Hydrogeochemical processes controlling the salinity of surface water and groundwater in an inland saline-alkali wetland in western Jilin, China
- 1Northeast Institute of Geography and Agroecology, Chinese Academy of Sciences, Changchun, China
- 2School of Geographical Sciences, Changchun Normal University, Changchun, China
Understanding the hydrochemical evolutionary mechanisms of surface water and groundwater in saline-alkali wetlands in arid and semi-arid regions is necessary for assessing how wetland water resource utilization and restoration processes may affect the natural interface between wetland salinity and water. The Momoge National Nature Reserve (MNNR) is an inland wetland in northeastern China that is mainly fed by irrigation water and floods from the Nenjiang River. The purpose of the present study is to describe the spatial distribution characteristics of surface water and groundwater hydrochemistry and salinity in the MNNR and analyze the main processes controlling these parameters. The composition of stable isotopes (δ2H and δ18O) and water chemistry, including the levels of Na, K, Ca, Mg, HCO3, SO4, and Cl, of 156 water samples were analyzed. The results show that the lake water in the MNNR is at a risk of salinization owing to a high degree of evaporation. The analysis of the ion ratio and mineral saturation index showed that the ions in water are primarily derived from aquifer leaching, and the precipitation of Ca2+ and Mg2+ resulted in lower Ca2+ and Mg2+ levels in lake water than in groundwater. Hydrogen and oxygen stable isotope and deuterium excess analyses show that evaporation is the dominant factor controlling the hydrochemistry and salinity of lake water in the MNNR. Long-term effective monitoring of lake water and groundwater must be developed to provide an early warning for the salinization of lake water and a scientific basis for the protection and restoration of wetland ecosystem functions within the MNNR.
Introduction
Wetland ecosystems have a variety of unique functions and structures between terrestrial and aquatic ecosystems. Inland saline-alkali wetlands are an important type of wetlands. They are mostly distributed in arid, semi-arid, and sub-humid transition areas and characterized by inundation or shallow-surface water environments, where saline-alkali soils and halobiotic communities develop and constitute a unique geographical complex of inland saline-alkali land ecosystems (Sun et al., 2000; Zhao et al., 2021). Multiple threats from climate change, population growth, land use, and hydraulic engineering have resulted in inland wetlands being exposed to the risk of increased saline inflows (Fowler et al., 2014; Herbert et al., 2015). Salinization is an important factor that affects and drives the ecological characteristics of the water environment in saline-alkali wetlands. The salinization of wetland water bodies in arid and semi-arid regions has attracted attention worldwide (Lyons et al., 2007; Nachshon et al., 2014; Liu et al., 2018a; Chamberlain et al., 2020). Owing to the stress of wetland water salinization, the metabolism and carbon sequestration of wetland aquatic plants have been seriously affected, resulting in a gradual weakening in the functionality of wetlands as carbon pools (Wen et al., 2017).
Climate, topography, water-rock interactions, and surface water-groundwater (SW-GW) interactions play important roles in the variation in salinity and hydrochemistry of wetlands. Owing to the influence of these factors, the increase in water salinity may be a slow process, lasting thousands to tens of thousands of years (Skrzypek et al., 2013). High evapotranspiration directly affects the salinity of wetland water and contributes to the formation of soluble salts, which are incorporated into wetland water by groundwater or tidal flow (Alvarez Mdel et al., 2015). Wetlands are often located at lower elevations, which exposes them to the risk of secondary salinization because of rising water table levels. Furthermore, highly dynamic surface-groundwater interactions in wetlands drive the salinity, which can have positive or negative impacts on wetland ecology (Jolly et al., 2008; Xin et al., 2022). Stable isotopes and major ions in water are important tracers for understanding the process of salinity change, and they can be used to analyze the causes of water salinity in coastal or inland wetlands (Galliari et al., 2021).
The saline-alkali soil region in western Jilin Province, China, is a plain surrounded by mountains and watersheds. The source of soil salinity is the accumulation of soluble salts in the parent rock where groundwater flows from the surrounding uplands to the depressions. The Momoge National Nature Reserve (MNNR) is located in northwest Jilin, where groundwater from the surrounding uplands converges. In 2013, it was included in the list of wetlands of international importance under the Ramsar Convention. The main geochemical processes that control the salinity and hydrochemical characteristics of the MNNR surface water and groundwater need to be explored. The objectives of this study are to (1) explore the salinity and hydrochemical distribution characteristics of surface water and groundwater in the MNNR and (2) determine the main geochemical processes that drive water chemistry and salinity in water bodies within the MNNR.
Materials and methods
Study area
The MNNR is located in the western region of Jilin Province, China, with an area of approximately 1,440 km2 and a geographic range between 45°42′25″–46°180″ N and 123°27′0″–124°4′33.7″ E (Figure 1). The MNNR is a typical reserve established to protect inland wetlands and aquatic ecosystems in northeastern China. It is an important migratory stop for the endangered species Grus leucogeranus and was included in the list of wetlands of international importance in 2013. The altitude of the reserve is between 100 and 171 m, which is high in the northwest and low in the southeast. The uplands in the western MNNR surround many small and large lakes, while the eastern region is relatively flat with an elevation difference of 2–5 m.
