, etc. (including biotic or abiotic sources), and the time-horizon during which performance in monitored, remains a puzzling issue (Mitri and Foster, 2013; Chomicki et al., 2020). Secondly, there are examples in which removal of the partner results in a short-term decrease in performance, or can even be lethal, but this may be due to the shared long-term co-evolutionary history, rather than evolved interspecies cooperation (De Mazancourt et al., 2005). Such an evolved dependence may be the result of adjusting to the presence of a non-beneficial (neutral or harmful) partner from which there is no getting rid of, and after long evolutionary time, the removal of this partner can now be detrimental for the host (Jeon, 1995, 2004; Weinbauer, 2004; Fellous and Salvaudon, 2009; Mitri and Foster, 2013; Zug and Hammerstein, 2014; Weinersmith and Earley, 2016). But this does not mean that the host couldn’t perform well without the non-beneficial partner, only means that the observed genotype is not adopted to the absence of it (De Mazancourt et al., 2005). Compared to such an evolved dependence, in true ultimate mutualisms real benefits are associated with the partnership and indeed none could have performed as well without the other (De Mazancourt et al., 2005; Zug and Hammerstein, 2014; Chomicki et al., 2020). Note that true mutualism can also evolve from evolved dependence, as we see instances of antagonisms evolving into mutualistic symbiosis (Fellous and Salvaudon, 2009; Sachs et al., 2011, 2013; Chomicki et al., 2020).
In a cross-feeding microbial community, the metabolic performance, and therefore the fitness of each cell depends directly on a subset of community members (those in direct interaction with the focal cell, metabolically or otherwise), and indirectly on the connected community as a whole (Khandelwal et al., 2013). Although formally the interaction network consists of pairwise interactions of cells (Faust and Raes, 2012), it can also enable higher-order interactions that may play a critical stabilizing role in complex microbiomes (Levine et al., 2017). While the interactions are mostly cooperative (West et al., 2006, 2007a), a shared metabolism can also be antagonistic (Machado et al., 2021). There exist highly cooperative communities, typically composed of members with smaller genomes and a diversity of auxotrophies. But there are also highly competitive communities with larger genomes, overlapping nutritional requirements, and higher antimicrobial activity (Machado et al., 2021). It seems that competitive communities can better resist species invasion but not nutrient shift, whereas cooperative communities are more susceptible to species invasion but resilient to nutrient changes (Machado et al., 2021).
The division of metabolic labor may trigger niche emergence or construction by opening novel, more efficient, or previously inhibited pathways enabling new metabolic phenotypes (Pearman et al., 2008; Colwell and Rangel, 2009; Großkopf et al., 2016; Ponomarova et al., 2017; Gatti et al., 2018, 2020; San Roman and Wagner, 2018; Oña et al., 2021). At the same time, division of labor results in a social interaction that have fitness consequences for both the producer and the recipient and leads to collective functionality (Crespi, 2001; West et al., 2006; Ackermann, 2015; Hays et al., 2015). For example, in the H2-mediated syntrophic interactions in methanogens, the metabolism of the secondary degrader inhibits further growth unless the syntrophic partner consumes H2 (Cavaliere et al., 2017), hence growth is only possible under coexistence.
It has been demonstrated that the evolved members of a syntrophic consortia are fitter than the non-evolved ancestral strain and that the consortium itself demonstrates enhanced biomass productivity, often interwoven with reduced byproduct accumulation (Bernstein et al., 2012; D’Souza and Kost, 2016; Harcombe et al., 2018; Preussger et al., 2020; Yang et al., 2020). Furthermore, coevolution of obligate mutualist bacteria can result in a faster growth and increase in productivity (Hillesland and Stahl, 2010; Summers et al., 2010). Growth advantage (increased Darwinian fitness) due to division of metabolic labor can be measured relative to prototrophic wild-type cell cultures (Pande et al., 2013; Pande and Kost, 2017). Enhanced metabolic activity is another sign of the synergistic effect of cross-feeding compared to cultures in which all reactions take place within one organism (Martínez et al., 2016). Densely connected syntrophic networks may partly suppress competition within guilds whose members would be in strong competition with each other for a common resource otherwise (Goldford et al., 2018).
Naturally, the fitness effects (i.e., costs and benefits) of metabolic interactions are often context dependent (Bronstein, 1994; Chamberlain et al., 2014; Hoek et al., 2016; Zengler and Zaramela, 2018; Chomicki et al., 2020) and may shift back and forth on the mutualism-antagonism continuum (Drew et al., 2021). Factors, such as intermediate cell densities and medium level of spatial proximity (Kim et al., 2008; Bull and Harcombe, 2009; D’Souza, 2020), presence of other parties, cooperative or exploiter types, in the community (Harcombe et al., 2016; van Tatenhove-Pel et al., 2021), as well as resource availability are all expected to affect the net benefit (Drew et al., 2021).
How Major Transitions May Emerge in Microbial Communities
A central tenet of transition theory and multilevel selection is that cooperation of lower level units realize group-level synergies that can be selected for at a higher level, ultimately establishing a new, higher level unit of evolution, the group (Szathmáry, 2015). There are a multitude of multicellular microbial communities based on metabolite-based cooperative aid where related cells stay together after division or aggregate via signals. Staying together may lead to canonical multicellularity, simple or complex, of a single species as in, e.g., cyanobacteria or metazoa. There are several social microbial species that evolved such an aggregative multicellularity (Rainey and Rainey, 2003; West et al., 2007a; Boyle et al., 2013). For an overview of the different community types, see Table 1.
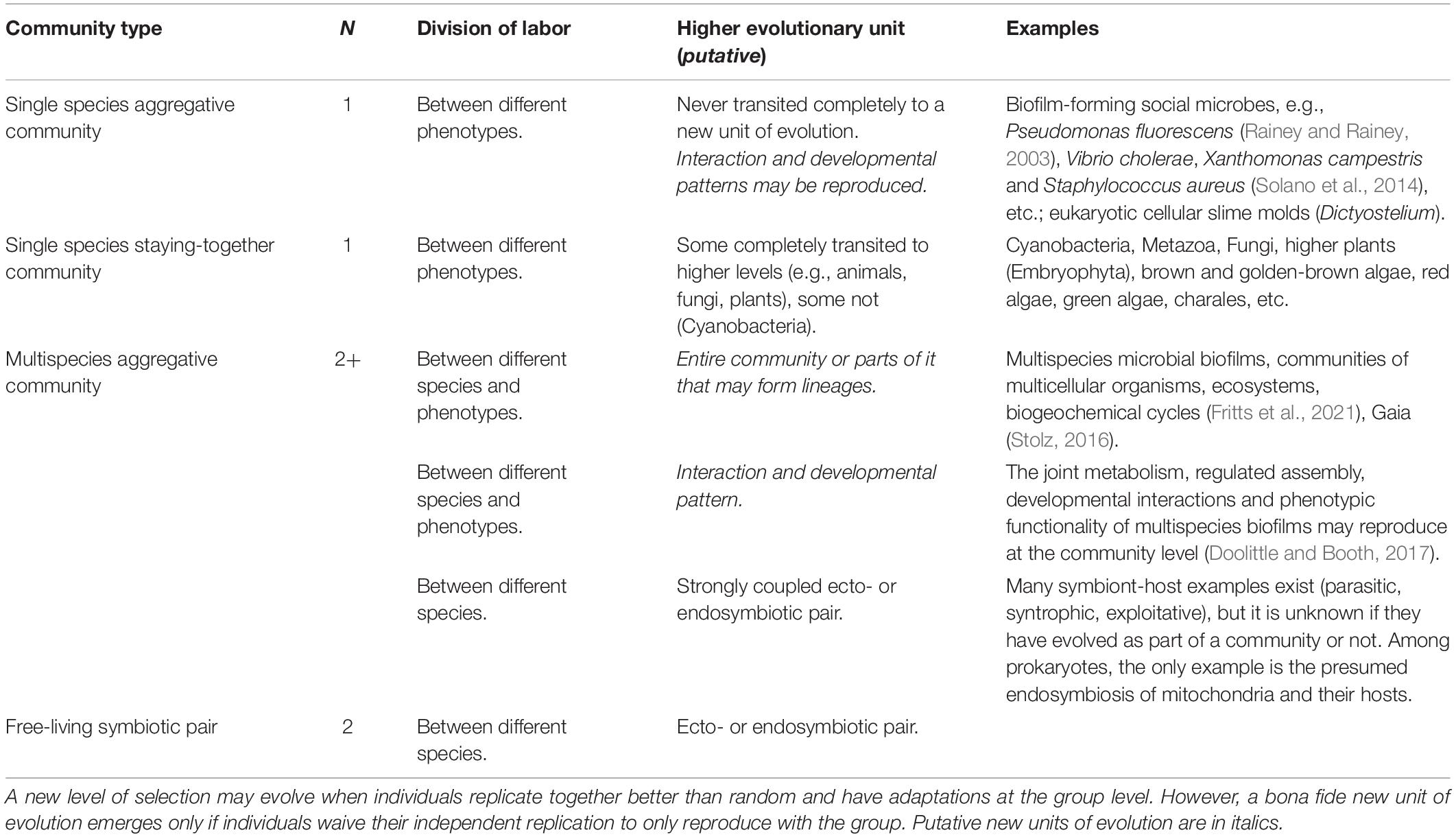
Table 1. Properties and examples of various types of community-forming and transitions in individuality.
However, there is a great difference between a single-(social) species and a multispecies community. Social multicellularity depends on the cooperative behavior of members, potentially having different phenotypes. Metazoa represent a typical case of fraternal major transition (Queller, 2000), where sister cells waived their reproductive ability for the common good of reproducing the multicellular body, with sophisticated epigenetic inheritance methods (Jablonka and Lamb, 1989, 1995). Interestingly, no multispecies microbial community seems to have ever made this transition and evolved to inherit epigenetic information, possibly because they involve cooperation of genetically unrelated partners which would require an egalitarian transition entailing conflicts that are hard to police. Here, we focus on evolutionary routes, that can potentially lead to higher level selection in communities, further integrating partners and may (at least theoretically) open a door to a transition in individuality (see Figures 2, 3). We set out to explore potential heritable information of multispecies communities.
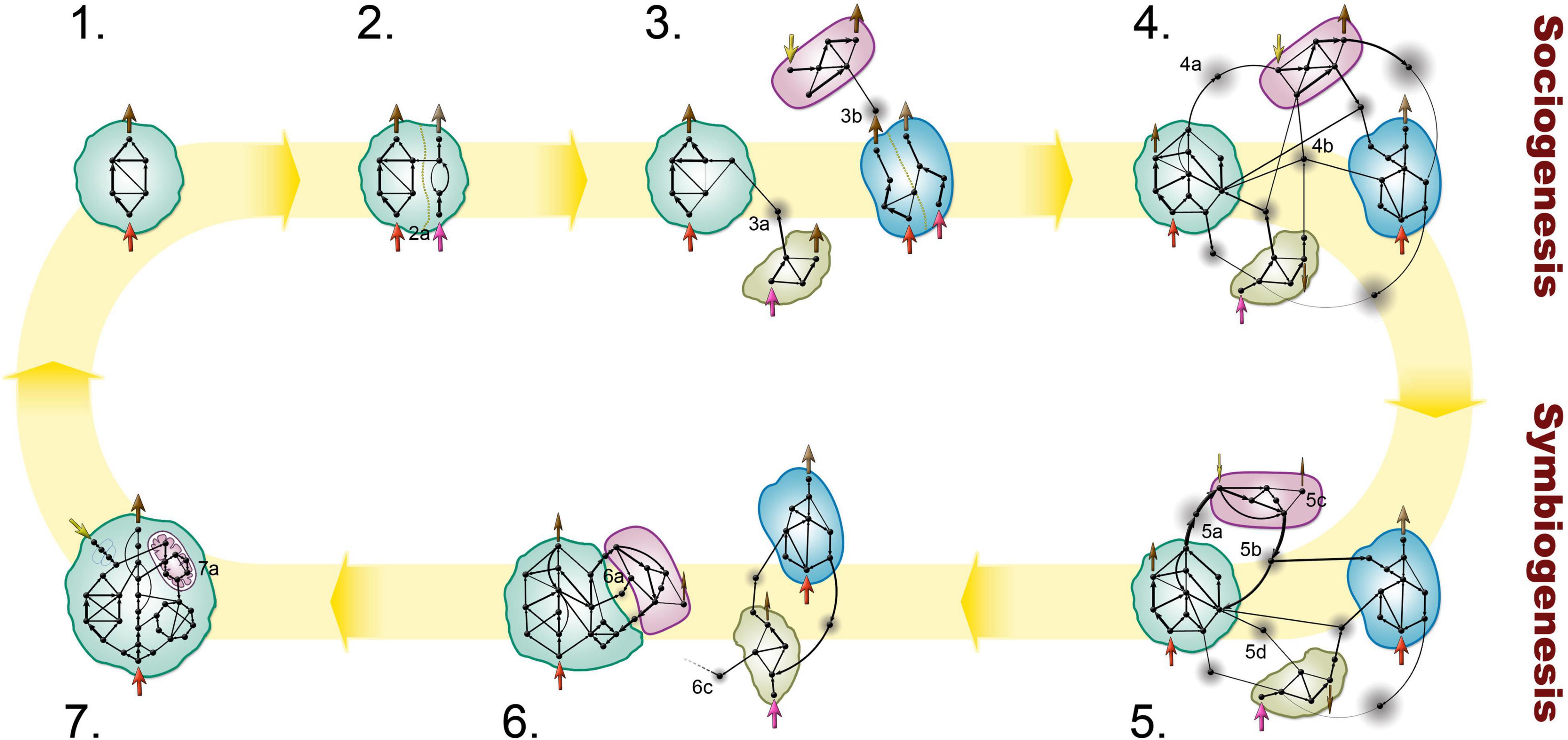
Figure 2. A theoretical scenario of the evolution of a microbial facilitation network. (1) Microbes (colored blobs) reproduce independently with self-sustaining metabolisms. Metabolism is represented as a network of metabolites (black dots) and reactions (black arrows) indicating the main directionality and weight of the metabolic flow. Inflow and outflow are depicted as arrows pointing in and out of cells, respectively. The yellow background cycle represents a possible evolutionary route; note that the route can branch or reverse at any point. (2) Phenotypic plasticity allows the organisms to adapt to environmental changes. E.g., mutually exclusive metabolic pathways within an individual (step 2, separated by a dotted line) can utilize different resources efficiently, but not both (differently colored inflows at 2a). (3) In a community, different species may specialize on a resource while relying on community members for the utilization of other resources. According to the Black Queen hypothesis, metabolic division of labor can evolve when the shared metabolism is partitioned and distributed among species that complement each other via diffusible, leaky products (gray nodes between cells indicate diffusion of released products; 3a, 3b). (4) Selection can further increase cross-dependency, resulting in a multi-context division of labor with several types of mutual dependencies (i.e., nutritional, protection). Public goods can be exclusively used by two species (4a), or by anyone in the community (4b). (5) Symbiosis may emerge if two organisms separate from the community by relying less on public goods (5d), by downregulating pathways relying on third party resources (5c), and strengthening reciprocal cooperation by increased production rates of compounds that benefit the partner (5a, 5b). Such compounds can still serve as public goods (5b) giving rise to free-riders. (6) Partners can secure a more efficient exchange to exclude free-riders by privatizing resources (6a). Symbiotic pairs can gradually decouple from the community (6c) which may still provide a stable environment for them. (7) The transition in individuality becomes complete when no partner can reproduce alone anymore (e.g., endosymbiont gets fully integrated; (7a). The new unit of evolution acts as a single individual, and the cycle may continue at step 1 (ignoring its higher internal complexity). Ecological and evolutionary timescales are not separated.
