Linking animal behavior to ecosystem change in disturbed environments
- Organismal and Evolutionary Biology Research Program, University of Helsinki, Helsinki, Finland
Environmental disturbances often cause individuals to change their behavior. The behavioral responses can induce a chain of reactions through the network of species interactions, via consumptive and trait mediated connections. Given that species interactions define ecosystem structure and functioning, changes to these interactions often have ecological repercussions. Here, we explore the transmission of behavioral responses through the network of species interactions, and how the responses influence ecological conditions. We describe the underlying mechanisms and the ultimate impact that the behavioral responses can have on ecosystem structure and functioning, including biodiversity and ecosystems stability and services. We explain why behavioral responses of some species have a larger impact than that of others on ecosystems, and why research should focus on these species and their interactions. With the work, we synthesize existing theory and empirical evidence to provide a conceptual framework that links behavior responses to altered species interactions, community dynamics, and ecosystem processes. Considering that species interactions link biodiversity to ecosystem functioning, a deeper understanding of behavioral responses and their causes and consequences can improve our knowledge of the mechanisms and pathways through which human activities alter ecosystems. This knowledge can improve our ability to predict the effects of ongoing disturbances on communities and ecosystems and decide on the interventions needed to mitigate negative effects.
Introduction
Environments around the world are rapidly changing mainly because of human activities. Habitat destruction, climate change, pollution, invasion of foreign species, and harvesting are drastically altering the living conditions for species (Ceballos et al., 2015; Blowes et al., 2019). The changes influence species directly as well as indirectly through interactions with other affected species and altered abiotic conditions (Tylianakis et al., 2008; Gilman et al., 2010). These direct and indirect impacts influence in turn population dynamics and thereby ecosystem structure, function, and stability (i.e., the ability of the ecosystem to resist change or return to the original state following a perturbation) (Ives and Carpenter, 2007).
The impact that environmental changes have on populations is often mediated by behavioral responses of individuals, particularly at the early stage of environmental change before evolutionary changes have occurred (Tuomainen and Candolin, 2011; Sih, 2013; Wong and Candolin, 2015). The behavioral responses can be direct reactions to the environmental change, such as when animals move away from a disturbed area, or a consequence of changes in physiology or life history traits, such as higher stress levels or reduced size at maturity. These behavioral responses influence in turn other species in the community and can cause cascading effects that ripple through the community via the network of species interactions (Tylianakis et al., 2008; Hoover and Tylianakis, 2012; Palkovacs and Dalton, 2012; Bartley et al., 2019). These cascading effects increase the pathways through which an environmental change reaches a species, and they can have a larger impact on species than the direct impact of the environmental change (Ockendon et al., 2014). The impact of the behavioral responses can be further altered by feedbacks among species and time lags, and result in complex changes to the species community.
The impact that behavioral responses of individuals have on community structure and ecosystem function depends on the traits and abundance of the species, with some species have a larger impact than others, such as keystone species (Paine, 1966; Power et al., 1996), dominant species (Avolio et al., 2019), foundation species (Ellison, 2019), and ecosystem engineers (Jones et al., 1994; Wright and Jones, 2006). Such species have been termed ‘biotic multipliers’ (Zarnetske et al., 2012; Urban et al., 2017). Focusing research on these species and their behavioral responses can provide essential information of the effects that environmental disturbances have on ecosystems and the underlying mechanisms (Urban et al., 2017).
Here, the aim is to give an overview of our current knowledge of how changes in the behavior of ecologically central species influence species interaction networks and thereby ecosystem structure, function, and stability. We provide a conceptual framework that links behavioral responses to species interactions, communities, and ecosystems, and discuss the factors that influence these links (Figure 1). We begin by explaining the central role that behavioral responses of biotic multipliers have in mediating impacts of environmental change on ecosystems. We then sketch out the impact that various environmental changes have on the behavior of species, and how these behavioral responses in turn influence species interactions and community composition. Next, we explain how changes in the behavior of individuals and species interactions influence ecological processes, such as biogeochemical cycles, biodiversity, and ecosystem stability and services. We end by outlining key avenues and priorities for future research.
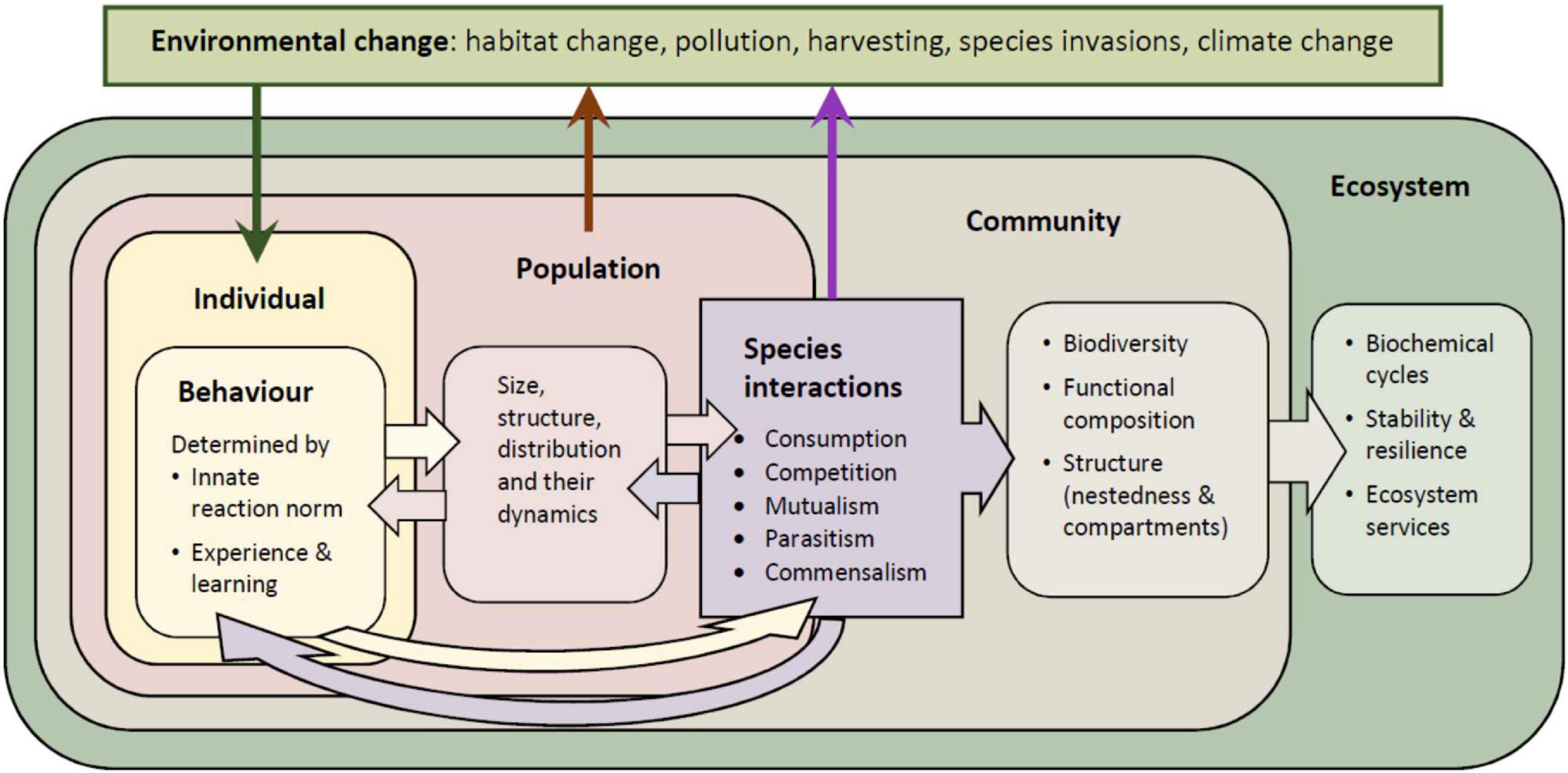
Figure 1. A conceptual overview of the impact that behavioral responses to environmental change can have on populations, communities, and ecosystems, mediateds by species interactions.
Biotic multipliers of disturbances
Biotic multipliers are ecologically important species that regulate or influence the abundance and function of a range of other species, directly or indirectly (Zarnetske et al., 2012). Changes in their behavior can initiate a cascade of effects through species interaction networks. Such species are keystone species, ecosystem engineers, and dominant species. These concepts partly overlap as a species can have multiple functions and belong to several groups (de Visser et al., 2013).
Keystone species
Keystone species are those with a disproportionately large effect on the ecosystems relative to their low biomass (Paine, 1966; Power et al., 1996). They have low functional redundancy, with no other species being able to fill their ecological niche should they disappear from the ecosystem. Thus, without them, the ecosystem would be dramatically different. Typical keystone species are top predators that regulate the abundance and distribution of several prey species and, hence, cause top-down effects that can be transmitted down to primary producers. A classic example is wolves (Canis lupus) that control the abundance of herbivores (if wolf populations are allowed to grow large enough and are not decimated by humans). These keystone predators are not necessarily large, or even at the top of the food web, as demonstrated by the sea star Pisaster ochraceus, which was the first species to be assigned a keystone role in regulating the biodiversity of intertidal plains (Paine, 1966). Similarly, pollinators can be keystone species as they are vital for plant reproduction and diversity.
