A three-step approach for co-locating nature-based solutions within offshore wind farms
- 1Resilient Coasts Ltd, Plymouth, United Kingdom
- 2College of Life and Environmental Sciences, University of Exeter, Exeter, United Kingdom
The extent of seabed licensed for offshore renewables is being expanded with the global requirement to reduce carbon emissions. The opportunity for Nature-based Solutions for restoration, conservation, mariculture, infrastructure protection, and carbon sequestration initiatives are being explored internationally. Co-location of marine renewable or structures with conservation initiatives offers the opportunity to support populations of threatened species and contribute to wider ecosystem services and benefits. Building on experience from a North Sea project, we explore the feasibility to co-locate bivalve species at offshore wind farms. We present a three-step approach to identify offshore wind farm sites with the potential to co-locate with compatible species within a marine licensed area, based on environmental and physical conditions and biological tolerances. These steps are, (1) information collection and data synthesis, (2) data analysis through site suitability and species compatibility assessments, and (3) numerical modelling approaches to test the feasibility of pilot studies and scale-up planned operations. This approach supports feasibility assessment by identification of sites where Nature-based Solution project success is more likely or certain, thereby reducing project costs and risk of failure. An example case study is provided using Gunfleet Sands offshore wind farm (southeast England) and the restoration and conservation of the commercially valuable European Flat Oyster (Ostrea edulis).
1. Introduction
The call to action to slow global warming is being communicated internationally (IPCC, 2019) and, as a result, the renewable energy sector is being expanded (Rodrigues et al., 2015; Quitzow et al., 2019). The European Commission (2020) has estimated that offshore wind power needs to contribute 240–450 GW of energy by 2050 to keep the global temperature increase below 1.5°C. Europe presently dominates the offshore windfarm (OWF) market, holding 84% of global installations (deCastro et al., 2019). The UK has the largest installed capacity of offshore wind in the world, with a commitment to generate up to 30GW of energy from arrays by 2030 (BEIS, 2020).
OWFs are considered by a variety of organizations (i.e., Blue Marine Foundation Plc., World Wildlife Fund, The Nature Conservancy, The Wildlife Trusts in the UK) to be ideal areas to co-locate Nature-based Solutions (NbS) for marine habitat restoration and generate associated co-benefits (Robertson et al., 2020; Bennun et al., 2021; The Nature Conservancy and Inspire Environmental, 2021; WWF European Policy Office, 2021). Under current licensing, OWF areas are mostly closed to large-scale commercial fishing activities, thereby restricting bottom trawling and dredging practices known to negatively impact benthic habitats and reduce biodiversity (Eigaard et al., 2015; Ashley et al., 2018; Fragkopoulou et al., 2021; Wang et al., 2021), which could inhibit restoration or enhancement efforts. In addition, the materials used to construct, stabilize and protect wind turbines can be designed in a way that optimizes growth of marine habitats and species populations (Lengkeek et al., 2017; Coolen et al., 2019). The shelter and protection the renewable activities and turbine structures offer may also provide areas for mariculture initiatives (Christie et al., 2014). The development of scour protection could include artificial reef structures or fish aggregation devices, which have the potential for aggregating fish or increasing abundance (Rendle and Rodwell, 2014).
Improving the sustainability credentials of offshore wind by opening the door to NbS initiatives are of considerable interest to operators. Utilizing licensed sites for additional activities that will enhance or restore local marine life (Wilson and Elliott, 2009; Ashley et al., 2014; Gimpel et al., 2015), develop sustainable aquaculture and/or for carbon sequestration (Buck et al., 2017) are being investigated worldwide. Co-location of NbS within OWFs has benefits which parallel the increase in competition for marine space (Christie et al., 2014), among ecosystem services and benefits. Co-location offers the opportunity to boost threatened populations, provision of food, materials or cultural services which have ecological, economic or social value (e.g., seaweed, coral or shellfish, and further detailed later). The expansion and development of existing and new OWFs provides the opportunity to explore co-location with conservation and restoration initiatives that provide environmental, economic, and societal improvements.
The growth in OWF licensed areas offers the opportunity to embed NbS for ecosystem engineering and habitat or species recovery within the planning and expansion programs, maximizing the dual environmental benefits of indirect reduction of CO2 emissions and direct enhancement of marine ecosystem services of these sites. While there are various clear advantages of co-location of OWFs with conservation initiatives, challenges must be considered, such as the potential for increased risk, responsibilities and costs for OWF developers (Christie et al., 2014). Other considerations include; the risk of contamination of aquaculture from OWF generated pollution, the increased risk of biofouling for the OWF, interruptions of operations and maintenance for the OWF, and a requirement for a specialized work force in NbS and/or mariculture (van den Burg et al., 2016).
Bivalve species, such as mussels (Mytilus spp. and Modiolus spp.) and oysters (Ostrea sp. and Crassostrea sp.), are considered ecosystem engineers due to their formation of biogenic reefs that contribute substantially to inshore biodiversity and provide protection and nursery grounds for juvenile fish and other species (Coen et al., 2007; zu Ermgassen et al., 2020). They can also play an important socio-economic role in local coastal communities as wild harvested or cultured fisheries (Smaal et al., 2019). Evidence of widespread decline of these bivalve species (Beck et al., 2011; Fariñas-Franco et al., 2018; Helmer et al., 2019; Pogoda, 2019) has motivated restoration projects (McLeod et al., 2019). Over the past 5 years, pilot projects testing reef restoration and caged species introduction have been developed at OWFs in the Dutch North Sea (Kamermans, 2018), with considerable success in reef creation and survival rates (Didderen et al., 2018, 2020).
Although NbS projects offer an attractive proposition in terms of mariculture or habitat restoration, challenges remain regarding OWF operation and maintenance. The risks to, and success in, NbS projects are complex and site and/or species specific, thus an intervention using NbS must be transdisciplinary in concept design. OWFs are typically built in highly exposed regions, advantageous for the energy they harness, but often sub-optimal for restoration activities. Sites vary in physical processes and climate; the sediment transport and energy exposure levels differ vastly between sites. Operation and maintenance procedures differ from site to site, however common themes include maintaining a well-controlled and predictable maritime space, prioritization of OWF infrastructure risk and security, and avoidance of incidental vessel collision. Planning of NbS must be designed around the physical parameters of the structures and the operation and maintenance activities at individual OWFs.
The North Sea Agreement outlines co-location of sustainable projects within OWFs, which includes activities such as mariculture and seaweed farming in Netherlands (Noordzeeloket, 2020; OFL, 2020; Vincent et al., 2020). Seaweed cultivation is currently being trialed in the “Norther OWF” in the North Sea, Belgium, where 2 ha will be installed (AtSeaNova, 2020; Durakovic, 2020). However, food safety standards, operational risk governance, cost to produce, and ecological benefits related knowledge gaps remain to be explored at these pilot project sites (van den Burg et al., 2016, 2020; Jansen et al., 2018; Banach et al., 2020). Internationally, where conflict for maritime space exists, interest is growing for OWFs to coexist with commercial fisheries and marine protected areas (Lacroix and Pioch, 2011; Kyriazi et al., 2015, 2016; Schupp et al., 2019). Ecologically appropriate and sustainable species should be selected that are native in origin, sensitive to the surrounding areas, and ideally be included in the habitats risk assessment review within the OWF environmental statement (EU Habitat’s Directive, 1992).
Biological, chemical and physical environmental conditions will influence the success of NbS projects, which will result in financial loss or reputational damage if an initiative is attempted at an unsuitable site. Benassai et al. (2014) and Di Tullio et al. (2018) introduce a sustainability index of co-location, utilizing remote-sensing data to evaluate physical and biological parameters, in order to identify suitable sites for OWF and mussel farm co-location. These studies demonstrate the importance of considering both physical (wind velocity, depth and sea surface temperature anomalies) and biological (chlorophyll-a and particle organic carbon concentration) parameters in identifying suitable sites for co-location, stating that their methodology would require further biological information, and use of numerical modelling, to further improve understanding of site-species compatibility.
To improve wider understanding of the capacity or suitability of an OWF licensed area to co-locate, or be retrofitted, for species restoration efforts or mariculture initiatives, we present a three-step approach. This approach uses site specific physical environmental conditions and species biological tolerances to conduct suitability and compatibility assessments. Subsequently, numerical modelling is conducted to better estimate the viability of sites found to be compatible for a specific species, and which offer evidence in support for implementing pilot project trials. This stepwise approach increases likelihood of project success, ensuring incompatible sites or species are identified, reducing the risk of project failure via economic and biological loss. A case study is presented utilizing the three-step approach, applied to a sample of existing UK OWF sites and then testing the feasibility and viability to use a selected OWF in the restoration of a nearby designated marine conservation zone. This approach can be used for assessing NbS co-location compatibility at other marine renewable sites or coastal structural assets. This approach is proposed for practitioners to follow in reviewing asset-species compatibility in NbS project design or scaling-up NbS interventions.
2. Three-step approach to evidence the co-location of species and OWFs
The presented three-step approach (Figure 1) provides a pragmatic decision-making tool to advise whether a specific species could survive and reproduce successfully, given the environmental characteristics of an OWF site. The resulting evidence informs the feasibility phase of the design process of NbS for habitat enhancement, reducing the risk of loss during pilot studies or trial and error approaches. The evidence-based approach has parallel components (two grey boxes) for site identification and species selection to inform pilot design and installation, through Step 1 data and information synthesis (blue), Step 2 data analysis (yellow), and Step 3 data modelling (green), which are further described in the following sub-sections. The three-step approach detailed within this section is applied to a case study in section 3.
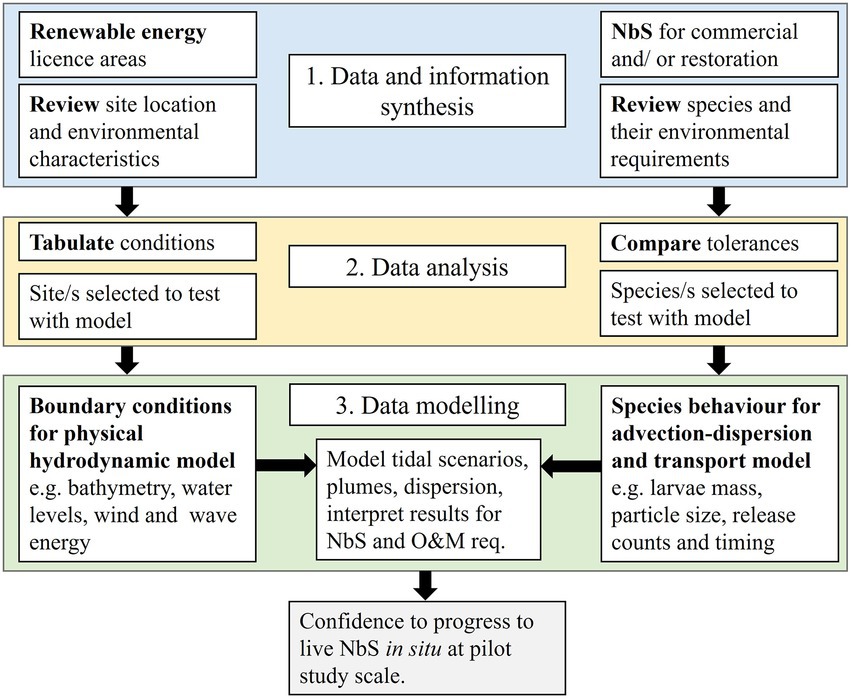
Figure 1. Flow diagram of the three-step approach to an evidence-based decision on co-location of species by retrofitting NbS into renewable energy licensed areas (arrows leading to examples from the case study example provided herein; Ørsted operated OWF and Ostrea edulis).
2.1. Step 1: Data and information synthesis
Firstly, physical and environmental characteristics of candidate OWF sites and the biophysical tolerance ranges of bivalve species of interest are obtained. Information and data to describe the environmental characteristics of OWF sites (see Case Study; Tables 1, 2) include; distance offshore, water depths, tidal range, wave descriptors, current velocity, turbidity, sediment composition and morphology, suspended sediment or particulate matter, sea surface temperature, chlorophyll a (chl a) concentration, salinity, and oxygen concentrations. Required data can be collated from a range of sources, including: OWF site specific technical reports, e.g., risk and mitigation, or design and tolerance documents; OWF environmental impact assessment reports; local authority reports on coastlines, fisheries and critical infrastructure vulnerability; and literature on local environment and species.
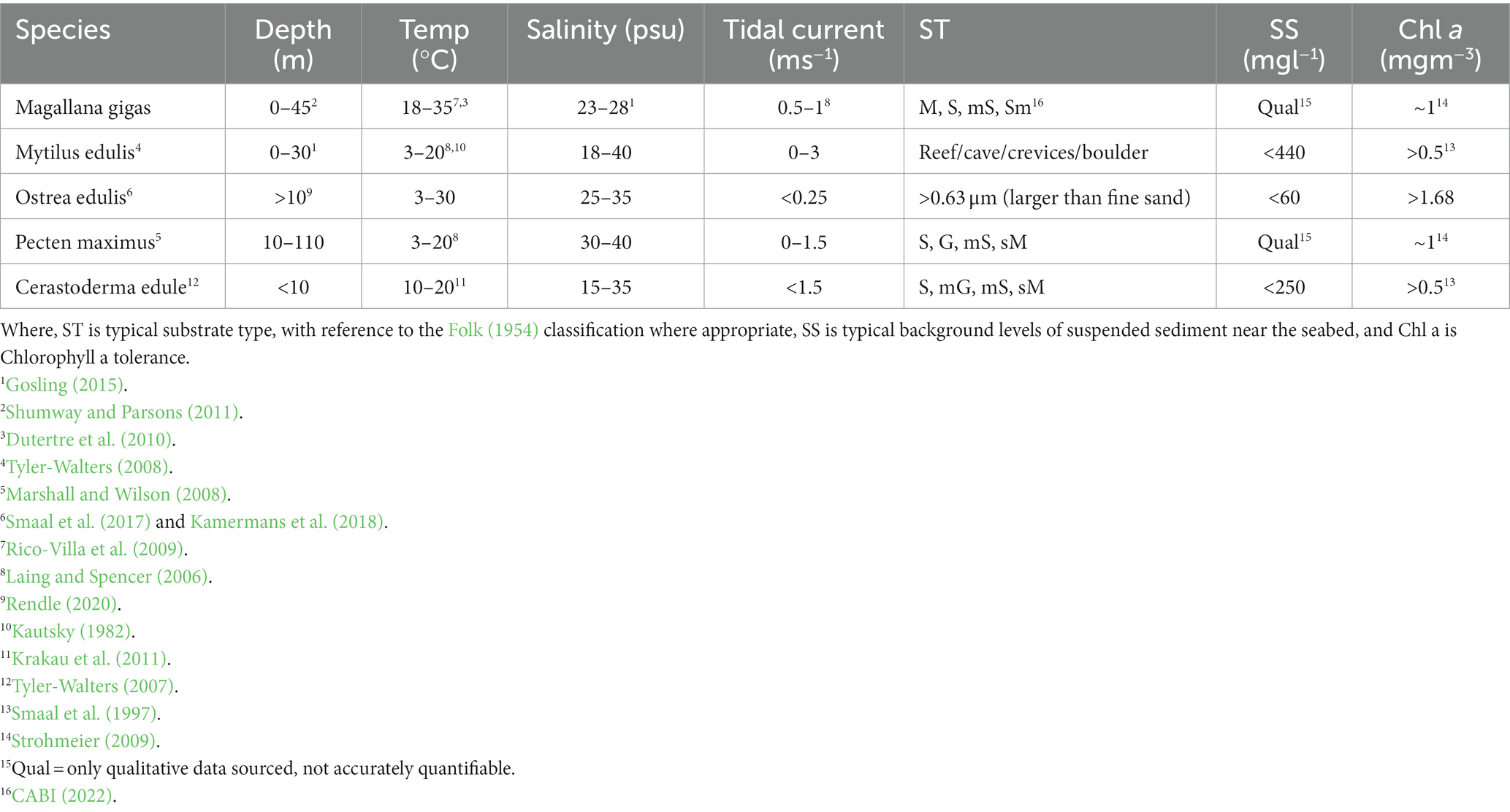
Table 2. Environmental tolerances of five bivalve mollusc species of ecological and commercial significance in Europe: Pacific oyster (Magallana gigas, previously Crassostrea gigas), Blue mussel (Mytilus edulis), European flat oyster (Ostrea edulis), the Great scallop (Pecten maximus), and the Common cockle (Cerastoderma edule).
