- 1Department of Bioengineering, School of Engineering and Applied Science, University of Pennsylvania, Philadelphia, PA, United States
- 2Pulmonary and Sleep Medicine, Children’s Hospital of Philadelphia, Philadelphia, PA, United States
- 3Department of Information Engineering and Research Center E. Piaggio, University of Pisa, Pisa, Italy
- 4Division of Otolaryngology, Department of Surgery, Children's Hospital of Philadelphia, Philadelphia, PA, United States
- 5Department of Otorhinolaryngology, Head and Neck Surgery, Perelman School of Medicine, University of Pennsylvania, Philadelphia, PA, United States
- 6Department of Orthopaedic Surgery, Perelman School of Medicine at the University of Pennsylvania, Philadelphia, PA, United States
- 7Ri.MED Foundation, Palermo, PA, Italy
Introduction: Arthritis, a disease affecting over 50 million adults in the United States, encompasses many different conditions involving joints and surrounding tissues. Disease development, progression, and subsequent treatment is dependent on many different factors, including the relationship between adjacent tissues and the immunological signals involved. A major contributor to disease regulation is the crosstalk between the cartilage and the bone in joints, as well as their reaction to immune factors such as cytokine signaling and macrophage mediation. Studying cartilage-bone crosstalk in arthritis development can be difficult, as controlling immunological factors in vivo is challenging, but in vitro models often lack multi-tissue relevancy.
Methods: To fix this, we developed an in vitro micro-physiological system using a biphasic bioreactor that supports modeling of multiple tissues. We generated cartilage and vascularized-bone analogs and combined them in the bioreactor to allow diffusion and signaling between them. Using this system, we directly induced inflammation in the cartilage region and studied how crosstalk between the two adjacent tissues contributed to disease progression.
Results: We showed that conditioned media from pro-inflammatory macrophages generated a different inflammatory profile than a simple inflammatory cytokine cocktail. We also showed that the vascularized-bone region became inflamed in response to the cartilage inflammation, verifying crosstalk in the system and successfully modeling the relationship between cartilage and bone in an arthritic environment.
Discussion: This model can be used to further probe the crosstalk between bone and cartilage in arthritis, allowing researchers to tease out the effect of specific inflammatory agents or therapeutics in vitro.
1 Introduction
Arthritis is a complex disease group that affects bone, cartilage, and the surrounding tissues in articular joints (1, 2). Arthritis is a major cause of disability and afflicts over 50M adults in the United States (3). There are currently few successful therapies for arthritis, most of which are palliative in nature such as physical therapy and non-steroidal anti-inflammatory drugs (4). There has been some progress with disease-modifying drugs that can prevent continued progression of arthritis, especially thanks to the use of monoclonal antibodies, however, none can completely reverse the effects (5). Therefore, continued study of disease progression and therapeutic efficacy is critical.
To design better solutions for the treatment of arthritis, it is important to acknowledge that an articular joint is a complex organ consisting of multiple tissues such as cartilage, bone, vasculature, and synovium (6). Articular cartilage is responsible for dispersing and distributing the load on the joint (7). The primary cells in cartilage are chondrocytes, which are sparsely distributed in a dense extracellular matrix (ECM) whose main characteristic components are collagen type II, glycosaminoglycans (GAGs), and aggrecan (1). Below cartilage and in stark contrast is the subchondral bone, innervated and vascularized, rich in minerals and collagen type I, and populated with osteocytes, osteoblasts, and osteoclasts (8). Bone also encapsulates the bone marrow cavity, which is rich in nutrients and stem cells (9, 10). The synovium, lining the articular joint capsule, contains macrophage-like cells and fibroblast-like cells that clear debris and secrete ECM components and cytokines (11, 12). In a healthy joint, the cartilage, bone, vasculature, and the joint space components undergo constant crosstalk to function as an articular unit. Nutrients from the joint space help support cartilage health and function. The vascularization drives bone remodeling (13), and in turn the bone supports cartilage structure and function (14). These processes can be compromised in arthritis, thus dysfunction in one tissue can negatively affect any of the others. This crosstalk plays an important role in the development and progression of arthritis in articular joints. Inflammatory cytokines in the joint space affect the phenotype of the resident cartilage and bone cells and propagate the disease (11, 15, 16). The cartilage responds to inflammatory cues with the degradation and progressive loss of critical ECM components such as GAGs and collagen type II (16–19). As a result, the subchondral bone experiences changes in mineralization and turnover, and local lesions appear (20–23). Functionally, the cartilage loses its mechanical integrity, and its erosion leads to bone-on-bone contact, which is associated with significant pain for patients. It is clear that when studying arthritic disorders or developing treatments, it is critical to consider tissue crosstalk in response to inflammatory signals and how they may affect each other as well as the ultimate clinical outcomes.
Given the complexity of the interactions in arthritic articular joints, animal models are commonly used to capture the full breadth of physiological functions (24–26). However, animal models have varying degrees of biological similarity compared to humans in both joint structure and immunological profile (27, 28). An example of differing joint mechanics is that human knees can reach full extension, whereas neither cow, sheep, goat, pig, dog, or rabbit knees can even surpass 20° extension (28). Immunologically, there are many differences in immune cell populations and responses between common animal models and humans. Specific examples relevant to this work include the toll-like receptor (TLR) pathway; Seok et al. found that in response to inflammation, activation of genes within the TLR pathway of a mouse model could not accurately predict the activation of genes in humans (29). Notably, mouse monocytes have been shown to lack a TLR4 response to lipopolysaccharide (LPS) (30). More broadly, Vijayan et al. found that mouse macrophages favored oxidative phosphorylation after LPS activation, whereas human macrophages reprogrammed to increase glycolysis (31). Another relevant example is that mouse endothelial cells have been shown to express P-selectin in response to tumor necrosis factor (TNF) and LPS, whereas human endothelial cells were nonresponsive (32). Considering the differences in immune response between human and animal models, it is not surprising that many therapeutics that are successfully tested in animals only have limited success in the clinic (5, 33). For example, mouse and rat studies of a disintegrin and metallopeptidase with thrombospondin (ADAMTS) type 5 inhibitor showed successful prevention of cartilage loss (34), but there was no statistically significant improvement in a human clinical trial (35). In fact, even a Food and Drug Administration (FDA) approved drug, anakinra, only shows modest efficacy in the clinic but poses a risk of infection (36, 37). Similarly, FDA-approved infliximab for TNF inhibition only has about a 50% success rate in clinic, and the response is subtle (38). Hence, while in vitro models lose in complexity compared to animals, they offer the unique possibility of using human cell sources, thus gaining biological similarity in that respect. Organ-on-a-chip and in vitro models have been advocated for as complementary tools alongside animal models. Additionally, animal models may be costly or inaccessible to many researchers around the globe, and there is increasing drive to reduce or replace the use of animals in scientific research (39, 40).
Given the shortcomings of animal models discussed above, there has been a growing body of research on the use of in vitro models to study arthritis progression. However, many of the currently available in vitro models have yet to recapitulate the complexity of the multiple tissues in articular joints. It is a challenge to co-culture many different cell types in different matrices, perfuse them with different media to match each cell’s needs, and target the delivery of inflammatory agents. As mentioned earlier, there are important biochemical cues from the synovial fluid that lead to disease progression in cartilage, as well as between the cartilage and the subchondral bone (16, 41, 42). The inflammatory agents in the synovial fluid play a role in arthritis development (12), and the constant signaling between the synovium, cartilage, and bone is thought to sustain disease progression (43). Thus, a more veritable in vitro model should be able to capture the crosstalk between bone and cartilage in order to accurately depict disease progression. One approach has been using transwell plates to co-culture combinations of mesenchymal stem cells (MSCs), chondrocytes, and synoviocytes (44), and study the effect that each cell type has on the others in a healthy or diseased environment. However, transwells do not prevent media mixing and cells cultured in monolayers do not capture the three-dimensional (3D) structure of the joint tissues (45). Human cartilage is over 2mm thick, and subchondral bone is similar if not thicker. Diffusion of growth factors and cytokines across such relatively long distances compared to the scale of a cell, and through dense matrices critical to tissue function, is not frequently modeled in vitro. To account for this, Samavedi et al. adapted co-culture models in transwells to accommodate a hydrogel with encapsulated macrophages in one compartment and chondrocytes in a hydrogel in the other transwell compartment, to study crosstalk between the two cell types in a more biomimetic, 3D environment (46). The shortcoming of this model is that the two constructs do not interface, so the diffusion of signaling factors occurs through the common medium, whereas in vivo the signaling factors diffuse more directly through the tissue (47, 48). To account for direct tissue-tissue contact, biphasic constructs have successfully been created to model the cartilage-bone interface, however the whole construct was still cultured in a single common medium. This does not account for the specific cues each tissue needs or is exposed to in vivo, nor does it eliminate the confounding crosstalk factor of signaling diffusion through the medium rather than across the cartilage-bone interface (49, 50). So, while great progress has been made to model arthritis of the articular joint in vitro, there is much room for improvement.
In arthritis, the joint is often inflamed, and synovium inflammation, possibly associated with macrophage polarization to a pro-inflammatory phenotype, is thought to be a major driver of arthritic development. The synovial fluid directly interfaces with cartilage; however, few in vitro models can separately deliver inflammatory cues to either cartilage or bone. In this work, we adopted one of few approaches leveraging our previously described biphasic bioreactor that allows us to perfuse two different types of media through a biphasic construct without mixing (51, 52). Therefore, we perfused the cartilaginous region of a construct with chondrogenic medium, and the osseous region with osteogenic medium, separately. For our in vitro osteochondral construct, we generated cartilage and vascularized bone analogs as described in our previous work (41, 42). The constructs differentiated in the bioreactor independently while maintaining contact to allow crosstalk between the cartilaginous and vascularized osseous regions. Furthermore, the primary key benefit of the bioreactor is the possibility to perfuse the cartilage region only with inflammatory cues modeling an inflamed synovial fluid. We then probed the effects of the inflammatory agents on the cartilaginous layer, and the subsequent response of the vascularized osseous layer to an inflamed cartilage analog. While delivering synovial fluid in our bioreactor is beyond the scope of the study and not feasible due to limited access to samples, we perfused the cartilage region with inflammatory cytokines that are commonly found in synovial fluid of arthritic patients (11). Furthermore, rather than just delivering one or more inflammatory cytokines to the constructs, to better mimic some of the complexity of in vivo inflammation, we also tested the effect of macrophage conditioned medium (MCM) to represent the inflammatory signals secreted by synovial-resident macrophages during arthritis (15, 16). Thus, we compared the response to a combination of cytokines that are highly implicated in arthritis with the response to a pro-inflammatory macrophage conditioned medium that represents a complex biological cocktail more similar to synovial fluid (53, 54). We aimed to validate crosstalk in the proposed system and to determine how to best mimic in vivo inflammation. We hypothesized that MCM will evoke an inflammatory profile similar but not identical to that of the cytokine cocktail, and that there will be detectable responses in the vascularized-bone region after pro-inflammatory activation of the cartilage region.
2 Materials and methods
Reagents were purchased from Thermo Fisher Scientific (Waltham, MA) unless otherwise specified.
2.1 Bioreactor fabrication
The bioreactor was fabricated according to Ianetti et al. and Nichols et al. Briefly, the bioreactor was designed using SolidWorks (Waltham, MA) computer-aided design modeling software. The bioreactor was 3D printed using a stereolithography apparatus from 3Dsystems Viper si2 (Rock Hill, SC) with Somos WaterShed XC 111222 (Elgin, IL) resin (51, 52, 55).
2.2 Cell culture
Bone marrow derived human MSCs (BM-hMSC) were purchased from Rooster Bio (Frederick, MD) at passage 2 and cultured until passage 5 in Rooster-Nourish expansion media (Rooster Bio, Frederick, MD). Three different donor BM-hMSCs were pooled to create each biological replicate, with a total of three biological replicates total (total number of pooled donors n=9, see Table 1). BM-hMSCs were seeded into experimental constructs at passages 5 to 7.