Ecosystem engineers
Ecosystem engineers are species that modify the habitat and directly or indirectly modulate the availability of resources to other species (Jones et al., 1994). Typical examples are beavers (Castor sp.) and earthworms (Lumbricus sp.). They cause physical changes to biotic and abiotic components of the environment, either via their own physical structures, which can be living or dead (autogenic engineers), or by transforming living or non-living materials from one state to another by mechanical or other means (allogenic engineers). Foundation species, which create conditions required for the persistence of other species, are a subgroup of ecosystem engineers (Jones et al., 1994).
Dominant species
Dominant species are abundant species whose effects on the community and ecosystem is proportional to their abundance (Avolio et al., 2019). They are linked to many species and are main contributors to ecosystem function, such as bioturbation and carbon storage and flows (Dangles and Malmqvist, 2004; Solan et al., 2004; Taylor et al., 2006). Thus, they underpin the provision of many ecosystem services to people. Some dominant species are also ecosystem engineers and foundation species. Changes in their abundance and behavior can significantly impact ecosystem functioning. Examples of dominant species are large bodied zooplankton in lakes without fish predators.
Influence of environmental change on behavior
Mechanisms behind behavioral responses
Human activities that alter abiotic or biotic components of an ecosystem often cause behavioral changes in animals either directly or indirectly through the responses of other individuals or species. How animals respond depends on their genetically determined innate reaction norms, and their experiences and learning possibilities during lifetime (Tuomainen and Candolin, 2011; Sih, 2013; Wong and Candolin, 2015). In some species, experiences of earlier generations can additionally influence responses through transgenerational effects (Bell and Hellmann, 2019)(Figure 1).
Behavioral responses can be adaptive or maladaptive, much depending on the similarity of the new conditions to the past ones; responses are more likely to be adaptive when the environmental change extends earlier encountered conditions, such as a rise in temperature, than when it results in completely new conditions, such as exposure to traffic noise. Yet, the extension of earlier encountered conditions can also result in maladaptive responses, especially when non-linear responses are required to improve fitness. For instance, an animal that linearly increases its activity with increasing temperature may suffer from reduced fitness if the adaptive response would be decrease activity when temperature rises above a certain threshold.
Behavioral responses to disturbances
Animals may respond to environmental change immediately or after a time lag, depending on whether physiological modifications or learning is needed, and whether the change affects them directly (such as higher temperature) or indirectly through interactions with other affected organisms. In addition, the phenotype of an animal influences responses, such as age, sex, body condition, personality, and social dominance (Sih, 2013; McCallum et al., 2021).
Response can be modified over time, depending on the responses of other individuals and species, and on gradual changes in environmental conditions (Tuomainen and Candolin, 2011). In addition, feedback among biotic and abiotic components can modify the responses, as can other environmental disturbances (Jackson et al., 2021). Thus, individuals in a population can show large individual, as well as spatial and temporal variation, in responses.
In the following, we outline the main responses of animals to ongoing human-induced disturbances. We describe their underlying mechanisms and consequences, drawing on theoretical predictions and empirical examples from the literature.
Harvesting
The removal of individuals from a population can influence the behavior of remaining individuals, either by inducing direct behavioral responses or through the selective removal of individuals with particular characteristics, such as bolder individuals (Diaz Pauli and Sih, 2017; Palkovacs et al., 2018; Sbragaglia et al., 2021b). For instance, fisheries that remove the individuals most vulnerable to the specific fishing techniques used alter the composition and behavior of the remaining population (Sbragaglia et al., 2021a).
Harvesting can also alter social interactions, such as aggression, mate choice, and parental care, through effects on the density, structure, or distribution of the population. These changes in social interactions can have further implications for population characteristics, through effects on birth and death rates and dispersal (Diaz Pauli and Sih, 2017; Palkovacs et al., 2018). Similarly, changes in population characteristics, such as its size or age structure, can influence interspecific interactions and influence the dynamics of the involved populations (Hoover and Tylianakis, 2012).
Correspondingly, the decimation of other species, or changes in their populations structure, can alter both intra- and interspecific behavior, such as competition for resources both within and between populations.
Habitat change
Changes in habitat characteristics - through modification, fragmentation, and destruction of the habitat - are common causes of behavioral modifications. For instance, the removal of shielding vegetation causes predators to alter their predatory tactics to prevent prey from detecting them too early in the hunting process (Michel and Adams, 2009). Behavioral responses that influence birth or mortality rates, or dispersal, can in turn influence population characteristics and thereby both intra- and interspecific interactions, as discussed for harvesting.
The responses of other species to changes in the habitat can similarly influence behavior. For instance, the disappearance of prey because of altered vegetation structure may force predators to alter their prey selection (Michel and Adams, 2009).
Pollution
Pollution takes many forms, such as noise, light, and chemical pollution. It creates novel conditions that influence the behavior of individuals, such as their habitat choice and activity (Swaddle et al., 2015; Shannon et al., 2016). Pollution that influences physiological processes, often through hormonal changes, can induce a range of behavioral modifications, from altered activity to reduced investment in reproductive behaviors (Zala and Penn, 2004; Wingfield, 2013; Candolin, 2019a; Candolin and Wong, 2019).
In addition to the direct effects of pollution, indirect effects through species interactions cause behavioral modifications (Willems et al., 2022). In particular, the trophic transmission of chemical pollutants can influence species not directly exposed to the pollutant (Arnot and Gobas, 2006; Previsic et al., 2021). Many compounds, such as heavy metals, pesticides, pharmaceuticals, and microplastics, accumulate in the tissue of organisms, with the concentration increasing for each trophic level (Gall et al., 2015; Puckowski et al., 2016).
Non-native species
Human activities are promoting the spread of species and their possibility of successfully invading new areas (Pysek et al., 2020). These invaders influence the behavior of native species as novel predators, prey, competitors, parasites, and mutualists (Simberloff et al., 2013). Native species may respond with adaptive or maladaptive behavioral responses, which may cause further changes to their population dynamics and species interactions (Simberloff et al., 2013; Pysek et al., 2020).
Non-native species that outcompete native species in resource acquisition often force native species to alter their behavior, such as habitat choice, activity, or aggression (Ricciardi et al., 2013). Similarly, the appearance of a new predator often induce behavioral changes (Sih et al., 2010), as does new parasites by altering the body condition of the species they infect (Barber, 2007). Indirect effects of invaders, through effects on other species, are especially common, given that invaders often alter the structure of food webs and species interactions (Candolin et al., 2018).
Climate change
Global warming and linked effects on the climate, such as altered precipitation and wind patterns, influence the behavior of species, particularly through effects on habitat quality and physiological processes (Buchholz et al., 2019). The impact is often mediated by altered resource availability and quality (Rosenblatt and Schmitz, 2016). For instance, changes in temperature and water availability alters the nutrient content of plants, which influence the foraging behavior of herbivores. If the alteration in behavior influences birth and death rates, or dispersal, the characteristics of the population may change and induce further behavioral modifications (Pelletier and Garant, 2012).
Climate change that alters metabolic rate is another common cause of behavioral modifications, especially in ectothermic species. Warming and enhanced metabolism often increase feeding rate through faster locomotion, more frequent encounters with prey, or faster prey capture, handling time, or ingestion (Rall et al., 2012; Rosenblatt and Schmitz, 2016). On the other hand, if energetic demands increase faster than feeding rates, climate change may eventually lead to starvation and population decline, with further consequences for behavior (Boukal et al., 2019).
Climate change also influences the timing of life-history events, such as the timing of reproduction and migration, given that these are often sensitive to abiotic conditions (Both et al., 2009; Buchanan and Partecke, 2012). Changes in the timing can in turn have implications for population dynamics and species interactions (Kharouba et al., 2018).
Plastic or evolutionary responses?
Animals often respond to a change in the environment with an immediate plastic modification of their behavior (Candolin and Wong, 2012). With time, evolutionary (genetic) changes may take place and result in behaviors that are better adapted to the new conditions. The possibility of genetic adaptation depends, however, on a range of factors, such as the generation time of the species, the presence and nature of genetic variation in the behavior, the rate at which new mutations arise, the size of the population, and the dispersal of individuals and gene flow (Hoffmann and Sgro, 2011; Bell, 2017).
Adaptive behavioral responses can facilitate genetic adaptation by preventing rapid population decline and thereby providing more time for genetic changes (Tuomainen and Candolin, 2011). Behavioral responses can also expose genetic variation that selection can then act on (Candolin and Jensen, 2021). Maladaptive behavioral responses, on the other hand, can have the opposite effect, eventually resulting in population decline and even extinction.
Species with a central role in regulating communities are often larger species with longer generation times. These are unlikely to be able to adapt genetically to rapid human-induced environmental changes, but have to rely on phenotypic plasticity and earlier evolved behavioral reaction norms. Species with shorter generation times and large genetic diversity, often combined with large population sizes, are more likely to adapt genetically to rapid environmental changes (Bell, 2017). Thus, many ecologically important species, such as many top predators with longer generation times, have to rely on behavioral reaction norms that evolved before the environment changed, which increases the probability of maladaptive responses.
The transmission of effects through species interaction networks
Mechanisms
Behavioral alterations by one species influence other species via the network of species interactions (Figure 2). Behavioral responses can also alter the disturbance itself. For instance, increased foraging activity by some consumers in an anthropogenically eutrophied habitat binds nutrients into biomass, which mitigates the impact of eutrophication on other species (Candolin, 2019b).
