- Institute of Earth Sciences, University of Applied Sciences and Arts of Southern Switzerland, Mendrisio, Switzerland
Cross-boundary prey subsidies can propagate the effects of human impacts from streams to terrestrial ecosystems, but effects during winter are poorly known. Here I focused on this season and investigated the effects of pollution due to a sewage treatment plant (STP) on aquatic insect emergence and wintering insectivorous birds in a Swiss stream. At sites downstream of the STP, a combination of nutrient (phosphorus), organic (biochemical oxygen demand), and thermal pollution led to higher aquatic emergence compared to upstream sites (6× higher). In turn, the higher emergence led to a strong aggregational response by wintering insectivorous birds (8× higher linear densities compared to upstream sites). Polluted sites also had a different bird assemblage, which included rare wintering species that forage largely on aerial insects. A comparison between the polluted (downstream) sites and a nearby unpolluted stream produced similar differences. The magnitude and consistency of the effects illustrate how strongly stream alterations can propagate to birds through changes in aquatic emergence. Moreover, the results provide insights into the responses of linked stream-terrestrial food webs to other environmental issues that cause warming and/or pollution, including urbanization and climate change.
Introduction
Stream and terrestrial food webs are so interlinked that impacts on one propagate to the other (Nakano and Murakami, 2001). The reciprocal flux of invertebrate prey plays a key role. While stream fishes obtain up to half of their annual dietary needs by feeding on terrestrial insects, terrestrial consumers like bats, birds, and spiders feed extensively on emerging aquatic insects (Baxter et al., 2005; Xiang et al., 2016). Consequently, changes in the flux of emerging aquatic insects, either natural or human-caused, can alter the abundance, distribution, and behavior of terrestrial consumers. For example, reduced aquatic emergence due to river regulation, pollution, or climate warming can decrease the abundance of insectivorous birds during the nesting period (Jonsson et al., 2012; Manning and Sullivan, 2021; Shipley et al., 2022). Here I explored the lesser-known impacts of stream pollution on wintering insectivorous birds, who may be especially sensitive to stream alterations because they rely strongly on aquatic subsidies.
In temperate climates, winter availability of terrestrial insects is severely limited (Nakano and Murakami, 2001), which is probably why most insectivorous birds migrate elsewhere (Lack, 1968). The few wintering species struggle to meet dietary needs and often experience high mortality (Newton, 1998). During winter, ice-free temperate streams remain more productive than surrounding terrestrial landscapes and yield small, but critical fluxes of emerging insects (Jackson and Fisher, 1986; Nakano and Murakami, 2001). At these times, insectivorous birds can be highly dependent on these subsidies (Nakano and Murakami, 2001). Presumably as a result, in winter, insectivorous birds often aggregate around ice-free lakes, rivers, and sewage treatment plants (STPs) (Fuller and Glue, 1978; Maumary et al., 2007).
In temperate streams, winter emergence largely consists of chironomid midges (Diptera: Chironomidae), which are among the few aquatic insects that develop into winged adults throughout the year (Anderson and Ferrington, 2013). Chironomids are tolerant of pollution and occur in high numbers in lakes and streams polluted with organic matter (Losos, 1984), e.g., due to STPs (Provost, 1958). Therefore, in winter, polluted streams might be particularly important sources of aquatic emergence and could potentially elicit aggregative responses (immigration of predators into areas with higher prey abundance) by wintering birds.
Aquatic emergence may also influence the functional makeup of birds. Birds belong to different feeding guilds (Jonsson et al., 2012) and display specific foraging methods (Murakami and Nakano, 2000) that influence their response to aquatic emergence. For example, Jonsson et al. (2012) suggested that a guild of “small-insect foragers” (feeding on adult black flies) responded more strongly to variation in aquatic-insect emergence (largely black flies) than a guild of “large-invertebrate and/or seed foragers,” a group that does not feed on black flies but may instead view them as a nuisance. Iwata et al. (2003) showed that meandering streams increased production of aquatic emerging insects and abundance of insectivorous birds per unit area, but “flycatchers” and “gleaners” responded more strongly than “bark probers.” In winter, aquatic emergence could especially favor species feeding on aerial insects, a resource nearly unavailable in this season away from streams or lakes.