Human umbilical vein endothelial cells (HUVECS) were purchased from Angio-Proteomie (Boston, MA) and expanded in monolayer on a 0.2% gelatin coating in endothelial growth medium 2 (EGM-2, Angio-Proteomie, Boston, MA). HUVECs were seeded into osteochondral constructs at passage 5.
2.3 Construct fabrication
2.3.1 Cartilage construct
Methacrylated gelatin (GelMA) (PhotoGel® ~95% DOM, Advanced Biomatrix, Carlsbad, CA) and Methacrylated hyaluronic acid (MeHA) (PhotoHA® Stiff, Advanced Biomatrix, Carlsbad, CA) were reconstituted to 10% weight/volume (w/v) and 5% w/v respectively, in sterile phosphate buffered saline (PBS) containing 0.15% w/v lithium phenyl-2,4,6-trimethylbenzoylphosphinate (LAP) (Millipore Sigma, Burlington, MA) overnight in a 37°C shaker. Reconstituted PhotoGel® and PhotoHA® were combined 1:1 to create a final solution containing 5% w/v GelMA, 2.5% w/v MeHA, and 0.15% LAP photo-initiator.
BM-hMSCs were suspended in the GelMA/MeHA/LAP solution at 15 million cells/mL, pipetted into ⌀4mm x 2mm cylindrical silicone molds, and photo-crosslinked using a near-ultraviolet (UV) flashlight (395nm, 8 mW/cm2) for 1 minute each from the top and bottom. Constructs were cultured in Dulbecco’s Modified Eagle Medium (DMEM) containing 10% volume/volume (v/v) fetal bovine serum (FBS) (VWR, Radnor, PA) and 2% v/v Antibiotic-Antimycotic (Anti-Anti) for 24 hours then changed to chondrogenic medium containing DMEM, 2% v/v Anti-Anti, 10μg/mL insulin-transferrin-selenium (ITS), 40μg/mL L-proline (Millipore Sigma, Burlington, MA), 50μg/mL L-ascorbic acid 2-phosphate (Millipore Sigma, Burlington, MA), and 10ng/mL transforming growth factor-β3 (TGF-β3). The cartilage constructs were differentiated in the plate on a rotating shaker for 14 days prior to the next step, where they either continued differentiation for 14 more days on the plate or were combined with the vascularized bone construct to generate a complete osteochondral construct.
2.3.2 Vascularized bone construct
The bone scaffold was fabricated as described in our previous work (41, 42). Briefly, biomaterial ink containing 10% w/v type A porcine gelatin (Millipore Sigma, Burlington, MA), 50% w/v nanohydroxyapatite (Nano-HAp) (Fluidinova, Maia, Portugal), and 0.2% w/v genipin (Challenge Bioproduct Co., Yun-Lin Hsien, Taiwan) in PBS was 3D printed using a piston-driven extruder 3D bioprinter with sacrificial support material Pluronic acid. 100K BM-hMSCs were seeded onto each bone scaffold and cultured in Rooster-Nourish expansion medium for 7 days. The medium was then replaced with osteogenic medium containing DMEM, 10% v/v FBS, 2% v/v Anti-Anti, 10mM β-glycerophosphate, 50μg/mL L-ascorbic acid 2-phosphate, and 10nM 1α, 25-Dihydroxyvitamin D3 (Enzo Biochem, Farmingdale, NY) for 14 days (Supplementary Figure 1). To vascularize the bone construct, HUVECs and BM-hMSCs (120K and 30K per scaffold, respectively) were seeded in the pores of the bone scaffold in a hydrogel composed of 5% w/v GelMA, 0.075% w/v LAP, and diluted Tisseel fibrin sealant (0.0025% w/v fibrinogen, 0.00004% w/v thrombin suspension) (Baxter Inc., Deerfield, IL) in PBS. Hydrogels were thermally crosslinked then photo-crosslinked for 2 minutes each.
2.3.3 Osteochondral construct
Complete osteochondral constructs were fabricated after 14 days of pre-differentiation of both the cartilage and the bone analogs. The cartilage analog was placed on top of the vascularized bone construct prior to cross-linking of the fibrin/GelMA with the HUVECs/BM-hMSCs. The construct was thermally crosslinked at room temperature for 2 minutes, then photocrosslinked using a near-UV flashlight for 1 minute each from the top and bottom. The osteochondral construct was then loaded into the biphasic bioreactor and differentiated for another 14 days with chondrogenic medium perfusing the upper chamber and 1:1 osteogenic and vasculogenic media (EGM-2) perfusing the lower chamber (Figure 1).
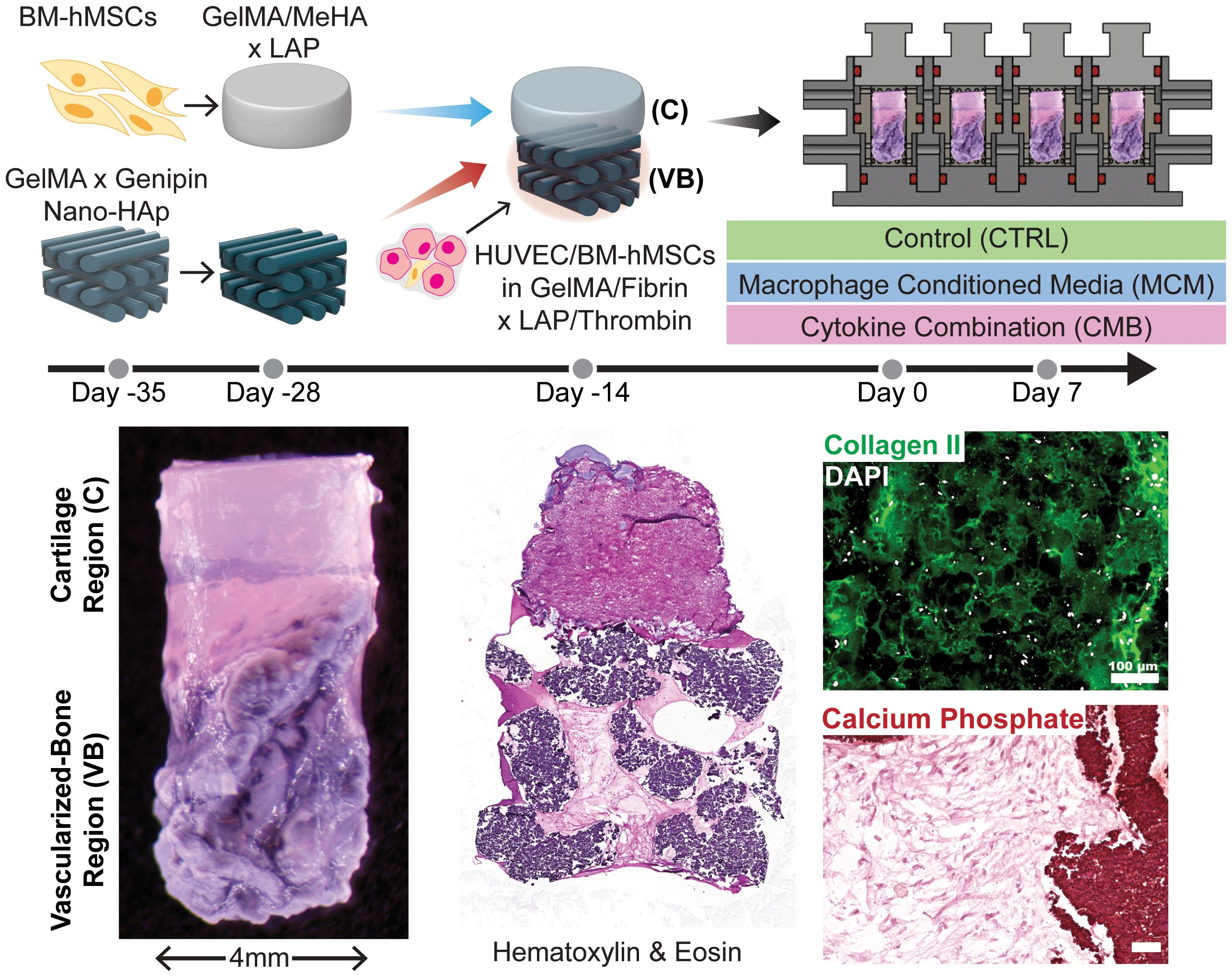
Figure 1. Graphical abstract. Diagram depicting the timeline of setting up the experiment, the experimental conditions, and images showing the construct, with staining for general structure, collagen II in the cartilage region, and calcium phosphate in the osseous region (Scale = 100μm). CTRL medium contains DMEM, 2% v/v Anti-Anti, 10μg/mL ITS, 40μg/mL L-proline, 50μg/mL L-ascorbic acid 2-phosphate, and 10ng/mL TGF-β3. CMB medium contains CTRL medium + 20 ng/mL IL-1β, 100 ng/mL IL-6, and 1000 ng/mL TNF-α. MCM contains CTRL medium + 20x concentrated macrophage conditioned medium (described in 2.4.2).
2.4 Experimental setup
2.4.1 Bioreactor setup
The bioreactor was connected via silicone tubing (5054K304, McMaster-Carr, Elmhurst, IL) and polypropylene male luer locks (51525K142, McMaster-Carr, Elmhurst, IL) to two 20mL syringes on one side, and two medium perfusion bags (Kiyatec, Greenville, SC) on the other. The system was loaded onto a KDS 220/220P Legacy Syringe Pump (KD Scientific, Holliston, MA) in a cell culture incubator. The medium perfusion bags were filled with 20mLs of the desired medium and the syringe pump was set to withdraw continuously at a rate of 1.2μL/min.
2.4.2 Macrophage conditioned media
Tohoku Hospital Pediatrics-1 (THP-1) monocytes (ATCC, Manassas, VA) were differentiated into macrophages in Roswell Park Memorial Institute (RPMI) medium containing 10% v/v FBS, 1% v/v Anti-Anti, and 100 nM phorbal-12-myristate 13-acetate. Cells adhered in 48 hours. The medium was replaced with complete RPMI-1640 (10% FBS, 1% PSF) for 24 hours. Medium was then changed to polarization medium (fluorobrite-DMEM, 1 mM sodium pyruvate, Minimum Essential Medium Non-Essential Amino Acids, 45mg/L Glutamax, 20 ng/mL interferon gamma (IFN-γ), 100 ng/mL LPS), a highly validated protocol for polarizing macrophages (56, 57). Cells were polarized for 72 hours. Macrophage polarization was confirmed by real time quantitative polymerase chain reaction (RT-qPCR) for M1 genes cluster of differentiation 80 (CD80) (58) and C-C chemokine receptor type 7 (CCR7) (59), and M2 genes C-C chemokine ligand 18 (CCL18) (60) and C-C chemokine ligand 22 (CCL22/MDC) (61) (Supplementary Figure 2A). Conditioned medium was collected, pelleted at 500xg to remove cell debris, and the supernatant was transferred to a new tube. Enzyme-linked immunosorbent assay (ELISA) kits for cytokines interleukin-1β (IL-1β), interleukin-6 (IL-6), and tumor necrosis factor-α (TNF-α) were performed according to the manufacturer’s instructions (Peprotech, Cranbury, NJ, Supplementary Figure 2B). In each experiment, three dilutions (1:10, 1:100, 1:1000) of the conditioned medium were analyzed by ELISAs. The dilution within the standard curve was chosen for downstream calculations. The conditioned medium was then concentrated 20X using protein concentration columns (3K molecular weight cutoff) per the manufacturer’s instructions. ELISAs were repeated to confirm accurate concentration and endotoxin detection assays were performed to ensure removal of LPS and IFN-γ.