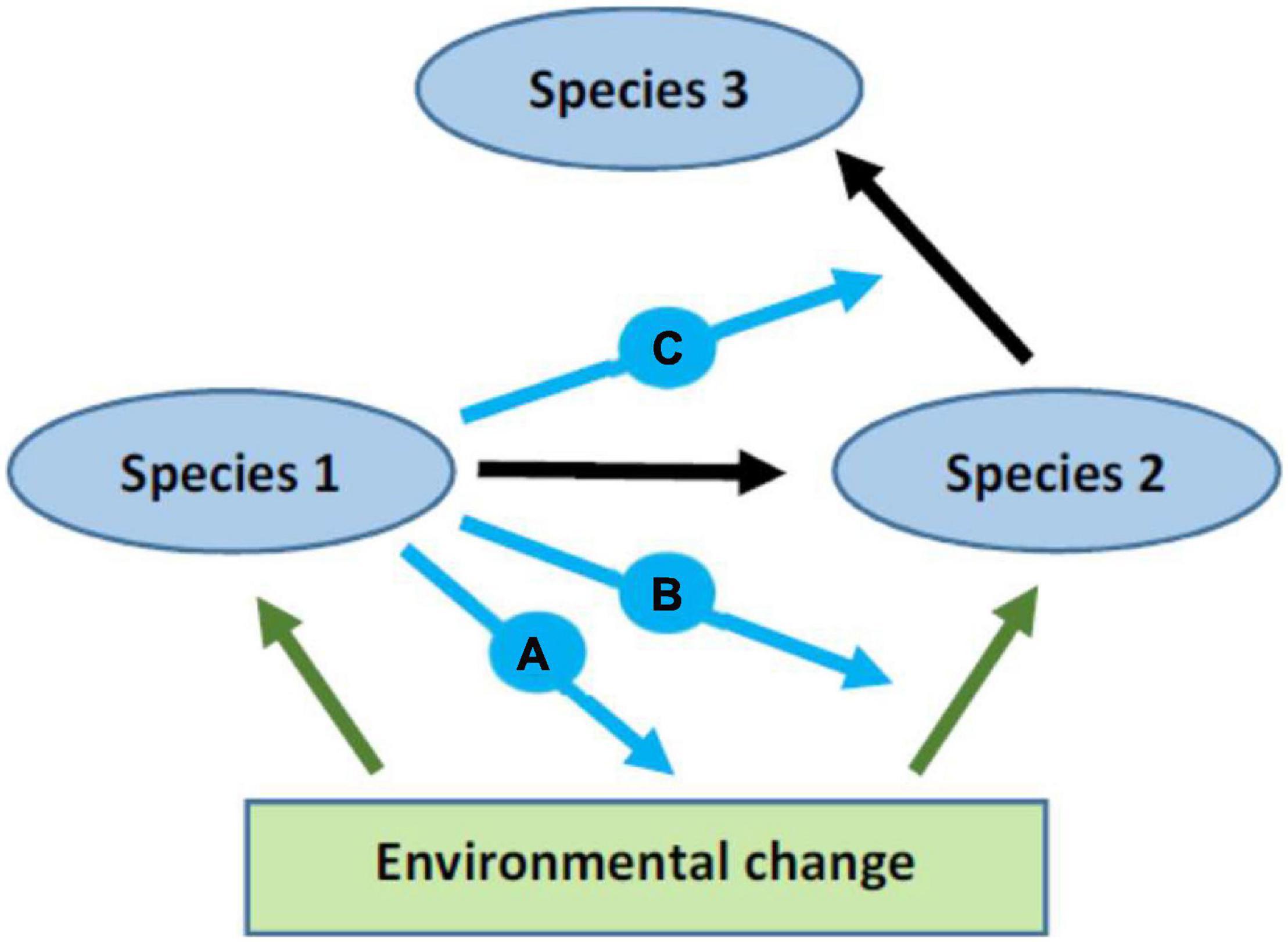
Figure 2. Illustration of the direct and indirect pathways through which changes in the behavior of one species can influence other species. Environmental change influences species 1 and 2 directly (green arrows), and changes in the behavior of species 1 influence in turn species 2 (black arrow), for instance through predation. Species 1 can influence species 2 also by altering the effect of the environmental change on species 2 (blue arrows a and b). Examples are (A) declining prey abundance that is amplified by the behavioural response of species 1, and (B) influence of species 1 on the distribution of species 2 and thereby on its exposure to the environmental change. Changes in species 2 influence in turn species 3 (black arrow), and the effect can be modulated by species 1 (blue arrow c), for instance by species 1 altering the environment and, thus, encounters between species 2 and 3. Feedbacks between the species can further alter the impacts of species 1 on the two other species.
The impact that behavioral responses have on linked species can be trait or density-mediated, respectively (Abrams, 1995; Werner and Peacor, 2003; Schmitz and Trussell, 2016). Trait-mediated effects arise when the behavioral response of a species directly influences other species. Density-mediated effects arise when the behavioral response alters the density of the species itself, through impact on birth, death, or dispersal rates, and this influences other species (Carpenter and Kitchell, 1988; Lima and Dill, 1990). These trait- and density-mediated effects can result in cascades of effects that ripple through the species interaction network, through top-down, bottom-up, and lateral effects, as well as through changes in nutrient recycling and abiotic conditions (Leroux and Loreau, 2010; Heath et al., 2014).
Top-down effects
Top consumers are important regulators of food webs. Their removal often has cascading impacts on lower trophic levels, which alters the abundance, biomass, and distribution of species (Ripple et al., 2014; Rasher et al., 2020). In particular, the current extinction and decline of top consumers – termed trophic downgrading - is triggering waves of secondary extinctions and the disassembly of communities (Estes et al., 2011; Donohue et al., 2017). For instance, mild winters have decreased the ability of wolves (Canis lupus) to kill moose (Alces alces) on Isle Royale in Michigan, which has reduced their abundance and allowed the moose population to grow (Wilmers et al., 2006).
The often profound impact that the decline of top consumers have on species communities are due their low diversity and functional redundancy - their loss and function cannot be compensated by other species (Duffy, 2003). Top consumers are also more sensitivity to environmental changes than most species at lower trophic levels, because of their larger body size and higher metabolic demands and resource requirements (Urban et al., 2017). Thus, many human-caused ecosystem changes are mediated by the decline of top consumers.
The cascading effects that the decline of top consumers have on species interaction networks can amplify the impact of other environmental changes on communities (Shurin et al., 2012). For instance, the loss of a keystone predator, the sea otter Enhydra lutris, from subarctic marine ecosystems has interacted with ocean warming in eroding calcareous reefs: the loss has promoted the growth of herbivore populations, which graze on the algae that build the calcareous reefs, resulting in overgrazing and the acceleration of the negative effects of warming on the reefs (Rasher et al., 2020).
Not all consumers are negatively affected by environmental change. Some benefit and cause in turn declines in consumers. For instance, a mesocosm experiment showed that warming advances zooplankton phenology and that this causes an earlier top-down control of phytoplankton and, hence, a strong reduction in their abundance (Velthuis et al., 2017).
Top consumers that are not affected by environmental change can moderate the effect of environmental change on lower trophic levels. For instance, the freshwater sculpin Cottus nozawae - a dominant predator in temperate stream communities - buffers the negative effects of heatwaves on benthic algae by reducing macroinvertebrate densities (Ross et al., 2022).
Bottom-up effects
Bottom-up effects - mediated by nutrient availability - are important regulators of community composition in ecosystems with low producer redundancy and where consumers are highly specialized in their diet (Hunter and Price, 1992). For instance, a simulation study showed that the extinction of primary producers in ecosystems with low connectance (complexity) can cause more secondary extinctions than the removal of herbivores or top consumers (Eklof and Ebenman, 2006). Similarly, environmental changes that alter the nutrient content of plants can change the growth rates of consumers and their population dynamics, resulting in effects that can propagate upwards in the food web through altered foraging behavior of higher trophic levels (Cross et al., 2015).
Interplay between top-down and bottom-up effects
Top-down effects countervail bottom-up effects and their interaction determines the ultimate impact of behavioral responses on food webs. The interaction is often complex with feedbacks between trophic levels. For instance, climate change that improves the nutrient content of primary producers and promotes the growth of consumers also increases the consumption rate of the consumers, which can feed back to influence primary producers (Rosenblatt and Schmitz, 2016). Such opposing effects of top-down and bottom-up effects can avert larger shifts in community structure, (Lynam et al., 2017). This is especially the case when ecosystems are exposed to several stressors. For instance, the influence of eutrophication on primary production is counteracted by global warming that increases foraging activity of consumers (Kratina et al., 2012).
Top-down and bottom-up effects do not always oppose each other but can also act additively (Shurin et al., 2012). For instance, nutrient enrichment that promotes primary production through a bottom-up effect can be strengthened by global warming that reduces the size of predators and their foraging pressure (Jochum et al., 2012). This can allow the biomass of primary producers to increase even further.
Generally the removal of top consumers has a larger impact on food webs than the removal of herbivores or primary producers, given that top consumers generally are more sensitive to environmental changes than species at lower trophic levels (Duffy, 2003; Voigt et al., 2003; Borrvall and Ebenman, 2006).
Lateral effects
Interactions within a trophic level, i.e., non-trophic, lateral interactions (such as competitive, mutualistic and commensalistic interactions), determine together with trophic interactions (consumptive and parasitic interactions) the structure of species interaction networks (Kefi et al., 2012). Competition, in particular, can drastically alter trophic interactions (Sih et al., 1998; Davenport and Chalcraft, 2013). For instance, the intensity of competition among freshwater insect predators for prey determines their top-down effect on the prey community, such as the composition of zooplankton (Sentis et al., 2017b).
Trophic and non-trophic (lateral) interactions often differ in their sensitivity to environmental change, with trophic interactions generally being more sensitive and, hence, more often having negative effects on communities (Sentis et al., 2017b; Vesely et al., 2019). Non-trophic, lateral interactions are more dependent on species traits, which can stabilize ecological communities by weakening disrupted trophic interactions and dampening population oscillations (Rall et al., 2008).
