- 1Department of Neurology, The Second Xiangya Hospital, Central South University, Changsha, China
- 2National Health Commission Key Laboratory of Birth Defect for Research and Prevention, Hunan Provincial Maternal and Child Health Care Hospital, Changsha, China
Studies have demonstrated the potential of mesenchymal stem cell (MSC) administration to promote functional recovery in preclinical studies of multiple sclerosis (MS), yet the effects of MSCs on remyelination are poorly understood. We wished to evaluate the therapeutic effects of MSCs on functional and histopathological outcomes in MS; therefore, we undertook an updated systematic review and meta-analysis of preclinical data on MSC therapy for MS. We searched mainstream databases from inception to July 15, 2021. Interventional studies of therapy using naïve MSCs in in vivo rodent models of MS were included. From each study, the clinical score was extracted as the functional outcome, and remyelination was measured as the histopathological outcome. Eighty-eight studies published from 2005 to 2021 met the inclusion criteria. Our results revealed an overall positive effect of MSCs on the functional outcome with a standardized mean difference (SMD) of −1.99 (95% confidence interval (CI): −2.32, −1.65; p = 0.000). MSCs promoted remyelination by an SMD of −2.31 (95% CI: −2.84, −1.79; p = 0.000). Significant heterogeneity among studies was observed. Altogether, our meta-analysis indicated that MSC administration improved functional recovery and promoted remyelination prominently in rodent models of MS.
Introduction
Multiple sclerosis (MS) is a devastating disease that presents in young adults and can cause progressive physical disability and cognitive impairment (1). The etiology of MS is not known, but its pathophysiology has been reported to be associated with the formation of autoreactive lymphocytes and antigen-presenting cells in the body. The inflammatory cytokines generated by these cells in turn promote the recruitment of immune cells in the central nervous system (CNS), which destroy myelin sheaths and axon neurons, contributing to inflammation, demyelination, and massive loss of neurons in the CNS (2).
MS comprises mainly a relapsing–remitting phase and a progressive phase (3). Better understanding regarding the mechanisms of relapsing–remitting MS (RRMS) has led to the development of several disease-modifying therapeutic regimens that reduced the frequency and severity of acute relapses through direct action on the immune system, while progression in the treatment of progressive MS (PMS) remains relatively lacking (4). In general, the exploitation regarding new treatment options that promote favorable CNS repair in MS will be of great significance and is promising.
Potent immunomodulatory properties and pleiotropic effects have made mesenchymal stem cell (MSC) candidates for MS treatment (5–8). Abundant data have indicated that transplantation of MSCs may be efficacious in animal models of MS. Studies have shown that MSCs can induce autoantigen immunotolerance, enhance remyelination, maintain and remodel axons, and promote functional improvement in animal models of MS (9). Conversely, other studies have reported that MSC administration did not ameliorate the neurological deficit of MS in animal models (10, 11). These differences may be related to the cell type, transplantation route, and dose of MSCs.
As the questions about the optimal pattern of MSC therapy in MS remain unanswered, the analysis that evaluates the overall therapeutic effect of MSCs in animal models of MS among different experimental conditions will be prospective. And such analysis is also necessary for the guidance of clinical translation. A recent meta-analysis by Yang and colleagues demonstrated the functional promotion effects of MSC transplantation in the experimental autoimmune encephalomyelitis (EAE) model of MS, but the database was only until October 1, 2017 (12). In addition, the meta-analysis that investigates the histopathological efficiency of MSC transplantation for MS is also lacking. Myelin repair-promoting therapy has been recognized as a well-acknowledged approach to prevent disease progression of MS, and myelin regeneration is increasingly measured as the structure outcomes of several studies (13–15). Thus, remyelination was measured as the histopathological outcome in our meta-analysis.
The aim of our study was to provide evidence relating to the therapeutic effects of MSCs on the functional and histopathological outcomes in rodent models of MS, through performing an updated systematic review and meta-analysis.
Materials and Methods
The present meta-analysis followed the guidelines of the Preferred Reporting Items for Systematic Reviews and Meta-Analyses (PRISMA) checklist (Supplementary Table 1) (16).
Search Strategy
We searched PubMed, Embase, and Web of Science databases from inception to July 15, 2021, using the following search strategy: (“mesenchymal stem cells” OR “mesenchymal stromal cells” OR “mesenchymal stem cell” OR “mesenchymal stromal cell”) AND (“Multiple sclerosis” OR “MS” OR “Experimental Autoimmune Encephalomyelitis” OR “Experimental Allergic Encephalomyelitis”). Besides, the reference lists of eligible studies were also reviewed to identify other relevant articles.
Inclusion and Exclusion Criteria
The eligibility criteria were set up according to the PICOS scheme (population, intervention, control, outcome and study design) (17). Studies were included if they met the following criteria: i) a rodent model of MS was induced; ii) the effect of unmodified MSCs was tested in at least one experimental group; iii) studies provided adequate data on clinical scores or remyelination; iv) experimental studies were presented in original research and published in peer-reviewed journals; and v) studies are published in English.
The exclusion criteria were as follows: i) studies that did not involve in vivo testing; ii) the outcome did not include the clinical score or remyelination; iii) cells were administered before the animal model induction; iv) studies that used only extracellular vesicles (EVs), conditioned medium derived from MSCs, or differentiated MSCs; and v) studies that reported sample size incompletely.
Study Selection
After removal of duplicated data, two independent investigators screened titles and abstracts for inclusion. Non-relevant studies were excluded if the two researchers agreed. Those deemed “potentially relevant studies” were recorded, and the full text was acquired. Then, the two researchers screened the full-text articles independently to evaluate the final eligibility according to the inclusion and exclusion criteria stated above. Disagreement was addressed by discussion with a third investigator, if needed.
Data Collection
The following items from the eligible studies were extracted and documented independently by two investigators: general information (first author, publication year, and country); experimental methods (numbers of animals per group for individual comparisons; species and strain of animals; gender; methods of MS induction in the animal model; sources and types of MSCs; dose of MSCs; delivery route of MSCs; time of administration [days post immunization (DPI)]; duration of follow-up; functional outcome (clinical score); and histopathological outcome (remyelination).
Data regarding the mean and standard deviation (SD) of a particular parameter from the MSC-treatment group and control group were extracted independently by the two researchers. If data were presented only graphically, the mean and SD from graphs were measured using a “digital ruler” (Graph Digitizer 2.26; GetData; http://getdata-graph-digitizer.com/download.php/). If SD was not reported, we calculated it through multiplying the standard error (SE) by the square root of the group size. If a control group served more than one experimental group differentiated by a different dose, delivery route, or administration time, then the size of control group was divided by the number of experimental groups served. Moreover, if an outcome observation was carried out at different times, only the data from the longest time were extracted. Disagreement between two investigators was solved by checking the data in the publications together.