2.4.3 Inflammatory conditions
After 14 days of continued biphasic differentiation in the bioreactor, inflammation was initiated by perfusing the cartilaginous layer with either control medium (CTRL) (chondrogenic medium), MCM (chondrogenic medium with concentrated pro-inflammatory polarized macrophage conditioned medium diluted 20X), or a combination of inflammatory cytokines (CMB) (chondrogenic media with 20 ng/mL IL-1β, 100 ng/mL IL-6, and 1000 ng/mL TNF-α) (STEMCELL Technologies, Cambridge, MA). The lower chamber remained perfused with 1:1 osteogenic and vasculogenic medium. Inflammatory medium was perfused for 7 days with medium refreshed on day 3 and 5. Similarly for cartilage only experiments, the chondrogenic medium was switched out to inflammatory conditions, with medium changed on days 3 and 5.
2.5 Histology and immunofluorescence
Samples were fixed in 10% w/v formalin, and cryo-embedded after stepwise washes in 10%-30% w/v sucrose solution. 8μm sections were then re-fixed with 4% w/v paraformaldehyde prior to staining. Hematoxylin and Eosin (Epredia, Kalamazoo, MI) staining was performed to visualize overall structure. Collagen Type II immunofluorescence was performed using rabbit Anti-Collagen II (ab34712, Abcam, Cambridge, UK) and goat anti-rabbit IgG Alexa Fluor™ 555 per the manufacturer’s instructions. For all immunostaining, antigen retrieval was performed with proteinase K for 1 hour at RT, followed by 0.15U/mL chondroitinase (Millipore Sigma, Burlington, MA) and 0.75mg/mL hyaluronidase for 30 minutes at RT. Slides were imaged using a Keyence BZ-X800 (Itasca, IL) microscope with a Nikon (Tokyo, Japan) camera.
2.6 RT-qPCR
Samples were flash-frozen and stored dry at -80°C until use. For total ribonucleic acid (RNA) extraction, samples were homogenized in TriZol using a microhomogenizer, then purified using the RNeasy Plus mini kit (Qiagen, Hilden, Germany). Reverse transcription was performed using the SuperScript IV kit. RT-qPCR was performed on a QuantStudio 7 with SYBR Green Master Mix. Primer pairs (Integrated DNA Technologies, Coralville, IA) are reported in Table 2. PCR data was reported as -ΔΔCT, normalized to housekeeping gene (HKG) ribosomal protein L13a (RPL13a) and pool/donor matched day 0 untreated timepoint. Data is not normalized to the CTRL condition, which is a day 7 untreated timepoint, as some CTRL samples continue to differentiate during 7 days of treatment and this trend is important to show.
2.7 Biochemical assays
For all biochemical assays, spent medium was collected from the cartilage only plate, as well as the syringes from the bioreactor system. Medium was centrifuged at 10000xg for 10 minutes and the supernatant was collected. SensoLyte 520 Generic matrix metalloproteinase (MMP) Activity Kit Fluorimetric (AnaSpec, Fremont, CA) was performed per the manufacturer’s instructions. DuoSet ELISA for total human MMP-1, MMP-3, and MMP-13 (R&D Systems, Minneapolis, MN) were performed per manufacturer’s instructions on spent medium diluted up to 1000X as necessary.
2.8 Statistical analysis
Normalized values of all assays were imported into GraphPad Prism 10 (La Jolla, CA) for plotting and analysis. RM Friedman test (paired, nonparametric) with Dunn’s posthoc was performed for all datasets. Due to high standard deviations and donor variability, p-values < 0.2 were reported on the graphs in grey, and p-values < 0.05 were reported in black. Any different statistical test performed were stated in the respective figure caption.
3 Results
3.1 Inflammatory conditions negatively affect cartilage anabolic markers
To first establish the baseline effects that different inflammatory conditions have on cartilage, we administered either MCM or CMB to cartilage constructs cultured in a multi-well plate for 7 days. RT-qPCR showed downregulation of chondrogenic genes for both inflammatory conditions compared to the control of chondrogenic medium without any inflammatory agents (Figure 2A). Specifically, we looked at SRY-Box Transcription Factor 9 (SOX9), the master regulator of chondrogenesis, ACAN, a gene encoding for the proteoglycan aggrecan that is abundant in cartilage, and COL2A1, the gene for collagen type II, the primary collagen in cartilage ECM. To corroborate, there was decreased staining for collagen type II in the matrix of the cartilage gel constructs in the inflammatory conditions (Figure 2B, Supplementary Figure 3), specifically the MCM condition.
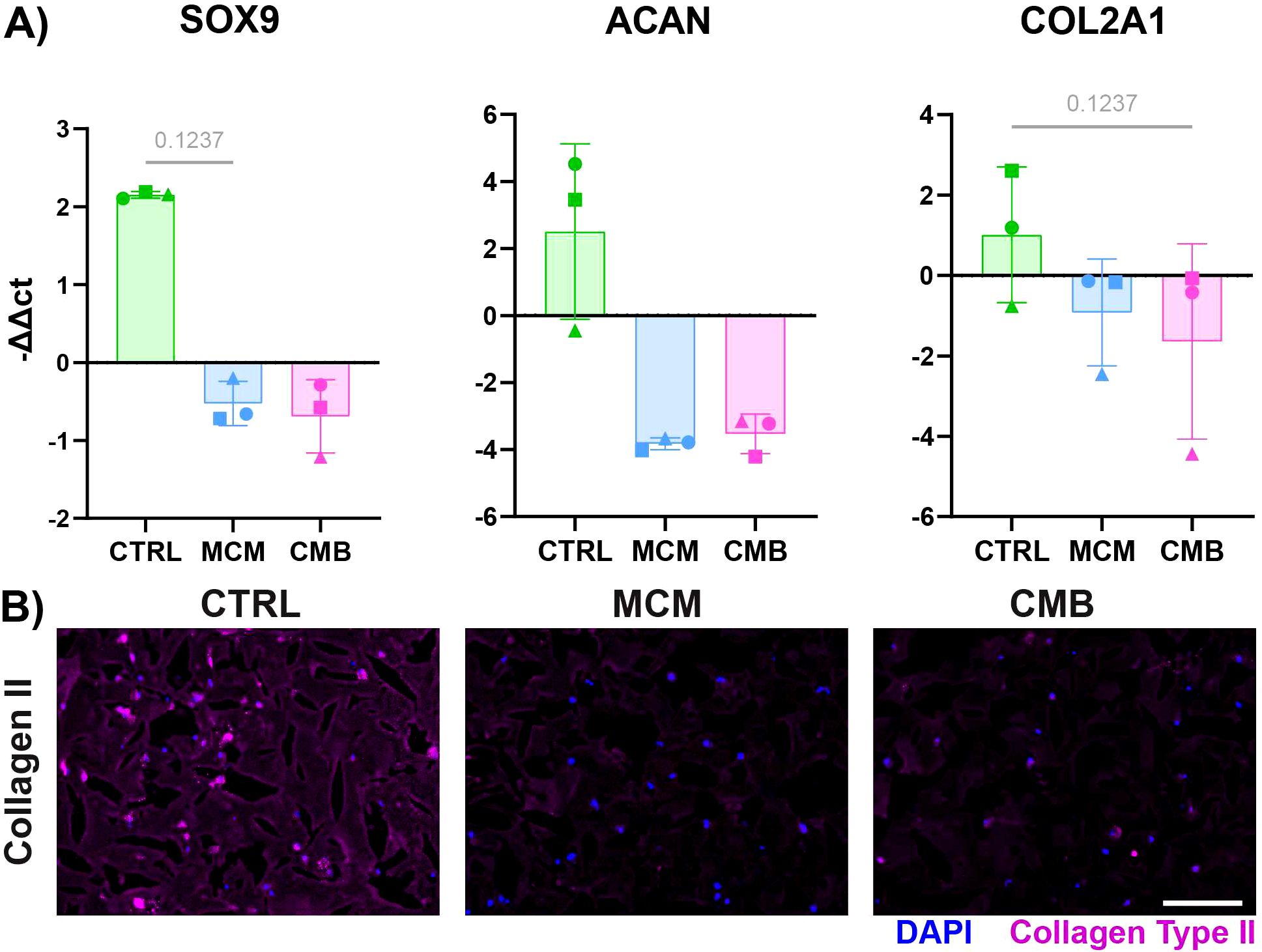
Figure 2. Inflammatory conditions decrease cartilage anabolism in isolated cartilage constructs. (A) RT-qPCR data showing a decrease in expression of chondrogenic genes SOX9, ACAN, COL2A1 under inflammatory conditions (n=3 pools, 3 donors/pool, normalized to D0 untreated and HKG RPL13a, Friedman P values: SOX9 = 0.1944, ACAN = 0.1944, COL2A1 = 0.1944). (B) Immunofluorescent staining for collagen type II showing a decrease in signal under inflammatory conditions (Scale = 50μm).
3.2 Macrophage conditioned media causes strong matrix remodeling in isolated cartilage constructs
The change in matrix composition and matrix remodeling factors such as MMPs were also measured in the individually cultured cartilage gel constructs in response to inflammatory cues. There was upregulation of MMP1, MMP3, MMP13, ADAMTS4, and ADAMTS5 in the inflammatory conditions compared to control (Figure 3A). MMP1 and ADAMTS5 were significantly upregulated in the MCM condition over the CMB condition. There was significant downregulation of COL1A1, the gene encoding for collagen type I, in the CMB group. To corroborate the gene expression findings, Pan-MMP assay and ELISAs were performed on the collected spent media. Pan-MMP assay showed continuous secretion of MMPs over the 7-day course of inflammatory cues delivery, with the highest MMP secretion detected in the MCM group (Figure 3B, Supplementary Figure 4). The Pan-MMP assay showed the abundance of the entire MMP family in the supernatant. To determine the specific activity of key MMPs implicated in arthritis, we performed ELISAs for MMP1, MMP3, and MMP13. The results from the ELISAs mirrored this trend for all key MMPs, showing greater secretion in the MCM group compared to the CMB group (Figure 3B, Supplementary Figure 4). There was little to no secretion of MMPs in the CTRL group as expected.