Phenological asynchronies
Human-induced environmental changes that alter the timing of behaviors, such as migration or reproductive behaviors, can cause mismatches with optimal abiotic and biotic conditions (Kharouba et al., 2018; Renner and Zohner, 2018). For example, birds that breed earlier because of climate change can suffer from food shortage if the advancement differs from that of their prey, which can lead to failed reproduction (Visser et al., 2012). Such mismatches are often caused by species using cues that differ in their sensitivity to environmental conditions to time their activities.
Other common causes of mismatches in the timing of activities are differences in response rate or time lag among species, because of constraints or costs associated with the responses (Both et al., 2009; Chen et al., 2019). In addition, species may differ in their recovery rate, as higher trophic levels are generally slower in responding to environmental changes than lower levels (Thackeray et al., 2016). Phenological mismatches also occur when the environmental change occurs at a time of the year that promotes one trophic level over another (Straile et al., 2015). For, instance, higher temperature during the period when zooplankton populations start to grow increases their population sizes, but results in food limitation later in the season (Wagner and Benndorf, 2007).
Finally, abiotic factors can cause mismatches by limiting the ability of species to respond to environmental change. For example, while grazers increase their grazing pressure in response to rising temperature, primary producers may be unable to respond because of the lack of nutrients, resulting in overgrazing (Thackeray et al., 2016). This overgrazing can in turn feed back to influence the grazers and result in complex changes to the food web.
Individual variation in behavioral responses
Individuals within a population often vary in their behavioral responses to environmental change, depending on traits such as physiology, developmental stage, sex, social status, and personality (Bolnick et al., 2011; Schmitz and Trussell, 2016). This variation influences species interactions and the dynamics of populations. For instance, the impact of a predator on the prey community is smaller if only some of the individuals switch to an alternative prey when their main prey declines than if all individuals, or none of them, altered their prey selection (Toscano and Griffen, 2014; Belgrad and Griffen, 2016; Sommer and Schmitz, 2020).
Similarly, variation among individuals in responses to altered resource availability can influence competitive interactions by alter niche overlap (Violle et al., 2012). Species with more variable responses may broaden their niches more than other species and gain a competitive advantage (Hogle et al., 2022). Likewise, environmental changes that alter the frequency of specific behavioral types, for instance through the emigration of bold individuals, can influence species interactions (Pelletier and Garant, 2012). In addition, changes in the presence of individuals at different developmental stages often influence interactions, given that ontogeny and individual development is a common cause of temporal changes in behavior both within and between species (Persson and de Roos, 2013; Toscano and Rudolf, 2021).
Restructuring of species interaction networks
Variation among species in their behavioral responses to environmental change often restructures species interaction networks. For instance, increased consumption and attack rate by consumers because of global warming augments the biomass of higher trophic levels while reducing that of lower levels (Shurin et al., 2012; Dell et al., 2014). Warming also decreases the size of individuals, which further modifies consumer-resource interactions (Horne et al., 2015; Sentis et al., 2017a). Such changes can reduce the importance of earlier dominant or keystone species in favor of other species species (Tanentzap et al., 2020).
Restructuring also occurs when some species amplify or dampen the impact of environmental change on other species (Urban et al., 2016). For example, the negative impact of warming on the survival of a dominant tadpole species, Hyla versicolor, is dampened by predation by a dragonfly on a competing tadpole species, as this reduces competitive interactions between the two tadpole species (Rudolf and Roman, 2018). Correspondingly, the impact of climate change on vegetation can be amplified by the grazing activity of herbivores (Boulangeat et al., 2018).
The restructuring of species interaction networks can in turn facilitate the invasion of new species (Pysek et al., 2020). Invasion success depends on the behavior of both the invader and the native species, and changes in either because of environmental change can influence the invasion success (Chapple et al., 2012). For instance, aggressive interactions between an invading shrimp, Palaemon elegans, and a native fish, the three-spined stickleback, Gasterosteus aculeatus, reduces the ability of the shrimp to invade habitats occupied by the fish (Jakubaviciute and Candolin, 2021). However, the aggression of the fish depends on its population density, which implies that changes in its density - because of human activities - can influence the invasion success of the shrimp and, hence, its impact on the community (Candolin et al., 2018).
The invasion of new species can also introduce non-native pathogens, which can alter species interaction and affect population sizes markedly. An example is the outbreak of the Squirrelpox disease in the native red squirrel (Sciurus vulgaris) in the United Kingdom with the introduction of the non-native gray squirrels (S. carolinensis), which carries the virus. The outbreak has altered the competitive interaction between the two species, favoring the non-native gray squirrel (Chantrey et al., 2014).
Ecological consequences: Impact of behavioral responses on abiotic components, biodiversity, and ecosystem services
Biogeochemical cycles and abiotic conditions
Changes in behaviors that alter species interactions influence ecological processes, such as the flow of energy and matter through the food web and between habitats and ecosystems. These ecological effects are often mediated by consumers, including detritivores, (Ren et al., 2022). For instance, a change in the foraging behavior and top-down control of consumers influences not only the biomass of lower trophic levels, but also biogeochemical cycles, such as the cycling of nutrients, carbon, and water through the ecosystem.
If altered consumer behavior influences the biomass of primary producers, the rate of assimilation can change and further alter biogeochemical cycles (Bakker et al., 2016; Ren et al., 2022). Similarly, changes in the behavior of consumers can influence respiration rate and the carbon dissimilatory process, through changes in their own metabolism and in the abundance and activity of other species. Changes in consumer behavior can also influence decomposition and abiotic conditions, such as oxygen availability (Ren et al., 2022). For instance, reduced burial activity of polychetes in aquatic sediments (bioturbation) reduces oxygen availability for other organisms in the sediments, which in turn reduces their decomposition activity (Kristensen, 2000). Changes in the activity of terrestrial detritivores can again alter soil formation and its characteristics. For example, the ingestion of microplastics by earthworms and other detritivores increases their mortality rate, which reduces their activity, especially when combined with other contaminants, such as heavy metals (Bhagat et al., 2021).
Changes in the consumption by herbivores of vegetation can in turn alter light conditions, both within and below the vegetation. Similarly, changes in the consumption by zooplankton of phytoplankton can alter water turbidity and light penetration and, hence, assimilation in deeper water. Reduced assimilation can in turn cause hypoxia and alter the benthic community. Likewise, changes in the activity of terrestrial herbivores can change the influx of nutrients to aquatic ecosystems and affect algae blooms and light penetration. For instance, the replacement of large wildlife with livestock on the African savanna has increased the transfer of organic matter and nutrient from terrestrial to aquatic ecosystems, with further consequences for these ecosystems (Iteba et al., 2021). The impact of consumers on vegetation can also alter the climate, given that the biomass and composition of vegetation influences humidity, temperature, and the release of water vapor and cloud formation (Green et al., 2017).
The transfer of energy and matter between habitats and ecosystems is obviously also sensitive to changes in animal movements. Shifts in where animals stay, what they feed on, and where they die, alter biogeochemical processes within habitats and, thus, ecological processes and components, such as primary production and biodiversity (Peller et al., 2022).
Biodiversity and ecosystem stability
Species and their behavioral activities and interactions with other species determine biodiversity and ecological processes, which in turn influence the stability of ecosystems - the ability to resist changes or return to the original state following a disturbance (Loreau and de Mazancourt, 2013; Hautier et al., 2015). In particular, the current decline of top predators and relaxation of their top-down control is likely to destabilize ecosystems, given that predation moderates competition among species, which otherwise can cause large fluctuations in population sizes (Loreau and de Mazancourt, 2013).
Changes in the behavior of dominant and common species can similarly cause cascades of effects that alter ecosystem stability (Gaston, 2010). Common species shape their environments and are involved in a large number of biotic interactions, and behavioral changes in such species are especially likely to cause extinction cascades that reduce the stability of species interaction networks (Dunne et al., 2002).
The behavior of species and their interactions can also buffer the effects of environmental change on communities and improve ecosystem stability. This is especially likely when species show redundancy in their ecological functions, as this gives rise to an ‘insurance effect’ (Yachi and Loreau, 1999). Species rich communities with multiple interactions, both within and between trophic levels, are consequently expected to be most resilient against environmental perturbations (Neutel et al., 2007; Rooney and McCann, 2012; Oliver et al., 2015). Correspondingly, ecosystems with few but highly specialized interactions are more sensitive to environmental disturbances. Thus, the decline of behavioral interactions among species can reduce the ability of ecosystems to resist large-scale changes in the face of human activities.
Ecosystem services
Ecosystems provide a range of services to humans, such as food security, control of pests and parasites, clean air and water, and recreational possibilities (Pecl et al., 2017). These services depend on the behavior of species and changes in these - because of human activities – can consequently have negative ramifications for the services.
Food security, in particular, is highly dependent on the behavior of species. Many plant species, including crops, depend on insect pollinators. Thus, the current decline of pollinators is expected to negatively affect pollination success and crop yield (Wagner et al., 2021). Similarly, the dispersal of seeds depends on animal resource use and movements, and changes in these behaviors can consequently influence the population dynamics of plants (McConkey et al., 2012).
Clear air and water are similarly dependent on the behavior of species. Plants both bind and emit volatile compounds, which implies that changes in the behavior of grazers and detritivores can alter these dynamics (Wang et al., 2018). Correspondingly, changes in the foraging behavior and resource use of consumers can influence their waste products and thereby water, air, and soil quality (Angerer et al., 2021).
