- 1School of Chemistry, University of New South Wales Sydney, Sydney, NSW, Australia
- 2Australian Centre for Astrobiology, University of New South Wales Sydney, Sydney, NSW, Australia
For the continuation and evolution of life, primitive membranes formed from prebiotically available components must have fulfilled certain essential requirements. Candidate amphiphiles, such as straight-chain fatty acids, that can self-assemble into cell-like structures have been shown to be capable of performing many life-like functions. However, obstacles that preclude a cohesive description of the evolution of modern-day cells from the origins of primitive membranes remain. Terpenoids are uniquely placed in terms of their derivation and chemical motifs to play an important role in primitive membranes, as they do in extant cells. Here, we discuss the principles behind primitive membrane formation and offer a biophysics perspective regarding the potential role of terpenoids in membrane function. By doing so, we identify opportunities in the realm of protocell research.
1 Introduction
Bilayer membranes perform essential functions that support cellular life as we know it. Despite drastic changes in Earth’s geochemistry since the emergence of life (Gözen et al., 2022; Saha et al., 2022), the principal roles of the lipid bilayer have been preserved: compartmentalizing and regulating the internal environment with respect to its surroundings and enabling stable propagation through growth and division (Wang and Szostak, 2019). In protocells, such roles must have been fulfilled from prebiotically available constituents (Gözen et al., 2022). Fatty acids (FAs) with straight alkyl chains, from which the hydrophobic moieties of modern phospholipids are derived, are the simplest molecules that can self-assemble into a protocellular bilayer membrane (Martin and Douliez, 2021). Indeed, model FA protocells have been shown to encapsulate genetic material (Chen et al., 2005), grow by incorporating new monomers from micelles (Chen and Szostak, 2004), and divide (Zhu and Szostak, 2009). However, unresolved challenges remain, such as robustness to pH changes (Lowe et al., 2022), compatibility with catalytic metal ions (Monnard et al., 2002), maintenance of ionic gradients, and stable propagation to daughter cells whilst retaining replicated genetic material (Xu et al., 2019). A targeted exploration of prebiotically available molecules that possess the required biophysical characteristics may help overcome these challenges.
Terpenoids, which are also known as isoprenoids, represent a broad class of molecules that are imperative to the functioning of extant cell membranes, either as the primary component (archaeal lipids) or as regulators through adjustments to membrane packing or localized partitioning (Hillier and Lathe, 2019; Řezanka et al., 2023). The biosynthesis of terpenoids requires isoprene units, from which a vast array of molecules are made through stages of chain elongation, structural modification and/or chemical functionalization (Hoshino and Villanueva, 2023), as depicted in Figure 1. In extant cells, isoprene monomer supply has its origins in glycolysis whilst the subsequent step-wise synthesis of terpenoids is catalyzed by a suite of enzymes, predominantly prenyl transferases (Liang et al., 2002; Chang et al., 2021). These pathways have distinct evolutionary origins, which is comprehensively covered in the review by Hoshino and Villanueva (Hoshino and Villanueva, 2023). In the context of protocells, prebiotic supply is necessary, which remains speculative (though for an overview of the progress thus far, we refer readers to the work by Nakatani et al., 2014). Hence, we focus on simpler analogues of extant membrane terpenoids, which, assuming their presence on prebiotic Earth (Jordan et al., 2019a), may have played an integral role in the origins of life.