Projects assessing the marine areas licensed for OWF development or the existing OWFs licensed to expand have benefitted from recent technological advancements. Improvements include survey equipment, techniques and regimes, remote sensing, satellite information, data analysis and numerical modelling capability providing ever more accurate representation of the marine areas. The OWF environmental assessments and design reports which hold these data may be made public or are typically available on request through the regulator (e.g., The Crown Estate, 2020) or license area operator unless commercially sensitive. Other UK repositories include Cefas (2021), DEFRA (2021), JNCC (2021), Natural England (2021), and MEDIN (2021). A technical expert or marine consultant will help bridge any gaps where data is lacking to provide an estimate, representative or indicative value range for a given parameter, or these data maybe found within an environmental report from a nearby location.
The species environmental tolerance ranges are equivalent or proxy to the aforementioned OWF characteristics. It is recommended to collate and tabulate these data to enable easy comparison and analysis (see Case Study; Tables 1, 2). Generating a list of species of interest to review will generate criteria to describe their natural or native distribution, any conservation concerns, or potential for commercial mariculture and/or carbon sequestration. Whilst not all species will have an entire spectrum of information published, much of this information is available in the literature, particularly for species providing ecosystem services and co-benefits. In some instances, large databases can be found for numerous species or tolerance ranges (Brennan et al., 2016; Bennett et al., 2018). An extensive literature review is required to collate data regarding specific species, typically dispersed in multiple articles.
Defining the end goal of the NbS will enable the appropriate species to be selected for comparison, prompting the questions: What are the aims and goals of the installation? What are the measurable success indicators? In commercial mariculture projects, a monoculture approach with wider socio-economic benefits for coastal communities might be considered, therefore understanding the route to market is paramount (Soto, 2009; Smaal et al., 2019). Where operations remain at sea, NbS activities have a lesser reliance on the coastal community, however transport, route to market and the value chain remain critical. A variety of species could be developed as an integrated multi-trophic aquaculture initiative, where polyculture efforts help to mitigate impacts of farming one species (Kremen and Miles, 2012), much like the principles of permaculture on land (Holmgren, 2002; Mcmanus, 2010). Producing an overview of ecosystem services for the species of interest would also support the next step and decision making.
These data collated and then tabulated within Step 1 (see Case Study; Tables 1, 2) make up the baseline evidence for each OWF site, enabling Step 2 comparisons to be carried out against specific species of interest.
2.2. Step 2: Data analysis – Site suitability and species compatibility
Having collated the desired information within Step 1, the next step involves an assessment of the suitability of the OWFs for restoration, enhancement, or mariculture initiatives. This can be conducted with respect to a singular OWF site of interest to determine compatibility, or alternatively a matrix may be developed to enable comparison at numerous sites and selection of the most suitable site/s (see Case Study; Table 3).
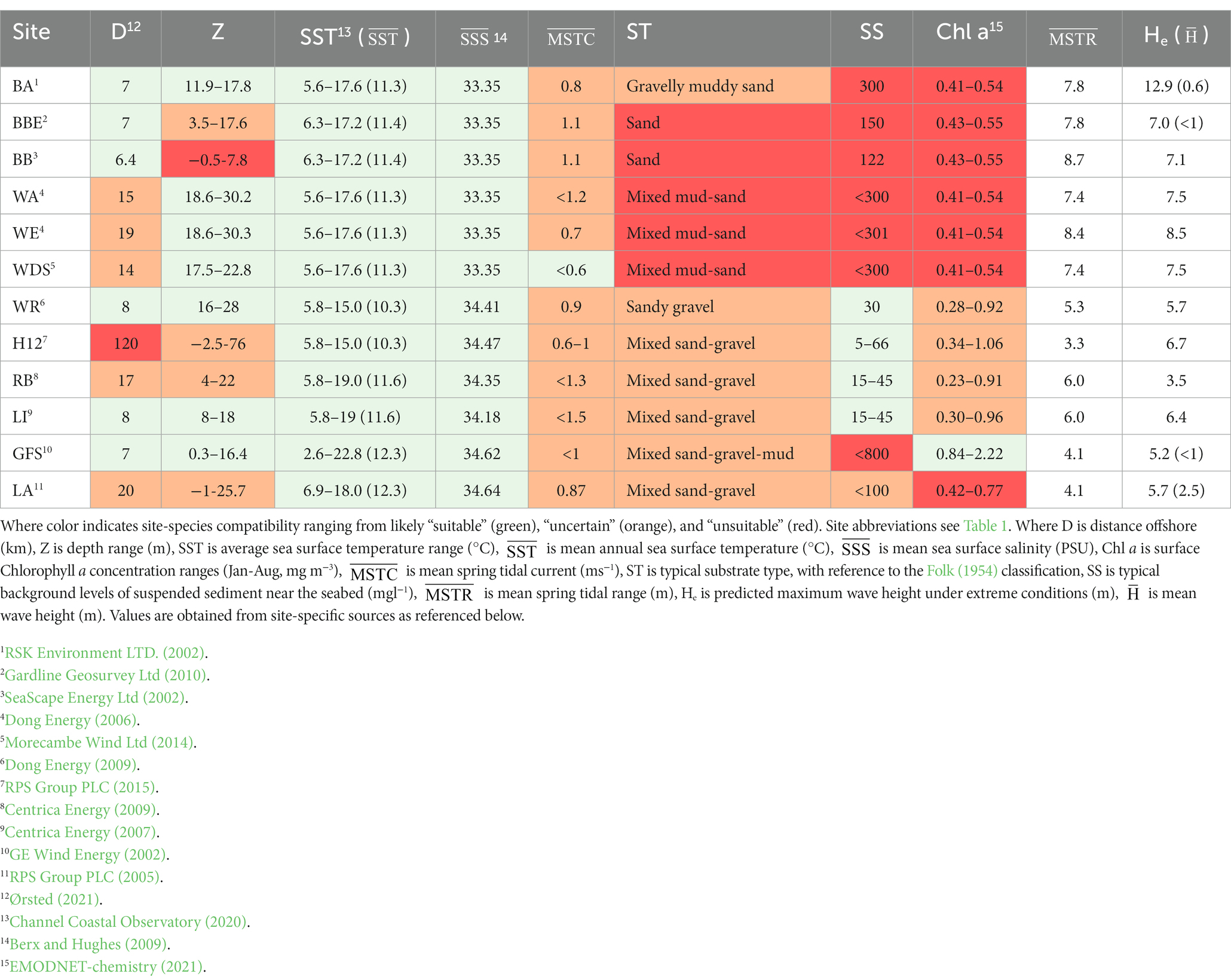
Table 3. Matrix of physical and environmental characteristics of Ørsted’s UK windfarms comparison of physical characteristics with species (Ostrea edulis) tolerances.
Initially, sites should be identified with regards to physical practicalities. Subsequently, a comparison with the biological tolerances of the candidate species can be conducted by tabulating their unique environmental requirements. A “traffic light system” is useful where multiple sites and species are compared to highlight those which match well and those that do not match (see Case Study; Table 3). Green indicates suitability, red represents unsuitability, and orange indicates uncertainty, where species tolerance will be tested by the environmental conditions. The orange sites may be retained to be considered suitable by further investigation, or careful location within the site and/or engineering of appropriate housings, where spatial variability exists within the OWF site. Unshaded columns may represent additional parameters, such as exposure through mean spring tidal current and the maximum wave height. Whilst not directly comparable with species tolerances, these are important indicators for likely survival and reproductive success (Smaal et al., 2017; Kamermans et al., 2018), as well as NbS project design (Rendle, 2020).
Water depth is important in site selection, influencing the practicalities of introducing a species, the construction or implementation of a project, ongoing monitoring and maintenance of any housing structures, and the exposure of species to the high energy environment. Therefore, total water depth over a site should be calculated, along with the duration of maximum and minimum water depth or coverage. There may be a range of depths at sites of interest with varying seabed morphological features, including drying areas at low tide exposing sand banks; whereby the shallowing over banks influences currents, increasing turbulence and exposure. Depths >40 m would restrict some setup or installation methods and incur additional cost for a proposed NbS initiative (Benassai et al., 2014).
Another characterization parameter that requires consideration is distance to shore and the potential for undesirable weather or rough sea state further offshore, which helps to project the escalation of resource requirements (e.g., additional workforce effort and renting or fueling of vessels and equipment).
Sediment type is a critical consideration for NbS, where a sandy gravel seabed is likely to be more mobile, particularly at the shallow, exposed sand or gravel banks which typify OWF locations in the UK (Kenyon and Cooper, 2005). Fine sediment combined with high tidal flows or wave energy, for example, could lead to high background suspended sediment levels which can impact the feeding efficiency and hinder growth of filter feeders (Kamermans et al., 2018) and increase the risk of smothering (Rendle, 2020). Depth-averaged current speed or sediment concentration values which typify environmental reports, while useful, should be treated with caution since the background suspended sediment levels and currents are likely to vary significantly through the water column (Kamermans et al., 2018). Applying depth-averaged values may result in an under estimation of the total sediment load or exposure to smothering or scouring risks.
Mean spring tidal range and significant wave height (Hs) offer good indication of the magnitude of energy exposure (Kamermans et al., 2018), and certain species may require a specific flow speed (e.g., to influence direction/distance of larval transport). The greater the bed slope, tidal range or wave height, the greater the shear stress experienced at the seabed. Therefore, greater depths could offer protection from orbital wave energy, so wave height may not be a limiting factor where regions of an OWF have adequate depth (>10 m for sites exposed to Hs > 2 m, as a guide). The calculation of mean total water depth, i.e., the sum of water depth, tidal range, and wave height, at various points within a site of interest is therefore critical, particularly where the planned housing is suspended from the seabed or fixed on scour protection.
The “traffic light system” presented here offers a simple method suitable for any user to repeat, however this may be taken a step further to achieve a quantifiable suitability index, through methods such as fuzzy classification (Gimpel et al., 2015) or a multi-criteria evaluation technique (Benassai et al., 2014; Di Tullio et al., 2018). Finally, a numerical modelling method, described in Step 3, can further assist in scenario testing and decision making for promoting or discounting certain species.
2.3. Step 3: Data modelling – Feasibility and viability assessment
Once OWF sites are either discounted or identified as holding potential compatibility with candidate species (following Step 2), further investigation using numerical modelling approaches should be considered for feasibility and viability assessments to bridge data gaps and aid understanding. These are robust approaches that help an expert to inform the project designers on likely success, or how to lower the risk or losses ahead of pilot trials using cultivated or wild organisms, thereby increasing success rates and reducing uncertainty. Whilst recognizing that modelling does not fully replicate the complexity of the physical processes, modelling enables the NbS design parameters and environmental extremes to be explored in scenario-based test runs. If the outcome of these tests demonstrate that the NbS objectives are unobtainable, then the project should not move forward.
Hydrodynamic and spectral wave modelling at renewable energy sites is common for structural engineering and impact assessments (Day et al., 2015; Venugopal and Nemalidinne, 2015). Turbulence, seabed shear stress and scour potential data is typically available at most OWF sites (Lu et al., 2005; Chen and Lam, 2014; Kamermans et al., 2018) and should be systematically reviewed using the model or reporting for feasibility and viability, in terms of wind, wave and current derived shear stress. This is also inherently important in calculating combined load, deriving tolerances for a NbS housing design, and quantifying suspended sediment levels (bedload).
Estuarine and coastal hydrodynamic processes, such as circulation and transport, are sometimes neglected in the design and planning of restoration actions (Khangaonkar and Yang, 2011). Numerical modelling of energy and friction coefficients will further support the understanding of the physical processes at the site, through providing information on tidal currents and bed shear stress (Dyer, 1986; Soulsby and Whitehouse, 1997; Whitehouse, 1998; Sumer et al., 2001), advection and dispersion for waste or larvae (Kwon et al., 2005; Ali et al., 2011), hindcast and forecast wave parameters aiding design of structures (Thomas and Dwarakish, 2015), or understanding smothering issues from fine sediments in more sheltered environments (Manning et al., 2010). Understanding the physical processes at a site will improve the outcome of an in-situ pilot study, by informing any housing engineering or design elements, the installation methods and monitoring regime.
A hydrodynamic model supports feasibility studies for species survival, reproduction success, and larval dispersion (North et al., 2008; Herbert et al., 2012). Modelling aids the design of a well-informed pilot study for implementing a test population. A hydrodynamic model coupled with particle tracking or dispersion modules, or a behavior model, can help to assess success indicators of a planned pilot study pre-installation (e.g., larval dispersion) or mitigate consequential impacts. Likely survival for the larval stage of different species can be assessed and compared against parameters such as dispersion and, more specifically, in understanding and/or mitigating risks in habitat connectivity (Carr et al., 2017).
Guidance (van Bussel and Bierbooms, 2003) and numerical tools (Gintautas et al., 2016; Seyr and Muskulus, 2019) have likely previously been developed for OWF design or operation and maintenance. Where the hydrodynamic model is made available to the NbS project, the initial costs of model set-up and calibration can be saved. Where hydrodynamic model/s or output data are not made available to the study, or this effort cannot be completed due to costs, manual calculation is recommended. An oceanographer or coastal engineer can support an NbS project by calculating the bed shear stress (e.g., Kamermans et al., 2018) and design loads (e.g., Buck et al., 2008) using extreme physical conditions over a grid of the site of interest, using the appropriate standard equations.
The quantity of information available and whether surface or seabed installation is perceived, will dictate the model to be used. A two-dimensional, depth-averaged approach [e.g., see section 3.3; (Smyth et al., 2016)] provides detailed sea surface and current information. At greater computational expense, a three-dimensional approach will provide stratification through the water column, enabling an improved understanding of the loading at different depths and sediment dynamics at the seabed (Mangor, 2004). Once setup, the hydrodynamic model can be used to run scenario tests for environmental conditions and inform logistics for NbS installation, ease for sampling and maintenance, operations limitations, site accessibility, decision making related to weather downtime and support cost:benefit consequence analysis.
3. Case study: offshore wind farms and bivalve NbS
The three-step approach was applied to 12 OWFs located within UK waters in the Irish and North Seas (Figure 2). As a developer-operator of OWFs, Ørsted were interested in investigating the likely success rate of introducing the European flat oyster (Ostrea edulis) into Gunfleet Sands OWF licensed area. An initial feasibility review was conducted for Blue Marine Foundation Plc by Resilient Coasts Ltd. (Rendle, 2020; Robertson et al., 2020). Linear modelling techniques helped to describe and explain the implications of the highly exposed environment on survival, reproduction, and larval transport, and therefore the limited likely success of the NbS initiative. The linear approach offered an indicative solution, with significant limitations. Thus, beyond the scope of the initial feasibility review (Rendle, 2020), a hydrodynamic model was developed for Gunfleet Sands to further the initial investigation and support improved understanding around physical processes for interested stakeholders. Herein, we demonstrate the three-step approach to evidence the co-location of O. edulis at Gunfleet Sands.