Changes in behavior can also influence the dynamics of pathogens and parasites, particularly by altering encounters between individuals and species and, hence, the transmission rate of these organisms, as well as by influencing the physical condition of individuals and their ability to resist infection or recover from it (Budria and Candolin, 2014). Increased transmission or reduced resistance can promote the introduction and rapid spread of pests that destroy resources, such as crops and forest products, and allow the transmission of parasites and pathogens to humans and other animals and cause pandemics (McNeely, 2021).
Last but not least, human health and well-being depends on the recreational value of nature. Behavioral responses to human-induced environmental changes are both a cause and a consequence of the current loss of species and species interactions. Thus, human-induced behavioral responses are further boosting the impact that we humans are having on ecosystems and their use for recreation and well-being (Pecl et al., 2017).
In general, the resilience of ecosystem services to human-induced environmental changes depends on the fragility of the network of species interactions, i.e., the number of species functional traits and links between them (Ross et al., 2021). More diverse communities with many behavioral links between functionally different species are expected to provide the most robust ecosystem services (Ross et al., 2021). Thus, the current loss of biodiversity and behavioral interactions is expected to reduce the provisioning of the goods and services that we humans depend on.
Outlook and future avenues of research
Behavior underlies interactions within and between species and with the abiotic environment. Behavior consequently links biotic and abiotic factors together and determines biodiversity and ecosystem processes. Thus, changes in the behavior of animals can influence ecosystem structure, dynamics, and function. Yet, few studies on the impact of human disturbances on communities and ecosystems consider the behavior of animals. In this review, we have shown that critical information on the mechanisms behind ecosystem changes can be gained by considering the behavior of animals. Information on the causes, mechanisms, and consequences of behavioral responses to environmental disturbances, and how the responses scale up to influence communities and ecosystems, can improve our ability to assess and predict the consequences of human activities for ecosystems, as well as to inform ecosystem management.
Unraveling the links between species and assessing their sensitivity to human activities is, however, challenging. Species are linked by a multitude of direct and indirect interactions, which can be differentially affected by environmental change. Thus, research has to concentrate on some species and their interactions. Top predators, ecosystem engineers, and dominant species are obvious candidates, given the large number of species they interact with, directly and indirectly, and the major impact they have on ecological processes. In this review, we have provided a conceptual framework for understanding the links between behavior and ecosystem processes and the factors that influence these links.
The research challenge is further complicated by the multitude of disturbances animals are exposed to. Future studies need to consider interactions among these and their combined effects. Moreover, given the multitude of pathways through which environmental disturbances influence animals and their interactions, collaboration among researchers is needed, as well as open access to data. Only through open, collaborative research can we gradually gain a deeper understanding of the pathways and mechanisms through which human activities influence ecosystems.
Author contributions
TR and UC conceptualized the manuscript, performed the literature search, and wrote the manuscript. Both authors contributed to the article and approved the submitted version.
Funding
This research was funded by Jenny and Antti Wihuri Foundation (to TR, grant no: 210290) and Swedish Cultural Foundation in Finland (to UC, grant no: 170152).
Conflict of Interest
The authors declare that the research was conducted in the absence of any commercial or financial relationships that could be construed as a potential conflict of interest.
Publisher’s Note
All claims expressed in this article are solely those of the authors and do not necessarily represent those of their affiliated organizations, or those of the publisher, the editors and the reviewers. Any product that may be evaluated in this article, or claim that may be made by its manufacturer, is not guaranteed or endorsed by the publisher.
References
Abrams, P. A. (1995). Implications of dynamically variable traits for identifying, classifying, and measuring direct and indirect effects in ecological communities. Am. Nat. 146, 112–134. doi: 10.1086/285789
Angerer, V., Sabia, E., von Borstel, U. K., and Gauly, M. (2021). Environmental and biodiversity effects of different beef production systems. J. Environ. Manag. 289:112523. doi: 10.1016/j.jenvman.2021.112523
Arnot, J. A., and Gobas, F. (2006). A review of bioconcentration factor (BCF) and bioaccumulation factor (BAF) assessments for organic chemicals in aquatic organisms. Environ. Rev. 14, 257–297. doi: 10.1139/a06-005
Avolio, M. L., Forrestel, E. J., Chang, C. C., La Pierre, K. J., Burghardt, K. T., and Smith, M. D. (2019). Demystifying dominant species. New Phytol. 223, 1106–1126. doi: 10.1111/nph.15789
Bakker, E. S., Wood, K. A., Pages, J. F., Veen, G. F., Christianen, M. J. A., Santamaria, L., et al. (2016). Herbivory on freshwater and marine macrophytes: a review and perspective. Aquat. Bot. 135, 18–36. doi: 10.1016/j.aquabot.2016.04.008
Barber, I. (2007). Parasites, behaviour and welfare in fish. Appl. Anim. Behav. Sci. 104, 251–264. doi: 10.1016/j.applanim.2006.09.005
Bartley, T. J., McCann, K. S., Bieg, C., Cazelles, K., Granados, M., Guzzo, M. M., et al. (2019). Food web rewiring in a changing world. Nat. Ecol. Evol. 3, 345–354.
Belgrad, B. A., and Griffen, B. D. (2016). Predator - prey interactions mediated by prey personality and predator hunting mode. Proc. R. Soc. B Biol. Sci. 283:20160408. doi: 10.1098/rspb.2016.0408
Bell, A. M., and Hellmann, J. K. (2019). “An Integrative Framework for Understanding the Mechanisms and Multigenerational Consequences of Transgenerational Plasticity,” in Annual Review of Ecology. Evolution, and Systematics, ed. D. J. Futuyma (Palo Alto: Annual Reviews), 97–118.
Bell, G. (2017). “Evolutionary Rescue,” in Annual Review of Ecology. Evolution, and Systematics, ed. D. J. Futuyma (Palo Alto: Annual Reviews), 605–627.
Bhagat, J., Nishimura, N., and Shimada, Y. (2021). Toxicological interactions of microplastics/nanoplastics and environmental contaminants: current knowledge and future perspectives. J. Hazard. Mater. 405:123913. doi: 10.1016/j.jhazmat.2020.123913
Blowes, S. A., Supp, S. R., Antao, L. H., Bates, A., Bruelheide, H., Chase, J. M., et al. (2019). The geography of biodiversity change in marine and terrestrial assemblages. Science 366, 339–345. doi: 10.1126/science.aaw1620
Bolnick, D. I., Amarasekare, P., Araujo, M. S., Burger, R., Levine, J. M., Novak, M., et al. (2011). Why intraspecific trait variation matters in community ecology. Trends Ecol. Evol. 26, 183–192. doi: 10.1016/j.tree.2011.01.009
Borrvall, C., and Ebenman, B. (2006). Early onset of secondary extinctions in ecological communities following the loss of top predators. Ecol. Lett. 9, 435–442. doi: 10.1111/j.1461-0248.2006.00893.x
Both, C., van Asch, M., Bijlsma, R. G., van den Burg, A. B., and Visser, M. E. (2009). Climate change and unequal phenological changes across four trophic levels: constraints or adaptations? J. Anim. Ecol. 78, 73–83. doi: 10.1111/j.1365-2656.2008.01458.x
Boukal, D. S., Bideault, A., Carreira, B. M., and Sentis, A. (2019). Species interactions under climate change: connecting kinetic effects of temperature on individuals to community dynamics. Curr. Opin. Insect Sci. 35, 88–95. doi: 10.1016/j.cois.2019.06.014
Boulangeat, I., Svenning, J. C., Daufresne, T., Leblond, M., and Gravel, D. (2018). The transient response of ecosystems to climate change is amplified by trophic interactions. Oikos 127, 1822–1833. doi: 10.1111/oik.05052
Buchanan, K. L., and Partecke, J. (2012). “The endocrine system: can homeostasis be maintained in a changing world?,” in Behavioural responses to a changing world. Mechanisms and consequences, eds U. Candolin and B. B. M. Wong (Oxford: Oxford University Press), 32–45.
Buchholz, R., Banusiewicz, J. D., Burgess, S., Crocker-Buta, S., Eveland, L., and Fuller, L. (2019). Behavioural research priorities for the study of animal response to climate change. Anim. Behav. 150, 127–137. doi: 10.1016/j.anbehav.2019.02.005
Budria, A., and Candolin, U. (2014). How does human-induced environmental change influence host-parasite interactions? Parasitology 141, 462–474. doi: 10.1017/s0031182013001881
Candolin, U. (2019a). Mate choice in a changing world. Biol. Rev. 94, 1246–1260. doi: 10.1111/brv.12501
Candolin, U. (2019b). The threespine stickleback (Gasterosteus aculeatus) as a modifier of ecological disturbances. Evol. Ecol. Res. 20, 167–191.
Candolin, U., Bertell, E., and Kallio, J. (2018). Environmental disturbance alters the ecological impact of an invading shrimp. Funct. Ecol. 32, 1370–1378. doi: 10.1111/1365-2435.13078
Candolin, U., and Jensen, I. (2021). Phenotypic plasticity in courtship exposed to selection in a human-disturbed environment. Evol. Appl. 14, 2392–2401. doi: 10.1111/eva.13225
Candolin, U., and Wong, B. B. M. (2012). Behavioural Responses to a Changing World. Mechanisms and Consequences. Oxford: Oxford University Press.