Lake distribution
MNNR is located on the western edge of the Songnen Plain in the northern region of the Songliao subsidence belt. Due to the Mesozoic rifted basin and the combined action of paleogeography and paleoenvironment, alluvial, lacustrine plains, and scattered lakes were formed. At the beginning of the Quaternary Period, the Songnen Plain sank slowly, and erosion was strengthened, causing transported gray-white sand and gravel to be deposited at the bottom of the Quaternary loose layer of the Songnen Plain. In the Early Pleistocene, the western part of the Songnen Plain continued to sink and formed a large ancient lake. In the Late Pleistocene, due to the slow uplift of the watershed, the subsidence center of the Songnen Plain continued to shrink and migrate westward. As a result of the Wurm glacial stage, the cold climate spread to the Songnen Plain, and the resulting cold and dry state caused the ancient lake to gradually decline. The lake area shrank, and it was divided into scattered small lakes. The rivers that entered the plain became unconfined, and the paleohydrological network underwent major changes, forming inland river systems and lakes, accompanied by extensive saline-alkali flatlands. Driven by severe cold and high pressure, the anticyclonic winds were strong, and wind erosion formed ancient sand dunes, sand ridges, and wind-eroded depressions, which became the present day saline-alkali lake bubbles. Since the Late Pleistocene, the lake area has gradually decreased and been divided into smaller lakes. Therefore, MNNR is a lacustrine alluvial plain formed by the combined action of lakes and rivers. The continuous development and multiple river diversions have resulted in numerous lakes.
Meteorology and hydrology
MNNR is located in a semi-arid region and has a temperate continental monsoon climate with an average annual temperature of 4.2°C. The highest temperature occurs in July, with an average of 23.5°C, and the lowest temperature occurs in January, with an average of –17.4°C. The average annual precipitation is 391.8 mm. Generally, precipitation is concentrated from June to September, reaching 300 mm and accounting for 76.6% of the annual precipitation. The average annual evaporation is 1585.1 mm, with the highest evaporation in May.
The MNNR is bounded by two natural rivers to the south and east. The Nenjiang River, to the east, flows through the reserve for 111.50 km, with a drainage area of more than 300 km2. The Taoer River, to the south, flows through the reserve for 60 km and then joins the Yueliangpao Reservoir. There are two seasonal rivers that flow through the reserve: the Erlongtao and Huerda rivers (Figure 1). Many lakes have developed within this reserve under the combined influence of topography and surface runoff. The west is dominated by brackish lakes dammed by piedmont fan-edge depressions, the east is dominated by floodplain freshwater lakes affected by floods, the middle is dominated by low plains, and the lower reaches of the river form a number of water passages from north to south, developing a series of beaded freshwater lakes. The Qianhang Drainage Station on the northern boundary of the reserve begins to discharge farmland water into the reserve annually in May and continues to discharge downstream through the sluice gate of Baihe Lake.
Sample collection and field monitoring
Groundwater and surface water in the reserve were sampled three times during one hydrological year in 2019 (Figure 1). The samples included (1) groundwater from 43 wells, (2) surface water from 18 lakes, and (3) water from major rivers flowing into the reserve. The samples were collected before the wet period (June), during the wet period (September), and during the dry period (November). All groundwater samples were collected after pumping three times the volume of water in the pipe. Groundwater sampling was concentrated in the central and western parts of the reserve as there are few boreholes in the eastern floodplain of the MNNR. Twenty-one sub-basins were divided based on the topography to ensure that groundwater sampling points were evenly distributed in each sub-basin in these areas. During the sampling campaign, the groundwater table was measured at each sampling point. These results provided a basis for assessing the effects of precipitation and hydrodynamic conditions on the isotopic and chemical compositions of lake and alluvial aquifer groundwater.
A total of 156 water samples were analyzed for stable isotope (δ2H and δ18O) and hydrochemical characteristics. The pH, dissolved oxygen (DO), electrical conductivity (EC), and total dissolved solids (TDS) were tested and recorded on-site using multi-parameter water quality analyzers (HANNA HI98121 and HI98129, Italy). Laboratory analysis was completed at the Northeast Institute of Geography and Agroecology, Chinese Academy of Sciences. The δ2H and δ18O values of the water samples were measured using a stable isotope mass spectrometer (MAT253, United States), and the measurement results were corrected with Vienna Standard Mean Ocean Water (VSMOW). The accuracies of δ2H and δ18O were found to be 0.3‰ and 0.1‰, respectively. The K+, Na+, Ca2+, and Mg2+ concentrations of the water samples were determined using inductively coupled plasma spectrometry (ICP-OES735E, United States), and HCO3–, Cl–, and SO42– were determined using ion chromatography (ICS-2100, United States).
Data analysis
The varying trends of precipitation, temperature, and evaporation in the MNNR from 1960 to 2019 were analyzed. The local deuterium excess was calculated using the equation proposed by Dansgaard (1964). Using the weighted linear regression model in SPSS software, a correlation analysis of the main ions and environmental parameters in surface water and groundwater in MNNR was conducted, based on which the causes of water salinity were inferred. The possible hydrogeochemical processes and the causes of surface water and groundwater chemistry were determined using a Piper diagram, main ion relationship analysis, and mineral saturation index calculation.
Results
Hydrochemical characteristics
Alkaline water occupied most of the depression wetlands in the MNNR, and the pH varied from 7.17 to 9.44. The groundwater was practically neutral, with a pH ranging from 6.93 to 7.88. The groundwater and surface water varied from freshwater (0.11 g/L) to brackish (3.97 g/L). The TDS of the Nenjiang River water were between 0.10 and 0.15 g/L, while the TDS of groundwater and lake water (including reservoirs) was considerably higher than that of river water. The average TDS of the sampling points in November was 0.80 g/L, which was nearly 0.20 g/L higher than that in September (0.61 g/L). In addition, the groundwater TDS at each sampling point did not change significantly during the three sampling campaigns. Water in the Nenjiang River was dominated by HCO3–, Ca+, Mg2+, and Na+, and the types of cations varied seasonally. The lake water was dominated by HCO3– and Na+, which accounted for approximately 80% of the main ions in the lake water. HCO3-Na•-Ca type water occurred continuously in the reservoir water. Finally, most of the groundwater was dominated by HCO3–, Na+, and Ca+, and the cations in a small fraction of the groundwater were dominated by Mg2+ (Table 1 and Figure 2).