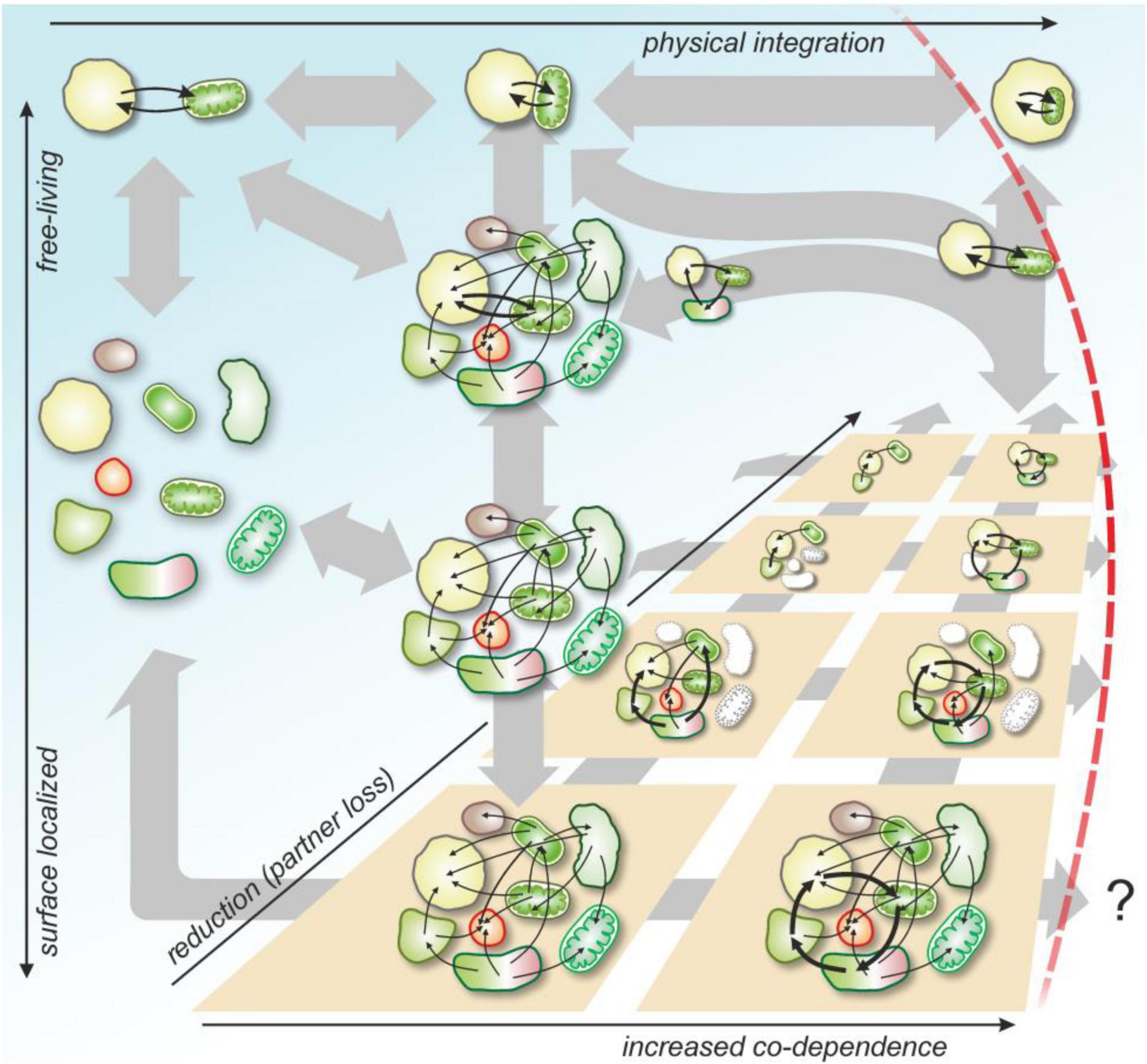
Figure 3. Possible routes for microbial communities to achieve transition in individuality. Communities may be either surface bounded (brown panels) or not (blue phase). Metabolic, cooperative, and inhibitive interactions evolve between members (for sake of simplicity, arrows denote unspecified positive ecological interactions). Certain interactions may strengthen, if selection favors them (thicker arrows), certain partners may be lost due to replacement, competition or instability (grayed out cells). A symbiotic pair may emerge from free-living cells, from a loosely coupled metabolic network or from a surface-bound biofilm. The organized spatiotemporal structure of biofilms bound to surfaces may facilitate co-dependence and the emergence of cooperative cycles (or pairs), that can be selected for at the biofilm level (if biofilms compete as lineages). A transition in individuality (red separator) happens when integration of the endosymbiont becomes irreversible and the pair can only reproduce together. Alternatively (and putatively), a biofilm (or any community) may evolve means to stably replicate as a group and waive the replication of its independent cells (question mark). Ecological and evolutionary timescales are not separated.
If there is a higher level of selection, there must be some form of population structure, which can facilitate cooperation (Nowak, 2006). Since cooperators sustain the structure (community, biofilm, group), they can spread better under selection. It would be informative to approach the problem at hand from a more abstract viewpoint. One may extend the concept of reciprocal cooperation of syntrophic microbes to more than two species. Accordingly, a metabolic community is a set of cooperative species, where cooperative help is mediated by produced public goods. The aid may chain (A helps B, B helps C) and form a network, that would stabilize member species. Ultimately, but not necessarily, a cycle may form (C helps A). It is formally called a hypercycle, a theoretical proposal to explain how (prebiotic) replicators could have coexisted via cross-catalysis (Eigen, 1971). Theoretically, such a cross-catalysis between organisms can itself be autocatalytic (multiply), and would feed back positively on all of the member species and on the collective itself. If so, it can be superior compared to linear (or non-) cooperative networks, as it can grow in numbers or compensate against loss via degradation, side reactions, mutations (Maynard Smith, 1979). If adaptive traits benefiting the collective emerge and are inherited, they can be selected for at the collective’s level. In the microbial domain, it means that adaptations that strengthen the group (via nonrandom recruitment, association and co-occurrence of partners) may accumulate and lead to the stabilization of the population structure. We emphasize that no hypercycle is known to exist in the microbial world. The real question is, can anything higher than species inherit changes in a real mutual microbial community? And can an evolutionary transition in individuality (West et al., 2015) happen? The answer is not trivial and is thoroughly debated.
Biofilm as Replicator
A long-standing debate of evolutionary biology is concerned with the “true” units of evolution (Williams, 1966; Wilson, 1975; Maynard Smith, 1987). A particularly interesting case is about the potential reproduction of multispecies microbial metabolic communities, namely, biofilms. According to some, biofilms are true reproducing units of evolution on their own right (Ereshefsky and Pedroso, 2012, 2015; Doolittle, 2013), while others maintain that they lack the necessary mechanisms and inheritance pathway to stably pass on information (Nadell et al., 2009; Clarke, 2016), e.g., changes in biofilm composition akin to a mutation in the genetic code.
Multiple arguments seem to support biofilms as units of evolution. Members exhibit coordinated activity (Davies, 2011; Lyons and Kolter, 2015), and cooperate in a reciprocal and network-like topology. They make costly investments that are beneficial for the producer and for others too (Jimenez and Scheuring, 2021). Benefits may be realized at the collective’s level (e.g., extracellular matrix) (Mitri and Foster, 2013; Ren et al., 2015). There may be further biofilm-level adaptations, however, Clarke has found these not verifiable (Clarke, 2016). Lichens share many similarities to biofilms (Carr et al., 2021; Libby and Ratcliff, 2021), and they clearly maintain characteristic types, that carry on the specific mycobiont and photobiont in successive lineages, and appear to involve multiple (prokaryotic) partners (Nelsen et al., 2020; Carr et al., 2021). Lichens provide an interesting analogy to biofilms as units of selection (Libby and Ratcliff, 2021), while their development is clearly differing from the sometimes ad hoc assembly of microbial biofilms.
Clarke has assessed the possibility that (multispecies) biofilms (or arbitrary subparts of it) may be group-selected units of evolution (Clarke, 2016), and has convincingly argued, that they lack crucial aspects to be bona fide groups under selection. Biofilm cells do not differentiate terminally, and there is not reproductive division of labor (no lineage loses irreversibly its reproductive capacity). Members can often be freely exchanged with functionally equivalent species interactions not being species-specific. Component biofilm lineages do not migrate collectively to new niches (Kolenbrander et al., 2010). There is no real group structure in the biofilm on which group selection could act, only varied local interaction networks. Without a macrobiotic host, colonization does not happen via vertical transfer (as in termites), neither do they form multispecies-spores, lacking thus an essential mechanism for stable co-occurrence of species. Hence, they do not form lineages that compete and in which biofilm-level adaptations are inherited from parent to offspring biofilm. This is because multispecies biofilms may not reproduce their structure due to fragmentation (cf. founder effect, see (Brislawn et al., 2019)) but via successive assembly during which members are horizontally recruited from the local environment (Moran and Sloan, 2015; Clarke, 2016; Douglas and Werren, 2016).
This is the appropriate time to elucidate on a subtle difference, that is often confused by those claiming that anything that is not a conventional organism (bounded by skin or vesicle and reproducing as a unit) cannot be subject to selection. We point out, that multilevel selection should not be interchanged with group formation and especially with evolutionary individuality. Selection can act on collectives, given that there is some better than random chance that members reappear together from time to time. Selection can act not only on compartmentalized (bounded) replicators, but also on temporally compartmentalized (Wilson, 1975, 1979; Matsumura et al., 2016), or no compartmentation at all in the conventional sense (e.g., spatial models, like metabolically coupled replicator systems (Czárán et al., 2015)), albeit admittedly with weaker effect. Their dynamics can be described by models of multilevel selection of the first type (MLS1), compared to the group selection dynamics of real evolutionary individuals that are themselves collectives of true, bounded reproducing units (MLS2) (Damuth and Heisler, 1988; Szathmáry, 2015; Szilágyi et al., 2017). The point is that groups of entities (biofilms) may be under selection while they may not necessarily correspond to evolutionary individuals in the conventional sense (cells). Population or spatial structure may ensure that such entities reappear better than random from time to time and can acquire and inherit adaptations (i.e., can maintain information). If so, they may evolve to be bona fide groups of selection, i.e., real units of evolution in the Maynard Smithian sense (Maynard Smith, 1987), realizing an evolutionary transition in individuality (West et al., 2015).
While biofilms are likely not individually replicating units of evolution, their members may be subject to selection at multiple levels. Community-level properties can be selected for and inherited in lab environments (Hansen et al., 2007; Ren et al., 2015), which may happen in nature, given strong selection at a higher level. Multilevel selection facilitates cooperation that further benefits the collective. Ultimately, there may just be some evolutionary potential in a biofilm. The question is then, what is the information that is stably reproduced from time to time by the community? Clarke claims, that “there will rarely be enough genetic heritability across biofilm generations to support a response to selection” (Clarke, 2016). This claim needs to find support from explicit statistical measurements of the heritability of potentially adaptive community-level traits. Investigating inheritance (if any) of biofilms or lichen thalli might provide insight on how multispecies communities evolve mechanisms to ensure group selection and inheritance. Something that can and should be done in the lab.
Community Interaction Pattern as a Replicator
A phylogenetically independent metabolic profile is present in all sorts of natural and synthetic microbial communities (Burke et al., 2011; Louca et al., 2016, 2018; Goldford et al., 2018; Cui et al., 2020; Estrela et al., 2022) and potentially in non-microbial communities like higher-level ecosystems (Veldhuis et al., 2018) and biogeochemical cycles (Levin, 1998; Braakman et al., 2017). In other words, the functional profile of microbial communities seems to be generally more stable or ecologically resilient than are their taxonomic compositions (Doolittle and Inkpen, 2018). Most of such communities develop in a successive manner, where species with fitting functional capacities appear as a niche emerges, but the specific metabolic functionality rather than a specific set of species, is required in the process. This is akin the idea of guilds: a set of different taxonomic units that occupy the same metabolic niche (i.e., has the same biochemical capacity (Burke et al., 2011)) and has the same competitive ability (Estrela et al., 2022). Guilds contain substantial taxonomic variation, independent of their initial starting composition (Goldford et al., 2018; Leventhal et al., 2018), due to multiple causes, such as the founder effect (random sampling from the initial species pool into new habitats) (Goldford et al., 2018), neutral community dynamics due to individual guild-member species being equivalent functionally and fitness-wise (Aguirre de Cárcer, 2019), dynamic multistability in population dynamics, and the existence of alternative stable states (Fukami, 2015) potentially caused by mutual exclusion (Leventhal et al., 2018). There is an ongoing debate about the role of selective effects vs. neutral factors during microbial community assembly (Rosindell et al., 2011; Cira et al., 2018). While community structure may be shaped by deterministic factors, such as competition or speciation, structural patterns can also be explained by neutral forces, such as stochastic birth and death processes and chance driven immigration (Hubbell, 2006; Sloan et al., 2006; Rosindell et al., 2011) or by a combination of the two types (Stegen et al., 2012; Cira et al., 2018). While high variability is often observed at the species level, there appears to be a consistency in the topological structure and in the community composition focusing on higher taxonomic levels (Goldford et al., 2018).
