- The Institute of Low Temperature Science, Hokkaido University, Sapporo, Japan
Microbes play essential roles in arsenic transformation in the environment. Microbial arsenite oxidation is catalyzed by either of two distantly related arsenite oxidases, referred to as AIO and ARX. The arx genes encoding ARX and its regulatory proteins were originally defined in the genomes of gammaproteobacteria isolated from an alkaline soda lake. The arx gene cluster has been identified in a limited number of bacteria, predominantly in gammaproteobacteria isolated from lakes characterized by high pH and high salinity. In the present study, a novel arsenite-oxidizing betaproteobacterium, strain M52, was isolated from a hot spring microbial mat. The strain oxidized arsenite under both microaerophilic and nitrate-reducing conditions at nearly neutral pH. Genome analysis revealed that the strain possesses the arx gene cluster in its genome and lacks genes encoding AIO. Inspection of the bacterial genomes available in the GenBank database revealed that the presence of this gene cluster is restricted to genomes of Proteobacteria, mainly in the classes Gammaproteobacteria and Betaproteobacteria. In these genomes, the structure of the gene cluster was generally well-conserved, but genes for regulatory proteins were lacking in genomes of strains belonging to a specific lineage. Phylogenetic analysis suggested that ARX encoded in the genomes can be divided into three groups, and strain M52 belongs to a group specific for organisms living in low-salt environments. The ArxA protein encoded in the genome of strain M52 was characterized by the presence of a long insertion, which was specifically observed in the same group of ARX. In clone library analyses with a newly designed primer pair, a diverse ArxA sequence with a long insertion was detected in samples of lake water and hot spring microbial mat, characterized by low salinity and a nearly neutral pH. Among the isolated bacterial strains whose arsenite oxidation has been demonstrated, strain M52 is the first betaproteobacterium that possesses the arx genes, the first strain encoding ARX of the group specific for low-salt environments, and the first organism possessing the gene encoding ArxA with a long insertion.
Introduction
Despite their toxic nature, compounds of arsenic are utilized by some prokaryotes. Microbial transformation of arsenic includes respiratory As(V) reduction and As(III) oxidation for autotrophic growth, which are referred to as “arsenotrophy” (Oremland et al., 2009). The interconversion of arsenate and arsenite in arsenotrophy is catalyzed by one of the three enzymes of the dimethyl sulfoxide (DMSO) reductase family, arsenate reductase (ARR) and two distantly related arsenite oxidases, AIO and ARX (Zargar et al., 2012).
ARX is encoded by the arxAB genes, which were initially defined in Alkalilimnicola ehrlichii MLHE-1. This bacterium was isolated from anoxic bottom water of an alkaline-saline lake and can grow chemolithoautotrophically by anaerobic arsenite oxidation coupled with nitrate reduction (Oremland et al., 2002). The arx genes have also been identified in the genomes of phototrophic bacterial strains isolated from alkaline-saline environments, such as Ectothiorhodospira sp. PHS-1 and Ectothiorhodospira sp. BSL-9. These strains are capable of arsenite oxidation coupled to anoxygenic photosynthesis, which is referred to as photoarsenotrophy. The essential role of the arxA gene in chemoautotrophic and phototrophic arsenite oxidation was demonstrated by mutagenesis experiments with A. ehrlichii MLHE-1 and Ectothiorhodospira sp. BSL-9, respectively (Zargar et al., 2010; Hoeft McCann et al., 2017). In the genome of A. ehrlichii MLHE-1, the arx genes form a gene cluster consisting of structural and regulatory components. The structural component is comprised of five genes, arxB2ABCD. The other group of genes transcribed in the opposite direction, arxXRS, are thought to encode a regulatory system (Zargar et al., 2010). The same arrangement of the arx genes was also found in Ectothiorhodospira sp. PHS-1 (Zargar et al., 2012) and BSL-9 (Hernandez-Maldonado et al., 2016, 2017). To date, only a few arsenite-oxidizing bacteria have been reported to possess the arx genes or arxA. They are predominantly gammaproteobacteria isolated from lakes characterized by high pH and high salinity, including Halomonas sp. ANA-440 (Hamamura et al., 2014), Halorhodospira halophila SL1 (Challacombe et al., 2013; Hernandez-Maldonado et al., 2017), Ectothiorhodospira sp. MLW-1 (Hoeft McCann et al., 2017), and the three strains mentioned above. As an exceptional case, Desulfotomaculum sp. TC-1 (in the phylum Firmicutes) was isolated from a sulfidic hot spring at pH 5.5 (Wu et al., 2017).
As mentioned above, most current knowledge regarding ARX came from studies on gammaproteobacterial strains from environments with high pH and high salinity. On the other hand, however, the arx genes have also been identified in the genome of other bacteria whose ability for arsenite oxidation has not been demonstrated. One such strain, Sulfuricella denitrificans skB26, is a sulfur-oxidizing betaproteobacterium isolated from an artificial freshwater lake. In its genome, the arx genes form a gene cluster corresponding to that of A. ehrlichii MLHE-1. The protein encoded by the arxA gene of S. denitrificans skB26 is phylogenetically distinct from those of the halophilic and alkaliphilic gammaproteobacteria. In addition, it has a unique inserted amino acid sequence (Watanabe et al., 2014). In some previous studies, partial sequences of the arxA gene were obtained with culture-independent approaches. In one such study, arxA gene sequences closely related to that of S. denitrificans skB26 were frequently detected in samples characterized by low salinity and a nearly neutral pH (Ospino et al., 2018). These results suggest the presence of a lineage of ARX specific for freshwater environments, but arsenite oxidation by the ARX of S. denitrificans skB26 or other members of this lineage has not been demonstrated.
In this study, an arsenite-oxidizing betaproteobacterium possessing the arx genes was isolated. This isolate has the arxA gene belonging to the lineage frequently detected in freshwater environments. In addition, the diversity of the arx gene cluster was investigated by using genome sequences available in public databases.
Materials and Methods
Isolation of an Arsenite-Oxidizing Bacterium, Strain M52
To obtain a novel arsenite-oxidizing bacterium, a dark green microbial mat developed on an inclined concrete wall was obtained from the Jozankei hot spring located in Hokkaido, Japan. The mat is identical to that used in previous studies (Kojima et al., 2017; Ospino et al., 2018). A piece of mat was inoculated in a synthetic basal medium (pH 7.0 and 341.0 mg/L of NaCl), which was prepared as described previously (Connon et al., 2008). Prior to sterilization, the medium was purged with N2 or CO2 gas to enrich anaerobic organisms. Just before inoculation, stock solutions of sodium arsenite and sodium chlorate were added to the medium to final concentrations of 0.5 and 3 mM, respectively. The headspace of the bottle was filled with N2 gas, and incubation was performed in the dark at 32°C. The enrichment was subject to successive transfers to fresh media with an increased concentration of arsenite, 1 mM. During the enrichment culture procedure, the presence of organisms with the arxA gene was monitored by the PCR-based method previously described (Ospino et al., 2018). After the fifth transfer, a pure culture was obtained by repeated agar shake dilution (Widdel and Bak, 1992). For the isolation, another medium was used with Na2S2O3 and NaNO3 as an electron donor and acceptor, as described previously (Kojima et al., 2017).
Genome Sequencing and Phylogenetic Analysis of Strain M52
Genomic DNA was extracted from strain M52 using a Wizard® Genomic DNA purification kit (Promega, Madison, WI, United States). Genome sequencing and assembly were performed as described previously (Umezawa et al., 2016). The obtained genome was annotated with Rapid Annotation using Subsystem Technology (RAST) (Overbeek et al., 2014). The annotation overview of the genome was made in the SEED Viewer version 2.0 (online). The assembled genome was subject to inspection for genes involved in arsenic metabolism. The genome of strain M52 is available in GenBank under the Accession No. NZ_AP019373.
The closest cultured relatives of the novel strain were identified by comparison to the rRNA database in BLASTn, and a phylogenetic tree was constructed. The sequences were aligned with MAFFT version 7. Gaps and poorly aligned regions were excluded using Gblocks v0.91b. The tree topology was inferred by neighbor-joining using MEGA 6.
Culturing Experiments With Arsenic
Arsenite oxidation ability of strain M52 and effects of arsenic on its growth were examined with culturing experiments at 45°C, as described below.
To verify whether strain M52 was capable of oxidizing arsenite anaerobically, culturing experiments were performed under anoxic conditions by using the basal medium used for the enrichment culture. The medium was supplemented with 0.2% (w/v) NaCl, 1–2 mg/L yeast extract, 0.5 mM sodium molybdate, 1 mM sodium arsenite and 10 mM sodium nitrate. For measurements of arsenite and arsenate concentrations, approximately 500 μL of medium was aseptically collected from each bottle at 0, 6, and 18 days of incubation. The collected samples were immediately filtered through 0.22 μm pore size filters and stored at -30°C until the measurements. The concentrations of arsenite and arsenate were determined by high-performance liquid chromatography (HPLC), as described previously (Watanabe et al., 2017).