Distribution of H and O stable isotopes
The local meteoric water line (LMWL) was determined to be δ2H = 5.75δ18O-16.85 by fitting the annual stable isotopes for precipitation measured data from 2019. The slope of the LMWL was smaller than that of the global meteoric water line (GMWL), reflecting the semi-arid characteristics of the area. Evaporation of precipitation from the surface accounts for a large proportion of the local water vapor source. The average values of δ2H and δ18O in the groundwater, lake water, and river water were –76.3, –9.1, and –50.8% and –4.7, –55.5, and –5.3%, respectively (Figure 3). The isotope values of groundwater, lake water, and river water in the MNNR were distributed along the LMWL, indicating the interaction between different water bodies was driven by precipitation recharge. The large water surface and arid climate contributed to the strong surface evaporation that occurred in the lake and the tail of the Erlongtao River, resulting in strong δ2H and δ18O enrichment, while the stable isotopes of groundwater and Nenjiang River water were relatively depleted.
Source of water solute
Driven by topography, groundwater flows from the northwest to southeast in the study area. During this process, water and rock interactions are dominated by leaching from fresh groundwater, with a TDS of approximately 0.5 g/L. Owing to the fine particle size of wetland sediments, the effect of groundwater recharge was weaker than that of the concentration caused by evaporation. This led to a significant increase in the TDS of the isolated wetland to 2.3 g/L. However, for Baihe Lake and other lakes fed by irrigation drainage, the effect of water mixing was stronger than that of the concentration, and the TDS of the lake water became stable at approximately 150 mg/L due to the large amount of freshwater input from the irrigation channel. The TDS concentrations correlated with that of Cl– and Na+ in groundwater (R > 0.90) (Figure 4), indicating the dissolution of halite in the aquifer. However, the TDS concentrations were primarily correlated with that of HCO3– and Na+ in the lake water (R = 0.99), which was different from groundwater. This indicated that groundwater is not the only recharge source of lake water and that river water may be a larger potential source. HCO3– is continuously input into the lake water from the river water and gradually accumulates under strong evaporation. In addition, pH was an important factor affecting the water chemistry of lake water, and it significantly correlated with the concentrations of HCO3– and Na+, and the pH range of lake water, resulting in HCO3– dominating the carbonate balance.
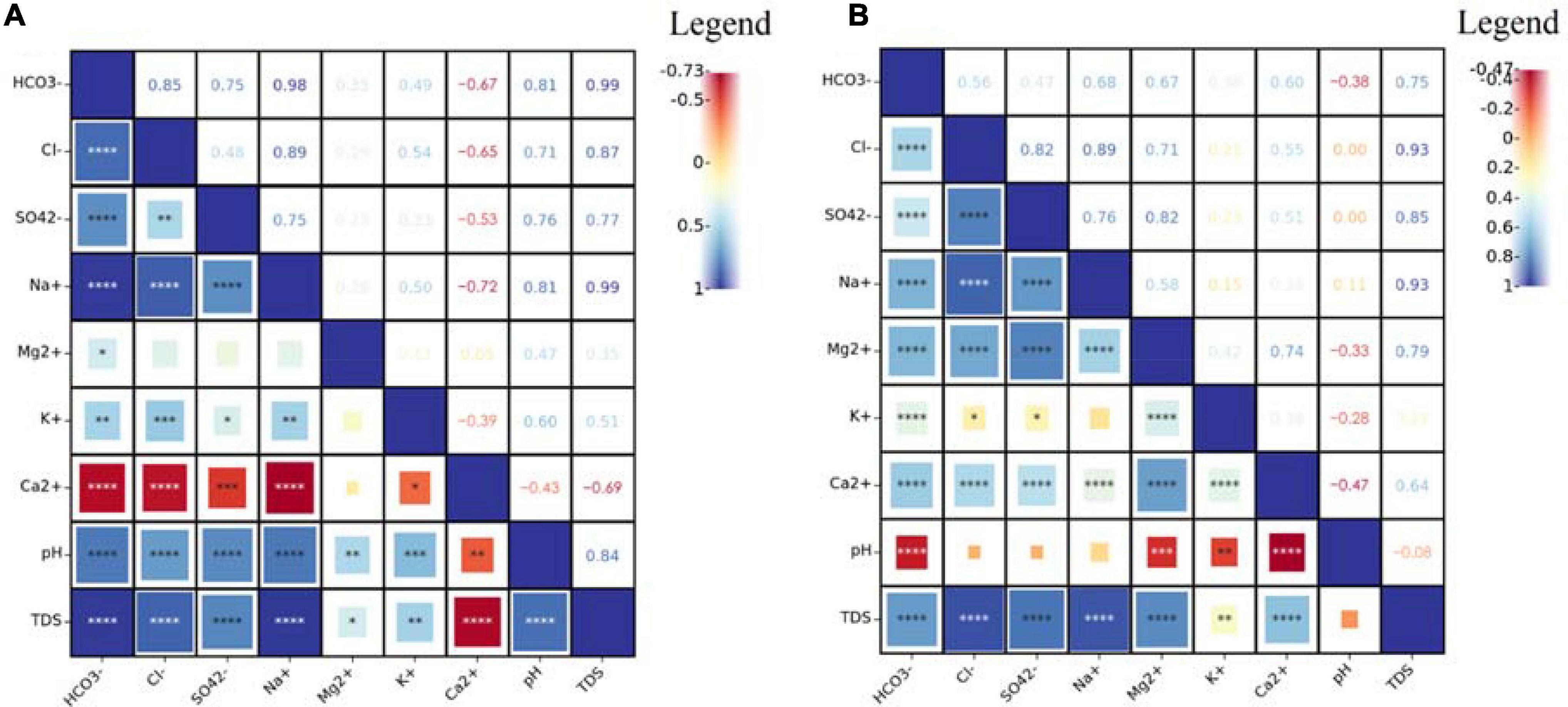
Figure 4. Correlation matrices of hydrochemistry and environmental parameters (A: lake water; B: groundwater; the number of * from 1 to 4 indicate the significance levels less than 0.05, 0.01, 0.001, and 0.0001 respectively).
