- 1Immunology, Microbiology and Infectious Diseases, Instituto Murciano de Investigación Biosanitaria (IMIB)-Pascual Parrilla, Murcia, Spain
- 2Centro de Investigación Biomédica en Red de Enfermedades Raras (CIBERER), Instituto de Salud Carlos III, Madrid, Spain
- 3Departamento de Biología Celular e Histología, Facultad de BiologíaUniversidad de Murcia, Murcia, Spain
Introduction: Mutations in the gene encoding the protein containing the sterile alpha motif and the HD domain (SAMHD1) have been implicated in the occurrence of type I interferonopathies. SAMHD1 is also involved in blocking the replication of retroviruses and certain DNA viruses by reducing the intracellular amount of deoxynucleotide triphosphates (dNTPs). It has also been suggested that SAMHD1 negatively regulates interferon (IFN) and the inflammatory responses to viral infections; however, the functions and mechanisms of SAMHD1 in modulating innate immunity are still under study.
Methods: In our laboratory, we have generated Samhd1-deficient zebrafish larvae using CRISPR-Cas9 and studied its role in the activation of nuclear factor kappa B (NF-κB) and the induction of type I IFN (IFN-I).
Results: It was shown that Samhd1 deficiency results in the overactivation of the IFN-I response, assayed as the increased transcript levels of the Interferon Stimulated Genes (ISGs), but only if the larvae were stimulated with suboptimal doses of IFN-I. However, Samhd1-deficient larvae showed robust spontaneous activation of NF-κB, which led to increased larval resistance to Salmonella enterica serovar Typhimurium (STM) infection. Genetic experiments further showed that the activation of NF-κB in macrophages mediated the resistance of Samhd1-deficient larvae against STM.
Discussion: These findings highlight the evolutionary conserved functions of SAMHD1 in the negative regulation of the inflammatory response of vertebrates and reveal, for the first time, a critical role for SAMHD1 in the regulation of NF-κB in macrophages to clear intracellular bacterial infection.
Introduction
Homozygous mutations in the sterile alpha motif and HD domain-containing protein 1 (SAMHD1) gene are linked to Aicardi–Goutières syndrome (AGS), a rare inflammatory neurological disorder. This syndrome is characterized by the spontaneous production of type I interferon (IFN-I) and the upregulation of IFN-stimulated genes (ISGs), which resemble those of congenital viral infections (1–3).
SAMHD1 is a protein that plays a critical role in the immune response against viral infections (3–5), its deoxynucleoside triphosphate (dNTP) triphosphohydrolase (dNTPase) activity being one of its most studied antiviral characteristics. This activity plays a crucial role in regulating the DNA precursor pools in mammalian cells (6). In non-dividing cells of the myeloid lineage and in resting CD4-positive T cells, SAMHD1 restricts the infection of human immunodeficiency virus type 1 (HIV-1). However, in proliferating cells, SAMHD1 is phosphorylated by cyclin-dependent kinases (CDKs), which deactivates its restriction function and makes these cells susceptible to HIV-1 infection. Despite some controversy, SAMHD1 has also been proposed to exhibit nuclease activity on single-stranded RNAs, adding another layer to its antiviral features (7, 8). Beyond its direct antiviral activities, SAMHD1 has been suggested to play a role in the suppression of the innate immune responses to viral infections and inflammatory stimuli (5, 9, 10). This suppression is achieved through interactions with key proteins in the nuclear factor kappa B (NF-κB) and IFN-I pathways. Specifically, the SAMHD1 protein interacts with NF-κB1/2, reducing the phosphorylation of IκBα (NF-κB inhibitor alpha) and inhibiting the activation of NF-κB. SAMHD1 also reduces the induction of IFN-I by inhibiting the IKKϵ (inhibitor-κB kinase ϵ) phosphorylation of IRF7 (5, 11). Through these diverse mechanisms, SAMHD1 emerges as a crucial regulator of both antiviral defenses and inflammatory responses, underscoring its importance in maintaining immune homeostasis.
While SAMHD1 is associated with the immune response to viral infections, little is known about its possible role in immunity against bacterial pathogens. Similarly to viruses, bacteria have evolved to adapt to and counteract host inflammatory responses. Salmonella enterica serovar Typhimurium (STM) is a widely studied bacterial infection system due to the range of diseases caused. During infection, STM invades multiple cell types, including dendritic cells, epithelial cells, and macrophages. While macrophages typically serve as the frontline defense against invading bacterial pathogens, like the start of the restriction of STM infection (12, 13), they are essential for the establishment of systemic disease in a susceptible host (14–16). In macrophages, STM activates the canonical and non-canonical signaling pathways of NF-κB, which is the most important transcriptional regulator activating the inflammatory responses in the host against STM (17). In addition, one of the strategies that STM uses to evade the immune response and to favor its infection is the inhibition of the transduction pathways of NF-κB by the effector molecule SpvB from the pathogenicity island 2 type III secretion system (T3SS-2) (18).
To investigate the role of SAMHD1 during STM infection, we developed a Samhd1-deficient zebrafish model and examined the involvement of the NF-κB signaling pathway. The findings demonstrated that Samhd1 deficiency induced an increased IFN-I response only when larvae were exposed to a suboptimal dose of zebrafish IFN-I. Furthermore, a marked activation of NF-κB was observed, which was characterized by an increased NF-κB transcriptional activity. Notably, the enhanced NF-κB activation in Samhd1-deficient larvae correlated with increased resistance to STM infection, a response mediated by macrophages.
Materials and methods
Animals
Zebrafish (Danio rerio H.) were obtained from the Zebrafish International Resource Center and were mated, staged, raised, and processed as described (Westerfield, 2000 #23). The Tg(NFkB-RE:eGFP)sh235 line, referred to as nfkb:eGFP (19) and Tg(UAS:dn-nfkbiaa)sd35 (20), has been previously described. The experiments performed complied with the Guidelines of the European Union Council (Directive 2010/63/EU) and the Spanish RD 53/2013. The experiments and procedures were performed as approved by the Bioethical Committee of the University of Murcia (approval no. 669/2020).
Salmonella Typhimurium infection assays
STM 12023 (wild type, WT) was used. Overnight cultures in Luria–Bertani (LB) broth were diluted 1:5 in LB with 0.3 M NaCl, incubated at 37°C until 1.5 optical density at 600 nm was reached, and finally diluted in sterile phosphate-buffered saline (PBS). Larvae at 2 days post-fertilization (dpf) were anesthetized in embryo medium with 0.16 mg/ml tricaine, and 10 bacteria per larvae were microinjected in the yolk sac. The larvae were allowed to recover in egg water at 28–29°C and were monitored for clinical signs of disease or mortality over 5 days.
Analysis of gene expression
Total RNA was extracted from a pool of 25 zebrafish larvae (3 dpf) with the TRIzol reagent (Invitrogen, Carlsbad, CA, USA) following the manufacturer’s instructions and treated with DNase I, amplification grade (1 U/μg RNA; Invitrogen). The SuperScript VILO cDNA Synthesis Kit (Invitrogen) was used to synthesize first-strand cDNA with a random primer from 1 μg of the total RNA at 50°C for 50 min. Real-time PCR was performed with an ABI PRISM 7500 instrument (Applied Biosystems, Foster City, CA, USA) using SYBR Green PCR Core Reagents (Applied Biosystems). The reaction mixtures were incubated for 10 min at 95°C, followed by 40 cycles of 15 s at 95°C, 1 min at 60°C, and finally 15 s at 95°C, 1 min at 60°C, and 15 s at 95°C. For each mRNA, the gene expression was normalized to the ribosomal protein S11 gene (rps11) content in each sample using the Pfaffl method (27). The sequences of the used primers are listed in Supplementary Table S1. In all cases, each PCR was performed with triplicate samples and repeated at least twice with independent samples.
