- 1Division of Ecology and Evolution, Research School of Biology, Australian National University, Canberra, ACT, Australia
- 2CSIRO Land and Water, Wembley, WA, Australia
Anthropogenic climate change is increasing the frequency and intensity of heat waves, thereby threatening biodiversity, particularly in hot, arid regions. Although free-ranging endotherms can use behavioral thermoregulation to contend with heat, it remains unclear to what degree behavior can buffer organisms from unprecedented temperatures. Thermoregulatory behaviors that facilitate dry heat loss during moderate heat become maladaptive once environmental temperatures exceed body temperature. Additionally, the costs associated with behavioral thermoregulation may become untenable with greater heat exposure, and effective cooling may be dependent upon the availability of specific microhabitats. Only by understanding the interplay of these three elements (responses, costs and habitat) can we hope to accurately predict how heat waves will impact wild endotherms. We quantified the thermoregulatory behaviors and microhabitat use of a small passerine, the Jacky Winter (Microeca fascinans), in the mallee woodland of SE Australia. At this location, the annual number of days ≥ 42°C has doubled over the last 25 years. The birds’ broad repertoire of behavioral responses to heat was nuanced and responsive to environmental conditions, but was associated with reduced foraging effort and increased foraging costs, accounting for the loss of body condition that occurs at high temperatures. By measuring microsite surface temperatures, which varied by up to 35°C at air temperatures > 44°C, we found that leaf-litter coverage and tree size were positively correlated with thermal buffering. Large mallee eucalypts were critical to the birds’ response to very high temperatures, providing high perches that facilitated convective cooling, the coolest tree-base temperatures and the greatest prevalence of tree-base crevices or hollows that were used as refuges at air temperatures > 38°C. Tree-base hollows, found only in large mallees, were cooler than all other microsites, averaging 2°C cooler than air temperature. Despite the plasticity of the birds’ response to heat, 29% of our habituated study population died when air temperatures reached a record-breaking 49°C, demonstrating the limits of behavioral thermoregulation and the potential vulnerability of organisms to climate change.
Introduction
Anthropogenic climate change has led to an increase in the frequency and intensity of extreme weather events, particularly heat waves (Stillman, 2019). Record-breaking temperatures are having severe consequences for biodiversity, with mass mortality events (Welbergen et al., 2008; McKechnie and Wolf, 2010; McKechnie et al., 2021), the loss of arid-zone communities (Riddell et al., 2019) and reduced population viability (Thomas et al., 2006; Ruthrof et al., 2018; Stillman, 2019).
Physiologically informed models predict severe losses for birds in hot, arid environments due to acute heat stress (Conradie et al., 2020), but they do not take into account the positive effect of many thermoregulatory behaviors, which may be substantial based on the few studies that have evaluated the survival of wild individuals during heatwaves (Cooper et al., 2019; Sharpe et al., 2019). At high temperatures, behavioral thermoregulation serves two functions: the minimization of heat gain and the facilitation of heat dissipation. Given that solar radiation is the primary source of heat load (Mitchell et al., 2018), with 55% of the sun’s energy comprised of non-visible radiant heat (Stuart-Fox et al., 2017), avoiding sun exposure through shade use and altered activity time budgets is an almost universal behavioral response (Hetem et al., 2012; Hall and Chalfoun, 2019). However, this is only a partial solution because reflected solar radiation and radiant heat emanating from sun-warmed surfaces also contribute to heat load. Air temperatures that exceed body temperature also result in heat gain, which endotherms avoid by using cooler microhabitats (Williams et al., 1999; Walde et al., 2009; Carroll et al., 2015; Ruth et al., 2020) but this strategy relies upon thermal heterogeneity within the organism’s environment. Behaviors that curtail metabolic heat production, such as inactivity and fasting, also reduce heat gain (Beale et al., 2018) but may adversely impact energy balance (Youngentob et al., 2021).
At high environmental temperatures, endotherms must dissipate heat. They can do this via sensible (dry) heat transfer or latent (evaporative) heat transfer. All forms of dry heat transfer (conduction, convection and radiation) depend upon the existence of a temperature gradient between the surface of the animal and its environment: the steeper the gradient, the more effective the heat transfer. Conductive cooling occurs when objects of differing temperature touch, and it is enhanced by maximizing bodily contact with cool, poorly insulated substrates, such as sprawling in newly excavated sand, hugging bare tree trunks or clasping cool perches (Dawson, 1973; Muiruri and Harrison, 1991; Briscoe et al., 2014). Convective heat transfer occurs via the currents within a fluid or gas (e.g., air) which are generated by temperature-related differences in the medium’s density. Losing heat via convection is impaired by an endotherm’s plumage or pelage and tends to be ineffectual unless the velocity of the air flow is artificially increased, by wind or rapid movement (i.e., “forced” convective cooling; Mitchell et al., 2018). Long, narrow objects and those with a large surface area to mass ratio, lose heat more rapidly (Mitchell et al., 2018), so animals can maximize convective cooling by altering their posture and orientation, reducing the insulative properties of their fur or feathers by ptilo- or piloretraction, and by exploiting windy microsites. In radiant heat transfer, animals lose heat by emitting infrared electromagnetic waves. Although mechanisms that maximize radiant heat loss are usually under autonomic control (e.g., the vasodilation of capillaries in uninsulated body parts such as beaks, ears, legs or facial skin; Tattersall et al., 2009; Weissenböck et al., 2010), the efficiency of these thermal windows can be enhanced behaviorally via movement (e.g., ear flapping), changes in posture and orientation, and by microsite choice.
Once environmental temperatures equal or exceed body temperature, interactive strategies that facilitate sensible (dry) cooling will become detrimental, serving to expediate the acquisition of heat instead. Although endotherms can use regulated hyperthermia (allowing body temperature to rise above normal levels) to maximize and prolong the efficiency of dry cooling mechanisms (Tieleman and Williams, 1999), there is a limit to how high body temperature can safely rise (McKechnie and Wolf, 2019). When environmental temperature exceeds body temperature, organisms must resort to evaporative (or latent) cooling to prevent lethal heat stress. Behaviors that facilitate evaporative cooling include bathing, mud-wallowing, licking saliva on the body and urohidrosis (using excreta to wet parts of the body), while the primary physiological mechanisms are panting, sweating in some mammals, or gular fluttering in some birds (Ingram, 1965; Dawson, 1973; Arad et al., 1989; Mole et al., 2016; McKechnie and Wolf, 2019). Rates of evaporative cooling can be accelerated by behaviors that enhance convective heat loss, but, for animals with limited access to water, the loss of body water poses a serious dehydration risk (Conradie et al., 2020). During prolonged heat exposure, behavioral strategies that constrain evaporative water loss to levels required for thermal homeostasis will aid survival, and animals may thus seek out microsites with lower wind velocity and greater humidity (Walde et al., 2009; Luna et al., 2020).
Although we know that endotherms can adopt a wide array of thermoregulatory behaviors, the breadth of individual species’ behavioral repertoires are largely unknown, especially in small species, and it is unclear to what degree responses to heat are flexible or fixed. The extent to which organisms can modify their thermoregulatory behavior under global warming is critical, given that behavioral adjustment can buffer organisms from lethal effects and provide time for genetic adaptations to evolve (Tuomainen and Candolin, 2011). There are, however, limitations. Firstly, an organism’s behavioral repertoire is the product of selection pressures generated by past environments, so its responses may be insufficient, or simply inappropriate, to meet novel conditions (Tuomainen and Candolin, 2011). Many thermoregulatory behaviors cannot simply be “scaled up” in response to increasing temperature because behaviors that facilitate dry heat loss in moderate heat are maladaptive (promoting heat gain) once environmental temperatures become extreme. Additionally, the costs associated with thermoregulatory behaviors, such as dehydration risk from panting or sweating, or loss of body condition from changed foraging patterns, may become untenable with greater heat exposure (Albright et al., 2017; Cunningham et al., 2021; Youngentob et al., 2021). Finally, many thermoregulatory behaviors are dependent upon the thermal heterogeneity of an organism’s environment, yet anthropogenic environmental change can alter the thermal profile of habitat, reducing the availability of critical thermally buffered microsites (Chen et al., 1999; Neel and McBrayer, 2018). Only by obtaining a clear understanding of how organisms respond behaviorally to heat, the costs associated with these behaviors and which elements of habitat are essential for these behaviors, will we be able to effectively predict the impact of heat waves and begin to develop appropriate mitigation strategies.
This study focusses on a small passerine, the Jacky Winter (Microeca fascinans), living in a semi-arid region of southern Australia experiencing unprecedented heat wave events (Figure 1). Prior work at the study site found that wild individuals (with no access to water) can withstand > 10 h exposure to air temperatures ≥ 40°C, even though we would expect them to succumb to lethal dehydration based on the thermal tolerance of similar sized passerines in the laboratory (Sharpe et al., 2019). The aim of this study was to determine the behavioral strategies the birds use and identify factors that may limit their ability to cope with rising environmental temperatures in the future. We quantified the breadth of the birds’ repertoire of thermoregulatory behaviors, from mild to near-lethal temperatures, to assess whether their responses were nuanced, flexible and responsive to environmental conditions. We hypothesized that at high air temperatures the birds would adopt heat dissipation behaviors, such as wing-spreading and panting, reduce high intensity motor behaviors and alter their foraging time budget to avoid feeding. We predicted that increasing air temperature would lead to changes in microhabitat use, with potential shifts in perch height, shade use and the size of perch trees. We identified the potential costs of these temperature-related changes in behavior, in relation to foraging effort. We also examined to what degree the birds’ thermoregulatory behaviors were dependent on particular microhabitats and quantified the associated thermal benefits of these microsites. Without a detailed mechanistic understanding of how small endotherms are utilizing habitat during heat waves, the costs involved, and which components of the habitat serve as critical thermal buffers, it is impossible to accurately predict the impact of climate change on population persistence or devise conservation strategies that protect vulnerable biodiversity.
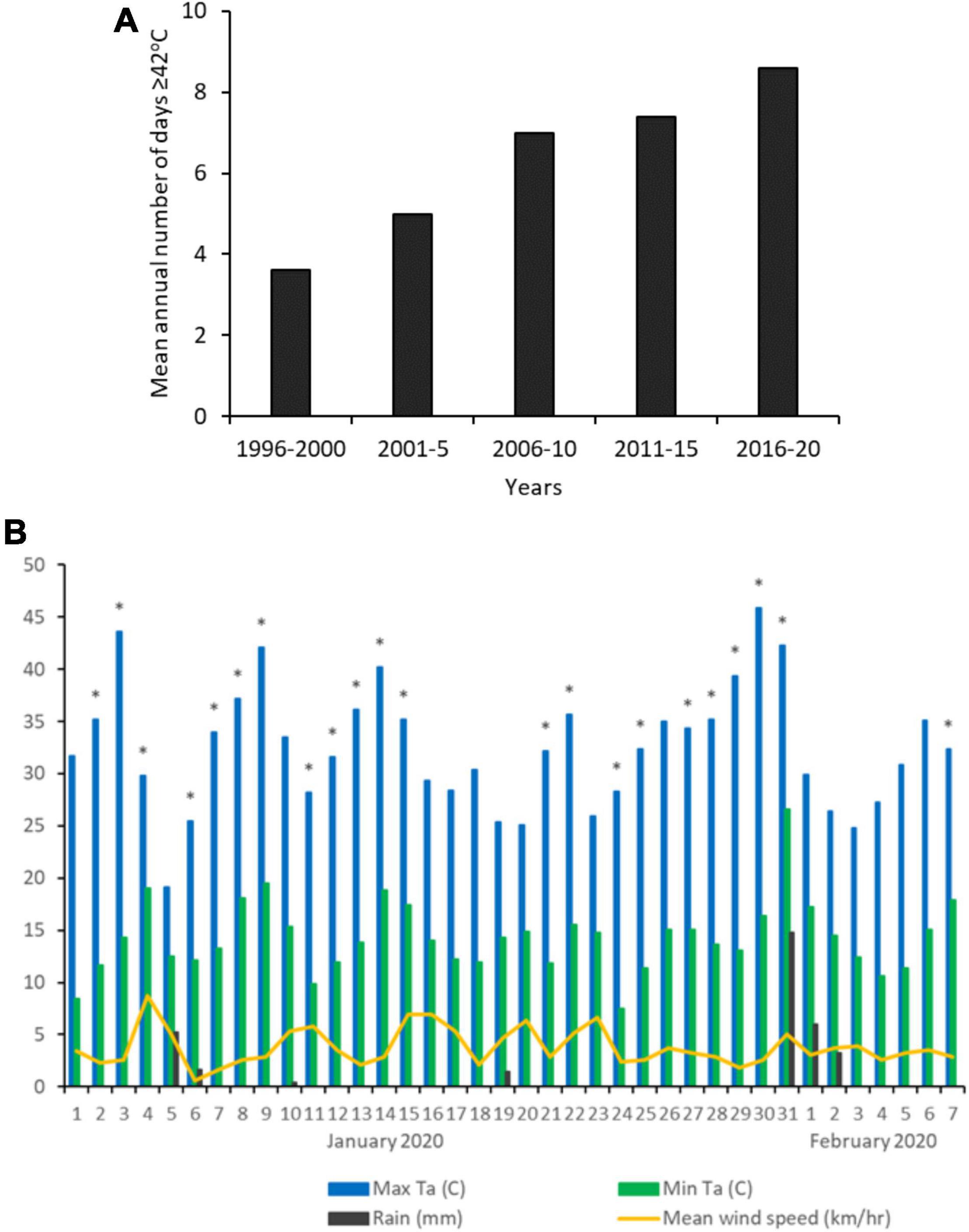
Figure 1. (A) Mean annual number of days when maximum air temperature was ≥ 42°C, 1996 to 2020 (Renmark; BOM). (B) Weather at the study site during the data collection period (Jan–Feb 2020). Asterisks indicate days upon which behavioral data were collected.
Materials and Methods
Study Site
The study was undertaken in semi-arid mallee woodland at Calperum Station, South Australia (34°03′S 140°38′E) during the austral summer (January–February) of 2020. The vegetation at the study site is comprised of low, multi-stemmed mallee eucalypts (Eucalyptus oleosa, E. dumosa, E. incrassata, E. socialis) and sugarwood (Myoporum platycarpum) above a sparse understorey of Senna and Acacia shrubs and porcupine grass (Triodia scariosa). All study groups lived in woodland that had not been burnt within the last 50 years. Mean annual rainfall is 251 mm, distributed irregularly across the year, and mean daily maximum temperatures in January and February are 33.8 and 32.7°C, respectively [data for Renmark, 1996–2020; Bureau of Meteorology, Australia (BOM)]. The frequency of extreme heat events at this location has been increasing, with the annual number of days ≥ 42°C more than doubling in the last 25 years (Figure 1A; Renmark, BOM).
Study Species
The study species—the Jacky Winter (Microeca fascinans)–is a small (14–20g) Australasian robin (Petroicidae) found in woodlands throughout Australia (Higgins and Peter, 2002). It is a “perch and pounce” predator, snatching tiny to small arthropods, either on the wing, from the ground surface or—less frequently—from vegetation (Recher and Davis, 1998). Although not a desert-adapted species, Jacky Winters rely entirely on dietary water, and surface water was unavailable to the study population during the data collection period. Pairs are territorial year-round (territory size is 1.7 hectares in southern Queensland; Wood et al., 2008) although they occasionally form cooperative groups of up to six birds (Higgins and Peter, 2002). Breeding occurs from September to January, with multiple clutches (comprised of two eggs) laid in open cup nests (Donaghey and Donaghey, 2017). At high temperatures, Jacky Winters use wing-spreading to facilitate convective heat loss and panting for evaporative cooling (Sharpe et al., 2019, 2021).