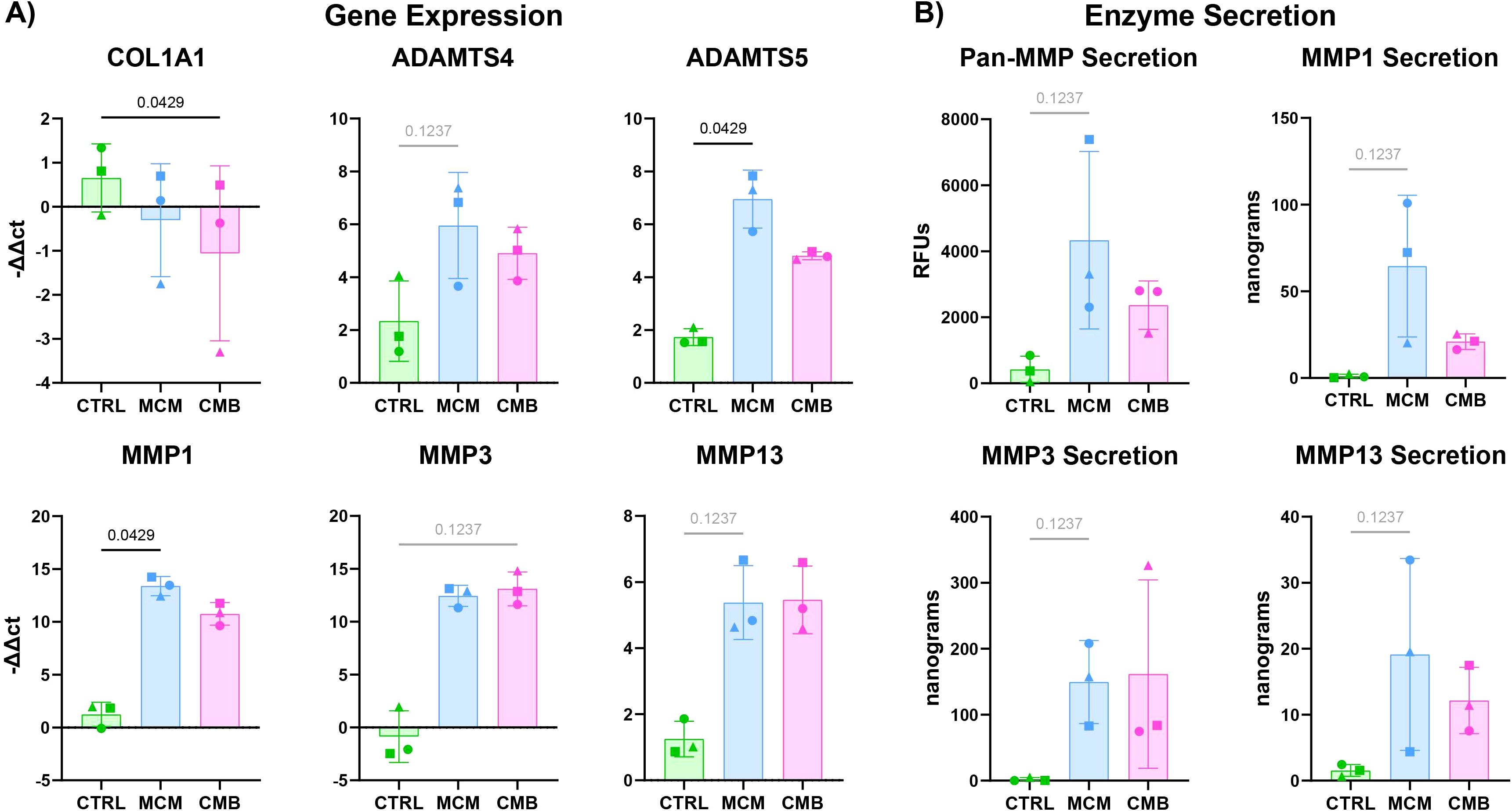
Figure 3. Inflammatory conditions increase catabolic genes in isolated cartilage constructs. (A) RT-qPCR data showing an increase in catabolic genes in the ADAMTS family and MMP family in inflammatory conditions (n=3 pools, 3 donors/pool, normalized to D0 untreated and HKG RPL13a, Friedman P values: COL1A1 = 0.0278, ADAMTS4 = 0.1944, ADAMTS5 = 0.0278, MMP1 = 0.0278, MMP3 = 0.1944, MMP13 = 0.1944). (B) Pan-MMP assay and MMP1, 3, and 13 ELISA data showing an increase in MMP secretion under inflammatory conditions (n=3 pools, 3 donors/pool, blanked with media and normalized to volume and construct number, Friedman P values: Pan-MMP = 0.1944, MMP1 = 0.1944, MMP3 = 0.1944, MMP13 = 0.1944).
3.3 Inflammatory conditions cause catabolic response in the cartilage component of the cartilage-vascularized bone constructs, but with a different profile than isolated cartilage constructs
To determine if the addition of the vascularized-bone construct affected the response of the cartilage analogs to inflammatory cues, complete osteochondral constructs were loaded into the bioreactor and underwent inflammatory stimulation for 7 days (Supplementary Figure 5). The inflammatory agents in the MCM and CMB groups were perfused only through the cartilage construct in the upper chamber. The constructs were collected for RT-qPCR and histology, and the spent medium was used for biochemical assays (Figure 4A). We saw that chondrogenesis is more robust in the bioreactor coculture versus the isolated cartilage constructs in a plate (Supplementary Figure 6). RT-qPCR revealed a similar trend to that in the isolated cartilage constructs, with a downregulation of chondrogenic genes SOX9, ACAN, and COL2A1 for both MCM and CMB conditions (Figure 4B). We similarly saw downregulation of COL1A1 in MCM group, and significant upregulation of ADAMTS5 in the MCM, and MMP3 in the CMB. Notably, expression of MMP1, MMP13, and ADAMTS4 was ameliorated in the inflammatory conditions in coculture. Pan-MMP assay showed higher overall MMP secretion for all groups compared to isolated cartilage constructs, but with lesser differences (Figure 4C). Interestingly, while the MCM elicited the greatest MMP 1, 3, and 13 secretions in the isolated cartilage constructs, it appeared that the CMB group drove the greatest MMP 1 and 13 secretion in the presence of the vascularized-bone construct, whereas MMP1 was significantly upregulated in the MCM group. The reduction of collagen II immunofluorescent staining in the inflammatory groups mirrored that of the isolated cartilage construct (Figure 4D, Supplementary Figure 7). We also compared the COL2A1/COL1A1 ratio of isolated versus cocultured cartilage constructs, but saw no clear trend (Supplementary Figure 8A). Additionally, we compared the expression of endochondral ossification genes bone morphogenetic protein 2 (BMP2), vascular endothelial growth factor (VEGF), Indian hedgehog homolog (IHH), and collagen type 10 (COL10A1) (62–64) in both the isolated cartilage construct and the coculture cartilage constructs. We saw significant upregulation of BMP2 in isolated cartilage constructs under MCM inflammation, but this decreased in cocultured constructs.
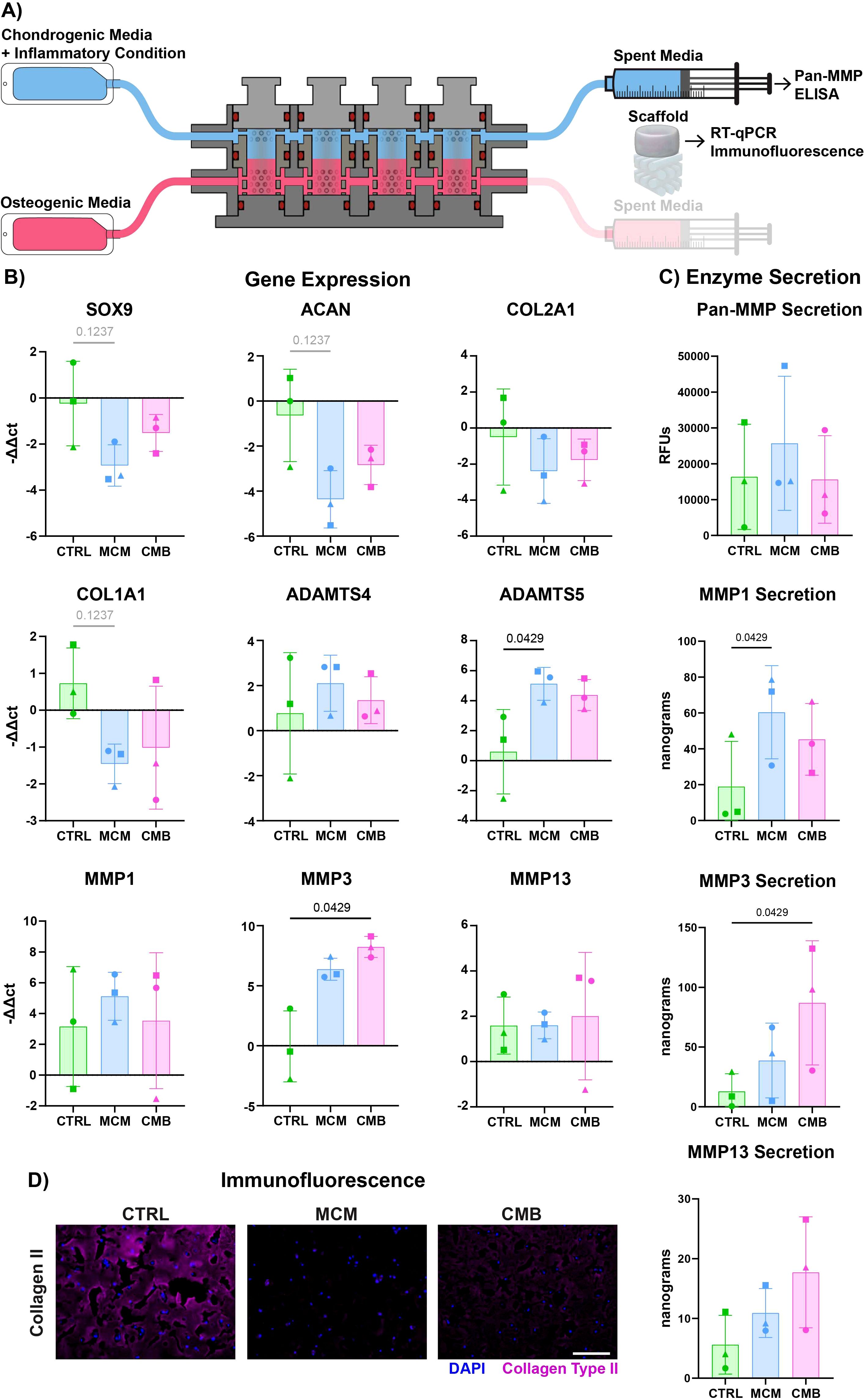
Figure 4. Inflammatory profile differs slightly in coculture cartilage constructs. (A) Diagram explaining the sample and media collection from the bioreactor coculture. (B) RT-qPCR data showing both a decrease in cartilage anabolic genes and increase in cartilage catabolic genes in inflammatory conditions (n=3 pools, 3 donors/pool, normalized to D0 untreated and HKG RPL13a, Friedman P values: SOX9 = 0.1944, ACAN = 0.1944, COL2A1 = 0.3611, COL1A1 = 0.1944, ADAMTS4 = 0.9444, ADAMTS5 = 0.0278, MMP1 = 0.9444, MMP3 = 0.0278, MMP13 = 0.9444). (C) Pan-MMP assay and MMP1, 3, and 13 ELISA data showing an increase in MMP secretion under inflammatory conditions (n=3 pools, 3 donors/pool, blanked with media and normalized to volume and construct number, Friedman P values: Pan-MMP = 0.3611, MMP1 = 0.0278, MMP3 = 0.0278, MMP13 = 0.3611). (D) Immunofluorescent staining for Collagen Type II showing a decrease in signal under inflammatory conditions (Scale = 50μm).
3.4 Inflamed cartilage causes both anabolic and catabolic response in the vascularized-bone matrix
To determine if there was evidence of crosstalk induced inflammation in the vascularized-bone region, we repeated RT-qPCR and enzyme assays on the vascularized-bone constructs and supernatant (Figure 5A). We saw downregulation of bone anabolic genes alkaline phosphatase biomineralization associated (ALPL), secreted phosphoprotein 1 (SPP1), and bone gamma-carboxyglutamate protein (BGLAP) in inflammatory conditions (Figure 5B). We saw upregulation of osteogenic genes runt-related transcription factor 2 (RUNX2) and integrin binding sialoprotein (IBSP) in the MCM condition only. There was a decrease of COL1A1 and an increase of MMP3 and MMP13 in the CMB condition, and an increase of MMP1 in CMB and more so MCM. We saw highest Pan-MMP and MMP13 expression in CMB condition, and significantly higher MMP3 expression in the CMB condition (Figure 5C).