Methodological Quality of Studies
The quality of each study was assessed according to the Collaborative Approach to Meta-Analysis and Review of Animal Data from Experimental Studies (CAMARADES) checklist. This comprises i) publication in a peer-reviewed journal; ii) reporting of a sample size calculation; iii) allocation of randomized treatment; iv) allocation concealment; v) blind assessment of outcome; vi) use of suitable animal models; vii) avoidance of anesthetic agents with significant intrinsic neuroprotective activity (e.g., ketamine); viii) statement of compliance with regulatory requirements; ix) statements describing temperature control; and x) declarations of potential conflicts of interest. Therefore, the total score of each study ranged from 0 through 10, where “10” represents the greatest methodological strength. The sum of the quality scores was recorded for each research by the two investigators independently.
Statistical Analysis
The meta-analysis was carried out using Stata 15.1 (StataCorp, College Station, TX, USA) and Cochrane Review Manager 5.3 (Cochrane Collaboration; www.cochrane.org/). The combined effect size was calculated as standardized mean differences (SMDs) with 95% confidence interval (CI) between MSC-treatment group and control group. Hedge’s statistic was used to estimate the effect size (18). Forest plots were generated to display the SMD and 95% CI of each study, and the pooled mean difference by combining all studies. p-Value < 0.05 was considered statistically significant.
Statistical heterogeneity was assessed using the I2 statistic. I2 that ranged from 0% to 40%, 30% to 60%, 50% to 90%, and 75% to 100% was defined as “low,” “moderate,” “substantial,” and “considerable” heterogeneity, respectively (19). A random-effects model was used if substantial heterogeneity (I2 > 50%, p < 0.05) was observed. Sensitivity analyses were undertaken to remove extreme values thought to be driving the overall effect. Subgroup analysis and multivariate meta-regression analysis were carried out to identify the source of the heterogeneity.
Publication bias was detected using funnel plots (20). Asymmetry was evaluated through Egger’s test and “trim-and-fill” method (21).
Results
Study Selection
The literature search identified 4,680 potential studies at the primary retrieval: 1,043 records in PubMed, 2,303 in Embase, and 1,334 in Web of science. After review and exclusion, 139 full-text articles remained and were evaluated for inclusion eligibility. From these, 51 records were excluded due to the reasons given in Figure 1. Finally, data from 88 studies published from 2005 to 2021 were included in the meta-analysis.
Study Characteristics
The overall study characteristics are outlined in Supplementary Table 2, and the reference lists of included studies are summarized in the Supplementary Materials. All research was undertaken in rats and mice, and female animals were used in most of the studies. The animal model of MS was induced via myelin oligodendrocyte glycoprotein (MOG), proteolipid protein (PLP), guinea pig spinal cord homogenate (GPSCH), myelin basic protein (MBP), or cuprizone, with MOG accounting for the greatest proportion. The vast majority of studies used bone marrow-derived MSC (BM-MSC) and adipose tissue-derived MSC (ASC), or umbilical cord MSC (UC-MSC) derived from mice, rats, or humans. The remaining studies used placenta-derived MSC (PMSC), embryonic stem cell-derived MSC (ES-MSC), amnion MSC (AMC), decidua-derived MSC (DMSC), periodontal ligament-derived MSC (PDLSC), and murine endometrial-derived MSC (meMSC). The number of MSCs also varied greatly among these studies, with 1 × 106 being the most common, and those MSCs were transplanted via intravenous (IV) or intraperitoneal (IP) injection in most studies. Furthermore, MSCs were administered from 0 days to 13 weeks after MS induction, and the duration of follow-up ranged from 18 to >315 days.
Functional outcomes were assessed in 75 studies using clinical score. In addition, the Morris water maze, basket test, footprint analysis, track visualizations, and Rotarod™ experiments were undertaken to measure functional outcomes in some publications. Forty-eight studies evaluated the histopathological outcomes (remyelination) using transmission electron microscope (TEM), Luxol Fast Blue (LFB) staining, MBP staining, Spielmeyer staining, Solochrome Cyanine staining, hematoxylin and eosin (H&E) staining, or magnetic resonance imaging (MRI). Intriguingly, six studies measured the oligodendrocyte precursor cell (OPC) count, and nine reports detected the oligodendrocyte count (Supplementary Table 3).
Study Quality
The quality assessment of the included studies is outlined in Table 1; the detailed information of each study is listed in Supplementary Table 4. The quality score of the studies ranged from 2 to 8. All studies were published in peer-reviewed journals and used suitable animal models. Also, 85.23% of studies reported a statement of compliance with regulatory requirement. Only 2.27% reported a calculation for the sample size, 39.77% of studies reported allocation of randomized treatment, 11.36% reported allocation concealment, and 42.05% of studies declared a blind assessment of outcome. In addition, the percentage of studies that avoided neuroprotective anesthetic agents, stated a temperature control, and a declared conflict of interest was 6.82%, 15.91%, and 70.45%, respectively.
Meta-Analysis
MSC administration exhibited a favorable effect on the clinical score and remyelination. More specifically, the composite weighted mean of clinical score was −1.99 (95% CI: −2.32 to −1.65, p = 0.000, 75 studies, 101 comparisons; Figure 2A), and remyelination was −2.31 (95% CI: −2.84 to −1.79, p = 0.000, 48 studies, 59 comparisons; Figure 2B). We also undertook a pooled analysis for the OPC count and oligodendrocyte count: the composite weighted mean was −3.66 (95% CI: −6.44 to −0.88, p = 0.000, six studies, six comparisons) (Supplementary Figure 1A) and −3.64 (95% CI: −5.14 to −2.14, p = 0.000, nine studies, nine comparisons) (Supplementary Figure 1B), respectively. These findings provided evidence of the advantageous effect of MSCs on MS models. The I2 statistic suggested remarkable heterogeneity among comparisons of clinical score and remyelination (p = 0.000).