The results of the relationship between the main ions (Figure 5) show that the groundwater points of HCO3– and SO42– against Ca2+ and Mg2+ were distributed around the 1:1 line, indicating the dissolution of calcite, gypsum, and other carbonate and sulfate minerals. However, the Ca2+ and Mg2+ concentrations in the lake water were considerably lower than those in the groundwater, and the proportion of Na+ in the lake water markedly increased. This was due to the precipitation of Ca2+ and Mg2+ in the lake water during repeated evaporation processes, which is the main process forming the saline-alkali soil in the MNNR.
Discussion
Mineral dissolution and precipitation
The saturation index (SI) of minerals in water is helpful in determining the dissolution and precipitation state of minerals to describe the hydrochemical origin and salinization process of the water body. The absence of Ca2+ and Mg2+ in the surface water (Figure 5) was due to the precipitation of calcite and dolomite (Figures 6A,B), as they were oversaturated in the surface water because of high evaporation. The Ca2+ produced by the dissolution of anhydrite and gypsum could not resist the precipitation of calcite and dolomite (Figures 6C,D). The SI values of halite in the groundwater and lake water were negative and similar, indicating that halite still had the potential to dissolve further and produce more Na+ and Cl– (Figure 6E).
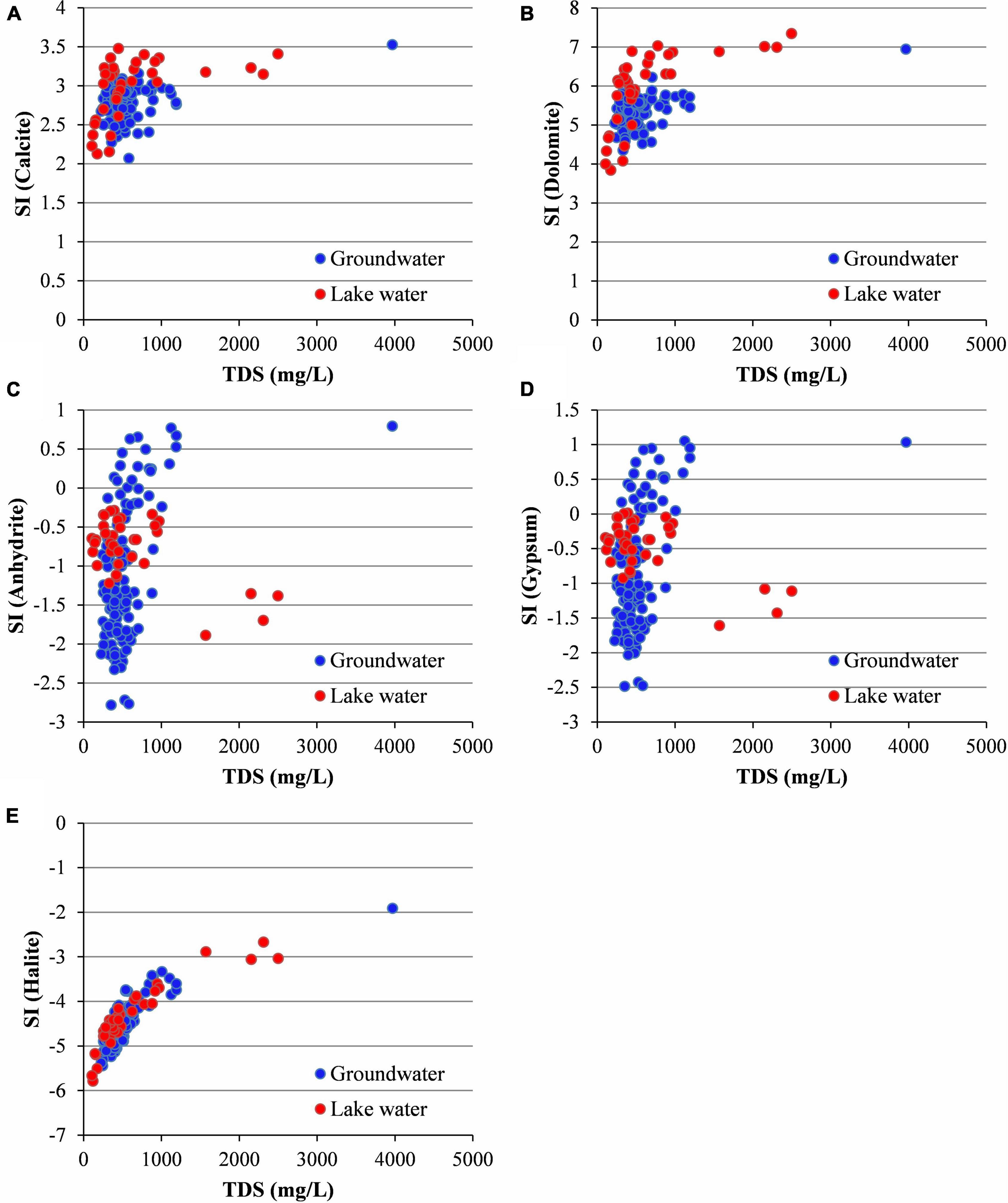
Figure 6. Saturation indices (SI) of water samples for selected minerals. (A) Calcite; (B) dolomite; (C) anhydrite; (D) gypsum; (E) halite.