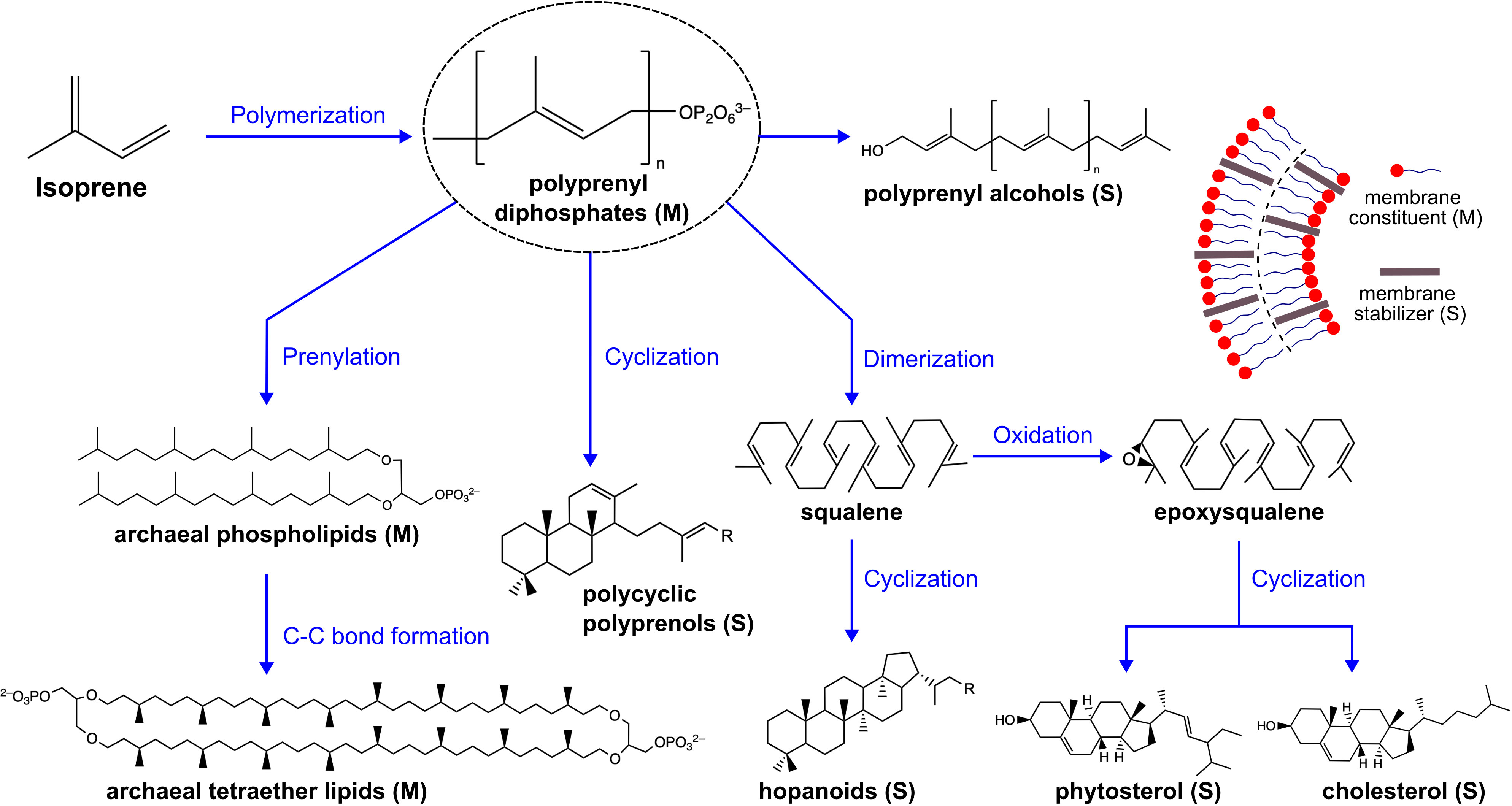
Figure 1 Terpenoids are derived from isoprene through sequential stages of chain elongation and structural modification, leading to a diverse array of molecules. Membrane functional terpenoids can act as primary membrane constituents or as membrane stabilizers that integrate into and alter membrane properties. The membrane terpenoids shown here are not exhaustive. M, membrane constituent and S, membrane stabilizer.
There are physical requirements that must have been fulfilled by early protocells without evolutionarily-advanced biochemistry, namely proteins. The structural motifs of membrane terpenoids provide functional diversity that could be useful in protocells. By taking a biophysical perspective, we seek to provide an overview of protocell formation and functionality that is underpinned by principles of self-assembly, and to identify the potential role of terpenoids in the field moving forward.
2 Principles of lipid bilayer membrane formation
Understanding why bilayers form is central to understanding primitive cell formation. Bilayer membranes are thermodynamically favored structures that can be formed by self-assembly of amphiphilic molecules, such as phospholipids or fatty acids (FAs) (Bergström, 2011). Depicted schematically in Figure 2, these molecules contain a hydrophilic “head-group” covalently bonded to one or two hydrophobic “tail-groups”. The main driver of self-assembly is the hydrophobic effect from the release of solvating water molecules around tail-groups as they aggregate together, thus increasing water entropy (Eastoe and Tabor, 2014). The hydrophilic nature of the head-group necessitates that it remain in aqueous contact. A complex suite of forces are involved in head-group interactions, such as, electrical double-layer, hydration, and steric forces (Israelachvili et al., 1980). Both tail-group and head-group interaction forces determine the likelihood of aggregation and the preferred aggregate type (Eastoe, 2005), which is crucial in the context of primitive cell formation.
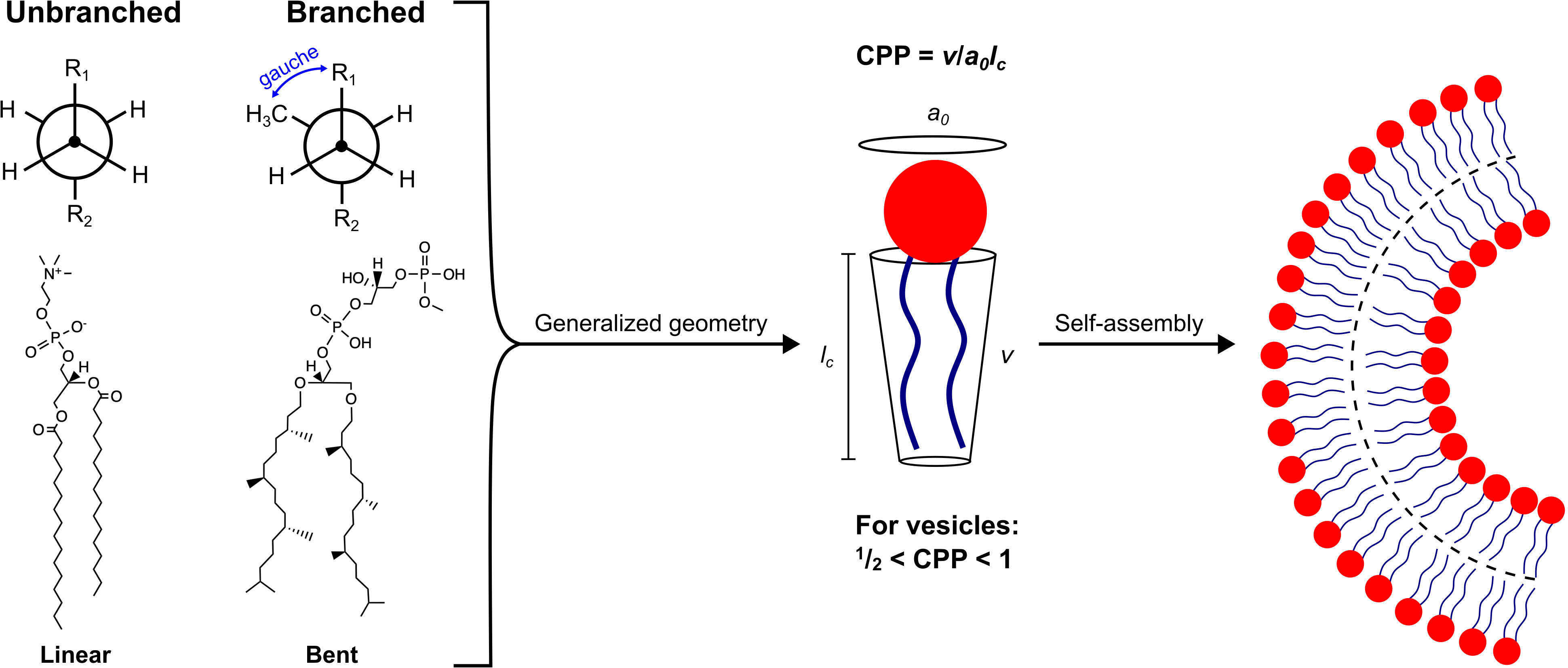
Figure 2 Lipid packing geometry for the formation of cell-like vesicles. Left: Structural comparison between a straight-chain, FA-derived phospholipid, 1,2-dipalmitoyl-sn-glycero-3-phosphocholine (DPPC), and a branched-chain, terpenoid-derived phospholipid, phosphatidyl glycerophosphate methyl ester (PCP-Me). DPPC adopts a linear conformation, whereas PGP-Me favors a bent conformation due to additional steric bulk from the methyl side-chains that greatly diminishes the energy barrier between gauche and anti conformers (Yamagami et al., 2019). Right: Both lipids can self-assemble into curved bilayers, which can be rationalized by considering their critical packing parameter, CPP.
Geometric properties of a given amphiphile can be summarized into parameters such as optimal area per head-group (a0), molecular volume (v), and chain length (lc). These parameters are used to define the critical packing parameter, CPP = v/a0lc(Israelachvili et al., 1980; Mitchell and Ninham, 1981). As shown in Figure 2, the formation of vesicles requires that , which restricts the type of molecule capable of forming cell-like structures through self-assembly. In this way, thermodynamics is invoked to demonstrate why aggregation is favored, whilst molecular geometry is used to rationalize the type of aggregate that is formed. Therefore, potential protocell constituents are constrained in terms of amphiphilicity and the preferred packing arrangement of their hydrophilic and hydrophobic moieties.
3 Dynamic requirements of primitive membranes