In vivo imaging
To study the reporter activity of NF-κB, 2-dpf nfkb:egfp larvae were anesthetized in embryo medium with 0.16 mg/ml buffered tricaine. Images of complete larvae were taken using a Leica MZ16FA fluorescence stereomicroscope. The fluorescence intensity from three biological replicates, each containing several larvae, was obtained and analyzed with ImageJ (FIJI) software.
CRISPR/Cas9, plasmid injections, and chemical treatments in zebrafish
The CRISPR RNA (crRNA) for zebrafish samhd1 (Supplementary Table S2) and the negative control crRNA (catalog no. 1072544, crSTD), as well as the tracrRNA (trans-activating tracrRNA; catalog no. 1072533), purchased from Integrated DNA Technologies (IDT, Coralville, IA, USA), were resuspended in nuclease-free duplex buffer to 100 µM. Of each, 1 μl was mixed and incubated for 5 min at 95°C for duplexing. After removing from the heat and cooling to room temperature, 1.43 µl of the nuclease-free duplex buffer was added to the duplex [guide RNA (gRNA) and crRNA + tracrRNA], giving a final concentration of 1,000 ng/µl. The injection mix was then prepared by mixing 1 µl of the duplex, 2.55 µl of the nuclease-free duplex buffer, 0.25 µl of Cas9 nuclease V3 (IDT, catalog no. 1081058), and 0.25 µl of phenol red, giving final concentrations of the gRNA duplex (250 ng/µl) and of Cas9 (500 ng/µl). The prepared mix was microinjected into the yolk of one-cell-stage embryos (0.5–1 nl per embryo) using a microinjector (Narishige, Amityville, NY, USA). The same amounts of gRNA were used in all the experimental groups. The efficiency of the gRNA was checked by amplifying the target sequence with a specific pair of primers (Supplementary Table S1) and the TIDE webtool (https://tide.nki.nl/) (Supplementary Figure S1).
pcDNA-IFNphi3 (GenBank accession no. NM_001111083) (21) was microinjected into the yolk of one-cell-stage embryos (1 pg/embryo). The 1-dpf embryos were manually dechorionated and 24 h post-fertilization treated by chemical bath immersion at 28°C. Baricitinib (Bar; MedChemExpress, Monmouth Junction, NJ, USA) was added to the water at 10 µM and BAY11-7082 (MedChemExpress) at 10 nM and renewed every day for the duration of the experiment (6 days). Incubation was carried out in 10-ml plates containing ≈100 larvae/well in egg water (including 60 μg/ml sea salts in distilled water) supplemented with 0.1% dimethyl sulfoxide (DMSO).
Statistical analyses
All statistical analyses were performed in GraphPad Prism 8. Data are shown as the mean ± SEM and were analyzed using one-way analysis of variance (ANOVA) and Tukey’s multiple range test to determine differences between groups. The differences between two samples were analyzed using Student’s t-test. A log-rank test was used to calculate the statistical differences in the survival of the different experimental groups.
Results
IFN stimulation is required to trigger an IFN-I response in Samhd1-deficient zebrafish larvae
An annotated samhd1 ortholog gene (Gene ID: 553453) to the human gene SAMHD1 was found in chromosome 23 of the zebrafish genome (ENSDARG00000071288.4) (Figure 1A). In addition, two zebrafish samhd1 paralogs were also annotated as LOC793232 (Gene ID: 793232) and si:ch211-233g6.2 (Gene ID: 100333959), both located in chromosome 1 (ENSDARG00000099421.2 and ENSDARG00000104626.2), which shared between them 94% of identity, while 58% and 57%, respectively, with the original samhd1 gene. Importantly, the two samhd1-like sequences lacked the SAM domain (Figure 1A), which is critical for the regulation and activation of mammalian SAMHD1 (22), suggesting their specialization in other functions. Consequently, in this work, we only focused on the samhd1 gene.
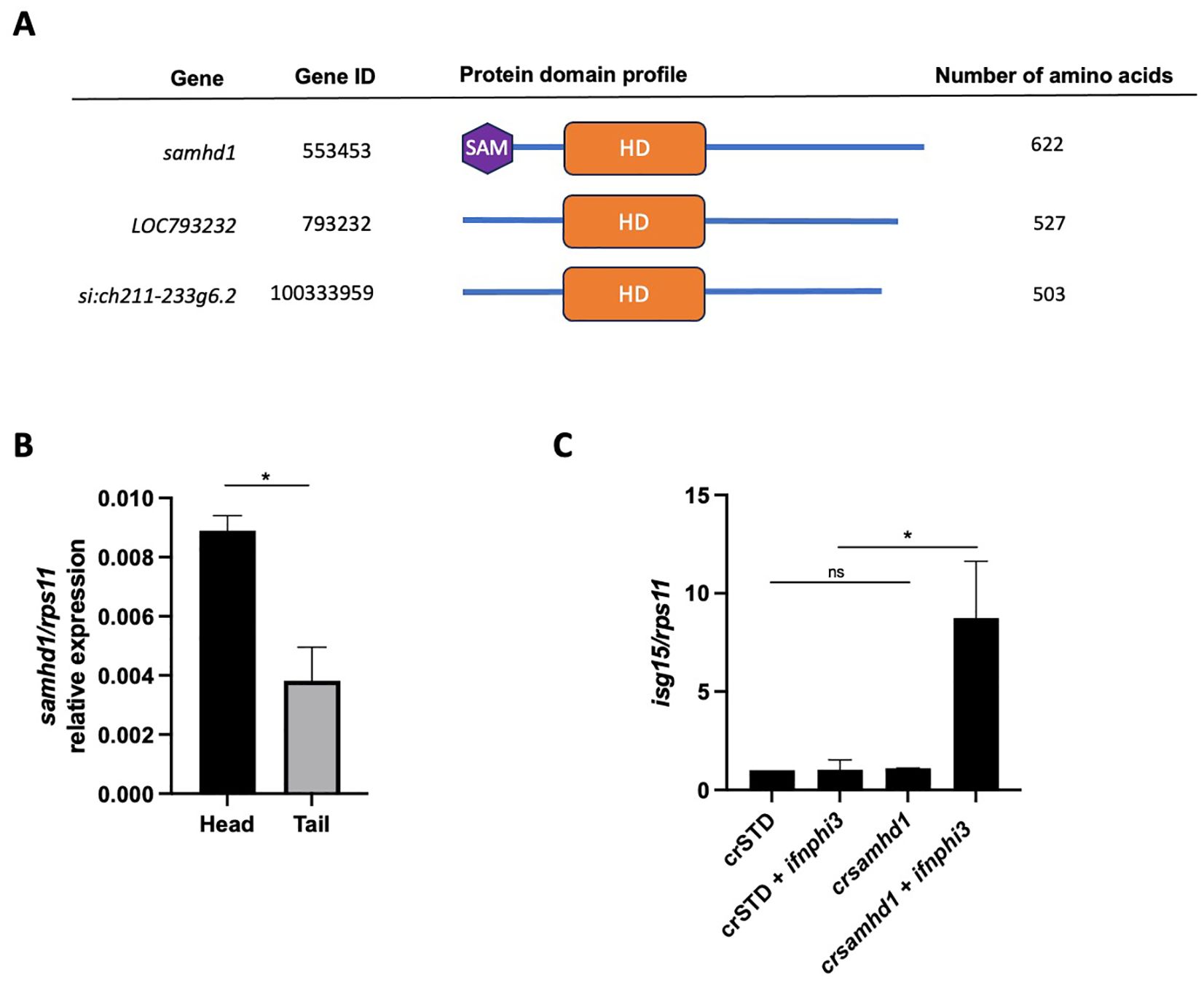
Figure 1. Interferon (IFN) stimulation is required to trigger interferon-stimulated gene (ISG) upregulation in Samhd1-deficient zebrafish larvae. (A) Diagram indicating the protein domains. (B) Transcript levels of samhd1 by reverse transcription PCR (RT-PCR) in the head and tail of zebrafish larvae. The average from three independent experiments, each with 25 pooled heads or tails, is shown. (C) Transcript levels of isg15 by reverse transcription quantitative PCR (RT-qPCR) in the head of zebrafish larvae injected with gRNA (samhd1 or STD)/Cas9 complexes with or without 1 pg/egg of pcDNA-IFNphi3. Bars show the mean ± SEM of three independent experiments, each with 25 pooled heads. Data are represented as fold change from control (STD). P-values were calculated using Student’s t-test in (B) and using one-way ANOVA and Tukey’s multiple range test in (C). ns, not significant. *p ≤ 0.05.