Indication of d-excess characteristics
Studies have shown that evaporation leads to the fractional enrichment of 2H and 18O in water bodies, which is accompanied by an increase in TDS (Jin et al., 2018; Chen et al., 2020). The positive correlation between TDS and δ18O in the MNNR lake water (R2 = 0.4031) was not evident in the groundwater (Figure 7A). As the degree of evaporation increased, the δ18O in the lake water reached 2.26‰, and the TDS reached 2,499 mg/L. In contrast to the d-excess proposed by Dansgaard (1964), the LMWL equation was used to calculate the local d-excess. The local d-excess and TDS of lake water were negatively correlated (R2 = 0.2197), whereas the d-excess and TDS of groundwater were not significantly correlated (Figure 7B). The d-excess is important for identifying the mechanism of water salinization. After seawater evaporates, underground and surface runoff form through the hydrological cycle, and depression lake wetlands form in the discharge area. After the lake water evaporates again, the d-excess continues to decrease, and the salinity increases owing to continuous accumulation. This negative correlation between d-excess and TDS can explain the effect of evaporation on the stable isotopes, salinity, and the on-going salinization of the water body. The lake water in the MNNR undergoes salinization due to evaporation, and the groundwater salinity is mainly affected by aquifer leaching in the water flow path.
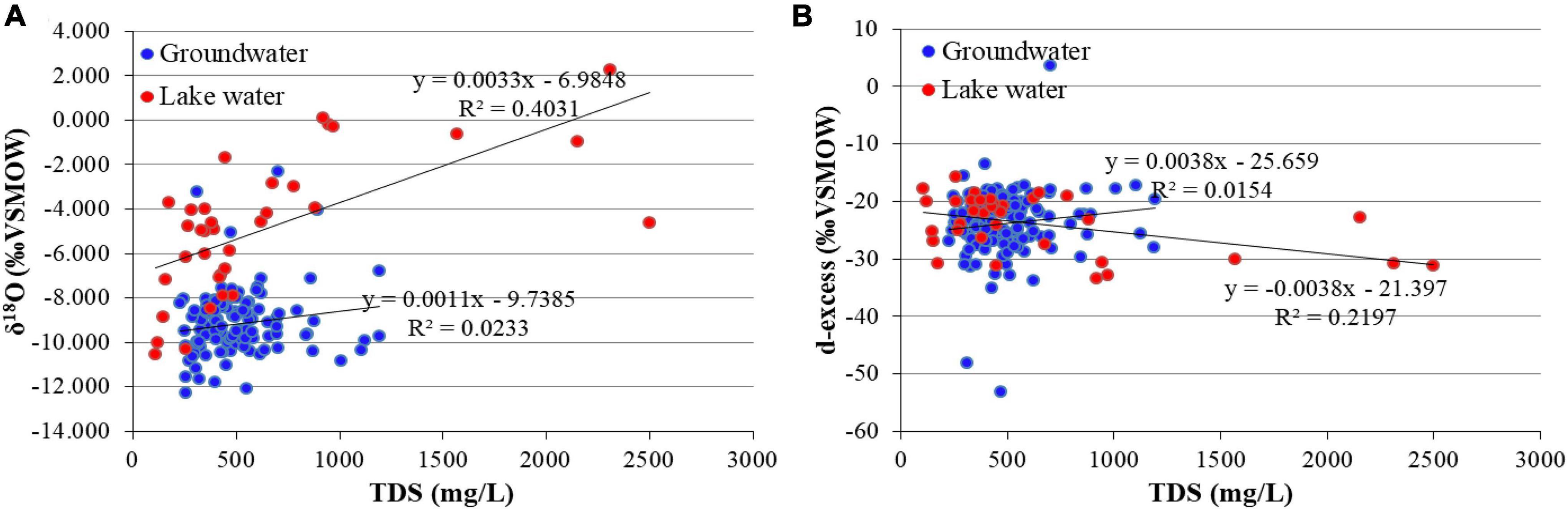
Figure 7. Relationships between TDS and H and O stable isotope parameters. (A) TDS and δ18O; (B) TDS and d-excess.
The precipitation, evaporation, and temperature data from Baicheng Station, a meteorological station near the study area, showed that from 1960 to 2019, the annual precipitation was in the range of 123–726 mm, with a gradual trend of decline. The annual evaporation was in the range of 1,137–2,240 mm. The 60-year average annual evaporation is nearly 4.4 times the average annual precipitation, which confirms the semi-arid climatic conditions and strong evaporation in the region. As evidence of global warming in the MNNR, the annual average temperature in the study area has gradually increased at an average rate of 0.053°C per year, but the annual evaporation in the study area has exhibited a decreasing trend (Figure 8). This is due to a decrease in the amount of solar radiation due to a decrease in the number of sunshine hours globally.