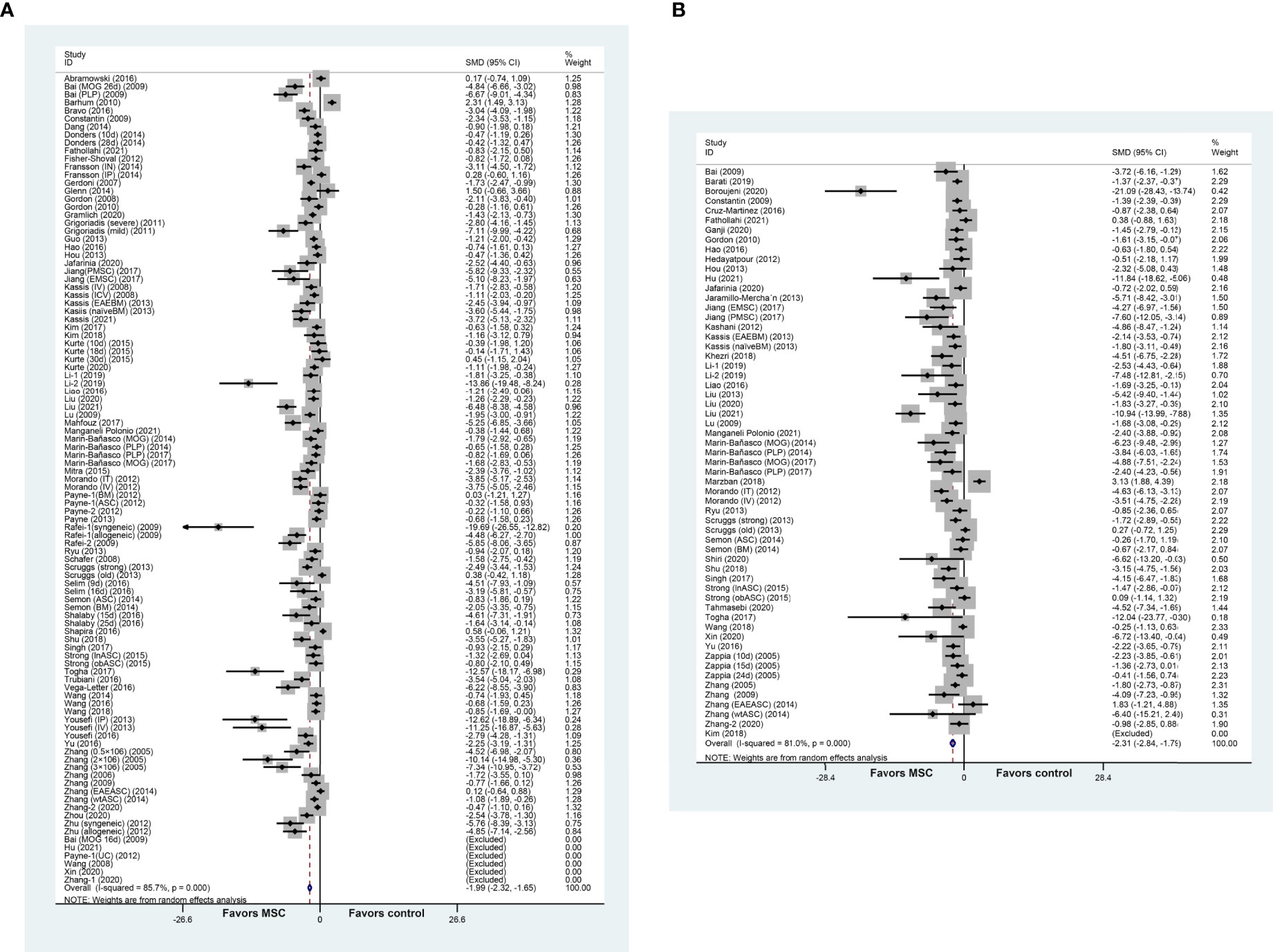
Figure 2 Forest plot shows the mean effect size and 95% confidence interval (CI) for (A) clinical score and (B) remyelination between MSC treatment group and control group in all studies. The mean value or standard deviation (SD) in Bai et al. (22), Hu et al. (23), Payne et al. (10), Wang et al. (24), Xin et al. (25), Zhang et al. (26), and Kim et al. (27) was 0. MSC, mesenchymal stem cell; SMD, standardized mean difference; MOG, myelin oligodendrocyte glycoprotein.
Sensitivity Analysis
We performed a sensitivity analysis to identify the stability of results by sequential omission of each study because of the notable heterogeneity. As depicted in Figures 3A, B, the pooled SMD of clinical score and remyelination was not affected by any study.
Stratified Analysis and Meta-Regression Analysis
We did a further subgroup analysis for the clinical score and remyelination based on different categories, which are summarized in Tables 2 and 3, respectively. In general, the prominent efficacy of MSCs intervention was observed in most subgroups, but significance was not seen in a few individual subgroups (p ≥ 0.05). Significant differences between groups were found in some stratified analyses, but the source of heterogeneity was not identified.
Subsequently, multivariate meta-regression analysis was employed to explore potential contributions to the heterogeneity of the items mentioned in Tables 2 and 3. For clinical score, the source of heterogeneity was not discovered, but the source of MSCs may be the cause of heterogeneity in remyelination (p = 0.022).
Publication Bias
The funnel plot was asymmetrical for the comparisons of the clinical score and remyelination (Figures 4A, B). Egger’s test demonstrated a conspicuous publication bias (p = 0.000). Then, we adopted the trim-and-fill approach to evaluate missing studies and recalculated the overall estimate of the pooled effect. Both of the imputed effect estimates of clinical score and remyelination were consistent with the previous one (SMD: −1.99, 95% CI: −2.32 to −1.65, p = 0.000; SMD: −2.31, 95% CI: −2.84 to −1.79, p = 0.000, respectively), which implied no “missing” studies (Figures 4C, D).
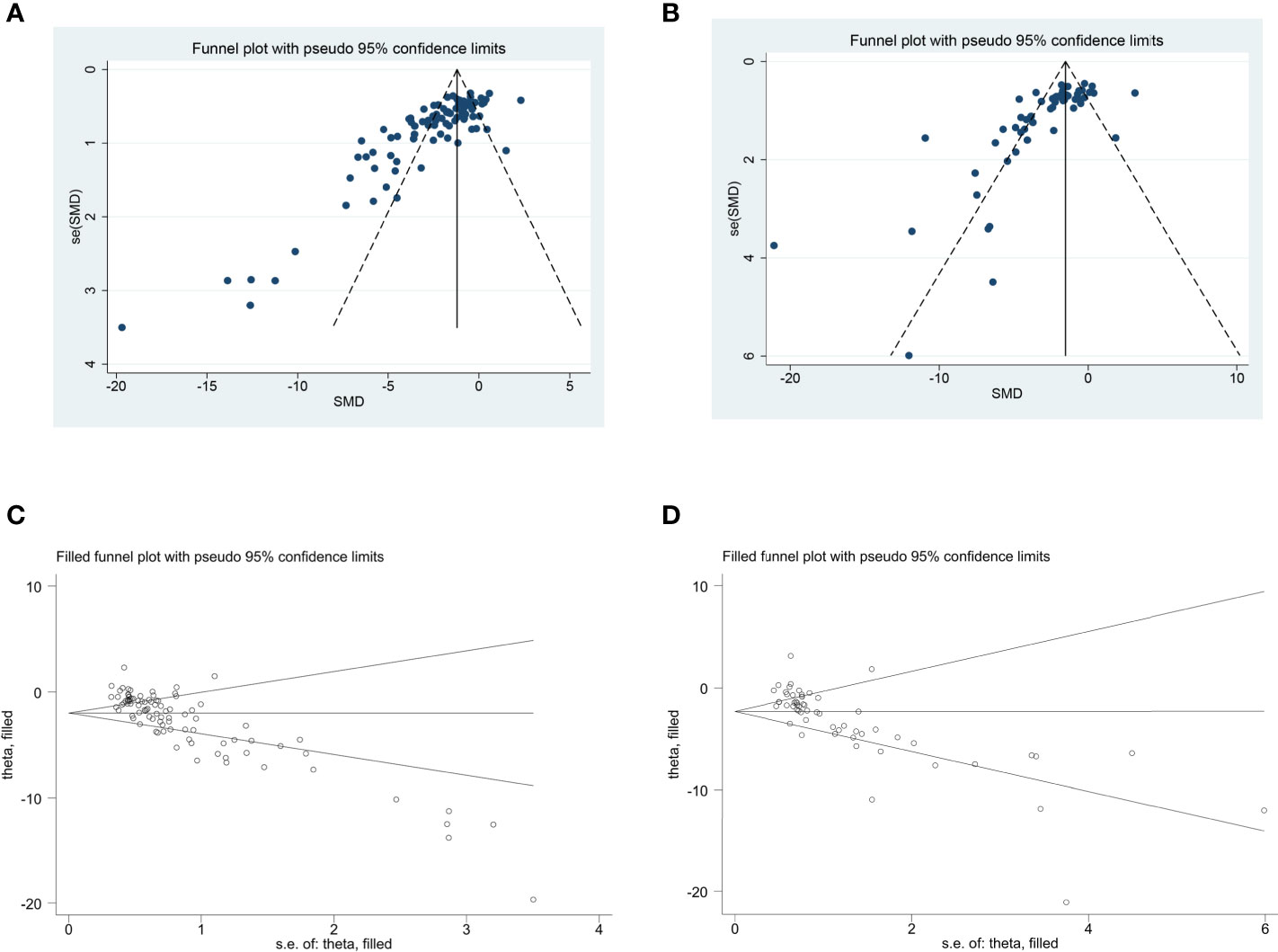
Figure 4 The evaluation of publication bias. Funnel plots for clinical score (A) and remyelination (B). Each dot in the figure represents a study, with the y-axis signifying study quality and the x-axis showing the study results. (C, D) Trim-and-fill method was used to evaluate the missing studies in clinical score and remyelination. SMD, standardized mean difference.