I was inspired to start this study when I observed unusual numbers of scarce-to-irregular wintering insectivorous birds (e.g., Common chiffchaff, Phylloscopus collybita, and Eurasian crag martin, Ptyonoprogne rupestris) in a section of a temperate stream (a tributary of Lake Lugano, Switzerland) that receives the effluent of a STP. In addition, the foraging methods used by these birds (several were catching insects in the air) was unusual for the region, because aerial insects are rare in winter. I hypothesized that STP pollution favors winter-emerging midges and creates a resource haven for otherwise rare wintering insectivorous birds (especially the ones that forage on aerial insects). I examined this hypothesis by comparing benthic insect larvae, aquatic insect emergence, wintering insectivorous birds, and bird foraging methods between sites upstream and downstream of the effluent, and between the impacted stream and a nearby unpolluted (reference) stream.
Methods
Study sites
The study was conducted on the Vedeggio (impacted by STP effluent) and Magliasina (an unpolluted reference) streams (Supplementary Table 1 and Supplementary Figure 1). Both streams are tributaries to Lake Lugano, Switzerland (45° 59′0′′ N, 8°58′0′′ E, 271 m above sea level). The Vedeggio receives the effluent of a large STP (serving more than 120,000 people) 1.4 km upstream of its mouth. On this stream, I set up three study sites (i.e., longitudinal sections measuring 0.5–1.0 km, Supplementary Table 1) downstream of the effluent (VE-DS5–7) and four sites upstream of the effluent (VE-US1–4). The Vedeggio is a uniform, channelized stream with narrow strips (~10 m wide) of riparian vegetation on both banks (Supplementary Table 1 and Supplementary Figure 2). All sites belong to the same longitudinal river zone (rhithron) and share similar in-stream and riparian characteristics, except that VED-DS sites are approximately 2× wider than VED-US and MAG sites due to the additional inflow from the STP (Supplementary Table 1).
The Magliasina does not receive STP effluents or other sources of pollution. On this stream, I set up a study site near the mouth (0.3 km, MA-DS) and another site 2.5 km upstream of the mouth (MA-US). These sites lie at comparable distances from the lake to VE-DS and VE-US sites, respectively. The choice of single reference upstream and downstream sites (instead of multiple sites as in the Vedeggio) was forced by land-use and land-access constraints. At the study sites, the Magliasina is bordered by woodland, except for the upper half of the site MA-DS, which is channelized and bordered by narrow riparian strips.
Data collection
Water-quality data and water temperature data were available thanks to a watershed monitoring program.1 I used data from a station (MAm) located on the Magliasina between MA-US and MA-DS to represent both MA sites (Supplementary Table 1). I also used data from two stations located on the Vedeggio, one just upstream (VED-USm) and one downstream (VED-DSm) of the STP effluent, to represent VED-US and VED-DS sites, respectively. I reported data on water temperature, total phosphorus concentration (PTOT, an index of nutrient enrichment), and Biochemical Oxygen Demand (BOD5, an index of organic pollution) measured monthly between December 2021 and February 2022. To complement monitoring data, I measured air temperature at all sites on one day (13 March 2022), under weather conditions similar to those encountered during sampling. Temperature (in °C) was measured in the shade at chest height using an electronic thermometer. At one site (VE-US4), I additionally measured temperature in seven haphazardly-selected spots to estimate within-site variability.
I conducted the study in January and February before the start of bird spring migration, which usually starts in March. To strengthen the data, surveys of birds, aquatic emergence, and benthic larvae were conducted twice at each site, on different days and at different hours of the day. The results were averaged to produce a single value for each combination of variable and site.
I conducted bird surveys by walking on the banks along the study sites and noting all the birds I could identify based on sight (aided by 8× binoculars) or sound. I included birds in riparian areas, in the channel, or flying overhead. Results were expressed in number of birds per unit stream length, i.e., linear density (ind 100 m–1). I focused on a guild of birds likely to consume winter aquatic emergence (Table 1). The guild included nine species of primarily insectivorous birds present at the study sites [European crag martin, Chiffchaff, Long-tailed tit (Aegithalos caudatus), Short-toed treecreeper (Certhia brachydactyla), Winter wren (Troglodytes troglodytes), European robin (Erithacus rubecula), Black redstart (Phoenicurus ochruros), White wagtail (Motacilla alba), and Gray wagtail (Motacilla cinerea)]. I observed all these birds (except for Short-toed treecreepers, which were rare) feeding on emerging aquatic insects during the study (I identified aquatic prey when birds were picking insects from the stream’s surface or rising from it; in other cases, I concluded that the prey consisted of aquatic insects because inspections of foraging habitats revealed only chironomids or mayflies).