Behavioral Data
Our color banded population of 40 breeding groups of Jacky Winters has been studied since May 2018. A subset of 16 groups have been habituated to the presence of an observer accompanying the birds at 3–5 m to allow accurate and unimpaired behavioral observations.
Behavioral data were collected from non-breeding birds between 8:00 and 16:00 during January and February 2020. Air temperatures during observation periods ranged from 15 to 43°C (Figure 1B; see “Weather and surface temperature data” for measurement details). We undertook 20-minute focal observation sessions, during which an observer and a scribe documented the following behaviors of the focal bird: all predator scares, social interactions (intra and intergroup squabbles and chases), non-foraging flights > 5 m and prey catching attempts (distance from perch to location of prey; location of prey: ground, air, leaves, trunk). Due to the very small size of the Jacky Winters’ prey, we were unable to ascertain whether prey catching attempts were successful. We also documented the start and stop times of all bouts of preening, panting and wing-spreading (bouts were considered ended if the behavior ceased for > 10 s or was replaced by a different behavior). We recognized three wing-spreading postures: wing-raising (wings held slightly away from the sides of body), wing-drooping (wings held further away from body and wing-tips lowered beneath the level of the tail) and wind-surfing (wings held out horizontally, similar to flying position). We also noted changes to body posture and ptiloerection but did not quantify these due to the difficulty of defining them objectively. Additionally, every 2 min throughout the 20-min observation period, we recorded details of the focal individual’s perch, estimating height above ground, degree of sun exposure (full sun, shade, dappled or overcast), direction faced (to nearest 45° compass point, using a Garmin Etrex 30x GPS), whether the bird was > 0.5 m from a living, naked (i.e., without dead fibrous bark) trunk/branch > 8 cm in diameter, and size of tree (diameter of the tree’s largest trunk at 1.5 m). Prior to data collection, all observers were trained to estimate distances and heights accurately using a Bosch digital laser distance measure. During observation periods, we paused all data collection if the focal individual ceased to be visible and resumed when the bird could be seen again. If the focal bird could not be relocated within 10 min-, we aborted the observation session. Sessions of < 5 min were discarded.
We collected behavioral observations for 17 adult, non-breeding Jacky Winters (nine males, eight females) from 10 habituated groups. To ensure we obtained a balanced sample across all individuals, time of day and temperatures, we divided the 8-h data collection period into four 2-h blocks and classified days as either mild (<35°C), warm (35–38°C) or hot (>38°C) based on the maximum temperature for that day. To select which bird to sample, we chose individuals randomly from the pool of unsampled birds for that specific temperature/time of day category. Every individual was sampled during all four time periods and on cool, warm and hot days, with an average of 14.4 ± 0.8 observation sessions obtained for each bird (range 11–21). In total, we collected 79 h of behavioral data and 2,379 records of perch choice.
Weather and Surface Temperature Data
To assess how weather variables influenced the birds’ behavior we used weather data collected every 30 min within 6 km of our study site by the Calperum-Chowilla OzFlux land-atmosphere observatory, operated by the Terrestrial Ecosystem Research Network (TERN). Windspeed and wind direction were measured 2 m above ground by a RM-Young Wind Sentry. At a height of 20 m, vapor pressure was measured by a LI-COR LI-7500 infra-red gas analyzer, and incoming shortwave radiation, by a large Kipp and Zonen CNR4. Air temperature in the shade was measured at a height of 2 m by a Vaisala HMP-45C temperature probe. We used this OzFlux weather data for all analyses of the birds’ behavior. Because our behavioral observation sessions were shorter than the weather logging interval (20 versus 30 min), when allocating weather data to a session that occurred between weather readings, we selected the weather reading closest to the observation period (i.e., always within 10 min).
To obtain an indication of the thermal profile of the bird’s habitat, we measured surface temperatures at 83 trees (all mallee eucalypts) within 10 Jacky Winter territories. All territories were within areas that had remained unburnt for at least 50 years. Temperatures were measured on clear afternoons during January 2020 (16:00 −19:30) when air temperature was ≥ 35°C (mean 39.5°C; range 35–47°C). We used a Digitech (model QM7221) infrared laser digital thermometer gun at a distance of 2cm, to measure all surface temperatures.
When choosing which trees to sample, we randomly selected up to five GPS waypoints from within each group’s territory (waypoints represent a location where the territory-owners have been observed, and a pool of approximately 35 waypoints were available for each group). At the waypoint location, we measured air temperature at a height of 1.5 m using a Falcon Kestrel 2000 pocket wind and temperature meter (0.5°C accuracy) positioned within our own shade. We also took three ground surface temperature measurements (sun-exposed sand, shaded sand and shaded leaf litter), all sampled > 2 m from a tree trunk. We then selected two trees: the nearest to the waypoint and the largest (based on trunk circumference) within a 20 m radius of the waypoint. At each tree, we identified the thickest trunk (because mallee eucalypts are multi-stemmed) and measured the shaded surface temperature of this trunk at its base and at a height of 1.5 and 2.5 m. We also recorded its circumference at 1.5 m. We identified any potential refuges (crevices, spouts, hollows, etc.) at the tree’s base and measured the surface temperature within these refuges at the deepest/shadiest location. We estimated the extent of leaf litter cover beneath the tree (the percentage of ground covered by leaf litter within a circle, 1 m in diameter, centered on the largest trunk) and measured the shaded surface temperature of this leaf litter.
Statistical Models
We constructed a series of linear and zero-inflated mixed models to explore relationships between behavior, habitat characteristics and weather. Explanatory variables were chosen based on hypothesized drivers, including potential non-linear effects and interactions.
1. Foraging behavior. To test the hypothesis that foraging behavior will decline with increasing heat load (Youngentob et al., 2021), we constructed two separate models, with rate of prey capture attempts and distance flown to obtain prey as response variables. In each model we included the following fixed effects: air temperature and solar radiation (contributors to heat load; Mitchell et al., 2018), wind speed (because wind reduces heat load due to enhanced cooling; Wolf and Walsberg, 1996) and two-way interactions between these weather variables. Because microhabitat choice may impact foraging behavior (Cunningham et al., 2015), we also included perch height, perch height × air temperature and proportion of perches in shade as fixed effects. We included individual and group identity as random terms, to account for multiple sampling of the same birds and groups. We controlled for the following variables by including them as fixed effects: time of day (as the number of minutes after sunrise), the bird’s sex (male or female) and observer identity (to account for any differences among observers). Although observers were trained in length estimation prior to data collection, we found that one observer slightly, but consistently, over-estimated while another slightly, but consistently, under-estimated. This resulted in a significant difference between observers in the quantification of parameters that involved estimating distance or trunk width. By including “observer” as a fixed effect, the model takes into account this source of variation so that it does not impact upon our findings relating to weather variables. In one model (Table 1B), we included observer as a random effect, rather than a fixed effect, because the model otherwise failed to converge. In this case, it was preferable to include observer as a random term to account for differences in the number of behavioral watches undertaken by each observer. In the model assessing distance flown to prey, we also included the location of the potential prey item (four categories) as a fixed effect. In a third model, we tested the hypothesis that birds will pre-emptively increase their foraging effort on the mornings of very hot days, using rate of prey catching attempts between 08.00 and 10.00 h as the response variable. We included the day’s maximum air temperature as a categorical variable (>38 or <38°C) and the same random terms as above.
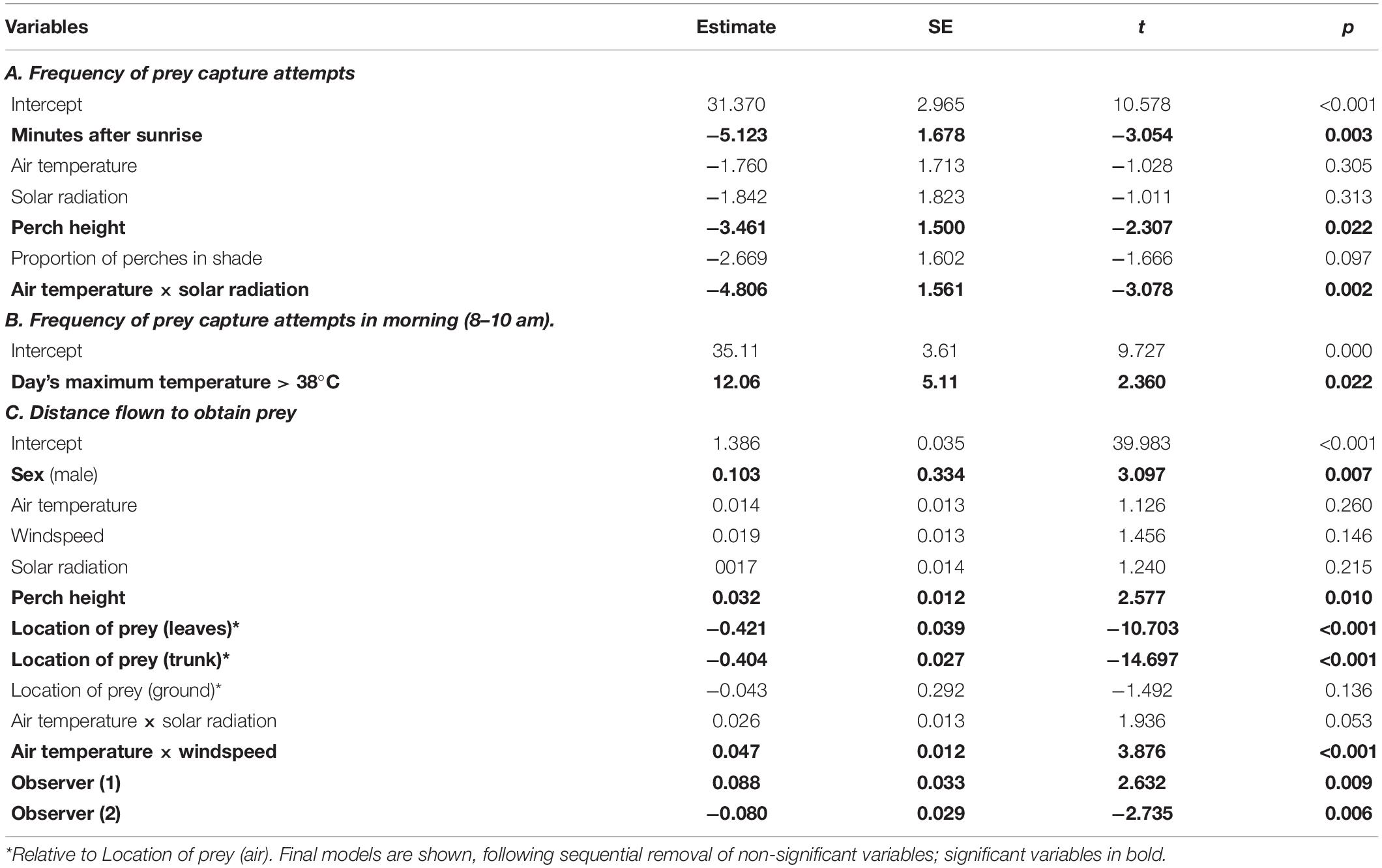
Table 1. Results of REML mixed effects models testing for factors associated with foraging behavior.
2. Non-foraging behavior. To test the hypothesis that non-foraging motor activities will decline with increasing heat load (Cunningham et al., 2021), we constructed three separate models with the response variables: rate of non-foraging flights (>5 m), rate of social interactions and proportion of time spent preening. We included the three weather variables and their two-way interactions as fixed effects, controlled for sex and time of day, and used the same random terms as (1).
3. Heat dissipation behaviors. To assess the influence of weather variables on heat dissipation behaviors we ran two models with the following response variables: the proportion of time spent panting and the proportion of time spent wing-spreading (combining wing-raising, wing-drooping and wind-surfing into a single variable). In both models we included the same terms as in (2) plus a quadratic term for air temperature because we expected a non-linear response due to threshold-related responses. In the panting model we fitted vapor pressure, and its interaction with temperature, as additional fixed effects because humidity is known to affect panting behavior at high temperatures (Mitchell et al., 2018).
4. Perch choice. We hypothesized that the birds’ choice of microsite will alter with increasing heat load, so we tested how the three weather variables were related to the following response variables: likelihood of perching in the sun, perch height, size of the perch tree, whether perched within 0.5 m of a naked branch/trunk (Briscoe et al., 2014) and whether facing the wind (the last two variables were categorical: yes/no). We tested each of these response variables in a separate model. We fitted the same fixed effects, including interactions and random effects, as in (2) and included air temperature as a quadratic term because we expected responses to be non-linear due to threshold effects.
5. Surface temperatures of mallee eucalypts. To test the hypothesis that the relative surface temperature of a tree’s microsites is negatively related to tree size and leaf-litter ground cover, we ran five separate models with the following five response variables: surface temperature of leaf-litter at tree-base, tree-base refuges (crevices and hollows), tree trunk at its base, tree trunk at 1.5 m and tree trunk at 2.5 m. We fitted air temperature, tree size (trunk circumference at 1.5 m) and percentage leaf-litter cover as fixed effects. Models were fitted using the lm function because there were no repeat measures.
Model Fitting
For each model we fitted all potential explanatory variables (as described above), then removed non-significant terms sequentially, starting with interactions, to arrive at a final model with only significant or near-significant terms. The model with the lowest AIC score was chosen, which always only included significant or near-significant variables. To avoid multicollinearity among explanatory variables we first estimated pair-wise Pearson’s correlation coefficients and confirmed that correlations were not high (|r| < 0.7). Model fit was checked using diagnostic plots to confirm model residuals were distributed around zero. For linear models we used residual plots and normality plots to check for deviations from normality among residuals, and for zero-inflated models we used DHARMa package (Hartig, 2020) to check for overdispersion.
Linear mixed models were fitted with REML (Restricted maximum likelihood) using the lmer function in the lme4 package (Bates et al., 2015) and generalized linear mixed models (GLMM) were fitted using the glmer function in the lme4 package (Venables and Ripley, 2002). Zero-inflated models (used for heat dissipation models) were fitted using the glmmTMB package with beta-binomial distribution. All models were fitted in R 3.3.2 (R Development Core Team).
Other Statistical Analyses
To compare the surface temperatures of different microsites within mallee eucalypt trees (except for potential refuges), we used the full data set and conducted a Repeated One-way ANOVA and Tukey tests for all pairwise comparisons, using R 3.3.2. We also undertook a second Repeated ANOVA to compare the surface temperatures of potential refuges with other microsites, restricting the analysis to trees that contained refuges. To test whether the difference between surface temperatures and air temperature changed with air temperature, we used Pearson correlation coefficients to compare the variable “surface temperature minus air temperature” with air temperature. These tests, and all other non-modeling statistical tests were conducted in Microsoft Excel 2016 v2111. Means are presented ± 1 SE.
Results
Foraging Behavior
Although there was no overall effect of either air temperature or solar radiation on foraging effort, when both these variables were simultaneously high, Jacky Winters substantially reduced their foraging effort, after controlling for time of day (Table 1A: air temperature × solar radiation interaction; Figure 2A). At >35°C, the birds averaged only 12 (±2) prey capture attempts per hour compared with 35 (±2) at < 28°C. Hourly rate of attempts was also negatively related to perch height and showed a negative trend with the proportion of a bird’s perches that were in the shade (Table 1A).