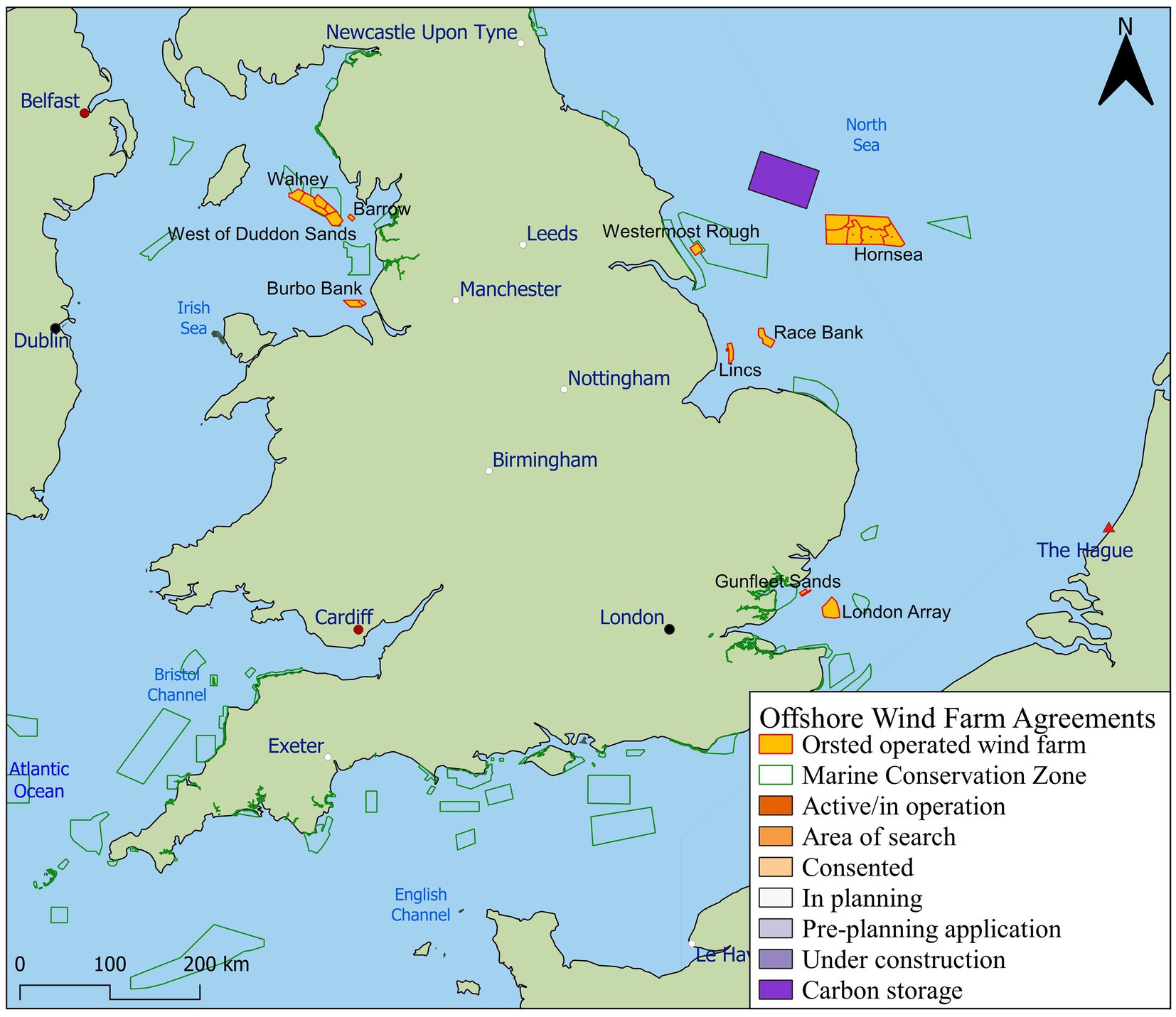
Figure 2. The Crown Estate (2020) licensed offshore wind farm areas in English waters with active, consented, or planned activity. Sites operated by Ørsted are labelled shapes, orange with red outline, as listed in Table 1. Marine Conservation Zones are depicted in green (Natural England, 2020).
3.1. Step 1: Data and information synthesis
3.1.1. OWF licensed areas
The initial step required collation of the physical and environmental characteristics of OWF sites (section 2.1). Twelve wind farms were considered within this review, mapped in Figure 2 and further described in Table 1. The OWFs reviewed for this study represent a habitat range from 6.4 km to 120 km offshore and in depths ranging from −2.5 m to 76 m. The extreme wave height ranges between sites from 3.5 m to 12.9 m and mean spring tidal current ranges from 0.6 ms−1 to 1.5 ms−1. All sites are located in well-mixed water bodies, due to the energetic environmental conditions associated with OWF sites. Therefore, an assumption was made regarding stratification in the water column of salinity and chl a, since both parameters will be influential for locating filter feeding organisms. Data regarding turbulence or mixing in the depth profile of water bodies can be a useful indicator of where salinity and/or chl a stratification is likely.
Environmental characteristics established in Step 1 influence species survival, and therefore the feasibility and suitability to introduce NbS to the site. Distance to shore is largely important due to ease of access to the site, which consequently impacts the cost or feasibility of equipment deployment, monitoring, inspection, and maintenance. Data collated within this step will enable Step 2 (data analysis) to take place. The majority of information collated within this step is presented and analyzed in Table 3 (section 3.2), to avoid repetition.
3.1.2. Bivalve species
Following collation of site-specific data, Step 1 then requires the physical and environmental tolerances of potential species of interest. The tolerances of five bivalve mollusc species of commercial importance and/or conservation concern are presented as part of this case study (Table 2). Scallops (Pecten maximus) currently hold the most value in the UK, worth £34 million per annum and contributing to 30% of the total weight landings from commercial bivalve species (Laing and Spencer, 2006), while Cerastoderma edule (Common cockle) is Europe’s primary wild-harvested bivalve species, with an economic value of up to £10 million per annum (Carss et al., 2020). Investigations surrounding the feasibility of cultivating the Common cockle have been undertaken, with clear signs of potential (Pronker et al., 2015). The cultivation of species through developing mariculture practices within OWFs may alleviate pressure on wild populations or provide a mechanism to restore lost habitats.
A particularly important species in Europe is Ostrea edulis (European flat oyster), which has suffered significant population collapse from overfishing, pollution, disease, and invasive species related pressures (Heral, 1989; Helmer et al., 2019). Research estimates a global loss of over 85% of oyster reef ecosystems, with oyster populations in the Irish and North Seas considered “poor” and “functionally extinct,” respectively (Beck et al., 2011). The economically important fishery landings fell from 40 million to 3 million oysters landed between the 1920s and 1960s (Edwards, 1997). The habitat loss has affected marine ecosystems and coastal community health, demonstrated by a decline in coastal species biodiversity (Laing et al., 2006). In response, oyster restoration initiatives have been growing worldwide (Harding et al., 2016), and O. edulis is listed as an OSPAR priority species (Haelters and Kerckhof, 2009) under the UK Biodiversity Action Plan (Gosling, 2015) to meet global targets set out by the UN Convention on Biological Diversity.
The environmental tolerances gathered from the literature (Table 2) show that inter-species tolerances vary. Therefore, restoration or cultivation sites must be selected based on species-specific requirements. Bivalve species have specific temperature and salinity ranges required for optimum growth and reproduction, and tolerable energy exposure is also species dependent (Table 2). The bivalve species reviewed in this study thrive in sheltered regions with low tidal current velocities (Laing and Spencer, 2006; Smaal et al., 2017), therefore focusing on areas with lowest flows will likely improve survival rates.
3.2. Step 2: Data analysis – Site suitability and species compatibility
The physical environmental characteristics of 12 UK OWFs operated by Ørsted were established through detailed review of all publicly available reports and literature (Figure 2).
Following collation of site characteristics, species established in Step 1 (Table 2) were compared against the environmental conditions at each site, thereby identifying compatible sites and selecting species. For the purpose of this case study example, the non-reef forming O. edulis was selected and carried forward due to the significant interest in the restoration of this declining population (Haelters and Kerckhof, 2009; Gosling, 2015; Harding et al., 2016). Thus, Table 3 was color-coordinated according to compatibility of sites with O. edulis tolerances. This could be repeated with any of the species described in Table 2.
The conservation group “Essex Native Oyster Restoration Initiative” is working to restore and conserve O. edulis for ecosystem services and co-benefits within the EU-designated Marine Conservation Zone of “Blackwater, Crouch, Roach and Colne Estuaries” (ENORI, 2021). This conservation zone represents the largest UK inshore designated area covering 284 km2. The 172 MW Gunfleet Sands OWF is located 7 km off the east coast at Clacton-on-Sea, UK (Figure 3), within proximity of the marine conservation zone. It is therefore an ideal NbS pilot study location due to its proximity to restoration sites and ease of NbS installation and maintenance.
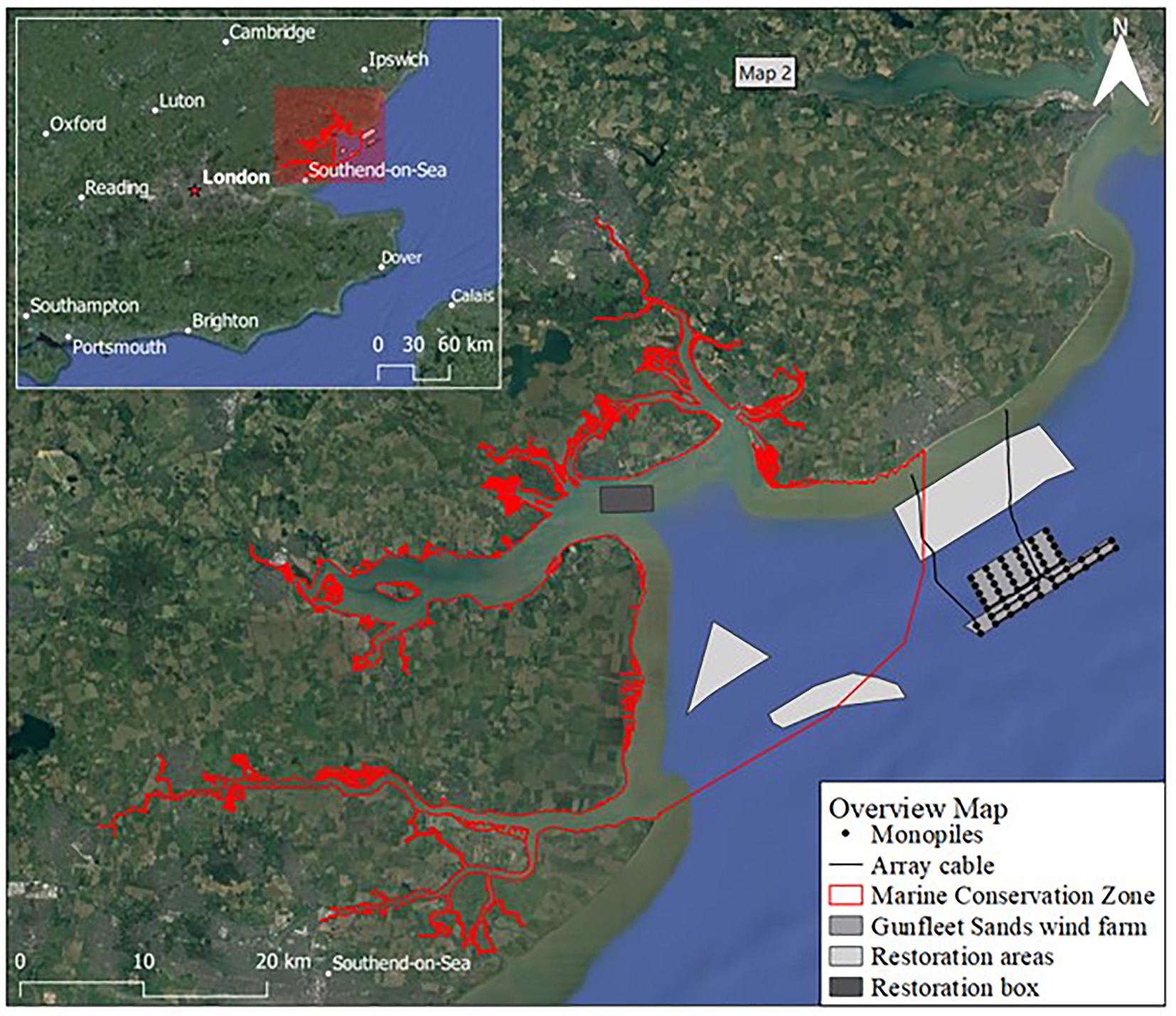
Figure 3. Map of Gunfleet Sands offshore wind farm in Essex UK, with monopile locations and cables for reference, with Marine Conservation Zone (MCZ) in white and the sites of interest for restoration and repopulation identified.
The environmental conditions at Gunfleet Sands were compared to the biological tolerances for O. edulis, established in Step 2. Water temperature, flow (wave and tide driven), chl a content, lunar and tidal cycles all influence larval occurrence, growth and/or dispersion (Wilson, 1987; Smaal et al., 2017; Kamermans et al., 2018; Maathuis et al., 2020). The conditions for oyster habitat are summarized in Table 4, based upon previous restoration pilot studies.
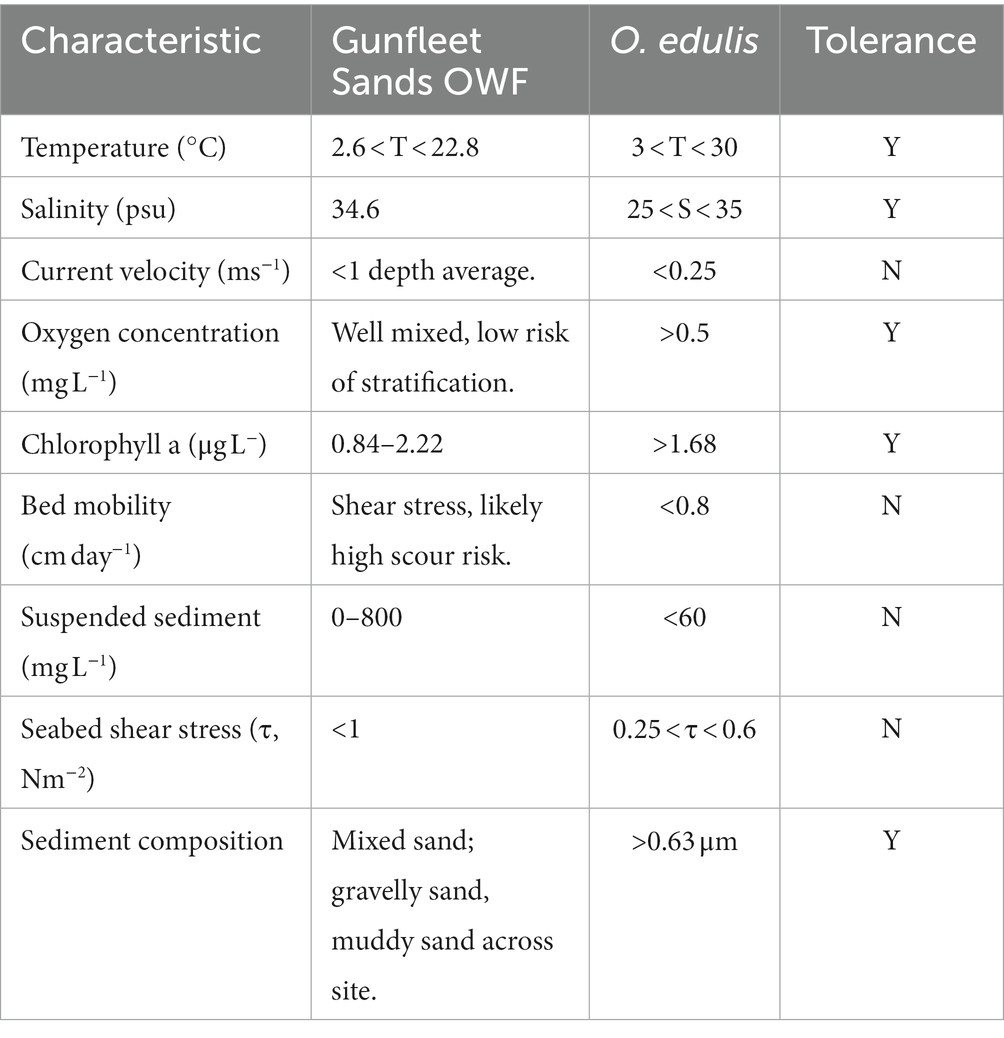
Table 4. Tolerance range of Ostrea edulis for survival, growth and reproduction, adapted from Kamermans et al. (2018) and Smaal et al. (2017).