Arsenite oxidation was also examined in the basically same medium which contained no nitrate, under anoxic, microoxic, and oxic conditions. For test of anoxic conditions, the medium was prepared as above, and headspace was filled with N2 gas. The medium for microoxic conditions were prepared as same as the above, but filter-sterilized air was added to the culture bottles after autoclaving, to obtain final oxygen concentrations of 1 or 2% (v/v) in the headspace. For oxic conditions, the medium was prepared without purging with N2 gas and head space was filled with air. Arsenite and arsenate were measured with HPLC just after inoculation and after 6 days incubation without shaking.
Effects of arsenic on growth were investigated under nitrate-reducing conditions, by monitoring turbidity along with concentrations of arsenate and nitrate. The experiments were performed with the medium same as that used for the first experiment, but concentration of yeast extract was 5 mg/L. Turbidity was measured as optical density at 660 nm, with a spectrophotometer. Arsenate and nitrate were quantified with ion chromatography equipped with conductivity detector (ICS-1500, Dionex), equipped with a column for anion analyses (IonPac AS12A, Dionex). The experiments were performed in the presence and absence of acetate, to assess heterotrophic and autotrophic growth. The experiment with acetate was started with medium containing 5 mM sodium acetate and 1 mM sodium arsenite. For comparison, bottles without arsenite were also prepared. In case of the experiments without acetate, the medium was initially supplemented with 0.65 mM sodium bicarbonate and 0.5 mM arsenite. These compounds were successively added to the medium during the incubation, by injection of dense solutions. At the latest stage of the experiments, 5 mM sodium acetate was added to the culture.
Tolerance to arsenite was tested in the basal medium, supplemented with sodium acetate (5 mM), sodium nitrate (5 mM), and 5 mg/L yeast extract. The strain M52 was cultured in presence of sodium arsenite with different concentrations (1, 2, 5, and 10 mM).
Detection of the arx Gene Cluster in Prokaryotic Genomes
To explore the arx genes in prokaryotic genomes, amino acid sequences encoded by the arxXRSB2ABCD genes of A. ehrlichii MLHE-1 were used as queries to identify homologous proteins in the non-redundant protein database of the National Center for Biotechnology Information (NCBI)1. The identifications were made using Diamond v 0.9.14 (Buchfink et al., 2014), and two proteins were considered to be homologous based on the following thresholds: an amino acid identity of ≥ 30%, a spanning alignment of ≥ 60% of the length of the query, and an e-value ≤ 1e-3. In addition to sequences in the protein database, good-quality genome sequences without functional annotation were collected for the analysis from the “Microbial Genomes resource” in NCBI2. They were selected based on the following criteria: consist of contigs ≤ 300, have an N50 ≥ 20 kb and contain ≤ 10 kb of ambiguous base pairs. In the collected genomes, protein coding regions were deduced using Prodigal v2.6.3 in normal mode (with default parameters) (Hyatt et al., 2010), and the predicted proteins were used to build a custom database for identification of homologous proteins with the method described above. The data collection from NCBI databases occurred in January 2018. Furthermore, three genome sequences obtained in our laboratory were also included in the analysis. They are genomes of three strains isolated from the same microbial mat: strain M52 obtained in this study, Sulfuritortus calidifontis J1A (Kojima et al., 2017) and Sterolibacteriaceae bacterium strain J5B (Watanabe et al., 2019). They were analyzed with the same methods used for the unannotated genome sequences as described above.
Phylogenetic Analyses
The identified proteins, homologous to ArxA of A. ehrlichii MLHE-1, were subjected to phylogenetic analysis, including the putative subunits of other enzymes from the DMSO reductase family. The amino acid sequences were aligned with MUSCLE (Edgar, 2004). Gaps were allowed within an appropriate block by Gblocks v0.91b (Talavera and Castresana, 2007). The tree was inferred with the distance criterion by the BioNJ algorithm using FastME 2.0 (Lefort et al., 2015). The inference included 1000 bootstraps replicates (Gascuel, 1997). Phylogenetic trees of proteins encoded by the arxA, arxB, and arxD genes were constructed as below. The amino acid sequences were separately aligned and curated as described above. The trees were reconstructed by the maximum-likelihood algorithm with the LG + G + I substitution model using PhyML (v3.0) (Guindon et al., 2010). The gamma shape parameter was estimated directly from the data. The aLRT test (SH-Like) was used to calculate the support values (Anisimova and Gascuel, 2006). To construct the ArxAB consensus tree, the alignments of ArxA and ArxB were concatenated using MEGA version 6 (Tamura et al., 2013) and then subjected to the remaining steps as explained above. All of the phylogenetic analyses were performed on the Phylogeny.fr platform (Dereeper et al., 2008).
Phylogenetic analysis of organisms possessing the arx genes was conducted on the basis of ribosomal proteins (RPs). The following 10 RPs were used for the analysis: L2, L3, L4, L13, L23, S2, S4, S9, S10, and S11 (see Supplementary Table S1 for detailed information). One of the identified genomes lacked some of these proteins, and thus it was excluded from the analysis. Each ribosomal protein was independently aligned using MAFFT (Katoh et al., 2017) with default parameters. The poorly aligned regions were removed using the Gblocks v0.91b program, allowing gaps located in less than 50% of the sequences. The resulting 10 alignments were concatenated using MEGA version 6. The tree was inferred by neighbor-joining under the p-distance model in MEGA 6. Support values were calculated using 1000 bootstrap replicates. The final trees were visualized using the R package ggtree (Yu et al., 2017).
Identification of Conserved Motifs and Prediction of Secondary Structure
In the proteins encoded by arxAB genes, conserved motif regions were identified by Pfam online (Finn et al., 2014) and visualized in the multiple sequences alignment on MEGA 6. The presence and location of twin-arginine signal peptide cleavage sites were identified using the TatP 1.0 server online (Bendtsen et al., 2005). Secondary structure prediction was performed for the ArxA proteins with insertion, using the Jpred 4 server (Drozdetskiy et al., 2015).
Clone Library Construction and Analysis of a Long Insertion in the arxA Gene
To explore the diversity of the ArxA proteins which have insertions, a set of degenerate primers was newly designed. The primers were designed based on the ArxA amino acid sequences characterized by the presence of a long insertion, encoded in the genomes of S. denitrificans skB26, Sulfuritortus calidifontis J1A, Gammaproteobacteria RIFOXYD12 FULL_61_37, Gammaproteobacteria RIFOXYA12 FULL_61_12, and Betaproteobacteria CG2_30_59_46. The primers were designed at positions flanking the insertion to obtain the full length of the insertion (Figure 8A). The primers are arxA_G2_F (AARCGTACCAAYCCSAAVAAGG) and arxA_G2_R (GTTCTTGGCGTAGTCRTCCAT). PCR was performed in a 25 μL volume reaction mixture containing 0.5 μmol/L of each primer, 1× Ex Taq Buffer (Takara, Shiga, Japan), 0.2 mmol/L dNTPs (Takara), 0.625 U of Ex Taq (Takara), 3% DMSO and template DNA solution. The PCR conditions were as follows: 94°C for 3 min; 34 cycles of denaturing at 94°C for 45 s, annealing at 55°C for 45 s, extension at 72°C for 1.5 min; and then a final extension step at 72°C for 7 min. Two DNA samples obtained in previous studies were used as templates for PCR amplification with newly designed primers: one water sample was obtained at a depth of 40 m in Lake Mizugaki (Watanabe et al., 2017), and a dark green microbial mat sample was retrieved from Jozankei hot spring (Ospino et al., 2018). The resulting PCR products were analyzed with agarose gel electrophoresis, and bands corresponding to expected sizes (ca. 1200 bp and ca. 900 bp, with and without insertion) were excised from the gel. From the pieces of gel, DNA was extracted with QIAquick Gel Extraction Spin Kit (Qiagen). The purified amplicons were cloned into pCR2.1-TOPO vector and transformed into TOP10 cells (Invitrogen), according to the manufacturer’s instructions. The resulting clone libraries were analyzed as described previously (Ospino et al., 2018), and operational taxonomic units (OTUs) were assigned at a cutoff value of 0.02 in Mothur (Schloss et al., 2009). The most abundant sequence of each OTU was selected as representative for phylogenetic analysis. The representative sequences were aligned with ArxA reference sequences using MUSCLE. The alignment was trimmed manually, excluding gaps. Phylogenetic relationships were inferred based on distance criteria using the BioNJ algorithm to construct a Neighbor-joining tree with 1000 bootstrap replicates in FastME 2.0. The ArrA of Alkaliphilus oremlandii OhILAs and Halarsenatibacter silvermanii were used as the outgroup. The nucleotide sequences obtained in this study have been deposited under the accession numbers LC439110 to LC439195.