Candolin, U., and Wong, B. B. M. (2019). Mate choice in a polluted world: consequences for individuals, populations and communities. Philos. Trans. R. Soc. B Biol. Sci. 374:20180055. doi: 10.1098/rstb.2018.0055
Carpenter, S. R., and Kitchell, J. F. (1988). Consumer control of lake productivity. Bioscience 38, 764–769. doi: 10.2307/1310785
Ceballos, G., Ehrlich, P. R., Barnosky, A. D., Garcia, A., Pringle, R. M., and Palmer, T. M. (2015). Accelerated modern human-induced species losses: entering the sixth mass extinction. Sci. Adv. 1:e1400253. doi: 10.1126/sciadv.1400253
Chantrey, J., Dale, T. D., Read, J. M., White, S., Whitfield, F., Jones, D., et al. (2014). European red squirrel population dynamics driven by squirrelpox at a gray squirrel invasion interface. Ecol. Evol. 4, 3788–3799. doi: 10.1002/ece3.1216
Chapple, D. G., Simmonds, S. M., and Wong, B. B. M. (2012). Can behavioral and personality traits influence the success of unintentional species introductions? Trends Ecol. Evol. 27, 57–64. doi: 10.1016/j.tree.2011.09.010
Chen, C., Gols, R., Biere, A., and Harvey, J. A. (2019). Differential effects of climate warming on reproduction and functional responses on insects in the fourth trophic level. Funct. Ecol. 33, 693–702. doi: 10.1111/1365-2435.13277
Cross, W. F., Hood, J. M., Benstead, J. P., Huryn, A. D., and Nelson, D. (2015). Interactions between temperature and nutrients across levels of ecological organization. Glob. Chang. Biol. 21, 1025–1040. doi: 10.1111/gcb.12809
Dangles, O., and Malmqvist, B. (2004). Species richness-decomposition relationships depend on species dominance. Ecol. Lett. 7, 395–402. doi: 10.1111/j.1461-0248.2004.00591.x
Davenport, J. M., and Chalcraft, D. R. (2013). Nonconsumptive effects in a multiple predator system reduce the foraging efficiency of a keystone predator. Ecol. Evol. 3, 3063–3072. doi: 10.1002/ece3.691
de Visser, S., Thebault, E., and de Ruiter, P. C. (2013). “Ecosystem Engineers, Keystone Species,” in Ecological Systems, ed. R. Leemans (New York, NY: Springer Science), 59–68.
Dell, A. I., Pawar, S., and Savage, V. (2014). Temperature dependence of trophic interactions are driven by asymmetry of species responses and foraging strategy. J. Anim. Ecol. 83, 70–84. doi: 10.1111/1365-2656.12081
Diaz Pauli, B., and Sih, A. (2017). Behavioural responses to human-induced change: why fishing should not be ignored. Evol. Appl. 10, 231–240. doi: 10.1111/eva.12456
Donohue, I., Petchey, O. L., Kefi, S., Genin, A., Jackson, A. L., Yang, Q., et al. (2017). Loss of predator species, not intermediate consumers, triggers rapid and dramatic extinction cascades. Glob. Chang. Biol. 23, 2962–2972. doi: 10.1111/gcb.13703
Duffy, J. E. (2003). Biodiversity loss, trophic skew and ecosystem functioning. Ecol. Lett. 6, 680–687. doi: 10.1046/j.1461-0248.2003.00494.x
Dunne, J. A., Williams, R. J., and Martinez, N. D. (2002). Network structure and biodiversity loss in food webs: robustness increases with connectance. Ecol. Lett. 5, 558–567. doi: 10.1046/j.1461-0248.2002.00354.x
Eklof, A., and Ebenman, B. (2006). Species loss and secondary extinctions in simple and complex model communities. J. Anim. Ecol. 75, 239–246. doi: 10.1111/j.1365-2656.2006.01041.x
Ellison, A. M. (2019). Foundation Species, Non-trophic Interactions, and the Value of Being Common. Iscience 13, 254–268. doi: 10.1016/j.isci.2019.02.020
Estes, J. A., Terborgh, J., Brashares, J. S., Power, M. E., Berger, J., Bond, W. J., et al. (2011). Trophic downgrading of planet earth. Science 333, 301–306. doi: 10.1126/science.1205106
Gall, J. E., Boyd, R. S., and Rajakaruna, N. (2015). Transfer of heavy metals through terrestrial food webs: a review. Environ. Monit. Assess. 187:201. doi: 10.1007/s10661-015-4436-3
Gilman, S. E., Urban, M. C., Tewksbury, J., Gilchrist, G. W., and Holt, R. D. (2010). A framework for community interactions under climate change. Trends Ecol. Evol. 25, 325–331. doi: 10.1016/j.tree.2010.03.002
Green, J. K., Konings, A. G., Alemohammad, S. H., Berry, J., Entekhabi, D., Kolassa, J., et al. (2017). Regionally strong feedbacks between the atmosphere and terrestrial biosphere. Nat. Geosci. 10, 410–414. doi: 10.1038/ngeo2957
Hautier, Y., Tilman, D., Isbell, F., Seabloom, E. W., Borer, E. T., and Reich, P. B. (2015). Anthropogenic environmental changes affect ecosystem stability via biodiversity. Science 348, 336–340. doi: 10.1126/science.aaa1788
Heath, M. R., Speirs, D. C., and Steele, J. H. (2014). Understanding patterns and processes in models of trophic cascades. Ecol. Lett. 17, 101–114. doi: 10.1111/ele.12200
Hoffmann, A. A., and Sgro, C. M. (2011). Climate change and evolutionary adaptation. Nature 470, 479–485. doi: 10.1038/nature09670
Hogle, S. L., Hepolehto, I., Ruokolainen, L., Cairns, J., Hiltunen, T., and Chase, J. (2022). Effects of phenotypic variation on consumer coexistence and prey community structure. Ecol. Lett. 25, 307–319. doi: 10.1111/ele.13924
Hoover, S. E. R., and Tylianakis, J. M. (2012). “Species interactions,” in Behavioural Responses to a Changing World: Mechanisms and Consequences, eds U. Candolin and B. B. M. Wong (Oxford: Oxford University Press), 129–142.
Horne, C. R., Hirst, A. G., and Atkinson, D. (2015). Temperature-size responses match latitudinal-size clines in arthropods, revealing critical differences between aquatic and terrestrial species. Ecol. Lett. 18, 327–335. doi: 10.1111/ele.12413
Hunter, M. D., and Price, P. W. (1992). Playing chutes and latters - heterogeneity and the relative roles of bottom-up and top-down forces in natural communities. Ecology 73, 724–732.
Iteba, J. O., Hein, T., Singer, G. A., and Masese, F. O. (2021). Livestock as vectors of organic matter and nutrient loading in aquatic ecosystems in African savannas. PLoS One 16:e0257076. doi: 10.1371/journal.pone.0257076
Ives, A. R., and Carpenter, S. R. (2007). Stability and diversity of ecosystems. Science 317, 58–62. doi: 10.1126/science.1133258
Jackson, M. C., Pawar, S., and Woodward, G. (2021). The Temporal Dynamics of Multiple Stressor Effects: From Individuals to Ecosystems. Trends Ecol. Evol. 36, 402–410. doi: 10.1016/j.tree.2021.01.005
Jakubaviciute, E., and Candolin, U. (2021). Density-dependent behavioural interactions influence coexistence between a native and a non-native mesopredator. Biol. Invasions 23, 3427–3434. doi: 10.1007/s10530-021-02585-6
Jochum, M., Schneider, F. D., Crowe, T. P., Brose, U., and O’Gorman, E. J. (2012). Climate-induced changes in bottom-up and top-down processes independently alter a marine ecosystem. Philos. Trans. R. Soc. B Biol. Sci. 367, 2962–2970. doi: 10.1098/rstb.2012.0237
Jones, C. G., Lawton, J. H., and Shachak, M. (1994). Organisms as ecosystem engineers. Oikos 69, 373–386. doi: 10.2307/3545850
Kefi, S., Berlow, E. L., Wieters, E. A., Navarrete, S. A., Petchey, O. L., Wood, S. A., et al. (2012). More than a meal. integrating non-feeding interactions into food webs. Ecol. Lett. 15, 291–300. doi: 10.1111/j.1461-0248.2011.01732.x
Kharouba, H. M., Ehrlen, J., Gelman, A., Bolmgren, K., Allen, J. M., Travers, S. E., et al. (2018). Global shifts in the phenological synchrony of species interactions over recent decades. Proc. Natl. Acad. Sci. U.S.A. 115, 5211–5216. doi: 10.1073/pnas.1714511115
Kratina, P., Greig, H. S., Thompson, P. L., Carvalho-Pereira, T. S. A., and Shurin, J. B. (2012). Warming modifies trophic cascades and eutrophication in experimental freshwater communities. Ecology 93, 1421–1430. doi: 10.1890/11-1595.1
Kristensen, E. (2000). Organic matter diagenesis at the oxic/anoxic interface in coastal marine sediments, with emphasis on the role of burrowing animals. Hydrobiologia 426, 1–24. doi: 10.1023/a:1003980226194
Leroux, S. J., and Loreau, M. (2010). Consumer-mediated recycling and cascading trophic interactions. Ecology 91, 2162–2171. doi: 10.1890/09-0133.1
Lima, S. L., and Dill, L. M. (1990). Behavioral decisions made under the risk of predation: a review and prospectus. Can. J. Zool. 68, 619–640.