The fitness of any primitive compartment would have been tied to its ability to encapsulate replicating genetic material, and then propagate – processes that are governed by enzymes in modern biology. Primitive membranes may have been leakier than modern membranes to allow the import of genetic building blocks and nutrients, but not so permeable as to lose genetic polymers (Mansy, 2010). Fatty acids (FAs) are considered likely membrane components in protocells, however, they have major limitations due to their instability under high pH, high salinity or in the presence of certain metal cations. Recent work indicates that a mixture of FAs and terpenoids may help address these issues (Jordan et al., 2019a; Jordan et al., 2019b).
Growth of a membrane compartment requires lipid insertion into the bilayer, which necessitates moderate solubility of the lipid in aqueous solution. The extremely low solubility of modern phospholipids, for example, means that free lipids rapidly aggregate into de novo structures, rather than insert into pre-existing membranes. By contrast, FA vesicles have been shown to grow in the presence of supplied FA micelles, or other FA vesicles with a different composition. Lipid exchange thus not only enables membrane growth, but also competition between vesicles (Chen et al., 2004) and thus the evolution of membrane composition (Chen et al., 2004; Budin and Szostak, 2011). Consequently, protocell membrane lipids must have had higher solubility than modern lipids, which would also decrease the energy barriers required for membrane remodeling processes such as fission or compartment fusion. Terpenoid-based amphiphiles, such as polyprenyl phosphates or polyprenyl alcohols, are prebiotically plausible membrane components but are relatively underexplored in this context. Further study is required to determine whether vesicles consisting of amphiphilic polyprenyls can grow as FA-based vesicles do. Additionally, a membrane containing a mixture of FA and terpenoid-based amphiphiles may induce unique curvatures that favor division. As protocells evolved enzymes, the requirement of the membranes to be dynamic would have decreased dramatically.
4 Impact of methyl branching
Methyl branching is a common feature of membrane functional terpenoid molecules, as shown in Figure 1, and has been shown to enhance specific membrane properties, many of which are advantageous under extreme environmental conditions. For example, methyl branching is well known to fluidize the membrane by disrupting close packing between lipid tail-groups, leading to a stable fluid membrane phase across temperature ranges as wide as −120 °C to 120 °C (Lindsey et al., 1979). Increased membrane fluidity induced by methyl side-chains is supported by a computational study by Poger and coworkers wherein various branched lipids were incorporated into a lipid bilayer composed of 1,2-dipalmitoyl-sn-glycero-3-phosphocholine (DPPC) (Poger et al., 2014). As an example, inclusion of a DPPC derivative with branching in one tail-group at carbon position eight caused an increase in the area per lipid (APL) within the bilayer (from 0.623 to 0.668 nm2), a reduction in membrane thickness (from ∼3.59 nm to ∼3.39 nm), and a decrease in the order parameter (from ∼0.2 to ∼0.1 at the branch point) (Poger et al., 2014). An unsaturated tail-group can also fluidize the membrane, however, methyl branching has the benefit of being chemically stable under oxidative conditions (Bhattacharya, 2022). In addition to disrupting inter-chain packing, methyl branching also affects the preferred intra-chain conformation. The steric repulsion of methyl branches essentially removes the energy barrier between anti and gauche rotational isomers (see Figure 2). A “bent”-like structure establishes an opportunity for neighboring chains to intertwine. This property has been proposed to confer excellent stability to high temperatures and very saline conditions in archaea (Yamagami et al., 2019).