The phylogenetically conserved functionality of guilds (Estrela et al., 2022) provides an advantage when colonizing new habitats by providing robustness against stochastic partner loss and allowing communities to self-assemble given any member of the required guild is available. However, it also means that community members are not specifically required and guild-members could replace each other. While the global interaction network is built up more deterministically, the particular species that take the functional role may be driven neutrally. Moreover, in general, negative interactions (competition, antibiosis) tend to reduce the potential diversity of colonizers, while positive interactions (producing public goods or ecosystem engineering) are thought to increase it (Canon et al., 2020; Ratzke et al., 2020). It is often the colonization sequence of certain functional types that shapes the topology and not the set of species (Stephens et al., 2015). In general, microbiomes are characterized functionally rather than taxonomically, and while the overall structure, metabolic network, guild ratios are similar, the specific composition is not reproduced stably (Figure 4).
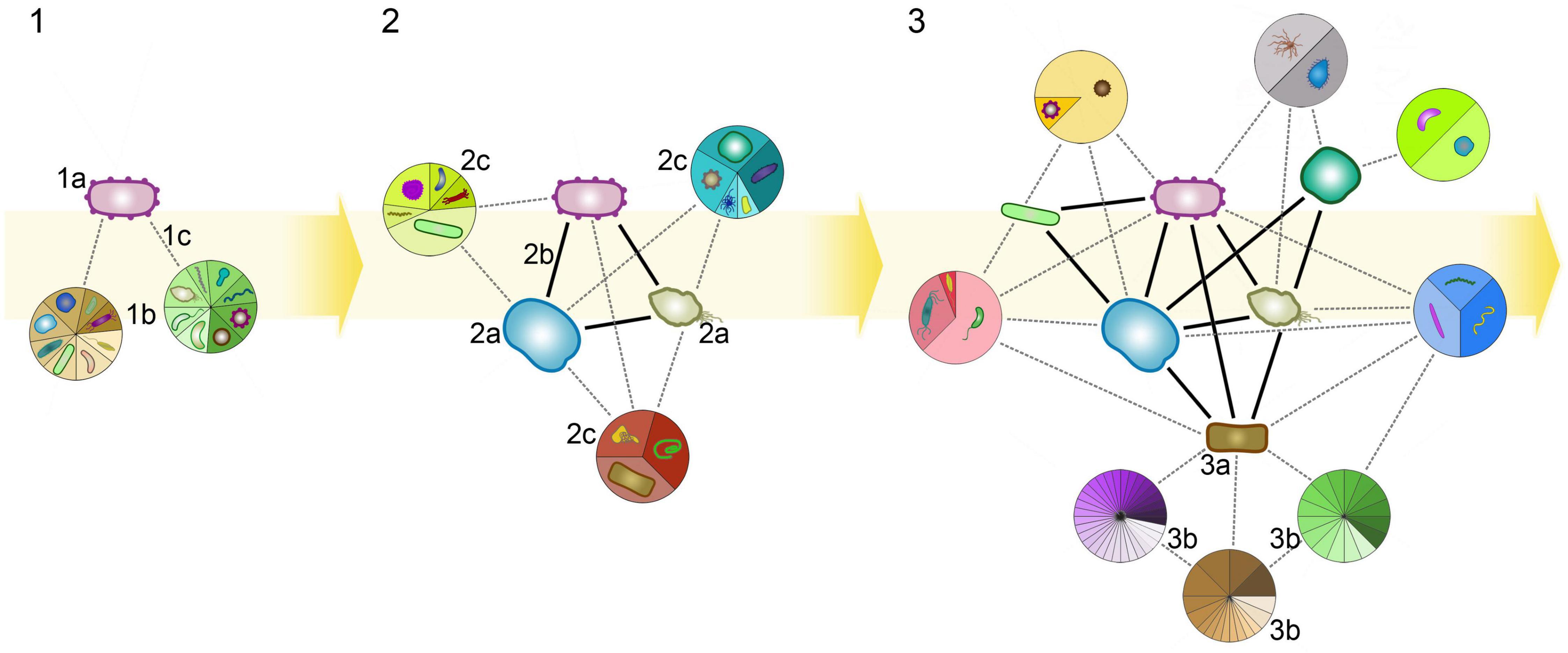
Figure 4. A potential scenario of microbial community assembly driven by deterministic and neutral factors. The colonization of a new habitat may set off from a specific species (purple founder cell). Options for subsequent partners may have a wide range (pie charts), that correspond to functional types or guilds (color schemes) that fit into the emerging interaction profile (dotted lines). The particular species recruited from a guild can be due to random chance. Initially, many alternative pioneers are possible (1b), giving rise to multiple alternative topologies (not shown). Once a recruitment happens (2a), the interaction is realized (2b; solid lines). After integration, some species can be exchanged (by another species from the same pie chart) without changing the community topology or functional profile. As species gets integrated into the community (1a, 2a, 3a), the number of further options often declines (2c), as the community presents an increasingly specific environment to join into. On the other hand, a new species (3a; e.g., ecosystem engineers) may create a local environment favorable for many new species (3b).
But then why would biofilms be considered replicators on their own right at all? The only requirement for a biofilm to be a higher-level replicator is that it stably reproduce some relevant aspect of the community in successive generations, presumably due to some form of autocatalytic multiplication. The interaction pattern is possibly such an aspect as it certainly persists for a long time, causally derived from the biofilm, and it may inherit changes. In this regard, this approach is very similar to the replicator definition of Godfrey-Smith, where the copy of a replicator is defined as something that is causally derived and is similar to the parent “in some relevant aspect” (Godfrey-Smith, 2000). This aspect does not have to be subjectively specified. It is the selection process that differentiates between classes of objects, where class members are equivalent to each other under selection, i.e., has the same fitness value in the given local time and space (Zachar and Szathmáry, 2010). The difference between classes defines the variability that should be inherited. If a reproductive system can inherit variants through successive generations of autocatalytic growth and selection, then the set of variants (classes) is, by definition, the “genotypic information” of this replicator system (Zachar and Szathmáry, 2010). For example, the functional profile of a community may be the property that is selected for; all else that is unseen by selection (e.g., the taxonomic composition of the community) is not expected to be heritable and exhibit neutral dynamics (subject to phenotypic exchange or plasticity). Taxonomic composition thus cannot be part of the genotype of the higher-level replicator. The question is, then, whether there is indeed a higher-level heritable “genotype” that can be stably maintained for an indefinite time and whether it can be selected for?
In the recent decade, Ford Doolittle has elaborated on the idea that something related to the coupled metabolism of species replicates in a metabolic community, other than the exact taxonomic composition (Doolittle, 2013). Clarke (2016) has pointed out, that neither the species composition nor the metabolic capacity of the community is constant in time, changing with the environment or due to internal mechanisms (Doolittle and Booth, 2017). Biofilms may inherit not only the metabolic profile but a characteristic community interaction pattern or “interactome” (the joint metabolism, developmental interactions and phenotypic functionality of constituents). It is responsible for the development of the same functional composition (set of guilds) at subsequent habitats (Doolittle, 2013; Doolittle and Booth, 2017). Do such patterns show heritable variation in fitness that justifies considering them replicators?
According to Doolittle (Doolittle, 2013; Doolittle and Booth, 2017), the crucial mechanism that ensures replication at the biofilm level is not vertical inheritance of biofilm lineages, but the adaptive, genetically encoded recruitment of species (or guild-members) and lateral gene transfer. The latter may ensure the dispersal of adaptive genes within the guild or community (Song et al., 2021). Selective recruitment may emerge from co-aggregation mechanisms (partner recognition and preferential association and adherence (Katharios-Lanwermeyer et al., 2014)) and niche transformation. As the niche is successively transformed by the successive cooperative communities (Gatti et al., 2018), such mechanisms may more effectively regulate what species (or functional guilds) and in what order can join the aggregation. Ultimately, these traits, encoded genetically in member species, may increase the probability of co-occurrence (Periasamy and Kolenbrander, 2009; Clarke, 2016). Goldford et al. (2018) have found in the lab that starting bacterial communities self-assembled into stable multispecies communities, stabilized by cross-feeding, regardless of a high taxonomic variance in composition.
Lateral gene transfer occurs frequently in biofilms (Song et al., 2021). Constant gain and loss of genes result in a distribution of the essential pan-genome between strains of the community (Fullmer et al., 2015), in which all members carry a set of core genes, and only a fraction carry accessory genes (Fullmer et al., 2015; Booth et al., 2016). The pan-genome is therefore the shared genomic resource and the associated cooperative meta-cell interaction network (Fullmer et al., 2015). Several mechanisms are hypothesized to shape pan-genomes, such as gene-gene or gene-by-environment interactions, positive and negative frequency-dependent selection, or the Black Queen mechanism (Domingo-Sananes and McInerney, 2021). Indeed, a considerable proportion of microbes have disproportionately small genomes with limited metabolic capacity (Booth et al., 2016; Stolz, 2016) hence must rely on obligate partners. Such evidence puts light on the distributed nature of heritable information in microbial communities and may question how we define ‘cellular’ life and mutual dependency (Stolz, 2016).
As a speculative conclusion, such communities may exhibit a form of reproduction, from colonization to colonization, as they reproduce not only the metabolic pattern of the community, but also the pan-genome (for some extent) and succession order and the (terminal) ratio of functional guilds (Estrela et al., 2022). Species do this by relying on genes in members of the broader phylogenomic group of a guild, encoding responsible metabolic and niche-constructing traits (Odling-Smee et al., 2003; Laland et al., 2016) other guilds may benefit from. This way the metabolic effects exerted on the environment (leaked metabolites, transformed external resources) become cooperative interactions as species realize cross-feeding not necessarily simultaneously but successively in time, as the community assembles and develops.
The succession of guilds and species may yield multi-stable population dynamics with alternative stable states within a functional guild (Estrela et al., 2022). This results in different attractors of community composition, and the evolutionary route depends heavily on initial conditions and perturbations. If initial and transient stages can be stably inherited (which we do not know), then the community-level information (e.g., functional profile) is not maintained by taxonomic composition or by a conservative storage (as DNA stores genetic information), but in the attractors of the dynamical system including populational, aggregational, and environmental conditions. The added novelty, in case of microbial biofilms, is that communities themselves modify their environment as they successively develop, on a much faster timescale. Experiments show that a niche constructing strain might have higher fitness in a self-modified environment than in the original environment (Callahan et al., 2014). Genetic traits encoding for ordered niche construction are directly responsible for the canalizing effect during the succession of guilds and maturing of biofilms. They may even spread during colonization (or via LGT) and are selected for, as they ensure that the same guild succession-pattern emerges.
Ecosystems (microbial or above) can persist, reappear and function stably for a long time, maintaining a homeostatic local environment. In one dominant view, biotic feedback cycles (autocatalytic networks (Gatti et al., 2018)) can drive persistence-based selection (Lenton et al., 2021). Such feedback cycles include unrelated components performing different functions. Such cross-cooperation may allow the expansion or emergence of niches (Gatti et al., 2018, 2020) as was demonstrated in microbial communities (Großkopf et al., 2016; Gatti et al., 2018; San Roman and Wagner, 2018; Oña et al., 2021). For physiological adaptations to happen in co-evolution, prolonged stability of the environment is required, so that ecological and evolutionary contexts (balance of benefit and cost) are kept constant (Herre et al., 1999; Gomulkiewicz et al., 2003; Hillesland, 2017; O’Brien et al., 2021). A homeostatic microbial ecosystem may provide a temporal buffer for co-evolutionary processes (Bateman, 2020).
Symbiotic Pair as a Replicator
There is another option for microbes to transit from a multispecies shared metabolic community to an integrated new unit of evolution. A real example of egalitarian multicellularity, albeit not in the conventional meaning of multicellularity, is obligate endosymbioses (Szathmáry, 2015). Internalization of a symbiont involves many problems the partners are likely not preadapted for [selfishness, asynchronous cell cycles, transport, see Zachar and Boza (2020)]. Microbial partners can nevertheless benefit form synergies (see the many prokaryotic (Zachar and Boza, 2020) and protist ectosymbioses (Husnik et al., 2021)). Such partnerships may ultimately physically integrate to escape competition at the lower level (Figure 3). It must be noted, that waiving independent replication often occurs for the (bacterial) symbiont only (Sachs et al., 2011), which may indicate that integration has not been concluded. In obligate endosymbiosis, (i) no species can leave or reproduce without the other; and (ii) both species retain their metabolic identities without being eaten or degraded. There are endosymbionts which engage in syntrophic interactions with their hosts (e.g., H2-scavanging methanogenic archaea of anaerobic protists (Wagener and Pfennig, 1987; Fenchel and Finlay, 1992; Gutiérrez et al., 2017; Beinart et al., 2018; Lind et al., 2018), sulfate-oxidizing ε-proteobacteria that colonize their surface (Edgcomb et al., 2011)).
Microbial endosymbiosis to protist hosts is diverse and ubiquitous (Lowe et al., 2016; Husnik et al., 2021), as one would expect, due mostly to phagocytosis (Nowack and Melkonian, 2010; Speijer, 2021). However, it is unknown if any eukaryotic nutritional endosymbiosis arose from an initially mutual partnership, either as a separate pair or part of a larger syntrophic community. In more general terms, we do not know from either in vivo, in vitro or in silico examples, if a multispecies metabolic community (especially prokaryotic) has ever given rise to endosymbiosis (Zachar and Boza, 2020) or at least to exclusively pairwise syntrophic symbiosis. Neither do we know for certain, if any microbial endosymbiosis has ever originated from an initially mutually syntrophic pair. While prokaryotic cross-feeding (communities) are ubiquitous, no prokaryote is capable of phagocytosis. Hence the possibility of endosymbiosis among prokaryotes and the origin of mitochondria remains a conundrum.