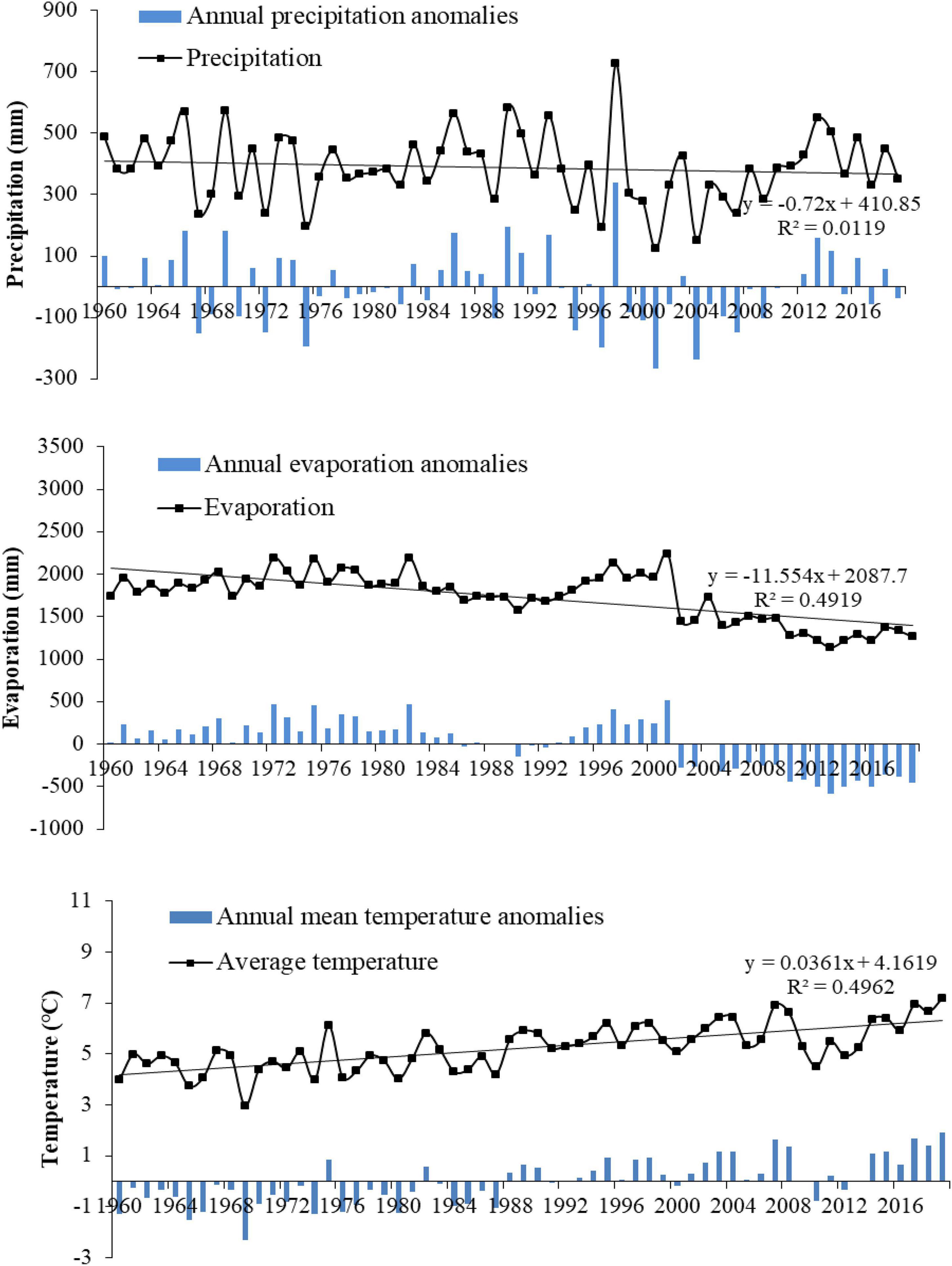
Figure 8. Variation of precipitation, evaporation, and temperature in the study area from 1960 to 2019.
Ecological consequences of wetland salinization
Salinization poses a significant threat to the structure and ecological functions of inland and coastal wetlands. Climate change, human modification, or destruction of the natural hydrological cycle has changed the balance of water and salinity, resulting in increased soil and water salinity in a number of wetlands at a higher rate than natural levels (Skrzypek et al., 2013). Natural changes in wetland salinity may occur over time scales of 10,000 years, whereas secondary salinization caused by anthropogenic factors may only occur over several decades. By analyzing the hydrochemical and isotopic characteristics of groundwater and surface water in the Manas River Basin, Liu et al. (2018b) concluded that mineral dissolution, transpiration, and agricultural irrigation activities are the main causes of groundwater salinization, which is consistent with the findings of this study. Elevated soil salinity during secondary salinization has the potential to increase the link between soil respiration and moisture content (Drake et al., 2014). Through laboratory-controlled experiments on peat deposits in coastal wetlands, van Dijk et al. (2015) found that soil and water salinization have an important impact on soil biogeochemical processes, and sediment cation exchange and brackish water supply can alter nitrogen and phosphorus availability and methane production rate Albecker et al. (2019) found that changes in water salinity affect frog skin microbial communities, thereby increasing the survival and health of the host frog These and other studies have displayed the causes of wetland salinization and the possible consequences for wetland ecology. From macro-to micro-perspectives, salinization plays an important role in the wetland carbon cycle, biological community distribution, and biogeochemical processes. Thus, the importance of freshwater wetlands should be clearly recognized. The effective monitoring and assessment of the sali process of freshwater wetlands are two important steps in protecting their ecological environment.