One curious feature of terpenoids is their ability to increase membrane fluidity and APL whilst reducing membrane permeability. A smaller APL usually corresponds to tighter lipid packing and reduced water permeation. Tristram-Nagle and coworkers used small-angle X-ray scattering and small-angle neutron scattering to probe the properties of 1,2-diphytanoyl-sn-glycero-3-phosphocholine (DPhyPC) membranes. Compared to straight-chained counterparts with similar water permeability, DPhyPC had at least 30% larger APL (Tristram-Nagle et al., 2010). In other words, DPhyPC had a similar water permeability as dimyristoyl-PC (saturated C14 chain), but a larger APL than dioleoyl-PC (unsaturated C18 chain). Similarly, membrane thickness is usually an approximate predictor for solute permeability because a thicker membrane usually corresponds to a larger energy barrier for defect formation, lipid flip-flop, and solute diffusion. However, branched-chain lipids form thinner bilayers (Kučerka et al., 2011; Poger et al., 2014) that are less permeable to salt (Yamauchi et al., 1992). Extant plant cells, for example, use phytosterols (structurally similar to cholesterol but with additional branching; both are shown in Figure 1) to support the maintenance of ionic gradients (Haines, 2001). Thus, properties that appear correlated in phospholipid systems are not necessarily correlated when there is branching in the lipids. The difference is attributed to fast intra-chain dynamics, but slow inter-chain dynamics and slow lateral diffusion in the hydrophobic core owing to methyl branches intertwining (Shinoda et al., 2003). In primitive membranes, a polyprenyl alcohol, such as farnesol, may have helped provide fluidity and maintenance of ionic gradients.
Methyl branching also impacts surface energies and the capacity to aggregate, both of which are critical to bilayer formation. Amphiphiles containing phytanyl chains appear more interfacially active than their straight-chained counterparts, whether double-chained (Kitano et al., 2003) or single-chained (Yamauchi et al., 1994). Straight-chained amphiphiles have suboptimal packing of the lipid tails at air–water interfaces, leading to limiting surface tensions of at least 35 mN/m (Yamauchi et al., 1997; Czajka et al., 2015). Adding methyl branches has been shown to decrease the surface energies of surfactants, approaching the limiting surface tension of a pure hydrocarbon–air interface, ∼25 mN/m (Czajka et al., 2015). This could be due to the fact that methyl groups have intrinsically lower surface energies than CH2 groups, and that branching can also increase the hydrophobic packing efficiency of surfactant monolayers (Czajka et al., 2015). Phytanyl surfactants also have higher critical micelle concentrations and thus higher solubility than their straight-chained counterparts (Arvidsson et al., 1971; Yamauchi et al., 1997), partly attributed to branching decreasing the total hydrophobic surface area. This enhanced solubility means terpenoids may be able to migrate more readily from one aggregate structure to another via the solution phase and participate in membrane growth.
Finally, terpenoids need not reside within the leaflets of lipids bilayers. Squalane (a saturated derivative of squalene) has been shown to reside in the hydrophobic midplane of lipid bilayers, whereby it prevents proton leakage (Hauß et al., 2002). The non-polar, branched nature of squalane means that residing completely within the hydrophobic membrane domain causes less disruption to the membrane leaflets.
5 The lipid divide
There is an ongoing debate regarding the origins of the lipid divide that led to the distinct structure of lipids in Archaea and Bacteria/Eukarya. Two key differences (head-group chirality and tail-group derivation), are briefly discussed.
Distinct head-group chirality exists across the domains of life – bacterial and eukaryotic lipids possess sn-glycerol-3-phosphate (G3P) backbone whereas archaeal lipids possess sn-glycerol-1-phosphate-type (G1P) backbone. The lack of an extant cell containing a heterochiral mixture of lipids (G3P and G1P) led to a theory that such a membrane is not viable, however, recent reports suggest otherwise. Shimada and Yamagishi made liposomes with a heterochiral mixture of lipids and found that certain combinations were stable to temperatures up to 120 °C with sufficient permeability to sustain life (Shimada and Yamagishi, 2011). Examples of viable bacteria with heterochiral membranes have also emerged. Caforio and coworkers used lipid engineering of Escherichia coli to produce a stable mixed-type membrane containing between 20–30% archaeal lipids that was more robust to temperature extremes than non-engineered forms of the bacteria (Caforio et al., 2018). Intriguingly, it has also been reported that the Fibrobacteres-Chlorobi-Bacteroidetes group of bacteria possess genes for both G3P and G1P-type lipids (Villanueva et al., 2021). It has even been theorized that ancestral cells had a mixture of archaeal and bacterial features (Jordan et al., 2019a; Řezanka et al., 2023).