Loreau, M., and de Mazancourt, C. (2013). Biodiversity and ecosystem stability: a synthesis of underlying mechanisms. Ecol. Lett. 16, 106–115. doi: 10.1111/ele.12073
Lynam, C. P., Llope, M., Mollmann, C., Helaoutet, P., Bayliss-Brown, G. A., and Stenseth, N. C. (2017). Interaction between top-down and bottom-up control in marine food webs. Proc. Natl. Acad. Sci. U.S.A. 114, 1952–1957. doi: 10.1073/pnas.1621037114
McCallum, E. S., Dey, C. J., Cerveny, D., Bose, A. P. H., and Brodin, T. (2021). Social status modulates the behavioral and physiological consequences of a chemical pollutant in animal groups. Ecol. Appl. 31:e02454. doi: 10.1002/eap.2454
McConkey, K. R., Prasad, S., Corlett, R. T., Campos-Arceiz, A., Brodie, J. F., Rogers, H., et al. (2012). Seed dispersal in changing landscapes. Biol. Conserv. 146, 1–13. doi: 10.1016/j.biocon.2011.09.018
McNeely, J. A. (2021). Nature and COVID-19: the pandemic, the environment, and the way ahead. Ambio 50, 767–781. doi: 10.1007/s13280-020-01447-0
Michel, M. J., and Adams, M. M. (2009). Differential effects of structural complexity on predator foraging behavior. Behav. Ecol. 20, 313–317. doi: 10.1093/beheco/arp005
Neutel, A. M., Heesterbeek, J. A. P., van de Koppel, J., Hoenderboom, G., Vos, A., Kaldeway, C., et al. (2007). Reconciling complexity with stability in naturally assembling food webs. Nature 449, 599–511. doi: 10.1038/nature06154
Ockendon, N., Baker, D. J., Carr, J. A., White, E. C., Almond, R. E. A., Amano, T., et al. (2014). Mechanisms underpinning climatic impacts on natural populations: altered species interactions are more important than direct effects. Glob. Chang. Biol. 20, 2221–2229. doi: 10.1111/gcb.12559
Oliver, T. H., Heard, M. S., Isaac, N. J. B., Roy, D. B., Procter, D., Eigenbrod, F., et al. (2015). Biodiversity and Resilience of Ecosystem Functions. Trends Ecol. Evol. 30, 673–684. doi: 10.1016/j.tree.2015.08.009
Paine, R. T. (1966). Food web complexity and species diversity. Am. Nat. 100, 65–75. doi: 10.1086/282400
Palkovacs, E. P., and Dalton, C. M. (2012). “Ecosystem consequences of behavioural palsticity and contemporary evolution,” in Behavioural Responses to a Changing World. Mechansims and Consequences, eds U. Candolin and B. B. M. Wong (Oxford: Oxford University Press), 175–189.
Palkovacs, E. P., Moritsch, M. M., Contolini, G. M., and Pelletier, F. (2018). Ecology of harvest-driven trait changes and implications for ecosystem management. Front. Ecol. Environ. 16, 20–28. doi: 10.1002/fee.1743
Pecl, G. T., Araujo, M. B., Bell, J. D., Blanchard, J., Bonebrake, T. C., Chen, I. C., et al. (2017). Biodiversity redistribution under climate change: impacts on ecosystems and human well-being. Science 355:eaai9214. doi: 10.1126/science.aai9214
Peller, T., Marleau, J. N., and Guichard, F. (2022). Traits affecting nutrient recycling by mobile consumers can explain coexistence and spatially heterogeneous trophic regulation across a meta-ecosystem. Ecol. Lett. 25, 440–452. doi: 10.1111/ele.13941
Pelletier, F., and Garant, D. (2012). “Population consequences of individual variation in behaviour,” in Behavioural Responses to a Changing world. Mechansims and Consequences, eds U. Candolin and B. B. M. Wong (Oxford: Oxford University Press), 159–174.
Persson, L., and de Roos, A. M. (2013). Symmetry breaking in ecological systems through different energy efficiencies of juveniles and adults. Ecology 94, 1487–1498. doi: 10.1890/12-1883.1
Power, M. E., Tilman, D., Estes, J. A., Menge, B. A., Bond, W. J., Mills, L. S., et al. (1996). Challenges in the quest for keystones. Bioscience 46, 609–620. doi: 10.2307/1312990
Previsic, A., Vilenica, M., Vuckovic, N., Petrovic, M., and Rozman, M. (2021). Aquatic Insects Transfer Pharmaceuticals and Endocrine Disruptors from Aquatic to Terrestrial Ecosystems. Environ. Sci. Technol. 55, 3736–3746. doi: 10.1021/acs.est.0c07609
Puckowski, A., Mioduszewska, K., Lukaszewicz, P., Borecka, M., Caban, M., Maszkowska, J., et al. (2016). Bioaccumulation and analytics of pharmaceutical residues in the environment: a review. J. Pharm. Biomed. Anal. 127, 232–255. doi: 10.1016/j.jpba.2016.02.049
Pysek, P., Hulme, P. E., Simberloff, D., Bacher, S., Blackburn, T. M., Carlton, J. T., et al. (2020). Scientists’ warning on invasive alien species. Biol. Rev. 95, 1511–1534. doi: 10.1111/brv.12627
Rall, B. C., Brose, U., Hartvig, M., Kalinkat, G., Schwarzmuller, F., Vucic-Pestic, O., et al. (2012). Universal temperature and body-mass scaling of feeding rates. Philos. Trans. R. Soc. B-Biol. Sci. 367, 2923–2934. doi: 10.1098/rstb.2012.0242
Rall, B. C., Guill, C., and Brose, U. (2008). Food-web connectance and predator interference dampen the paradox of enrichment. Oikos 117, 202–213. doi: 10.1111/j.2007.0030-1299.15491.x
Rasher, D. B., Steneck, R. S., Halfar, J., Kroeker, K. J., Ries, J. B., Tinker, M. T., et al. (2020). Keystone predators govern the pathway and pace of climate impacts in a subarctic marine ecosystem. Science 369, 1351–1354. doi: 10.1126/science.aav7515
Ren, L. J., Jensen, K., Porada, P., and Mueller, P. (2022). Biota-mediated carbon cycling-A synthesis of biotic-interaction controls on blue carbon. Ecol. Lett. 25, 521–540. doi: 10.1111/ele.13940
Renner, S. S., and Zohner, C. M. (2018). “Climate Change and Phenological Mismatch in Trophic Interactions Among Plants, Insects, and Vertebrates,” in Annual Review of Ecology, Evolution, and Systematics, ed. D. J. Futuyma (Palo Alto: Annual Reviews), 165–182.
Ricciardi, A., Hoopes, M. F., Marchetti, M. P., and Lockwood, J. L. (2013). Progress toward understanding the ecological impacts of nonnative species. Ecol. Monogr. 83, 263–282. doi: 10.1890/13-0183.1
Ripple, W. J., Estes, J. A., Beschta, R. L., Wilmers, C. C., Ritchie, E. G., Hebblewhite, M., et al. (2014). Status and Ecological Effects of the World’s Largest Carnivores. Science 343:1241484. doi: 10.1126/science.1241484
Rooney, N., and McCann, K. S. (2012). Integrating food web diversity, structure and stability. Trends Ecol. Evol. 27, 40–46. doi: 10.1016/j.tree.2011.09.001
Rosenblatt, A. E., and Schmitz, O. J. (2016). Climate Change, Nutrition, and Bottom-Up and Top-Down Food Web Processes. Trends Ecol. Evol. 31, 965–975. doi: 10.1016/j.tree.2016.09.009
Ross, S., Arnoldi, J. F., Loreau, M., White, C. D., Stout, J. C., Jackson, A. L., et al. (2021). Universal scaling of robustness of ecosystem services to species loss. Nat. Commun. 12:5167. doi: 10.1038/s41467-021-25507-5
Ross, S., Molinos, J. G., Okuda, A., Johnstone, J., Atsumi, K., Futamura, R., et al. (2022). Predators mitigate the destabilising effects of heatwaves on multitrophic stream communities. Glob. Chang. Biol. 28, 403–416. doi: 10.1111/gcb.15956
Rudolf, V. H. W., and Roman, A. (2018). Trophic structure alters consequences of environmental warming. Oikos 127, 1646–1656. doi: 10.1111/oik.05535
Sbragaglia, V., Lopez-Olmeda, J. F., Frigato, E., Bertolucci, C., and Arlinghaus, R. (2021b). Size-selective mortality induces evolutionary changes in group risk-taking behaviour and the circadian system in a fish. J. Anim. Ecol. 90, 387–403. doi: 10.1111/1365-2656.13372
Sbragaglia, V., Jolles, J. W., Coll, M., and Arlinghaus, R. (2021a). Fisheries-induced changes of shoaling behaviour: mechanisms and potential consequences. Trends Ecol. Evol. 36, 885–888. doi: 10.1016/j.tree.2021.06.015
Schmitz, O. J., and Trussell, G. C. (2016). Multiple stressors, state-dependence and predation risk - foraging trade-offs: toward a modern concept of trait-mediated indirect effects in communities and ecosystems. Curr. Opin. Behav. Sci. 12, 6–11. doi: 10.1016/j.cobeha.2016.08.003
Sentis, A., Gemard, C., Jaugeon, B., and Boukal, D. S. (2017b). Predator diversity and environmental change modify the strengths of trophic and nontrophic interactions. Glob. Chang. Biol. 23, 2629–2640. doi: 10.1111/gcb.13560
Sentis, A., Binzer, A., and Boukal, D. S. (2017a). Temperature-size responses alter food chain persistence across environmental gradients. Ecol. Lett. 20, 852–862. doi: 10.1111/ele.12779
Shannon, G., McKenna, M. F., Angeloni, L. M., Crooks, K. R., Fristrup, K. M., Brown, E., et al. (2016). A synthesis of two decades of research documenting the effects of noise on wildlife. Biol. Rev. 91, 982–1005. doi: 10.1111/brv.12207