Similarly to Whiters et al. (23), we decided to measure the ISG expression in larval heads, given that the expression of samhd1 was higher in heads than in tails (Figure 1B). We next inactivated samhd1 using the CRISPR/Cas9 technology in one-cell-stage embryos (with a knockdown efficiency of 83.1%) (Supplementary Figure S1). We were not able to detect any significant difference in the expression levels of isg15 between the Samhd1-deficient and the control zebrafish larvae (Figure 1C). However, the addition of a suboptimal dose of the zebrafish IFN-I ifnphi3 was able to robustly increase the isg15 transcript levels in Samhd1-deficient larvae, but not in their sibling controls (Figure 1C). Of note is that a suboptimal dose of 1 pg/egg of IFN-I ifnphi3 was used in the experiment (Supplementary Figure S2).
Samhd1-deficient zebrafish larvae show spontaneous activation of NF-κB and high resistance to Salmonella Typhimurium infection
To study the inflammatory process in Samhd1-deficient zebrafish larvae, the transcriptional activity of NF-κB was analyzed using the reporter line nfkb:eGFP. As seen in Figures 2A, B, Samhd1-deficient larvae showed stronger NF-κB reporter activity than control larvae. As NFKB protects murine models from STM infection and this pathogen is able to hijack NF-κB with its effector protein SpvB (18), we next studied whether Samhd1-deficient zebrafish larvae were more resistant to STM infection than their WT counterparts. The results confirmed this hypothesis, showing that Samhd1-deficient zebrafish larvae were more resistant to STM infection, with almost a 30% higher survival than WT larvae (Figure 2C). Moreover, the gene expression analysis after 24 h of infection showed that the transcript levels of nfkb1 significantly increased in Samhd1-deficient infected larvae (Figure 3A) compared with control larvae. In addition, the expression of the il1b and isg15 transcripts also increased in Samhd1-deficient larvae following infection (Figures 3B, C). Interestingly, the mRNA levels of tnfα and ifng1r from the Samhd1-deficient infected larvae did not overcome those of the control post-infection (Figures 3D, E). Finally, the transcript levels of cxcl8a were unaffected by Samhd1 deficiency independently of infection (Figure 3F). Collectively, these findings indicate that Samhd1-deficient larvae display a heightened inflammatory state, facilitating a more robust and accelerated response to pathogen infection compared with control larvae.
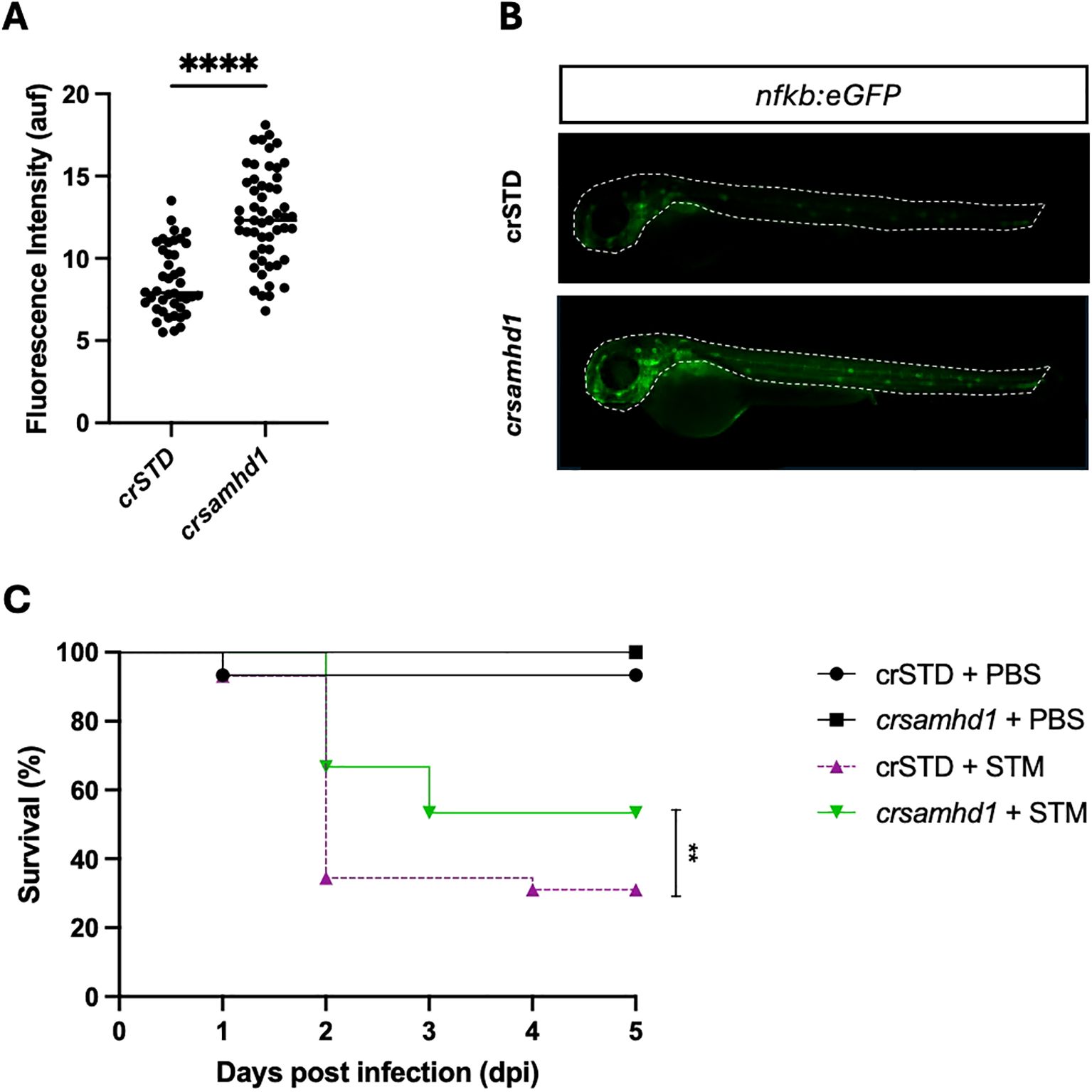
Figure 2. Samhd1 deficiency results in the spontaneous activation of nuclear factor kappa B (NF-κB) and hyperresistance to infection with Salmonella enterica serovar Typhimurium (STM). (A) Real-time visualization of NF-κB activation in Samhd1-deficient zebrafish larvae. The nfkb:eGFP reporter zebrafish line injected with gRNA (samhd1 or STD)/Cas9 complexes were analyzed using fluorescence microscopy and quantified. Each dot represents a larva, and the mean ± SEM for each experimental group is also shown. (B) Representative images of whole larvae are shown, and the region of interest (ROI) used to quantify the fluorescence in the nfkb:eGFP reporter line is indicated as a dot line in the images. (C) Zebrafish one-cell embryos were injected with gRNA (samhd1 or STD)/Cas9 complexes, dechorionated, and infected at 2 days post-fertilization via the yolk sac with STM at a multiplicity of infection (MOI) of 10 or phosphate-buffered saline (PBS), with the number of surviving larvae counted daily during the next 5 days. A total number of >100 specimens/treatment. P-values were calculated using Student’s t test in (A) and long-range test in (C). **p ≤ 0.01, ****p ≤ 0.0001.