Data on the winter status of the birds in this guild (irregular, rare, common) were obtained from a published database and an atlas of wintering birds (Table 1). Data on foraging methods were obtained by observing the birds feeding during two afternoons at VED-DS sites and assigning the methods observed to four broad categories (sallying, hawking, ground gleaning, and tree gleaning; definitions in Supplementary Table 2).
I sampled emerging aquatic insects using a dip net (width: 21 cm, mesh size. 0.3 mm) to skim the surface of the water. Sampling time was standardized at 3 min per sample. Compared to the more common method based on the use of emergence nets, this method was not designed to quantify emergence fluxes over long time scales (e.g., weeks), but to provide an unbiased index of emergence for between-site comparisons. The reproducibility of the method was supported by the two replicate surveys, which produced similar results even though the order of visit of the sites was reshuffled; results not shown). Emergence samples were collected in similar “run” habitats (i.e., in relatively fast, but not turbulent water) across sites. Only two taxa emerged during the study period and were reported in this study: chironomids and the mayfly Baëtis rhodani. Results were expressed as individuals per unit stream width per minute (ind m–1 min–1).
I sampled larval insects by agitating sediments by foot in a small area (0.08 m2) in front of a hand net (width: 28 cm, mesh size: 0.2 mm) held immediately downstream. Samples were collected from a representative run within each site. Samples were preserved in ethanol and taken to the laboratory, where chironomid larvae and Baëtis rhodani nymphs were sorted, identified, and counted under a dissecting microscope. Results were expressed as individuals per unit area (ind m–2).
Data analysis
I compared water quality, water temperature, and air temperature descriptively based on the magnitude of between-site differences. I compared bird densities, benthic larval densities, and numbers of emerging insects using ANOVA followed by Tukey’s pairwise comparisons (statistical package: PAST; Hammer et al., 2001). For significant differences, I calculated effect sizes using Cohen’s d (difference between group means divided by the pooled standard deviation). I additionally tested for associations between bird density, benthic larval density, and number of emerging insects across sites using Pearson’s correlation coefficient, r. I complemented this approach by using multiple regression analyses to explore the association between birds and emerging insects while controlling for channel width (the total flux of emerging insects crossing the stream-riparian boundary may increase with channel width; Benjamin et al., 2011). Bird data were explored further to compare the responses of (1) birds of different winter status (re-classified into “rare,” including scarce and irregular species, or “common,” including fairly common and common species; Table 1), and (2) different foraging methods. Since individual species can use more than one method, foraging methods were quantified using fuzzy coding. I assigned fuzzy indexes to quantify the extent to which each species exhibited each method (Table 1). Then, I multiplied these indexes by the abundance of the species and aggregated the resulting values across species. In all statistical comparisons, the two sites on the reference stream Magliasina, which were similar, were pooled into one group.
Results
Based on monitoring data, the Vedeggio downstream of the STP effluent had higher PTOT concentrations and temperatures than upstream of the STP or the reference stream Magliasina (Table 2). The average BOD5 concentration was also slightly higher, although the difference was less marked. I also observed differences in biofilm composition. The Vedeggio downstream of the STP effluent was carpeted with sewage fungus (a growth of filamentous bacteria and other associated organisms), which was not observed at the other sites. Based on my own measurements, Vedeggio sites upstream or downstream of the STP effluent had similar air temperature (the average difference, 0.2°C, was smaller than the within-site variability, 1.4°C; Table 2). Between-stream differences were slightly larger, with the Magliasina showing temperatures ~ 2°C lower than the Vedeggio (Table 2).