Phylogenetic Analysis of Partial Sequences of the arxA Gene Reported in Previous Studies
In some previous studies, partial sequences of the arxA gene were obtained with the primer pair arxA_Deg_F_B/arxA_Deg_R_B (Zargar et al., 2012; Hamamura et al., 2014; Wu et al., 2017) (Table 1). The phylogenetic positions of these sequences were reexamined with reference ArxA sequences collected in this study. In the phylogenetic analysis, partial sequences obtained in this study were also included. The alignment was made using MUSCLE and was trimmed manually to exclude the gaps. A neighbor-joining tree was reconstructed using the BioNJ algorithm with 1000 bootstrap replicates in FastME 2.0. The arsenate reductase ArrA sequences were used as the outgroup.
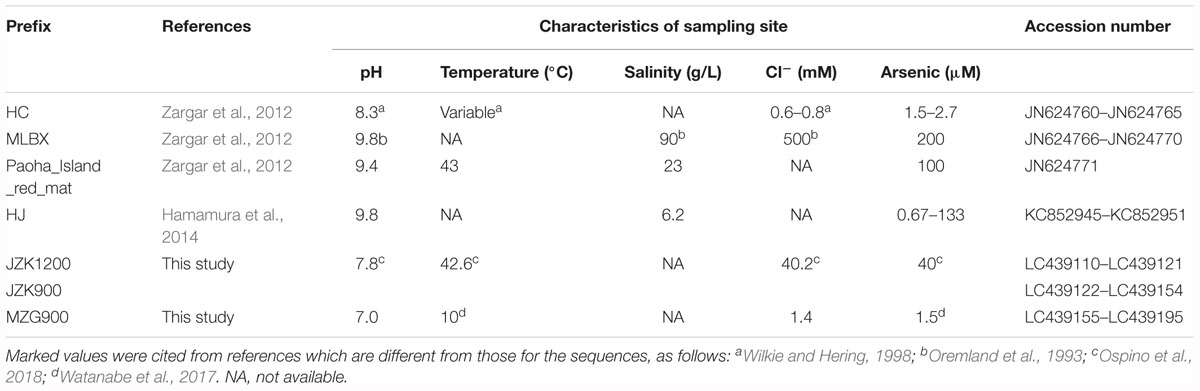
Table 1. Partial arxA gene sequences of uncultured organisms included in phylogenetic analysis in this study (Supplementary Figure S8).
Results
Isolation and Characterization of Arsenite-Oxidizing Bacterium Strain M52
From the microbial mat, arsenite-oxidizing enrichment cultures were established. The presence of an arxA-carrying organism in culture was confirmed with PCR amplification and sequencing with a previously reported primer pair. By analyzing the 16 rRNA gene, it was revealed that the cultures were dominated by a bacterium related to Sterolibacteriaceae bacterium strain J5B (Watanabe et al., 2019). The dominating organism was isolated by using the same methods that were used for the isolation of strain J5B, and the resulting isolate was designated strain M52. During the isolation procedures, strain M52 showed anaerobic and chemolithoautotrophic growth depending on nitrate and thiosulfate. The strain J5B is facultatively autotrophic bacterium which can grow on some organic acids (Watanabe et al., 2019). Among the organic substrates which support growth of strain J5B, acetate and lactate were tested with strain M52. Both these substrates supported anaerobic growth of strain M52 under nitrate reducing conditions.
The complete genome of strain M52 consisted of a single circular chromosome with a length of 2.74 Mb and a G+C content of 63.6%. In the genome, 2794 coding sequences were predicted. There are two copies of the rrn operons which contain the 16S rRNA genes with slightly different lengths. BLASTn searches revealed that the most closely related species with validly published names were Sterolibacterium denitrificans and Georgfuchsia toluolica, with sequence identities of 94–95%. By constructing a phylogenetic tree, it was confirmed that strain M52 is a novel member of the family Sterolibacteriaceae (Figure 1).
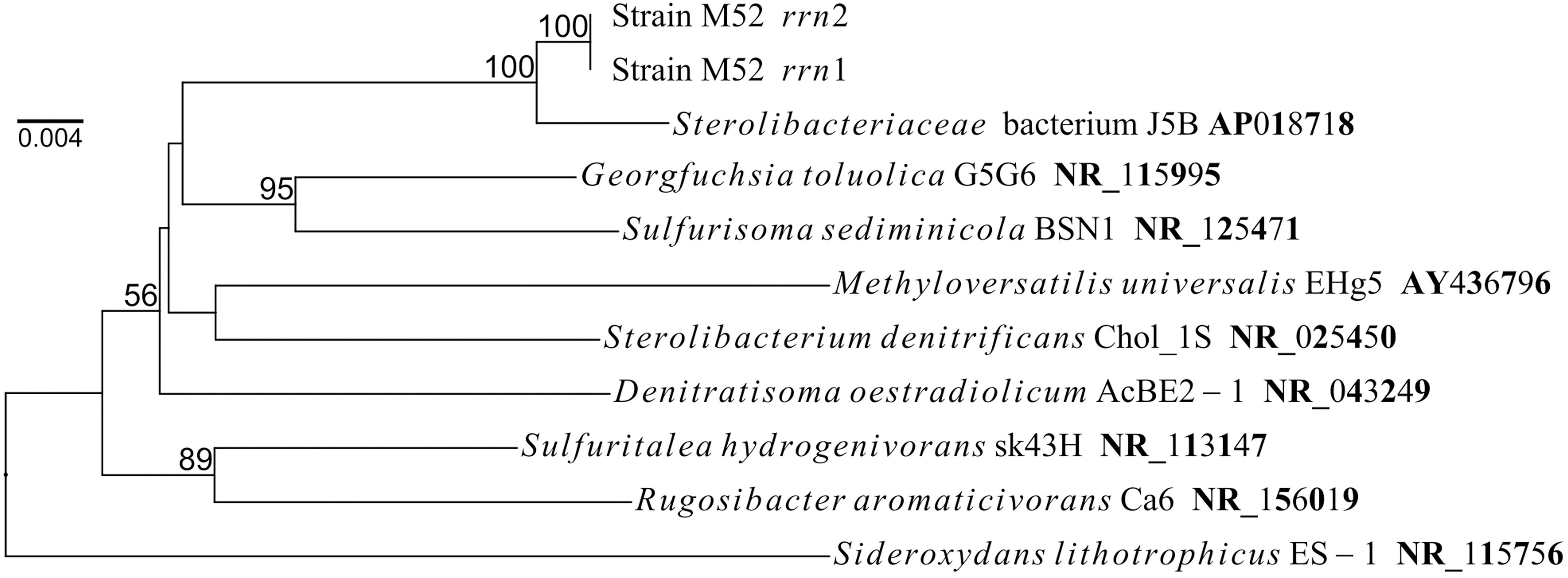
Figure 1. Phylogenetic position of two copies of the 16S rRNA gene in the genome of strain M52. Numbers on nodes represent percentage values (greater than 50%) of 1000 bootstrap resampling.
In the genome of strain M52, the arxA gene was identified, and its nucleotide sequence perfectly matched the partial sequence detected in the enrichment cultures. The arxA gene of strain M52 encoded a protein that has 92 extra amino acids compared to that of strain MLHE-1, but the protein is similar to its homolog in S. denitrificans skB26. In addition to arxA, the other arx genes were also identified nearby arxA. Some differences in arrangement of these genes were observed, as described later in more detail.
Regarding other genes related to arsenic metabolism, strain M52 had genes for energy-dependent arsenic detoxification: arsC (detoxifying arsenate reductase), arsR (transcriptional repressor) and acr3 (arsenite–specific efflux pump). On the other hand, the arxM gene, which is involved in arsenic methylation, was absent in the genome. The aio genes, which encode another type of arsenite oxidase, and arrAB, which encodes respiratory arsenate reductase, were not identified in the genome of strain M52.
Further inspection of the genome revealed that strain M52 has genes required for respiratory nitrate reduction. They include the genes encoding catalytic subunits of periplasmic nitrate reductase (napA), nitrite reductase (nirS), nitric-oxide reductase (norB) and nitrous oxide reductase (nosZ). The genome also harbors genes required for respiration with oxygen, the ccoNOQP genes encoding cytochrome c bb3-oxidase and the cydAB genes encoding cytochrome bd oxidase. Although the strain was isolated from enrichment culture with chlorate, genes for chlorate reduction were not identified in the genome.
Arsenic Metabolism of Strain M52
Under anoxic conditions, strain M52 oxidized arsenite to arsenate in the medium containing nitrate as sole electron acceptor (Figure 2A). In the uninoculated control, changes in concentrations of arsenate or arsenite were not observed. Arsenite oxidation by strain M52 was also tested in the medium without nitrate. In the absence of nitrate, arsenite oxidation was not observed under anoxic conditions (Figure 2B). In the same medium, strain M52 oxidized arsenite when incubation was performed under microoxic conditions with 1 or 2% oxygen in the headspace (Figure 2B). The oxygen-dependent arsenite oxidation was not observed under atmospheric oxygen level (20%).