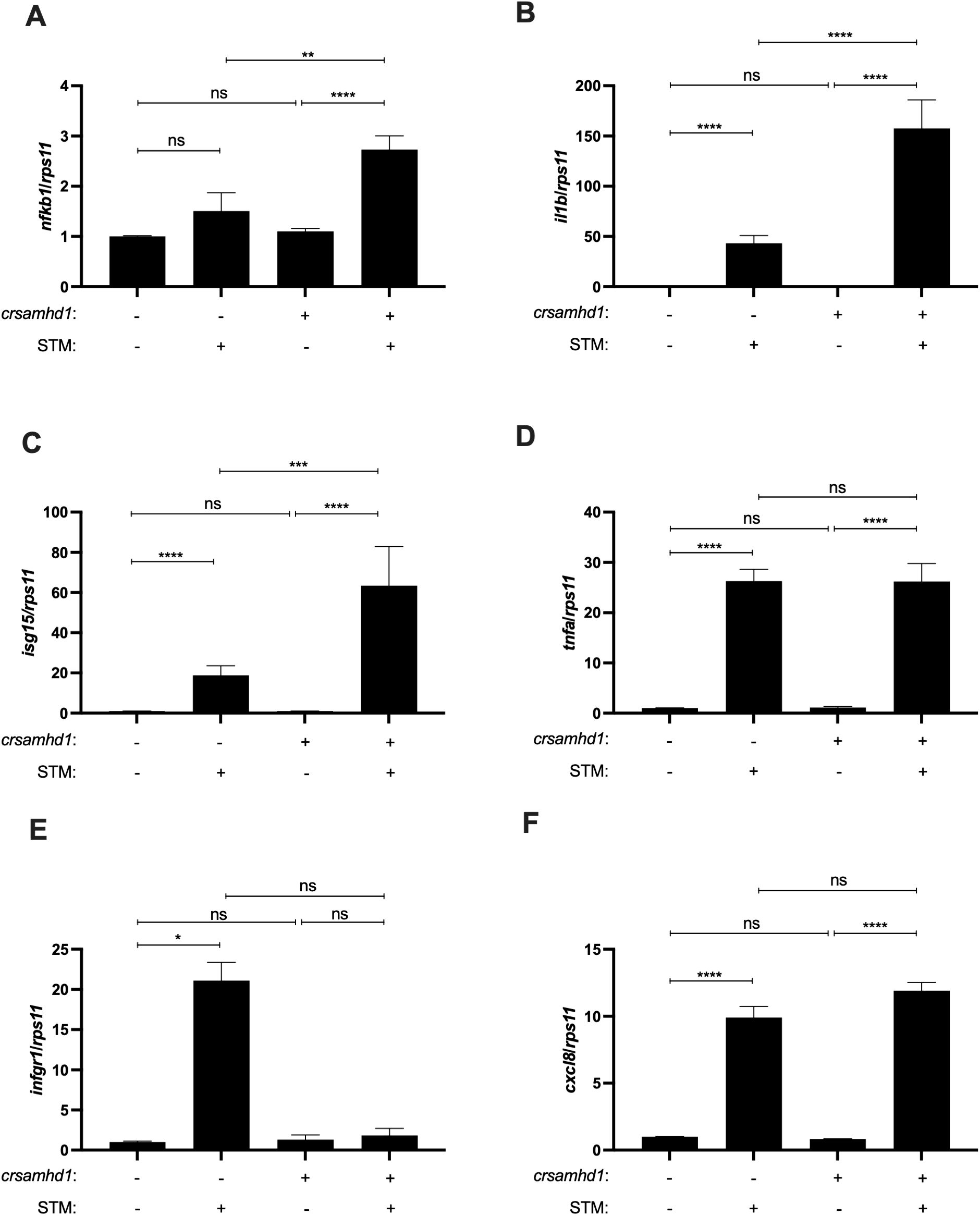
Figure 3. Samhd1 deficiency leads to an activated inflammatory state. (A–F) Transcript levels of nfkb1 (A), il1b (B) , isg15 (C), tnfα (D) , ifng1r (E), and cxcl8a (F) in larvae injected with gRNA (samhd1 or STD)/Cas9 complexes infected or not with Salmonella enterica serovar Typhimurium (STM) for 24 h assayed using reverse transcription quantitative PCR (RT-qPCR). Bars show the mean ± SEM of three independent experiments, each with 25 pooled larvae. Data are represented as fold change from control. P-values were calculated using one-way ANOVA. ns, not significant. *p ≤ 0.05, **p ≤ 0.01, ***p ≤ 0.001, ****p ≤ 0.0001.
Pharmacological inhibition of NF-κB abolishes the protective effect of Samhd1 deficiency in zebrafish larvae against Salmonella Typhimurium infection
To further validate the role of NF-κB in the resistance of Samhd1-deficient larvae to infection, we treated the larvae with BAY11-7082, an NF-κB inhibitor, and conducted a challenge with STM. As shown in Figure 4A, although the Samhd1-deficient larvae treated with the NF-κB inhibitor exhibited mortality rates comparable to those of WT larvae, the treatment fully abolished the increased bacterial resistance of Samdh1-deficient larvae (Figure 4A). Moreover, we used baricitinib, a JAK1/JAK2 inhibitor, which is able to suppress the IFN-I response in zebrafish larvae (24). Consistent with our expectations, treatment with baricitinib did not alter the susceptibility of Samhd1-deficient larvae to STM infection, which showed a survival rate approximately 30% higher than that of WT larvae and similar to that of untreated Samhd1-deficient larvae (Figure 4B). These findings support the hypothesis that the activation of NF-κB is crucial for the enhanced survival observed in Samhd1-deficient larvae.