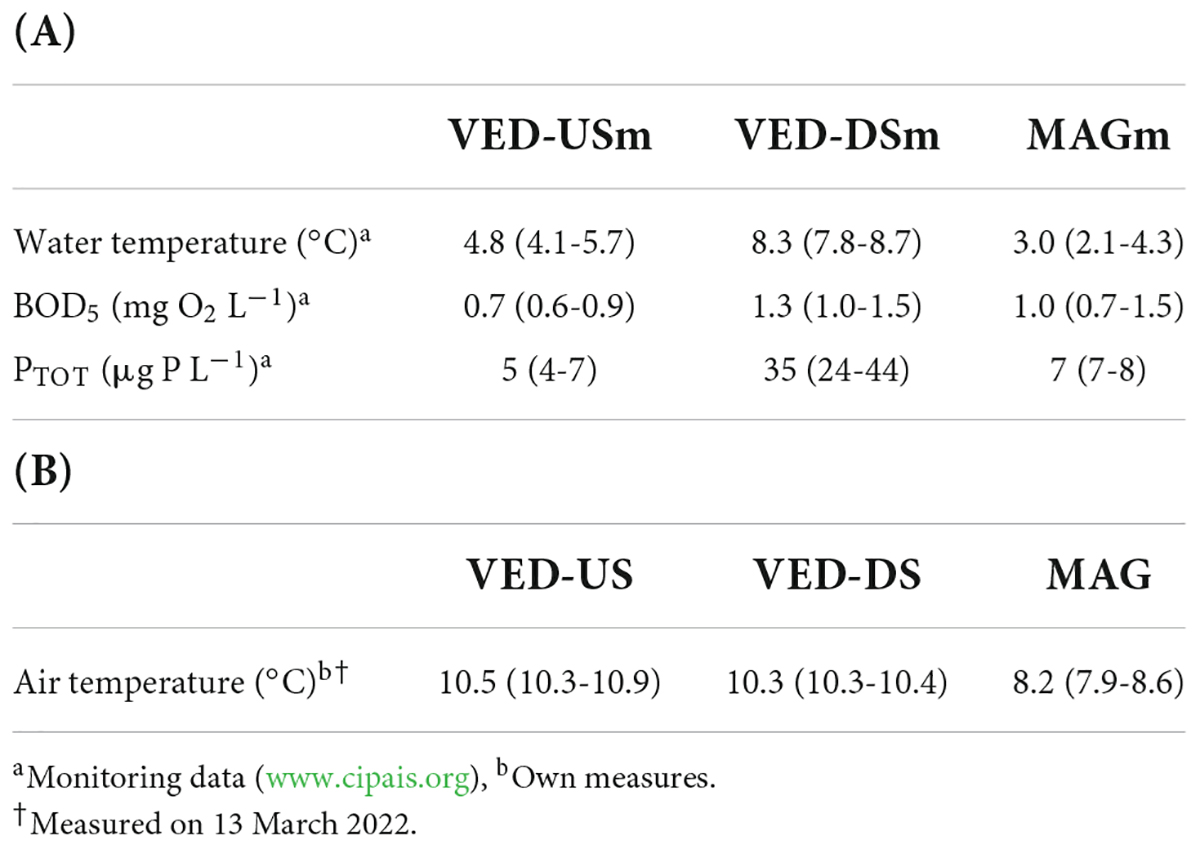
Table 2. (A) Differences in water temperature and water chemistry (biochemical oxygen demand, BOD5, and total phosphorus, PTOT) among monitoring sites (values in parenthesis indicate min and max of monthly measures within stations). (B) Differences in air temperature among study sites (values in parenthesis indicate min and max within site groups VE-US, VE-DS, and MA).
The impacted VED-DS sites had on average 7× higher densities of chironomid larvae, 6× higher emergence of chironomid adults, and 8× higher densities of insectivorous birds than the VED-US sites (Figure 1; ANOVAs significant at p ≤ 0.02; Table 3; data in Supplementary Table 3). Emergence of the mayfly B. rhodani was also 6× greater downstream than upstream of the STP effluent, although larval densities were not significantly different (Figure 1 and Table 3). Likewise, the sites VED-DS had 10× greater larval chironomid density, 25× higher emergence of chironomid adults, and 4× higher bird density than the reference MAG sites (Figure 1; ANOVAs significant at p ≤ 0.02, Table 3). No emerging B. rhodani were sampled at MAG sites (Figure 1). Across all sites, the density of insectivorous birds was strongly associated with the numbers of emerging chironomids (r = 0.94; p < 0.001) and B. rhodani (r = 0.95; p < 0.001; Supplementary Table 4). These associations remained significant after controlling for channel width (Supplementary Table 5). Pairwise correlations also indicated that chironomid emergence and chironomid benthic density were strongly associated (r = 0.91; p < 0.001), whereas the association between B. rhodani emergence and B. rhodani benthic density was non-significant (r = -0.39, p > 0.1).