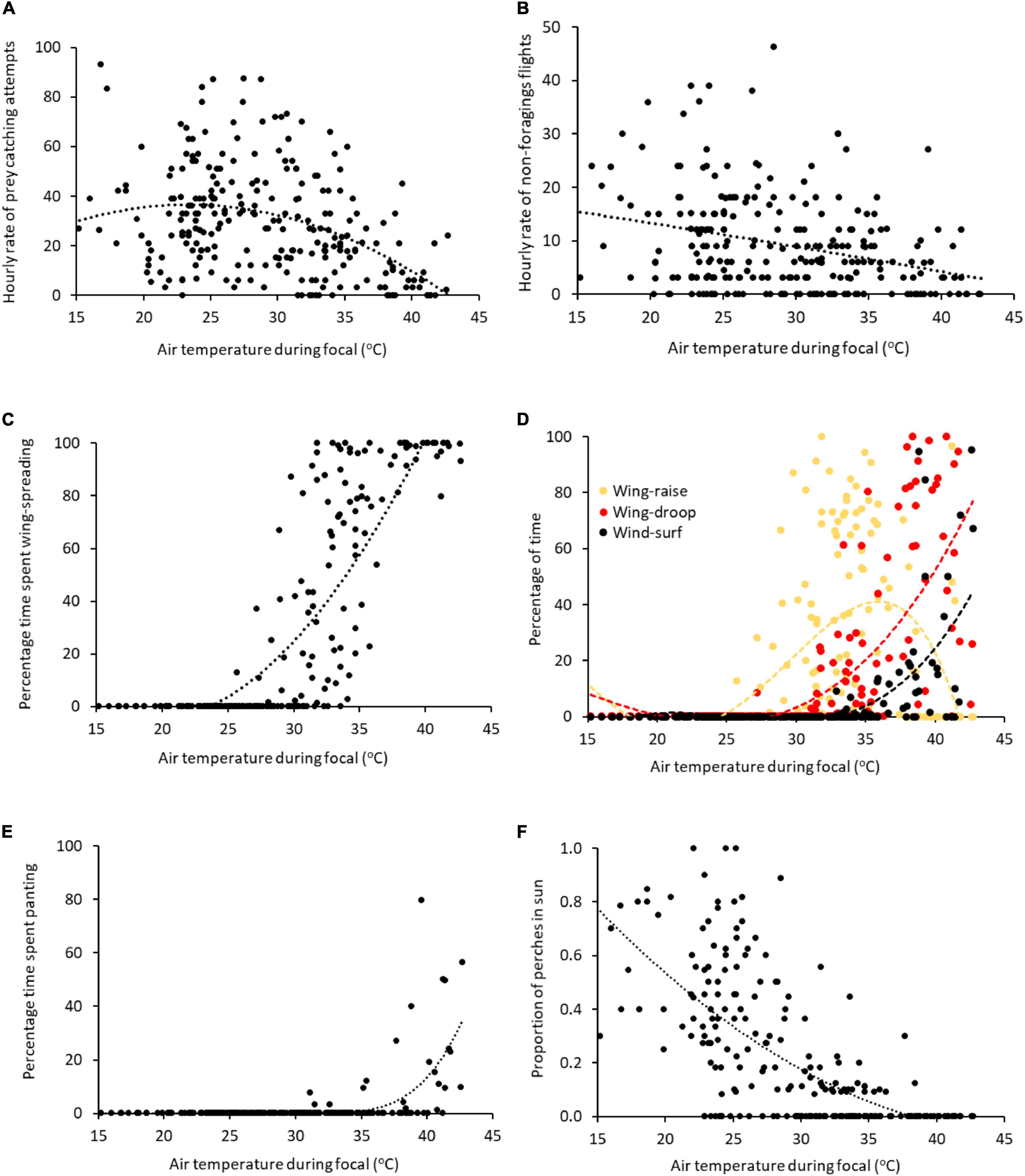
Figure 2. The relationship between air temperature and (A) hourly rate of prey catching attempts and (B) non-foraging flights > 5 m, and percentage of time devoted to (C) any form of wing-spreading, (D) wing-raising, wing-drooping and wind surfing, and (E) panting, and (F) proportion of perches located in direct sun. Showing raw data with polynomial trend lines generated by Microsoft Excel v2111.
To compensate for reduced foraging effort at high temperatures, individuals modified their time budget. On days > 38°C, they increased their rate of foraging attempts in the mornings (8–10 am) by 30% and reduced afternoon foraging rates by 70%, (as compared with days < 35°C) (Table 1B and Figure 3). To test if this increase in foraging effort was genuinely pre-emptive and that the birds were not simply compensating for a deficit of foraging on the previous afternoon, we focused on the hourly rate of foraging attempts at 8–10 am on days with a maximum temperature > 38°C, comparing days that were preceded by a warm day (35–38°C), when the birds foraged normally, and days preceded by a > 38°C day, when they abstained from afternoon foraging. There was no significant difference between the two (t-test: t11 = 0.77, p = 0.46; hourly attempts: warm: 50 ± 5; hot: 42 ± 9).
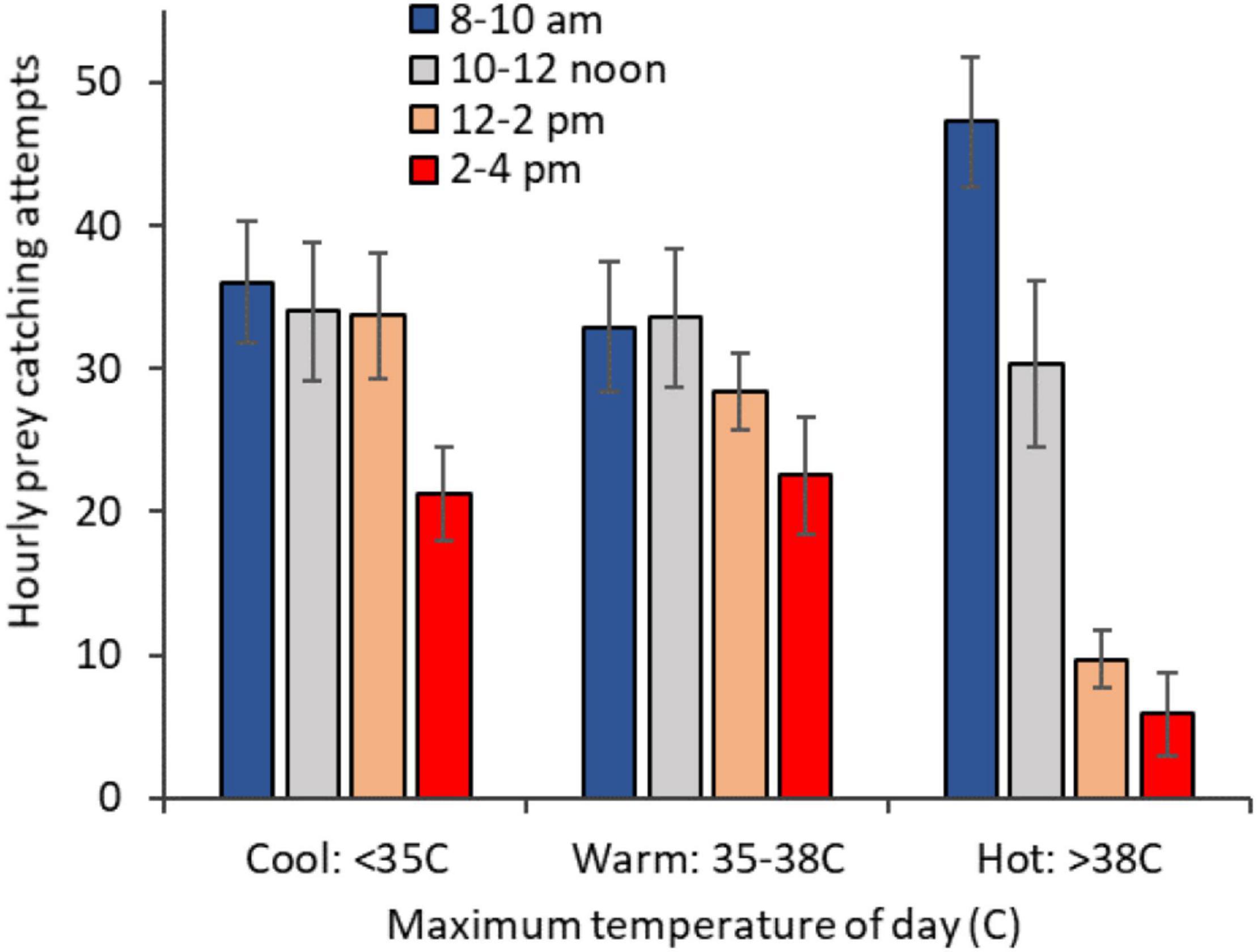
Figure 3. Hourly rate of prey catching attempts by time of day, on days with a maximum temperature that was mild (< 35°C, n = 91 observation sessions), warm (35–38°C; n = 83) or hot (> 38°C; n = 71). Error bars show SE.
Jacky Winters did not modify the distance they flew to catch prey in response to air temperature, windspeed or level of solar radiation, although they flew further at high temperatures when the wind was strong (Table 1C). The distance the birds flew to obtain prey was, however, positively correlated with perch height, but unrelated to whether the bird was perched in the shade. Males flew further than females. Flight distance also varied with the prey’s location, with birds flying furthest for aerial or ground prey but shorter distances for prey on leaves or tree trunks (Table 1C).
Other Activities
The hourly rate of non-foraging flights (>5 m) was negatively related to air temperature, after controlling for time of day (Figure 2B). This negative effect of increasing temperature on non-foraging flights tended to be exacerbated as solar radiation and windspeed increased (Table 2A: air temperature × solar radiation interaction, air temperature × windspeed interaction, respectively). Males moved more frequently than females (Table 2A). Hourly rates of social interactions were also negatively related to air temperature and to windspeed (Table 2B). However, the proportion of time spent preening showed a non-linear relationship with air temperature, increasing with temperature until the mid 30°Cs, then decreasing. Preening was also positively related to solar radiation, and this relationship was stronger as temperature increased (Table 2C: air temperature × solar radiation interaction).
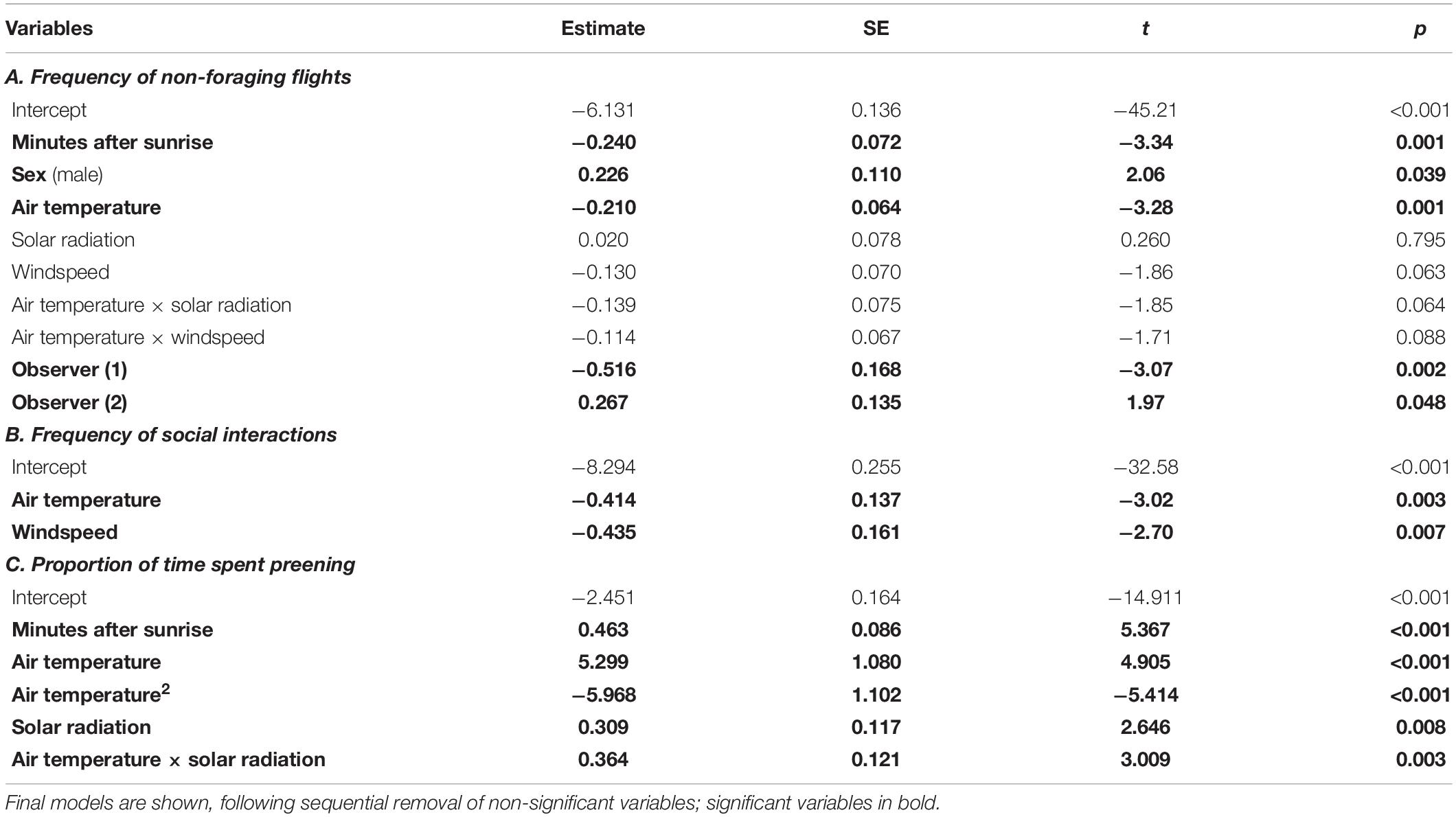
Table 2. Results of REML mixed effects models testing for factors associated with non-foraging activities.
Heat Dissipation Behaviors
The proportion of time spent wing-spreading (i.e., wing-raising, wing-drooping or wind-surfing) was positively associated with air temperature, although the relationship had a non-linear component (Table 3A and Figure 2C). The birds began wing-raising at air temperatures as low as 26°C and wing-drooping at about 32°C. Wing-raising was the most prevalent form of wing-spreading until around 35°C, when wing-drooping became more prevalent (Figure 2D). Proportion of time spent wing-spreading declined as windspeed increased, but the declining effect of windspeed on wing-spreading was reduced as air temperatures increased (Table 3A: air temperature × windspeed interaction).
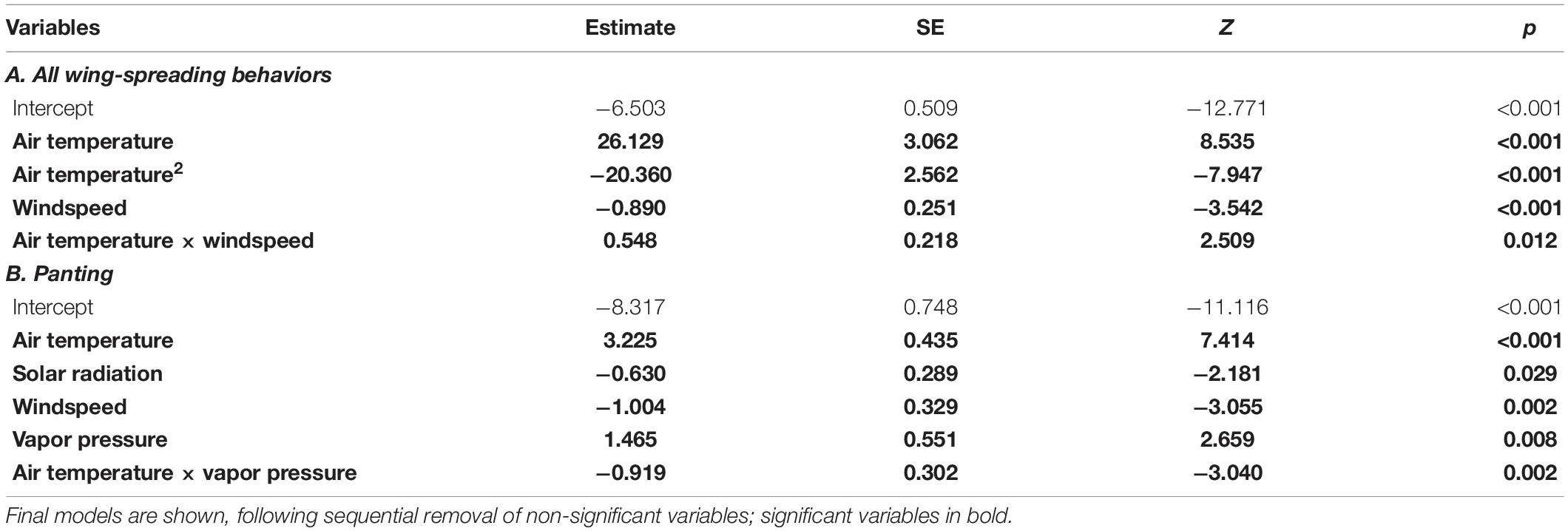
Table 3. Results of zero-inflated models with beta-binomial distribution (glmmTMB) testing for factors associated with the proportion of time spent undertaking heat dissipation behaviors.