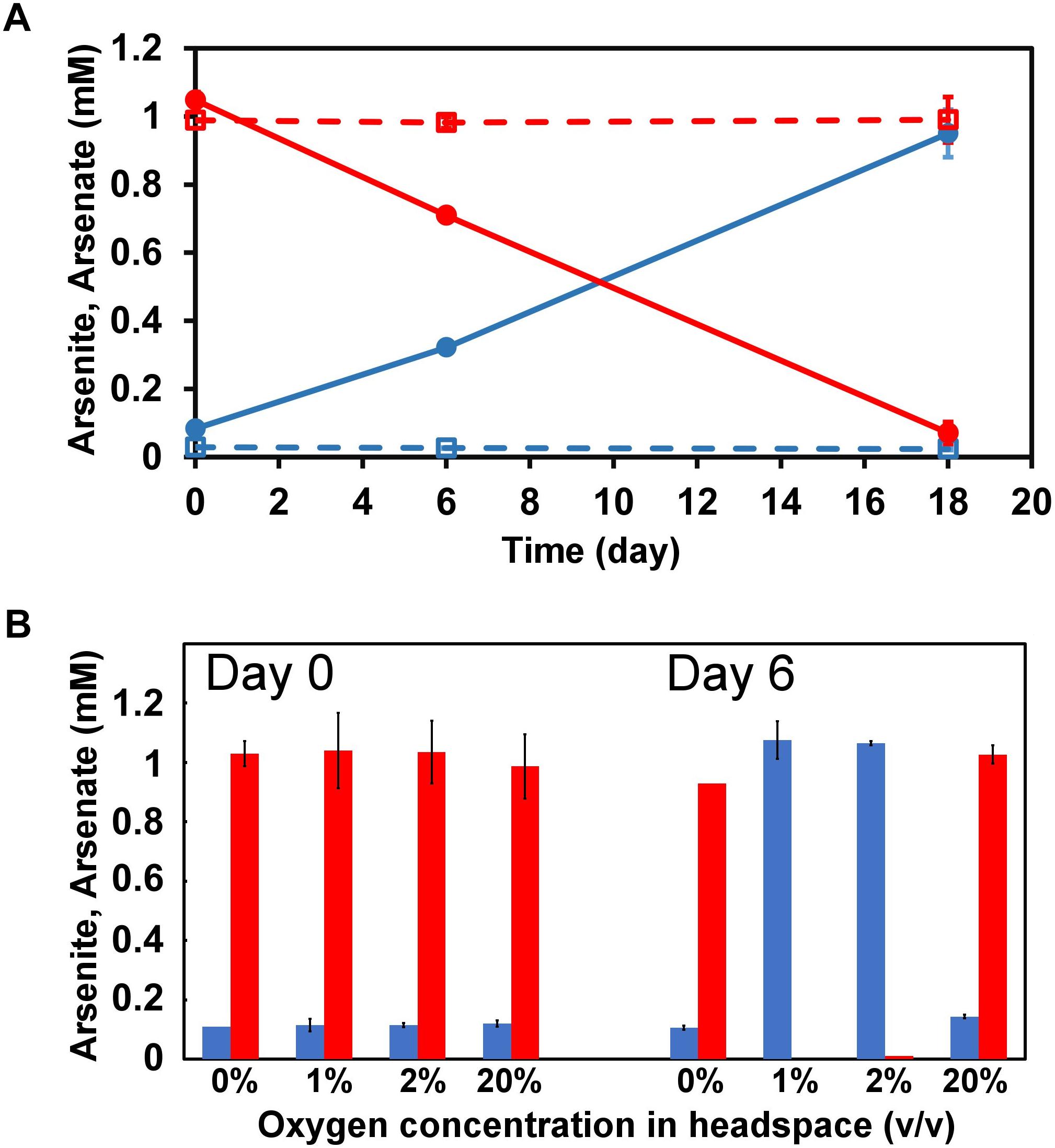
Figure 2. Arsenite oxidation to arsenate by strain M52. (A) Changes in concentrations of arsenite (red) and arsenate (blue) in medium containing nitrate, incubated under anoxic conditions. Error bars represent standard deviation from two independent assays. Dashed lines represent uninoculated control. (B) Effect of oxygen concentration in headspace on arsenite oxidation in medium without nitrate. Red and blues bars represent concentrations of arsenite and arsenate, respectively. Error bars represent standard deviation from triplicates (20%) or duplicates (the others).
To assess effects of arsenic on anaerobic growth of strain M52, cell density was monitored along with changes in concentrations of nitrate and arsenate. As shown in Figure 3A, 1 mM of arsenite showed negative effect on heterotrophic growth on acetate. In the presence of acetate, arsenate was generated in the early stage of growth, but it seemed to be reduced back into arsenite in the later stage of incubation possibly by the ars system. The effects on autotrophic growth was tested with gradual supplement of arsenite to abate toxicity (Figure 3B). With a serial addition of arsenite, continuous production of arsenate was observed throughout the incubation. A small increase in turbidity was observed until day 5, but no obvious growth was observed during the period from day 5 to day 19. In this period, supplement of inorganic carbon source did not enhanced growth. After the measurements at day 19, acetate was added to the culture. After that, cell density increased within the following 5 days, suggesting that viability was retained during the experiment (Figure 3B). In these experiments, nitrate was monitored with ion chromatography which can detect nitrite as well. In all samples analyzed, however, nitrite was not detected.
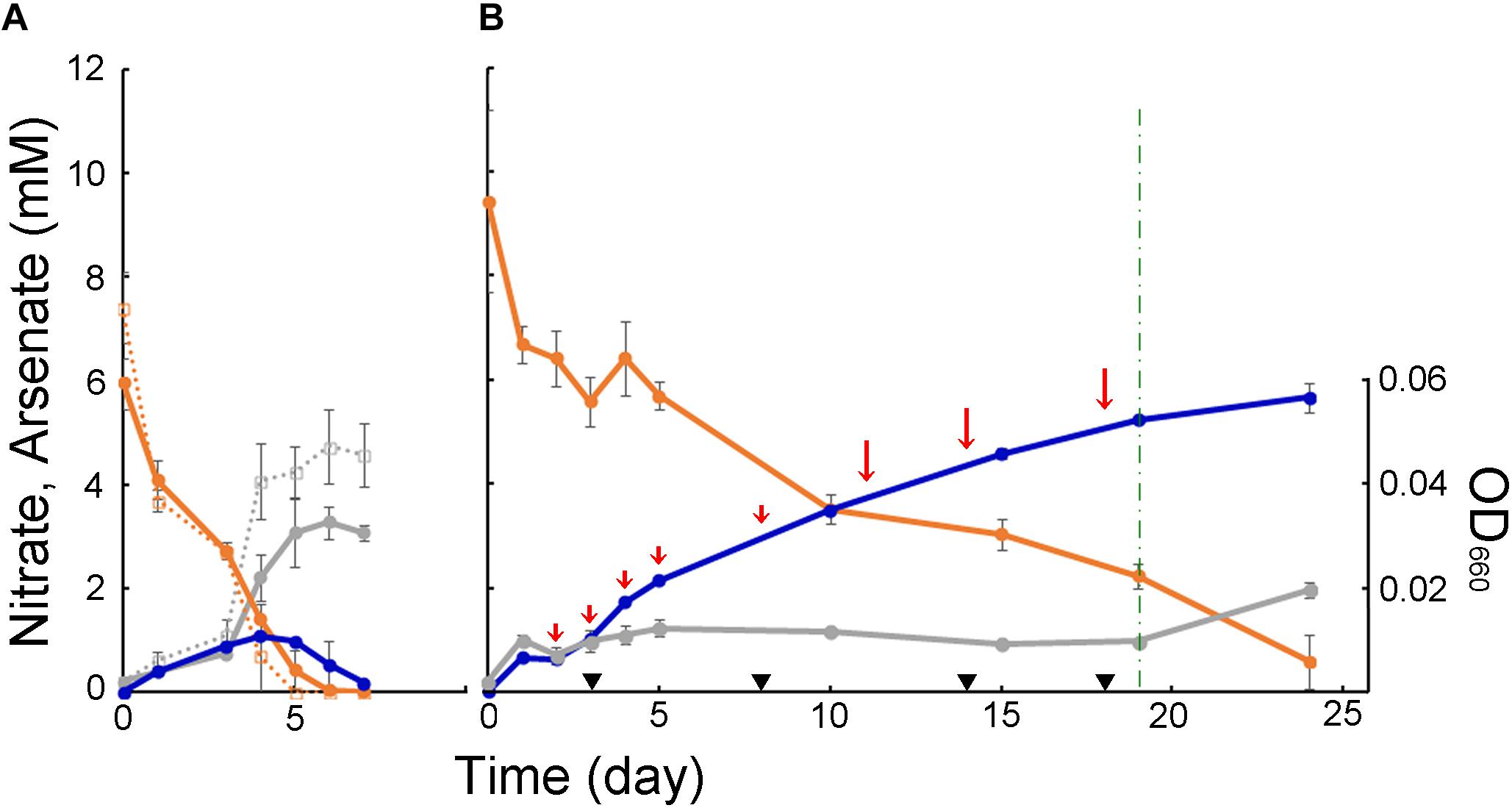
Figure 3. Anaerobic growth of strain M52 assessed as optical density at 660 nm (gray), and changes in concentrations of nitrate (orange) and arsenate (blue). Bars represent standard deviation from triplicates. (A) Experiments in medium containing acetate. Dashed lines represent culture without arsenite. (B) Experiments in medium without acetate. Short and long red arrows indicate time points of addition of arsenite, 0.5 and 1 mM, respectively. Black triangles and dashed green line indicate time points of addition of bicarbonate (0.65 mM) and acetate (5 mM), respectively.