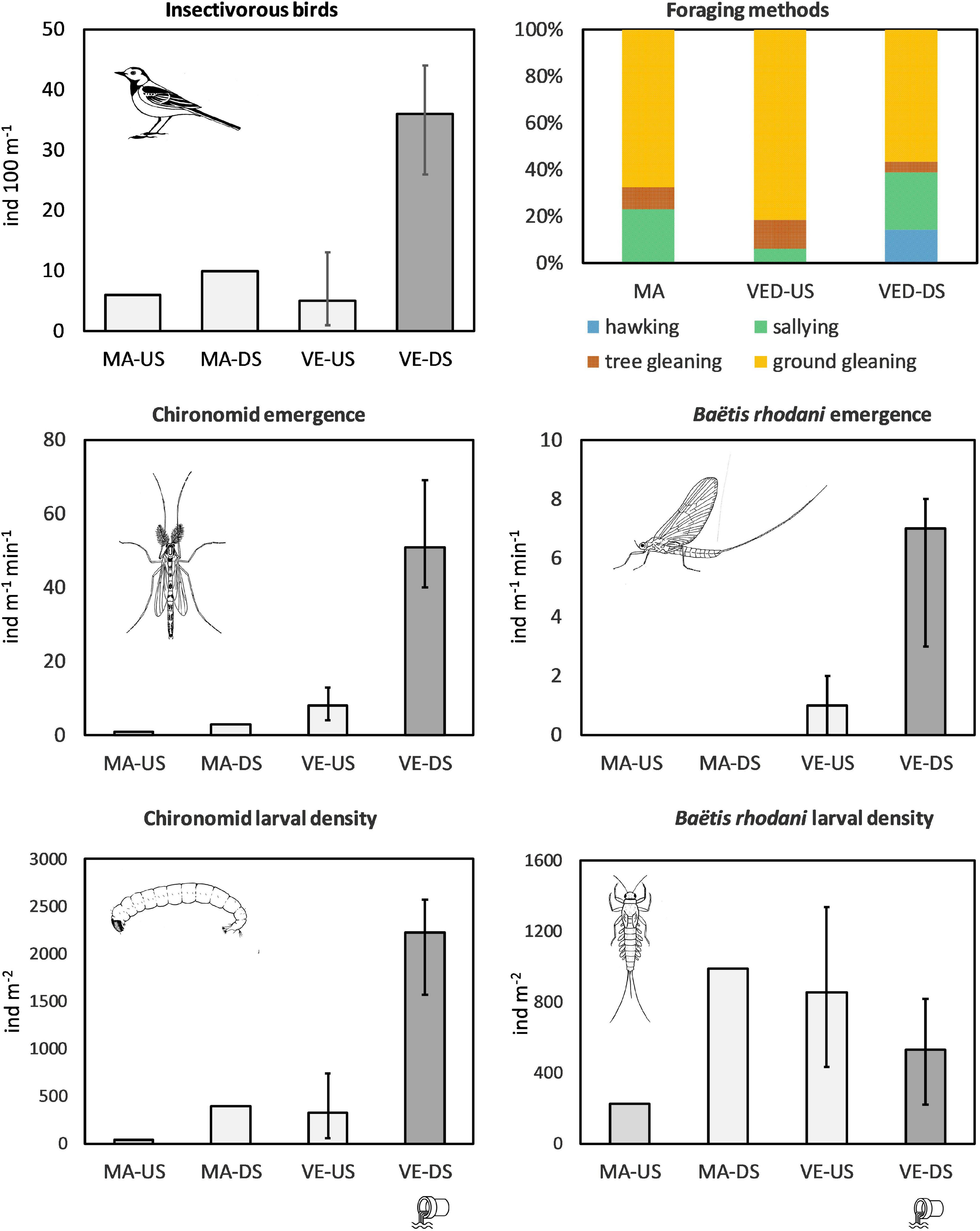
Figure 1. Top right: bird foraging methods in percentage (average within MA, VED-US, and VED-DS sites). Other charts: average (VED-US and VED-DS) or total (MAG-US and MAG-DS) insectivorous-bird linear density, larval chironomid and Baëtis rhodani density, and chironomid and Baëtis rhodani emergence rate. Error bars indicate maximum and minimum values within VED-US sites (n = 4) and VED-DS sites (n = 3).