Discussion
Main Findings
Overall, our updated meta-analysis comprising 88 studies suggested that rodents with MS benefited from MSC therapy as manifested by significant amelioration of functional and histopathological outcomes. Subgroup analysis provided more detailed information.
Species, Gender, and Methods of MS Induction in Rodents
Commonly used experimental systems of MS includes EAE (28), virus-induced demyelination/inflammation (29), and toxin-induced demyelination models with cuprizone (30) or ethidium bromide (31). In general, distinct histopathological discrepancies are recognized among these models. EAE and virus-induced demyelination/inflammation model reproduce well the disseminated and inflammatory characteristics of MS, whereas toxin-induced demyelination models are more suitable for simulating the specific mechanism of myelin decline and regeneration (32).
EAE is induced in susceptible animal strains either through active immunization with CNS tissue or myelin peptides (e.g., MOG, GPSCH, PLP, and MBP) or by passive transfer of encephalitogenic T cells (33). C57BL/6 mice, SJL/J mice, NOD mice, Lewis rats, and Dark Agouti (DA) rats are frequently used rodent species. Individual models of EAE can resemble corresponding types of MS clinical forms. Immunization of SJL/J mice with PLP139-151 induces a relapsing–remitting disease course, while C57BL/6 mice often develop a chronic disease after being immunized with a high dose of MOG35-55. Interestingly, immunization of NOD mice with MOG35-55 results in an initial acute episode of EAE followed by a secondary progressive EAE course, which is reminiscent of the secondary progressive phase of MS patients (28, 34). And EAE displays a sex bias that parallels MS whereby females are more susceptible than males (35).
Animal studies can differ widely in terms of experimental conditions. Hence, the species, gender, and methods of MS induction in rodents were applied as the items of stratified analysis in our meta-analysis. No difference in the effect size of species was observed. Males seemed to be more sensitive to MSC treatment than females in terms of the clinical score and remyelination. MSC administration exhibited similar beneficial effects on remyelination among different MS models, but the rodents that were immunized with GPSCH responded best to MSC therapy in terms of the clinical score, followed by PLP immunization group and MOG immunization group. Those outcomes may be related to the small sample size.
Source and Type of MSCs
Several sources of MSCs have been used in experimental models of MS, but most studies used BM-MSCs or ASC from humans, mice, or rats (9). In general, previous data demonstrating the beneficial effects of MSCs were not dependent on the MSCs source (6). Zhu et al. observed no difference in the amelioration of EAE clinical signs between administration of syngeneic and allogeneic MSCs in animal models of MS (36). Similarly, another study suggested that PMSC and ES-MSC exhibited a similarly favorable effect on MS models (37). Payne et al. reported no beneficial effect of BM-MSC, ASC, and UC-MSC in animal models of MS when MSCs were administered after symptom onset (10). In our meta-analysis, the source of MSC was correlated with the effect size in clinical score and remyelination. Allogeneic MSCs displayed the greatest efficacy, followed by syngeneic MSCs and then xenogeneic MSCs. And the types of the MSC also had impact on the effect size in terms of the clinical score and remyelination; however, the number of studies included in several subgroups was too small, and larger preclinical studies are needed to explore this issue in-depth.
Dose and Delivery Route
Delivery dose and route are typically the topic of concern when MSC therapy is applied in clinical situations. The literature suggests that the MSC dose administered in rodent models of MS is in the range from 3 × 105 to 1 × 107. These doses exerted protective effects when administered alone or in multiple injections (6). Yousefi et al. attempted to compare the therapeutic impact of ASC injected via IP and IV routes but did not document the disparity in improvement of the clinical score using these two routes (38). Morando and colleagues also did not observe the significant difference when they compared the clinical course of mice IV injected with those treated intrathecally (IT) (39). The meta-analysis by Yang and colleagues did not find a significant difference in the effect size with regard to the dose and the delivery methods of MSCs (12). Inversely, we observed that a high dose of MSCs (>1 × 106) improved the functional and histopathological outcomes better than a low dose (≤1 × 106). Meanwhile, the delivery route contributed to apparent differences in the clinical score and remyelination. For the clinical score, IT injection seems to show the greatest efficacy, followed by intra-cerebroventricular (ICV), IP, intranasal (IN), and IV injections. In addition, ICV injection was discovered to be the most effective administration route to promote remyelination in comparison with IT, IV, or IP injection. IN and intra-cerebroparenchymal (ICP) injections were also favorable, but large CIs and small sample sizes diminished the robustness of the data.
Time of Administration and Duration of Follow-Up
It is also considerable to determine the role of MSCs in the light of the time of administration and duration of follow-up. In studies that explored the therapeutic effects of MSCs in MS models, MSCs were administered at the onset, peak, or after EAE stabilization. The optimal time of MSC transplantation was controversial. Several studies demonstrated the ability of MSCs to promote functional recovery regardless of the injection time (22, 40). Conversely, other research concluded that the beneficial effect of MSCs was negatively correlated with the time of administration. More specifically, MSC injection induced a significant improvement in the clinical score in animals suffering from EAE at the onset or peak of the disease, with more sustained effects being documented at disease onset (41, 42). Later administration of MSCs had little or no effect on clinical signs or remyelination (41, 43, 44). However, our meta-analysis showed a positive correlation between the effect size and administration time in the clinical score, but not for remyelination. And no difference in the effect size of follow-up duration was observed.
The findings of subgroup analysis are speculative because they are based on the reanalysis of published data, rather than the data from a well-designed randomized controlled trial. Therefore, although the subgroup analysis provided updated evidence, the results of subgroup analysis should be viewed with caution.