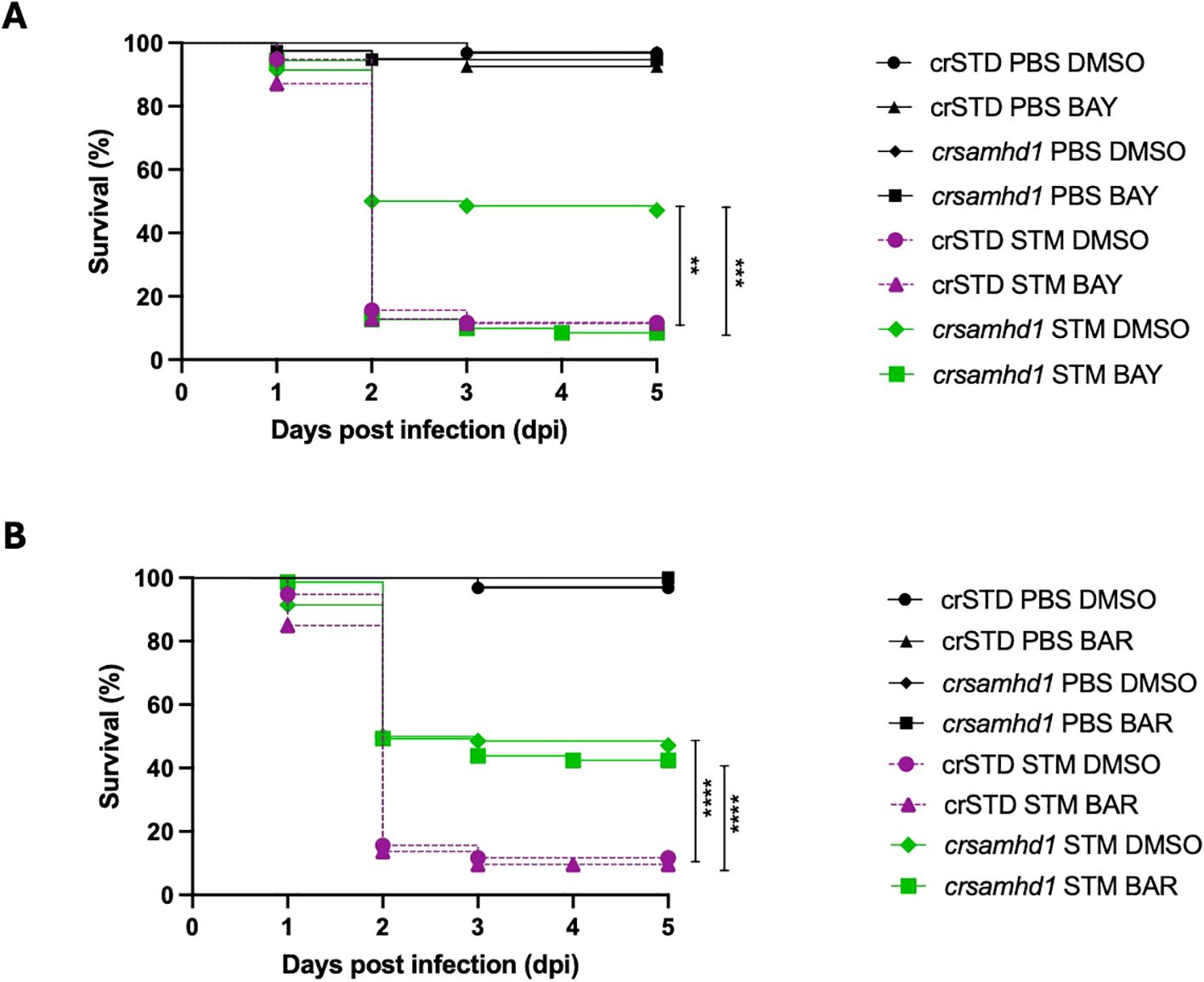
Figure 4. Pharmacological inhibition of nuclear factor kappa B (NF-κB) abolishes the protection of Samhd1-deficient zebrafish larvae to infection with Salmonella enterica serovar Typhimurium (STM). Zebrafish one-cell embryos were injected with gRNA (samhd1 or crSTD)/Cas9 complexes, dechorionated at 24 h, and treated by bath immersion with 10 nM BAY11-7082 (BAY) (A) or with 10 μM baricitinib (B). Drugs were renewed every day for the next 6 days. Controls were incubated with 0.1% dimethyl sulfoxide (DMSO). Larvae were infected at 2 days post-fertilization via the yolk sac with STM at a multiplicity of infection (MOI) of 10, with the number of surviving larvae counted daily during the next 5 days. A total number of >100 specimens/treatment. P-values were calculated using a long-range test. **p ≤ 0.01, ***p ≤ 0.001, ****p ≤ 0.0001.
NF-κB activation in macrophages is responsible for the resistance of Samhd1-deficient zebrafish larvae to Salmonella Typhimurium infection
The above results, together with the critical dual role of macrophages in STM dissemination and clearance (12–16), prompted us to examine whether the activation of NF-κB in the macrophages of Samhd1-deficient larvae mediated their hyperresistance to STM infection. To achieve this, we used the transgenic line Tg(UAS:dn-nfkbiaa), which expresses a dominant negative (DN) form of the nuclear factor of kappa light polypeptide gene enhancer in B-cell inhibitor, alpha a (nfkbiaa) and functions as an inhibitor of NF-κB (20). This line was outcrossed with the Tg(mpeg1:gal4) line, which allows exclusively expressing the DN-Nfkbiaa in macrophages. One-cell-stage embryos were injected with samhd1 crRNA/tracrRNA/Cas9 complexes and subsequently infected with STM, as previously described. Larvae with macrophages expressing DN-Nfkbiaa lost their hyperresistance to infection (Figure 5A), behaving similarly to control larvae, indicating that the activation of NF-κB in Samhd1-deficient macrophages is essential for their enhanced resistance to STM infection. Figure 5B shows a schematic model of the restriction of NF-κB activation by Samhd1 in WT macrophages and the hyperresistance of Samhd-1-deficient macrophages to STM infection through the induction of NF-κB activation.
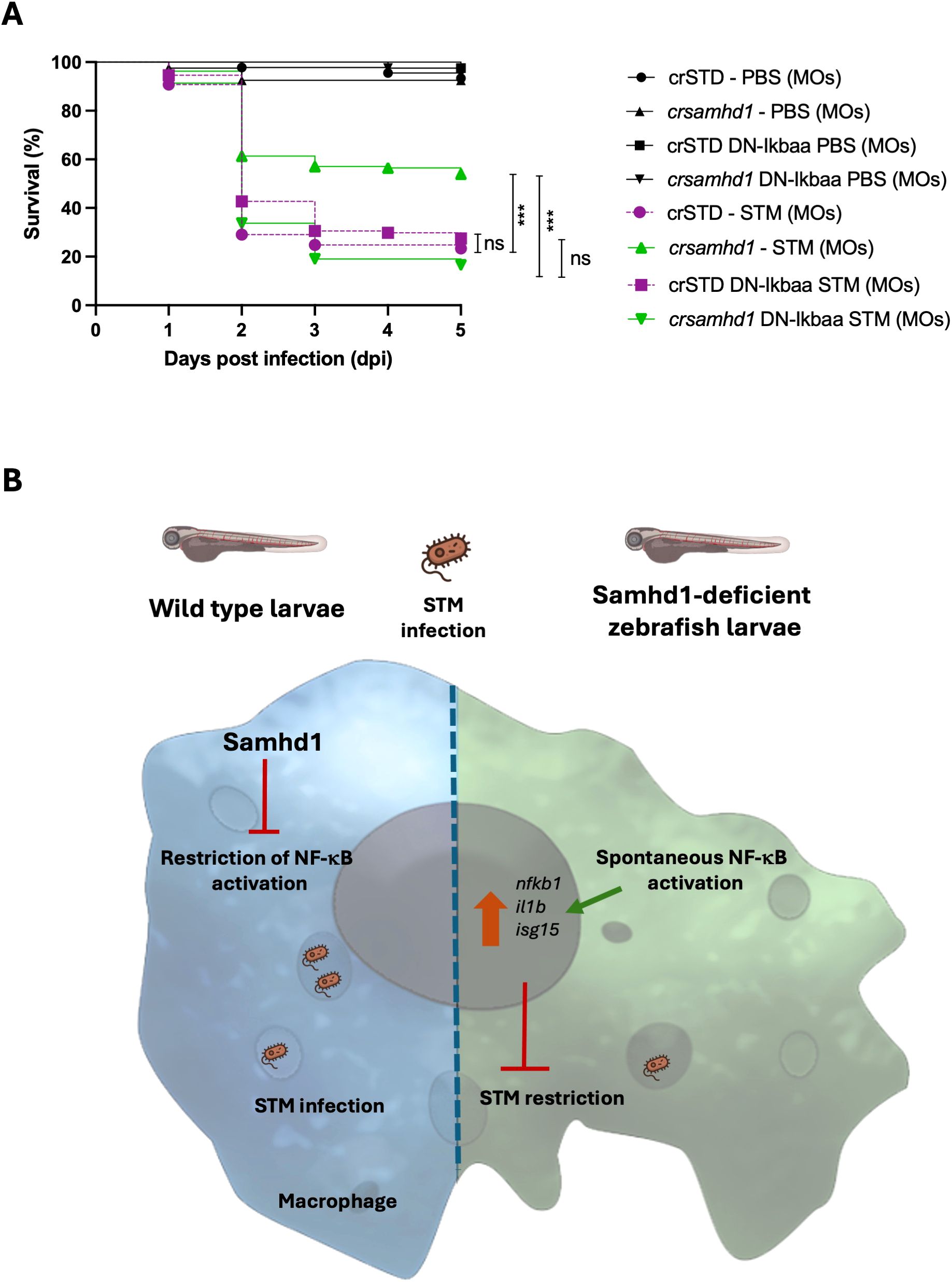
Figure 5. Genetic inhibition of nuclear factor kappa B (NF-κB) in macrophages abolishes the hyperresistance of Samhd1-deficient zebrafish larvae to infection with Salmonella enterica serovar Typhimurium. (A) The zebrafish line expressing a dominant negative (DN) form of Nfkbiaa, Tg(UAS:dn-nfkbiaa), was outcrossed with the transgenic line Tg(mpeg1:gal4), and one-cell-stage embryos were injected with gRNA (samhd1 or STD)/Casp9 complexes and infected with STM as previously described. The average from two independent experiments with >80 larvae per group is shown. P-values were calculated using a long-range test. ns, not significant. ***p ≤ 0.001. (B) Model showing the restriction of NF-κB activation by Samhd1 in wild-type macrophages and the hyperresistance of Samhd-1deficient macrophages to STM infection through the induction of NF-κB activation.