The membrane lipids of Archaea and Bacteria/Eukarya also differ in their tail-groups: the former are terpenoid-derived, whereas the latter are fatty acid-derived. Although there are certain characteristics of terpenoid-based archaeal lipids that confer membrane stability in extreme temperature and salt conditions (as mentioned above), such features do not fully account for the modern lipid divide. The presence of archaea in moderate environmental conditions and bacteria in extreme conditions negates such an argument (Konings et al., 2002; Řezanka et al., 2023). Therefore, the basis of the lipid divide remains elusive and contested.
The curious origins of the homochirality that exists in modern day cells has been hypothesized to be evolutionarily driven by interactions with proteins (Sojo, 2019). Thus, the evolution of membrane proteins, and their functionality-dependent interactions with lipids, may have been a driver for the lipid divide. Homochiral lipid bilayers are enantioselectively permeable (Hu et al., 2021), and this may have also conferred an advantage if enantioselective enzymes are encapsulated. However, before the emergence of proteins or lipids with chirality, protocells with mixed membrane composition may not only have been viable, but could have had a selective advantage.
6 Potential significance of terpenoids in prebiotic membrane research
There are numerous potential research avenues to investigate the putative role of terpenoids early in life’s history. As discussed above, terpenoids can exhibit unique functional attributes in bilayer membranes. Here, we outline how these attributes could be applied to prebiotic membrane research.
Lipid bilayer membranes composed of single-tailed species are of interest in prebiotic membrane research because they are simpler than double-tailed lipids and thus more prebiotically plausible. Preliminary work shows that single-chained terpenoids may be able to form bilayers on their own (Ourisson and Nakatani, 1994; Yamauchi et al., 1994; Nakatani et al., 2014). There are also numerous examples of viable membranes comprising combinations of terpenoids and fatty acids (FAs). A mixed saturated FA/terpenoid membrane was demonstrated by Jordan and coworkers, who made 1:1 geraniol:decanoic acid mixed membranes (Jordan et al., 2019a) as well as membranes from 1:1:1 C10–C15 FA/1-alkanol/C10 isoprenoid mixtures (Jordan et al., 2019b). Lowe and coworkers used phytanyl tethers to form tethered bilayer membranes that were 10% isoprenoid and 90% unsaturated FA (Lowe et al., 2022). Squalane has also been shown to integrate into oleic acid vesicles (Morigaki and Walde, 2002) at a level that was not quantified, but significant enough to affect vesicle morphology. Thus, the next steps are to attempt growth and division experiments on membranes containing terpenoids, and to further characterize their permeability and underlying mechanical and biophysical properties.
Enhanced membrane fluidity at lower temperatures is an invaluable property that is as yet unavailable to non-terpenoid membranes. Decanoic acid, which is often used in protocell studies, has a melting temperature of over 30 °C as a solid, and just under 20 °C in membrane form in aqueous solution. Including C10–C15 terpenoid derivatives in the bilayer could ensure membrane fluidity and integrity are retained at cooler temperatures and during freeze–thaw cycles, which are known to help critical processes for RNA copying (Zhang et al., 2022) but also induce content loss (Litschel et al., 2018).
One feature of life that appears intrinsically challenging to recapitulate in membranes containing FAs is the maintenance of ion and proton gradients. In living cells, the energy from these gradients is usually converted into other forms of energy, making gradients core features of metabolism. While the insertion of free FAs into lipid bilayers has been used to generate proton gradients (Kamp and Hamilton, 1992; Chen and Szostak, 2004), the proton gradient can also be dissipated by the flip-flop of the same FAs: neutral FAs migrate from one lipid leaflet to the other, then release the proton to ionize (Zeng et al., 1998; Chen and Szostak, 2004). One strategy to prevent dissipation could be to use terpenoids that could potentially incorporate into the midplane of the bilayers, as squalane does (Hauß et al., 2002), to create an additional barrier against ion transport. Phytanic acid should be avoided, because it has been shown to enhance proton conductance more effectively than straight-chained FAs (Gutknecht, 1988). An alternative strategy for maintaining a proton gradient could involve working at lower temperatures to slow down FA flip-flop and membrane pore formation, whilst incorporating terpenoids to help retain lateral membrane fluidity.
In general, primitive cells may have needed to be able to withstand environmental changes, such as dramatic shifts in temperature or pH. For this purpose, stabilizer molecules may have performed a crucial role in primitive cells. In existing cells, cholesterol (a terpenoid-derived molecule) fulfills this requirement. Cholesterol can relieve bending stresses by flip-flopping across the bilayer (Bruckner et al., 2009) and increases orientational order in the liquid crystalline state, which provides greater mechanical strength (Ohvo-Rekilä, 2002). The intercalation of cholesterol in lipid bilayers is favored by local non-polar and polar interactions with lipids. There is some precedent in the literature that simpler terpenoids could have performed a similar function in primitive cells (Nakatani et al., 2014). The altered APL from incorporating terpenoids into a membrane could also affect the pKa of FA aggregates (Phoeung et al., 2010), and thus potentially promote bilayer stability across a broader range of pHs. Prior to the advent of homeostasis, the ability for primitive membranes to withstand some variation in environmental conditions would have been critical.