Mechanisms of the MSC Therapies in MS
Studies have suggested that MSC transplantation may aid in neuroprotection, anti-inflammation, neuroregeneration, and tissue repair in animal models of MS (45, 46). MSCs confer benefit mainly via the release of soluble trophic factors that stimulate intrinsic tissue restoration mechanisms and the production of EV that enables cell-to-cell communication.
MSC transplantation results in reduction of demyelination and axonal loss through production of neurotrophic factors, such as fibroblast glial factor (FGF), brain-derived neurotropic factor (BDNF), and neural growth factor (NGF) (47). Increased oligodendrogenesis, remyelination, and neurogenesis can be attributed to the neuroregeneration ability of MSCs. The anti-inflammation effect of MSCs not only aids the decline in the numbers of inflammatory cell infiltration but also modulates systematic immune cells (48). Barati et al. noted that MSC infusion diminished neuroinflammation by suppressing activation of astrocytes and microglia as well as promoting a shift of proinflammatory subtypes of microglia (M1) into anti-inflammatory microglia (M2) (49). In addition, another study demonstrated that MSCs attenuated MS by reducing oxidative stress and improving mitochondrial homeostasis (50). Overall, the mechanism of MSC-derived therapeutic effects in MS is depicted in Figure 5.
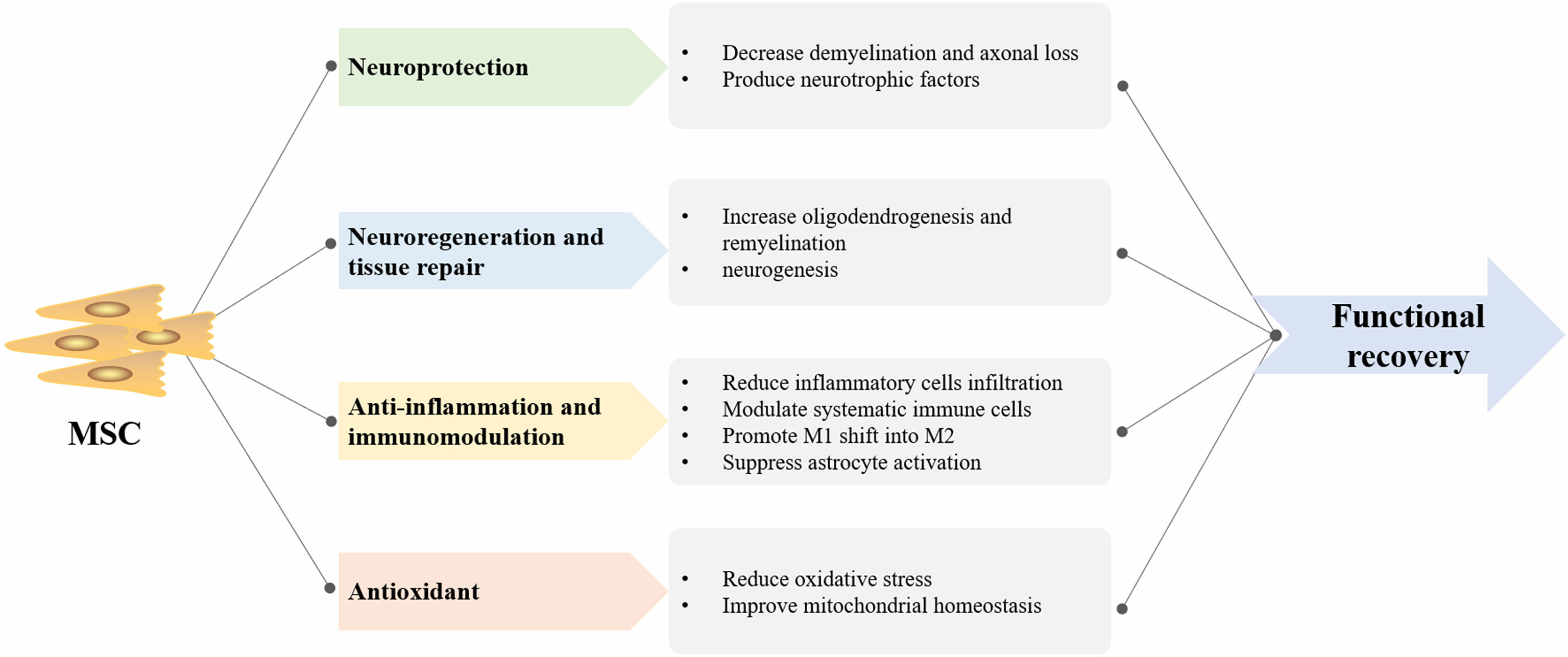
Figure 5 The summary of mesenchymal stem cell (MSC)-derived therapeutic mechanism in multiple sclerosis (MS). M1, proinflammatory subtypes of microglia; M2, anti-inflammatory subtypes of microglia.
Clinical Perspective
To date, a considerable number of small clinical trials involving MSCs in MS with inspiring results have been completed (51–56). Those trials have supported the safety, tolerability, and feasibility of MSC transplantation in MS disorders. Most studies used autologous BM-MSC, and allogeneic PMSC or UC-MSC was applied in a few trials. The infusion routes of MSCs were IV and IT. However, the optimal choice of the source, dose, and transplantation route of MSCs is inconclusive. Data have shown that systemic delivery of MSCs provided safer and beneficial effects (57), but a recent double-blind phase II trial involving 48 patients with PMS found that IT injection was more efficacious than IV injection for several parameters of the outcome (58). Intriguingly, another open prospective study, which was conducted by the same group, noted that multiple IT injections of autologous MSC in patients with PMS were shown safe at the short/intermediate term and induced clinical benefits (59). As many open issues are still unsolved, larger and longer-term trials are warranted to determine the more favorable parameter of MSC transplantation and to further evaluate the potential of cellular therapy in MS.
Limitations
The conclusions from our systematic review were limited by several factors. Firstly, because of a lack of rigor in study design or methodology in parts of a particular study, our conclusions are tempered by the low quality of the studies included in our analysis. Secondly, all data were extracted from the eligible studies that published as original research; therefore, we did not gain access to unpublished results including conference abstract, and this may contribute to the obvious publication bias. Thirdly, there was considerable heterogeneity in statistical analysis across studies.
Conclusion
In summary, our systematic review and meta-analysis provide a comprehensive, up-to-date evaluation of the available data concerning the efficacy of MSC therapy for MS in preclinical settings. MSC administration demonstrated a considerable beneficial effect with regard to functional outcome and histopathological outcome. Future preclinical studies should consider the study design and methodological rigor. A well-designed study will contribute to a better determination of the effect regarding MSC therapy in MS treatment.
Data Availability Statement
The original contributions presented in the study are included in the article/Supplementary Material. Further inquiries can be directed to the corresponding author.
Author Contributions
ZH supervised the project. JH, YH, and XT analyzed the data. JL and ZL extracted the data. JH and ZH wrote the paper. All authors contributed to the article and approved the submitted version.
Funding
This work was supported by the National Natural Science Foundation of China [grant numbers 81974213, 81801188] and Natural Science Foundation of Hunan Province, China [grant number 2019JJ40421].