Differences also concerned winter-status groups (rare vs. common wintering species) and foraging methods. Rare species were only detected at VED-DS sites, where they made up 30% of the total insectivorous bird assemblage (by linear density). Observations revealed that hawking and sallying (both involving catching insects in the air) were used more frequently (sallying) or exclusively (hawking) by rare wintering species, whereas gleaning was predominant among common species (Table 1). Ground gleaning was also the most common foraging method across sites. At sites downstream of the STP effluent, feeding methods were more diverse due to higher frequency of sallying and hawking. At VE-DS sites, the combination of these two feeding methods were 6× times more frequent than at VED-US sites (36% vs. 6%) and 2× times more frequent than at MAG sites (36% vs. 23%; ANOVA significant at p = 0.019, VED-DS significantly different from VED US but not from MAG; Table 3).
Discussion
Pollution by the STP effluent caused a strong aggregational response by wintering insectivorous birds (4–8× more abundant along polluted sites VED-DS than unpolluted sites VED-US and MAG) due to increased aquatic emergence. A causal effect was supported by three lines of evidence: The differences in these variables (insect emergence and bird density) between the upstream and downstream sites, the differences between the impacted and the reference stream, and the existence of a plausible mechanism, i.e., greater food availability in times of shortage. The strong correlation between aquatic emergence and birds across all sites further indicates that insectivorous birds closely tracked the availability of food. The magnitude and consistency of effects throughout the “larvae→emergence→birds” pathway support the occurrence of a link between wintering insectivorous birds and aquatic emergence (Nakano and Murakami, 2001) and illustrate how strongly stream alterations propagate through this nexus.
Was the aggregation of birds only caused by organic pollution favoring chironomids? The results suggest there are additional factors. First, the sites downstream of the STP effluent showed not only organic pollution, but also nutrient enrichment (more phosphorus), and warming (higher temperature). In fact, differences in BOD5 between polluted and unpolluted sites were slight, although the observation of sewage fungus downstream of the STP effluent suggests substantial organic pollution (Curtis, 1969). Second, the mayfly Baëtis rhodani also contributed to the aggregation of birds, even though this species was not favored by organic pollution (larval densities were similar upstream and downstream of the STP effluent). A better explanation is that greater aquatic emergence at polluted sites was caused by a taxon-dependent combination of thermal, nutrient, and organic pollution. For B. rhodani, which usually emerge from March onward (Sartori and Landolt, 1999), higher emergence was probably caused by higher water temperature, which accelerated development and advanced emergence by 1–2 months. For chironomids, higher emergence was probably caused jointly by organic pollution, which increased larval populations, and higher temperature, which allowed a greater proportion of the larvae to complete their development in winter (Pinder, 1986).
The aggregation of insectivorous birds at polluted sites was not a purely numerical response (8× higher densities of the same birds), but involved changes in species composition and foraging methods. Rare wintering species (Crag martin, Chiffchaff, and Redstart) were particularly favored, likely because of their foraging method. These birds fed largely on aerial insects (by hawking or sallying), a resource available in substantial amounts only at polluted sites (in addition to the measured difference in emergence, at these sites I also noted swarms of chironomids in the air). Temperate streams can produce emergence throughout the winter (Nakano and Murakami, 2001), but in short supply. At polluted sites, the supply was probably sufficient to make hawking and sallying energetically profitable, creating a winter haven for a functional category of birds that would otherwise migrate further South (with few exceptions).
The nutrient, organic, and thermal pollution caused by the STP effluent mirrors the effects of other environmental issues causing pollution or warming, including urbanization and climate change (Lepori et al., 2015). Therefore, in temperate regions, some of the effects observed in this study (increased winter emergence, increased abundance of rare wintering birds) might have become or might become widespread. Circumstantial evidence already exists. In Switzerland, wintering Chiffchaffs, White wagtails, and Black redstarts have become more abundant since the 1980s–1990s (Maumary et al., 2007). This increase may be partly explained by an increase in winter air temperature. However, because these birds feed on insects and tend to aggregate around lakes and rivers in the winter (Maumary et al., 2007), they probably also benefited from increased aquatic emergence due to warmer water temperatures.