Protect wetlands from salinization
As freshwater demand increases, the control and remediation of freshwater salinization becomes increasingly important (Vörösmarty et al., 2010). Moreover, once salinization or secondary salinization occurs in freshwater wetlands, the original plant community is irreversibly degraded (Wang et al., 2019), causing the wetland to gradually change from an oasis to a desert. Researchers have attempted to use a variety of methods to slow the process of wetland salinization, providing several good case studies (Zalidis, 1998; Litalien and Zeeb, 2020; Wang et al., 2021). However, as each anthropogenic source of freshwater salinization is associated with a specific set of ions, there is currently no global standard for evaluating the salinization of freshwater wetlands (Schuler et al., 2018; Hintz et al., 2022). In the future, establishing standards and legislation for wetland salinization may be an effective way to slow the process of wetland salinization, as they play an important positive role in wetland salinization assessment and the reduction of anthropogenic impacts.
Conclusion
A portion of the lake water in the MNNR has been converted to brackish water or is near the threshold range for fresh and brackish water. In addition, the location and climatic conditions of the MNNR place it at risk for lake salinization. Groundwater brings salinity into depression lakes through aquifer leaching along the flow path, and semi-arid climatic conditions and high evaporation lead to the precipitation of Ca2+ and Mg2+ in lake waters. Although the discharge of irrigation water dilutes the water and forms drainage channels in certain downstream lakes, reducing the risk of lake salinity, it may increase the downstream nitrogen and phosphorus loads. Lakes lacking recharge water sources and drainage channels have now become brackish. Long-term effective monitoring of lake water and groundwater should be suggested to provide early warning of salinization of lake water within the MNNR, and active measures such as formulating relevant evaluation standards and regulations should be taken to avoid further salinization, which has a large impact on wetland ecosystems.
Data availability statement
The original contributions presented in this study are included in the article/supplementary material, further inquiries can be directed to the corresponding author.
Author contributions
YL: investigation, data curation, writing—original draft preparation, and funding acquisition. GC: software, visualization, validation, and writing—review and editing. ST: conceptualization, supervision, and methodology. All authors contributed to the manuscript and approved the submitted version.
Funding
This research was funded by the Chinese Academy of Sciences (XDA23060402), China Postdoctoral Science Foundation (2021M693154), and the Jilin Association of Science and Technology (QT202029). The authors express their deep gratitude to the funders for supporting this research.
Conflict of interest
The authors declare that the research was conducted in the absence of any commercial or financial relationships that could be construed as a potential conflict of interest.
Publisher’s note
All claims expressed in this article are solely those of the authors and do not necessarily represent those of their affiliated organizations, or those of the publisher, the editors and the reviewers. Any product that may be evaluated in this article, or claim that may be made by its manufacturer, is not guaranteed or endorsed by the publisher.
References
Albecker, M. A., Belden, L. K., and McCoy, M. W. (2019). Comparative analysis of anuran amphibian skin microbiomes across Inland and Coastal Wetlands. Microb. Ecol. 78, 348–360. doi: 10.1007/s00248-018-1295-9
Alvarez Mdel, P., Carol, E., and Dapena, C. (2015). The role of evapotranspiration in the groundwater hydrochemistry of an arid coastal wetland (Peninsula Valdes, Argentina). Sci. Total Environ. 50, 299–307. doi: 10.1016/j.scitotenv.2014.11.028
Chamberlain, S. D., Hemes, K. S., Eichelmann, E., Szutu, D. J., Verfaillie, J. G., and Baldocchi, D. D. (2020). Effect of drought-induced salinization on wetland methane emissions, gross ecosystem productivity, and their interactions. Ecosystems 23, 675–688.
Chen, J., Qian, H., Gao, Y., Wang, H., and Zhang, M. (2020). Insights into hydrological and hydrochemical processes in response to water replenishment for lakes in arid regions. J. Hydrol. 581:124386.
Drake, P. L., McCormick, C. A., and Smith, M. J. (2014). Controls of soil respiration in a salinity-affected ephemeral wetland. Geoderma 22, 96–102. doi: 10.1016/j.geoderma.2014.01.010
Fowler, D. N., King, S. L., and Weindorf, D. C. (2014). Evaluating abiotic influences on soil salinity of inland managed wetlands and agricultural croplands in a semi-arid environment. Wetlands 34, 1229–1239.
Galliari, J., Santucci, L., Misseri, L., Carol, E., and Alvarez, M. D. P. (2021). Processes controlling groundwater salinity in coastal wetlands of the southern edge of South America. Sci. Total Environ. 754:141951. doi: 10.1016/j.scitotenv.2020.141951
Herbert, E. R., Boon, P., Burgin, A. J., Neubauer, S. C., Franklin, R. B., Ardón, M., et al. (2015). A global perspective on wetland salinization: Ecological consequences of a growing threat to freshwater wetlands. Ecosphere 6, 1–43.
Hintz, W. D., Arnott, S. E., Symons, C. C., Greco, D. A., McClymont, A., Brentrup, J. A., et al. (2022). Current water quality guidelines across North America and Europe do not protect lakes from salinization. Proc. Natl. Acad. Sci. U.S.A. 119:e2115033119. doi: 10.1073/pnas.2115033119
Jin, K., Rao, W., Tan, H., Song, Y., Yong, B., Zheng, F., et al. (2018). HO isotopic and chemical characteristics of a precipitation-lake water-groundwater system in a desert area. J. Hydrol. 559, 848–860.