The single presumed example of endosymbiosis among prokaryotes is the possible syntrophic origin of mitochondria, according to syntrophic theories (Martin et al., 2015; Spang et al., 2019; Imachi et al., 2020; López-García and Moreira, 2020). These theories posit an initially mutual metabolic cooperation between the ancestors of mitochondria and host, instead of exploitation by parasitism (White et al., 2018) or by phagocytosis (Martijn and Ettema, 2013; Zachar et al., 2018). Syntrophy ultimately lead to the unspecified engulfment of the bacterial partner. Such an inclusion between syntrophic prokaryotes (or protists for that matter) has never been documented. On the other hand, the sheer amount of syntrophies among prokaryotes proves that mutual syntrophy is an extremely common and stable interaction when external. Protists either receive their numerous endosymbionts via eukaryote-specific ways (via phagocytosis) or via parasitism, which are both well documented (Sachs et al., 2011; Speijer, 2021). The primary plastid of all plants probably had a single origin (Adl et al., 2018), at which point the host was likely already phagocytic, even if only rudimentarily (Mills, 2020). Later re-uptakes of plastid-bearing cells by protists sometimes retained the phagosomal membrane as a proof of phagocytic inclusion (Keeling, 2013; Speijer, 2021). On the other hand, according to a common view, the vast majority of extant host-bacteria associations are mostly parasitic of origin, and are costly for the host (Sachs et al., 2011; Keeling and McCutcheon, 2017; Husnik et al., 2021). If syntrophy has indeed enabled and facilitated endosymbiosis of mitochondria, one must ask, why such transitions are not seen more often among prokaryotes?
We hypothesize that the lack of (endo)symbiotic examples originating from a syntrophic community is due to multiple factors. For eukaryotes, it is mostly because those protists capable of phagocytosis (i.e., has a mechanism to integrate a symbiont) are never participating in biofilm formation or syntrophic communities. Parasitic endosymbionts capable of entering a host by themselves prefer heterotrophic hosts for energetic reasons, again not members of biofilms. The issue is clearer in case of prokaryotic symbioses, where phagocytosis is not an option to acquire the partner. We do not know how many of the pairwise syntrophic ectosymbioses of prokaryotes emerged from larger communities, but there is no example of bona fide endosymbiosis other than possibly mitochondria (Zachar and Boza, 2020), and even in that case it is not clear whether they started out as an exclusive pair or part of a community. The cause must be inherent to metabolic network topology and stability of communities (prokaryotic and eukaryotic alike in this regard).
The alternative hypothesis, at least for eukaryotes, is that endosymbiosis does happen more frequently in larger communities, but then the pair quickly decouples from or dominates the network due to the pair benefiting the most under selection (Figures 2, 3). A third option is that syntrophic endosymbioses do not come from syntrophic communities. They may have evolved from syntrophic pairs that were never part of a bigger community; or syntrophy may evolve after integration (like the ATP-ADP exchange of mitochondria and host, as is believed so (Zachar and Szathmáry, 2017)), assuming the initial interaction was not mutualism (Zachar et al., 2018). These hypotheses could be tested, by modeling the emergence of pairwise symbiosis within or without communities and testing their stability against internal and external perturbation.
One may ask, why exclusively pairwise interactions are not more common in microbial communities. Syntrophic mutualism is the microbial realization of a 2-membered cooperative cycle where both species benefit from the interaction. A natural extension then is to assume that a 3 or N-membered cooperative cycle can also emerge, stabilize and be selected for at the group level. However, no multimembered circularly cooperating microbial metabolic network is known. There exist communities with cyclic cooperation (e.g., 3-species Utricularia system (Ulanowicz, 1995; Ulanowicz et al., 2014)), but microbes usually form more complex networks: positive interactions are not necessarily reciprocated (directly, specifically or exclusively) and a cooperative chain is not guaranteed to close. Most importantly, direct interactions are often competitive (even inhibitive) leading to complicated interaction networks, that can still lead to community stability (Kato et al., 2005). Circular competition is common, but exclusively circular mutualism is rare, possibly nonexistent, for N > 2. Naturally, the larger a cooperative cycle is, the more prone it is to selfish cheaters and destabilization. It is unknown (and unlikely) if such a network can give rise to a specific mutual pair that can stably emerge, without strong means of vertical co-inheritance (like engulfment).
In summary, it is yet unknown if endosymbiosis could or have ever evolved in multispecies communities from mutual syntrophy. Based on observable data, we believe that, on one hand, endosymbiotic integration of mutually syntrophic prokaryotes is extremely rare. On the other hand, protists likely acquire their endosymbionts via pairwise interactions (e.g., phagocytosis) and not as being part of a community or biofilm. This, of course, does not mean that any of it is impossible. The singular case of long term stable endosymbiosis emerging from mutual syntrophy, as theorized for mitochondria, could be the result of an extremely improbable event.
Conclusion
We have reviewed how microbial cooperation can emerge and stabilize communities and how they can potentially facilitate a major transition. We pointed out, that the metabolic topology (cross-feeding) itself is not enough to analyse cooperation, as it only defines a trophic interaction mediated by metabolites. One must know the cooperative topology, the second order catalytic aid that provides a benefit. We reviewed how cooperation may emerge via metabolic interactions and public goods in microbial communities, and have considered the theoretically possible routes how a microbial community may achieve higher integration due to cooperation and transition in individuality. Finally, we have investigated how a multispecies community may undergo group selection and inherit community-level variations.
If biofilms could somehow undergo a transition in individuality, there would be a theoretically possible escape route from the microbial hurdle toward higher level integration and a major transition, potentially available for prokaryotes too. Admittedly, some of these ideas are speculative, but they serve a dual purpose. Firstly, they clearly distinguish between the various aspects of a community that may or may not carry heritable information. There may be some epigenetic information (even if limited) encoded not in genes, that can be subjects of selection at the level of the collective. Secondly, they identify the possible routes that may be more realistic in other domains, where similarly coupled Darwinian systems exist. Biofilms may be a domain-specific case of a more general idea of replicators coupled through (metabolic) facilitation, and while they may have at best limited heredity that we know of, they may have a potential for increasing this limit or facilitating a possible future transition (Robin et al., 2021).
Certain features of a biofilm do contribute to the growth, survival, and multiplication of the community. While total information content of a community likely cannot reappear deterministically from time to time, some subset of this information may do so, despite severe ecological and evolutionary fluctuations. One can even state, that these features, responsible for this subset of information, are replicators as they re-appear with the biofilm. Indeed, the extended phenotype (the interaction pattern or the constructed niche of the biofilm) may be autocatalytically generated and may cross-catalytically help the biofilm. There is certainly a positive feedback loop (catalysis) between the properties of the biofilm and biofilm species (their genes) that benefit the biofilm as their extended phenotype, leading to positive selection. However, autocatalysis should not be mistaken for informational replication: biofilm-level adaptations do not inherit their variations. Without variability that can be causally and stably reproduced, these extended cycles cannot inherit information and are thus non-informational replicators (Zachar and Szathmáry, 2010). The nest of the bird, the dam of the beaver, the habitat of microbiome may catalyze the replication of their respective replicators (bird, beaver, microbes), but they cannot stably inherit variations.
There may be properties of a biofilm, that are replicated from time to time as the community spreads (guild amounts, ratios, metabolic capacity, interaction pattern, constructed niche, etc.). Can any of these inherit changes? This can only be ascertained if one measures the response to selection and the heritability of community traits of a biofilm “population” on a statistical basis. We must emphasize, that niche construction, causing persistent conditions of the external environment, should only be considered if persistent external entities can pass on acquired variation. In reality, variations of the external conditions likely cause the extinction of the community or won’t affect its replication: while there may be a causal arrow, there is no informational arrow from habitat to community.
Recently, there have been advances in artificial engineering and directed selection of microbial communities (Castle et al., 2021). Artificial community selection aims at finding a proper map between community composition and community function, to improve desired functionalities via selection (Chang et al., 2021). Theoretical models present promising results regarding the heritability of community functions (Xie and Shou, 2021), however, success in establishing long-lasting modifications in engineered functionalities are yet to be seen in experimental systems (Albright et al., 2021). Note, that when the environment repeatedly enforces a single outcome (as often does under artificial selection), it should not be called replication, as relevant variations are not inherited.
Is there a chance for a major transition in a multispecies biofilm then? Certainly. One can imagine directed selection in the lab that enhances existing mechanisms of association and prefers novel adaptations that further ensures better together-replication of partners against competing biofilms. Could it happen in the wild? We see no theoretical objection to it. Is there any such living microbial community that have been transited or are in transition? Not that we know of. But there are microbial communities that reappear more frequently than others from time to time (especially, when the species count is as low as 2; explored in Zachar and Boza, 2020), indicative of their reproductive and associative stability. Has it ever happened to a biofilm? Not that we know of. At least not in the sense one would imagine the compartmented replication of groups of microbes of the community. One can argue, that if syntrophy can lead to the integration of individually replicating species, as mitochondriogenesis theories assume, then it may provide a particular route how aggregative evolutionary transitions may happen in the microbial world. On the other hand, the observed fact that metabolically coupled communities fail to transit to higher levels of selection and form bona fide groups may be an indication that this transition is exceptionally hard, at least in the prokaryotic and protist domains. Maybe, there are other domains where formally analogous systems can form and have better chances to achieve a transition.
Doolittle and Booth (2017) claim, that interaction patterns do vary and that their variation is heritable and causes differential fitness, leading to the evolution of these networks by natural selection. That is, there is an “epigenetic” source of heritability in biofilms, that could support evolution of the biofilm at a group level. It may turn out that syntrophic communities in this regard can be considered informational replicators, encoding something about their dependent metabolism and interactions at the group level. Likely, this information has limited variability (the amount of selectively different attractors defined by different functional profiles), hence biofilms may only have limited hereditary potential (Szathmáry, 2000). If this is so, we hypothesize, that the transition from this limited hereditary state to unlimited heredity is not possible due to the attractor-based inheritance system of the community. There is just not enough variability for evolution to select for and improve upon. There is, however, a lack of modeling to support this at the moment.
But let’s assume for a moment, that some information is heritable. The biofilm thus likely have at least limited heredity. If there is heredity, there is differential selection of the variants. If there is selection, there may be adaptations that affect the collective and are selected for. Epigenetic systems do exist, complementing genetic inheritance (Jablonka and Szathmáry, 1995; Jablonka et al., 1998). They also have limited heredity, so there is nothing unorthodox about assuming that a (microbial) community may pass on aggregate-level information to new colonies. At this point we do not claim that any community property qualifies as a heritable property, we only claim that we do not see a theoretical objection that such things may or may have existed. Whether such limited heredity would ever become practically unlimited and whether it would enable a classical transition in individuality are unlikely due to the competitive, distributed and highly variable nature of microbial communities. If, however, one accepts that such properties could exist, one has to follow the rabbit hole all to the end, to the full Darwinian suite that leads to open ended evolution of a new level of informational inheritance system and thus to a potential major transition.
Author Contributions
IZ and GB contributed to the writing and the editing of the manuscript and the designing of the figures. Both authors contributed to the article and approved the submitted version.
Funding
The authors acknowledge support from the Hungarian Research Fund under grant numbers NKFIH #132250 (GB), #128289 (GB), #140901 (IZ), and #129848 (IZ), from the Volkswagen Stiftung initiative “Leben?-Ein neuer Blick der Naturwissenschaften auf die grundlegenden Prinzipien des Lebens” (IZ), and from the European Union’s Horizon 2020 Research and Innovation Programme under grant agreement no. 952914 (GB).
Conflict of Interest
The authors declare that the research was conducted in the absence of any commercial or financial relationships that could be construed as a potential conflict of interest.
Publisher’s Note
All claims expressed in this article are solely those of the authors and do not necessarily represent those of their affiliated organizations, or those of the publisher, the editors and the reviewers. Any product that may be evaluated in this article, or claim that may be made by its manufacturer, is not guaranteed or endorsed by the publisher.
Acknowledgments
We are thankful for Eörs Szathmáry for inspiring ideas and comments on the manuscript and István Scheuring for discussions.
Footnotes
- ^ According to cooperation theory, increasing population density of the partner species increases the relative fitness of receiver species (Dobay, 2014). In microbial context, this amounts to the assumption that products realize the cooperative benefit, and the cooperative efficiency of a product depends on its concentration. Since product concentration usually depends on the density of producers, ultimately the benefit of cooperation depends on the density (and production rate) of producers, as was assumed by, e.g. (Jimenez and Scheuring, 2021). The benefit of a species is thus ultimately realized in the increased growth rate or carrying capacity in such a model.