Arsenite tolerance of strain M52 was tested in presence of nitrate and acetate under anoxic conditions. Anaerobic growth on acetate was observed in the presence of 5 mM or lower concentration of arsenite, and higher concentrations resulted in slower growth of the strain. No growth was observed in the presence of 10 mM arsenite.
Distribution, Composition, and Arrangement of the arx Gene Cluster
By using the sequences of A. ehrlichii MLHE-1 as queries, a total of 46 genomes were identified to contain full-length genes homologous to the arxAB genes (see Supplementary Table S2 for more detail). Among them, five genomes were those of archaea, and the others were bacterial genomes. Detailed descriptions of the genomes are presented in Supplementary Table S3. All 42 bacterial genomes (41 identified and A. ehrlichii) were from organisms belonging to the phylum Proteobacteria (Figure 4). Among them, 25 are members of the class Gammaproteobacteria, including 15 strains in the family Ectothiorhodospiraceae (in the order Chromatiale). This group included four strains whose ability to oxidize arsenite was demonstrated: A. ehrlichii MLHE-1, Halorhodospira halophila SL1, Ectothiorhodospira sp. PSH-1, and Ectothiorhodospira sp. BSL9. The other 11 strains of this family were members of the genus Thioalkalivibrio. As another major group in the class Gammaproteobacteria, 7 strains were identified in the order Oceanospirillales. This group included the genera Halomonas, Marinospirillum, and Nitrincola (Figure 4). The majority of these gammaproteobacterial strains were isolated from high saline and/or high-pH environments, but two gammaproteobacterial genomes identified were reconstructed from metagenomic data of a groundwater sample (Supplementary Table S3). In the class Betaproteobacteria, six cultured strains possessed the genes, along with seven metagenome-assembled genomes (MAGs). The remaining bacterial genomes were affiliated with the classes Alphaproteobacteria, Deltaproteobacteria, and Candidatus Muproteobacteria (Figure 4). On the other hand, all archaeal genomes identified were those of Candidatus Methanoperedens in the order Methanosarcinales. They were MAGs from anaerobic bioreactors performing methane oxidation coupled to nitrate reduction (Supplementary Table S3).
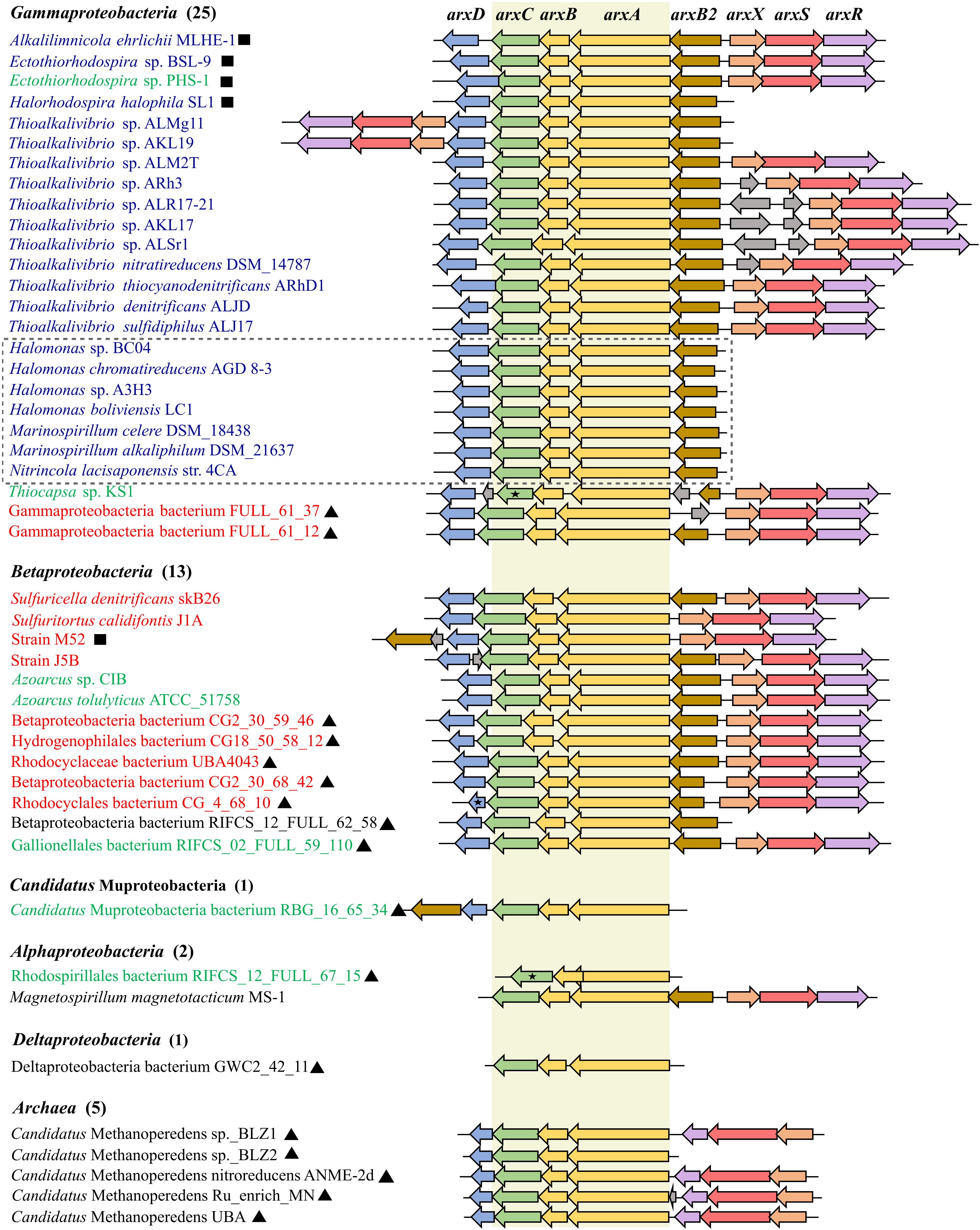
Figure 4. Arrangement of the arx gene cluster found in the genomes. Arrows with different colors represent the following genes: yellow for arxAB, green for arxC, blue for arxD, gold for arxB2, peach for arxX, coral for arxS, purple for arxR, and gray for hypothetical proteins. The number of genomes for each class-level taxonomy of bacteria is shown in parentheses. Name of organisms with ARX of group 1, group 2, and group 3 are shown in blue, green and red respectively. Names with black square correspond to organism reported as arx-arsenite-oxidizing bacterium, including strain M52. Names with black triangle represent the metagenome-assembled genomes (MAGs). Black star represents the genes encoding products annotated as partial protein. Names of organisms affiliated to the Order Oceanospirillum are enclosed in a gray dashed square. The conserved arxABC genes are highlighted in beige color.
In all the genomes identified, the arxABC-like genes were conserved in the same order and direction. As well, arrangement of genes around them showed significant similarities among the genomes (Figure 4). Among the genomes, 17 genomes had gene organization identical to that of the arx genes in A. ehrlichii MLHE-1 (Figure 4). For the proteins encoded by the gene homologous to arxA, a phylogenetic tree was constructed to identify their phylogenetic positions within the DMSO reductase family (Figure 5 and Supplementary Figure S1). In the tree, the proteins encoded in the identified genomes formed two distinct clades, corresponding to those of bacteria and archaea. The former encompasses ArxA of A. ehrlichii MLHE-1 and Ectothiorhodospira sp. PHS-1, which were included in the original definition of ARX. On the other hand, there is no evidence regarding the function of the proteins forming the latter cluster, and arsenic metabolism by Candidatus Methanoperedens has not been reported. Based on these results, the archaeal genomes were excluded from the following analyses. Hereafter, the bacterial genes homologous to the arx genes of A. ehrlichii MLHE-1 are simply referred to as arx genes.