A mixed sand bank morphology typifies the seabed at Gunfleet Sands, which mostly consists of a firm substrate formed by partially lithified cross-bedded sands, covered by up to 2 m of mobile sand and sandy mud (Dong Energy, 2007). Levels of suspended sediment can be high during periods of high wave energy; 0–400 mgl−1 on the northern flank, and 0–800 mgl−1 on the southern flank (GE Wind Energy, 2002). The region experiences a bi-modal wave climate, with principal wave directions from the northeast and southwest accounting for 31% and 33% of waves, respectively. The site is moderately exposed (significant wave height < 1 m and wave period <6 s), with larger waves from the southwest and predicted extreme wave height reaching 4.5 m (GE Wind Energy, 2002). A nearby waverider buoy demonstrates a mean temperature of 12.3°C (range of 2.6°C to 22.8°C), measured over a 12-year period (Felixstowe; Channel Coastal Observatory, 2020). The windfarm and surrounding regions are well-mixed, meaning that stratification is unlikely to occur, which can limit oxygen and chl a at the seabed. The tidal current and wave derived bed shear stress at shallows are likely to cause increased bed mobility and risks, making GFS a complex selection for housing the oyster restoration initiative. A typical O. edulis habitat is less exposed than the Gunfleet Sands site, therefore it was determined that installation at depth (>10 m) is advised to house O. edulis below surface wave energy, thereby providing shelter at depth.
The chances of O. edulis larvae survival are small (Korringa, 1946) with survival rates of 5% in favorable conditions (Maathuis et al., 2020), however the deployment of old shell and gravel (cultch) improves settlement success and survival rates (Laing et al., 2006), hence the restoration box identified within the marine conservation zone has been prepared with clutch (Figure 3).
3.3. Step 3: Data modelling; feasibility assessment
Presuming O. edulis survive and reproduce when co-located in Gunfleet Sands, the next challenge is to ensure the larvae travel to the restoration areas within the marine conservation zone. Given a relative particle size, the tidal flow could feasibly transport larvae towards the estuary. Rendle (2020) and Robertson et al. (2020) applied a linear depth-averaged approach for Gunfleet Sands, which assumed released larvae are transported passively with the prevailing flows. This indicated a short window of opportunity for circulation near the edge of the marine conservation zone and over potential restoration zones, before being carried rapidly offshore. The linear approach was highly simplified to assess the capacity for a given grain size and weight due to gravity to be carried over a specified distance, offering an indicative calculation to predict larval transport. The dispersion of the larval stage oysters is likely to be influenced by other parameters, such as wind and wave energy, which require a more complex modelling approach.
To further investigate, a hydrodynamic model of Gunfleet Sands was produced to demonstrate larval direction and dispersion for site suitability and feasibility, representing Step 3. While larval dispersion is the main criteria of interest in this case study, other uses for the hydrodynamic model include energy exposure, habitat design parameters and loadings, and bed shear stress. An off-the-shelf industry standard numerical model was utilized within this study and run with open source regional and global data. The procedure presented herein is representative of the model set-up (section 3.3.1) and calibration/validation (section 3.3.2) for reviewing any OWF or renewable energy site of interest to reduce uncertainty around pilot project planning.
3.3.1. Model set-up and boundary conditions
DHI MIKE 21 is a 2D modelling software package for the modelling of hydrodynamics, waves, sediment dynamics and water quality. Hydrodynamic and particle-tracking modules within DHI’s MIKE21 Flow Model (DHI, 2017) were used to simulate local tide-driven currents, wind-wave interaction and larval dispersion from a release point within Gunfleet Sands OWF. Standard settings were selected, resulting in a depth-averaged, barotropic flow assumption. To ensure an accurate representation of the region of interest, the shallow sand banks are represented at a high resolution (Figure 4). A lower resolution mesh is utilized for deeper offshore regions to provide a suitable balance between accuracy and computational efficiency. The bathymetry at the offshore model boundaries was artificially deepened to further improve model stability. This is not expected to influence results within the region of interest, as demonstrated through validation processes (section 3.3.2). Tidal elevations form the northern, eastern, and southern vertical boundaries, as predicted by DHI’s Global Tide Model, which has a resolution of 0.125o and includes 10 tidal constituents.
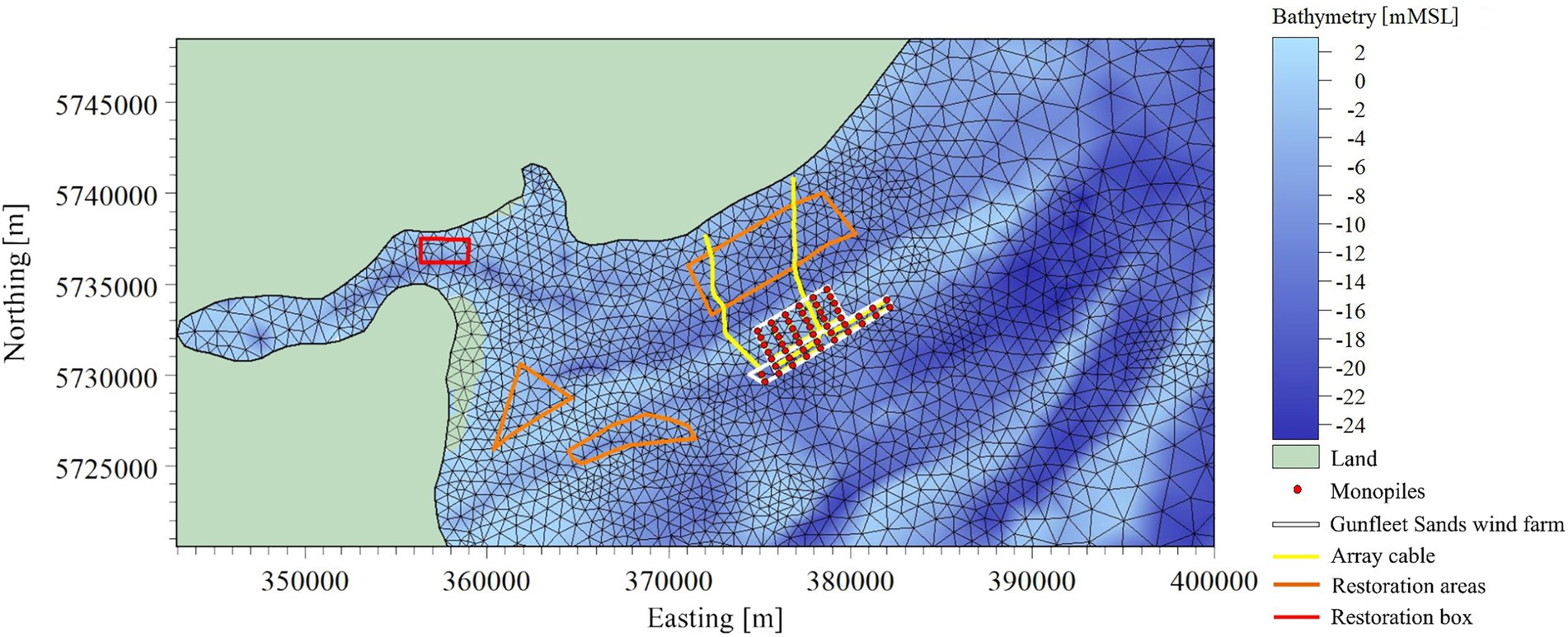
Figure 4. DHI Mike21 flexible mesh set up with bathymetry projected (blue scale), utilized for hydrodynamic modelling of the Gunfleet Sands offshore wind farm (red points and blue extent for turbines, green for landing cables), with the oyster restoration sites (in red and purple) within the local Marine Conservation Zone (MCZ). The generated 2-dimensional flexible triangular mesh consists of 3,272 nodes and 6,287 elements.
3.3.2. Model validation
Since observational water level data was not available within the model boundaries, UKHO (2020) data was extracted from the regional EasyTide (UKHO, 2020) model and utilized for model validation. This was compared with the nearby Felixstow wave buoy to cross reference the tidal signal. The model was then calibrated to improve the replication of tidal flow. Given this study is focusing on dispersion patterns and indicative values, as opposed to parameters for detailed design, this method is considered appropriate for model validation. The modelled surface elevation is relatively well represented; the tide is in-phase and compared well against predicted data at Whitaker Beacon and Bradwell Waterside, where a root mean squared error of 0.355 m and 0.702 m were achieved, respectively (Figure 5). A degree of variance is expected due to the hydrodynamic model being run with and validated against modelled datasets. The current flow at the Gunfleet Sands OWF region is tide dominated in a northeast direction with ebb tide and southeast direction with flood tide. The tidal currents tend to flow along the shore-parallel sandbanks, funneled by the channels in the seabed morphology, with circulation blocked by sand bars (Figure 6).
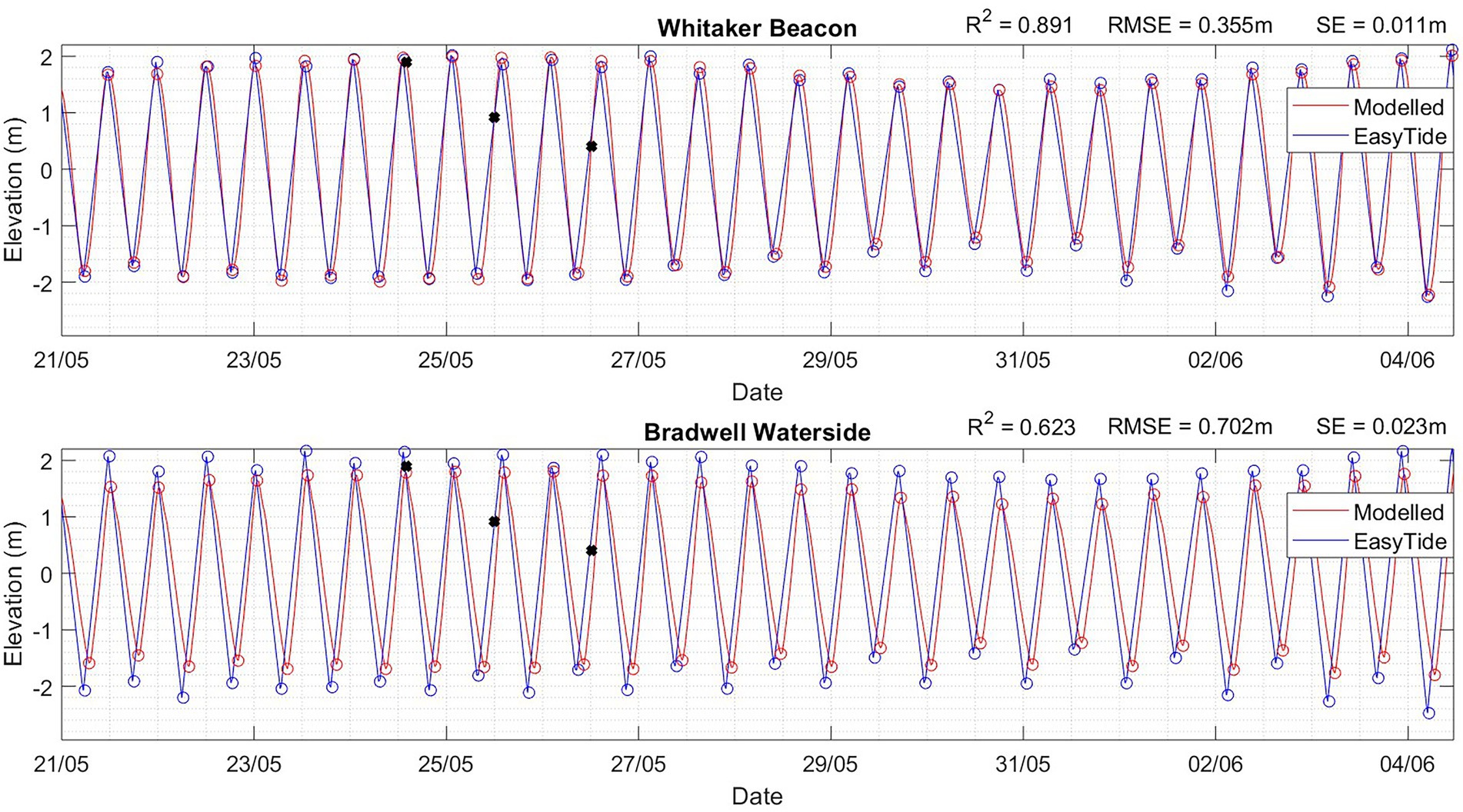
Figure 5. Surface water level elevation from the hydrodynamic model (red) and previously validated Easy Tide (UKHO, 2020) data between 21/05/20–04/06/20 at (A) Whitaker Beacon and (B) Bradwell Waterside. The root mean square error (RMSE), standard error (SE), and R2 values are presented. Black asterisks mark the three larval release times associated with spring tide.
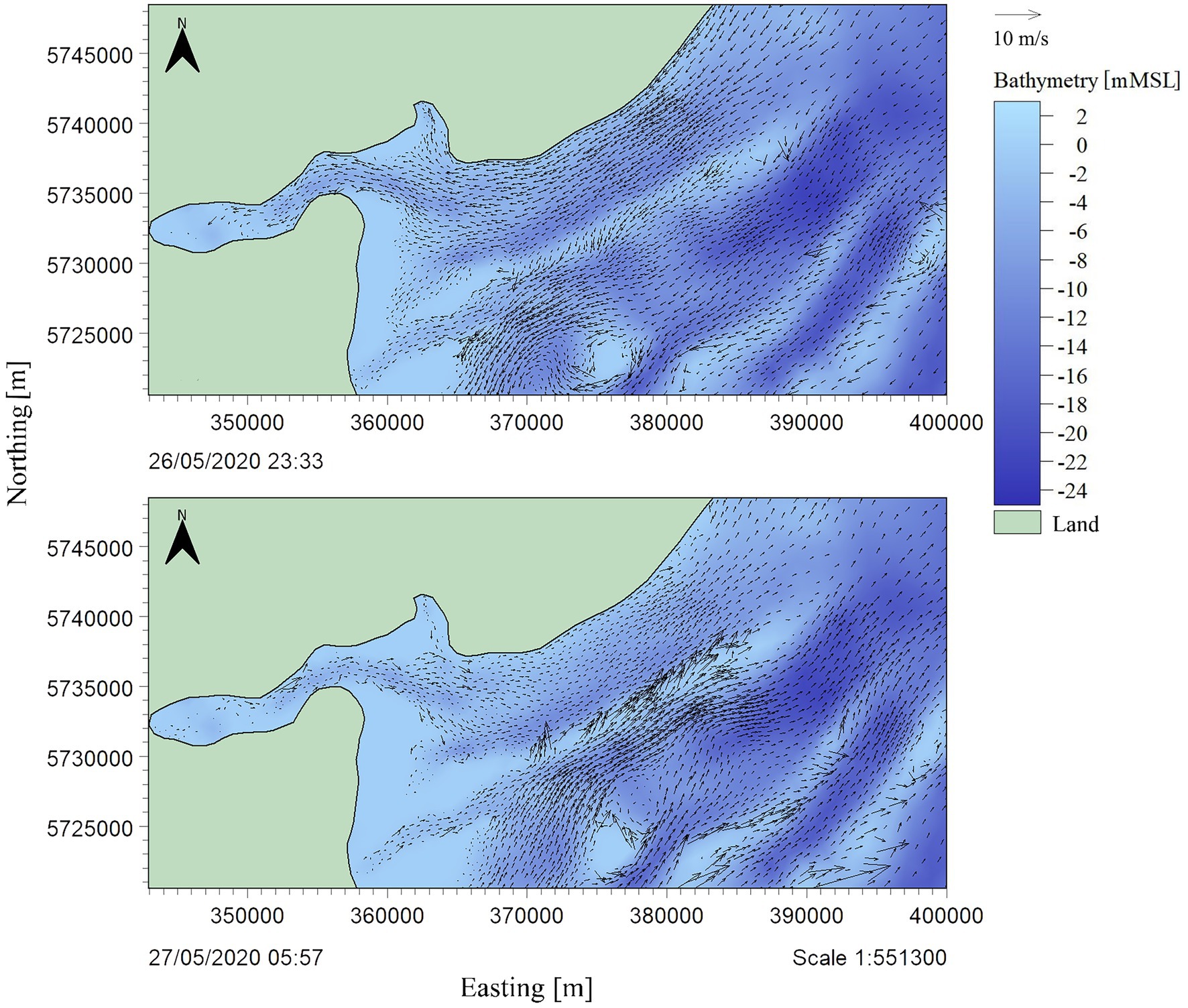
Figure 6. Bathymetry of the Gunfleet Sands offshore wind farm, with vectors demonstrating the modelled tidal current flow (ms−1) on (A) a flooding tide (HW-3.0 h) directional flow southwest, and (B) an ebbing tide (HW + 3.5 h), directional flow northeast. Where HW is high water.