Discussion
The role of SAMHD1 in modulating the immune response has been extensively studied in human and mouse models, where it has been shown to negatively regulate both the innate and adaptive immunity following viral infections or inflammatory stimuli, primarily through its interactions with proteins in the NF-κB and IFN-I pathways (4, 5, 11). As in other studies in zebrafish, in our model of Samhd1-deficient larvae, the upregulation of ISGs was moderated compared with that in human or mouse models and was only observed when a suboptimal stimulus was added (23). However, our zebrafish model, similar to the mouse model, did not develop any observable neurological phenotype (25, 26), contrary to what occurred in other zebrafish models (23, 27). The variability of the phenotypes found between studies could be due to the different techniques used to achieve the model, as transient gene knockdown by morpholinos (27) versus a stable mutant zebrafish line (23) or knockdown using CRISPR/Cas9 in this study. It is also important to consider that zebrafish live in a highly pathogen-enriched environment and that they possess compensatory mechanisms to avoid a permanent inflammatory state (28–31), and this could also be a reason for the type I interferonopathy models in zebrafish being very difficult to study and to determine a robust phenotype. Moreover, it is also logical to think that the knockdown samhd1 model in this study does not show neurological phenotypes as it does not have a constitutive activation of IFN-I, which might be directly related to the overproduction of IFN-I (23, 27), as also mentioned in other AGS mouse studies (32, 33). These results also suggest that the characteristics of the animal facilities could also play a role in the study of the activation of the innate immune system in zebrafish, as the presence of microbial infections, particular microbiota, or other pro-inflammatory circumstances may contribute to AGS development.
One of the most interesting results in this study is the increased activation of NF-κB in Samhd1-deficient larvae. Similarly, this phenotype was also found after the depletion of SAMHD1 in human and mouse models (4, 5, 25, 34, 35). This feature has been widely studied in viral infection systems to elucidate the restriction mechanism of SAMHD1, with different, even contradictory, results being obtained depending on the pathogen used. On the one hand, Kim et al. (4) observed that SAMHD1 deficiency activated the NF-κB innate immune pathway, resulting in increased viral replication through transcriptional activation of the human cytomegalovirus (HCMV) MIE gene promoter. On the other hand, Chen et al. (5) demonstrated that Sendai virus (SeV)-infected SAMHD1-silenced human monocytic cells or primary macrophages led to the increased nuclear accumulation of NF-κB, induction of IFN-I, and reduction of SeV nucleoprotein mRNA, which was attributed to the IFN-I-mediated inhibition of SeV replication.
All of these studies with SAMHD1 focused on the immune response to viral infections; however, little is known about its possible role in immunity against bacterial pathogens. In this context, we performed a challenge against STM in which the Samhd1-deficient larvae were more resistant to the infection. To further study the mechanism, we wanted to determine which cells were implicated in the activation of NF-κB and, hence, in the increased survival rate of Samhd1-deficient larvae. It is well known that SAMHD1 is highly expressed in macrophages, dendritic cells, and CD4+ T cells, where it restricts retroviral infections (36–39). It has been recently reported that SAMHD1 disrupts the interaction between the upstream kinase TAK1 and IKKα or IKKβ, inhibiting the phosphorylation of IκBα and the activation of NF-κB (11). Furthermore, it is also well known that STM uses macrophages to replicate and establish systemic diseases (14–16, 40–43) and that its SpvB of the T3SS-2 has a potent and specific ability to prevent the activation of NF-κB by targeting IKKβ (18). Taking all these together, we decided to specifically block the activation of NF-κB in the macrophages of Samhd1-deficient larvae by expressing a DN-Nfkbiaa. The results demonstrated that the activation of NF-κB in macrophages was responsible for the high resistance of Samhd1-deficient larvae to STM infection.
In summary, this study is the first to reveal that Samhd1 plays a crucial role in restricting the macrophage-mediated clearance of STM infection. While previous studies have focused on the role of SAMHD1 in modulating the immune responses to viral infections, the findings of this study highlight its importance in bacterial immunity. It was demonstrated that Samhd1-deficient zebrafish larvae exhibit enhanced resistance to STM, which is driven by the increased activation of NF-κB in macrophages. This suggests that the loss of Samhd1 function allows for a more robust inflammatory response, facilitating a more effective pathogen clearance. These results open new avenues for exploring the role of SAMHD1 in bacterial infections and its potential as a therapeutic target in host–pathogen interactions.
Data availability statement
The raw data supporting the conclusions of this article will be made available by the authors, without undue reservation.
Ethics statement
The animal study was approved by Bioethical Committee of the University of Murcia (approval number #669/2020). The study was conducted in accordance with the local legislation and institutional requirements.
Author contributions
AM-L: Investigation, Methodology, Supervision, Writing – original draft, Writing – review & editing. SDT: Conceptualization, Investigation, Methodology, Visualization, Writing – review & editing. FM-M: Conceptualization, Data curation, Investigation, Writing – review & editing. CA-O: Investigation, Writing – review & editing. JL-G: Investigation, Methodology, Writing – review & editing. SC: Conceptualization, Methodology, Supervision, Writing – review & editing. VM: Funding acquisition, Investigation, Resources, Supervision, Validation, Visualization, Writing – review & editing. DG-M: Conceptualization, Funding acquisition, Methodology, Resources, Supervision, Visualization, Writing – review & editing, Writing – original draft.
Funding
The author(s) declare that financial support was received for the research and/or publication of this article. This study has been funded by Instituto de Salud Carlos III (ISCIII) through the project "PI22/00879” and "CP21/00028" and co-funded by the European Union to DG-M and Consejería de Salud de la Región de Murcia (ZEBER project to VM), Instituto de Salud Carlos III (ISCIII) to SDT through the project "CP23/00049". The funders had no role in the study design, data collection and analysis, decision to publish, or preparation of the manuscript.
Acknowledgments
We thank I. Fuentes and P. J. Martínez for their excellent technical assistance, S.A.
Conflict of interest
The authors declare that the research was conducted in the absence of any commercial or financial relationships that could be construed as a potential conflict of interest.