Birds began panting at air temperatures in the 30°Cs, and proportion of time spent panting increased with air temperature (Figure 2E). Vapor pressure was also positively associated with time panting, but its effect on panting was less pronounced as air temperature increased (Table 3B: air temperature × windspeed interaction). Conversely, windspeed was negatively related to time spent panting: birds panted less when the wind was strong (Table 3B and Figure 4). Solar radiation was also negatively related to time panting.
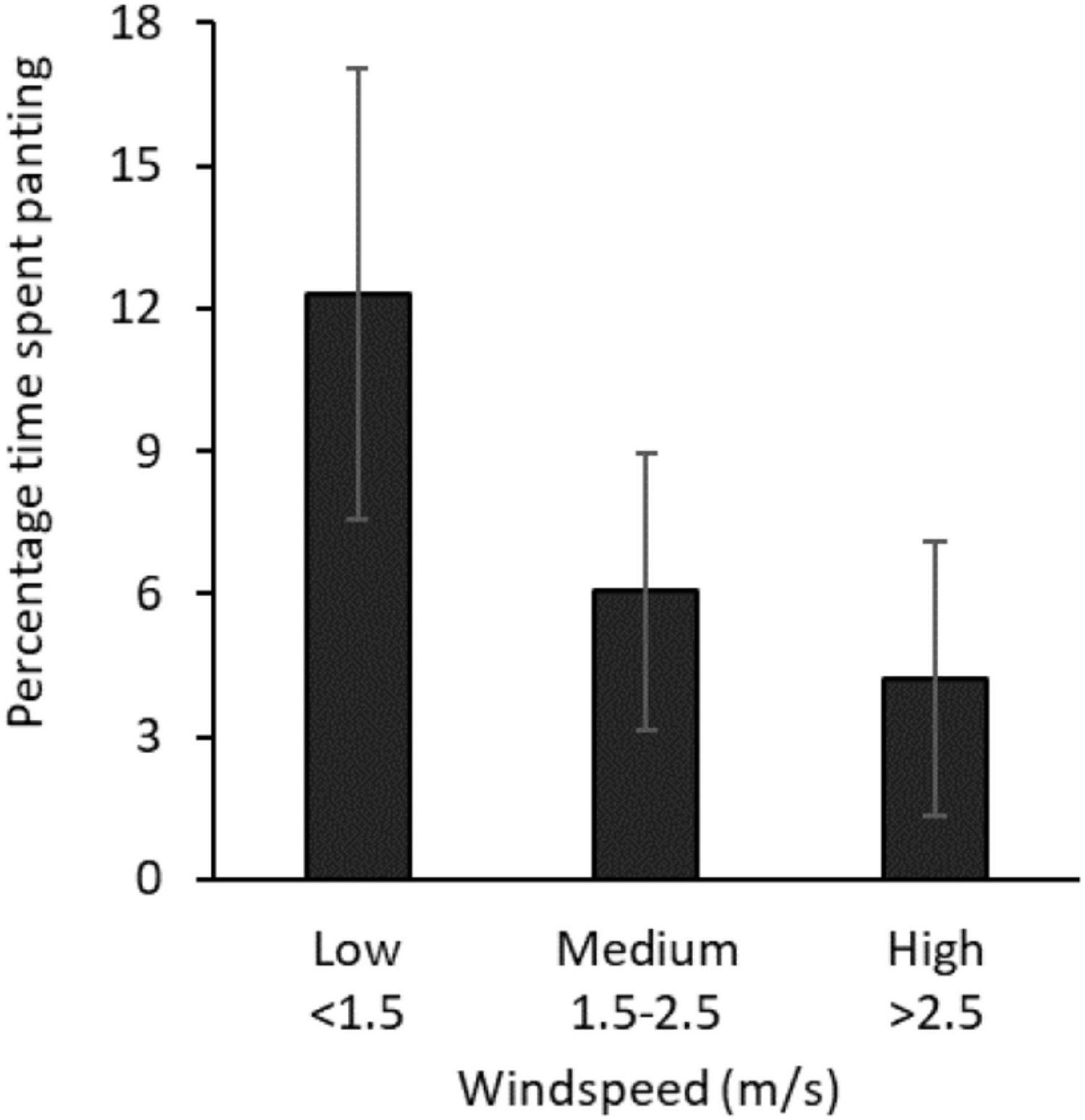
Figure 4. Mean percentage of time birds spent panting at air temperatures > 35°C, by windspeed (m/s). Error bars show SE.
Microsite Choice
The likelihood of a bird choosing to perch in direct sunlight was negatively correlated with both air temperature and level of solar radiation (Table 4A). In sunny conditions, birds began using shaded perches at air temperatures > 28°C. Only 1% of perches were in sun at temperatures > 35°C (compared with 47% at < 28°C; Figure 2F). Birds also shifted to shaded perches in windy conditions (Table 4A).
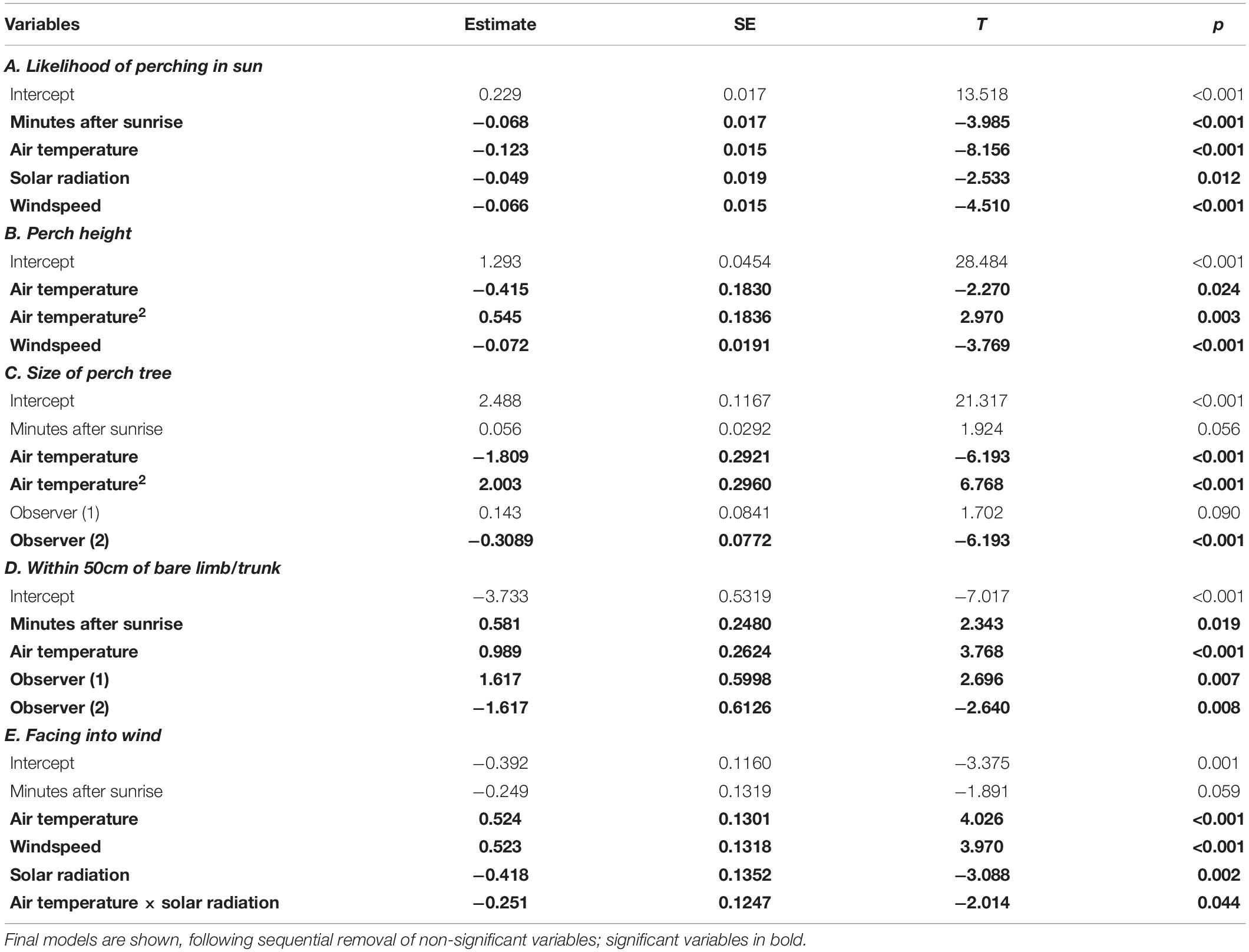
Table 4. Results of REML or GLMM mixed effects models testing for factors associated with perch choice.
There was a non-linear relationship between the height of a bird’s perch and air temperature, with the birds switching to elevated perches at temperatures > 35°C, when mean perch height almost doubled (from 2.7 to 5.1 m; Figure 5A and Table 4B). Perch height was negatively related to windspeed, with birds perching lower in high winds (Table 4B). Birds choosing high perches favored larger trees, with a positive correlation between perch height and perch tree size (Pearson’s correlation: r1978 = 0.34, p < 0.001). The size of the perch tree was also related to air temperature, with birds favoring large trees at the highest temperatures (Figure 5B and Table 4C). Air temperature was also positively related to the likelihood of the birds perching within 0.5 m of a naked trunk or large branch (Table 4D).
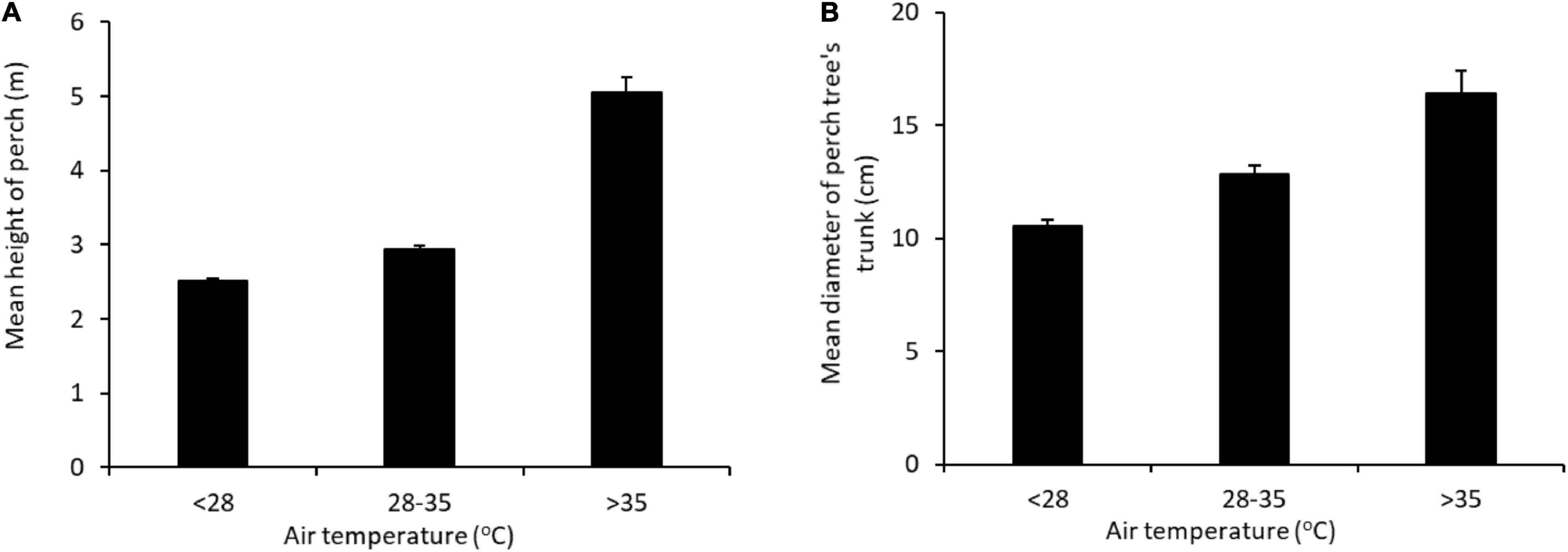
Figure 5. Relationship between air temperature and (A) the mean height of perches and (B) the mean size of the perch tree (diameter of largest trunk at 1.5 m). Error bars show SE.
Birds perched facing into the wind more often than expected by chance (44 versus 25%; Chi2 goodness of fit: X2 = 434.38, n = 2,322, p < 0.001) and this became more pronounced at high temperatures (67% at > 40°C; Table 4E). Facing into the wind was also positively related to windspeed, but negatively associated with level of solar radiation (Table 4E). Birds were also twice as likely to face into the wind if it was coming from the North (i.e., from the hot central Australian desert) as compared with other directions (78 versus 38%; Figure 6).