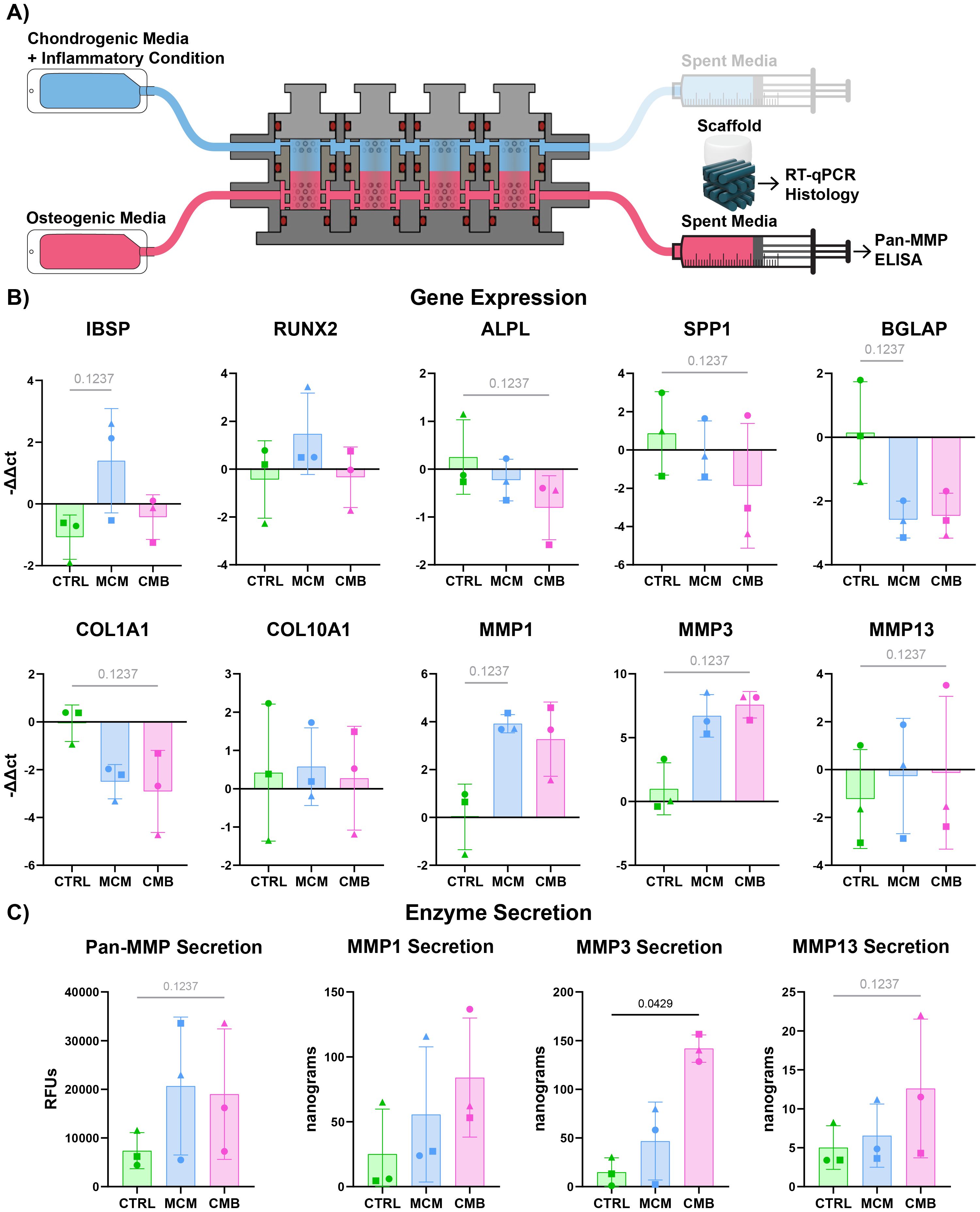
Figure 5. Inflammatory profile of vascularized-bone construct shows evidence of cartilage-bone crosstalk. (A) Diagram explaining the sample and media collection from the bioreactor coculture. (B) RT-qPCR data showing both a decrease in most bone anabolic genes and increase in bone catabolic genes in inflammatory conditions (n=3 pools, 3 donors/pool, normalized to D0 untreated and HKG RPL13a, Friedman P values: IBSP = 0.1944, RUNX2 = 0.9444, ALPL = 0.1944, SPP1 = 0.1944, BGLAP = 0.1944, COL1A1 = 0.1944, COL10A1 > 0.9999, MMP1 = 0.1944, MMP3 = 0.1944, MMP13 = 0.1944). (C) Pan-MMP assay and MMP1, 3, and 13 ELISA data showing an increase in MMP secretion under inflammatory conditions (n=3 pools, 3 donors/pool, blanked with media and normalized to volume and construct number, Friedman P values: Pan-MMP = 0.1944, MMP1 = 0.5278, MMP3 = 0.0278, MMP13 = 0.1944).
4 Discussion
In this study, we developed an in vitro micro-physiological system to model cartilage-bone crosstalk in arthritic environments. To study the inflammatory response of the cartilage analog, we exposed isolated cartilage constructs in a plate to our pro-inflammatory macrophage conditioned medium and cytokine cocktail and compared to the control. Then, we exposed the cartilage region of an osteochondral construct to the same inflammatory conditions and compared the response of the coculture cartilage to the isolated cartilage to determine how the coculture affected the inflammatory response profile. Lastly, to probe crosstalk in our system, we analyzed which evidence of an inflammatory response was shown in the osseus region when the cartilage region was exposed to inflammatory conditions.
We first exposed isolated cartilage constructs to pro-inflammatory stressors to tease out the different responses between CMB and the MCM. In vivo during arthritis, cartilage degrades via aggrecanases and metalloproteinases, losing critical ECM components such as collagen type II and GAGs (17). These phenotypes and their associated gene expression are what we looked for when analyzing the response to inflammatory agents of our cartilage constructs. From RT-qPCR and biochemical assays we observed that both inflammatory conditions resulted in cartilage degradation, although the MCM and CMB conditions presented different inflammatory profiles. There was an increase in MMPs and ADAMTSs, and a decrease in Collagen II and aggrecan. The cartilage construct response to MCM seemed to have greater variability, suggesting a potential stronger response. Furthermore, immunofluorescent staining showed that MCM drove a more substantive decrease in collagen type II signal than the CMB condition. This suggests that the MCM comprises inflammatory agents beyond the inflammatory cytokines in the CMB that may contribute to arthritis initiation and progression. However, isolated cartilage constructs are a somewhat limited in vitro model when the whole articular joint is involved.
We then repeated the test of the same pro-inflammatory conditions within our biphasic bioreactor combining the cartilage constructs with a vascularized osseous construct in a vascularized osteochondral model. We assessed the impact of crosstalk with the adjacent vascularized-bone construct on the cartilage layers when exposed to a pro-inflammatory environment. We put particular care in including HUVECs within the bone scaffold, so that the construct was both vascularized and mineralized. In fact, previous work from our group when developing the vascularized-bone construct (41) showed that the addition of a vascular component to the bone analog supported more robust osteogenesis via paracrine signaling (65–67). In fact, in vivo, vasculature plays a critical role in bone development, maintenance, and regeneration (13), besides supplying oxygen and nutrients from blood. Recently, the Zelzar lab has even proposed that vasculature supports bone growth during development by acting as a collagen I template guiding osteoblast growth and mineralization (68). On the other hand, the MSCs included in our constructs acted as pericytes and stabilized the forming vessels (69), and the MSC-derived osteoblasts in the construct secreted VEGF to support endothelial cell growth and differentiation (70–72). This interconnected relationship between angiogenesis and osteogenesis is preserved in in vitro engineered bone constructs (73). Thus, we included vascularization in our model to ensure robust osteogenesis and a more realistic in vitro analog.
Bone and cartilage work together in vivo during both homeostasis and disease, so we expected the addition of the vascularized-bone construct to alter the response of the cartilage construct to inflammation. BMPs secreted by osteoblasts and osteocytes (74) have been shown to protect cartilage from inflammation (75). In contrast, Hopkins et al. showed that damaged subchondral bone tissue inhibited cartilage GAG production in vitro (76). The vascularization in the bone also affects cartilage. Endothelial cells that support osteogenesis may also activate the receptor activator of nuclear factor-κB ligand (RANKL) pathway that regulates cartilage resorption (77) and secrete MMPs that remodel both bone and cartilage. Nagao et al. showed that targeting VEGF, a critical factor in osteogenesis and angiogenesis coupling, attenuates osteoarthritis progression in cartilage (78). Furthermore, vasculature has been shown to activate the phosphatidylinositol 3-kinase and protein kinase B (PI3K/Akt) and BMP pathways (42, 67, 79, 80), which help chondrogenic differentiation both in vivo during development, and in vitro. Whether the vascularized-bone construct ameliorated or worsened the cartilage construct response to inflammation, we anticipated the crosstalk between the two regions to change in inflammation. Interestingly, we saw the secretion of MMPs was greater in the CMB condition compared to the MCM, which differed from the isolated cartilage results. This suggests that crosstalk between the bone and cartilage does in fact contribute to cartilage inflammatory response, and that factors secreted by the vascularized-bone construct regulate the cartilage construct’s inflammatory response profile. This reinforces the concept that an isolated cartilage construct is hardly sufficient to recapitulate the dynamics occurring in arthritis, and that a robust in vitro model should include the critical articular tissues of vascularized-bone.
This also raises interest in the difference between MCM and CMB, and how they elicit different responses in the cartilage construct and the vascularized-bone construct. When deciding what cytokines to include in the combined cytokine cocktail, it was critical to focus on cytokines most implicated in arthritis (54, 81–83). Among these, IL-1β, IL-6, and TNF-α play a substantive role in both rheumatoid arthritis and osteoarthritis (82, 83). Inflammation in the synovium is the driver of cytokine production in joint disease (81), with synovial fibroblasts producing IL-6 (23) during arthritis progression, whereas macrophages produce IL-1β and TNF-α (11, 54). In the design of the CMB, we started with the concentrations of these three cytokines in the MCM and multiplied tenfold. Arthritis development in vivo occurs over decades with physiological levels of these cytokines, so we then chose to consistently use supraphysiological levels to ensure a response in our in vitro model in experimentally reasonable time frames. Supraphysiological levels of cytokine are often necessary to elicit a response in 3D cultures due to cytokine interaction with and diffusion through the gel (84, 85), and the protective nature of 3D culture (86, 87). The tenfold multiplication placed the cytokine concentrations in the ranges found most commonly in literature for 3D studies (55, 88, 89) and kept it proportional to the levels in our MCM to maintain consistency. We chose to use a combination of the cytokines instead of testing them individually to represent the in vivo environment more closely. Interestingly, there is evidence of crosstalk between the IL-6 and IL-1β pathways (90, 91), and IL-6 has been shown to regulate the IL-1β and TNF-α signaling (92). The cytokines work synergistically to drive disease and should then be studied together. While the cytokines chosen in this study have been shown to have the strongest effect on inflammation, there are of course far more than 3 cytokines that contribute to arthritis. The modularity and convenience of our system means that many different cytokines could be tested. Moving forward, one might consider teasing out the role of other cytokines hypothesized to have a role in arthritis such as IL-10, IL-12, and IL-8, as well as chemokines such as CCL20 and CCL2 (23, 81, 83).
This very same reasoning is also why we chose to test pro-inflammatory macrophage conditioned medium as an inflammatory agent. In vivo, synovial macrophages release many pro-inflammatory signals that are major drivers of arthritis. While there has been significant research into the secretome of synovial macrophages, the specifics of its composition and effects are still being decoded (18, 93–95). Nevertheless, we used macrophage conditioned medium to test if it may better represent in our model the in vivo arthritic conditions, driving a response more analogous to in vivo inflammation. Although, macrophages polarized in vitro differ from macrophages in vivo (96), we hypothesized that the macrophage conditioned medium would still be sufficient to drive a response in cartilage. We saw that the MCM induces inflammation similar to—and in some cases greater than—that of the CMB condition. For simplicity, using a combination of cytokines successfully induced an inflammatory response in vitro, however, the MCM more severely depleted extracellular collagen II protein expression. Since loss of critical ECM components is a hallmark of cartilage degradation in arthritis, this suggests that the MCM can derive an inflammatory response that is more functional and clinically relevant. As for why the MCM may drive a greater inflammatory response, pro-inflammatory macrophages secrete an abundance of proteins (97) that account for many more signaling pathways than those activated by TNF-α, IL-6, and IL-1β. We then hypothesized that the other cytokines secreted by the macrophages, such as IL-12 or IL-23, or chemokines, such as CXC chemokine ligand 1 (CXCL1) and CXCL2 (98, 99), contributed to further response by the construct. Another hypothesis is that pro-inflammatory macrophages secreted nitric oxide (NO) (100), and NO has long been associated with degradation of articular cartilage in arthritis (101). Ideally, the MCM would be a more accurate representation of the synovial fluid in the joint space than the cytokine cocktail, but further secretory analysis of the MCM, and especially the synovial fluid of arthritic patients would be necessary to support this. At this point, we know that synovial fluid from arthritic patients contain cytokines like IL-1, IL-6 (82), and IL-20 (83), and proteases like MMP1 and MMP3 (102). Pro-inflammatory macrophages similarly express MM1 and MMP3 (103), and we confirmed here that the conditioned media contains cytokines IL-1β, IL-6, and TNF-α. There are clearly similarities between conditioned media from pro-inflammatory macrophages and arthritic synovial fluid, but more research is required to precisely determine the full extent of this similarity.