Ultimately, lipid bilayers must strike a balance between the competing requirements of having sufficient integrity as a barrier, and maintaining dynamic enough behavior to enable out-of-equilibrium processes essential to life. Condensing the packing of the lipid tails to improve integrity has intrinsic limits, because a gel or crystalline phase can no longer support processes such as cellular growth and division. Terpenoids appear to be a class of molecules that can integrate into or form bilayer membranes and maximize the packing of the hydrophobic components, but be sufficiently internally dynamic and structurally different from straight-chain lipids such that crystallization is inhibited. Exploring their prebiotic synthesis and ability to modify primitive membrane properties, with a focus on permeability, stability to environmental fluctuations, and fluidity, will be fruitful directions for further research.
Author contributions
JK: Conceptualization, Project administration, Writing – original draft, Writing – review & editing, Visualization. AW: Conceptualization, Funding acquisition, Project administration, Writing – original draft, Writing – review & editing.
Funding
The author(s) declare financial support was received for the research, authorship, and/or publication of this article. AW acknowledges support from the Australian Research Council (DE210100291) and the Human Frontier Science Program (RGP0029/2020 to AW).
Acknowledgments
The authors thank Dr Albert Fahrenbach and Lauren Lowe for discussions.
Conflict of interest
The authors declare that the research was conducted in the absence of any commercial or financial relationships that could be construed as a potential conflict of interest.
Publisher’s note
All claims expressed in this article are solely those of the authors and do not necessarily represent those of their affiliated organizations, or those of the publisher, the editors and the reviewers. Any product that may be evaluated in this article, or claim that may be made by its manufacturer, is not guaranteed or endorsed by the publisher.
References
Arvidsson E. O., Green F. A., Laurell S. (1971). Branching and hydrophobic bonding. J. Biol. Chem. 246, 5373–5379. doi: 10.1016/S0021-9258(18)61917-9
Bergström L. M. (2011). “Thermodynamics of self-assembly,” in Application of thermodynamics to biological and materials science. Ed. M. Tadashi (Croatia: InTechOpen), 289–315. doi: 10.5772/13711
Bhattacharya A. (2022). Self-assembly and biophysical properties of archaeal lipids. Emerging Topics Life Sci. 6, 571–582. doi: 10.1042/ETLS20220062
Bruckner R. J., Mansy S. S., Ricardo A., Mahadevan L., Szostak J. W. (2009). Flip-flop-induced relaxation of bending energy: implications for membrane remodeling. Biophys. J. 97, 3113–3122. doi: 10.1016/j.bpj.2009.09.025
Budin I., Szostak J. W. (2011). Physical effects underlying the transition from primitive to modern cell membranes. Proc. Natl. Acad. Sci. 108, 5249–5254. doi: 10.1073/pnas.1100498108
Caforio A., Siliakus M. F., Exterkate M., Jain S., Jumde V. R., Andringa R. L. H., et al. (2018). Converting Escherichia coli into an archaebacterium with a hybrid heterochiral membrane. Proc. Natl. Acad. Sci. 115, 3704–3709. doi: 10.1073/pnas.1721604115
Chang H.-Y., Cheng T.-H., Wang A. H.-J. (2021). Structure, catalysis, and inhibition mechanism of prenyltransferase. IUBMB Life 73, 40–63. doi: 10.1002/iub.2418
Chen I. A., Roberts R. W., Szostak J. W. (2004). The emergence of competition between model protocells. Science 305, 1474–1476. doi: 10.1126/science.1100757
Chen I. A., Salehi-Ashtiani K., Szostak J. W. (2005). RNA catalysis in model protocell vesicles. J. Am. Chem. Soc. 127, 13213–13219. doi: 10.1021/ja051784p
Chen I. A., Szostak J. W. (2004). Membrane growth can generate a transmembrane pH gradient in fatty acid vesicles. Proc. Natl. Acad. Sci. U.S.A. 101, 7965–7970. doi: 10.1073/pnas.0308045101
Czajka A., Hazell G., Eastoe J. (2015). Surfactants at the design limit. Langmuir 31, 8205–8217. doi: 10.1021/acs.langmuir.5b00336
Eastoe J. (2005). “Surfactant aggregation and adsorption at interfaces,” in Colloid science. Ed. Cosgrove T. (Oxford, UK: Blackwell Publishing Ltd), 50–76. doi: 10.1002/9781444305395.ch4
Eastoe J., Tabor R. F. (2014). “Surfactants and nanoscience,” in Colloidal foundations of nanoscience (Amsterdam, Netherlands: Elsevier), 135–157. doi: 10.1016/B978-0-444-59541-6.00006-0
Gözen I., Köksal E. S., Põldsalu I., Xue L., Spustova K., Pedrueza-Villalmanzo E., et al. (2022). Protocells: milestones and recent advances. Small 18, 2106624. doi: 10.1002/smll.202106624
Gutknecht J. (1988). Proton conductance caused by long-chain fatty acids in phospholipid bilayer membranes. J. Membrane Biol. 106, 83–93. doi: 10.1007/BF01871769