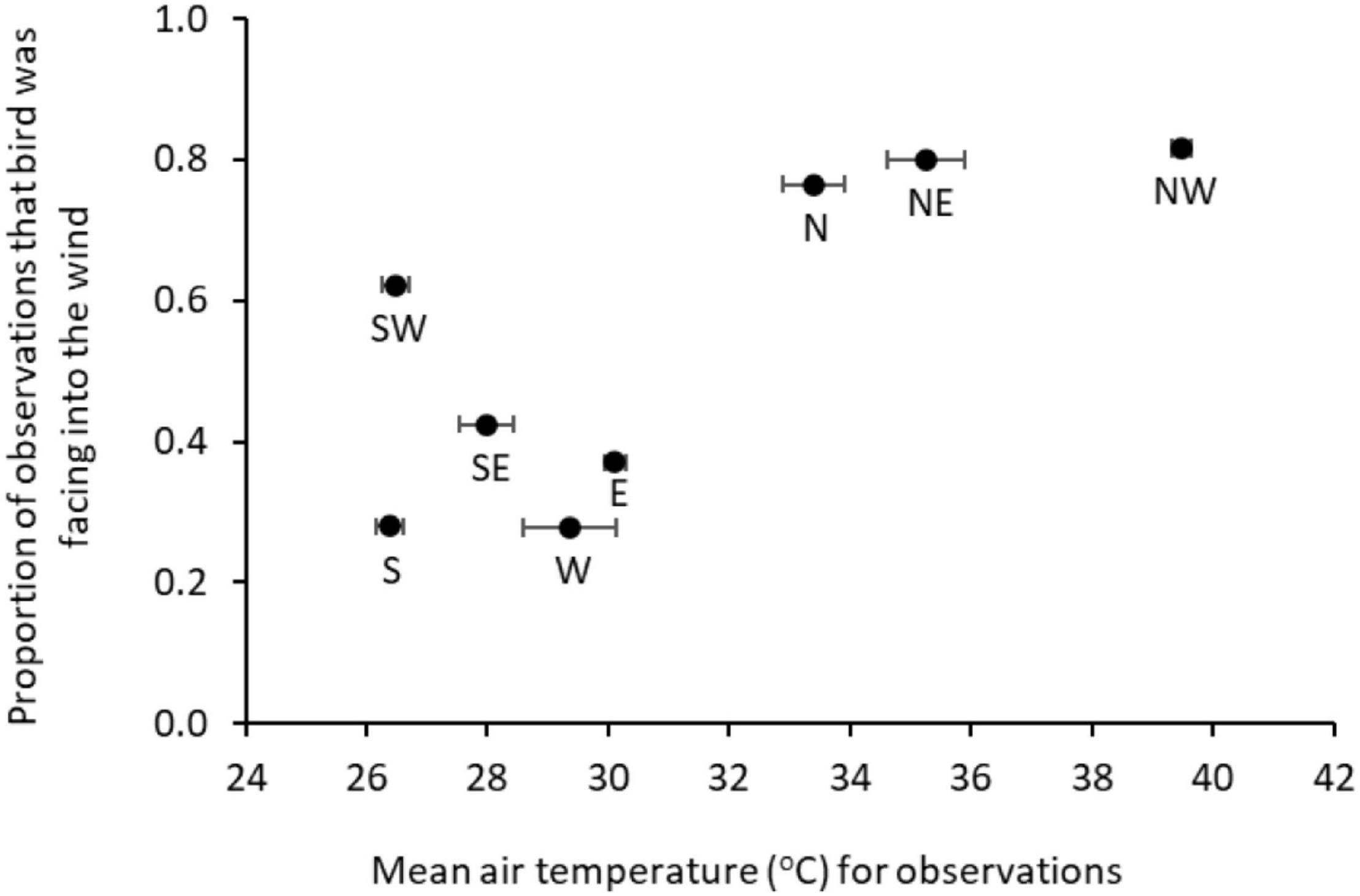
Figure 6. Wind direction: mean air temperature recorded for observations made when the wind was coming from a particular direction, and proportion of those observations in which the bird was facing into the wind (25% expected by chance). Error bars show SE for air temperature.
Once air temperature reached 39–40°C, Jacky Winters usually moved to the ground, sheltering in shaded crevices and depressions at the base of large trees. They did not perch on the ground under any other circumstances. At ≥ 39°C, 39% of our focal individuals retreated to a tree-base refuge, but this is a recognized underestimate because, once birds were within a refuge, we were unable to locate them to begin focal observations, so our sample was entirely biased to individuals that remained out of refuges. In total, we observed nine instances of Jacky Winters moving into tree-base refuges (eight individuals from four groups) but, at air temperatures ≥ 41°C, we observed only two individuals (from one group) remaining aloft.
Thermal Profile of Habitat
There was considerable thermal heterogeneity in the birds’ habitat at air temperatures > 35°C (Figure 7). Sun exposure greatly increased surface temperatures, with sun-exposed sand 15.9 (±1.2)°C hotter than air temperature and 7.3 (±0.9)°C hotter than shaded sand, at an average air temperature of 39.5°C (Repeated one-way ANOVA for surface temperatures, excluding refuges: F6,81 = 189.1, p < 0.001; Tukey: p < 0.001). The temperature difference between sun-exposed sand and air temperature increased as air temperature increased (Pearson’s correlations: r42 = 0.442, p = 0.003).
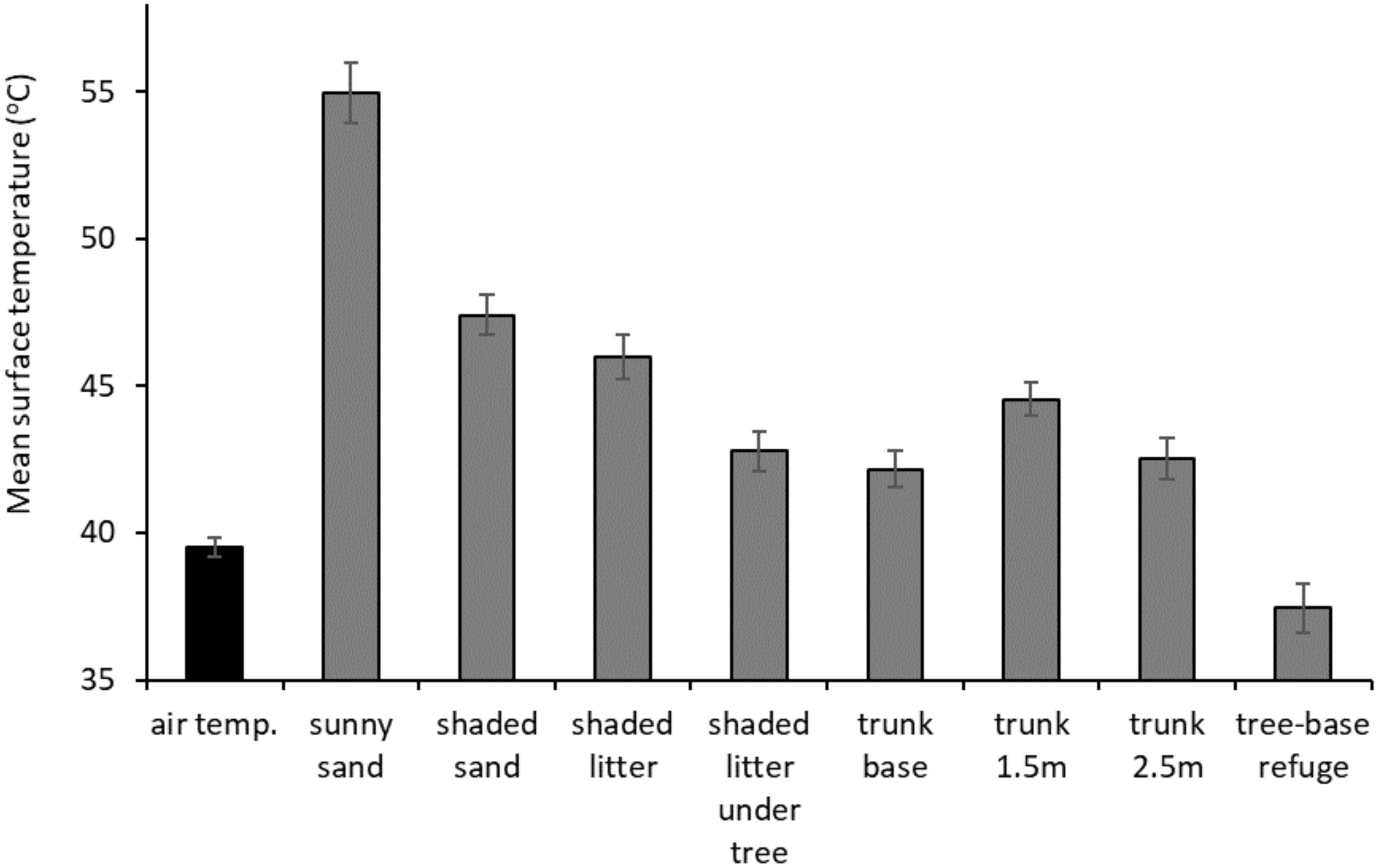
Figure 7. Mean surface temperature of habitat substrates at a mean air temperature of 39.5°C on sunny afternoons. Error bars show SE. (Repeated ANOVA: F6,81 = 189.1, p < 0.001; Tukey: all pairwise comparisons between substrate categories: p < 0.05, except for shaded litter under tree versus trunk base and trunk at 2.5 m, and trunk base versus trunk at 2.5 m).
Trees reduced ground surface temperatures, with shaded leaf-litter at the base of a tree 3.6 (± 0.4)°C cooler than shaded leaf-litter > 2 m from a tree (Tukey: p < 0.001). The average temperature of shaded, tree-base litter was the same as air temperature at air temperatures ≤ 38°C but rose to 6°C above air temperature at air temperatures ≥ 45°C (Pearson’s: r76 = 0.481, p < 0.0001). The size of the tree (circumference of the tree’s largest trunk) was negatively related to surface temperatures beneath the tree, with larger trees being cooler at all measured locations, except for the trunk at 2.5 m (Tables 5A–D and Figure 8).
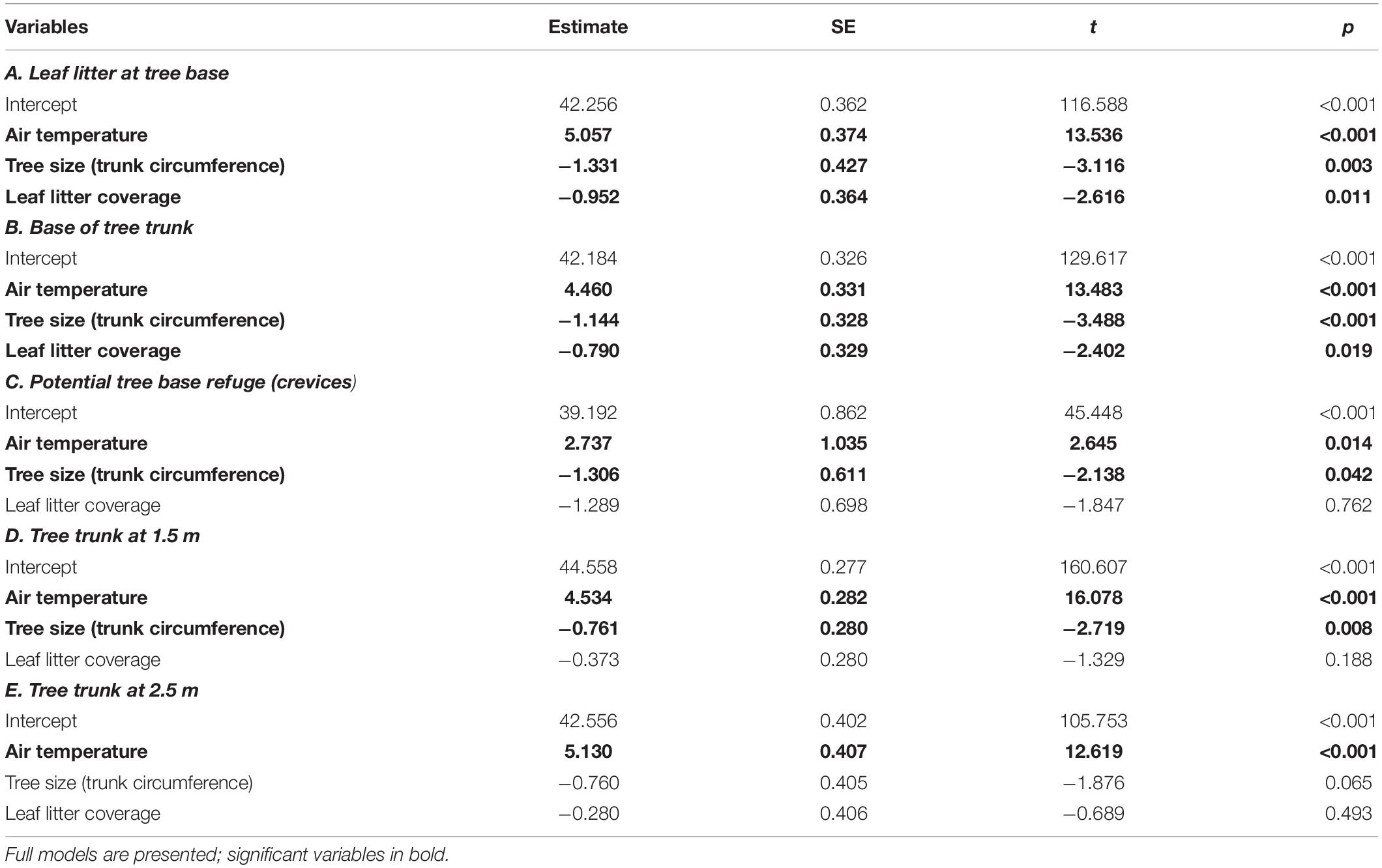
Table 5. Results from linear models (lm) testing for factors associated with surface temperatures of mallee eucalypt trees, at air temperatures > 35°C.
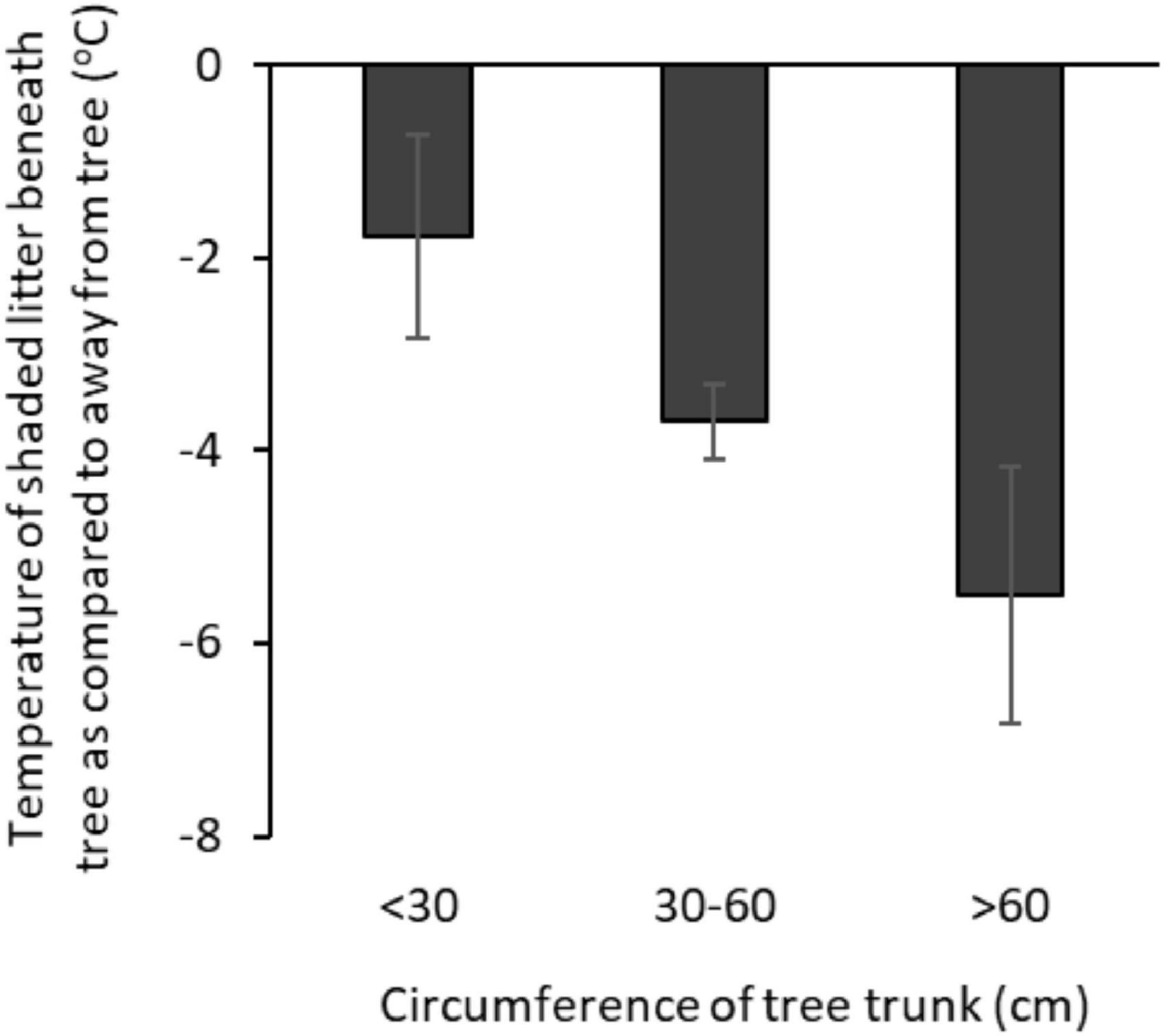
Figure 8. The thermal benefit of trees: the difference between the surface temperature of shaded leaf-litter beneath a tree as compared with shaded leaf-litter > 2 m from a tree (°C), by tree size (circumference of largest trunk at 1.5 m) at mean air temperature of 39.5°C. Error bars show SE.