Conflict of Interest
The authors declare that the research was conducted in the absence of any commercial or financial relationships that could be construed as a potential conflict of interest.
Publisher’s Note
All claims expressed in this article are solely those of the authors and do not necessarily represent those of their affiliated organizations, or those of the publisher, the editors and the reviewers. Any product that may be evaluated in this article, or claim that may be made by its manufacturer, is not guaranteed or endorsed by the publisher.
Supplementary Material
The Supplementary Material for this article can be found online at: https://www.frontiersin.org/articles/10.3389/fimmu.2021.711362/full#supplementary-material
Abbreviations
MS, multiple sclerosis; CNS, central nervous system; RRMS, relapsing–remitting MS; PMS, progressive MS; MSCs, mesenchymal stem cells; BM-MSC, bone marrow-derived MSC; ASC, adipose tissue-derived MSC; UC-MSC, umbilical cord MSC; PMSC, placenta-derived MSC; EAE, experimental autoimmune encephalomyelitis; PRISMA, Preferred Reporting Items for Systematic Reviews and Meta-Analyses; EV, extracellular vesicles; DPI, days post immunization; SD, standard deviation; SE, standard error; CAMARADES, Collaborative Approach to Meta-Analysis and Review of Animal Data from Experimental Studies; SMD, standardized mean differences; CI, confidence interval; MOG, myelin oligodendrocyte glycoprotein; PLP, proteolipid protein; GPSCH, guinea pig spinal cord homogenate; ES-MSC, embryonic stem cell-derived MSC; AMC, amnion MSC; DMSC, decidua-derived MSC; PDLSC, periodontal ligament-derived MSC; meMSC, murine endometrial-derived mesenchymal stem cell; IV, intravenous; IP, intraperitoneal; ICV, intra-cerebroventricular; IN, intranasal; ICP, intra-cerebroparenchymal; IT, intrathecal; TEM, transmission electron microscope; LFB, Luxol Fast Blue; MBP, myelin basic protein; H&E, hematoxylin and eosin; MRI, magnetic resonance imaging; OPC, oligodendrocyte precursor cell; DA, Dark Agouti; FGF, fibroblast glial factor; BDNF, brain-derived neurotropic factor; NGF, neural growth factor; M1, promoting proinflammatory subtypes of microglia; M2, anti-inflammatory microglia.
References
1. McGinley MP, Goldschmidt CH, Rae-Grant AD. Diagnosis and Treatment of Multiple Sclerosis: A Review. JAMA (2021) 325(8):765–79. doi: 10.1001/jama.2020.26858
2. Simkins TJ, Duncan GJ, Bourdette D. Chronic Demyelination and Axonal Degeneration in Multiple Sclerosis: Pathogenesis and Therapeutic Implications. Curr Neurol Neurosci Rep (2021) 21(6):26. doi: 10.1007/s11910-021-01110-5
3. Correale J, Gaitan MI, Ysrraelit MC, Fiol MP. Progressive Multiple Sclerosis: From Pathogenic Mechanisms to Treatment. Brain (2017) 140(3):527–46. doi: 10.1093/brain/aww258
4. Zeydan B, Kantarci OH. Impact of Age on Multiple Sclerosis Disease Activity and Progression. Curr Neurol Neurosci Rep (2020) 20(7):24. doi: 10.1007/s11910-020-01046-2
5. Andrzejewska A, Dabrowska S, Lukomska B, Janowski M. Mesenchymal Stem Cells for Neurological Disorders. Adv Sci (Weinh) (2021) 8(7):2002944. doi: 10.1002/advs.202002944
6. Gugliandolo A, Bramanti P, Mazzon E. Mesenchymal Stem Cells in Multiple Sclerosis: Recent Evidence From Pre-Clinical to Clinical Studies. Int J Mol Sci (2020) 21(22):8662. doi: 10.3390/ijms21228662
7. Uccelli A, Moretta L, Pistoia V. Mesenchymal Stem Cells in Health and Disease. Nat Rev Immunol (2008) 8(9):726–36. doi: 10.1038/nri2395
8. Uccelli A, Laroni A, Freedman MS. Mesenchymal Stem Cells as Treatment for MS - Progress to Date. Mult Scler (Houndmills Basingstoke England) (2013) 19(5):515–9. doi: 10.1177/1352458512464686
9. Lotfy A, Ali NS, Abdelgawad M, Salama M. Mesenchymal Stem Cells as a Treatment for Multiple Sclerosis: A Focus on Experimental Animal Studies. Rev Neurosci (2020) 31(2):161–79. doi: 10.1515/revneuro-2019-0040
10. Payne NL, Sun G, McDonald C, Layton D, Moussa L, Emerson-Webber A, et al. Distinct Immunomodulatory and Migratory Mechanisms Underpin the Therapeutic Potential of Human Mesenchymal Stem Cells in Autoimmune Demyelination. Cell Transplant (2013) 22(8):1409–25. doi: 10.3727/096368912x657620
11. Fransson M, Piras E, Wang H, Burman J, Duprez I, Harris RA, et al. Intranasal Delivery of Central Nervous System-Retargeted Human Mesenchymal Stromal Cells Prolongs Treatment Efficacy of Experimental Autoimmune Encephalomyelitis. Immunology (2014) 142(3):431–41. doi: 10.1111/imm.12275
12. Yang YW, Ge ML, Zhang YX, Hao QK, Dong BR. Mesenchymal Stem Cells in Experimental Autoimmune Encephalomyelitis Model of Multiple Sclerosis: A Systematic Review and Meta-Analysis. Mult Scler Relat Dis (2020) 44:102200. doi: 10.1016/j.msard.2020.102200
13. Lubetzki C, Zalc B, Williams A, Stadelmann C, Stankoff B. Remyelination in Multiple Sclerosis: From Basic Science to Clinical Translation. Lancet Neurol (2020) 19(8):678–88. doi: 10.1016/s1474-4422(20)30140-x
14. Plemel JR, Liu WQ, Yong VW. Remyelination Therapies: A New Direction and Challenge in Multiple Sclerosis. Nat Rev Drug Discov (2017) 16(9):617–34. doi: 10.1038/nrd.2017.115
15. Rivera FJ, Aigner L. Adult Mesenchymal Stem Cell Therapy for Myelin Repair in Multiple Sclerosis. Biol Res (2012) 45(3):257–68. doi: 10.4067/s0716-97602012000300007
16. Moher D, Liberati A, Tetzlaff J, Altman DG. Preferred Reporting Items for Systematic Reviews and Meta-Analyses: The PRISMA Statement. BMJ (Clinical Res ed) (2009) 339:b2535. doi: 10.1136/bmj.b2535
17. Riva JJ, Malik KM, Burnie SJ, Endicott AR, Busse JW. What Is Your Research Question? An Introduction to the PICOT Format for Clinicians. J Can Chiropr Assoc (2012) 56(3):167–71.