We then looked at the effect of the inflammatory agents on the vascularized-bone analog to determine if the cartilage construct’s inflammation would initiate a response in the vascularized-bone construct. It is important to emphasize that the vascularized-bone construct was never directly perfused with inflammatory agents, and the effect of inflammatory agents onto the vascularized-bone region would be mediated by the cartilage construct. In vivo, during arthritic disruption, inflammation dysregulates the RANKL signaling and produces inflammatory cytokines that cause osteoclast-mediated bone erosion (20). Furthermore, IL-1β blocks mineralization and disrupts osteoblasts (20), and results in strong secretion of MMPs by both osteocytes and endothelial cells in arthritic environments (10). Therefore, we expected to see a decrease in bone mineralization agents and an increase in MMP secretion in our vascularized-bone region in response to inflammation. Notably, we did see an increase in MMP1, 3, and 13 gene expression and protein secretion in the vascularized-bone construct in both CMB and MCM inflammatory conditions. We also saw a decrease in osteogenic genes in the CMB condition. Unexpectedly, we observed a slight increase in RUNX2 and IBSP in the MCM group, implying there may be something in the MCM that promotes expression of those genes. One potential explanation is that inflamed and resorbed bone release BMPs (104), and BMPs can trigger both RUNX2 and subsequent IBSP upregulation (105). Since the vascularized-bone construct presented an inflammatory response profile even when MCM or CMB were delivered only to the cartilage construct, this suggests that the inflamed cartilage construct signals to the bone construct and propagates the inflammation and disease. Thus, we confirmed that our in vitro model allows for crosstalk between the cartilage analog and the bone analog, recapitulating some aspects of the crosstalk that occurs in vivo during disease.
As an even closer model to in vivo circumstances, one might consider using explants in the biphasic bioreactor, and several research groups have created successful ex vivo models using tissue explants (106, 107). However, animal explants suffer from the same limitation as the use of animal cells in terms of lower biological similarity to humans. Human explants would be ideal, but they are more challenging to source in sufficient number for screening and can more easily be obtained by joint replacement surgeries, hence, from joints that present varying and inhomogeneous degrees of tissue degeneration. Therefore, creating a biomimetic osteochondral construct with human cell sources is a good option to create a biologically similar human analog while allowing for significant replicates appropriate for the future screening of therapeutics.
Overall, the results of our study showed that our micro-physiological system is a valid model of crosstalk between bone and cartilage in an arthritic environment. We showed that macrophage conditioned media generated an inflammatory response profile in the cartilage constructs that may be more clinically relevant compared to a cytokine cocktail, suggesting that the latter may not capture the full extent of the inflammatory profile of the synovium in arthritis. Furthermore, we showed that the combination with a vascularized-bone analog in an osteochondral construct modulated the cartilage analog’s response to inflammatory agents, pointing to crosstalk between the cartilaginous and vascularized-osseous components. Notably, the main benefit of our model is the ability to deliver agents to one compartment within the biphasic bioreactor to study the effect of crosstalk between the two tissues. This unique feature could be leveraged to explore the effects of specific molecules in modeling or modulating arthritis, or to test the efficacy of therapeutics in a good throughput system prior to preclinical animal studies.
Data availability statement
The original contributions presented in the study are included in the article/Supplementary Material. Further inquiries can be directed to the corresponding author.
Ethics statement
Ethical approval was not required for the studies on humans in accordance with the local legislation and institutional requirements because only commercially available established cell lines were used.
Author contributions
KS: Conceptualization, Formal analysis, Methodology, Project administration, Visualization, Writing – original draft, Writing – review & editing. SF: Conceptualization, Formal analysis, Investigation, Methodology, Writing – review & editing. HW: Investigation, Methodology, Writing – review & editing. IC: Investigation, Methodology, Resources, Writing – review & editing. GV: Resources, Supervision, Writing – review & editing. CD: Resources, Supervision, Writing – review & editing. RG: Conceptualization, Funding acquisition, Methodology, Project administration, Supervision, Writing – review & editing.
Funding
The author(s) declare that financial support was received for the research and/or publication of this article. NIH NIAMS R44AR072169 and CHOP Airway Frontier Program to RG, NIH T32-AR007132 and Fontaine Fellowship to KS, UPENN CHA to SF. NIH NIAMS P30AR069619 for Core Facility use.
Acknowledgments
The authors would like to acknowledge useful discussions and advice from Dr. Kevin Roehm and the CFD Research team, Dr. Louis Soslowsky, and Dr. George Dodge.
Conflict of interest
RG is an inventor on an issued patent for the bioreactor technology. All other authors declare that the research was conducted in the absence of any commercial or financial relationships that could be construed as a potential conflict of interest.
Publisher’s note
All claims expressed in this article are solely those of the authors and do not necessarily represent those of their affiliated organizations, or those of the publisher, the editors and the reviewers. Any product that may be evaluated in this article, or claim that may be made by its manufacturer, is not guaranteed or endorsed by the publisher.
Supplementary material
The Supplementary Material for this article can be found online at: https://www.frontiersin.org/articles/10.3389/fimmu.2025.1495613/full#supplementary-material
References
1. Mankin HJ, Mow VC, Buckwalter JA, Iannotti JP, and Ratcliffe A. Articular cartilage structure, composition, and function. Orthop basic Sci. (2000) 443–470.
2. Ng JY and Azizudin AM. Rheumatoid arthritis and osteoarthritis clinical practice guidelines provide few complementary and alternative medicine therapy recommendations: a systematic review. Clin Rheumatol. (2020) 39:2861–73. doi: 10.1007/s10067-020-05054-y
3. Fallon EA, Boring MA, Foster AL, Stowe EW, Lites TD, Odom EL, et al. Morbidity and mortality weekly report prevalence of diagnosed arthritis-United States 2019-2021. (2019). Available online at: https://www.cdc.gov/mmwr/mmwr_continuingEducation.html (Accessed August 19, 2024).
4. Huang H, Lou Z, Zheng S, Wu J, Yao Q, Chen R, et al. Intra-articular drug delivery systems for osteoarthritis therapy: shifting from sustained release to enhancing penetration into cartilage. Drug Deliv. (2022) 29:767–91. doi: 10.1080/10717544.2022.2048130
5. Oo WM, Little C, Duong V, and Hunter DJ. The development of disease-modifying therapies for osteoarthritis (DMOADs): the evidence to date. Drug Des Devel. Ther. (2021) 15:2921–45. doi: 10.2147/DDDT.S295224
6. Pacifici M, Koyama E, and Iwamoto M. Mechanisms of synovial joint and articular cartilage formation: Recent advances, but many lingering mysteries. Birth Defects Res Part C - Embryo Today Rev. (2005) 75:237–48. doi: 10.1002/bdrc.20050
7. Patel JM, Wise BC, Bonnevie ED, and Mauck RL. A systematic review and guide to mechanical testing for articular cartilage tissue engineering. Tissue Eng. - Part C Methods. (2019) 25:593–608. doi: 10.1089/ten.tec.2019.0116
8. Florencio-Silva R, Sasso GRDS, Sasso-Cerri E, Simões MJ, and Cerri PS. Biology of bone tissue: structure, function, and factors that influence bone cells. BioMed Res Int. (2015) 2015(1):1–17. doi: 10.1155/2015/421746
9. Stewart HL and Kawcak CE. The importance of subchondral bone in the pathophysiology of osteoarthritis. Front Vet Sci. (2018) 5:178. doi: 10.3389/fvets.2018.00178
10. Hu Y, Chen X, Wang S, Jing Y, and Su J. Subchondral bone microenvironment in osteoarthritis and pain. Bone Res. (2021) 9:1–13. doi: 10.1038/s41413-021-00147-z
11. Scanzello CR. Synovial fluid analysis and the evaluation of patients with arthritis. (2022) 3–19. Springer. doi: 10.1007/978-3-030-99612-3
12. Knights AJ, Farrell EC, Ellis OM, Song MJ, Appleton CT, and Maerz T. Synovial macrophage diversity and activation of M-CSF signaling in post-traumatic osteoarthritis. bioRxiv Prepr. Serv. Biol. (2023). doi: 10.1101/2023.10.03.559514
13. Filipowska J, Tomaszewski KA, Niedźwiedzki Ł, Walocha JA, and Niedźwiedzki T. The role of vasculature in bone development, regeneration and proper systemic functioning. Angiogenesis. (2017) 20:291–302. doi: 10.1007/s10456-017-9541-1
14. Wen C, Lu WW, and Chiu KY. Importance of subchondral bone in the pathogenesis and management of osteoarthritis from bench to bed. J Orthop Transl. (2014) 2:16–25. doi: 10.1016/j.jot.2013.11.004
15. Otero M and Goldring MB. Cells of the synovium in rheumatoid arthritis. Chondrocytes. Arthritis Res Ther. (2007) 9:1–13. doi: 10.1186/ar2292
16. Goldring SR and Goldring MB. Changes in the osteochondral unit during osteoarthritis: Structure, function and cartilage bone crosstalk. Nat Rev Rheumatol. (2016) 12:632–44. doi: 10.1038/nrrheum.2016.148
17. Mankin H, Mow V, and Buckwalter J. Articular cartilage repair and osteoarthritis. Orthop basic Sci Found. Clin Pract. (2007), 472–88.
18. Lozito TP, Alexander PG, Lin H, Gottardi R, Cheng AW-M, and Tuan RS. Osteoarthritis is a disease of the osteochondral junction. (2010). Available online at: http://stemcellres.com/content/4/S1/S6 (Accessed August 19, 2024).