3.3.3. Particle tracking and larval dispersion
Since O. edulis larvae can remain suspended within the water column for around 10 days (Smaal et al., 2017; Kamermans et al., 2018), their destination is dominated by current flow, although they retain some mobility in the water column. Water-borne transport is a key factor controlling or regulating the dispersal of the larval stage of benthic marine invertebrates which have limited mobility (Smyth et al., 2016). It is necessary that the larvae settle on suitable substrate within proximity to the point of release. Thus, modelling the hydrodynamics, larvae dispersal and settlement are useful approaches for determining a successful site (see lifecycle, Figure 7). While larvae biological attributes, such as the velum, may influence factors such as buoyancy and thus vertical distribution (Knights et al., 2006), the nature of the depth-averaged model means location within the water column was not considered. We focused on the likelihood of the larvae reaching the proximity of a restoration area within the average time that larvae are predicted to survive in suspension at sea (circa 10-days). There were two key assumptions given the exposed conditions at OWF sites; (1) the larvae behave as passive, neutrally buoyant, spherical particles, and (2) the behavior of larvae within the water column were dominated by the exposed physical environment. These assumptions would not be appropriate in sheltered regions where larval shape and mobility will dominate (Rodriduez-Perez et al., 2020), enabling settling.
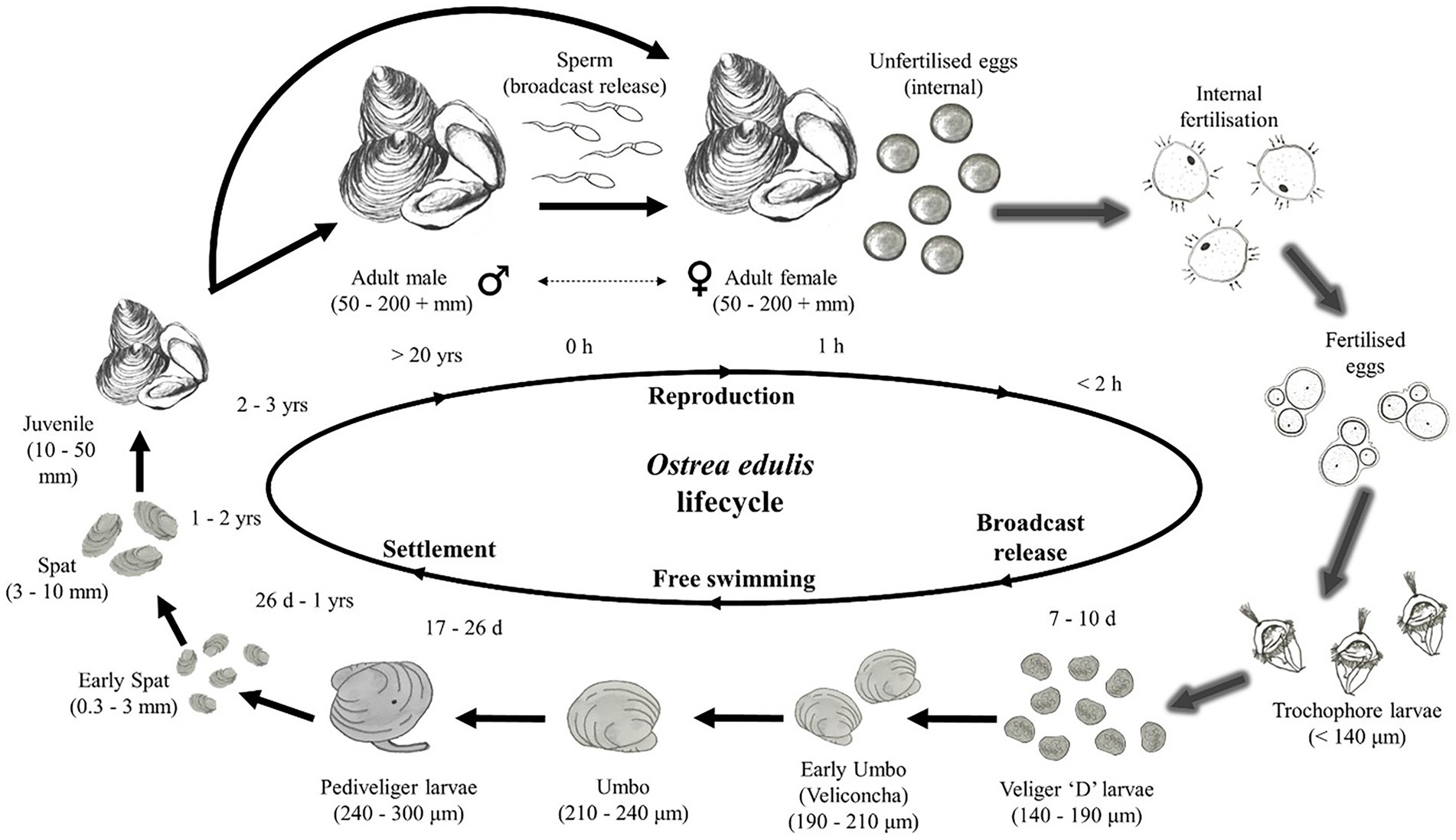
Figure 7. Stages of the Ostrea edulis lifecycle. Arrows with a shadow represent stages that occur within the oyster, the sharp arrows are external stages. Larvae are modelled as the Veliger “D” larvae stage shortly after being broadcast from the oyster, they can survive for 7–10 days at this stage. Figure sourced from Helmer et al. (2019).
A hydrodynamic model was utilized to simulate larval transport through two methods: particle tracking and dispersion. A theoretical population of 2,500 mature O. edulis installed at the Gunfleet Sands OWF were represented. The larvae released in the model per oyster was 15,000, based on an estimated survival rate of 1.5% (Cranfield and Allen, 1977). Therefore, 37,500,000 particles representing larvae were simulated in the dispersion model (Figure 7; Helmer et al., 2019). The larvae were released over a 3-day period over a spring tide from the southwestern extent of Gunfleet Sands OWF (Figure 8).
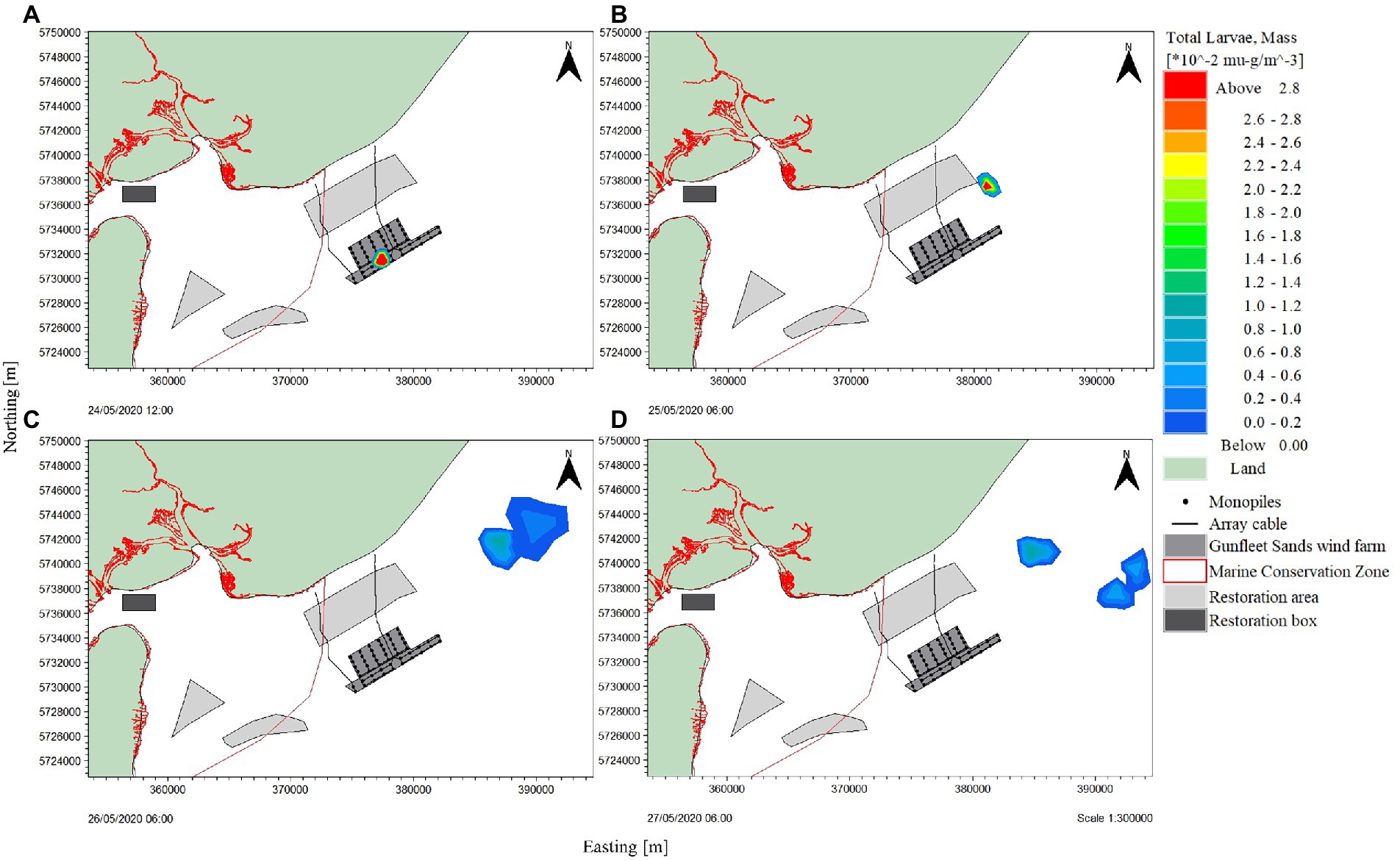
Figure 8. Distribution of total oyster larvae mass following release from Gunfleet Sands offshore wind farm, Essex. Larvae dispersal was simulated over a 3-day period between 24/05/20 to 26/05/2020. Image plotted in QGIS; WGS84, UTM Zone 31 N. Subplot (A) Larvae release showing dispersion plume at zero hours, (B) dispersion of larval plume after 18 h, (C) plume dispersion after 42 h, and (D) plume dispersion after 66 h.
Particle tracking enables simulation of individual inert particles being carried with a given settling velocity, determined according to Stokes’s (1851) law. The MIKE21 particle tracking module (DHI, 2017) was coupled with the hydrodynamic model to simulate larval transport within the study region, modelling the movement of individual particles. The input parameters used to describe the larvae in the MIKE21 particle tracking module were diameter 1.73 × 10−5 mm, minimum mass as 0.71 μg, and the maximum particle age utilized was 10 days (Labarta et al., 1999; Helmer et al., 2019).
The settling velocity was calculated to be 2.09 × 10−8 ms−1 using Stoke’s law (Stokes, 1851; Ferguson and Church, 2004):
where g is acceleration due to gravity (9.81 ms−1), d is the particle diameter (1.73 × 10−8 m), ρ1 and ρ are the mass density of the particle (2,000 kgm3) and fluid (1026.2 kgm3), respectively, and υ is the fluid viscosity (1.31 × 10−3 Pa s).
Multiple simulations were completed (Table 5) to test whether a suitable site could be identified, to result in larval transport towards or near the marine conservation zone. Different scenarios of likely wind-wave conditions over the modelled period (24/05/20–04/06/20) and various potential turbines within the OWF, whereby the oysters would release the larvae, were explored. All model-runs resulted in similar results, with no significant difference in general direction of larvae transport under different wind-wave conditions or release locations, dispersing the larvae away from the marine conservation zone. The hydrodynamic model’s depth-averaged assumptions occur regarding surface vs. bottom conditions; however, the results demonstrate no significant impact on direction or distance of larval transport with the addition of wind-wave interactions of the model, due to the region being tide dominated. The time of the tide and location of release within the site also did not significantly influence the average direction of particle movement.
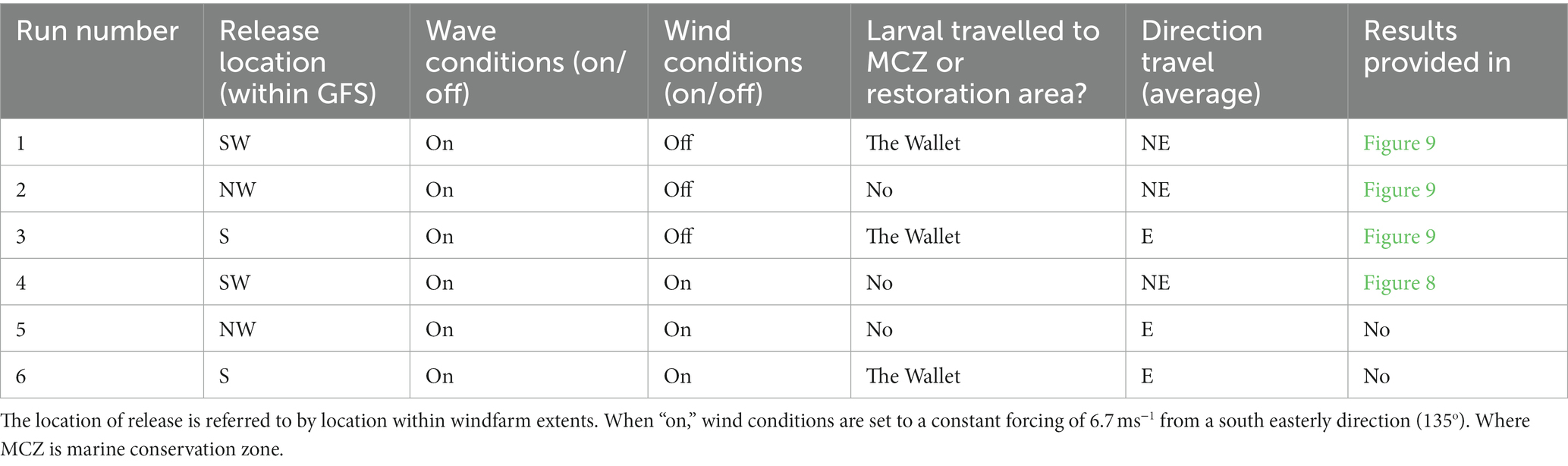
Table 5. Model runs (producing outputs for both particle tracking and dispersion) under varying modelled wind-wave conditions and release locations within Gunfleet Sands (GFS), tested to model direction of larval dispersion.
Three larvae release tracks from different turbine positions, with no wind forcing, are illustrated (Figure 9). Particle tracking from different locations within Gunfleet Sands demonstrated an offshore directional trend. Under certain release locations (e.g., southwestern or southern extent of Gunfleet Sands), it is possible for the larvae to reach “The Wallet” temporarily (restoration area to the north). However, the window of opportunity for larval settlement is short, given that the larvae are quickly carried offshore, away from this more exposed restoration site.