18. Cella M, Preti A, Edwards C, Dow T, Wykes T. Cognitive Remediation for Negative Symptoms of Schizophrenia: A Network Meta-Analysis. Clin Psychol Rev (2017) 52:43–51. doi: 10.1016/j.cpr.2016.11.009
19. Higgins JP, Thompson SG. Quantifying Heterogeneity in a Meta-Analysis. Stat Med (2002) 21(11):1539–58. doi: 10.1002/sim.1186
20. Sterne JA, Sutton AJ, Ioannidis JP, Terrin N, Jones DR, Lau J, et al. Recommendations for Examining and Interpreting Funnel Plot Asymmetry in Meta-Analyses of Randomised Controlled Trials. BMJ (Clinical Res ed) (2011) 343:d4002. doi: 10.1136/bmj.d4002
21. Egger M, Davey Smith G, Schneider M, Minder C. Bias in Meta-Analysis Detected by a Simple, Graphical Test. BMJ (Clinical Res ed) (1997) 315(7109):629–34. doi: 10.1136/bmj.315.7109.629
22. Bai LH, Lennon DP, Eaton V, Maier K, Caplan AI, Miller SD, et al. Human Bone Marrow-Derived Mesenchymal Stem Cells Induce Th2-Polarized Immune Response and Promote Endogenous Repair in Animal Models of Multiple Sclerosis. Glia (2009) 57(11):1192–203. doi: 10.1002/glia.20841
23. Hu RX, Lv WQ, Zhang SF, Liu YM, Sun B, Meng YT, et al. Combining miR-23b Exposure With Mesenchymal Stem Cell Transplantation Enhances Therapeutic Effects on EAE. Immunol Lett (2021) 229:18–26. doi: 10.1016/j.imlet.2020.11.007
24. Wang JH, Wang GY, Sun B, Li HL, Mu L, Wang Q, et al. Interleukin-27 Suppresses Experimental Autoimmune Encephalomyelitis During Bone Marrow Stromal Cell Treatment. J Autoimmun (2008) 30(4):222–9. doi: 10.1016/j.jaut.2007.10.001
25. Xin Y, Gao J, Hu R, Li H, Li Q, Han F, et al. Changes of Immune Parameters of T Lymphocytes and Macrophages in EAE Mice After BM-MSCs Transplantation. Immunol Lett (2020) 225:66–73. doi: 10.1016/j.imlet.2020.05.005
26. Zhang JM, Wang H, Fan YY, Yang FH. Effect of Mesenchymal Stem Cells Transplantation on the Changes of Oligodendrocyte Lineage in Rat Brain With Experimental Autoimmune Encephalomyelitis. Brain Behav (2021) 11(2). doi: 10.1002/brb3.1999
27. Kim MJ, Ryu CH, Kim SM, Lim JY, Kim WS, Jeun SS, et al. Combined Treatment with Methylprednisolone and Human Bone Marrow-Derived Mesenchymal Stem Cells Ameliorate Experimental Autoimmune Encephalomyelitis. Adv Exp Med Biol (2018) 15(2):183–94. doi: 10.1007/s13770-017-0101-y
28. Glatigny S, Bettelli E. Experimental Autoimmune Encephalomyelitis (EAE) as Animal Models of Multiple Sclerosis (Ms). Cold Spring Harb Perspect Med (2018) 8(11):a028977. doi: 10.1101/cshperspect.a028977
29. Mecha M, Carrillo-Salinas FJ, Mestre L, Feliú A, Guaza C. Viral Models of Multiple Sclerosis: Neurodegeneration and Demyelination in Mice Infected With Theiler's Virus. Prog Neurobiol (2013) 101-102:46–64. doi: 10.1016/j.pneurobio.2012.11.003
30. Kipp M, Clarner T, Dang J, Copray S, Beyer C. The Cuprizone Animal Model: New Insights Into an Old Story. Acta Neuropathol (2009) 118(6):723–36. doi: 10.1007/s00401-009-0591-3
31. Torre-Fuentes L, Moreno-Jiménez L, Pytel V, Matías-Guiu JA, Gómez-Pinedo U, Matías-Guiu J. Experimental Models of Demyelination and Remyelination. Neurologia (Barcelona Spain) (2020) 35(1):32–9. doi: 10.1016/j.nrl.2017.07.002
32. Hooijmans CR, Hlavica M, Schuler FAF, Good N, Good A, Baumgartner L, et al. Remyelination Promoting Therapies in Multiple Sclerosis Animal Models: A Systematic Review and Meta-Analysis. Sci Rep (2019) 9(1):822. doi: 10.1038/s41598-018-35734-4
33. Constantinescu CS, Farooqi N, O'Brien K, Gran B. Experimental Autoimmune Encephalomyelitis (EAE) as a Model for Multiple Sclerosis (MS). Br J Pharmacol (2011) 164(4):1079–106. doi: 10.1111/j.1476-5381.2011.01302.x
34. Rangachari M, Kuchroo VK. Using EAE to Better Understand Principles of Immune Function and Autoimmune Pathology. J Autoimmun (2013) 45:31–9. doi: 10.1016/j.jaut.2013.06.008
35. Tatar C, Bessert D, Tse H, Skoff RP. Determinants of Central Nervous System Adult Neurogenesis are Sex, Hormones, Mouse Strain, Age, and Brain Region. Glia (2013) 61(2):192–209. doi: 10.1002/glia.22426
36. Zhu J, Zhang J, Li Q, Du Y, Qiao B, Hu X. Transplanting of Mesenchymal Stem Cells May Affect Proliferation and Function of CD4+T Cells in Experimental Autoimmune Encephalomyelitis. Exp Clin Transplant (2012) 10(5):492–500. doi: 10.6002/ect.2011.0197
37. Jiang H, Zhang YY, Tian KW, Wang BB, Han S. Amelioration of Experimental Autoimmune Encephalomyelitis Through Transplantation of Placental Derived Mesenchymal Stem Cells. Sci Rep (2017) 7:41837. doi: 10.1038/srep41837
38. Yousefi F, Ebtekar M, Soleimani M, Soudi S, Hashemi SM. Comparison of In Vivo Immunomodulatory Effects of Intravenous and Intraperitoneal Administration of Adipose-Tissue Mesenchymal Stem Cells in Experimental Autoimmune Encephalomyelitis (EAE). Int Immunopharmacol (2013) 17(3):608–16. doi: 10.1016/j.intimp.2013.07.016
39. Morando S, Vigo T, Esposito M, Casazza S, Novi G, Principato M, et al. The Therapeutic Effect of Mesenchymal Stem Cell Transplantation in Experimental Autoimmune Encephalomyelitis Is Mediated by Peripheral and Central Mechanisms. Stem Cell Res Ther (2012) 3(1):3. doi: 10.1186/scrt94
40. Donders R, Vanheusden M, Bogie JF, Ravanidis S, Thewissen K, Stinissen P, et al. Human Wharton's Jelly-Derived Stem Cells Display Immunomodulatory Properties and Transiently Improve Rat Experimental Autoimmune Encephalomyelitis. Cell Transplant (2015) 24(10):2077–98. doi: 10.3727/096368914x685104