19. Li Y, Wei X, Zhou J, and Wei L. The age-related changes in cartilage and osteoarthritis. BioMed Res Int. (2013) 2013:1–12. doi: 10.1155/2013/916530
20. Karmakar S, Kay J, and Gravallese EM. Bone Damage in rheumatoid arthritis: Mechanistic insights and approaches to prevention. Rheumatol Dis Clin North Am. (2010) 36:385–404. doi: 10.1016/j.rdc.2010.03.003
21. Baker-LePain JC and Lane NE. Role of bone architecture and anatomy in osteoarthritis. Bone. (2012) 51:197–203. doi: 10.1016/j.bone.2012.01.008
22. Aho OM, Finnilä M, Thevenot J, Saarakkala S, and Lehenkari P. Subchondral bone histology and grading in osteoarthritis. PloS One. (2017) 12:1–16. doi: 10.1371/journal.pone.0173726
23. Komatsu N and Takayanagi H. Mechanisms of joint destruction in rheumatoid arthritis — immune cell–fibroblast–bone interactions. Nat Rev Rheumatol. (2022) 18:415–29. doi: 10.1038/s41584-022-00793-5
24. Barré-Sinoussi F and Montagutelli X. Animal models are essential to biological research: Issues and perspectives. Futur. Sci OA. (2015) 1(4). doi: 10.4155/fso.15.63
25. Kuyinu EL, Narayanan G, Nair LS, and Laurencin CT. Animal models of osteoarthritis: Classification, update, and measurement of outcomes. J Orthop Surg Res. (2016) 11(19):1–27. doi: 10.1186/s13018-016-0346-5
26. Zhao T, Xie Z, Xi Y, Liu L, Li Z, and Qin D. How to model rheumatoid arthritis in animals: from rodents to non-human primates. Front Immunol. (2022) 13:887460. doi: 10.3389/fimmu.2022.887460
27. Gregory MH, Capito N, Kuroki K, Stoker AM, Cook JL, and Sherman SL. A review of translational animal models for knee osteoarthritis. Arthritis. (2012) 2012:1–14. doi: 10.1155/2012/764621
28. Proffen BL, McElfresh M, Fleming BC, and Murray MM. A comparative anatomical study of the human knee and six animal species. Knee. (2012) 19:493–9. doi: 10.1016/j.knee.2011.07.005
29. Junhee Seok H, Warren S, Alex GC, Michael NM, Henry VB, Xu W, et al. Genomic responses in mouse models poorly mimic human inflammatory diseases. Proc Natl Acad Sci U. S. A. (2013) 110:3507–12. doi: 10.1073/pnas.1222878110
30. Bjornson-Hooper ZB, Fragiadakis GK, Spitzer MH, Chen H, Madhireddy D, Hu K, et al. A comprehensive atlas of immunological differences between humans, mice, and non-human primates. Front Immunol. (2022) 13:867015. doi: 10.3389/fimmu.2022.867015
31. Vijayan V, Pradhan P, Braud L, Fuchs HR, Gueler F, Motterlini R, et al. Human and murine macrophages exhibit differential metabolic responses to lipopolysaccharide - A divergent role for glycolysis. Redox Biol. (2019) 22:101147. doi: 10.1016/j.redox.2019.101147
32. Mestas J and Hughes CCW. Of mice and not men: differences between mouse and human immunology. J Immunol. (2004) 172:2731–8. doi: 10.4049/jimmunol.172.5.2731
33. Sridharan B, Sharma B, and Detamore MS. A road map to commercialization of cartilage therapy in the United States of america. Tissue Eng. Part B Rev. (2016) 22:15–33. doi: 10.1089/ten.teb.2015.0147
34. Clement-Lacroix P, Little CB, Smith MM, Cottereaux C, Merciris D, Meurisse S, et al. Pharmacological characterization of GLPG1972/S201086, a potent and selective small-molecule inhibitor of ADAMTS5. Osteoarthr. Cartil. (2022) 30:291–301. doi: 10.1016/j.joca.2021.08.012
35. Schnitzer T, Pueyo M, Deckx H, van der Aar E, Bernard K, Hatch S, et al. Evaluation of S201086/GLPG1972, an ADAMTS-5 inhibitor, for the treatment of knee osteoarthritis in ROCCELLA: a phase 2 randomized clinical trial. Osteoarthr. Cartil. (2023) 31:985–94. doi: 10.1016/j.joca.2023.04.001
36. Mertens M and Singh JA. Anakinra for rheumatoid arthritis: A systematic review. J Rheumatol. (2009) 36:1118–25. doi: 10.3899/jrheum.090074
37. Kim M, Choe YH, and Lee S. Lessons from the success and failure of targeted drugs for rheumatoid arthritis: perspectives for effective basic and translational research. Immune Netw. (2022) 22:1–20. doi: 10.4110/in.2022.22.e8
38. Wang Z, Huang J, Xie D, He D, Lu A, and Liang C. Toward overcoming treatment failure in rheumatoid arthritis. Front Immunol. (2021) 12:755844. doi: 10.3389/fimmu.2021.755844
39. Liguori GR, Jeronimus BF, De Aquinas Liguori TT, Moreira LFP, and Harmsen MC. Ethical issues in the use of animal models for tissue engineering: reflections on legal aspects, moral theory, three rs strategies, and harm’Benefit analysis. Tissue Eng. - Part C Methods. (2017) 23:850–62. doi: 10.1089/ten.tec.2017.0189
40. Kirk RGW. Recovering the principles of humane experimental technique. Science, Technology, & Human Values. (2018) 43:622–48. doi: 10.2307/26580457
41. Chiesa I, Maria C, Lapomarda A, Fortunato GM, Montemurro F, Di R, et al. Endothelial cells support osteogenesis in an in vitro vascularized bone model developed by 3D bioprinting. Biofabrication. (2020) 12:1–16. doi: 10.1088/1758-5090/ab6a1d
42. Pirosa A, Gottardi R, Alexander PG, Puppi D, Chiellini F, and Tuan RS. An in vitro chondro-osteo-vascular triphasic model of the osteochondral complex. Biomaterials. (2021) 272:1–13. doi: 10.1016/j.biomaterials.2021.120773
43. Ostrowska M, Maśliński W, Prochorec-Sobieszek M, Nieciecki M, and Sudoł-Szopińska I. Cartilage and bone damage in rheumatoid arthritis. Reumatologia. (2018) 56:111–20. doi: 10.5114/reum.2018.75523
44. Manferdini C, Maumus M, Gabusi E, Piacentini A, Filardo G, Peyrafitte JA, et al. Adipose-derived mesenchymal stem cells exert antiinflammatory effects on chondrocytes and synoviocytes from osteoarthritis patients through prostaglandin E2. Arthritis Rheumatol. (2013) 65:1271–81. doi: 10.1002/art.37908
45. Samvelyan HJ, Hughes D, Stevens C, and Staines KA. Models of osteoarthritis: relevance and new insights. Calcif. Tissue Int. (2021) 109:243–56. doi: 10.1007/s00223-020-00670-x
46. Samavedi S, Diaz-Rodriguez P, Erndt-Marino JD, and Hahn MS. A three-dimensional chondrocyte-macrophage coculture system to probe inflammation in experimental osteoarthritis. Tissue Eng. - Part A. (2017) 23:101–14. doi: 10.1089/ten.tea.2016.0007
47. Lee JI, Sato M, Ushida K, and Mochida J. Measurement of diffusion in articular cartilage using fluorescence correlation spectroscopy. BMC Biotechnol. (2011) 11:19. doi: 10.1186/1472-6750-11-19
48. Oliveira Silva M, Gregory JL, Ansari N, and Stok KS. Molecular signaling interactions and transport at the osteochondral interface: A review. Front Cell Dev Biol. (2020) 8:750. doi: 10.3389/fcell.2020.00750
49. Erickson AE, Sun J, Lan Levengood SK, Swanson S, Chang FC, Tsao CT, et al. Chitosan-based composite bilayer scaffold as an in vitro osteochondral defect regeneration model. Biomed Microdevices. (2019) 21(34):1–16. doi: 10.1007/s10544-019-0373-1
50. Scalzone A, Cerqueni G, Wang XN, Ferreira-Duarte A, Dalgarno K, Mattioli-Belmonte M, et al. An in vitro engineered osteochondral model as tool to study osteoarthritis environment. Adv Healthc. Mater. (2023) 12:1–12. doi: 10.1002/adhm.202202030
51. Iannetti L, D’Urso G, Conoscenti G, Cutrì E, Tuan RS, Raimondi MT, et al. Distributed and lumped parameter models for the characterization of high throughput bioreactors. PloS One. (2016) 11(9):1–25. doi: 10.1371/journal.pone.0162774
52. Nichols DA, Sondh IS, Litte SR, Zunino P, and Gottardi R. Design and validation of an osteochondral bioreactor for the screening of treatments for osteoarthritis. Biomed Microdevices. (2018) 20:4–11. doi: 10.1007/s10544-018-0264-x
53. Fahy N, de Vries-van Melle ML, Lehmann J, Wei W, Grotenhuis N, Farrell E, et al. Human osteoarthritic synovium impacts chondrogenic differentiation of mesenchymal stem cells via macrophage polarisation state. Osteoarthr. Cartil. (2014) 22:1167–75. doi: 10.1016/j.joca.2014.05.021
54. Zhang H, Cai D, and Bai X. Macrophages regulate the progression of osteoarthritis. Osteoarthr. Cartil. (2020) 28:555–61. doi: 10.1016/j.joca.2020.01.007
55. Lin H, Lozito TP, Alexander PG, Gottardi R, and Tuan RS. Stem cell-based microphysiological osteochondral system to model tissue response to interleukin-1B. Mol Pharm. (2014) 11:2203–12. doi: 10.1021/mp500136b
56. Spiller KJKL, Anfang R, Spiller KJKL, Ng J, Kenneth R, Daulton JW, et al. Improved angiogenesis in response to localized delivery of macrophage-recruiting molecules. PloS One. (2015) 6:1–12. doi: 10.1016/j.biomaterials.2014.02.012.The
57. Spiller KL, Wrona EA, Romero-Torres S, Pallotta I, Graney PL, Witherel CE, et al. Differential gene expression in human, murine, and cell line-derived macrophages upon polarization. Exp Cell Res. (2016) 347:1–13. doi: 10.1016/j.yexcr.2015.10.017
58. Raggi F, Pelassa S, Pierobon D, Penco F, Gattorno M, Novelli F, et al. Regulation of human Macrophage M1-M2 Polarization Balance by hypoxia and the Triggering receptor expressed on Myeloid cells-1. Front Immunol. (2017) 8:1097. doi: 10.3389/fimmu.2017.01097
59. Van Raemdonck K, Umar S, Palasiewicz K, Volkov S, Volin MV, Arami S, et al. CCL21/CCR7 signaling in macrophages promotes joint inflammation and Th17-mediated osteoclast formation in rheumatoid arthritis. Cell Mol Life Sci. (2020) 77:1387–99. doi: 10.1007/s00018-019-03235-w
60. Schraufstatter IU, Zhao M, Khaldoyanidi SK, and Discipio RG. The chemokine CCL18 causes maturation of cultured monocytes to macrophages in the M2 spectrum. Immunology. (2012) 135:287–98. doi: 10.1111/j.1365-2567.2011.03541.x
61. Sun J, Sun J, Song B, Zhang L, Shao Q, Liu Y, et al. Fucoidan inhibits CCL22 production through NF-κB pathway in M2 macrophages: A potential therapeutic strategy for cancer. Sci Rep. (2016) 6:1–11. doi: 10.1038/srep35855
62. Farrell E, Both SK, Odörfer KI, Koevoet W, Kops N, Brien FJO, et al. In-vivo generation of bone via endochondral ossification by in-vitro chondrogenic priming of adult human and rat mesenchymal stem cells. BMC Musculoskeletal Disorders. (2011) 12(31):1–9. doi: 10.1186/1471-2474-12-31
63. Mumme M, Scotti C, Papadimitropoulos A, Todorov A, Hoffmann W, Bocelli-Tyndall C, et al. Interleukin-1β modulates endochondral ossification by human adult bone marrow stromal cells. Eur Cells Mater. (2012) 24:224–36. doi: 10.22203/eCM.v024a16
64. Yang J, Andre P, Ye L, and Yang YZ. The Hedgehog signalling pathway in bone formation. Int J Sci. (2015) 7:73–9. doi: 10.1038/IJOS.2015.14
65. Chakraborty G, Jain S, and Kundu GC. Osteopontin promotes vascular endothelial growth factor-dependent breast tumor growth and angiogenesis via autocrine and paracrine mechanisms. Cancer Res. (2008) 68:152–61. doi: 10.1158/0008-5472.CAN-07-2126