This study joins a growing number of papers that indicate that human-caused streams alterations can propagate to woodland or forest birds through effects on aquatic emergence. Jonsson et al. (2012) found that, in Sweden, during the breeding season, two feeding groups of birds (seed and/or large insect eaters and small-insect eaters) were more abundant along free-flowing rivers versus regulated rivers, matching differences in aquatic insect emergence. In Sierra Nevada, introduction of non-native trout in some alpine lakes strongly reduced aquatic insects, causing a six-fold aggregation of Gray-crowned Rosy-Finches (Leucosticte tephrocotis dawsoni) around fishless lakes (Epanchin et al., 2010). Jointly, these studies indicate that aquatic emergence is important to insectivorous birds throughout the year, although presumably for different reasons, i.e., to improve adult survival in winter and increase breeding success in spring and summer.
Nakano and Murakami (2001) wrote that streams and terrestrial habitats are so interconnected that “degradation of one habitat may have more detrimental effects on neighboring communities.” The effect on the bird community described in this study (aggregation of wintering birds) may not appear detrimental, but it should be considered in the context of broader changes, some of which are undesirable. Earlier development and emergence of aquatic insects in winter due to warmer conditions might favor wintering birds, but could also shift peak emergence to earlier in the year, reducing the availability of aquatic insects and associated critical nutritional compounds (e.g., polyunsaturated fatty acids) during spring nesting (Shipley et al., 2022). In a similar vein, pollution might favor winter chironomids, but have negative impacts on the aggregated assemblage of emerging insects (which includes mayflies, stoneflies, caddisflies, and other dipterans), causing declines in breeding insectivorous birds (Manning and Sullivan, 2021). In addition, aquatic emergence from polluted streams and rivers may convey pollutants and pathogens that pose toxicological risks (Xiang et al., 2016). Research into these complex consequences is just developing. Systems amenable to relatively simple study, like the one studied for this paper, could continue to offer useful opportunities for learning.
Data availability statement
The raw data supporting the conclusions of this article will be made available by the authors, without undue reservation.
Ethics statement
Ethical review and approval was not required for the animal study because birds were surveyed by sight and sound from a distance, taking care in minimizing disturbance.
Author contributions
FL designed the study, carried out the field and laboratory work, and wrote the manuscript.
Conflict of interest
The author declares that the research was conducted in the absence of any commercial or financial relationships that could be construed as a potential conflict of interest.
Publisher’s note
All claims expressed in this article are solely those of the authors and do not necessarily represent those of their affiliated organizations, or those of the publisher, the editors and the reviewers. Any product that may be evaluated in this article, or claim that may be made by its manufacturer, is not guaranteed or endorsed by the publisher.
Supplementary material
The Supplementary Material for this article can be found online at: https://www.frontiersin.org/articles/10.3389/fevo.2022.926529/full#supplementary-material
Footnotes
References
Anderson, A. M., and Ferrington, L. C. (2013). Resistance and resilience of winter-emerging Chironomidae (Diptera) to a flood event: Implications for Minnesota trout streams. Hydrobiologia 707, 59–71. doi: 10.1007/s10750-012-1406-4
Baxter, C. V., Fausch, K. D., and Saunders, W. C. (2005). Tangled webs: Reciprocal flows of invertebrate prey link streams and riparian zones. Freshw. Biol. 50, 201–220. doi: 10.1111/j.1365-2427.2004.01328.x
Benjamin, J. R., Fausch, K. D., and Baxter, C. V. (2011). Species replacement by a nonnative salmonid alters ecosystem function by reducing prey subsidies that support riparian spiders. Oecologia 167, 503–512. doi: 10.1007/s00442-011-2000-6
Curtis, E. J. C. (1969). Sewage fungus: Its nature and effects. Water Res. 3, 289–311. doi: 10.1016/0043-1354(69)90084-0
Epanchin, P. N., Knapp, R. A., and Lawler, S. P. (2010). Nonnative trout impact an alpine-nesting bird by altering aquatic-insect subsidies. Ecol. 91, 2406–2415. doi: 10.1890/09-1974.1
Fuller, R. J., and Glue, D. E. (1978). Seasonal activity of birds at a sewage-works. Br. Birds 71, 235–244.