41. Kurte M, Bravo-Alegria J, Torres A, Carrasco V, Ibanez C, Vega-Letter AM, et al. Intravenous Administration of Bone Marrow-Derived Mesenchymal Stem Cells Induces a Switch From Classical to Atypical Symptoms in Experimental Autoimmune Encephalomyelitis. Stem Cells Int (2015) 2015:140170. doi: 10.1155/2015/140170
42. Selim AO, Selim SA, Shalaby SM, Mosaad H, Saber T. Neuroprotective Effects of Placenta-Derived Mesenchymal Stromal Cells in a Rat Model of Experimental Autoimmune Encephalomyelitis. Cytotherapy (2016) 18(9):1100–13. doi: 10.1016/j.jcyt.2016.06.002
43. Shalaby SM, Sabbah NA, Saber T, Abdel Hamid RA. Adipose-Derived Mesenchymal Stem Cells Modulate the Immune Response in Chronic Experimental Autoimmune Encephalomyelitis Model. IUBMB Life (2016) 68(2):106–15. doi: 10.1002/iub.1469
44. Zappia E, Casazza S, Pedemonte E, Benvenuto F, Bonanni I, Gerdoni E, et al. Mesenchymal Stem Cells Ameliorate Experimental Autoimmune Encephalomyelitis Inducing T-Cell Anergy. Blood (2005) 106(5):1755–61. doi: 10.1182/blood-2005-04-1496
45. Yousefi F, Lavi Arab F, Saeidi K, Amiri H, Mahmoudi M. Various Strategies to Improve Efficacy of Stem Cell Transplantation in Multiple Sclerosis: Focus on Mesenchymal Stem Cells and Neuroprotection. J Neuroimmunol (2019) 328:20–34. doi: 10.1016/j.jneuroim.2018.11.015
46. Uccelli A, Laroni A, Freedman MS. Mesenchymal Stem Cells for the Treatment of Multiple Sclerosis and Other Neurological Diseases. Lancet Neurol (2011) 10(7):649–56. doi: 10.1016/s1474-4422(11)70121-1
47. Cuascut FX, Hutton GJ. Stem Cell-Based Therapies for Multiple Sclerosis: Current Perspectives. Biomedicines (2019) 7(2):26. doi: 10.3390/biomedicines7020026
48. Baldassari LE, Feng J, Clayton BLL, Oh SH, Sakaie K, Tesar PJ, et al. Developing Therapeutic Strategies to Promote Myelin Repair in Multiple Sclerosis. Expert Rev Neurother (2019) 19(10):997–1013. doi: 10.1080/14737175.2019.1632192
49. Barati S, Ragerdi Kashani I, Moradi F, Tahmasebi F, Mehrabi S, Barati M, et al. Mesenchymal Stem Cell Mediated Effects on Microglial Phenotype in Cuprizone-Induced Demyelination Model. J Cell Biochem (2019) 120(8):13952–64. doi: 10.1002/jcb.28670
50. Shiri E, Pasbakhsh P, Borhani-Haghighi M, Alizadeh Z, Nekoonam S, Mojaverrostami S, et al. Mesenchymal Stem Cells Ameliorate Cuprizone-Induced Demyelination by Targeting Oxidative Stress and Mitochondrial Dysfunction. Cell Mol Neurobiol (2020). doi: 10.1007/s10571-020-00910-6
51. Karussis D, Karageorgiou C, Vaknin-Dembinsky A, Gowda-Kurkalli B, Gomori JM, Kassis I, et al. Safety and Immunological Effects of Mesenchymal Stem Cell Transplantation in Patients With Multiple Sclerosis and Amyotrophic Lateral Sclerosis. Arch Neurol (2010) 67(10):1187–94. doi: 10.1001/archneurol.2010.248
52. Yamout B, Hourani R, Salti H, Barada W, El-Hajj T, Al-Kutoubi A, et al. Bone Marrow Mesenchymal Stem Cell Transplantation in Patients With Multiple Sclerosis: A Pilot Study. J Neuroimmunol (2010) 227(1-2):185–9. doi: 10.1016/j.jneuroim.2010.07.013
53. Connick P, Kolappan M, Crawley C, Webber DJ, Patani R, Michell AW, et al. Autologous Mesenchymal Stem Cells for the Treatment of Secondary Progressive Multiple Sclerosis: An Open-Label Phase 2a Proof-of-Concept Study. Lancet Neurol (2012) 11(2):150–6. doi: 10.1016/S1474-4422(11)70305-2
54. Cohen JA, Imrey PB, Planchon SM, Bermel RA, Fisher E, Fox RJ, et al. Pilot Trial of Intravenous Autologous Culture-Expanded Mesenchymal Stem Cell Transplantation in Multiple Sclerosis. Mult Scler J (2018) 24(4):501–11. doi: 10.1177/1352458517703802
55. Harris VK, Stark J, Vyshkina T, Blackshear L, Joo G, Stefanova V, et al. Phase I Trial of Intrathecal Mesenchymal Stem Cell-Derived Neural Progenitors in Progressive Multiple Sclerosis. Ebiomedicine (2018) 29:23–30. doi: 10.1016/j.ebiom.2018.02.002
56. Riordan NH, Morales I, Fernández G, Allen N, Fearnot NE, Leckrone ME, et al. Clinical Feasibility of Umbilical Cord Tissue-Derived Mesenchymal Stem Cells in the Treatment of Multiple Sclerosis. J Trans Med (2018) 16(1):57. doi: 10.1186/s12967-018-1433-7
57. Barati S, Tahmasebi F, Faghihi F. Effects of Mesenchymal Stem Cells Transplantation on Multiple Sclerosis Patients. Neuropeptides (2020) 84:102095. doi: 10.1016/j.npep.2020.102095
58. Petrou P, Kassis I, Levin N, Paul F, Backner Y, Benoliel T, et al. Beneficial Effects of Autologous Mesenchymal Stem Cell Transplantation in Active Progressive Multiple Sclerosis. Brain (2020) 143(12):3574–88. doi: 10.1093/brain/awaa333
Keywords: multiple sclerosis, mesenchymal stem cell, remyelination, meta-analysis, animal model
Citation: He J, Huang Y, Liu J, Lan Z, Tang X and Hu Z (2021) The Efficacy of Mesenchymal Stem Cell Therapies in Rodent Models of Multiple Sclerosis: An Updated Systematic Review and Meta-Analysis. Front. Immunol. 12:711362. doi: 10.3389/fimmu.2021.711362
Received: 18 May 2021; Accepted: 05 August 2021;
Published: 26 August 2021.
Edited by:
Hideyuki Takeuchi, Yokohama City University, JapanReviewed by:
Ibrahim Kassis, Hadassah Medical Center, IsraelEmanuele D’Amico, University of Catania, Italy
Copyright © 2021 He, Huang, Liu, Lan, Tang and Hu. This is an open-access article distributed under the terms of the Creative Commons Attribution License (CC BY). The use, distribution or reproduction in other forums is permitted, provided the original author(s) and the copyright owner(s) are credited and that the original publication in this journal is cited, in accordance with accepted academic practice. No use, distribution or reproduction is permitted which does not comply with these terms.
*Correspondence: Zhiping Hu, emhpcGluZ2h1QGNzdS5lZHUuY24=