References
Ackermann, M. (2015). A functional perspective on phenotypic heterogeneity in microorganisms. Nat. Rev. Microbiol. 13, 497–508. doi: 10.1038/nrmicro3491
Adl, S. M., Bass, D., Lane, C. E., Lukeš, J., Schoch, C. L., Smirnov, A., et al. (2018). Revisions to the classification, nomenclature, and diversity of eukaryotes. J. Eukaryot. Microbiol. 66, 4–119. doi: 10.1111/jeu.12691
Aguirre de Cárcer, D. (2019). A conceptual framework for the phylogenetically constrained assembly of microbial communities. Microbiome 7:142. doi: 10.1186/s40168-019-0754-y
Albright, M. B. N., Louca, S., Winkler, D. E., Feeser, K. L., Haig, S.-J., Whiteson, K. L., et al. (2021). Solutions in microbiome engineering: prioritizing barriers to organism establishment. ISME J. 16, 331–338. doi: 10.1038/s41396-021-01088-5
Antonova, E. S., and Hammer, B. K. (2011). Quorum-sensing autoinducer molecules produced by members of a multispecies biofilm promote horizontal gene transfer to Vibrio cholerae. FEMS Microbiol. Lett. 322, 68–76. doi: 10.1111/j.1574-6968.2011.02328.x
Bateman, A. (2020). Division of labour in a matrix, rather than phagocytosis or endosymbiosis, as a route for the origin of eukaryotic cells. Biol. Direct 15:8. doi: 10.1186/s13062-020-00260-9
Beinart, R. A., Rotterová, J., Čepička, I., Gast, R. J., and Edgcomb, V. P. (2018). The genome of an endosymbiotic methanogen is very similar to those of its free-living relatives. Environ. Microbiol. 20, 2538–2551. doi: 10.1111/1462-2920.14279
Bernstein, H. C., Paulson, S. D., and Carlson, R. P. (2012). Synthetic Escherichia coli consortia engineered for syntrophy demonstrate enhanced biomass productivity. J. Biotechnol. 157, 159–166. doi: 10.1016/j.jbiotec.2011.10.001
Booth, A., Mariscal, C., and Doolittle, W. F. (2016). The modern synthesis in the light of microbial genomics. Annu. Rev. Microbiol. 70, 279–297. doi: 10.1146/annurev-micro-102215-095456
Boyle, K. E., Heilmann, S., van Ditmarsch, D., and Xavier, J. B. (2013). Exploiting social evolution in biofilms. Curr. Opin. Microbiol. 16, 207–212. doi: 10.1016/j.mib.2013.01.003
Braakman, R., Follows, M. J., and Chisholm, S. W. (2017). Metabolic evolution and the self-organization of ecosystems. Proc. Natl. Acad. Sci. U. S. A. 114, E3091–E3100. doi: 10.1073/pnas.1619573114
Brislawn, C. J., Graham, E. B., Dana, K., Ihardt, P., Fansler, S. J., Chrisler, W. B., et al. (2019). Forfeiting the priority effect: turnover defines biofilm community succession. ISME J. 13, 1865–1877. doi: 10.1038/s41396-019-0396-x
Bronstein, J. L. (1994). Conditional outcomes in mutualistic interactions. Trends Ecol. Evol. 9, 214–217. doi: 10.1016/0169-5347(94)90246-1
Bull, J. J., and Harcombe, W. R. (2009). Population dynamics constrain the cooperative evolution of cross-feeding. PLoS One 4:e4115. doi: 10.1371/journal.pone.0004115
Burger, J. R., Hou, C., Hall, C. A. S., and Brown, J. H. (2021). Universal rules of life: metabolic rates, biological times and the equal fitness paradigm. Ecol. Lett. 24, 1262–1281. doi: 10.1111/ele.13715
Burke, C., Steinberg, P., Rusch, D., Kjelleberg, S., and Thomas, T. (2011). Bacterial community assembly based on functional genes rather than species. Proc. Natl. Acad. Sci. U. S. A. 108, 14288–14293. doi: 10.1073/pnas.1101591108
Callahan, B. J., Fukami, T., and Fisher, D. S. (2014). Rapid evolution of adaptive niche construction in experimental microbial populations: experimental evolution of niche construction. Evolution 68, 3307–3316. doi: 10.1111/evo.12512
Canon, F., Nidelet, T., Guédon, E., Thierry, A., and Gagnaire, V. (2020). Understanding the mechanisms of positive microbial interactions that benefit lactic acid bacteria co-cultures. Front. Microbiol. 11:2088. doi: 10.3389/fmicb.2020.02088
Carr, E. C., Harris, S. D., Herr, J. R., and Riekhof, W. R. (2021). Lichens and biofilms: common collective growth imparts similar developmental strategies. Algal Res. 54:102217. doi: 10.1016/j.algal.2021.102217
Castelle, C. J., Wrighton, K. C., Thomas, B. C., Hug, L. A., Brown, C. T., Wilkins, M. J., et al. (2015). Genomic expansion of domain Archaea highlights roles for organisms from new phyla in anaerobic carbon cycling. Curr. Biol. 25, 690–701. doi: 10.1016/j.cub.2015.01.014
Castle, S. D., Grierson, C. S., and Gorochowski, T. E. (2021). Towards an engineering theory of evolution. Nat. Commun. 12:3326. doi: 10.1038/s41467-021-23573-3
Cavaliere, M., Feng, S., Soyer, O. S., and Jiménez, J. I. (2017). Cooperation in microbial communities and their biotechnological applications. Environ. Microbiol. 19, 2949–2963. doi: 10.1111/1462-2920.13767
Chamberlain, S. A., Bronstein, J. L., and Rudgers, J. A. (2014). How context dependent are species interactions? Ecol. Lett. 17, 881–890. doi: 10.1111/ele.12279
Chang, C.-Y., Vila, J. C. C., Bender, M., Li, R., Mankowski, M. C., Bassette, M., et al. (2021). Engineering complex communities by directed evolution. Nat. Ecol. Evol. 5, 1011–1023. doi: 10.1038/s41559-021-01457-5
Chomicki, G., Kiers, E. T., and Renner, S. S. (2020). The evolution of mutualistic dependence. Annu. Rev. Ecol. Evol. Syst. 51, 409–432. doi: 10.1146/annurev-ecolsys-110218-024629
Cira, N. J., Pearce, M. T., and Quake, S. R. (2018). Neutral and selective dynamics in a synthetic microbial community. Proc. Natl. Acad. Sci. U. S. A. 115, E9842–E9848. doi: 10.1073/pnas.1808118115
Clarke, E. (2016). Levels of selection in biofilms: multispecies biofilms are not evolutionary individuals. Biol. Philos. 31, 191–212. doi: 10.1007/s10539-016-9517-3
Cohen, A. A., Martin, L. B., Wingfield, J. C., McWilliams, S. R., and Dunne, J. A. (2012). Physiological regulatory networks: ecological roles and evolutionary constraints. Trends Ecol. Evol. 27, 428–435. doi: 10.1016/j.tree.2012.04.008
Colwell, R. K., and Rangel, T. F. (2009). Hutchinson’s duality: the once and future niche. Proc. Natl. Acad. Sci. U. S. A. 106, 19651–19658. doi: 10.1073/pnas.0901650106
Cooper, G. A., and West, S. A. (2018). Division of labour and the evolution of extreme specialization. Nat. Ecol. Evol. 2, 1161–1167. doi: 10.1038/s41559-018-0564-9
Cordero, O. X., Ventouras, L.-A., DeLong, E. F., and Polz, M. F. (2012). Public good dynamics drive evolution of iron acquisition strategies in natural bacterioplankton populations. Proc. Natl. Acad. Sci. U. S. A. 109, 20059–20064. doi: 10.1073/pnas.1213344109
Crespi, B. J. (2001). The evolution of social behavior in microorganisms. Trends Ecol. Evol. 16, 178–183. doi: 10.1016/S0169-5347(01)02115-2
Cui, Z., Huntley, R. B., Zeng, Q., and Steven, B. (2020). Temporal and spatial dynamics in the apple flower microbiome in the presence of the phytopathogen Erwinia amylovora. ISME J. 15, 318–329. doi: 10.1038/s41396-020-00784-y
Czárán, T., Könnyű, B., and Szathmáry, E. (2015). Metabolically coupled replicator systems: overview of an RNA-world model concept of prebiotic evolution on mineral surfaces. J. Theor. Biol. 381, 39–54. doi: 10.1016/j.jtbi.2015.06.002
Damuth, J., and Heisler, I. L. (1988). Alternative formulations of multilevel selection. Biol. Philos. 3, 407–430. doi: 10.1007/bf00647962
Davies, D. G. (2011). “Biofilm dispersion,” in Springer Series on Biofilms, eds K. P. Rumbaugh and T. Coenye (Berlin Heidelberg: Springer), 1–28. doi: 10.1007/978-3-642-19940-0_1
de Lorenzo, V., Sekowska, A., and Danchin, A. (2014). Chemical reactivity drives spatiotemporal organisation of bacterial metabolism. FEMS Microbiol. Rev. 39, 96–119. doi: 10.1111/1574-6976.12089
De Mazancourt, C., Loreau, M., and Dieckmann, U. (2005). Understanding mutualism when there is adaptation to the partner. J. Ecol. 93, 305–314. doi: 10.1111/j.0022-0477.2004.00952.x
Dionisio, F., and Gordo, I. (2006). The tragedy of the commons, the public goods dilemma, and the meaning of rivalry and excludability in evolutionary biology. Evol. Ecol. Res. 8, 321–332.
Dobay, A., Bagheri, H. C., Messina, A. Kümmerli, R., and Rankin, D. J. (2014). Interaction effects of cell diffusion, cell density and public goods properties on the evolution of cooperation in digital microbes. J. Evol. Biol. 27, 1869–1877. doi: 10.1111/jeb.12437
Doebeli, M. (2002). A model for the evolutionary dynamics of cross-feeding polymorphisms in microorganisms. Popul. Ecol. 44, 59–70. doi: 10.1007/s101440200008
Domingo-Sananes, M. R., and McInerney, J. O. (2021). Mechanisms that shape microbial pangenomes. Trends Microbiol. 29, 493–503. doi: 10.1016/j.tim.2020.12.004
Doolittle, W. F. (2013). Microbial neopleomorphism. Biol. Philos. 28, 351–378. doi: 10.1007/s10539-012-9358-7
Doolittle, W. F., and Booth, A. (2017). It’s the song, not the singer: an exploration of holobiosis and evolutionary theory. Biol. Philos. 32, 5–24. doi: 10.1007/s10539-016-9542-2
Doolittle, W. F., and Inkpen, S. A. (2018). Processes and patterns of interaction as units of selection: an introduction to ITSNTS thinking. Proc. Natl. Acad. Sci. U. S. A. 115, 4006–4014. doi: 10.1073/pnas.1722232115
Douglas, A. E., and Werren, J. H. (2016). Holes in the hologenome: why host-microbe symbioses are not holobionts. mBio 7:e02099. doi: 10.1128/mbio.02099-15
Dragoš, A., Kiesewalter, H., Martin, M., Hsu, C.-Y., Hartmann, R., Wechsler, T., et al. (2018). Division of labor during biofilm matrix production. Curr. Biol. 28, 1903–1913.e5. doi: 10.1016/j.cub.2018.04.046
Drew, G. C., Stevens, E. J., and King, K. C. (2021). Microbial evolution and transitions along the parasite–mutualist continuum. Nat. Rev. Microbiol. 19, 623–638. doi: 10.1038/s41579-021-00550-7
D’Souza, G., and Kost, C. (2016). Experimental evolution of metabolic dependency in bacteria. PLoS Genet. 12:e1006364. doi: 10.1371/journal.pgen.1006364
D’Souza, G., Shitut, S., Preussger, D., Yousif, G., Waschina, S., and Kost, C. (2018). Ecology and evolution of metabolic cross-feeding interactions in bacteria. Nat. Prod. Rep. 35, 455–488. doi: 10.1039/c8np00009c
D’Souza, G. G. (2020). Phenotypic variation in spatially structured microbial communities: ecological origins and consequences. Curr. Opin. Biotechnol. 62, 220–227. doi: 10.1016/j.copbio.2019.12.013
Edgcomb, V. P., Leadbetter, E. R., Bourland, W., Beaudoin, D., and Bernhard, J. M. (2011). Structured multiple endosymbiosis of bacteria and archaea in a ciliate from marine sulfidic sediments: a survival mechanism in low oxygen, sulfidic sediments? Front. Microbiol. 2:55. doi: 10.3389/fmicb.2011.00055
Eigen, M. (1971). Self-organization of matter and the evolution of biological macromolecules. Naturwissenschaften 58, 465–523. doi: 10.1007/BF00623322
Embley, T. M., and Finlay, B. J. (1994). Systematic and morphological diversity of endosymbiotic methanogens in anaerobic ciliates. Antonie van Leeuwenhoek 64, 261–271. doi: 10.1007/bf00873086
Ereshefsky, M., and Pedroso, M. (2012). Biological individuality: the case of biofilms. Biol. Philos. 28, 331–349. doi: 10.1007/s10539-012-9340-4
Ereshefsky, M., and Pedroso, M. (2015). Rethinking evolutionary individuality. Proc. Natl. Acad. Sci. U. S. A. 112, 10126–10132. doi: 10.1073/pnas.1421377112
Estrela, S., Kerr, B., and Morris, J. J. (2016). Transitions in individuality through symbiosis. Curr. Opin. Microbiol. 31, 191–198. doi: 10.1016/j.mib.2016.04.007
Estrela, S., Morris, J. J., and Kerr, B. (2015). Private benefits and metabolic conflicts shape the emergence of microbial interdependencies. Environ. Microbiol. 18, 1415–1427. doi: 10.1111/1462-2920.13028
Estrela, S., Vila, J. C. C., Lu, N., Bajic, D., Rebolleda-Gomez, M., Chang, C.-Y., et al. (2022). Metabolic rules of microbial community assembly. Cell Syst. 13, 29–42.e7. doi: 10.1016/j.cels.2021.09.011
Evans, C. R., Kempes, C. P., Price-Whelan, A., and Dietrich, L. E. P. (2020). Metabolic heterogeneity and cross-feeding in bacterial multicellular systems. Trends Microbiol. 28, 732–743. doi: 10.1016/j.tim.2020.03.008
Faust, K., and Raes, J. (2012). Microbial interactions: from networks to models. Nat. Rev. Microbiol. 10, 538–550. doi: 10.1038/nrmicro2832
Fellous, S., and Salvaudon, L. (2009). How can your parasites become your allies? Trends Parasitol. 25, 62–66. doi: 10.1016/j.pt.2008.11.010
Fenchel, T. (2006). “Eukaryotic life: anaerobic physiology,” in Evolution of Microbial Life, eds D. M. Roberts, P. Sharp, G. Alderson, and M. A. Collins (Cambridge: Cambridge University Press), 185–203.