Haines T. H. (2001). Do sterols reduce proton and sodium leaks through lipid bilayers? Prog. Lipid Res. 40, 299–324. doi: 10.1016/S0163-7827(01)00009-1
Hauß T., Dante S., Dencher N. A., Haines T. H. (2002). Squalane is in the midplane of the lipid bilayer: Implications for its function as a proton permeability barrier. Biochim. Biophys. Acta (BBA) - Bioenergetics 1556, 149–154. doi: 10.1016/S0005-2728(02)00346-8
Hillier S. G., Lathe R. (2019). Terpenes, hormones and life: Isoprene rule revisited. J. Endocrinol. 242, R9–R22. doi: 10.1530/JOE-19-0084
Hoshino Y., Villanueva L. (2023). Four billion years of microbial terpenome evolution. FEMS Microbiol. Rev. 47, fuad008. doi: 10.1093/femsre/fuad008
Hu J., Cochrane W. G., Jones A. X., Blackmond D. G., Paegel B. M. (2021). Chiral lipid bilayers are enantioselectively permeable. Nat. Chem. 13, 786–791. doi: 10.1038/s41557-021-00708-z
Israelachvili J. N., Marčelja S., Horn R. G. (1980). Physical principles of membrane organization. Q. Rev. Biophysics 13, 121–200. doi: 10.1017/S0033583500001645
Jordan S. F., Nee E., Lane N. (2019a). Isoprenoids enhance the stability of fatty acid membranes at the emergence of life potentially leading to an early lipid divide. Interface Focus 9, 20190067. doi: 10.1098/rsfs.2019.0067
Jordan S. F., Rammu H., Zheludev I. N., Hartley A. M., Maréchal A., Lane N. (2019b). Promotion of protocell self-assembly from mixed amphiphiles at the origin of life. Nat. Ecol. Evol. 3, 1705–1714. doi: 10.1038/s41559-019-1015-y
Kamp F., Hamilton J. A. (1992). pH gradients across phospholipid membranes caused by fast flip-flop of un-ionized fatty acids. Proc. Natl. Acad. Sci. 89, 11367–11370. doi: 10.1073/pnas.89.23.11367
Kitano T., Onoue T., Yamauchi K. (2003). Archaeal lipids forming a low energy-surface on air-water interface. Chem. Phys. Lipids 126, 225–232. doi: 10.1016/j.chemphyslip.2003.08.006
Konings W. N., Albers S.-V., Koning S., Driessen A. J. M. (2002). The cell membrane plays a crucial role in survival of bacteria and archaea in extreme environments. Antonie Van Leeuwenhoek 81, 61–72. doi: 10.1023/a:1020573408652
Kučerka N., Nieh M.-P., Katsaras J. (2011). Fluid phase lipid areas and bilayer thicknesses of commonly used phosphatidylcholines as a function of temperature. Biochim. Biophys. Acta (BBA) - Biomembranes 1808, 2761–2771. doi: 10.1016/j.bbamem.2011.07.022
Liang P.-H., Ko T.-P., Wang A. H.-J. (2002). Structure, mechanism and function of prenyltransferases. Eur. J. Biochem. 269, 3339–3354. doi: 10.1046/j.1432-1033.2002.03014.x
Lindsey H., Petersen N. O., Chan S. I. (1979). Physicochemical characterization of 1,2-diphytanoylsn-glycero-3-phosphocholine in model membrane systems. Biochim. Biophys. Acta (BBA) Biomembranes 555, 147–167. doi: 10.1016/0005-2736(79)90079-8
Litschel T., Ganzinger K. A., Movinkel T., Heymann M., Robinson T., Mutschler H., et al. (2018). Freeze-thaw cycles induce content exchange between cell-sized lipid vesicles. New J. Phys. 20, 055008. doi: 10.1088/1367-2630/aabb96
Lowe L. A., Kindt J. T., Cranfield C., Cornell B., Macmillan A., Wang A. (2022). Subtle changes in pH affect the packing and robustness of fatty acid bilayers. Soft Matter 18, 3498–3504. doi: 10.1039/D2SM00272H
Mansy S. S. (2010). Membrane transport in primitive cells. Cold Spring Harbor Perspect. Biol. 2, a002188. doi: 10.1101/cshperspect.a002188
Martin N., Douliez J.-P. (2021). Fatty acid vesicles and coacervates as model prebiotic protocells. ChemSystemsChem 3, 1–12. doi: 10.1002/syst.202100024
Mitchell D. J., Ninham B. W. (1981). Micelles, vesicles and microemulsions. J. Chem. Society Faraday Trans. 2 77, 601. doi: 10.1039/f29817700601
Monnard P.-A., Apel C. L., Kanavarioti A., Deamer D. W. (2002). Influence of ionic inorganic solutes on self-assembly and polymerization processes related to early forms of life: implications for a prebiotic aqueous medium. Astrobiology 2, 139–152. doi: 10.1089/15311070260192237
Morigaki K., Walde P. (2002). Giant vesicle formation from oleic acid/sodium oleate on glass surfaces induced by adsorbed hydrocarbon molecules. Langmuir 18, 10509–10511. doi: 10.1021/la026579r
Nakatani Y., Ribeiro N., Streiff S., Gotoh M., Pozzi G., Désaubry L., et al. (2014). Search for the most “primitive” membranes and their reinforcers: a review of the polyprenyl phosphates theory. Origins Life Evol. Biospheres 44, 197–208. doi: 10.1007/s11084-014-9365-6
Ohvo-Rekilä H. (2002). Cholesterol interactions with phospholipids in membranes. Prog. Lipid Res. 41, 66–97. doi: 10.1016/S0163-7827(01)00020-0
Ourisson G., Nakatani Y. (1994). The terpenoid theory of the origin of cellular life: The evolution of terpenoids to cholesterol. Chem. Biol. 1, 11–23. doi: 10.1016/1074-5521(94)90036-1