Jolly, I. D., McEwan, K. L., and Holland, K. L. (2008). A review of groundwater-surface water interactions in arid/semi-arid wetlands and the consequences of salinity for wetland ecology. Ecohydrology 1, 43–58. doi: 10.1002/eco.6
Litalien, A., and Zeeb, B. (2020). Curing the earth: A review of anthropogenic soil salinization and plant-based strategies for sustainable mitigation. Sci. Total Environ. 698:134235. doi: 10.1016/j.scitotenv.2019.134235
Liu, Y., Ding, Z., Bachofen, C., Lou, Y., Jiang, M., Tang, X., et al. (2018a). The effect of saline-alkaline and water stresses on water use efficiency and standing biomass of Phragmites australis and Bolboschoenus planiculmis. Sci. Total Environ. 644, 207–216. doi: 10.1016/j.scitotenv.2018.05.321
Liu, Y., Jin, M., and Wang, J. (2018b). Insights into groundwater salinization from hydrogeochemical and isotopic evidence in an arid inland basin. Hydrol. Process. 32, 3108–3127. doi: 10.1002/hyp.13243
Lyons, M. N., Halse, S. A., Gibson, N., Cale, D. J., Lane, J. A. K., Walker, C. D., et al. (2007). Monitoring wetlands in a salinizing landscape: Case studies from the Wheatbelt region of Western Australia. Hydrobiologia 591, 147–164. doi: 10.1007/s10750-007-0805-4
Nachshon, U., Ireson, A., Van Der Kamp, G., Davies, S., and Wheater, H. J. H. (2014). Impacts of climate variability on wetland salinization in the North American prairies. Hydrol. Earth Syst. Sci. 18, 1251–1263.
Schuler, M. S., Canedo-Arguelles, M., Hintz, W. D., Dyack, B., Birk, S., and Relyea, R. A. (2018). Regulations are needed to protect freshwater ecosystems from salinization. Philos. Trans. R. Soc. B Biol. Sci. 374:20180019. doi: 10.1098/rstb.2018.0019
Skrzypek, G., Dogramaci, S., and Grierson, P. F. (2013). Geochemical and hydrological processes controlling groundwater salinity of a large inland wetland of northwest Australia. Chem. Geol. 357, 164–177. doi: 10.1016/j.chemgeo.2013.08.035
Sun, G.-y, Luo, X.-z, Yi, F.-k, and Zhang, X.-p (2000). Concept, distribution law and formation machanism of inland saline-alkaline wetland. Chin. Geogr. Sci. 10, 254–260.
van Dijk, G., Smolders, A. J. P., Loeb, R., Bout, A., Roelofs, J. G. M., and Lamers, L. P. M. (2015). Salinization of coastal freshwater wetlands; effects of constant versus fluctuating salinity on sediment biogeochemistry. Biogeochemistry 126, 71–84. doi: 10.1007/s10533-015-0140-1
Vörösmarty, C. J., McIntyre, P. B., Gessner, M. O., Dudgeon, D., Prusevich, A., Green, P., et al. (2010). Global threats to human water security and river biodiversity. Nature 467, 555–561.
Wang, X., Zhang, D., Qi, Q., Tong, S., An, Y., Lu, X., et al. (2019). The restoration feasibility of degraded Carex Tussock in soda-salinization area in arid region. Ecol. Indic. 98, 131–136. doi: 10.1016/j.ecolind.2018.08.066
Wang, Z., Tan, W., Yang, D., Zhang, K., Zhao, L., Xie, Z., et al. (2021). Mitigation of soil salinization and alkalization by bacterium-induced inhibition of evaporation and salt crystallization. Sci. Total Environ. 755(Pt 1):142511. doi: 10.1016/j.scitotenv.2020.142511
Wen, B., Li, X., Yang, F., Lu, X., Li, X., and Yang, F. (2017). Growth and physiology responses of Phragmites australis to combined drought-flooding condition in inland saline-alkaline marsh. Northeast China. Ecol. Eng. 108, 234–239.
Xin, P., Wilson, A., Shen, C., Ge, Z., Moffett, K. B., Santos, I. R., et al. (2022). Surface water and groundwater interactions in salt marshes and their impact on plant ecology and coastal biogeochemistry. Rev. Geophys. 60, e2021RG00 0740.
Zalidis, G. (1998). Management of river water for irrigation to mitigate soil salinization on a coastal wetland. J. Environ. Manag. 54, 161–167.
Keywords: hydrogeochemical processes, inland wetland, salinity, hydrochemistry, stable isotope
Citation: Cui G, Liu Y and Tong S (2022) Hydrogeochemical processes controlling the salinity of surface water and groundwater in an inland saline-alkali wetland in western Jilin, China. Front. Ecol. Evol. 10:993849. doi: 10.3389/fevo.2022.993849
Received: 14 July 2022; Accepted: 22 August 2022;
Published: 07 September 2022.
Edited by:
He Yixin, Key Laboratory of Mountain Ecological Rehabilitation and Biological Resource Utilization, Chengdu Institute of Biology (CAS), ChinaReviewed by:
Qitao Xiao, Nanjing Institute of Geography and Limnology (CAS), ChinaGuan Bo, Ludong University, China
Copyright © 2022 Cui, Liu and Tong. This is an open-access article distributed under the terms of the Creative Commons Attribution License (CC BY). The use, distribution or reproduction in other forums is permitted, provided the original author(s) and the copyright owner(s) are credited and that the original publication in this journal is cited, in accordance with accepted academic practice. No use, distribution or reproduction is permitted which does not comply with these terms.
*Correspondence: Yan Liu, liuyan@iga.ac.cn