Hammer, Ø, Harper, D. A. T., and Paul, D. R. (2001). Past: Paleontological statistics software package for education anddata analysis. Palaeontol. Electron. 4:9.
Iwata, T., Nakano, S., and Murakami, M. (2003). Stream meanders increase insectivorous bird abundance in riparian deciduous forests. Ecography 26, 325–337. doi: 10.1034/j.1600-0587.2003.03355.x
Jackson, J. K., and Fisher, S. G. (1986). Secondary production, emergence, and export of aquatic insects of a Sonoran Desert stream. Ecology 67, 629–638. doi: 10.2307/1937686
Jonsson, M., Strasevicius, D., and Malmqvist, B. (2012). Influences of river regulation and environmental variables on upland bird assemblages in northern Sweden. Ecol. Res. 27, 945–954. doi: 10.1007/s11284-012-0974-0
Lepori, F., Pozzoni, M., and Pera, S. (2015). What drives warming trends in streams? A case study from the Alpine foothills. River Res. Appl. 31, 663–675. doi: 10.1002/rra.2763
Losos, B. (1984). The influence of pollution on the density and production of Chironomidae (Diptera) in running waters. Limnologica 15, 7–19.
Manning, D. W., and Sullivan, S. M. P. (2021). Conservation across aquatic-terrestrial boundaries: Linking continental-scale water quality to emergent aquatic insects and declining aerial insectivorous birds. Front. Ecol. Evol. 9:633160. doi: 10.3389/fevo.2021.633160
Maumary, L., Vallotton, L., and Knaus, P. (2007). Les oiseaux de Suisse. Sempach: Station ornithologique Suisse.
Murakami, M., and Nakano, S. (2000). Species-specific bird functions in a forest-canopy food web. Proc. R. Soc. Lond. B 267, 1597–1601. doi: 10.1098/rspb.2000.1184
Nakano, S., and Murakami, M. (2001). Reciprocal subsidies: Dynamic interdependence between terrestrial and aquatic food webs. Proc. Natl. Acad. Sci. U.S.A. 98, 166–170. doi: 10.1073/pnas.98.1.166
Pinder, L. C. V. (1986). Biology of freshwater Chironomidae. Annu. Rev. Entomol. 31, 1–23. doi: 10.1146/annurev.en.31.010186.000245
Sartori, M., and Landolt, P. (1999). Atlas de distribution des éphémères de Suisse (Insecta, Ephemeroptera). Fauna Helvetica 3. Neuchatel: Centre Suisse de Cartographie de la Faune and Schweizerische Entomologische Gesellschaft.
Shipley, J. R., Twining, C. W., Mathieu-Resuge, M., Parmar, T. P., Kainz, M., Martin-Creuzburg, D., et al. (2022). Climate change shifts the timing of nutritional flux from aquatic insects. Curr. Biol. 32, 1342–1349. doi: 10.1016/j.cub.2022.01.057
Keywords: aquatic emergence, aggregational response, chironomids, subsidies, sewage pollution, Swiss streams
Citation: Lepori F (2022) Stream pollution causes aggregation of wintering insectivorous birds through increased aquatic emergence. Front. Ecol. Evol. 10:926529. doi: 10.3389/fevo.2022.926529
Received: 22 April 2022; Accepted: 03 August 2022;
Published: 26 August 2022.
Edited by:
Andrea Sciarretta, University of Molise, ItalyReviewed by:
Irene Saavedra Garcés, Doñana Biological Station, Spanish National Research Council (CSIC), SpainHongyong Xiang, Yunnan University, China
Copyright © 2022 Lepori. This is an open-access article distributed under the terms of the Creative Commons Attribution License (CC BY). The use, distribution or reproduction in other forums is permitted, provided the original author(s) and the copyright owner(s) are credited and that the original publication in this journal is cited, in accordance with accepted academic practice. No use, distribution or reproduction is permitted which does not comply with these terms.
*Correspondence: Fabio Lepori, ZmFiaW8ubGVwb3JpQHN1cHNpLmNo