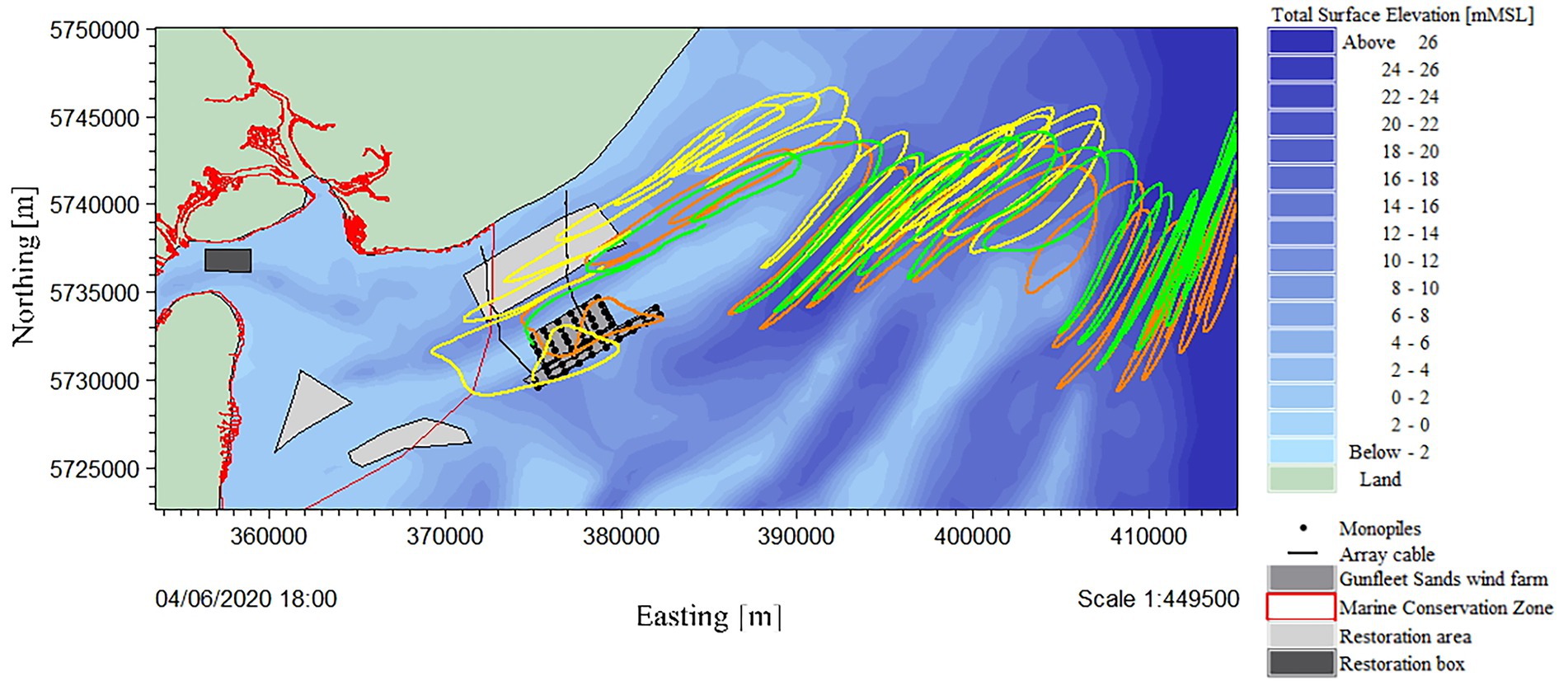
Figure 9. Modelled particle track of larvae over a 10-day period (24/05/20–04/06/20) released from Gunfleet Sands offshore wind farm on 24/05/20 at 12:00 to correspond with peak tide. Three turbine locations were modelled due to their scour protection and proximity to the Marine Conservation Zone (MCZ), the release points representing their relative positions: the center in orange, the southwest extent in yellow, and northwest extent in green.
The locally validated model was also applied to model particle advection and dispersion, expressed in relative terms as a fraction of the larvae released at a site. The shape of the spatial distribution provides information on dispersal (Kamermans et al., 2018). The larval dispersion demonstrated a northeast direction of travel, travelling with the tidal current parallel to the sandbanks. The larval plume travels from Gunfleet Sands OWF, dispersing towards the coast and northeast away from the marine conservation zone (Figure 8). After 24-h from initial release, the larvae had dispersed to low levels of detection at the release site, yet away from the site of interest. The majority of larvae had travelled in a northeast direction 42-h after release (Figure 8C), dispersing further after 66-h in both a north-northeast and east-northeast direction (Figure 8D). The interest in and argument for the ebb tidal oscillation carrying O.edulis larvae into the marine conservation area and other restoration sites is therefore considered unfounded.
3.4. Case study: conclusions
The results of this study highlight that, whilst Gunfleet Sands provides an attractive option for O. edulis co-location due to relative shelter at depth and hard substrate to attach housing, albeit finite available surface area, project success is unlikely. The model output demonstrated that the larval dispersion from different locations of interest within Gunfleet Sands is offshore, due to the direction of travel with current flow, and not toward the marine conservation zone, where the restoration of O. edulis is planned. Therefore, the restoration initiative for the marine conservation zone would not likely be successful given the low confidence in larvae reaching the restoration sites when released from Gunfleet Sands. This modelling approach cost a fraction of the planned pilot study and informed the decision to not continue with the pilot given the intended outcome would not be realized. The hydrodynamic modelling outlined the uncertainty and risks related to the planned project and enabled the conservation groups and operator to improve their understanding of success. Remaining project resources would be better utilized on local approaches for restoration, either through a focus on alternative methods, e.g., seeding with oyster spat within the restoration sites (Colsoul et al., 2021) or exploring other OWF sites that a model proves evidence for likely success.
4. Discussion
The three-step approach for a site-species feasibility assessment provides a logical guide to simplify the review and assessment of OWFs for site suitability in co-locating NbS projects. The presented approach demonstrates the ability to use open-source environmental data to provide a rapid site-species compatibility assessment for OWF licensed areas. A feasibility assessment of the co-location of NbS within OWF can be explored to improve understanding of success and sustainability. The presented approach empowers conservation groups and NbS practitioners to make cost-effective decisions that maximize likelihood of restoration success through a methodical review and synthesis of widely available information. This method improves the baseline evidence for business case development of in situ pilot studies. The approach results in an informed decision with the opportunity to test the outcome in a numerical environment, and develop then monitor success indicators.
Often projects are conducted by non-technical conservation experts, therefore refining a stepwise approach to help design pilot studies is key to both achieving success and helping with communication. The physical processes at identified sites of interest can be easily assessed to determine suitability to house and support NbS with one or more of the following goals: retrofitting for habitat restoration, co-location with mariculture, or carbon sequestration. The benefits of the approach include the speed of assessment and minimum resources required to generate baseline evidence when compared to in situ pilot studies alone. The produced evidence helps discuss and logically resolve key practical questions regarding NbS logistics, set-up and management. Whilst this paper provides a review using bivalves to demonstrate the case study, the three-step co-location approach can be adapted and applied to other NbS initiatives, for example, using fish, seagrass, seaweed, and coral.
Since the proposed method reviews the sites considered for restoration without accessing the site, it is more efficient. Resource reduction is estimated as an order of magnitude less through the review of physical processes and hydrodynamic modelling in the feasibility assessment ahead of a small-scale pilot field investigation (e.g., <£10,000 vs. >£100,000, respectively). The additional benefit is a deeper understanding of likely success in a more robust methodology than using the pilot project alone, which could monitor survival and reproduction, but not tracking larvae or provide details of larval dispersion with any confidence.
Numerical modelling and expert interpretation of the output results, with the support of geospatial mapping, will assist with understanding environmental complexity, for example the implications of the local meteorological and oceanographic climate, or the behavior of species and interaction with OWF infrastructure. The resulting baseline evidence provides a stepping-off point for discussion with stakeholders regarding potential for co-location and the results from the feasibility assessment. Pilot studies that are planned in consultation with stakeholders and designed with strong evidence for success are more likely to be supported by stakeholders. Additionally, projects will benefit from support gained from community, industry, and government initiatives, with parallel attempts to improve traction with funding bodies or trusts. The pilot stage remains an essential step prior to scaling-up NbS initiatives, as the practicalities and logistics of offshore and marine construction are specific to the location, plus housing trials can be completed in a laboratory or through in situ tests.
When applied to the presented OWF site (Gunfleet Sands) and species (O. edulis), the three-step approach helped to demonstrate that the proposed restoration initiative would not be successful given the low confidence in larvae reaching the restoration sites. While resulting in a negative outcome, this approach cost a fraction of the planned pilot study, which would not have achieved quantitative information or described the failure mechanism. This enables remaining project resources to be utilized for exploration into alternative NbS approaches or structural assets that may offer greater likelihood for project success. It is essential to acknowledge potential project failures (Catalano et al., 2019), and that NbS require the appropriate environment and conditions to be successful and avoid unnecessary risks and costs (Seddon et al., 2020), thus may not be the most appropriate solution in all cases.
A list of structural assets in UK waters will aid inter-site comparison and application of this approach for other NbS species of interest. A matrix of site-species compatibility for NbS projects to potentially co-locate with improved confidence of success, can then be taken forward to improve a business case proposal to OWF operators. The baseline evidence will influence decisions to co-locate NbS projects where cost:benefit is favorable and appropriately considers site logistics. Developing a strategic approach to align conservation interests with engaged OWF operators with clearly defined vision, measurable goals and success indicators will prove more successful than an ad hoc approach to NbS implementation. Whilst adaptation approaches work well on land and in the intertidal zone, key considerations for offshore implementation relate to higher costs and risks associated with relocating wild fauna.
Presently, during the OWF design phases, there are no secondary use considerations that could be environmentally, ecologically and/or economically beneficial. While it is logical to consider NbS early in the process, the installation of habitat-enhancing structures or substrate is presently only possible post-construction (Royal Haskoning DHV, 2020). NbS initiatives are not traditionally considered or incorporated into policy, legislative, regulatory, or organizational arrangements for OWFs. Given the emergent field, few experienced NbS practitioners exist and neither developers nor operators hold the awareness or key skills. The developer will perceive NbS retrofitting as an additional complexity and risk with wide uncertainties, until these are better defined and options to mitigate risk are presented.
A paradigm shift is required in order to co-locate NbS projects within OWF design and operation and maintenance. The information to design and support NbS projects requires consideration from OWF concept (Christie et al., 2014) or under the marine area licensing agreement. Equally, if an NbS project is not designed and moderated within standard procedures and operation and maintenance activities, it is unlikely to proceed. If NbS project benefits and constraints are integrated under an institutional arrangement of integrated management, they have the potential to be successful. Michler-Cieluch et al. (2009) discuss offshore co-management approaches for OWFs and mariculture sectors, which benefit through the integration and shared resources in the operation and maintenance activities. The main driver for improving the practice will be policies and guidelines relating to sustainable practices in co-location projects. Whilst retrofitting infrastructure or co-locating housing are desirable, designing OWF with NbS from the planning stage is preferable (Christie et al., 2014).
5. Conclusion
An approach to identify potential OWFs for the purpose of co-location with compatible species is introduced, with the aim of reducing complexity and guiding groups or stakeholders who may be interested in trialing NbS. The co-location approach developed for NbS in OWFs highlighted that distance offshore, depth and substrate type are key considerations for the practicalities of deploying instruments, housing or artificial habitat, site access, and installation of equipment. Additionally, consideration for OWF planning, design, operation and maintenance is critical. Any NbS project should be included in the planning consent process, within the environmental statement, to ensure concerns regarding overspill and combined interactions on ecology or socio-economic implications are accounted for and mitigated against.
The three-step approach introduced for co-locating NbS is designed to improve the project outcomes in terms of the aims, goals, and success indicators. Analysis can be carried out using a hydrodynamic model and other coupled models, to inform on wider aspects of designing and managing a project. Approximation of survival rates, reproduction capabilities, behavior, or socio-economic parameters for baseline evidence and business case development is advised. For example, to improve location selection based on depth and substrate within the OWF domain, and engineering load for the design of housings and culture systems. Logically, implementation costs will increase where an OWF is located further away from shore and transport routes to market, at depth, or is highly exposed.
It is necessary to identify potential failure mechanisms or high-risk issues that will hinder restoration or NbS success rates. This method provides the evidence base for pilot project site selection and implementation. Therefore, financial risk is reduced, and likelihood of project success improved. The results from this study can be used to form the case for additional feasibility studies on marine licensed areas and designing appropriate pilot projects within the renewables or other sectors.
Data availability statement
The raw data supporting the conclusions of this article will be made available by the authors, without undue reservation.
Author contributions
ER, EH, and AB contributed to the design of the approach and contributed to manuscript revision and review. ER planned the paper, performed the feasibility assessment, and managed data and information. ER and EH performed the numerical modelling assessment. EH wrote the first draft of the manuscript under the guidance of ER. AB contributed biological and structural guidance for drafts versions, and final revision. All authors contributed to the article and approved the submitted version.
Acknowledgments
This research was part-funded by a European Regional Development Fund (ERDF) grant funded internship through the University of Plymouth “STEM Graduate into Business” project, awarded to Resilient Coasts Ltd (RCL) and part-funded using internal RCL research and development funds. Danish Hydraulics Institute (DHI) awarded a student license for MIKE 21 to EH to conduct the modelling and analysis under the student internship alongside ER under RCL. The bathymetry data was sourced from the Channel Coastal Observatory. An initial feasibility study was commissioned by BLUE Marine Foundation Plc., who asked RCL to investigate the potential for a native oyster restoration pilot project at Gunfleet Sands. This research follows that study, yet was not funded by BLUE. Specific thanks to Morven Robertson, Matt Uttley, Jacob Kean Hammerson, Sophie Locke (BLUE), Hywel Roberts and Palle Martin Jensen (Ørsted), Pauline Kamersman (Wageningen Marine Research), Tom Benson (HR Wallingford), Alison Debney (Zoological Society of London), Tom Cameron and Alice Lown (University of Essex), and Thomas Prime (National Oceanography Centre), for the knowledge, literature, data, and input to the method development.
Conflict of interest
ER and EH were employed by the company Resilient Coasts Ltd.
The authors declare that this study received funding from Resilient Coasts Ltd. The funder had the following involvement in the study: co-funding the internship with University of Plymouth STEM graduates into Business (EU ERDF funding) to undertake the data analysis, numerical modelling, preparation of tables and figures, and drafting work associated with the paper development.
The remaining author declares that the research was conducted in the absence of any commercial or financial relationships that could be construed as a potential conflict of interest.
Publisher’s note
All claims expressed in this article are solely those of the authors and do not necessarily represent those of their affiliated organizations, or those of the publisher, the editors and the reviewers. Any product that may be evaluated in this article, or claim that may be made by its manufacturer, is not guaranteed or endorsed by the publisher.
References
Ali, A., Thiem, Ø., Berntsen, J., Ali, A., Thiem, Ø., Thiem, Ø., et al. (2011). Numerical modelling of organic waste dispersion from fjord located fish farms. Ocean Dyn. 61, 977–989. doi: 10.1007/s10236-011-0393-8
Ashley, M., Austen, M., Rodwell, L., and Mangi, S. C. (2018). “Co-locating offshore wind farms and marine protected areas: a United Kingdom perspective” in Offshore energy and marine spatial planning. eds. K. L. Yates and C. J. A. Bradshaw (London, UK: Routledge), 246–259.
Ashley, M., Mangi, S., and Rodwell, L. D. (2014). The potential of offshore windfarms to act as marine protected areas–a systematic review of current evidence. Mar. Policy 45, 301–309. doi: 10.1016/j.marpol.2013.09.002
AtSeaNova (2020). Projects - Weir & Wind. Available at” https://atseanova.com/projects/ [Accessed December 2, 2020].
Banach, J. L., van den Burg, S. W. K., and van der Fels-Klerx, H. J. (2020). Food safety during seaweed cultivation at offshore wind farms: An exploratory study in the North Sea. Mar. Policy 120:104082. doi: 10.1016/j.marpol.2020.104082
Beck, M. W., Brumbaugh, R. D., Airoldi, L., Carranza, A., Coen, L. D., Crawford, C., et al. (2011). Oyster reefs at risk and recommendations for conservation, restoration, and management. Bioscience 61, 107–116. doi: 10.1525/bio.2011.61.2.5
BEIS (2020). Offshore wind sector Deal. Available at” https://www.gov.uk/government/publications/offshore-wind-sector-deal/offshore-wind-sector-deal [Accessed March 22, 2021].