Leaf-litter also moderated ground surface temperatures with shaded leaf-litter 1.4 (±0.3)°C cooler than shaded sand (both measured > 2 m from a tree, at an air temperature of 39.5°C; Tukey: p = 0.047). The greater the proportion of ground covered by leaf-litter (within 1 m of the tree base), the cooler were the surface temperatures at the base of the tree (Tables 5A,B).
Tree-Base Refuges
We documented two types of potential thermal refuge at the base of mallee eucalypts: tree hollows and spouts (holes within a dead or living trunk < 50 cm from ground; n = 20; Figure 9a) and small crevices that provided partial shelter (nooks among the tree-base roots or depressions on the basal lignotuber; n = 11; Figure 9b). We included crevices in our sample because Jacky Winters used this refuge type most often.
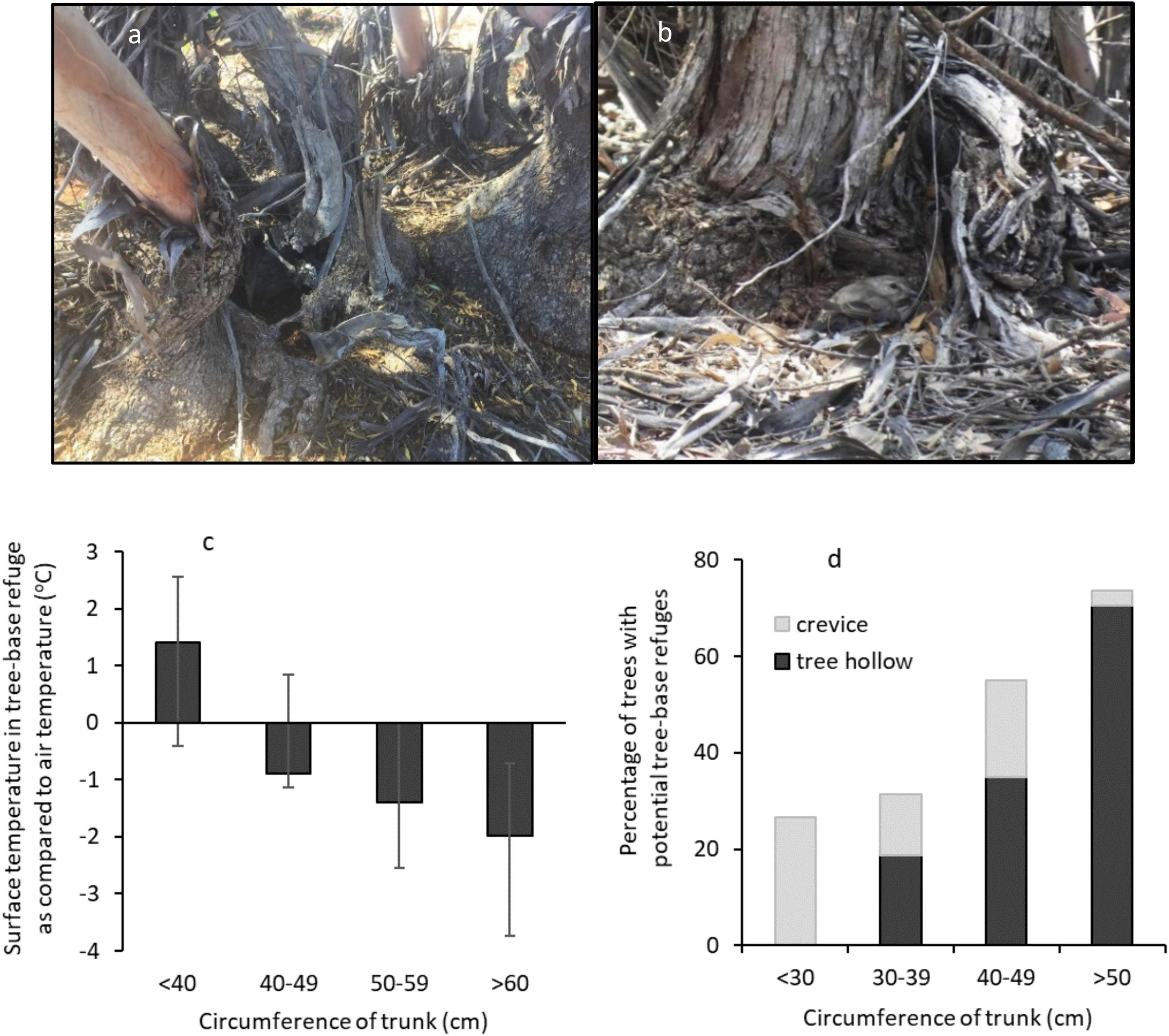
Figure 9. Tree-base refuges: (a) a tree hollow and (b) a Jacky Winter sheltering in a crevice/depression. (c) Mean difference between the surface temperature in a tree-base refuge and air temperature (at a mean air temperature of 39.5°C) and (d) the percentage of mallee eucalypts with potential tree-base refuges, by tree size. Error bars show SE.
The surface temperature within potential tree-base refuges (crevices and hollows) was significantly cooler than all other locations (Repeated one-way ANOVA: F7,31 = 189.1, p < 0.001; Figure 7). Tree-base hollows were 2.0 (± 0.8)°C cooler than air temperature, but tree-base crevices did not differ significantly from air temperature (Paired t-tests: hollows: t19 = 2.368, p = 0.029; crevices: t10 = −1.595, p = 0.142). Tree size affected the thermal benefit of tree-base crevices and hollows, with bigger trees providing cooler refuges (Table 5D and Figure 9c).
Large trees were also more likely to contain tree-base refuges (Figure 9d): refuge-bearing trees were significantly larger (trunk circumference) than those without (t-test: t25 = 3.016, p = 0.006). Tree-base hollows did not occur in mallees with a trunk circumference < 30 cm (at a height of 1.5 m). Overall, refuges were rare: 63% of trees offered no potential refuges, even though we biased the sample toward large trees (by sampling the largest tree within 20 m at each sampling location).
Discussion
Behavioral Responses to Heat
Jacky Winters exhibited a broad repertoire of thermoregulatory behaviors, which was nuanced, flexible and highly responsive to both air temperature and other abiotic factors, such as wind velocity and solar radiation. The sequence of their behavioral responses to heat is shown in Figure 10. At air temperatures > 28°C, the birds withdrew to shade and reduced physically demanding activities such as non-foraging flights and social interactions. Air temperature per se did not reduce foraging activity (except at temperatures ≥ 38°C), suggesting that energy and water gains from feeding outweighed the costs of increased thermogenesis (Wolf and Walsberg, 1996). However, this balance shifted when air temperature and solar radiation were high simultaneously, and the birds curtailed foraging in response to this greater heat load. This reduction in foraging may have been influenced by heat-related changes to prey availability (Holm and Edney, 1973), but experimental studies show that birds given unlimited food still do not forage at high temperatures (digestion generates metabolic heat; Beale et al., 2018), suggesting that heat, not food availability, is responsible (Ricklefs and Hainsworth, 1968; Olinger, 2017).
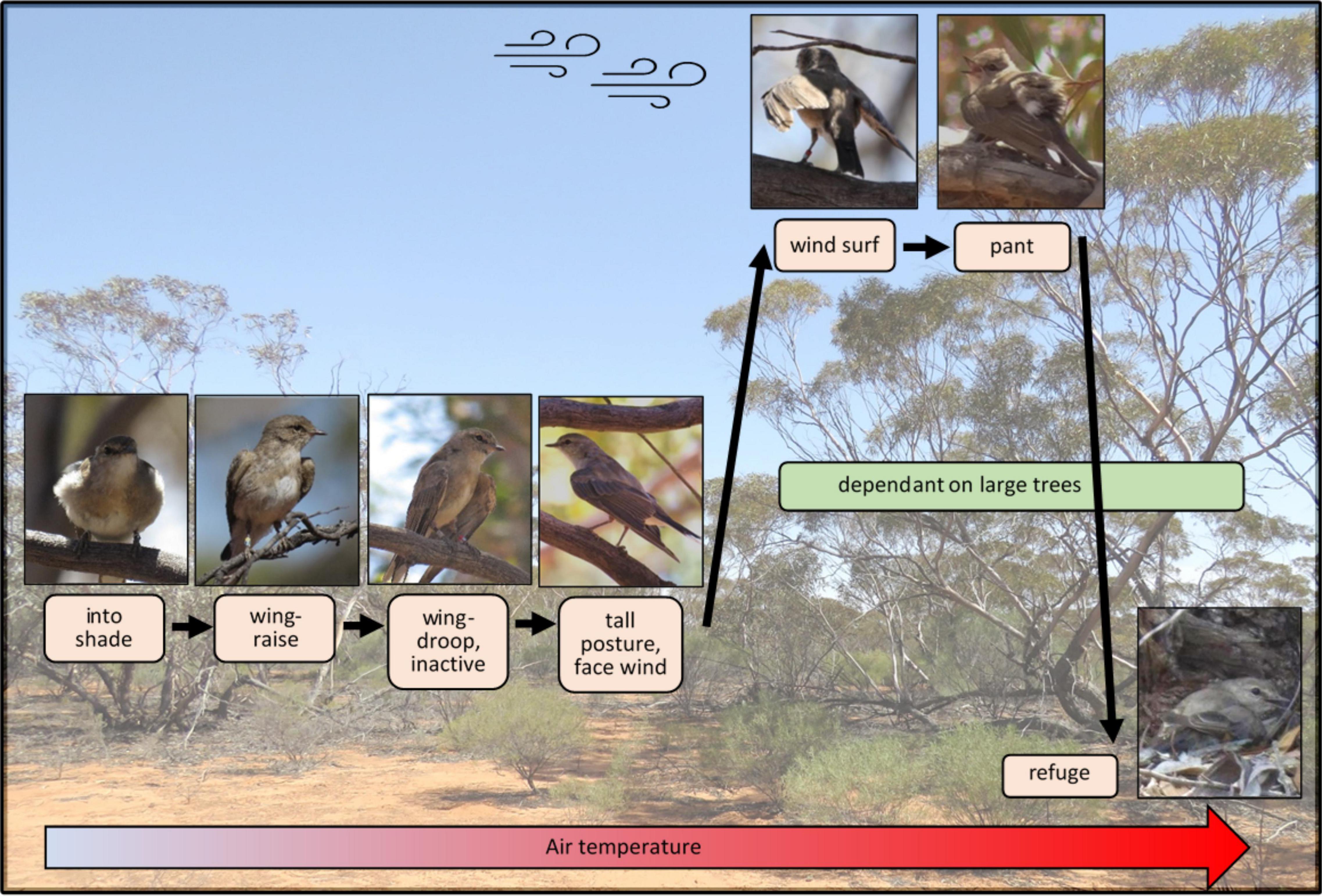
Figure 10. The effect of increasing air temperature on the cooling behaviors and microhabitat use of Jacky Winters in mallee woodland.
The Jacky Winters also began adopting behaviors that facilitated convective heat loss at air temperatures in the high 20°Cs. In addition to wing-raising, to increase surface area to mass ratio, the birds flattened their plumage to reduce its insulative properties, and adopted a tall, upright posture to elongate body shape (Figure 10). As air temperature rose, wing-spreading became more exaggerated (becoming wing-drooping; Figure 10) and, when > 35°C, the birds moved to elevated perches in the largest trees. This preference for high perches appeared to be related to wind velocity, which increases with elevation (Mcilveen, 1992). Average windspeed at our site doubled between the heights of 2 and 10 m. Choosing the windiest microsites allowed the birds to maximize “forced” convective cooling, and it is notable that they did not use elevated perches if the wind was already strong. The thermal benefit of higher wind velocity is evidenced by our finding that birds devoted less time to heat-dissipation behaviors (e.g., wing-spreading and panting) with increasing windspeed, which is consistent with laboratory findings (Wolf and Walsberg, 1996; Wolf et al., 2000). Jacky Winters were also three times more likely to perch facing into the wind at high air temperatures (>40 versus <20°C), probably to enhance convective heat loss, because narrow objects, pointing into the wind, lose heat more rapidly (Mitchell et al., 2018). Facing into the wind also facilitated wind-surfing (i.e., allowing the wind to lift the wings into flying position; Figure 10), enabling air flow over a greater surface area. By seeking out windy microsites and maximizing convective cooling, birds can reduce their heat load without relying on more costly evaporative cooling.
At air temperatures in the high 30°Cs, the birds also favored perches close to naked, living branches or trunks, and some individuals were seen lying with their bellies against the wood (a posture only adopted during extreme heat or incubation; Sharpe et al., 2021). This presumably facilitated conductive cooling because the surface temperature of living timber has been shown to be significantly cooler than air temperature, due to water flow in the tree’s phloem tissue and thermal inertia (Briscoe et al., 2014). The birds may also have favored perches close to large branches to capitalize on the dense shade cast by large limbs, because unbroken shade is rare in this habitat.
As air temperature approached body temperature (approximately 40°C; Boyles et al., 2011), the birds began panting intermittently to facilitate evaporative cooling. Time spent panting increased with air temperature but was relatively low (averaging 13 min per hour at air temperatures ≥ 40°C) presumably reflecting the costs associated with this behavior. Panting uses body water, with rates of water loss increasing linearly with temperature (McKechnie et al., 2017). At air temperatures of 40°C, a small bird loses about 3% of its body mass per hour (e.g. the Lesser Goldfinch, Spinus psaltria; Albright et al., 2017), so the risk of lethal dehydration during prolonged heat exposure is extreme for birds without access to water. Evaporative cooling is also less efficient in humid conditions (Mitchell et al., 2018) and Jacky Winters spent more time panting (an autonomic response to body temperature) when vapor pressure was high.
Once temperatures rose into the 40°Cs, and dry (sensible) cooling was impossible, most of the birds retreated to the ground, taking refuge in shaded crevices or depressions at the base of large trees. These crevices were significantly cooler than any other location we measured (Figure 7). Inside refuges, the birds huddled together and lay with their bellies contacting the sand to facilitate conductive cooling. Jacky Winters do not normally perch within 5cm of conspecifics (Sharpe LL, pers. obs.), yet we observed one pair huddling with a territorial intruder they had been chasing earlier in the day. A similar breakdown of territoriality occurs in Arizona Grasshopper Sparrows (Ammodramus savannarum ammolegus) at high temperatures when the birds congregate in dense shrubs (Ruth et al., 2020). Huddling reduces the amount of surface area an animal exposes to the environment (Gilbert et al., 2010), which lessens heat gain when environmental temperature exceeds body temperature. Huddling inside a refuge also reduces evaporative water loss, due to increased humidity within the refuge (Luna et al., 2020), which is potentially critical for survival during prolonged heat exposure. The thermal and water-saving benefits of huddling may explain why, on the hottest days, we repeatedly observed large, mixed-species aggregations of small passerines sheltering together within single tree-base refuges.