Fenchel, T., and Bernard, C. (1993b). Endosymbiotic purple non-sulphur bacteria in an anaerobic ciliated protozoon. FEMS Microbiol. Lett. 110, 21–25. doi: 10.1111/j.1574-6968.1993.tb06289.x
Fenchel, T., and Finlay, B. J. (1991). Endosymbiotic methanogenic bacteria in anaerobic ciliates: significance for the growth efficiency of the host. J. Protozool. 38, 18–22. doi: 10.1111/j.1550-7408.1991.tb04788.x
Fenchel, T., and Finlay, B. J. (1992). Production of methane and hydrogen by anaerobic ciliates containing symbiotic methanogens. Arch. Microbiol. 157, 475–480. doi: 10.1007/BF00276765
Fritts, R. K., McCully, A. L., and McKinlay, J. B. (2021). Extracellular metabolism sets the table for microbial cross-feeding. Microbiol. Mol. Biol. Rev. 85, 1–29. doi: 10.1128/mmbr.00135-20
Fröstl, J. M., and Overmann, J. (1998). Physiology and tactic response of the phototrophic consortium “Chlorochromatium aggregatum. Arch. Microbiol. 169, 129–135. doi: 10.1007/s002030050552
Fukami, T. (2015). Historical contingency in community assembly: integrating niches, species pools, and priority effects. Annu. Rev. Ecol. Evol. Syst. 46, 1–23. doi: 10.1146/annurev-ecolsys-110411-160340
Fullmer, M. S., Soucy, S. M., and Gogarten, J. P. (2015). The pan-genome as a shared genomic resource: mutual cheating, cooperation and the black queen hypothesis. Front. Microbiol. 6:728. doi: 10.3389/fmicb.2015.00728
Gatti, R. C., Fath, B., Hordijk, W., Kauffman, S., and Ulanowicz, R. (2018). Niche emergence as an autocatalytic process in the evolution of ecosystems. J. Theor. Biol. 454, 110–117. doi: 10.1016/j.jtbi.2018.05.038
Gatti, R. C., Koppl, R., Fath, B. D., Kauffman, S., Hordijk, W., and Ulanowicz, R. E. (2020). On the emergence of ecological and economic niches. J. Bioeconomics 22, 99–127. doi: 10.1007/s10818-020-09295-4
Germerodt, S., Bohl, K., Lück, A., Pande, S., Schröter, A., Kaleta, C., et al. (2016). Pervasive selection for cooperative cross-feeding in bacterial communities. PLoS Comput. Biol. 12:e1004986. doi: 10.1371/journal.pcbi.1004986
Giovannoni, S. J., Thrash, J. C., and Temperton, B. (2014). Implications of streamlining theory for microbial ecology. ISME J. 8, 1553–1565. doi: 10.1038/ismej.2014.60
Godfrey-Smith, P. (2000). The replicator in retrospect. Biol. Philos. 15, 403–423. doi: 10.1023/A:1006704301415
Goel, A., Wortel, M. T., Molenaar, D., and Teusink, B. (2012). Metabolic shifts: a fitness perspective for microbial cell factories. Biotechnol. Lett. 34, 2147–2160. doi: 10.1007/s10529-012-1038-9
Gogarten, J. P., Doolittle, W. F., and Lawrence, J. G. (2002). Prokaryotic evolution in light of gene transfer. Mol. Biol. Evol. 19, 2226–2238. doi: 10.1093/oxfordjournals.molbev.a004046
Goldford, J. E., Lu, N., Bajic, D., Estrela, S., Tikhonov, M., Sanchez-Gorostiaga, A., et al. (2018). Emergent simplicity in microbial community assembly. Science 361, 469–474. doi: 10.1126/science.aat1168
Gomulkiewicz, R., Nuismer, S. L., and Thompson, J. N. (2003). Coevolution in variable mutualisms. Am. Nat. 162, S80–S93. doi: 10.1086/378705
Gore, J., Youk, H., and van Oudenaarden, A. (2009). Snowdrift game dynamics and facultative cheating in yeast. Nature 459, 253–256. doi: 10.1038/nature07921
Großkopf, T., Consuegra, J., Gaffé, J., Willison, J. C., Lenski, R. E., Soyer, O. S., et al. (2016). Metabolic modelling in a dynamic evolutionary framework predicts adaptive diversification of bacteria in a long-term evolution experiment. BMC Evol. Biol. 16:163. doi: 10.1186/s12862-016-0733-x
Großkopf, T., and Soyer, O. S. (2016). Microbial diversity arising from thermodynamic constraints. ISME J. 10, 2725–2733. doi: 10.1038/ismej.2016.49
Gudelj, I., Beardmore, R. E., Arkin, S. S., and Maclean, R. C. (2007). Constraints on microbial metabolism drive evolutionary diversification in homogeneous environments. J. Evol. Biol. 20, 1882–1889. doi: 10.1111/j.1420-9101.2007.01376.x
Gudelj, I., Kinnersley, M., Rashkov, P., Schmidt, K., and Rosenzweig, F. (2016). Stability of cross-feeding polymorphisms in microbial communities. PLoS Comput. Biol. 12:e1005269. doi: 10.1371/journal.pcbi.1005269
Gudelj, I., Weitz, J. S., Ferenci, T., Claire Horner-Devine, M., Marx, C. J., Meyer, J. R., et al. (2010). An integrative approach to understanding microbial diversity: from intracellular mechanisms to community structure: multi-scale drivers of diversity. Ecol. Lett. 13, 1073–1084. doi: 10.1111/j.1461-0248.2010.01507.x
Gutiérrez, G., Chistyakova, L. V., Villalobo, E., Kostygov, A. Y., and Frolov, A. O. (2017). Identification of Pelomyxa palustris endosymbionts. Protist 168, 408–424. doi: 10.1016/j.protis.2017.06.001
Hall-Stoodley, L., Costerton, J. W., and Stoodley, P. (2004). Bacterial biofilms: from the Natural environment to infectious diseases. Nat. Rev. Microbiol. 2, 95–108. doi: 10.1038/nrmicro821
Hansen, S. K., Rainey, P. B., Haagensen, J. A. J., and Molin, S. (2007). Evolution of species interactions in a biofilm community. Nature 445, 533–536. doi: 10.1038/nature05514
Harcombe, W. R., Betts, A., Shapiro, J. W., and Marx, C. J. (2016). Adding biotic complexity alters the metabolic benefits of mutualism. Evolution 70, 1871–1881. doi: 10.1111/evo.12973
Harcombe, W. R., Chacón, J. M., Adamowicz, E. M., Chubiz, L. M., and Marx, C. J. (2018). Evolution of bidirectional costly mutualism from byproduct consumption. Proc. Natl. Acad. Sci. U. S. A. 115, 12000–12004. doi: 10.1073/pnas.1810949115
Hays, S. G., Patrick, W. G., Ziesack, M., Oxman, N., and Silver, P. A. (2015). Better together: engineering and application of microbial symbioses. Curr. Opin. Biotechnol. 36, 40–49. doi: 10.1016/j.copbio.2015.08.008
Helling, R. B. (2002). Speed versus efficiency in microbial growth and the role of parallel pathways. J. Bacteriol. 184, 1041–1045. doi: 10.1128/jb.184.4.1041-1045.2002
Herre, E., Knowlton, N., Mueller, U., and Rehner, S. (1999). The evolution of mutualisms: exploring the paths between conflict and cooperation. Trends Ecol. Evol. 14, 49–53. doi: 10.1016/s0169-5347(98)01529-8
Hillesland, K. L. (2017). Evolution on the bright side of life: microorganisms and the evolution of mutualism. Ann. N. Y. Acad. Sci. 1422, 88–103. doi: 10.1111/nyas.13515
Hillesland, K. L., and Stahl, D. A. (2010). Rapid evolution of stability and productivity at the origin of a microbial mutualism. Proc. Natl. Acad. Sci. U. S. A. 107, 2124–2129. doi: 10.1073/pnas.0908456107
Hoek, T. A., Axelrod, K., Biancalani, T., Yurtsev, E. A., Liu, J., and Gore, J. (2016). Resource availability modulates the cooperative and competitive nature of a microbial cross-feeding mutualism. PLoS Biol. 14:e1002540. doi: 10.1371/journal.pbio.1002540
Hubbell, S. P. (2006). Neutral theory and the evolution of ecological equivalence. Ecology 87, 1387–1398.
Hünken, M., Harder, J., and Kirst, G. O. (2008). Epiphytic bacteria on the Antarctic ice diatom Amphiprora kufferathii Manguin cleave hydrogen peroxide produced during algal photosynthesis. Plant Biol. 10, 519–526. doi: 10.1111/j.1438-8677.2008.00040.x
Husnik, F., Tashyreva, D., Boscaro, V., George, E. E., Lukeš, J., and Keeling, P. J. (2021). Bacterial and archaeal symbioses with protists. Curr. Biol. 31, R862–R877. doi: 10.1016/j.cub.2021.05.049
Imachi, H., Nobu, M. K., Nakahara, N., Morono, Y., Ogawara, M., Takaki, Y., et al. (2020). Isolation of an archaeon at the prokaryote–eukaryote interface. Nature 577, 519–525. doi: 10.1038/s41586-019-1916-6
Jablonka, E., and Lamb, M. J. (1989). The inheritance of acquired epigenetic variations. J. Theor. Biol. 139, 69–83. doi: 10.1016/S0022-5193(89)80058-X
Jablonka, E., and Lamb, M. J. (1995). Epigenetic inheritance and evolution: the Lamarckian dimension. Oxford/New York: Oxford University Press.
Jablonka, E., Lamb, M. J., and Avital, E. (1998). ‘Lamarckian’ mechanisms in Darwinian evolution. Trends Ecol. Evol. 13, 206–210. doi: 10.1016/S0169-5347(98)01344-5
Jablonka, E., and Szathmáry, E. (1995). The evolution of information storage and heredity. Trends Ecol. Evol. 10, 206–211. doi: 10.1016/S0169-5347(00)89060-6
Jeon, K. W. (1995). The large, free-living amoebae: wonderful cells for biological studies. J. Eukaryot. Microbiol. 42, 1–7. doi: 10.1111/j.1550-7408.1995.tb01532.x
Jeon, K. W. (2004). Genetic and physiological interactions in the amoeba-bacteria symbiosis. J. Eukaryot. Microbiol. 51, 502–508. doi: 10.1111/j.1550-7408.2004.tb00277.x
Jimenez, P., and Scheuring, I. (2021). Density-dependent private benefit leads to bacterial mutualism. Evolution 75, 1619–1635. doi: 10.1111/evo.14241
John, P., and Whatley, F. R. (1975). Paracoccus denitrificans and the evolutionary origin of the mitochondrion. Nature 254, 495–498. doi: 10.1038/254495a0
Johnson, D. R., Goldschmidt, F., Lilja, E. E., and Ackermann, M. (2012). Metabolic specialization and the assembly of microbial communities. ISME J. 6, 1985–1991. doi: 10.1038/ismej.2012.46
Kallus, Y., Miller, J. H., and Libby, E. (2017). Paradoxes in leaky microbial trade. Nat. Commun. 8:1361. doi: 10.1038/s41467-017-01628-8
Katharios-Lanwermeyer, S., Xi, C., Jakubovics, N. S., and Rickard, A. H. (2014). Mini-review: microbial coaggregation: ubiquity and implications for biofilm development. Biofouling 30, 1235–1251. doi: 10.1080/08927014.2014.976206
Kato, S., Haruta, S., Cui, Z. J., Ishii, M., and Igarashi, Y. (2005). Stable coexistence of five bacterial strains as a cellulose-degrading community. Appl. Environ. Microbiol. 71, 7099–7106. doi: 10.1128/AEM.71.11.7099-7106.2005
Keeling, P. J. (2013). The number, speed, and impact of plastid endosymbioses in eukaryotic evolution. Annu. Rev. Plant Biol. 64, 583–607. doi: 10.1146/annurev-arplant-050312-120144
Keeling, P. J., and McCutcheon, J. P. (2017). Endosymbiosis: the feeling is not mutual. J. Theor. Biol. 434, 75–79. doi: 10.1016/j.jtbi.2017.06.008
Khandelwal, R. A., Olivier, B. G., Röling, W. F. M., Teusink, B., and Bruggeman, F. J. (2013). Community flux balance analysis for microbial consortia at balanced growth. PLoS One 8:e64567. doi: 10.1371/journal.pone.0064567
Kim, H. J., Boedicker, J. Q., Choi, J. W., and Ismagilov, R. F. (2008). Defined spatial structure stabilizes a synthetic multispecies bacterial community. Proc. Natl. Acad. Sci. U. S. A. 105, 18188–18193. doi: 10.1073/pnas.0807935105
Kolenbrander, P. E., Palmer, R. J., Periasamy, S., and Jakubovics, N. S. (2010). Oral multispecies biofilm development and the key role of cell-cell distance. Nat. Rev. Microbiol. 8, 471–480. doi: 10.1038/nrmicro2381
Kollock, P. (1998). Social dilemmas: the anatomy of cooperation. Annu. Rev. Sociol. 24, 183–214. doi: 10.1146/annurev.soc.24.1.183
Laland, K., Matthews, B., and Feldman, M. W. (2016). An introduction to niche construction theory. Evol. Ecol. 30, 191–202. doi: 10.1007/s10682-016-9821-z
Lengeler, J. W., Drews, G., and Schlegel, H. G. (1999). Biology of the Prokaryotes. New York: Georg Thieme Verlag.
Lenton, T. M., Kohler, T. A., Marquet, P. A., Boyle, R. A., Crucifix, M., Wilkinson, D. M., et al. (2021). Survival of the systems. Trends Ecol. Evol. 36, 333–344. doi: 10.1016/j.tree.2020.12.003
Leventhal, G. E., Boix, C., Kuechler, U., Enke, T. N., Sliwerska, E., Holliger, C., et al. (2018). Strain-level diversity drives alternative community types in millimetre-scale granular biofilms. Nat. Microbiol. 3, 1295–1303. doi: 10.1038/s41564-018-0242-3
Levin, S. A. (1998). Ecosystems and the Biosphere as Complex Adaptive Systems. Ecosystems 1, 431–436. doi: 10.1007/s100219900037
Levine, J. M., Bascompte, J., Adler, P. B., and Allesina, S. (2017). Beyond pairwise mechanisms of species coexistence in complex communities. Nature 546, 56–64. doi: 10.1038/nature22898
Lewontin, R. C. (1970). The units of selection. Annu. Rev. Ecol. Syst. 1, 1–18. doi: 10.1146/annurev.es.01.110170.000245
Libby, E., Hébert-Dufresne, L., Hosseini, S.-R., and Wagner, A. (2019). Syntrophy emerges spontaneously in complex metabolic systems. PLoS Comput. Biol. 15:e1007169. doi: 10.1371/journal.pcbi.1007169
Libby, E., and Ratcliff, W. C. (2021). Lichens and microbial syntrophies offer models for an interdependent route to multicellularity. Lichenologist 53, 283–290. doi: 10.1017/S0024282921000256
Lind, A. E., Lewis, W. H., Spang, A., Guy, L., Embley, T. M., and Ettema, T. J. G. (2018). Genomes of two archaeal endosymbionts show convergent adaptations to an intracellular lifestyle. ISME J. 12, 2655–2667. doi: 10.1038/s41396-018-0207-9
Liu, J., Prindle, A., Humphries, J., Gabalda-Sagarra, M., Asally, M., Lee, D. D., et al. (2015). Metabolic co-dependence gives rise to collective oscillations within biofilms. Nature 523, 550–554. doi: 10.1038/nature14660
López-García, P., and Moreira, D. (2020). The Syntrophy hypothesis for the origin of eukaryotes revisited. Nat. Microbiol. 5, 655–667. doi: 10.1038/s41564-020-0710-4
Loreau, M. (1998). Ecosystem development explained by competition within and between material cycles. Proc. R. Soc. B Biol. Sci. 265, 33–38. doi: 10.1098/rspb.1998.0260
Louca, S., Jacques, S. M. S., Pires, A. P. F., Leal, J. S., Srivastava, D. S., Parfrey, L. W., et al. (2016). High taxonomic variability despite stable functional structure across microbial communities. Nat. Ecol. Evol. 1:0015. doi: 10.1038/s41559-016-0015
Louca, S., Polz, M. F., Mazel, F., Albright, M. B. N., Huber, J. A., O’Connor, M. I., et al. (2018). Function and functional redundancy in microbial systems. Nat. Ecol. Evol. 2, 936–943. doi: 10.1038/s41559-018-0519-1
Lowe, C. D., Minter, E. J., Cameron, D. D., and Brockhurst, M. A. (2016). Shining a light on exploitative host control in a photosynthetic endosymbiosis. Curr. Biol. 26, 207–211. doi: 10.1016/j.cub.2015.11.052
Lyons, N. A., and Kolter, R. (2015). On the evolution of bacterial multicellularity. Curr. Opin. Microbiol. 24, 21–28. doi: 10.1016/j.mib.2014.12.007
Machado, D., Maistrenko, O. M., Andrejev, S., Kim, Y., Bork, P., Patil, K. R., et al. (2021). Polarization of microbial communities between competitive and cooperative metabolism. Nat. Ecol. Evol. 5, 195–203. doi: 10.1038/s41559-020-01353-4
MacLean, R. C. (2008). The tragedy of the commons in microbial populations: insights from theoretical, comparative and experimental studies. Heredity 100, 233–239. doi: 10.1038/sj.hdy.6801073
Madigan, M. T., Martinko, J. M., Bender, K. S., Buckley, D. H., and Stahl, D. A. (2014). Brock biology of microorganisms, 14th Edn. San Francisco: Benjamin Cummings.