66. Nguyen LH, Annabi N, Nikkhah M, Bae H, Binan L, Park S, et al. Vascularized bone tissue engineering: Approaches for potential improvement. Tissue Eng. - Part B Rev. (2012) 18:363–82. doi: 10.1089/ten.teb.2012.0012
67. Rao RR, Vigen ML, Peterson AW, Caldwell DJ, Putnam AJ, and Stegemann JP. Dual-phase osteogenic and vasculogenic engineered tissue for bone formation. Tissue Eng. - Part A. (2015) 21:530–40. doi: 10.1089/ten.tea.2013.0740
68. Shoham AB, Rot C, Stern T, Krief S, Akiva A, Dadosh T, et al. Deposition of collagen type I onto skeletal endothelium reveals a new role for blood vessels in regulating bone morphology. Dev. (2016) 143:3933–43. doi: 10.1242/dev.139253
69. Correia C, Grayson WL, Park M, Hutton D, Zhou B, Guo XE, et al. In vitro model of vascularized bone: Synergizing vascular development and osteogenesis. PloS One. (2011) 6(12). doi: 10.1371/journal.pone.0028352
70. Infanger M, Grosse J, Westphal K, Leder A, Ulbrich C, Paul M, et al. Vascular endothelial growth factor induces extracellular matrix proteins and osteopontin in the umbilical artery. Ann Vasc Surg. (2008) 22:273–84. doi: 10.1016/j.avsg.2007.11.002
71. Carulli C, Innocenti M, and Brandi ML. Bone vascularization in normal and disease conditions. Front Endocrinol (Lausanne). (2013) 4:106. doi: 10.3389/fendo.2013.00106
72. de Silva L, Bernal PN, Rosenberg AJW, Malda J, Levato R, and Gawlitta D. Biofabricating the vascular tree in engineered bone tissue. Acta Biomater. (2023) 156:250–68. doi: 10.1016/j.actbio.2022.08.051
73. Lv N, Zhou Z, Hou M, Hong L, Li H, Qian Z, et al. Research progress of vascularization strategies of tissue-engineered bone. Front Bioeng. Biotechnol. (2023) 11:1291969. doi: 10.3389/fbioe.2023.1291969
74. Katagiri T and Watabe T. Bone morphogenetic proteins. Cold Spring Harb. Perspect Biol. (2016) 8. doi: 10.1101/cshperspect.a021899
75. Deng ZH, Li YS, Gao X, Lei GH, and Huard J. Bone morphogenetic proteins for articular cartilage regeneration. Osteoarthr. Cartil. (2018) 26:1153–61. doi: 10.1016/j.joca.2018.03.007
76. Hopkins T, Wright KT, Kuiper NJ, Roberts S, Jermin P, Gallacher P, et al. An in vitro system to study the effect of subchondral bone health on articular cartilage repair in humans. Cells. (2021) 10:1–23. doi: 10.3390/cells10081903
77. Chen J, Hendriks M, Chatzis A, Ramasamy SK, and Kusumbe AP. Bone vasculature and bone marrow vascular niches in health and disease. J Bone Miner. Res. (2020) 35:2103–20. doi: 10.1002/jbmr.4171
78. Nagao M, Hamilton JL, Kc R, Berendsen AD, Duan X, Cheong CW, et al. Vascular endothelial growth factor in cartilage development and osteoarthritis. Sci Rep. (2017) 7:1–16. doi: 10.1038/s41598-017-13417-w
79. Tsai TL, Wang B, Squire MW, Guo LW, and Li WJ. Endothelial cells direct human mesenchymal stem cells for osteo- and chondro-lineage differentiation through endothelin-1 and AKT signaling. Stem Cell Res Ther. (2015) 6:1–14. doi: 10.1186/s13287-015-0065-6
80. Lee PT and Li W-J. Chondrogenesis of embryonic stem cell-derived mesenchymal stem cells induced by TGFβ1 and BMP7 through increased TGFβ Receptor expression and endogenous TGFβ1 production. J Cell Biochem. (2017) 176:139–48. doi: 10.1002/jcb.25623.Chondrogenesis
81. Nees TA, Rosshirt N, Zhang JA, Reiner T, Sorbi R, Tripel E, et al. Synovial cytokines significantly correlate with osteoarthritis-related knee pain and disability: Inflammatory mediators of potential clinical relevance. J Clin Med. (2019) 8:1–13. doi: 10.3390/jcm8091343
82. Meehan RT, Regan EA, Hoffman ED, Wolf ML, Gill MT, Crooks JL, et al. Synovial fluid cytokines, chemokines and mmp levels in osteoarthritis patients with knee pain display a profile similar to many rheumatoid arthritis patients. J Clin Med. (2021) 10:1–14. doi: 10.3390/jcm10215027
83. Yang L, Chen Z, Guo H, Wang Z, Sun K, Yang X, et al. Extensive cytokine analysis in synovial fluid of osteoarthritis patients. Cytokine. (2021) 143:1–10. doi: 10.1016/j.cyto.2021.155546
84. Dogru S, Dai Z, Alba GM, Simone NJ, and Albro MB. Computational and experimental characterizations of the spatiotemporal activity and functional role of TGF-β in the synovial joint. J Biomech. (2023) 156:111673. doi: 10.1016/j.jbiomech.2023.111673
85. Wang T, Dogru S, Dai Z, Kim SY, Vickers NA, and Albro MB. Physiologic doses of transforming growth factor-β Improve the composition of engineered articular cartilage. Tissue Eng. - Part A. (2024) 31:56–68. doi: 10.1089/ten.tea.2023.0360
86. Edmondson R, Broglie JJ, Adcock AF, and Yang L. Three-dimensional cell culture systems and their applications in drug discovery and cell-based biosensors. Assay Drug Dev Technol. (2014) 12:207–18. doi: 10.1089/adt.2014.573
87. Jha R, Wu Q, Singh M, Preininger MK, Han P, Ding G, et al. Simulated microgravity and 3D culture enhance induction, viability, proliferation and differentiation of cardiac progenitors from human pluripotent stem cells. Sci Rep. (2016) 6:1–14. doi: 10.1038/srep30956
88. Richardson DW and Dodge GR. Effects of interleukin-1β β and tumor necrosis factor-α α on expression of matrix-related genes by cultured equine articular chondrocytes. American Journal of Veterinary Research. (2000) 61(6):624–30. doi: 10.2460/ajvr.2000.61.624
89. Scalzone A, Cerqueni G, Wang XN, Dalgarno K, Mattioli-Belmonte M, Ferreira-Duarte AM, et al. A cytokine-induced spheroid-based in vitro model for studying osteoarthritis pathogenesis. Front Bioeng. Biotechnol. (2023) 11:1167623. doi: 10.3389/fbioe.2023.1167623
90. Deon D, Ahmed S, Tai K, Scaletta N, Herrero C, Lee I-H, et al. Cross-talk between IL-1 and IL-6 signaling pathways in rheumatoid arthritis synovial fibroblasts 1. (2001). Available online at: http://journals.aai.org/jimmunol/article-pdf/167/9/5395/1143074/5395.pdf (Accessed September 2, 2024).
91. Hayer S, Niederreiter B, Kalkgruber M, Wanic K, Maißner J, Smolen JS, et al. Analysis of combined deficiency ofinterleukin-1 and -6 versus singledeficiencies in TNF-mediated arthritis and systemic bone loss. Bone Joint Res. (2022) 11:484–93. doi: 10.1302/2046-3758.117.BJR-2021-0481.R1
92. Favalli EG. Understanding the role of interleukin-6 (IL-6) in the joint and beyond: A comprehensive review of IL-6 inhibition for the management of rheumatoid arthritis. Rheumatology and Therapy. (2020) 7:473–516. doi: 10.6084/m9.figshare.12581825
93. Bai LK, Su YZ, Wang XX, Bai B, Zhang CQ, Zhang LY, et al. Synovial macrophages: past life, current situation, and application in inflammatory arthritis. Front Immunol. (2022) 13:905356. doi: 10.3389/fimmu.2022.905356
94. Zhao K, Ruan J, Nie L, Ye X, and Li J. Effects of synovial macrophages in osteoarthritis. Front Immunol. (2023) 14:1164137. doi: 10.3389/fimmu.2023.1164137
95. Wang C, De Francesco R, Lamers LA, Rinzema S, Frölich S, van Lent PLEM, et al. Transcriptomic profiling of osteoarthritis synovial macrophages reveals a tolerized phenotype compounded by a weak corticosteroid response. Rheumatology. (2024) 64(2):860–9. doi: 10.1093/rheumatology/keae161
96. Strizova Z, Benesova I, Bartolini R, Novysedlak R, Cecrdlova E, Foley LK, et al. M1/M2 macrophages and their overlaps - myth or reality? Clin Sci. (2023) 137:1067–93. doi: 10.1042/CS20220531
97. Beyer M, Mallmann MR, Xue J, Staratschek-Jox A, Vorholt D, Krebs W, et al. High-resolution transcriptome of human macrophages. PloS One. (2012) 7:1–16. doi: 10.1371/journal.pone.0045466
98. Duque GA and Descoteaux A. Macrophage cytokines: Involvement in immunity and infectious diseases. Front Immunol. (2014) 5:491. doi: 10.3389/fimmu.2014.00491
99. Chávez-Galán L, Olleros ML, Vesin D, and Garcia I. Much more than M1 and M2 macrophages, there are also CD169+ and TCR+ macrophages. Front Immunol. (2015) 6:263. doi: 10.3389/fimmu.2015.00263
100. Palmieri EM, McGinity C, Wink DA, and McVicar DW. Nitric oxide in macrophage immunometabolism: Hiding in plain sight. Metabolites. (2020) 10:1–34. doi: 10.3390/metabo10110429
101. Amin AR and Abramson SB. The role of nitric oxide in articular cartilage breakdown in osteoarthritis. Curr Opin Rheumatol. (1998) 10:263–8. doi: 10.1097/00002281-199805000-00018
102. Timur UT, Jahr H, Anderson J, Green DC, Emans PJ, Smagul A, et al. Identification of tissue-dependent proteins in knee OA synovial fluid. Osteoarthr. Cartil. (2021) 29:124–33. doi: 10.1016/j.joca.2020.09.005
103. Newby AC. Metalloproteinase expression in monocytes and macrophages and its relationship to atherosclerotic plaque instability. Arterioscler Thromb Vasc Biol. (2008) 28:2108–14. doi: 10.1161/ATVBAHA.108.173898
104. Huntley R, Jensen E, Gopalakrishnan R, and Mansky KC. Bone morphogenetic proteins: Their role in regulating osteoclast differentiation. Bone Rep. (2019) 10:1-9. doi: 10.1016/j.bonr.2019.100207
105. Phimphilai M, Zhao Z, Boules H, Roca H, and Franceschi RT. BMP signaling is required for RUNX2-dependent induction of the osteoblast phenotype. J Bone Miner. Res. (2006) 21:637–46. doi: 10.1359/jbmr.060109
106. Haltmayer E, Ribitsch I, Gabner S, Rosser J, Gueltekin S, Peham J, et al. Co-culture of osteochondral explants and synovial membrane as in vitro model for osteoarthritis. PloS One. (2019) 14:1–19. doi: 10.1371/journal.pone.0214709
Keywords: arthritis, in vitro models, tissue engineering, crosstalk, organ on a chip, disease modeling, cartilage, inflammation
Citation: Smith KWY, Fung SL, Wu H-F, Chiesa I, Vozzi G, De Maria C and Gottardi R (2025) Developing an in vitro osteochondral micro-physiological system for modeling cartilage-bone crosstalk in arthritis. Front. Immunol. 16:1495613. doi: 10.3389/fimmu.2025.1495613
Received: 12 September 2024; Accepted: 10 April 2025;
Published: 26 May 2025.
Edited by:
Elizabeth R. Balmayor, University Hospital RWTH Aachen, GermanyReviewed by:
Jay Patel, Emory University, United StatesTomas Gonzalez-Fernandez, Lehigh University, United States
Copyright © 2025 Smith, Fung, Wu, Chiesa, Vozzi, De Maria and Gottardi. This is an open-access article distributed under the terms of the Creative Commons Attribution License (CC BY). The use, distribution or reproduction in other forums is permitted, provided the original author(s) and the copyright owner(s) are credited and that the original publication in this journal is cited, in accordance with accepted academic practice. No use, distribution or reproduction is permitted which does not comply with these terms.
*Correspondence: Riccardo Gottardi, Z290dGFyZGlyQGNob3AuZWR1