Generative AI statement
The author(s) declare that no Generative AI was used in the creation of this manuscript.
Publisher’s note
All claims expressed in this article are solely those of the authors and do not necessarily represent those of their affiliated organizations, or those of the publisher, the editors and the reviewers. Any product that may be evaluated in this article, or claim that may be made by its manufacturer, is not guaranteed or endorsed by the publisher.
Supplementary material
The Supplementary Material for this article can be found online at: https://www.frontiersin.org/articles/10.3389/fimmu.2025.1509725/full#supplementary-material
Supplementary Figure 1 | Analysis of the efficiency of CRISPR/Cas9 edition. The edition efficiency of gsamhd1/Cas9 complexes was checked by amplifying the target sequence with a specific pair of primers (see Supplementary Table S1) and quantifying the rate of nonhomologous end joining mediated repair using the TIDE webtool (https://tide.nki.nl/). All insertions and deletions (INDELS) at the target site are shown.
Supplementary Figure 2 | IFN stimulation. Transcript levels of isg15 RT-qPCR in head of zebrafish larvae injected with gRNAs (samhd1 or STD)/Cas9 complexes with or without 10 pg of pcDNA-IFNphi3 or 75 pg in wild type eggs (A). Transcript levels of isg15 RT-qPCR in head of zebrafish larvae injected with gRNAs (samhd1 or STD)/Cas9 complexes with or without 1 pg of pcDNA-IFNphi3 (B). Data is represented as fold change from control. p-values were calculated using Student’s t-test; ***p≤ 0.001.
References
1. Rice GI, Bond J, Asipu A, Brunette RL, Manfield IW, Carr IM, et al. Mutations involved in Aicardi-Goutieres syndrome implicate SAMHD1 as regulator of the innate immune response. Nat Genet. (2009) 41:829–32. doi: 10.1038/ng.373
2. Rice GI, Forte GM, Szynkiewicz M, Chase DS, Aeby A, Abdel-Hamid MS, et al. Assessment of interferon-related biomarkers in Aicardi-Goutieres syndrome associated with mutations in TREX1, RNASEH2A, RNASEH2B, RNASEH2C, SAMHD1, and ADAR: a case-control study. Lancet Neurol. (2013) 12:1159–69. doi: 10.1016/S1474-4422(13)70258-8
3. Crow YJ, Rehwinkel J. Aicardi-Goutieres syndrome and related phenotypes: linking nucleic acid metabolism with autoimmunity. Hum Mol Genet. (2009) 18:R130–6. doi: 10.1093/hmg/ddp293
4. Kim ET, Roche KL, Kulej K, Spruce LA, Seeholzer SH, Coen DM, et al. SAMHD1 modulates early steps during human cytomegalovirus infection by limiting NF-kappaB activation. Cell Rep. (2019) 28:434–448 e6. doi: 10.1016/j.celrep.2019.06.027
5. Chen S, Bonifati S, Qin Z, St Gelais C, Kodigepalli KM, Barrett BS, et al. SAMHD1 suppresses innate immune responses to viral infections and inflammatory stimuli by inhibiting the NF-kappaB and interferon pathways. Proc Natl Acad Sci USA. (2018) 115:E3798–807. doi: 10.1073/pnas.1801213115
6. Franzolin E, Pontarin G, Rampazzo C, Miazzi C, Ferraro P, Palumbo E, et al. The deoxynucleotide triphosphohydrolase SAMHD1 is a major regulator of DNA precursor pools in mammalian cells. Proc Natl Acad Sci U S A. (2013) 110:14272–7. doi: 10.1073/pnas.1312033110
7. Beloglazova N, Flick R, Tchigvintsev A, Brown G, Popovic A, Nocek B, et al. Nuclease activity of the human SAMHD1 protein implicated in the Aicardi-Goutieres syndrome and HIV-1 restriction. J Biol Chem. (2013) 288:8101–10. doi: 10.1074/jbc.M112.431148
8. Ryoo J, Choi J, Oh C, Kim S, Seo M, Kim SY, et al. The ribonuclease activity of SAMHD1 is required for HIV-1 restriction. Nat Med. (2014) 20:936–41. doi: 10.1038/nm.3626
9. Behrendt R, Schumann T, Gerbaulet A, Nguyen LA, Schubert N, Alexopoulou D, et al. Mouse SAMHD1 has antiretroviral activity and suppresses a spontaneous cell-intrinsic antiviral response. Cell Rep. (2013) 4:689–96. doi: 10.1016/j.celrep.2013.07.037
10. Martinez-Lopez A, Martin-Fernandez M, Buta S, Kim B, Bogunovic D, Diaz-Griffero F. SAMHD1 deficient human monocytes autonomously trigger type I interferon. Mol Immunol. (2018) 101:450–60. doi: 10.1016/j.molimm.2018.08.005
11. Yang H, Espada CE, Phillips S, Martinez N, Kenney AD, Yount JS, et al. The host antiviral protein SAMHD1 suppresses NF-kappaB activation by interacting with the IKK complex during inflammatory responses and viral infection. J Biol Chem. (2023) 299:104750. doi: 10.1016/j.jbc.2023.104750
12. Niedergang F, Sirard JC, Blanc CT, Kraehenbuhl JP. Entry and survival of Salmonella typhimurium in dendritic cells and presentation of recombinant antigens do not require macrophage-specific virulence factors. Proc Natl Acad Sci U S A. (2000) 97:14650–5. doi: 10.1073/pnas.97.26.14650
13. Wick MJ, Harding CV, Twesten NJ, Normark SJ, Pfeifer JD. The phoP locus influences processing and presentation of Salmonella typhimurium antigens by activated macrophages. Mol Microbiol. (1995) 16:465–76. doi: 10.1111/j.1365-2958.1995.tb02411.x
14. Gog JR, Murcia A, Osterman N, Restif O, McKinley TJ, Sheppard M, et al. Dynamics of Salmonella infection of macrophages at the single cell level. J R Soc Interface. (2012) 9:2696–707. doi: 10.1098/rsif.2012.0163
15. Fields PI, Swanson RV, Haidaris CG, Heffron F. Mutants of Salmonella typhimurium that cannot survive within the macrophage are avirulent. Proc Natl Acad Sci U S A. (1986) 83:5189–93. doi: 10.1073/pnas.83.14.5189
16. Leung KY, Finlay BB. Intracellular replication is essential for the virulence of Salmonella typhimurium. Proc Natl Acad Sci U S A. (1991) 88:11470–4. doi: 10.1073/pnas.88.24.11470
17. Keestra-Gounder AM, Tsolis RM, Baumler AJ. Now you see me, now you don't: the interaction of Salmonella with innate immune receptors. Nat Rev Microbiol. (2015) 13:206–16. doi: 10.1038/nrmicro3428
18. Yang S, Deng Q, Sun L, Zhu Y, Dong K, Wu S, et al. Salmonella effector spvB inhibits NF-kappaB activity via KEAP1-mediated downregulation of IKKbeta. Front Cell Infect Microbiol. (2021) 11:641412. doi: 10.3389/fcimb.2021.641412
19. Kanther M, Sun X, Muhlbauer M, Mackey LC, Flynn EJ 3rd, Bagnat M, et al. Microbial colonization induces dynamic temporal and spatial patterns of NF-kappaB activation in the zebrafish digestive tract. Gastroenterology. (2011) 141:197–207. doi: 10.1053/j.gastro.2011.03.042
20. Espin-Palazon R, Stachura DL, Campbell CA, Garcia-Moreno D, Del Cid N, Kim AD, et al. Proinflammatory signaling regulates hematopoietic stem cell emergence. Cell. (2014) 159:1070–85. doi: 10.1016/j.cell.2014.10.031