Madsen, J. S., Burmølle, M., Hansen, L. H., and Sørensen, S. J. (2012). The interconnection between biofilm formation and horizontal gene transfer. FEMS Immunol. Med. Microbiol. 65, 183–195. doi: 10.1111/j.1574-695x.2012.00960.x
Margulis, L. (1981). Symbiosis in cell evolution: life and its environment on the early earth. San Francisco: Freeman.
Martijn, J., and Ettema, T. J. G. (2013). From archaeon to eukaryote: the evolutionary dark ages of the eukaryotic cell. Biochem. Soc. Trans. 41, 451–457. doi: 10.1042/BST20120292
Martin, W. F., Garg, S., and Zimorski, V. (2015). Endosymbiotic theories for eukaryote origin. Philos. Trans. R. Soc. Lond. B Biol. Sci. 370, 1–18. doi: 10.1098/rstb.2014.0330
Martínez, I., Mohamed, M. E.-S., Rozas, D., García, J. L., and Díaz, E. (2016). Engineering synthetic bacterial consortia for enhanced desulfurization and revalorization of oil sulfur compounds. Metab. Eng. 35, 46–54. doi: 10.1016/j.ymben.2016.01.005
Martínez-Cano, D. J., Reyes-Prieto, M., Martínez-Romero, E., Partida-Martinez, L. P., Latorre, A., Moya, A., et al. (2015). Evolution of small prokaryotic genomes. Front. Microbiol. 5:742. doi: 10.3389/fmicb.2014.00742
Matsumura, S., Kun, Á, Ryckelynck, M., Coldren, F., Szilágyi, A., Jossinet, F., et al. (2016). Transient compartmentalization of RNA replicators prevents extinction due to parasites. Science 354, 1293–1296. doi: 10.1126/science.aag1582
Maynard Smith, J. (1979). Hypercycles and the origin of life. Nature 280, 445–446. doi: 10.1038/280445a0
Maynard Smith, J. (1987). “How to model evolution,” in The latest on the best: essays on evolution and optimality, ed. J. Dupré (Cambridge, MA: MIT Press), 119–131.
Maynard Smith, J. (1991). “A Darwinian view of symbiosis,” in Symbiosis as a source of evolutionary innovation: speciation and morphogenesis, eds L. Margulis and R. Fester (Cambridge, MA: MIT Press), 26–39.
Maynard Smith, J., and Szathmáry, E. (1995). The major transitions in evolution. Oxford: Freeman & Co.
McCarty, N. S., and Ledesma-Amaro, R. (2019). Synthetic biology tools to engineer microbial communities for biotechnology. Trends Biotechnol. 37, 181–197. doi: 10.1016/j.tibtech.2018.11.002
Mee, M. T., Collins, J. J., Church, G. M., and Wang, H. H. (2014). Syntrophic exchange in synthetic microbial communities. Proc. Natl. Acad. Sci. U. S. A. 111, E2149–E2156. doi: 10.1073/pnas.1405641111
Meijer, J., van Dijk, B., and Hogeweg, P. (2020). Contingent evolution of alternative metabolic network topologies determines whether cross-feeding evolves. Commun. Biol. 3:401. doi: 10.1038/s42003-020-1107-x
Michod, R. E., and Nedelcu, A. M. (2003). On the reorganization of fitness during evolutionary transitions in individuality. Integr. Comp. Biol. 43, 64–73. doi: 10.1093/icb/43.1.64
Mills, D. B. (2020). The origin of phagocytosis in Earth history. Interface Focus 10:20200019. doi: 10.1098/rsfs.2020.0019
Mitri, S., and Foster, K. R. (2013). The genotypic view of social interactions in microbial communities. Annu. Rev. Genet. 47, 247–273. doi: 10.1146/annurev-genet-111212-133307
Moran, N. A., and Sloan, D. B. (2015). The hologenome concept: helpful or hollow? PLoS Biol. 13:e1002311. doi: 10.1371/journal.pbio.1002311
Mori, M., de-León, M. P., Peretó, J., and Montero, F. (2016). Metabolic complementation in bacterial communities: necessary conditions and optimality. Front. Microbiol. 7:1553. doi: 10.3389/fmicb.2016.01553
Morris, B. E. L., Henneberger, R., Huber, H., and Moissl-Eichinger, C. (2013). Microbial syntrophy: interaction for the common good. FEMS Microbiol. Rev. 37, 384–406. doi: 10.1111/1574-6976.12019
Morris, J. J., Lenski, R. E., and Zinser, E. R. (2012). The Black Queen Hypothesis: evolution of dependencies through adaptive gene loss. mBio 3, e00036–12. doi: 10.1128/mbio.00036-12
Nadell, C. D., Xavier, J. B., and Foster, K. R. (2009). The sociobiology of biofilms. FEMS Microbiol. Rev. 33, 206–224. doi: 10.1111/j.1574-6976.2008.00150.x
Nelsen, M. P., Lücking, R., Boyce, C. K., Lumbsch, H. T., and Ree, R. H. (2020). The macroevolutionary dynamics of symbiotic and phenotypic diversification in lichens. Proc. Natl. Acad. Sci. U. S. A. 117, 21495–21503. doi: 10.1073/pnas.2001913117
Netzker, T., Shepherdson, E. M. F., Zambri, M. P., and Elliot, M. A. (2020). Bacterial volatile compounds: functions in communication, cooperation, and competition. Annu. Rev. Microbiol. 74, 409–430. doi: 10.1146/annurev-micro-011320-015542
Nowack, E. C. M., and Melkonian, M. (2010). Endosymbiotic associations within protists. Philos. Trans. R. Soc. B Biol. Sci. 365, 699–712. doi: 10.1098/rstb.2009.0188
Nowak, M. A. (2006). Five rules for the evolution of cooperation. Science 314, 1560–1563. doi: 10.1126/science.1133755
O’Brien, A. M., Jack, C. N., Friesen, M. L., and Frederickson, M. E. (2021). Whose trait is it anyways? Coevolution of joint phenotypes and genetic architecture in mutualisms. Proc. R. Soc. B Biol. Sci. 288:20202483. doi: 10.1098/rspb.2020.2483
Odling-Smee, J., Laland, K. N., and Feldman, M. W. (2003). Niche construction. The neglected process in evolution. Princeton/Oxford: Princeton University Press.
Odum, E. P. (1969). The strategy of ecosystem development. Science 164, 262–270. doi: 10.1126/science.164.3877.262
Okasha, S. (2005). Multilevel selection and the major transitions in evolution. Philos. Sci. 72, 1013–1025. doi: 10.1086/508102
O’Malley, M. A. (2015). Endosymbiosis and its implications for evolutionary theory. Proc. Natl. Acad. Sci. U. S. A. 112, 10270–10277. doi: 10.1073/pnas.1421389112
Oña, L., Giri, S., Avermann, N., Kreienbaum, M., Thormann, K. M., and Kost, C. (2021). Obligate cross-feeding expands the metabolic niche of bacteria. Nat. Ecol. Evol. 5, 1224–1232. doi: 10.1038/s41559-021-01505-0
Pande, S., and Kost, C. (2017). Bacterial unculturability and the formation of intercellular metabolic networks. Trends Microbiol. 25, 349–361. doi: 10.1016/j.tim.2017.02.015
Pande, S., Merker, H., Bohl, K., Reichelt, M., Schuster, S., de Figueiredo, L. F., et al. (2013). Fitness and stability of obligate cross-feeding interactions that emerge upon gene loss in bacteria. ISME J. 8, 953–962. doi: 10.1038/ismej.2013.211
Pearman, P. B., Guisan, A., Broennimann, O., and Randin, C. F. (2008). Niche dynamics in space and time. Trends Ecol. Evol. 23, 149–158. doi: 10.1016/j.tree.2007.11.005
Periasamy, S., and Kolenbrander, P. E. (2009). Mutualistic biofilm communities develop with porphyromonas gingivalis and initial, early, and late colonizers of enamel. J. Bacteriol. 191, 6804–6811. doi: 10.1128/jb.01006-09
Pfeiffer, T., and Bonhoeffer, S. (2004). Evolution of cross-feeding in microbial populations. Am. Nat. 163, E126–E135. doi: 10.1086/383593
Pfeiffer, T., and Schuster, S. (2005). Game-theoretical approaches to studying the evolution of biochemical systems. Trends Biochem. Sci. 30, 20–25. doi: 10.1016/j.tibs.2004.11.006
Pfeiffer, T., Schuster, S., and Bonhoeffer, S. (2001). Cooperation and competition in the evolution of ATP-producing pathways. Science 292, 504–507. doi: 10.1126/science.1058079
Ponomarova, O., Gabrielli, N., Sévin, D. C., Mülleder, M., Zirngibl, K., Bulyha, K., et al. (2017). Yeast creates a niche for symbiotic lactic acid bacteria through nitrogen overflow. Cell Syst. 5, 345–357.e6. doi: 10.1016/j.cels.2017.09.002
Popat, R., Crusz, S. A., Messina, M., Williams, P., West, S. A., and Diggle, S. P. (2012). Quorum-sensing and cheating in bacterial biofilms. Proc. R. Soc. B Biol. Sci. 279, 4765–4771. doi: 10.1098/rspb.2012.1976
Preussger, D., Giri, S., Muhsal, L. K., Oña, L., and Kost, C. (2020). Reciprocal fitness feedbacks promote the evolution of mutualistic cooperation. Curr. Biol. 30, 3580–3590.e7. doi: 10.1016/j.cub.2020.06.100
Queller, D. C. (2000). Relatedness and the fraternal major transitions. Philos. Trans. R. Soc. Lond. B Biol. Sci. 355, 1647–1655. doi: 10.1098/rstb.2000.0727
Rainey, P. B., and Rainey, K. (2003). Evolution of cooperation and conflict in experimental bacterial populations. Nature 425, 72–74. doi: 10.1038/nature01906
Ratzke, C., Barrere, J., and Gore, J. (2020). Strength of species interactions determines biodiversity and stability in microbial communities. Nat. Ecol. Evol. 4, 376–383. doi: 10.1038/s41559-020-1099-4
Ren, D., Madsen, J. S., Sørensen, S. J., and Burmølle, M. (2015). High prevalence of biofilm synergy among bacterial soil isolates in cocultures indicates bacterial interspecific cooperation. ISME J. 9, 81–89. doi: 10.1038/ismej.2014.96
Robin, A. N., Denton, K. K., Lowell, E. S. H., Dulay, T., Ebrahimi, S., Johnson, G. C., et al. (2021). Major evolutionary transitions and the roles of facilitation and information in ecosystem transformations. Front. Ecol. Evol. 9:711556. doi: 10.3389/fevo.2021.711556
Rosindell, J., Hubbell, S. P., and Etienne, R. S. (2011). The unified neutral theory of biodiversity and biogeography at age ten. Trends Ecol. Evol. 26, 340–348. doi: 10.1016/j.tree.2011.03.024
Rumbaugh, K. P., and Sauer, K. (2020). Biofilm dispersion. Nat. Rev. Microbiol. 18, 571–586. doi: 10.1038/s41579-020-0385-0
Sachs, J. L., Mueller, U. G., Wilcox, T. P., and Bull, J. J. (2004). The evolution of cooperation. Q. Rev. Biol. 79, 135–160. doi: 10.1086/383541
Sachs, J. L., Skophammer, R. G., Bansal, N., and Stajich, J. E. (2013). Evolutionary origins and diversification of proteobacterial mutualists. Proc. R. Soc. B Biol. Sci. 281:0132146. doi: 10.1098/rspb.2013.2146
Sachs, J. L., Skophammer, R. G., and Regus, J. U. (2011). Evolutionary transitions in bacterial symbiosis. Proc. Natl. Acad. Sci. U. S. A. 108, 10800–10807. doi: 10.1073/pnas.1100304108
San Roman, M., and Wagner, A. (2018). An enormous potential for niche construction through bacterial cross-feeding in a homogeneous environment. PLoS Comput. Biol. 14:e1006340. doi: 10.1371/journal.pcbi.1006340
Schink, B. (2002). Synergistic interactions in the microbial world. Antonie van Leeuwenhoek 81, 257–261. doi: 10.1023/a:1020579004534
Searcy, D. G. (1992). “Origins of mitochondria and chloroplasts from sulfur-based symbioses,” in The origin and evolution of the cell, eds H. Hartman and K. Matsuno (Singapore: World Scientific), 47–78.