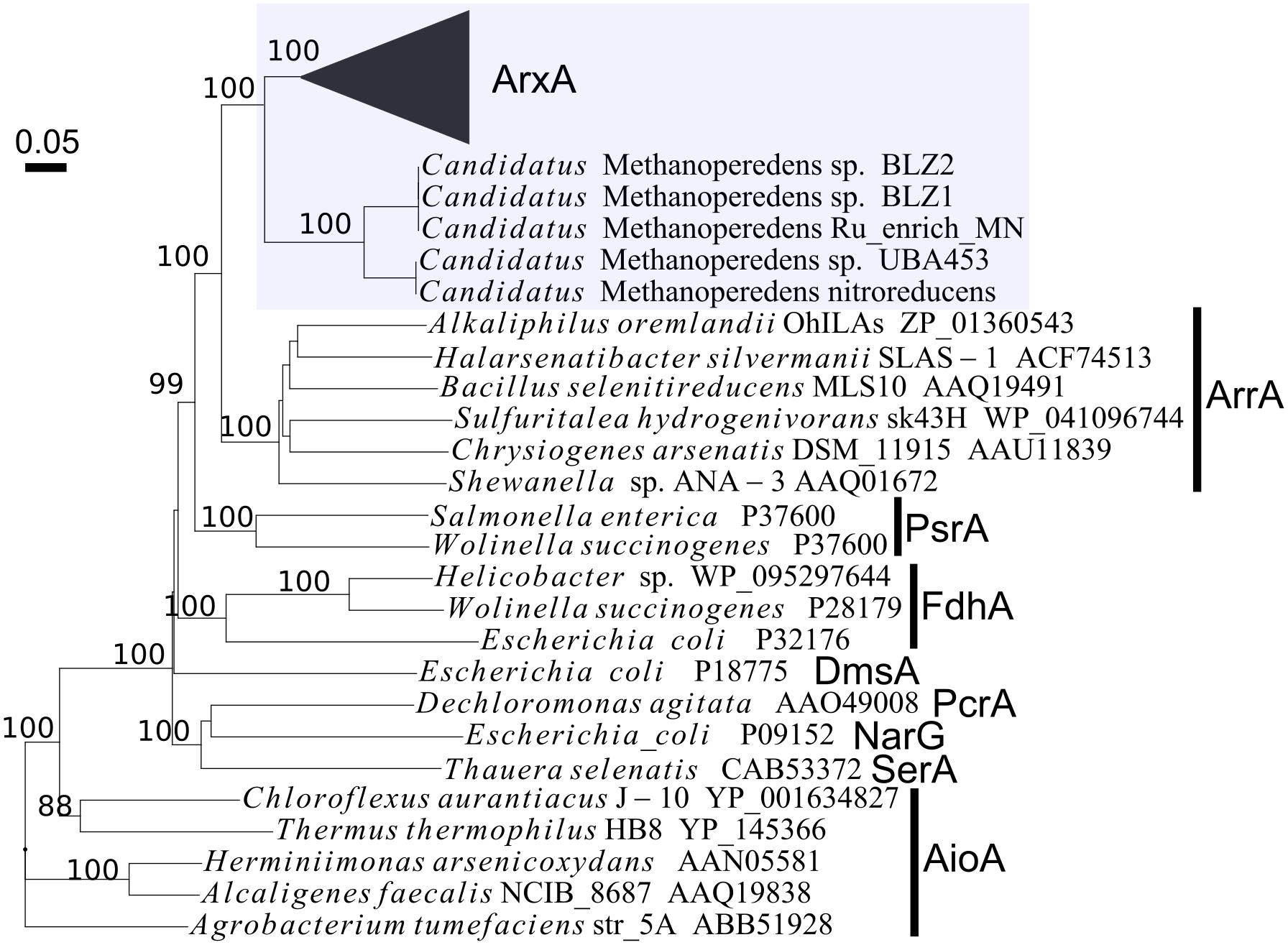
Figure 5. Phylogenetic positions of proteins homologous to ArxA of Alkalilimnicola ehrlichi MLHE-1, within the DMSO reductase family proteins. The ArxA of A. ehrlichi MLHE-1 and its homologs identified by BLAST searches are highlighted with a shaded box. An uncollapsed version of this tree is presented in Supplementary Figure S1. PsrA, polysulfide reductase; FdhA, formate dehydrogenase; DmsA, dimethyl sulfoxide reductase; SerA, selenate reductase; NarG, respiratory nitrate reductase; PcrA, perchlorate reductase.
The novel strain isolated in this study, strain M52, has arx gene cluster similar to that of A. ehrlichii MLHE-1, but the arxB2 gene is located downstream of arxABCD, similar to a MAG of Candidatus Muproteobacteria (Figure 4). The arxB2 gene was not identified in four bacterial genomes. The arxD gene was conserved in all the genomes except for three genomes from Alphaproteobacteria and Deltaproteobacteria.
Genes encoding regulatory proteins ArxXSR were commonly found upstream of the arxB2ABCD genes on the opposite strand. In the genomes of Thioalkalivibrio sp. ALMg11 and Thioalkalivibrio sp. AKL19, the arxXSR genes were identified downstream of arxB2ABCD on the same strand as exceptional cases (Figure 4). Halorhodospira halophila SL1 can oxidize arsenite, but the arxXSR genes were not identified in its genome. The arxXSR genes were consistently absent in the genomes of three genera in the order Oceanospirillales (Halomonas and Marinospirillum and Nintricola lacisaponensis) (Figure 4). The other genomes lacking arxXSR genes were distributed in Candidatus Muproteobacteria, Alphaproteobacteria, and Deltaproteobacteria.
Phylogeny of Proteins Encoded by the Arx Proteins
Phylogenetic relationships among the identified Arx proteins were further investigated in detail. At first, phylogenetic trees of ArxA and ArxB were separately constructed. The resulting trees showed phylogenetic congruence with minor exceptions, indicating the coevolution of these two subunits (Supplementary Figure S2). For a more reliable phylogenetic inference, an ArxAB consensus tree was constructed by concatenating the alignments of ArxA and ArxB (Figure 6). In the tree, the majority of ArxAB proteins were grouped into three groups corresponding to well-supported monophyletic clusters. In this study, these groups are referred to as group 1, group 2, and group 3, respectively (Figure 6). Correspondence between the grouping of ARX and the class-level taxonomy of the bacteria can be seen in Figures 4, 7. Direct comparisons between the phylogeny of bacteria (based on RPs) and ARX are shown in Supplementary Figure S3.
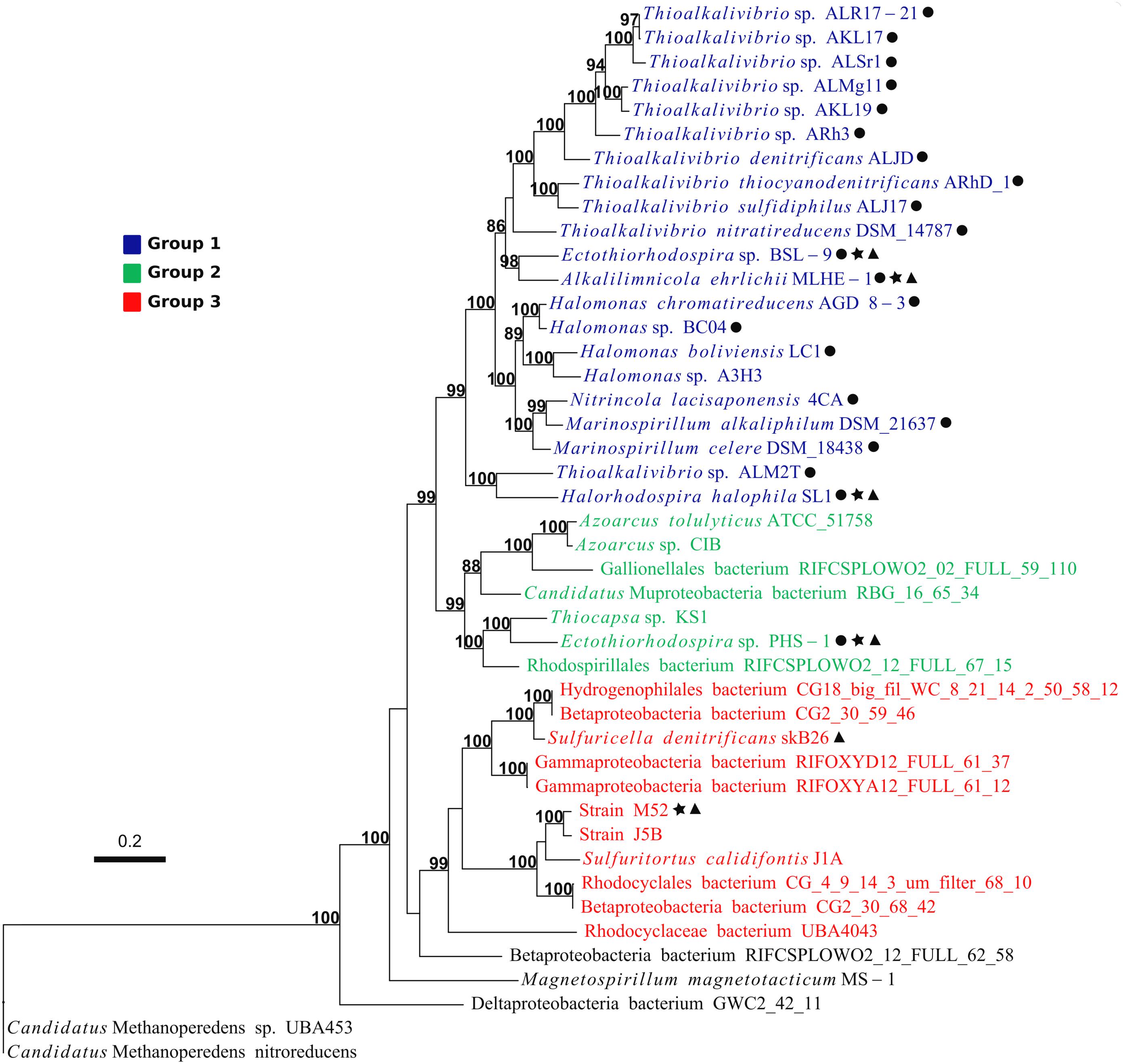
Figure 6. Phylogeny and grouping of ARX based on ArxAB sequences. Support values above 70 are shown. Names with: black circle, represent organisms isolated from high pH/high salinity environments; black star, represent arsenite-oxidizing bacteria; black triangle, correspond to organisms reported to hold an arx operon in the genome. Name of organisms with ARX of group 1, group 2, and group 3 are shown in blue, green and red respectively.