Benassai, G., Mariani, P., Stenberg, C., and Christoffersen, M. (2014). A sustainability index of potential co-location of offshore wind farms and open water aquaculture. Ocean Coast. Manag. 95, 213–218. doi: 10.1016/j.ocecoaman.2014.04.007
Bennett, J. M., Calosi, P., Clusella-Trullas, S., Martinez, B., Agar, A. C., Araujo, M. B., et al. (2018). GlobTherm, a global database on thermal tolerances for aquatic and terrestrial organisms. Sci. Data 5:180022. doi: 10.1038/sdata.2018.22
Bennun, L., van Bochove, J., Ng, C., Fletcher, C., Wilson, D., Phair, N., and Carbone, G. (2021) Mitigating biodiversity impacts associated with solar and wind energy development. Guidelines for project developers. Gland, Switzerland: IUCN and Cambridge, UK: The Biodiversity Consultancy.
Berx, B., and Hughes, S. L. (2009). Climatology of surface and near-bed temperature and salinity on the north-west European continental shelf for 1971-2000. Cont. Shelf Res. 29, 2286–2292. doi: 10.1016/j.csr.2009.09.006
Brennan, C. E., Blanchard, H., and Fennel, K. (2016). Putting temperature and oxygen thresholds of marine animals in context of environmental change: a regional perspective for the Scotian shelf and gulf of St. Lawrence. PLoS One 11:e0167411. doi: 10.1371/JOURNAL.PONE.0167411
Buck, B. H., Berg-Pollack, A., Assheuer, J., Zielinski, O., and Kassen, D. (2008) “Technical realization of extensive aquaculture constructions in offshore wind farms: consideration of the mechanical loads,” in International conference on offshore mechanics and Arctic engineering. Hamburg, Germany. pp. 691–697.
Buck, B. H., Nevejan, N., Wille, M., Chambers, M. D., and Chopin, T. (2017). “Offshore and multi-use aquaculture with extractive species: seaweeds and bivalves” in Aquaculture perspective of multi-use sites in the Open Ocean: The untapped potential for marine resources in the Anthropocene. eds. B. H. Buck and R. Langan (Switzerland: Springer).
CABI (2022) ‘Magallana gigas (Pacific oyster)’, Invasive species compendium: Detailed coverage of invasive species threatening livelihoods and the environment worldwide. Available at: https://www.cabi.org/isc/datasheet/87296 [Accessed March 26, 2023].
Carr, M. H., Robinson, S. P., Wahle, C., Davis, G., Kroll, S., Murray, S., et al. (2017). The central importance of ecological spatial connectivity to effective coastal marine protected areas and to meeting the challenges of climate change in the marine environment. Aquat. Conserv. Mar. Freshwat. Ecosyst. 27, 6–29. doi: 10.1002/aqc.2800
Carss, D. N., Brito, A. C., Chainho, P., Ciutat, A., de Montaudouin, X., Fernández Otero, R. M., et al. (2020). Ecosystem services provided by a non-cultured shellfish species: the common cockle Cerastoderma edule. Mar. Environ. Res. 158:104931. doi: 10.1016/j.marenvres.2020.104931
Catalano, A. S., Lyons-White, J., Mills, M. M., and Knight, A. T. (2019). Learning from published project failures in conservation. Biol. Conserv. 238:108223. doi: 10.1016/j.biocon.2019.108223
Cefas (2021). Cefas data hub. Available at: http://data.cefas.co.uk/ [Accessed April 1, 2021].
Channel Coastal Observatory (2020). Regional coastal monitoring programmes. Available at https://www.channelcoast.org/realtimedata/ [Accessed March 22, 2021].
Chen, L., and Lam, W. H. (2014). “Methods for predicting seabed scour around marine current turbine” in Renewable and sustainable Energy reviews Vol 29. ed. A. Foley (Amsterdam: Elsevier), 683–692.
Christie, N., Smyth, K., Barnes, R., and Elliott, M. (2014). Co-location of activities and designations: a means of solving or creating problems in marine spatial planning? Mar. Policy 43, 254–261. doi: 10.1016/j.marpol.2013.06.002
Coen, L. D., Brumbaugh, R. D., Bushek, D., Grizzle, R., Luckenbach, M. W., Posey, M. H., et al. (2007). Ecosystem services related to oyster restoration. Mar. Ecol. Prog. Ser. 341, 303–307. doi: 10.3354/meps341303
Colsoul, B., Boudry, P., Perez-Paralle, M. L., Cetinic, A. B., Hugh-Jones, T., Arzul, I., et al. (2021). Sustainable large-scale production of European flat oyster(Ostrea edulis) seed for ecological restoration andaquaculture: a review. Rev. Aquac. 13, 1423–1468. doi: 10.1111/raq.12529
Coolen, J. W. P., Lengkeek, W., van der Have, T., and Bittner, O. (2019). Upscaling positive effects of scour protection in offshore wind farms: Quick scan of the potential to upscale positive effects of scour protection on benthic macrofauna and associated fish species. Netherlands: Wageningen Marine Research.
Cranfield, H. I., and Allen, R. L. (1977). Fertility and larval production in an unexploited population of oysters, Ostrea lutaria Hutton, from Foveaux Strait. N. Z. J. Mar. Freshw. Res. 11, 239–253. doi: 10.1080/00288330.1977.9515675
Day, A. H., Babarit, A., Fontaine, A., He, Y. P., Kraskowski, M., Murai, M., et al. (2015). Hydrodynamic modelling of marine renewable energy devices: a state of the art review. Ocean Eng. 108, 46–69. doi: 10.1016/j.oceaneng.2015.05.036
deCastro, M., Salvador, S., Gómez-Gesteira, M., Costoya, X., Carvalho, D., Sanz-Larruga, F. J., et al. (2019). “Europe, China and the United States: three different approaches to the development of offshore wind energy” in Renewable and sustainable energy reviews Vol. 113. ed. A. Foley (Amsterdam: Elsevier Ltd), 55–70.
DEFRA (2021). Defra data services platform. Available at: https://environment.data.gov.uk/ [Accessed April 1, 2021].
DHI (2017). MIKE 21 flow model: Hydrodynamic module, user guide Available at: https://manuals.mikepoweredbydhi.help/2017/MIKE_21.htm [Accessed March 22, 2021].
Di Tullio, G. R., Mariani, P., Benassai, G., Di Luccio, D., and Grieco, L. (2018). Sustainable use of marine resources through offshore wind and mussel farm co-location. Ecol. Model. 367, 34–41. doi: 10.1016/j.ecolmodel.2017.10.012
Didderen, K., Lengkeek, W., Bergsma, J. H., Dongen, U.Van, Driessen, F. M. F., and Kamermans, P. (2020). ‘WWF & ARK Borkum reef ground oyster pilot: Active restoration of native oysters in the North Sea - monitoring September 2019’. Bureau Waardenburg. Available at: https://research.wur.nl/en/publications/wwf-amp-ark-borkum-reef-ground-oyster-pilot-active-restoration-of [Accessed March 19, 2021].
Didderen, K., Lengkeek, W., Kamermans, P., Deden, B., and Reuchlin-Hugenholtz, E. (2018). Pilot to actively restore native oyster reefs in the North Sea comprehensive report to share lessons learned in 2018 Available at: https://www.ark.eu/sites/default/files/media/Schelpdierbanken/Report_Borkumse_Stenen.pdf [Accessed March 22, 2021].
Durakovic, A. (2020). Norther to double as seaweed farm. Available at: https://www.offshorewind.biz/2020/07/15/norther-to-double-as-seaweed-farm/ [Accessed March 22, 2021].
Dutertre, M., Beninger, P. G., Barillé, L., Papin, M., and Haure, J. (2010). Rising water temperatures, reproduction and recruitment of an invasive oyster, Crassostrea gigas, on the French Atlantic coast. Mar. Environ. Res. 69, 1–9. doi: 10.1016/j.marenvres.2009.07.002
Eigaard, O. R., Bastardie, F., Breen, M., Dinesen, G. E., Hintzen, N. T., Laffargue, P., et al. (2015). Estimating seabed pressure from demersal trawls, seines, and dredges based on gear design and dimensions. ICES J. Mar. Sci. J. Cons. 73, i27–i43. doi: 10.1093/icesjms/fsv099
EMODNET-chemistry (2021). ‘European seas - DIVAnd 4D monthly analysis of water body chlorophyll-a 1960/2020 v2021’. Available at: https://www.emodnet-chemistry.eu/products/catalogue#/metadata/cb754d88-ad3a-11eb-3167-0ff42881d67d [Accessed March 25, 2023].
ENORI (2021). ENORI: Essex native oyster restoration initiative. Available at: https://essexnativeoyster.com/ [Accessed March 22, 2021].
EU Habitat’s Directive (1992). Council directive 92/43/EEC of 21 may 1992 on the conservation of natural habitats and of wild fauna and flora. Available at: https://eur-lex.europa.eu/legal-content/EN/TXT/?uri=CELEX:31992L0043 [Accessed April 2, 2021].
European Commission (2020). Energy - onshore and offshore wind. Available at: https://ec.europa.eu/energy/topics/renewable-energy/onshore-and-offshore-wind_en [Accessed March 22, 2021].
Fariñas-Franco, J. M., Pearce, B., Mair, J. M., Harries, D. B., MacPherson, R. C., Porter, J. S., et al. (2018). Missing native oyster (Ostrea edulis L.) beds in a European marine protected area: should there be widespread restorative management? Biol. Conserv. 221, 293–311. doi: 10.1016/j.biocon.2018.03.010
Ferguson, R. I., and Church, M. (2004). A simple universal equation for grain settling velocity. J. Sediment. Res. 74, 933–937. doi: 10.1306/051204740933
Folk, R. L. (1954). The distinction between grain size and mineral composition in sedimentary-rock nomenclature. J. Geol. 62, 344–359. doi: 10.1086/626171
Fragkopoulou, E., Serrão, E. A., Horta, P. A., Koerich, G., and Assis, J. (2021). Bottom trawling threatens future climate refugia of Rhodoliths globally. Front. Mar. Sci. 7:1246. doi: 10.3389/fmars.2020.594537
Gimpel, A., Stelzenmüller, V., Grote, B., Buck, B. H., Floeter, J., Núñez-Riboni, I., et al. (2015). A GIS modelling framework to evaluate marine spatial planning scenarios: co-location of offshore wind farms and aquaculture in the German EEZ. Mar. Policy 55, 102–115. doi: 10.1016/j.marpol.2015.01.012
Gintautas, T., Sørensen, J. D., and Vatne, S. R. (2016). “Towards a risk-based decision support for offshore wind turbine installation and operation & maintenance” in Energy Procedia Vol 94. eds. J. O. G. Tande, T. Kvamsdal and M. Muskulus (Amsterdam: Elsevier Ltd), 207–217.
Haelters, J., and Kerckhof, F. (2009). ‘Background document for Ostrea edulis and Ostrea edulis beds.’, in Biodiversity series, OSPAR convention.
Harding, S., Nelson, L., and Glover, T. (2016). Solent oyster restoration project. Available at: www.bluemarinefoundation.comwww.bluemarinefoundation.com [Accessed March 22, 2021].
Helmer, L., Farrell, P., Hendy, I., Harding, S., Robertson, M., and Preston, J. (2019, 2019). Active management is required to turn the tide for depleted Ostrea edulis stocks from the effects of overfishing, disease and invasive species. PeerJ 2:e6431. doi: 10.7717/peerj.6431
Herbert, R. J. H., Willis, J., Jones, E., Ross, K., Hübner, R., Humphreys, J., et al. (2012). Invasion in tidal zones on complex coastlines: modelling larvae of the non-native Manila clam, Ruditapes philippinarum, in the UK. J. Biogeogr. 39, 585–599. doi: 10.1111/j.1365-2699.2011.02626.x
Holmgren, D. (2002). Permaculture principles & pathways beyond sustainability. Holmgren Design Services, Hepburn, Australia. Available at: www.permaculture.co.uk [Accessed April 1, 2021].
IPCC (2019). Special report on the ocean and cryosphere in a changing climate. Edited by H. O. Pörtner, D. C. Roberts, V. Masson-Delmotte, P. Zhai, M. Tignor, E. Poloczanska, K. Mintenbeck, A. Alegría, M. Nicolai, A. Okem, J. Petzold, B. Rama, and N. M. Weyer. Available at: https://www.ipcc.ch/srocc/ [Accessed March 22, 2021].
Jansen, H. M., Tonk, L., Werd, A. V. D., Meer, I. V. D., Van Tuinen, S., Burg, S. V. D., et al. (2018). Development of offshore seaweed cultivation: Food safety, cultivation, ecology and economy doi: 10.18174/470706.
JNCC (2021). JNCC Open Data. Available at: https://data.jncc.gov.uk/ [Accessed April 1, 2021].
Kamermans, P. (2018) European flat oysters back in the North Sea - spotlight, Wageningen university & research. Available at: https://weblog.wur.eu/spotlight/european-flat-oysters-back-in-the-north-sea/ [Accessed March 22, 2021].
Kamermans, P., Walles, B., Kraan, M., van Duren, L. A., Kleissen, F., van der Have, T. M., et al. (2018). Offshore wind farms as potential locations for flat oyster (Ostrea edulis) restoration in the Dutch North Sea. Sustainability 10, 308–331. doi: 10.3390/su10113942
Kautsky, N. (1982). Growth and size structure in a Baltic Mytilus edulis population. Mar. Biol. 68, 117–133. doi: 10.1007/BF00397599
Kenyon, N. H., and Cooper, B. (2005) Sand banks, sand transport and offshore wind farms. Available at https://www.researchgate.net/publication/285584613_Sand_banks_sand_transport_and_offshore_wind_farms#fullTextFileContent. [Accessed March 22, 2021].
Khangaonkar, T., and Yang, Z. (2011). High-resolution hydrodynamic model of puget sound to support nearshore restoration feasibility analysis and design. Ecol. Restor. 29, 173–184. doi: 10.3368/er.29.1-2.173
Knights, A. M., Crowe, T. P., and Burnell, G. (2006). Mechanisms of larval transport: vertical distribution of bivalve larvae varies with tidal conditions. Mar. Ecol. Prog. Ser. 326, 167–174. doi: 10.3354/MEPS326167
Krakau, M., Jacobsen, S., Kurt Jensen, T., and Reise, K. (2011). The cockle Cerastoderma edule at Northeast Atlantic shores: Genetic signatures of glacial refugia doi: 10.1007/s00227-011-1802-8.
Kremen, C., and Miles, A. (2012). Ecosystem Services in Biologically Diversified versus conventional farming systems: benefits, externalities, and trade-offs. Ecol. Soc. 17, 1–25. doi: 10.5751/ES-05035-170440
Kwon, J., Jung, R., Kang, Y., An, K., and Lee, W. (2005). Environmental management of marine cage fish farms using numerical modelling. Sea 10, 181–195.
Kyriazi, Z., Lejano, R., Maes, F., and Degraer, S. (2015). Bargaining a net gain compensation agreement between a marine renewable energy developer and a marine protected area manager. Mar. Policy 60, 40–48. doi: 10.1016/J.MARPOL.2015.06.005
Kyriazi, Z., Maes, F., and Degraer, S. (2016). Coexistence dilemmas in European marine spatial planning practices. The case of marine renewables and marine protected areas. Energy Policy 97, 391–399. doi: 10.1016/J.ENPOL.2016.07.018
Labarta, U., Fernández-Reiriz, M. J., and Pérez-Camacho, A. (1999) ‘Larvae of Ostrea edulis (L.) during starvation: growth, energy and biochemical substrates’, Hydrobiologia, 405(0), pp. 125–131. doi:doi: 10.1023/A:1003851220515.
Lacroix, D., and Pioch, S. (2011). The multi-use in wind farm projects: more conflicts or a win-win opportunity? Aquat. Living Resour. 24, 129–135. doi: 10.1051/ALR/2011135
Laing, I., and Spencer, B. E. (2006). Bivalve cultivation: Criteria for selecting a site science series technical report no.136. Available at: www.hmso.gov.uk/copyright/licences/core/core_ [Accessed March 22, 2021].
Laing, I., Walker, P., and Areal, F. (2006). Aquatic living resources review return of the native-is European oyster (Ostrea edulis) stock restoration in the UK feasible? Aquat. Living Resour. 19, 283–287. doi: 10.1051/alr:2006029
Lengkeek, W., Didderen, K., Teunis, M., Driessen, F., Coolen, J. W. P., Bos, O. G., Vergouwen, S. A., Raaijmakers, T., Vries, M. B.De, and Koningsveld, M.Van (2017). ‘Eco-friendly design of scour protection: Potential enhancement of ecological functioning in offshore wind farms: Towards an implementation guide and experimental set-up’. Bureau Waardenburg. Available at: https://research.wur.nl/en/publications/eco-friendly-design-of-scour-protection-potential-enhancement-of- [Accessed February 21, 2021].