21. Lopez-Munoz A, Roca FJ, Meseguer J, Mulero V. New insights into the evolution of IFNs: zebrafish group II IFNs induce a rapid and transient expression of IFN-dependent genes and display powerful antiviral activities. J Immunol. (2009) 182:3440–9. doi: 10.4049/jimmunol.0802528
22. Buzovetsky O, Tang C, Knecht KM, Antonucci JM, Wu L, Ji X, et al. The SAM domain of mouse SAMHD1 is critical for its activation and regulation. Nat Commun. (2018) 9:411. doi: 10.1038/s41467-017-02783-8
23. Withers SE, Rowlands CF, Tapia VS, Hedley F, Mosneag IE, Crilly S, et al. Characterization of a mutant samhd1 zebrafish model implicates dysregulation of cholesterol biosynthesis in Aicardi-Goutieres syndrome. Front Immunol. (2023) 14:1100967. doi: 10.3389/fimmu.2023.1100967
24. Bernal-Bermudez B, Martinez-Lopez A, Martinez-Morcillo FJ, Tyrkalska SD, Martinez-Menchon T, Mesa-Del-Castillo P, et al. A zebrafish model of Ifih1-driven Aicardi-Goutieres syndrome reproduces the interferon signature and the exacerbated inflammation of patients. Front Immunol. (2023) 14:1294766. doi: 10.3389/fimmu.2023.1294766
25. Rehwinkel J, Maelfait J, Bridgeman A, Rigby R, Hayward B, Liberatore RA, et al. SAMHD1-dependent retroviral control and escape in mice. EMBO J. (2013) 32:2454–62. doi: 10.1038/emboj.2013.163
26. Behrendt R, Roers A. Mouse models for Aicardi-Goutieres syndrome provide clues to the molecular pathogenesis of systemic autoimmunity. Clin Exp Immunol. (2014) 175:9–16. doi: 10.1111/cei.12147
27. Kasher PR, Jenkinson EM, Briolat V, Gent D, Morrissey C, Zeef LA, et al. Characterization of samhd1 morphant zebrafish recapitulates features of the human type I interferonopathy Aicardi-Goutieres syndrome. J Immunol. (2015) 194:2819–25. doi: 10.4049/jimmunol.1403157
28. Berczi I, Bertok L, Bereznai T. Comparative studies on the toxicity of Escherichia coli lipopolysaccharide endotoxin in various animal species. Can J Microbiol. (1966) 12:1070–1. doi: 10.1139/m66-143
29. Suttle CA. Marine viruses–major players in the global ecosystem. Nat Rev Microbiol. (2007) 5:801–12. doi: 10.1038/nrmicro1750
30. Falkowski PG, de Vargas C. Genomics and evolution. Shotgun sequencing in the sea: a blast from the past? Science. (2004) 304:58–60. doi: 10.1126/science.1097146
31. Breitbart M, Bonnain C, Malki K, Sawaya NA. Phage puppet masters of the marine microbial realm. Nat Microbiol. (2018) 3:754–66. doi: 10.1038/s41564-018-0166-y
32. Ohto T, Tayeh AA, Nishikomori R, Abe H, Hashimoto K, Baba S, et al. Intracellular virus sensor MDA5 mutation develops autoimmune myocarditis and nephritis. J Autoimmun. (2022) 127:102794. doi: 10.1016/j.jaut.2022.102794
33. Emralino FL, Satoh S, Sakai N, Takami M, Takeuchi F, Yan N, et al. Double-stranded RNA induces mortality in an MDA5-mediated type I interferonopathy model. J Immunol. (2022) 209:2093–103. doi: 10.4049/jimmunol.2200367
34. Espada CE, St Gelais C, Bonifati S, Maksimova VV, Cahill MP, Kim SH, et al. TRAF6 and TAK1 contribute to SAMHD1-mediated negative regulation of NF-kappaB signaling. J Virol. (2021) 95(3):10.1128/jvi.01970-20. doi:10.1128/jvi.01970-20
35. Espada CE, Sari L, Cahill MP, Yang H, Phillips S, Martinez N, et al. SAMHD1 impairs type I interferon induction through the MAVS, IKKepsilon, and IRF7 signaling axis during viral infection. J Biol Chem. (2023) 299:104925. doi: 10.1016/j.jbc.2023.104925
36. Laguette N, Sobhian B, Casartelli N, Ringeard M, Chable-Bessia C, Segeral E, et al. SAMHD1 is the dendritic- and myeloid-cell-specific HIV-1 restriction factor counteracted by Vpx. Nature. (2011) 474:654–7. doi: 10.1038/nature10117
37. Pan X, Baldauf HM, Keppler OT, Fackler OT. Restrictions to HIV-1 replication in resting CD4+ T lymphocytes. Cell Res. (2013) 23:876–85. doi: 10.1038/cr.2013.74
38. Laguette N, Benkirane M. How SAMHD1 changes our view of viral restriction. Trends Immunol. (2012) 33:26–33. doi: 10.1016/j.it.2011.11.002
39. Luban J. Innate immune sensing of HIV-1 by dendritic cells. Cell Host Microbe. (2012) 12:408–18. doi: 10.1016/j.chom.2012.10.002
40. Jiang L, Wang P, Song X, Zhang H, Ma S, Wang J, et al. Salmonella Typhimurium reprograms macrophage metabolism via T3SS effector SopE2 to promote intracellular replication and virulence. Nat Commun. (2021) 12:879. doi: 10.1038/s41467-021-21186-4
41. Leiba J, Sipka T, Begon-Pescia C, Bernardello M, Tairi S, Bossi L, et al. Dynamics of macrophage polarization support Salmonella persistence in a whole living organism. Elife. (2024) 13:e89828. doi: 10.7554/eLife.89828
42. Eisele NA, Ruby T, Jacobson A, Manzanillo PS, Cox JS, Lam L, et al. Salmonella require the fatty acid regulator PPARdelta for the establishment of a metabolic environment essential for long-term persistence. Cell Host Microbe. (2013) 14:171–82. doi: 10.1016/j.chom.2013.07.010
Keywords: SAMHD1, Salmonella typhimurium, NF-kB, zebrafish, innate immunity
Citation: Martínez-López A, Tyrkalska SD, Martínez-Morcillo FJ, Abenza-Olmos C, Lozano-Gil JM, Candel S, Mulero V and García-Moreno D (2025) SAMHD1 deficiency enhances macrophage-mediated clearance of Salmonella Typhimurium via NF-κB activation in zebrafish. Front. Immunol. 16:1509725. doi: 10.3389/fimmu.2025.1509725
Received: 11 October 2024; Accepted: 28 March 2025;
Published: 25 April 2025.
Edited by:
Saba Valadkhan, Case Western Reserve University, United StatesReviewed by:
Marie Wrande, Uppsala University, SwedenHolly Stephenson, University of Plymouth, United Kingdom
Copyright © 2025 Martínez-López, Tyrkalska, Martínez-Morcillo, Abenza-Olmos, Lozano-Gil, Candel, Mulero and García-Moreno. This is an open-access article distributed under the terms of the Creative Commons Attribution License (CC BY). The use, distribution or reproduction in other forums is permitted, provided the original author(s) and the copyright owner(s) are credited and that the original publication in this journal is cited, in accordance with accepted academic practice. No use, distribution or reproduction is permitted which does not comply with these terms.
*Correspondence: Alicia Martínez-López, YWxpY2lhLm1hcnRpbmV6MzRAdW0uZXM=; Victoriano Mulero, dm11bGVyb0B1bS5lcw==; Diana García-Moreno, ZGlhbmFnbUB1bS5lcw==
†These authors have contributed equally to this work