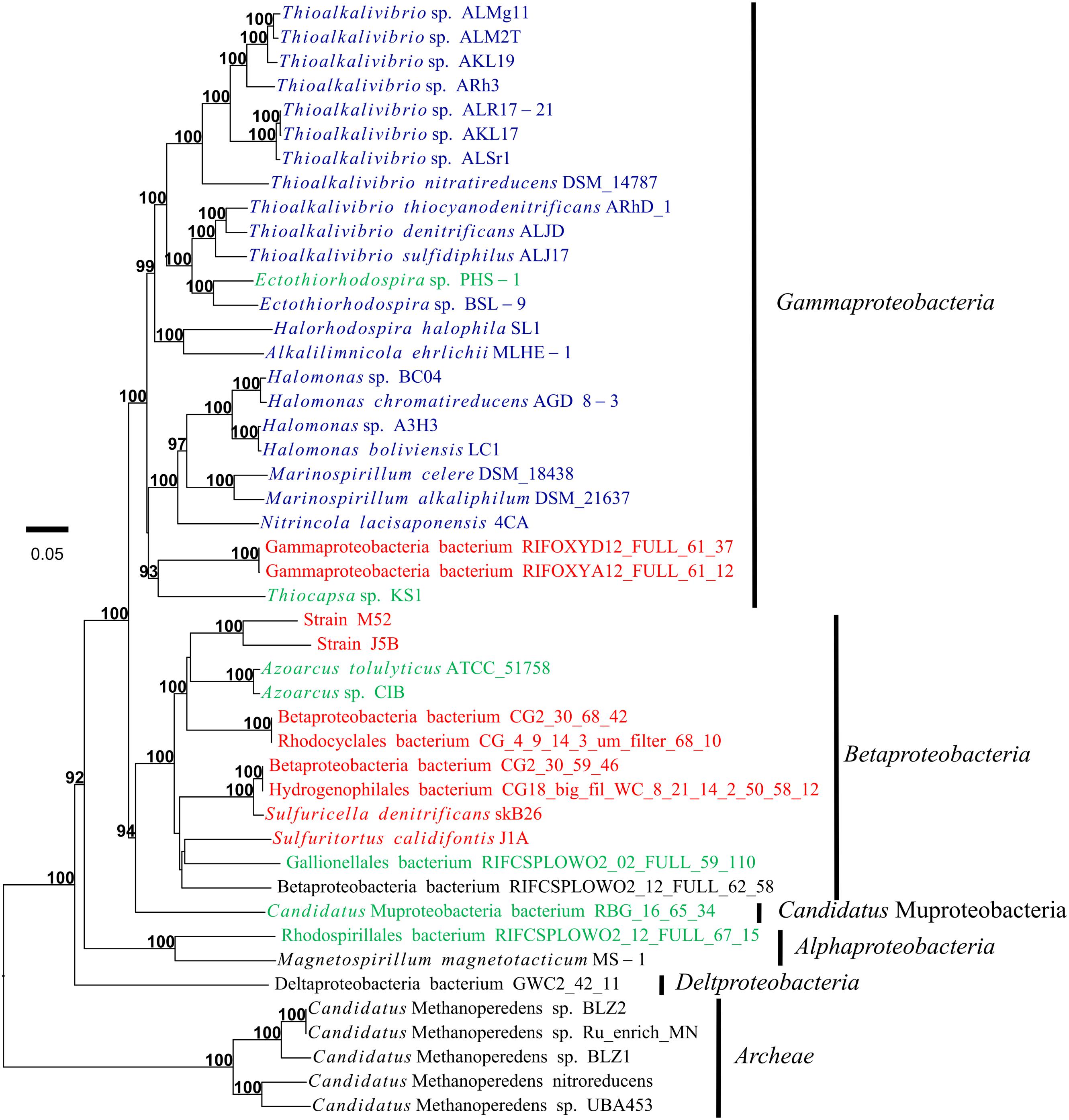
Figure 7. Phylogeny of the organisms possessing the arx genes, based on the concatenated amino acid sequences of 10 ribosomal proteins. Support values greater than 70% are indicated in bold. Rhodocyclaceae bacterium UBA4043 is not included due to absence of the majority of genes encoding ribosomal proteins. Name of organisms with ARX of group 1, group 2, and group 3 are shown in blue, green and red respectively.
The ARX of A. ehrlichii MLHE-1 was classified into the group 1. The ARX of this group was identified only in genomes of gammaproteobacterial haloalkaliphiles (Figures 3, 5). Ectothiorhodospira sp. PHS-1 is closely related to organisms with the ARX of group 1, but its ARX is phylogenetically distinct from those of the group 1. It was classified into group 2, together with the ARX of bacteria belonging to the classes Gammaproteobacteria, Betaproteobacteria, Alphaproteobacteria, and Ca. Muproteobacteria. The ARX of strain M52 belonged to group 3. This group consists of ARX encoded in bacteria isolated at a relatively low pH and low salt concentrations and MAGs from such environments.
A phylogenetic analysis of ArxD was also constructed (Supplementary Figure S4). In the tree, ArxD proteins associated with the ARX of group 1 and group 3 formed exclusive clusters. Phylogeny discordance with ArxD proteins was apparent in the ARX of group 2, but it was also observed in that of group 1. For instance, the positions of Thioalkalivibrio nitratireducens DSM 14787, Thioalkalivibrio denitrificans ALJD, and Thioalkalivibrio sp. ALM2T are considerably different in the phylogenetic trees of ArxD and ArxAB. In contrast, the phylogeny of ArxD proteins associated with ARX of group 3 was consistent with that of ArxAB, as indicated by the same branching pattern in the two trees (Supplementary Figure S4).
Conserved Regions in the ArxAB Amino Acid Sequences
Comparison among amino acid sequences of the ArxAB encoded in the identified genomes indicated that they share some characteristics with those of A. ehrlichii MLHE-1 and Ectothiorhodospira sp. PHS-1 reported in previous studies (Zargar et al., 2010, 2012; Van Lis et al., 2013; Badilla et al., 2018), as described below. The ArxA sequences had three conserved regions commonly identified in subunit A of the DMSO reductase family enzymes: an iron–sulfur [4Fe-4S] motif; a catalytic binding pocket sequence similar to that suggested in the ArxA of A. ehrlichii MLHE-1 and Ectothiorhodospira sp. PHS-1 (Zargar et al., 2012); and a twin-arginine signal peptide on the N-terminus (Supplementary Figure S5). Additionally, all the sequences had an XGRGWG motif located near the putative catalytic binding pocket. This motif is argued to be one of the conserved motifs that distinguish ArxA from the closely related arsenate reductase ArrA, characterized by a corresponding motif of (R/K)GRY (Glasser et al., 2018). The ArxB also share similarities to subunit B of the enzymes from the DMSO reductase family with at least three conserved iron–sulfur [4Fe-4S] clusters (Supplementary Figure S6).