Lu, L., Li, Y., and Qin, J. (2005). Numerical simulation of the equilibrium profile of local scour around submarine pipelines based on renormalized group turbulence model. Ocean Eng. 32, 2007–2019. doi: 10.1016/j.oceaneng.2005.04.004
Maathuis, M. A. M., Coolen, J. W. P., van der Have, T., and Kamermans, P. (2020). Factors determining the timing of swarming of European flat oyster (Ostrea edulis L.) larvae in the Dutch Delta area: implications for flat oyster restoration. J. Sea Res. 156:101828. doi: 10.1016/j.seares.2019.101828
Mangor, K. (2004). Shoreline Management Guidelines. Hørshølm, Denmark: DHI Water & Environment. Available at: https://www.scirp.org/(S(czeh2tfqyw2orz553k1w0r45))/reference/ReferencesPapers.aspx?ReferenceID=1455971 [Accessed April 1, 2021].
Manning, A. J., Baugh, J. V., Spearman, J. R., and Whitehouse, R. J. S. (2010). “Flocculation settling characteristics of mud: sand mixtures” in Ocean dynamics Vol 49. ed. T. Ezer (Amsterdam: Springer), 237–253.
Marshall, C., and Wilson, E. (2008). “Great scallop (Pecten maximus)” in Marine life information network: Biology and sensitivity key information reviews. eds. H. Tyler-Walters and K. Hiscock (Plymouth: Marine Biological Association of the United Kingdom)
McLeod, I. M., Zu Ermgassen, P. S. E., Gillies, C. L., Hancock, B., Humphries, A., Westby, S. R., et al. (2019). “Can bivalve habitat restoration improve degraded estuaries?” in Coasts and estuaries: The future. eds. E. Eric Wolanski, J. W. Day, M. Elliott and R. Ramachandran (Amsterdam: Elsevier), 427–442.
Mcmanus, B. (2010). An integral framework for permaculture. J. Sust. Dev. 3, 162–174. doi: 10.5539/jsd.v3n3p162
MEDIN (2021). Medin Portal. Available at: https://portal.medin.org.uk/portal/start.php [Accessed April 1, 2021].
Michler-Cieluch, T., Krause, G., and Buck, B. H. (2009). Reflections on integrating operation and maintenance activities of offshore wind farms and mariculture. Ocean Coast. Manag. 52, 57–68. doi: 10.1016/j.ocecoaman.2008.09.008
Natural England (2020). Marine conservation zones (England). Available at: https://naturalengland-defra.opendata.arcgis.com/search [Accessed March 12, 2021].
Natural England (2021). Natural England open data geoportal. Available at: https://naturalengland-defra.opendata.arcgis.com/ [Accessed April 1, 2021].
Noordzeeloket (2020). North Sea agreement. Available at: https://www.noordzeeloket.nl/en/policy/north-sea-agreement/ [Accessed March 22, 2021].
North, E., Schlag, Z., Hood, R., Li, M., Zhong, L., Gross, T., et al. (2008). Vertical swimming behavior influences the dispersal of simulated oyster larvae in a coupled particle-tracking and hydrodynamic model of Chesapeake Bay. Mar. Ecol. Prog. Ser. 359, 99–115. doi: 10.3354/meps07317
OFL (2020). The North Sea agreement. Available at: https://www.noordzeeloket.nl/publish/pages/184533/the_north_sea_agreement.pdf [Accessed December 2, 2020].
Ørsted (2021). Our offshore windfarms. Available at: https://orsted.com/en/our-business/offshore-wind/our-offshore-wind-farms [Accessed February 13, 2021].
Pogoda, B. (2019). Current status of European oyster decline and restoration in Germany. Humanities 8:9. doi: 10.3390/h8010009
Pronker, A. E., Peene, F., Donner, S., Wijnhoven, S., Geijsen, P., Bossier, P., et al. (2015). Hatchery cultivation of the common cockle (Cerastoderma edule L.): from conditioning to grow-out. Aquac. Res. 46, 302–312. doi: 10.1111/are.12178
Quitzow, R., Thielges, S., Goldthau, A., Helgenberger, S., and Mbungu, G. (2019). ‘Advancing a global transition to clean energy: the role of international cooperation’, economics: the open-access. Open-Assess. E-J. 13, 1–18. doi: 10.5018/economics-ejournal.ja.2019-48
Rendle, E. J. (2020). Feasibility study for native oyster restoration in a UK wind farm; to determine the suitability of Gunfleet Sands as a potential site for broodstock native oysters. Available at: https://resilientcoasts.com [Accessed March 25, 2023].
Rendle, E. J., and Rodwell, L. D. (2014). Artificial surf reefs: a preliminary assessment of the potential to enhance a coastal economy. Mar. Policy 45, 349–358. doi: 10.1016/j.marpol.2013.09.004
Rico-Villa, B., Pouvreau, S., and Robert, R. (2009). Influence of food density and temperature on ingestion, growth and settlement of Pacific oyster larvae, Crassostrea gigas. Aquaculture 287, 395–401. doi: 10.1016/j.aquaculture.2008.10.054
Robertson, M., Locke, S., Uttley, M., and Kean-Hammerson, J. (2020). Exploring the role of offshore wind in restoring priority marine habitats case study: Opportunities for native oyster (Ostrea edulis) restoration at the Gunfleet Sands offshore wind farm. Available at: https://www.bluemarinefoundation.com/wp-content/uploads/2021/01/BLUE-wind-farm-feasibility-study-report-FINAL.pdf [Accessed March 22, 2021].
Rodriduez-Perez, A., Sanderson, W. G., Moller, L. F., Henry, T. B., and James, M. (2020). “Return to sender: the influence of larval behaviour on the distribution and settlement of the European oyster Ostrea edulis” in Aquatic conservation: Marine and freshwater Ecosystems. Volume 30, issue 11: unlocking the blueprint for native oyster restoration in europe. eds. P. J. Boon and J. M. Baxter (New Jersey, US: John Wiley & Sons, Ltd)
Rodrigues, S., Restrepo, C., Kontos, E., Teixeira Pinto, R., and Bauer, P. (2015). “Trends of offshore wind projects” in Renewable and sustainable Energy reviews Vol 49. ed. A. Foley (Amsterdam: Elsevier Ltd), 1114–1135.
Royal Haskoning DHV (2020). Norfolk vanguard offshore wind farm habitats regulations derogation, provision of evidence appendix 3-Haisborough, Hammond and Winterton special area of conservation (SAC)-in principle compensation measures. Available at: https://group.vattenfall.com/uk/contentassets/e0aaa63956ad4fbf90788962b444e205/8.25-appendix-3-hhw-sac-in-principle-compensatory-measures.pdf [Accessed March 22, 2021].
RPS Group Plc (2005). Environmental Statement, vol. 1. London, UK: Offshore Works, London Array Limited.
RPS Group PLC (2015). Environmental statement. Non-technical summary - Hornsea offshore WindFarm: Project two. Available at: https://infrastructure.planninginspectorate.gov.uk/wp-content/ipc/uploads/projects/EN010053/EN010053-000321-7.1.0.a%20Non-technical%20Summary.pdf [Accessed March 25, 2023].
RSK Environment Ltd. (2002). Barrow offshore wind farm environmental impact statement. Warwick Energy Limited.
Schupp, M. F., Bocci, M., Depellegrin, D., Kafas, A., Kyriazi, Z., Lukic, I., et al. (2019). ‘Toward a common understanding of ocean multi-use’, Frontiers in marine. Science 6:165. doi: 10.3389/FMARS.2019.00165
SeaScape Energy Ltd. (2002). Burbo offshore environmental statement. Centre for Marine and Coastal Studies.
Seddon, N., Chausson, A., Berry, P., Girardin, C. A., and Smith, A. (2020). Understanding the value and limits of nature-based solutions to climate change and other global challenges. Philos. Trans. R. Soc. B 375:20190120. doi: 10.1098/rstb.2019.0120
Seyr, H., and Muskulus, M. (2019). Decision support models for operations and maintenance for offshore wind farms: a review. Appl. Sci. 9, 1–30. doi: 10.3390/app9020278
Shumway, S. E., and Parsons, G. J. (2011). Scallops: Biology, ecology and aquaculture. 2nd Edn. Amsterdam: Elsevier.
Smaal, A. C., Ferreira, J. G., Grant, J., Petersen, J. K., and Strand, Ø. (2019). Goods and services of marine bivalves. Cham, Switzerland: Springer International Publishing.
Smaal, A., Kamermans, P., Kleissen, F., Van Duren, L., and Van Der Have, T. (2017). ‘Flat oysters on offshore wind farms opportunities for the development of flat oyster populations on existing and planned wind farms in the Dutch section of the North Sea’.
Smaal, A. C., Vonck, A. P. M. A., and Bakker, M. (1997). Seasonal variation in physiological energetics of Mytilus Edulis and Cerastoderma Edule of different size classes. J. Mar. Biol. Assoc. U. K. 77, 817–838. doi: 10.1017/s0025315400036213
Smyth, D., Kregting, L., Elsäßer, B., Kennedy, R., and Roberts, D. (2016). Using particle dispersal models to assist in the conservation and recovery of the overexploited native oyster (Ostrea edulis) in an enclosed sea lough. J. Sea Res. 108, 50–59. doi: 10.1016/j.seares.2015.12.009
Soto, D. (2009). “Integrated mariculture: A global review” in Food and agriculture Organization of the United Nations. ed. D. Soto (Rome, Italy: FAO)
Soulsby, R. L., and Whitehouse, R. J. S. (1997). ‘Threshold of sediment Motion in coastal environments’, in Pacific Coasts and Ports 97: Proceedings of the 13th Australasian Coastal and Ocean Engineering Conference and the 6th Australasian Port and Harbour Conference. Available at: https://search.informit.org/doi/10.3316/INFORMIT.929741720399033 [Accessed April 1, 2021].
Stokes, G. G. (1851). On the effect of the internal friction of fluids on the motion of pendulums, transactions of the cambridge philosophical society. Available at: http://www.nawcc-index.net/Articles/Stokes-InternalFriction.pdf [Accessed April 1, 2021].
Strohmeier, T. (2009). Feeding behavior and bioenergetic balance of the great scallop (Pecten maximus) and the blue mussel (Mytilus edulis) in a low seston environment and relevance to suspended shellfish aquaculture. University of Bergen. Available at: https://bora.uib.no/bora-xmlui/bitstream/handle/1956/3713/Dr.thesis_ToreStrohmeier.pdf?sequence=1&isAllowed=y [Accessed March 22, 2023].
Sumer, B. M., Whitehouse, R. J. S., and Tørum, A. (2001). “Scour around coastal structures: a summary of recent research” in Coastal engineering Vol 44. eds. I. J. Losada, E. W. Bijker and H. F. Burcharth (Amsterdam: Elsevier), 153–190.
The Crown Estate (2020). Offshore wind site agreements (England, Wales & NI), the crown estate. Available at: https://opendata-thecrownestate.opendata.arcgis.com/datasets/thecrownestate::offshore-wind-site-agreements-england-wales-ni-the-crown-estate [Accessed March 22, 2021].
The Nature Conservancy and Inspire Environmental (2021). Turbine reefs: Nature-based designs for augmenting offshore wind structures in the United States. Available at: https://www.nature.org/content/dam/tnc/nature/en/documents/TurbineReefs_Nature-BasedDesignsforOffshoreWind_FinalReport_Nov2021.pdf [Accessed 25 March, 2023].
Thomas, T. J., and Dwarakish, G. S. (2015). Numerical wave modelling – a review. Aqua. Proc. 4, 443–448. doi: 10.1016/j.aqpro.2015.02.059
Tyler-Walters, H. (2007). ‘Common cockle (Cerastoderma edule)’, in marine life information network: Biology and sensitivity key information reviews. Plymouth: Marine Biological Association of the United Kingdom.
Tyler-Walters, H. (2008). “Mytilus edulis Common mussel” in Marine life information network: Biology and sensitivity key information reviews. eds. H. Tyler-Walters and K. Hiscock (Plymouth: Marine Biological Association of the United Kingdom)
UKHO (2020). Admiralty EasyTide, the United Kingdom hydrographic office admiralty EasyTide. Available at: http://www.ukho.gov.uk/easytide/EasyTide/index.aspx [Accessed August 26, 2020].
van Bussel, G. J. W., and Bierbooms, W. A. A. M. (2003). The DOWEC offshore reference windfarm: analysis of transportation for operation and maintenance. Wind Eng. 27, 381–391. doi: 10.1260/030952403322770986
van den Burg, S. W. K., Röckmann, C., Banach, J. L., and van Hoof, L. (2020). Governing risks of multi-use: seaweed aquaculture at offshore wind farms. Front. Mar. Sci. 7:60. doi: 10.3389/fmars.2020.00060
van den Burg, S. W. K., van Duijn, A. P., Bartelings, H., van Krimpen, M. M., and Poelman, M. (2016). The economic feasibility of seaweed production in the North Sea. Aquac. Econ. Manag. 20, 235–252. doi: 10.1080/13657305.2016.1177859
Venugopal, V., and Nemalidinne, R. (2015). Wave resource assessment for Scottish waters using a large scale North Atlantic spectral wave model. Renew. Energy 76, 503–525. doi: 10.1016/j.renene.2014.11.056
Vincent, A., Stanley, A., and Ring, J. (2020). Seaweed as a growth engine for a sustainable European future Available at: https://www.seaweedeurope.com/wp-content/uploads/2020/10/Seaweed_for_Europe-Hidden_Champion_of_the_ocean-Report.pdf [Accessed March 22, 2021].
Wang, Z., Leung, K. M. Y., Sung, Y.-H., Dudgeon, D., and Qiu, J.-W. (2021). Recovery of tropical marine benthos after a trawl ban demonstrates linkage between abiotic and biotic changes. Commun. Biol. 4:212. doi: 10.1038/s42003-021-01732-y
Whitehouse, R. (1998) Scour at marine structures: A manual for practical applications. London: Thomas Telford Publications.
Wilson, J. H. (1987). ‘Temporal and spatial distribution of Ostrea edulis larvae in Kilkieran’, Irish fisheries investigations, series B, 29. Available at: https://oar.marine.ie/handle/10793/316 [Accessed March 22, 2021].
Wilson, J. C., and Elliott, M. (2009). The habitat-creation potential of offshore wind farms. Wind Energy 12, 203–212. doi: 10.1002/we.324
WWF European Policy Office (2021). ‘Nature protection and offshore renewable energy in the European Union - position paper’, Position Paper. Available at: https://wwfeu.awsassets.panda.org/downloads/wwf_epo_position_paper_offshore_renewable_energy_and_nature.pdf [Accessed 25 March, 2023].
Keywords: restoration, mariculture, hydrodynamic modelling, renewable energy, nature-based solutions, conservation, engineering with nature
Citation: Rendle EJ, Hunt EL and Bicknell AWJ (2023) A three-step approach for co-locating nature-based solutions within offshore wind farms. Front. Ecol. Evol. 11:690382. doi: 10.3389/fevo.2023.690382
Edited by:
Ana B. Bugnot, Commonwealth Scientific and Industrial Research Organisation (CSIRO), AustraliaReviewed by:
Chris Hauton, University of Southampton, United KingdomMaria Vozzo, Commonwealth Scientific and Industrial Research Organisation (CSIRO), Australia
Copyright © 2023 Rendle, Hunt and Bicknell. This is an open-access article distributed under the terms of the Creative Commons Attribution License (CC BY). The use, distribution or reproduction in other forums is permitted, provided the original author(s) and the copyright owner(s) are credited and that the original publication in this journal is cited, in accordance with accepted academic practice. No use, distribution or reproduction is permitted which does not comply with these terms.
*Correspondence: Emma Jane Rendle, emma.rendle@resilientcoasts.com