Most Jacky Winters modified their behavior and microsite use to minimize convective heat transfer once air temperatures rose above body temperature. This behavioral transition, when heat gradients reverse, may prove to be critical for endotherms now experiencing unprecedented heat. Although we expect organisms to adopt behaviors that maximize their fitness, this is not always the case when individuals encounter novel conditions (e.g., responses to novel predators, artificial light, anthropogenic food; Tuomainen and Candolin, 2011). Although some species may have sufficient cognitive and behavioral flexibility to cope with this transition point (Ghalambor et al., 2007; Mills and McGraw, 2021), for others, air temperatures above body temperature may act as an insurmountable barrier to effective behavioral thermal regulation. Australian flying foxes (Pteropus spp.), for example, exhibit a suite of thermoregulatory behaviors (e.g., wing-fanning to maximize convective and radiant cooling, shade-use, panting and saliva-spreading; Welbergen et al., 2008) but in extreme heat they do not seek cooler microsites or adopt behaviors that minimize dry heat exchange. As a consequence, they succumb to lethal heat stress at air temperatures > 42°C, and 45,500 (in 600 colonies in SE Queensland) died on a single day in 2014 (Welbergen et al., 2014). This vulnerability to extreme heat may be much more widespread than we recognize, particularly among small endotherms (McKechnie et al., 2021) because few species are as readily observable as flying foxes, which roost in large, noisy colonies, and attempts to quantify individual responses to extreme heat are rare (Buchholz et al., 2019).
Potential Costs of Behavioral Thermoregulation
Even when lethal stress is avoided, thermoregulatory behaviors carry opportunity costs, with organisms known to reduce sociality, territorial defense, food intake or offspring care (Cunningham et al., 2021). Panting, for example, reduces foraging success in birds that excavate subterranean prey with their beak (du Plessis et al., 2012; van de Ven et al., 2019) and the utilization of cooler microhabitats is often associated with a reduction in forage quality or foraging success (Mason et al., 2017; van de Ven et al., 2019). With greater heat exposure, these costs may become unsustainable. For example, in Mexico’s Sceloporus lizards, the growing need for extended periods of inactivity during the breeding season, to avoid lethal heat stress, has resulted in the extinction of 12% of monitored populations (Sinervo et al., 2010). We found that the Jacky Winters’ use of elevated perches carried foraging costs, (after taking into account the effect of temperature), with birds making fewer prey catching attempts and flying further to capture prey. Perching in shade may also impair the birds’ ability to detect prey because they tended to make fewer foraging attempts from shaded perches. Southern Fiscals (Lanius collaris)—an African “perch and pounce” avian predator—consume 50% less prey when hunting from shaded perches (Cunningham et al., 2015), although this could be due to decreased foraging effort associated with temperature or time of day.
Reduced food intake—due to the necessity for inactivity and voluntary fasting at high temperatures—is a major cost of thermoregulatory behaviors in endotherms (Youngentob et al., 2021). By mounting video cameras at refuges, we found that Jacky Winters remained within refuges for up to 8 h and only emerged just before sunset (when air temperature dropped to about 38°C), precluding any afternoon foraging. Under these conditions, the birds lose > 2% of body mass per day (Sharpe et al., 2019), and similar losses occur in other wild avian species (du Plessis et al., 2012; Gardner et al., 2016; van de Ven et al., 2019). Such loss of body condition is likely to have long-term consequences for individual health, fitness and survival (Cunningham et al., 2021; Youngentob et al., 2021). Although Jacky Winters can partially recoup lost body condition during the interval between heat waves (Sharpe et al., 2019), the predicted increase in the frequency and duration of heat wave events is likely to have a detrimental impact.
In an effort to compensate for lost foraging time on very hot days, the Jacky Winters appeared to pre-emptively increase their foraging effort on the mornings of extremely hot days (Figure 3). Given that this species is reliant solely on dietary water, obtaining sufficient food prior to prolonged heat exposure will be critical for avoiding lethal dehydration. Pre-emptive foraging has also been observed in other avian species (Carroll et al., 2015; Cooper et al., 2019) but is reliant upon the birds’ ability to anticipate forthcoming conditions. Overnight temperature seems to be the most likely cue, as evidenced by an avian mass mortality event that occurred on a single, isolated hot day in Western Australia in 2010, when early morning temperatures were indistinguishable from those of previous, much cooler days (Cooper et al., 2019). The short-term predictability of heat waves may be critical to the survival of arid zone birds.
Thermally Buffered Microhabitats
The Jacky Winters’ use of different microhabitats altered markedly as temperatures increased and at high temperatures the birds used the largest trees available to them. Surface temperatures at different microsites varied by up to a 35°C on the hottest days (air: > 44°C; sun-exposed sand: 77°C; tree-base refuge: 42°C) but thermally buffered microsites were rare. Tree-base refuges, the coolest sites, were only, on average, 2°C cooler than air temperature. The presence of trees and leaf-litter ground cover reduced surface temperatures, but tree size had the greatest influence: the larger the mallee eucalypt, the cooler the surface temperatures at its base, including within refuges (Figures 8, 9), presumably due to their larger, multitiered canopies (Lindenmayer et al., 2014). This greater leaf area not only increases shade below the tree but amplifies levels of evapotranspiration (Sanusi et al., 2017). Transpiration from leaves directly reduces air temperature below a trees’ canopy by 1 to 8°C, depending on species and environmental conditions (Rahman et al., 2020).
Large, fully mature mallee eucalypts also provided the birds with the opportunity to perch higher (giving them access to higher wind velocities) and were significantly more likely to contain potential tree-base refuges. In fact, tree-base hollows, the best quality refuges, were found only in the largest mallees (trunk circumference > 30 cm). The importance of large trees to the survival of Jacky Winters became apparent when air temperatures rose into the high 40°Cs. Although only one individual succumbed to acute heat stress/dehydration during the data collection period, 29% of our habituated adults died during a severe heat wave 3 weeks prior to data collection, when air temperature attained a record-breaking 48.8°C. These deaths appeared to be concentrated in parts of our study area that did not contain large trees, and although we did not verify this quantitatively, it underscores the importance of thermally buffered refuges for organisms facing unprecedented heat exposure.
Large, old trees act as keystone structures in many ecosystems, providing cavity nest-sites and unique microhabitats, facilitating habitat regeneration, storing large quantities of carbon and modifying ecosystem hydrology and water table level (Lindenmayer et al., 2014). However, the potential thermal benefits provided by old, large trees have yet to be quantified and we could find only one published study: in Canada’s boreal forests, stands of mature conifers were significantly cooler than stands of younger trees or shrubland when ambient temperatures were highest, and grizzly bears (Ursus arctos horribilis) favored these old stands at high temperatures (Pigeon et al., 2017). Interspecific comparisons of urban trees reveal that size per se is not a determinant of a species’ thermal buffering properties because small tree species often have denser canopies, and it is leaf area—and rate of evapotranspiration—that dictate cooling effect (Sanusi et al., 2017; Rahman et al., 2020). Arid adapted species provide less thermal benefit because they have lower transpiration rates. Although the thermal buffering provided by mallee eucalypts at our semi-arid site would be constrained by their restricted evapotranspiration rates and limited shade generation (the leaves of arid-adapted eucalypts are vertically aligned to minimize sun exposure at the hottest time of day; King, 1997), the largest trees at our site may have accessed ground water unavailable to smaller conspecifics (Lindenmayer et al., 2014), potentially augmenting their value as thermal refuges.
Climate Change and Adaptation Management
Our finding that the availability and value of thermal refuges increases with the size of mallee eucalypts has important management implications. Mallee stems are usually killed by wildfire and high intensity planned burns, hence trunk size and tree height increase with time since fire (Yates et al., 2017; Clarke et al., 2021). Indeed, it takes 60 years for mallees to attain full canopy height, and 40–60 years to develop hollows in living timber (Haslem et al., 2011; Clarke et al., 2021), likely reflecting their value as thermal refuges. Climate change is expected to influence the incidence of wildfires in mallee habitat; in mallee regions of Victoria, for example, the number of days of extreme fire danger and the occurrence of dry lightning are predicted to increase (Clarke et al., 2021). Whether this leads to increased fire incidence depends on their interaction with reductions in fuel biomass and continuity, associated with increasing aridity (Bradstock, 2010). Nevertheless, any increase in fire frequency would prevent mallee eucalypts from attaining the large size required for thermal buffering.
The world’s dryland areas are predicted to become increasingly arid, with worsening droughts (IPCC, 2021), which reduce vegetation cover and can permanently alter habitat structure (Pritzkow et al., 2021). At our study site, densely leaved, green shrubs—identified as critical thermal refuges in other terrestrial habitats (Carroll et al., 2015; Anthony et al., 2020; Ruth et al., 2020)—were simply not present during the data collection period due to 2 years of severe drought. In previous summers, we had observed many small passerines (although not Jacky Winters) sheltering within such shrubs at high temperatures. The loss of this microhabitat, combined with the reduction in leaf area that eucalypts suffer during drought (Pritzkow et al., 2021), is likely to seriously impair the ability of avian species to survive heat wave events.
Options to help biodiversity adapt to climate change are limited, but the relationship between fire interval, tree size and thermal refuges offers an opportunity for “low regrets” intervention (Prober et al., 2019) in mallee ecosystems. Managing fire to maintain and increase the area of mallee in older age classes is likely to maximize the availability of thermal refuges, thereby reducing mortality during heat waves. Later seral stages in mallee also provide significant habitat structural elements that support a diversity of reptiles, birds and mammals (Yates et al., 2017; Clarke et al., 2021). Another option worth considering relates to habitat structures known to act as critical refuges in other hot, arid ecosystems. Underground burrows are exploited by a huge array of species during extreme heat: four lark species use lizard burrows in the Arabian Desert (Williams et al., 1999) and more than 50 vertebrate species shelter in gopher tortoise (Gopherus polyphemus) burrows in south-eastern United States (Walde et al., 2009). However, burrows are no longer present in SE Australia’s mallee woodlands due to the extirpation of at least three species of medium-sized, burrowing marsupial (Fleming et al., 2014). The reintroduction of burrowing species could greatly enhance the availability of thermally buffered microsites within this habitat.
Conclusion
Jacky Winters exhibited a broad and nuanced repertoire of thermoregulatory behaviors, which appeared “appropriate” to environmental conditions, based on principles of heat transfer. However, the effectiveness of their behavioral strategies was dependent on the quality and availability of specific microhabitats. There is an urgent need for studies that quantify how wild individuals respond behaviorally to heat waves and identify which fine-scale components of their habitat serve as thermal refuges (Buchholz et al., 2019). Only once we have this information can we hope to develop effective conservation strategies to help to mitigate the adverse impact of rising global temperatures on biodiversity.
Data Availability Statement
The raw data supporting the conclusions of this article will be made available by the authors, without undue reservation.
Ethics Statement
Ethics approval for this research was granted by the Australian National University Animal Experimentation Ethics Committee (A2018/29), and the work was approved by the South Australian Department of Environment and Water (E26755-1) and the Australian Bird and Bat Banding Scheme (153506).
Author Contributions
JG conceived the study and performed the statistical analyses. LS designed the methodology and wrote the manuscript. LS and JG collected the data. JG and SP reviewed the manuscript. All authors secured funding and approved the final manuscript.
Funding
This work was supported by the Australian Research Council (DP1810101235), the Wettenhall Environment Trust and the Centre for Biodiversity Analysis (Ignition Project grant). JG was supported by an Australian Research Council Future Fellowship (FT150100139).
Conflict of Interest
The authors declare that the research was conducted in the absence of any commercial or financial relationships that could be construed as a potential conflict of interest.
Publisher’s Note
All claims expressed in this article are solely those of the authors and do not necessarily represent those of their affiliated organizations, or those of the publisher, the editors and the reviewers. Any product that may be evaluated in this article, or claim that may be made by its manufacturer, is not guaranteed or endorsed by the publisher.
Acknowledgments
We would like to thank the Australian Landscape Trust for permission to work at Calperum Station and Belinda Cale for assistance in the field. We would also like to thank Victoria Sohm, Thalia Williamson, Kevin Yang and Kalya Subasinghe for help with data collection, and Peter Marsack for feedback on the manuscript.
References
Albright, T. P., Mutiibwaa, D., Gersond, A. R., Smithe, E. K., Talbote, W. A., O’Neille, J. J., et al. (2017). Mapping evaporative water loss in desert passerines reveals an expanding threat of lethal dehydration. PNAS 114, 2283–2288. doi: 10.1073/pnas.1613625114
Anthony, C. R., Hagen, C. A., Dugger, K. M., and Elmore, R. D. (2020). The effects of fire on the thermal environment of sagebrush communities. J. Thermal Biol. 89:102488. doi: 10.1016/j.jtherbio.2019.102488
Arad, Z., Midtgård, U., and Bernstein, M. H. (1989). Thermoregulation in turkey vultures. vascular anatomy, arteriovenous heat exchange, and behavior. Condor 91, 505–514. doi: 10.2307/1368103
Bates, D., Mächler, M., Bolker, B., and Walker, S. (2015). Fitting linear mixed-effects models using lme4. J. Statistical Software 67, 1–48.
Beale, P. K., Marsh, K. J., Foley, W. J., and Moore, B. D. (2018). A hot lunch for herbivores: physiological effects of elevated temperatures on mammalian feeding ecology. Biol. Rev. 93, 674–692. doi: 10.1111/brv.12364
BOM Bureau of Meteorology, Australia (BOM). Available online at: https://bom.gov.au
Boyles, J. G., Seebacher, F., Smit, B., and McKechnie, A. E. (2011). Adaptive thermoregulation in endotherms may alter responses to climate change. Integrative Comp. Biol. 51, 676–690. doi: 10.1093/icb/icr053
Bradstock, R. A. (2010). A biogeographic model of fire regimes in Australia: current and future implications. Glob. Ecol. Biogeog. 19, 145–158. doi: 10.1111/j.1466-8238.2009.00512.x
Briscoe, N. J., Handasyde, K. A., Griffiths, S. R., Porter, W. P., Krockenberger, A., and Kearney, M. R. (2014). Tree-hugging koalas demonstrate a novel thermoregulatory mechanism for arboreal mammals. Biol. Lett. 10:20140235. doi: 10.1098/rsbl.2014.0235
Buchholz, R., Banusiewicz, J. D., Burgess, S., Crocker-Buta, S., Eveland, L., and Fuller, L. (2019). Behavioural research priorities for the study of animal response to climate change. Animal Behav. 150, 127–137.