Searcy, D. G. (2002). “Nutritional syntrophies and consortia as models for the origin of mitochondria,” in Symbiosis: mechanisms and Model Systems, ed. J. Seckbach (Dordrecht: Springer Netherlands), 163–183. doi: 10.1007/0-306-48173-1_10
Searcy, D. G. (2014). Elemental sulfur reduction by eukaryotic cytoplasm consistent with an ancient sulfur symbiosis. Nitric Oxide 39:S13. doi: 10.1016/j.niox.2014.03.048
Searcy, D. G., Stein, D. B., and Green, G. R. (1978). Phylogenetic affinities between eukaryotic cells and a thermophilic mycoplasma. Biosystems 10, 19–28. doi: 10.1016/0303-2647(78)90024-2
Seiler, C., van Velzen, E., Neu, T. R., Gaedke, U., Berendonk, T. U., and Weitere, M. (2017). Grazing resistance of bacterial biofilms: a matter of predators’ feeding trait. FEMS Microbiol. Ecol. 93:fix112. doi: 10.1093/femsec/fix112
Shou, W., Ram, S., and Vilar, J. M. G. (2007). Synthetic cooperation in engineered yeast populations. Proc. Natl. Acad. Sci. U. S. A. 104, 1877–1882. doi: 10.1073/pnas.0610575104
Sloan, W. T., Lunn, M., Woodcock, S., Head, I. M., Nee, S., and Curtis, T. P. (2006). Quantifying the roles of immigration and chance in shaping prokaryote community structure. Environ. Microbiol. 8, 732–740. doi: 10.1111/j.1462-2920.2005.00956.x
Smith, N. W., Shorten, P. R., Altermann, E., Roy, N. C., and McNabb, W. C. (2019). The classification and evolution of bacterial cross-feeding. Front. Ecol. Evol. 7:153. doi: 10.3389/fevo.2019.00153
Smith, P., and Schuster, M. (2019). Public goods and cheating in microbes. Curr. Biol. 29, R442–R447. doi: 10.1016/j.cub.2019.03.001
Solano, C., Echeverz, M., and Lasa, I. (2014). Biofilm dispersion and quorum sensing. Curr. Opin. Microbiol. 18, 96–104. doi: 10.1016/j.mib.2014.02.008
Song, W., Wemheuer, B., Steinberg, P. D., Marzinelli, E. M., and Thomas, T. (2021). Contribution of horizontal gene transfer to the functionality of microbial biofilm on a macroalgae. ISME J. 15, 807–817. doi: 10.1038/s41396-020-00815-8
Sørensen, M. E. S., Lowe, C. D., Minter, E. J. A., Wood, A. J., Cameron, D. D., and Brockhurst, M. A. (2019). The role of exploitation in the establishment of mutualistic microbial symbioses. FEMS Microbiol. Lett. 366:fnz148. doi: 10.1093/femsle/fnz148
Sousa, F. L., Neukirchen, S., Allen, J. F., Lane, N., and Martin, W. F. (2016). Lokiarchaeon is hydrogen dependent. Nat. Microbiol. 1:16034. doi: 10.1038/nmicrobiol.2016.34
Spang, A., Stairs, C. W., Dombrowski, N., Eme, L., Lombard, J., Caceres, E. F., et al. (2019). Proposal of the reverse flow model for the origin of the eukaryotic cell based on comparative analyses of Asgard archaeal metabolism. Nat. Microbiol. 4, 1138–1148. doi: 10.1038/s41564-019-0406-9
Speijer, D. (2021). Zombie ideas about early endosymbiosis: which entry mechanisms gave us the “endo” in different endosymbionts? BioEssays 43:2100069. doi: 10.1002/bies.202100069
Staley, J. T., and Konopka, A. (1985). Measurement of in situ activities of nonphotosynthetic microorganisms in aquatic and terrestrial habitats. Annu. Rev. Microbiol. 39, 321–346. doi: 10.1146/annurev.mi.39.100185.001541
Stams, A. J. M., de Bok, F. A. M., Plugge, C. M., Eekert, M. H. A., van, Dolfing, J., et al. (2006). Exocellular electron transfer in anaerobic microbial communities. Environ. Microbiol. 8, 371–382. doi: 10.1111/j.1462-2920.2006.00989.x
Stams, A. J. M., and Plugge, C. M. (2009). Electron transfer in syntrophic communities of anaerobic bacteria and archaea. Nat. Rev. Microbiol. 7, 568–577. doi: 10.1038/nrmicro2166
Stegen, J. C., Lin, X., Konopka, A. E., and Fredrickson, J. K. (2012). Stochastic and deterministic assembly processes in subsurface microbial communities. ISME J. 6, 1653–1664. doi: 10.1038/ismej.2012.22
Stephens, W. Z., Wiles, T. J., Martinez, E. S., Jemielita, M., Burns, A. R., Parthasarathy, R., et al. (2015). Identification of population bottlenecks and colonization factors during assembly of bacterial communities within the zebrafish intestine. mBio 6, e01163–15. doi: 10.1128/mbio.01163-15
Stolz, J. F. (2016). Gaia and her microbiome. FEMS Microbiol. Ecol. 93:fiw247. doi: 10.1093/femsec/fiw247
Stoodley, P., Sauer, K., Davies, D. G., and Costerton, J. W. (2002). Biofilms as complex differentiated communities. Annu. Rev. Microbiol. 56, 187–209. doi: 10.1146/annurev.micro.56.012302.160705
Stoodley, P., Wilson, S., Hall-Stoodley, L., Boyle, J. D., Lappin-Scott, H. M., and Costerton, J. W. (2001). Growth and detachment of cell clusters from mature mixed-species biofilms. Appl. Environ. Microbiol. 67, 5608–5613. doi: 10.1128/aem.67.12.5608-5613.2001
Stump, S. M., Johnson, E. C., Sun, Z., and Klausmeier, C. A. (2018). How spatial structure and neighbor uncertainty promote mutualists and weaken black queen effects. J. Theor. Biol. 446, 33–60. doi: 10.1016/j.jtbi.2018.02.031
Stump, S. M., and Klausmeier, C. A. (2016). Competition and coexistence between a syntrophic consortium and a metabolic generalist, and its effect on productivity. J. Theor. Biol. 404, 348–360. doi: 10.1016/j.jtbi.2016.06.019
Summers, Z. M., Fogarty, H. E., Leang, C., Franks, A. E., Malvankar, N. S., and Lovley, D. R. (2010). Direct exchange of electrons within aggregates of an evolved syntrophic coculture of anaerobic bacteria. Science 330, 1413–1415. doi: 10.1126/science.1196526
Szathmáry, E. (2000). The evolution of replicators. Philos. Trans. R. Soc. Lond. B Biol. Sci. 355, 1669–1676. doi: 10.1098/rstb.2000.0730
Szathmáry, E. (2013). On the propagation of a conceptual error concerning hypercycles and cooperation. J. Syst. Chem. 4:1. doi: 10.1186/1759-2208-4-1
Szathmáry, E. (2015). Toward major evolutionary transitions theory 2.0. Proc. Natl. Acad. Sci. U. S. A. 112, 10104–10111. doi: 10.1073/pnas.1421398112
Szathmáry, E., and Maynard Smith, J. (1995). The major evolutionary transitions. Nature 374, 227–232. doi: 10.1038/374227a0
Szilágyi, A., Zachar, I., Scheuring, I., Kun, Á, Könnyű, B., and Czárán, T. (2017). Ecology and evolution in the RNA World: dynamics and stability of prebiotic replicator systems. Life 7:48. doi: 10.3390/life7040048
Tarnita, C. E. (2017). The ecology and evolution of social behavior in microbes. J. Exp. Biol. 220, 18–24. doi: 10.1242/jeb.145631
Tsoi, R., Wu, F., Zhang, C., Bewick, S., Karig, D., and You, L. (2018). Metabolic division of labor in microbial systems. Proc. Natl. Acad. Sci. U. S. A. 115, 2526–2531. doi: 10.1073/pnas.1716888115
Ulanowicz, R. E. (1995). Utricularia’s secret: the advantage of positive feedback in oligotrophic environments. Ecol. Model. 79, 49–57. doi: 10.1016/0304-3800(94)00032-d
Ulanowicz, R. E., Holt, R. D., and Barfield, M. (2014). Limits on ecosystem trophic complexity: insights from ecological network analysis. Ecol. Lett. 17, 127–136. doi: 10.1111/ele.12216
van Gestel, J., Vlamakis, H., and Kolter, R. (2015). Division of labor in biofilms: the ecology of cell differentiation. Microbiol. Spectr. 3:MB–0002–2014. doi: 10.1128/microbiolspec.mb-0002-2014
Van Hoek, M. J. A., and Merks, R. M. H. (2017). Emergence of microbial diversity due to cross-feeding interactions in a spatial model of gut microbial metabolism. BMC Syst. Biol. 11:56. doi: 10.1186/s12918-017-0430-4
van Tatenhove-Pel, R. J., de Groot, D. H., Bisseswar, A. S., Teusink, B., and Bachmann, H. (2021). Population dynamics of microbial cross-feeding are determined by co-localization probabilities and cooperation-independent cheater growth. ISME J. 15, 3050–3061. doi: 10.1038/s41396-021-00986-y
Veldhuis, M. P., Berg, M. P., Loreau, M., and Olff, H. (2018). Ecological autocatalysis: a central principle in ecosystem organization? Ecol. Monogr. 88, 304–319. doi: 10.1002/ecm.1292
Wagener, S., and Pfennig, N. (1987). Monoxenic culture of the anaerobic ciliate Trimyema compressum Lackey. Arch. Microbiol. 149, 4–11. doi: 10.1007/bf00423128
Weinbauer, M. G. (2004). Ecology of prokaryotic viruses. FEMS Microbiol. Rev. 28, 127–181. doi: 10.1016/j.femsre.2003.08.001
Weinersmith, K. L., and Earley, R. L. (2016). Better with your parasites? Lessons for behavioural ecology from evolved dependence and conditionally helpful parasites. Anim. Behav. 118, 123–133. doi: 10.1016/j.anbehav.2016.06.004
West, S. A., and Cooper, G. A. (2016). Division of labour in microorganisms: an evolutionary perspective. Nat. Rev. Microbiol. 14, 716–723. doi: 10.1038/nrmicro.2016.111
West, S. A., Griffin, A. S., and Gardner, A. (2007b). Social semantics: altruism, cooperation, mutualism, strong reciprocity and group selection. J. Evol. Biol. 20, 415–432. doi: 10.1111/j.1420-9101.2006.01258.x
West, S. A., Diggle, S. P., Buckling, A., Gardner, A., and Griffin, A. S. (2007a). The social lives of microbes. Annu. Rev. Ecol. Evol. Syst. 38, 53–77. doi: 10.1146/annurev.ecolsys.38.091206.095740
West, S. A., Fisher, R. M., Gardner, A., and Kiers, E. T. (2015). Major evolutionary transitions in individuality. Proc. Natl. Acad. Sci. U. S. A. 112, 10112–10119. doi: 10.1073/pnas.1421402112
West, S. A., Griffin, A. S., Gardner, A., and Diggle, S. P. (2006). Social evolution theory for microorganisms. Nat. Rev. Microbiol. 4, 597–607. doi: 10.1038/nrmicro1461
White, J. F., Kingsley, K., Harper, C. J., Verma, S. K., Brindisi, L., Chen, Q., et al. (2018). “Reactive oxygen defense against cellular endoparasites and the origin of eukaryotes,” in Transformative Paleobotany, eds M. Krings, C. Harper, N. Cuneo, and G. Rothwell (Amsterdam: Elsevier), 439–460. doi: 10.1016/b978-0-12-813012-4.00018-8
Williams, G. C. (1966). Adaptation and natural selection: a critique of some current evolutionary thought. Princeton, NJ: Princeton University Press.
Wilson, D. S. (1975). A theory of group selection. Proc. Natl. Acad. Sci. U. S. A. 72, 143–146. doi: 10.1073/pnas.72.1.143
Wilson, D. S. (1979). Structured demes and trait-group variation. Am. Nat. 113, 606–610. doi: 10.1086/283417
Wintermute, E. H., and Silver, P. A. (2010). Emergent cooperation in microbial metabolism. Mol. Syst. Biol. 6:407. doi: 10.1038/msb.2010.66
Wolfe, R. S., and Pfennig, N. (1977). Reduction of sulfur by spirillum 5175 and syntrophism with Chlorobium. Appl. Environ. Microbiol. 33, 427–433. doi: 10.1128/aem.33.2.427-433.1977
Xie, L., and Shou, W. (2021). Steering ecological-evolutionary dynamics to improve artificial selection of microbial communities. Nat. Commun. 12:6799. doi: 10.1038/s41467-021-26647-4
Yang, D.-D., Alexander, A., Kinnersley, M., Cook, E., Caudy, A., Rosebrock, A., et al. (2020). Fitness and productivity increase with ecotypic diversity among Escherichia coli strains that coevolved in a simple, constant environment. Appl. Environ. Microbiol. 86, e00051–20. doi: 10.1128/AEM.00051-20
Yurtsev, E. A., Chao, H. X., Datta, M. S., Artemova, T., and Gore, J. (2013). Bacterial cheating drives the population dynamics of cooperative antibiotic resistance plasmids. Mol. Syst. Biol. 9:683. doi: 10.1038/msb.2013.39
Zachar, I., and Boza, G. (2020). Endosymbiosis before eukaryotes: mitochondrial establishment in protoeukaryotes. Cell. Mol. Life Sci. 77, 3503–3523. doi: 10.1007/s00018-020-03462-6
Zachar, I., and Szathmáry, E. (2010). A new replicator: a theoretical framework for analyzing replication. BMC Biol. 8:21. doi: 10.1186/1741-7007-8-21
Zachar, I., and Szathmáry, E. (2017). Breath-giving cooperation: critical review of origin of mitochondria hypotheses. Biol. Direct 12:19. doi: 10.1186/s13062-017-0190-5
Zachar, I., Szilágyi, A., Számadó, S., and Szathmáry, E. (2018). Farming the mitochondrial ancestor as a model of endosymbiotic establishment by natural selection. Proc. Natl. Acad. Sci. U. S. A. 115, E1504–E1510. doi: 10.1073/pnas.1718707115
Zengler, K., and Zaramela, L. S. (2018). The social network of microorganisms - How auxotrophies shape complex communities. Nat. Rev. Microbiol. 16, 383–390. doi: 10.1038/s41579-018-0004-5
Keywords: cross-feeding, syntrophy, endosymbiosis, social evolution theory, origin of mitochondria, cooperation, public goods, major evolutionary transitions
Citation: Zachar I and Boza G (2022) The Evolution of Microbial Facilitation: Sociogenesis, Symbiogenesis, and Transition in Individuality. Front. Ecol. Evol. 10:798045. doi: 10.3389/fevo.2022.798045
Received: 19 October 2021; Accepted: 25 February 2022;
Published: 13 April 2022.
Edited by:
Karen Marie Kapheim, Utah State University, United StatesReviewed by:
Philippe Vandenkoornhuyse, University of Rennes 1, FrancePeter Conlin, Georgia Institute of Technology, United States
Copyright © 2022 Zachar and Boza. This is an open-access article distributed under the terms of the Creative Commons Attribution License (CC BY). The use, distribution or reproduction in other forums is permitted, provided the original author(s) and the copyright owner(s) are credited and that the original publication in this journal is cited, in accordance with accepted academic practice. No use, distribution or reproduction is permitted which does not comply with these terms.
*Correspondence: Gergely Boza, Ym96YS5nZXJnZWx5QGVjb2xyZXMuaHU=