Shurin, J. B., Clasen, J. L., Greig, H. S., Kratina, P., and Thompson, P. L. (2012). Warming shifts top-down and bottom-up control of pond food web structure and function. Philos. Trans. R. Soc. B Biol. Sci. 367, 3008–3017. doi: 10.1098/rstb.2012.0243
Sih, A. (2013). Understanding variation in behavioural responses to human-induced rapid environmental change: a conceptual overview. Anim. Behav. 85, 1077–1088. doi: 10.1016/j.anbehav.2013.02.017
Sih, A., Bolnick, D. I., Luttbeg, B., Orrock, J. L., Peacor, S. D., Pintor, L. M., et al. (2010). Predator-prey naivete, antipredator behavior, and the ecology of predator invasions. Oikos 119, 610–621. doi: 10.1111/j.1600-0706.2009.18039.x
Sih, A., Englund, G., and Wooster, D. (1998). Emergent impacts of multiple predators on prey. Trends Ecol. Evol. 13, 350–355. doi: 10.1016/s0169-5347(98)01437-2
Simberloff, D., Martin, J. L., Genovesi, P., Maris, V., Wardle, D. A., Aronson, J., et al. (2013). Impacts of biological invasions: what’s what and the way forward. Trends Ecol. Evol. 28, 58–66. doi: 10.1016/j.tree.2012.07.013
Solan, M., Cardinale, B. J., Downing, A. L., Engelhardt, K. A. M., Ruesink, J. L., and Srivastava, D. S. (2004). Extinction and ecosystem function in the marine benthos. Science 306, 1177–1180. doi: 10.1126/science.1103960
Sommer, N. R., and Schmitz, O. J. (2020). Differences in prey personality mediate trophic cascades. Ecol. Evol. 10, 9538–9551. doi: 10.1002/ece3.6648
Straile, D., Kerimoglu, O., and Peeters, F. (2015). Trophic mismatch requires seasonal heterogeneity of warming. Ecology 96, 2794–2805. doi: 10.1890/14-0839.1.sm
Swaddle, J. P., Francis, C. D., Barber, J. R., Cooper, C. B., Kyba, C. C. M., Dominoni, D. M., et al. (2015). A framework to assess evolutionary responses to anthropogenic light and sound. Trends Ecol. Evol. 30, 550–560. doi: 10.1016/j.tree.2015.06.009
Tanentzap, A. J., Morabito, G., Volta, P., Rogora, M., Yan, N. D., and Manca, M. (2020). Climate warming restructures an aquatic food web over 28 years. Glob. Chang. Biol. 26, 6852–6866. doi: 10.1111/gcb.15347
Taylor, B. W., Flecker, A. S., and Hall, R. O. (2006). Loss of a harvested fish species disrupts carbon flow in a diverse tropical river. Science 313, 833–836. doi: 10.1126/science.1128223
Thackeray, S. J., Henrys, P. A., Hemming, D., Bell, J. R., Botham, M. S., Burthe, S., et al. (2016). Phenological sensitivity to climate across taxa and trophic levels. Nature 535, 241–294. doi: 10.1038/nature18608
Toscano, B. J., and Griffen, B. D. (2014). Trait-mediated functional responses: predator behavioural type mediates prey consumption. J. Anim. Ecol. 83, 1469–1477. doi: 10.1111/1365-2656.12236
Toscano, B. J., and Rudolf, V. H. W. (2021). Developmental Change in Predators Drives Different Community Configurations. Am. Nat. 197, 719–731. doi: 10.1086/714049
Tuomainen, U., and Candolin, U. (2011). Behavioural responses to human-induced environmental change. Biol. Rev. 86, 640–657. doi: 10.1111/j.1469-185X.2010.00164.x
Tylianakis, J. M., Didham, R. K., Bascompte, J., and Wardle, D. A. (2008). Global change and species interactions in terrestrial ecosystems. Ecol. Lett. 11, 1351–1363. doi: 10.1111/j.1461-0248.2008.01250.x
Urban, M. C., Bocedi, G., Hendry, A. P., Mihoub, J. B., Pe’er, G., Singer, A., et al. (2016). Improving the forecast for biodiversity under climate change. Science 353:aad8466. doi: 10.1126/science.aad8466
Urban, M. C., Zarnetske, P. L., and Skelly, D. K. (2017). Searching for Biotic Multipliers of Climate Change. Integr. Comp. Biol. 57, 134–147. doi: 10.1093/icb/icx045
Velthuis, M., Domis, L. N. D., Frenken, T., Stephan, S., Kazanjian, G., Aben, R., et al. (2017). Warming advances top-down control and reduces producer biomass in a freshwater plankton community. Ecosphere 8:16. doi: 10.1002/ecs2.1651
Vesely, L., Boukal, D. S., Buric, M., Kuklina, I., Fort, M., Yazicioglu, B., et al. (2019). Temperature and prey density jointly influence trophic and non-trophic interactions in multiple predator communities. Freshw. Biol. 64, 1984–1993. doi: 10.1111/fwb.13387
Violle, C., Enquist, B. J., McGill, B. J., Jiang, L., Albert, C. H., Hulshof, C., et al. (2012). The return of the variance: intraspecific variability in community ecology. Trends Ecol. Evol. 27, 244–252. doi: 10.1016/j.tree.2011.11.014
Visser, M. E., te Marvelde, L., and Lof, M. E. (2012). Adaptive phenological mismatches of birds and their food in a warming world. J. Ornithol. 153, S75–S84. doi: 10.1007/s10336-011-0770-6
Voigt, W., Perner, J., Davis, A. J., Eggers, T., Schumacher, J., Bahrmann, R., et al. (2003). Trophic levels are differentially sensitive to climate. Ecology 84, 2444–2453. doi: 10.1890/02-0266
Wagner, A., and Benndorf, J. (2007). Climate-driven warming during spring destabilises a Daphnia population: a mechanistic food web approach. Oecologia 151, 351–364. doi: 10.1007/s00442-006-0554-5
Wagner, D. L., Grames, E. M., Forister, M. L., Berenbaum, M. R., and Stopak, D. (2021). Insect decline in the Anthropocene: death by a thousand cuts. Proc. Natl. Acad. Sci. U.S.A. 118:e2023989118. doi: 10.1073/pnas.2023989118
Wang, B., Shuman, J., Shugart, H. H., and Lerdau, M. T. (2018). Biodiversity matters in feedbacks between climate change and air quality: a study using an individual-based model. Ecol. Appl. 28, 1223–1231. doi: 10.1002/eap.1721
Werner, E. E., and Peacor, S. D. (2003). A review of trait-mediated indirect interactions in ecological communities. Ecology 84, 1083–1100.
Willems, J. S., Phillips, J. N., and Francis, C. D. (2022). Artificial light at night and anthropogenic noise alter the foraging activity and structure of vertebrate communities. Sci. Total Environ. 805:150223. doi: 10.1016/j.scitotenv.2021.150223
Wilmers, C. C., Post, E., Peterson, R. O., and Vucetich, J. A. (2006). Predator disease out-break modulates top-down, bottom-up and climatic effects on herbivore population dynamics. Ecol. Lett. 9, 383–389. doi: 10.1111/j.1461-0248.2006.00890.x
Wingfield, J. C. (2013). The comparative biology of environmental stress: behavioural endocrinology and variation in ability to cope with novel, changing environments. Anim. Behav. 85, 1127–1133. doi: 10.1016/j.anbehav.2013.02.018
Wong, B. B. M., and Candolin, U. (2015). Behavioral responses to changing environments. Behav. Ecol. 26, 665–673. doi: 10.1093/beheco/aru183
Wright, J. P., and Jones, C. G. (2006). The concept of organisms as ecosystem engineers ten years on: progress, limitations, and challenges. Bioscience 56, 203–209.
Yachi, S., and Loreau, M. (1999). Biodiversity and ecosystem productivity in a fluctuating environment: the insurance hypothesis. Proc. Natl. Acad. Sci. U.S.A. 96, 1463–1468. doi: 10.1073/pnas.96.4.1463
Zala, S. M., and Penn, D. J. (2004). Abnormal behaviours induced by chemical pollution: a review of the evidence and new challenges. Anim. Behav. 68, 649–664. doi: 10.1016/j.anbehav.2004.01.005
Keywords: anthropogenic disturbances, climate change, environmental change, food webs, keystone species, phenology, species interactions, trophic interactions
Citation: Rahman T and Candolin U (2022) Linking animal behavior to ecosystem change in disturbed environments. Front. Ecol. Evol. 10:893453. doi: 10.3389/fevo.2022.893453
Received: 10 March 2022; Accepted: 27 June 2022;
Published: 14 July 2022.
Edited by:
Neville Pillay, University of the Witwatersrand, South AfricaReviewed by:
Piotr Tryjanowski, Poznań University of Life Sciences, PolandBryony Tolhurst, University of Brighton, United Kingdom
Copyright © 2022 Rahman and Candolin. This is an open-access article distributed under the terms of the Creative Commons Attribution License (CC BY). The use, distribution or reproduction in other forums is permitted, provided the original author(s) and the copyright owner(s) are credited and that the original publication in this journal is cited, in accordance with accepted academic practice. No use, distribution or reproduction is permitted which does not comply with these terms.
*Correspondence: Ulrika Candolin, ulrika.candolin@helsinki.fi; https://orcid.org/0000-0001-8736-7793