Phoeung T., Aubron P., Rydzek G., Lafleur M. (2010). pH-triggered release from nonphospholipid LUVs modulated by the pKa of the included fatty acid. Langmuir 26, 12769–12776. doi: 10.1021/la1014829
Poger D., Caron B., Mark A. E. (2014). Effect of methyl-branched fatty acids on the structure of lipid bilayers. J. Phys. Chem. B 118, 13838–13848. doi: 10.1021/jp503910r
Řezanka T., Kyselová L., Murphy D. J. (2023). Archaeal lipids. Prog. Lipid Res. 91, 101237. doi: 10.1016/j.plipres.2023.101237
Saha A., Yi R., Fahrenbach A. C., Wang A., Jia T. Z. (2022). A physicochemical consideration of prebiotic microenvironments for self-assembly and prebiotic chemistry. Life 12, 1595. doi: 10.3390/life12101595
Shimada H., Yamagishi A. (2011). Stability of Heterochiral Hybrid Membrane Made of Bacterial sn -G3P Lipids and Archaeal sn -G1P Lipids. Biochemistry 50, 4114–4120. doi: 10.1021/bi200172d
Shinoda W., Mikami M., Baba T., Hato M. (2003). Molecular dynamics study on the effect of chain branching on the physical properties of lipid bilayers: structural stability. J. Phys. Chem. B 107, 14030–14035. doi: 10.1021/jp035493j
Sojo V. (2019). Why the lipid divide? Membrane proteins as drivers of the split between the lipids of the three domains of life. BioEssays 41, 1800251. doi: 10.1002/bies.201800251
Tristram-Nagle S., Kim D. J., Akhunzada N., Kučerka N., Mathai J. C., Katsaras J., et al. (2010). Structure and water permeability of fully hydrated diphytanoylPC. Chem. Phys. Lipids 163, 630–637. doi: 10.1016/j.chemphyslip.2010.04.011
Villanueva L., Von Meijenfeldt F. A. B., Westbye A. B., Yadav S., Hopmans E. C., Dutilh B. E., et al. (2021). Bridging the membrane lipid divide: Bacteria of the FCB group superphylum have the potential to synthesize archaeal ether lipids. ISME J. 15, 168–182. doi: 10.1038/s41396-020-00772-2
Wang A., Szostak J. W. (2019). Lipid constituents of model protocell membranes. Emerging Topics Life Sci. 3, 537–542. doi: 10.1042/ETLS20190021
Xu B.-Y., Xu J., Yomo T. (2019). A protocell with fusion and division. Biochem. Soc. Trans. 47, 1909–1919. doi: 10.1042/BST20190576
Yamagami M., Tsuchikawa H., Cui J., Umegawa Y., Miyazaki Y., Seo S., et al. (2019). Average conformation of branched chain lipid PGP-me that accounts for the thermal stability and high-salinity resistance of archaeal membranes. Biochemistry 58, 3869–3879. doi: 10.1021/acs.biochem.9b00469
Yamauchi K., Doi K., Kinoshita M., Kii F., Fukuda H. (1992). Archaebacterial lipid models: Highly salt-tolerant membranes from 1,2-diphytanylglycero-3-phosphocholine. Biochim. Biophys. Acta (BBA) - Biomembranes 1110, 171–177. doi: 10.1016/0005-2736(92)90355-P
Yamauchi K., Onoue Y., Tsujimoto T., Kinoshita M. (1997). Archaebacterial lipids: High surface activity of polyisoprenoid surfactants in water. Colloids Surfaces B: Biointerfaces 10, 35–39. doi: 10.1016/S0927-7765(97)00040-4
Yamauchi K., Yoshida Y., Moriya T., Togawa K., Kinoshita M. (1994). Archaebacterial lipid models: Formation of stable vesicles from single isoprenoid chain-amphiphiles. Biochim. Biophys. Acta (BBA) - Biomembranes 1193, 41–47. doi: 10.1016/0005-2736(94)90330-1
Zeng Y., Han X., Schlesinger P., Gross R. W. (1998). Nonesterified fatty acids induce transmembrane monovalent cation flux: host-guest interactions as determinants of fatty acid-induced ion transport. Biochemistry 37, 9497–9508. doi: 10.1021/bi980303u
Zhang S. J., Duzdevich D., Ding D., Szostak J. W. (2022). Freeze-thaw cycles enable a prebiotically plausible and continuous pathway from nucleotide activation to nonenzymatic RNA copying. Proc. Natl. Acad. Sci. 119, e2116429119. doi: 10.1073/pnas.2116429119
Keywords: terpenoids, primitive membranes, protocells, origins of life, self-assembly, biophysics
Citation: King JP and Wang A (2023) Putative roles of terpenoids in primitive membranes. Front. Ecol. Evol. 11:1272163. doi: 10.3389/fevo.2023.1272163
Received: 03 August 2023; Accepted: 30 October 2023;
Published: 22 November 2023.
Edited by:
Yosuke Hoshino, GFZ German Research Centre for Geosciences, GermanyReviewed by:
Ahanjit Bhattacharya, Stanford University, United StatesNemanja Cvjetan, University of Alberta, Canada
Copyright © 2023 King and Wang. This is an open-access article distributed under the terms of the Creative Commons Attribution License (CC BY). The use, distribution or reproduction in other forums is permitted, provided the original author(s) and the copyright owner(s) are credited and that the original publication in this journal is cited, in accordance with accepted academic practice. No use, distribution or reproduction is permitted which does not comply with these terms.
*Correspondence: Anna Wang, YW5uYS53YW5nQHVuc3cuZWR1LmF1; Joshua P. King, am9zaHVhLmtpbmcxQHVuc3cuZWR1LmF1