Carroll, J. M., Davis, C. A., Elmore, R. D., Fuhlendorf, S. D., and Thacker, E. T. (2015). Thermal patterns constrain diurnal behavior of a ground-dwelling bird. Ecosphere 6, 222–237. doi: 10.1890/ES15-00163.1
Chen, J. Q., Saunders, S. C., Crow, T. R., Naiman, R. J., Brosofske, K. D., and Franklin, J. F. (1999). Microclimate in forest ecosystem and landscape ecology – variations in local climate can be used to monitor and compare the effects of different management regimes. BioScience 49, 288–297. doi: 10.2307/1313612
Clarke, M. F., Kelly, L. T., Avitabile, S. C., Benshemesh, J., Callister, K. E., Driscoll, D. A., et al. (2021). Fire and its interactions with other drivers shape a distinctive, semi-arid ‘Mallee’. Ecosystem. Front. Ecol. Evol. 9:647557. doi: 10.3389/fevo.2021.647557
Conradie, S. R., Woodborne, S. M., Wolf, B. O., Pessato, A., Mariette, M. M., and McKechnie, A. E. (2020). Avian mortality risk during heat waves will increase greatly in arid Australia during the 21st century. Conserv. Physiol. 8:coaa048. doi: 10.1093/conphys/coaa048
Cooper, C. E., Withers, P. C., Hurley, L. L., and Griffith, S. C. (2019). The field metabolic rate, water turnover, and feeding and drinking behavior of a small avian desert granivore during a summer heatwave. Front. Physiol. 10:1405. doi: 10.3389/fphys.2019.01405
Cunningham, S. J., Gardner, J. L., and Martin, R. O. (2021). Opportunity costs and the response of birds and mammals to climate warming. Front. Ecol. Environ. 19:300–307. doi: 10.1002/fee.2324
Cunningham, S. J., Martin, R. O., and Hockey, P. A. R. (2015). Can behaviour buffer the impacts of climate change on an arid-zone bird? Ostrich 86, 119–126. doi: 10.2989/00306525.2015.1016469
Dawson, T. J. (1973). Thermoregulatory responses of the arid zone kangaroos, Megaleia rufa and Macropus robustus. Comp. Biochem. Physiol. A Comp. Physiol. 46, 153–169. doi: 10.1016/0300-9629(73)90568-90569
Donaghey, R., and Donaghey, C. (2017). Parental care and breeding strategies of the Jacky Winter and its life-history traits compared with other Australasian robins, and northern temperate and tropical songbirds. Australian Field Ornithol. 34, 98–110.
du Plessis, K. L., Martin, R. O., Hockey, P. A. R., Cunningham, S. J., and Ridley, A. R. (2012). The costs of keeping cool in a warming world: implications of high temperatures for foraging, thermoregulation and body condition of an arid-zone bird. Global Change Biol. 18, 3063–3070. doi: 10.1111/j.1365-2486.2012.02778.x
Fleming, P. A., Anderson, H., Prendergast, A. S., Bretz, M. R., Valentine, L. E., and Hardy, G. E. S. (2014). Is the loss of Australian digging mammals contributing to a deterioration in ecosystem function? Mammal Rev. 44, 94–108. doi: 10.1111/mam.12014
Gardner, J. L., Amano, T., Sutherland, W. J., Clayton, M., and Peters, A. (2016). Individual and demographic consequences of reduced body condition following repeated exposure to high temperatures. Ecology 97, 786–795. doi: 10.1890/15-0642.1
Ghalambor, C. K., McKay, J. K., Carroll, S. P., and Reznick, D. N. (2007). Adaptive versus non-adaptive phenotypic plasticity and the potential for contemporary adaptation in new environments. Funct. Ecol. 21, 394–407.
Gilbert, C., McCafferty, D., Le Maho, Y., Martrette, J., Giroud, S., Blanc, S., et al. (2010). One for all and all for one: the energetic benefits of huddling in endotherms. Biol. Rev. 85, 545–569. doi: 10.1111/j.1469-185X.2009.00115.x
Hall, L. E., and Chalfoun, A. D. (2019). Behavioural plasticity modulates temperature-related constraints on foraging time for a montane mammal. J. Anim. Ecol. 88, 363–375. doi: 10.1111/1365-2656.12925
Hartig, F. (2020). DHARMa: Residual Diagnostics for Hierarchical Multi-level/mixed) Regression Model. cran.R-project.org.
Haslem, A., Kelly, L. T., Nimmo, D. G., Watson, S. J., Kenny, S. A., and Bennett, A. F. (2011). Habitat or fuel? Implications of long-term, post-fire dynamics for the development of key resources for fauna and fire. J. Appl. Ecol. 48, 247–256. doi: 10.1111/j.1365-2664.2010.01906.x
Hetem, R. S., Strauss, W. M., Fick, L. G., Maloney, S. K., Meyer, L. C. R., Shobrak, M., et al. (2012). Activity re-assignment and microclimate selection of free-living Arabian oryx: responses that could minimise the effects of climate change on homeostasis? Zoology 115, 411–416. doi: 10.1016/j.zool.2012.04.005
Higgins, P. J., and Peter, J. M. (eds) (2002). Handbook of Australian, New Zealand & Antarctic Birds: Pardalotes to Shrike-Thrushes. Oxford: Oxford University Press.
Holm, E., and Edney, E. B. (1973). Daily activity of namib desert arthropods in relation to climate. Ecology 54, 45–56.
IPCC (2021). “Climate change 2021: the physical science basis,” in Proceedings of the Contribution of Working Group I to the 6th Assessment Report of the Intergovernmental Panel on Climate Change, (Cambridge: Cambridge University Press). doi: 10.1007/s10584-021-03233-7
King, D. A. (1997). The functional significance of leaf angle in Eucalypts. Australian J. Botany 45, 619–639. doi: 10.1071/bt96063
Lindenmayer, D. B., Laurance, W. F., Franklin, J. F., Likens, G. E., Banks, S. C., and Stein, J. A. R. (2014). New policies for old trees: averting a global crisis in a keystone ecological structure. Conservation Lett. 7, 61–69. doi: 10.1111/conl.12013
Luna, F., Sumbera, R., Okrouhlík, J., Mladenkova, N., and Antenucci, C. D. (2020). Evaporative water loss in seven species of fossorial rodents: does effect of degree of fossoriality and sociality exist? J. Thermal Biol. 89:102564. doi: 10.1016/j.jtherbio.2020.102564
Mason, T. H. E., Brivio, F., Stephens, P. A., Apollonio, M., and Grignolio, S. (2017). The behavioral trade off between thermoregulation and foraging in a heat-sensitive species. Behav. Ecol. 28, 908–918. doi: 10.1093/beheco/arx057
McKechnie, A. E., and Wolf, B. O. (2010). Climate change increases the likelihood of catastrophic avian mortality events during extreme heat waves. Biol. Lett. 6, 253–256. doi: 10.1098/rsbl.2009.0702
McKechnie, A. E., and Wolf, B. O. (2019). The physiology of heat tolerance in small endotherms. Physiology 34, 302–313. doi: 10.1152/physiol.00011.2019
McKechnie, A. E., Gerson, A. R., McWhorter, T. J., Smith, E. K., Talbot, W. A., and Wolf, B. O. (2017). Avian thermoregulation in the heat: evaporative cooling in five Australian passerines reveals within-order biogeographic variation in heat tolerance. J. Exp. Biol. 220, 2436–2444. doi: 10.1242/jeb.155507
McKechnie, A. E., Rushworth, I. A., Myburgh, F., and Cunningham, S. J. (2021). Mortality among birds and bats during an extreme heat event in eastern South Africa. Austral Ecol. 46, 687–691. doi: 10.1111/aec.13025
Mills, R., and McGraw, K. J. (2021). Cool birds: facultative use by an introduced species of mechanical air conditioning systems during extremely hot outdoor conditions. Biol. Lett. 17:20200813. doi: 10.1098/rsbl.2020.0813
Mitchell, D., Snelling, E. P., Hetem, R. S., Maloney, S. K., Strauss, W. M., and Fuller, A. (2018). Revisiting concepts of thermal physiology: predicting responses of mammals to climate change. J. Animal Ecol. 87, 956–973. doi: 10.1111/1365-2656.12818
Mole, M. A., DÁraujo, S. R., van Aarde, R. J., Mitchell, D., and Fuller, A. (2016). Coping with heat: behavioural and physiological responses of savanna elephants in their natural habitat. Conserv. Physiol. 4:cow044. doi: 10.1093/conphys/cow044
Muiruri, H. K., and Harrison, P. C. (1991). Effect of peripheral foot cooling on metabolic-rate and thermoregulation of fed and fasted chicken hens in a hot environment. Poult. Sci. 70, 74–79. doi: 10.3382/ps.0700074
Neel, L. K., and McBrayer, L. D. (2018). Habitat management alters thermal opportunity. Funct. Ecol. 32, 2029–2039. doi: 10.1111/1365-2435.13123
Olinger, R. (2017). How does Temperature Affect Fork-tailed Drongo, Dicrurus adsimilis, Foraging Effort, Nestling Provisioning and Growth Rates?. MSc thesis, South Africa: Univ. of Cape Town.MScthesis
Pigeon, K. E., Cardinal, E., Stenhouse, G. B., and Côté, S. D. (2017). Staying cool in a changing landscape: the influence of maximum daily ambient temperature on grizzly bear habitat selection. Oecologia 181, 1101–1116. doi: 10.1007/s00442-016-3630-5
Pritzkow, C., Szota, C., Williamson, V., and Arndt, S. K. (2021). Previous drought exposure leads to greater drought resistance in eucalypts through changes in morphology rather than physiology. Tree Physiol. 41:taa176. doi: 10.1093/treephys/tpaa176
Prober, S. M., Doerr, V., Broadhurst, L., Williams, K. J., and Dickson, F. (2019). Shifting the conservation paradigm - a synthesis of options for renovating nature under climate change. Ecol. Monographs 89:e01333. doi: 10.1002/ecm.1333
Rahman, M. A., Stratopoulos, L. M. F., Moser-Reischl, A., Zolch, T., Haberle, K., Rotzer, T., et al. (2020). Traits of trees for cooling urban heat islands: a meta-analysis. Building Environ. 170:106606. doi: 10.1016/j.buildenv.2019.106606
Recher, H., and Davis, W. (1998). The foraging profile of a wandoo woodland avifauna in early spring. Austral Ecol. 23, 514–527.
Ricklefs, R. E., and Hainsworth, F. R. (1968). Temperature dependent behavior of the cactus wren. Ecology 49, 227–233. doi: 10.2307/1934451
Riddell, E. A., Iknayan, K. J., Wolf, B. O., Sinervo, B., and Beissinger, S. R. (2019). Cooling requirements fuelled the collapse of a desert bird community from climate change. PNAS 116, 21609–21615. doi: 10.1073/pnas.1908791116
Ruth, J. M., Talbot, W. A., and Smith, E. K. (2020). Behavioral response to high temperatures in a desert grassland bird: use of shrubs as thermal refugia. Western North Am. Natural. 80, 265–275.
Ruthrof, K. X., Breshears, D. D., Fontaine, J. B., Froend, R. H., Matusick, G., et al. (2018). Subcontinental heat wave triggers terrestrial and marine, multi-taxa responses. Sci. Rep. 8:13094. doi: 10.1038/s41598-018-31236-5
Sanusi, R., Johnstone, D., Maya, P., and Livesley, S. J. (2017). Microclimate benefits that different street tree species provide to sidewalk pedestrians relate to differences in Plant Area Index. Landscape Urban Plann. 157, 502–511.
Sharpe, L. L., Bayter, C., and Gardner, J. L. (2021). Too hot to handle? Behavioural plasticity during incubation in a small, Australian passerine. J. Thermal Biol. 98:102921. doi: 10.1016/j.jtherbio.2021.102921
Sharpe, L. L., Cale, B., and Gardner, J. L. (2019). Weighing the cost: the impact of serial heatwaves on body mass in a small Australian passerine. J. Avian Biol. 50:e02355. doi: 10.1111/jav.02355
Sinervo, B., Méndez-de-la-Cruz, F., Miles, D. B., Heulin, B., Bastiaans, E., and Sites, J. W. (2010). Erosion of lizard diversity by climate change and altered thermal niches. Science 328, 894–899. doi: 10.1126/science.1184695
Stillman, J. H. (2019). Heat waves, the new normal: summertime temperature extremes will impact animals, ecosystems, and human communities. Physiology 34, 86–100. doi: 10.1152/physiol.00040.2018
Stuart-Fox, D., Newton, E., and Clusella-Trullas, S. (2017). Thermal consequences of colour and near-infrared reflectance. Phil. Trans. R. Soc. B 372:20160345. doi: 10.1098/rstb.2016.0345
Tattersall, G. J., Andrade, D. V., and Abe, A. S. (2009). Heat exchange from the toucan bill reveals a controllable vascular thermal radiator. Science 325, 468–470. doi: 10.1126/science.1175553
Thomas, C. D., Franco, A. M. A., and Hill, J. K. (2006). Range retractions and extinction in the face of climate warming. Trends Ecol. Evol. 21, 415–416. doi: 10.1016/j.tree.2006.05.012
Tieleman, B. I., and Williams, J. B. (1999). The role of hyperthermia in the water economy of desert birds. Physiol. Biochem. Zool. 72, 87–100. doi: 10.1086/316640
Tuomainen, U., and Candolin, U. (2011). Behavioural responses to human-induced environmental change. Biol. Rev. Camb. Philos. Soc. 86, 640–657. doi: 10.1111/j.1469-185X.2010.00164.x
van de Ven, T. M. F. N., McKechnie, A. E., and Cunningham, S. J. (2019). The costs of keeping cool: behavioural trade-offs between foraging and thermoregulation are associated with significant mass losses in an arid-zone bird. Oecologia 18, 3063–3070. doi: 10.1007/s00442-019-04486-x
Venables, W., and Ripley, B. (2002). Modern Applied Statistics with S, 2nd Edn. New York, NY: Springer.
Walde, A. D., Walde, A. M., Delaney, D. K., and Pater, L. L. (2009). Burrows of desert tortoises (Gopherus agassizii) as thermal refugia for horned larks (Eremophila apestris) in the Mojave desert. Southwestern Natural. 54, 375–381.
Weissenböck, N. M., Weiss, C. M., Schwammer, H. M., and Kratochvil, H. (2010). Thermal windows on the body surface of African elephants (Loxodonta africana) studied by infrared thermography. J. Therm. Biol. 35, 182–188.
Welbergen, J. A., Booth, C., and Martin, J. (2014). Killer Climate: Tens of Thousands of Flying Foxes Dead in a Day. Available online at: https://theconversation.com/killer-climate-tens-of-thousands-of-flying-foxes-dead-in-a-day-23227.
Welbergen, J. A., Klose, S. M., Markus, N., and Eby, P. (2008). Climate change and the effects of temperature extremes on Australian flying-foxes. Proc. R. Soc. B 275, 419–425. doi: 10.1098/rspb.2007.1385
Williams, J. B., Tieleman, B. I., and Shobrak, M. (1999). Lizard burrows provide thermal refugia for larks in the Arabian desert. Condor 101, 714–717. doi: 10.2307/1370208
Wolf, B. O., and Walsberg, G. E. (1996). Thermal effects of radiation and wind on a small bird and implications for microsite selection. Ecology 77, 2228–2236. doi: 10.2307/2265716
Wolf, B. O., Wooden, K. M., and Walsberg, G. E. (2000). Effects of complex radiative and convective environments on the thermal biology of the white-crowned sparrow (Zonotrichia leucophrys gambelii). J. Exp. Biol. 203, 803–811. doi: 10.1242/jeb.203.4.803
Wood, K., Thompson, N., and Ley, A. (2008). Breeding territories and breeding success of the jacky winter Microeca fascinans in South-eastern Queensland. Australian Field Ornithol. 25, 121–131.
Yates, C. J., Gosper, C. R., Hopper, S. D., Keith, D. A., Prober, S. M., and Tozer, M. (2017). “Mallee woodlands and shrublands – the mallee, muruk/muert and maalok vegetation of Southern Australia,” in Australian Vegetation, 3rd Edn, ed. D. Keith (Cambridge: Cambridge University Press), 570–598.
Keywords: behavioral thermoregulation, heat waves, heat dissipation, microhabitat, thermal profile, avian thermoregulation, large mallee trees, thermal refuge
Citation: Sharpe LL, Prober SM and Gardner JL (2022) In the Hot Seat: Behavioral Change and Old-Growth Trees Underpin an Australian Songbird’s Response to Extreme Heat. Front. Ecol. Evol. 10:813567. doi: 10.3389/fevo.2022.813567
Received: 12 November 2021; Accepted: 08 February 2022;
Published: 04 March 2022.
Edited by:
Andrew McKechnie, University of Pretoria, South AfricaReviewed by:
Edward Narayan, The University of Queensland, AustraliaMichael Fraser Clarke, La Trobe University, Australia
Copyright © 2022 Sharpe, Prober and Gardner. This is an open-access article distributed under the terms of the Creative Commons Attribution License (CC BY). The use, distribution or reproduction in other forums is permitted, provided the original author(s) and the copyright owner(s) are credited and that the original publication in this journal is cited, in accordance with accepted academic practice. No use, distribution or reproduction is permitted which does not comply with these terms.
*Correspondence: Lynda L. Sharpe, c2hhcnBlbHluZGFAaG90bWFpbC5jb20=
†Present address: Lynda L. Sharpe, Loch Sport, VIC, Australia