Diversity of arxA With Insertion
As reported previously, ArxA of S. denitrificans skB26 has a long insertion (Watanabe et al., 2014). Similar insertions were identified in five other genomes, including strain M52 isolated in this study. To evaluate the diversity of the insertion in ArxA, a new primer pair was designed (Figure 8A). The primers were tested with purified genomic DNA from S. denitrificans skB26, Sulfuritortus calidifontis J1A, A. ehrlichii MLHE-1, and Nitrincola lacisaponensis 4CA. Among them, strains skB26 and J1A have the arxA gene with a long insertion. As a bacterium possessing the arrA gene, Sulfuritalea hydrogenivorans sk43H was used to confirm the specificity of the primer pair. The primer pair, named arxA_G2_F/arxA_G2_R, generated PCR products of approximately 1200 bp in size from strains skB26 and J1A (Figure 8B, lanes 3 and 5). A product of approximately 900 bp was obtained from strain MLHE-1 (Figure 8B, lane 8) but not from strain 4CA (Figure 8B, lane 4). No PCR products were obtained from the genomic DNA of strain sk43H (Figure 8B, lane 9). Although strains M52 and J5B have the insertions, their arxA gene sequences were not used for the primer design, because genomes of these strains were not available when the primers were designed. At a later time, the primer pair was tested with these strains but generated no PCR products.
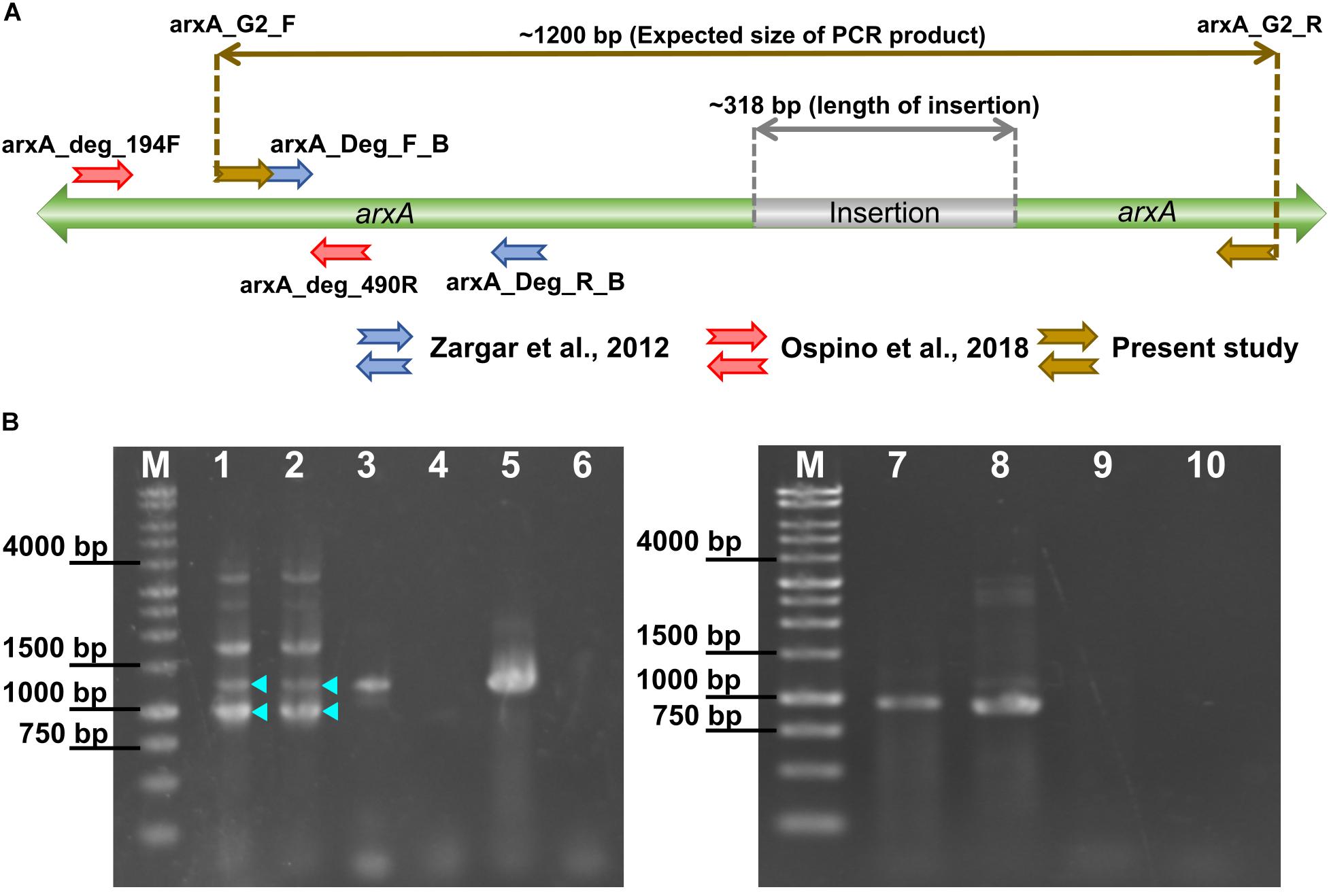
Figure 8. (A) Positions of PCR primers for the arxA gene and expected size of the PCR product. (B) Gel images showing the results of PCR with newly designed primers. Samples in lanes are: M, 10 kb DNA ladder as a size marker; (1–2) Jozankei microbial mat; (3) Sulfuritortus calidifontis J1A; (4) Nitrincola lacisaponensis 4CA; (5) Sulfuricella denitrificans skB26; (6) no template control; (7) Mizugaki water; (8) Alkalilimnicola ehrlichii MLHE-1; (9) Sulfuritalea hydrogenivorans sk43H; (10) no template control. In lanes 1 and 2, bands of expected sizes are marked with triangles.
Two environmental samples were analyzed with the new primer pair. They were water collected at a depth of 40 m in Lake Mizugaki (MZG) and a microbial mat obtained in Jozankei hot springs (JZK). A PCR product of the expected size with the insertion (1200 bp) was obtained only from the sample from JZK (Figure 8B, lanes 1 and 2). PCR amplicons of approximately 900 bp length were obtained in both JZK and MZG samples (Figure 8B, JZK: lanes 1 and 2; MZG: lane 7). With these PCR products, three clone libraries, named JZK1200, JZK900 and MZG900, were constructed (Table 1 and Supplementary Table S4). The phylogenetic tree in Supplementary Figure S7 shows the relationships between the partial ArxA sequences from the three libraries and the reference sequences. The neighbor-joining tree was reconstructed excluding gaps, using a final dataset of 310 compared amino acid positions. The tree indicated that five OTUs without insertions are clustered with ARX of group 2. The other OTUs were grouped with ARX of group 3. In the clone library of JZK1200, five OTUs with a long insertion were detected. Four of them are closely related to the ArxA of strains isolated from the same microbial mat (Sulfuritortus calidifontis J1A, strain M52 and strain J5B). The other OTU detected in this library was phylogenetically distinct from them and harbored the insertion with a clearly different sequence. In some OTUs detected in the library of 900 bp, insertions of another type were identified (Supplementary Figure S7). The insertions of this type, consisting of 12 amino acid residues, were also identified in ArxA of group 3 encoded in some MAGs.
The analysis of full ArxA sequences indicated that the insertions are distantly located from the putative functional conserved regions. No sequence motif was identified within the long insertion, but some amino acids were abundant and a few of them were conserved, such as lysine, alanine, glutamate, and leucine (Supplementary Figure S7). The analysis with Jpred4 predicted that the insertion sequences form alpha helices, with a high probability to form a coiled-coil structure. Coiled-coil is a common structural motif in proteins, formed by two or more strands of alpha-helices winded around each other in superhelical fashion (Lupas, 1997; Truebestein and Leonard, 2016). The above mentioned amino acids enriched in the insertions are known to be involved in formation of the coiled-coil frequently, because of their propensity to form alpha-helices (Monera et al., 2002; Kwok and Hodges, 2004; Surkont and Pereira-Leal, 2015). All the insertion sequences were cut out and individually subjected to BLAST analysis, but no homologous sequence was identified outside the ArxA.
Phylogenetic Reassessment of Partial arxA Gene Sequences Detected in Previous Studies
An additional phylogenetic analysis was performed to reassess the phylogenetic positions of previously reported partial sequences of the arxA gene. A neighbor-joining tree was reconstructed using a final dataset of 92 compared amino acid positions. The tree showed that all of the clones recovered from high-saline and high-pH samples are closely related to ArxA encoded in the genomes of haloalkaliphilic gammaproteobacteria (Supplementary Figure S8). The ArxA of Desulfotomaculum sp. TC-1 isolated from a hot spring was also related to these bacteria. In contrast, all the clones recovered from the Hot Creek riverbed sediment (HC) fell within a strongly supported cluster together with ARX of group 3 encoded in the genomes of bacteria isolated from the Jozankei hot spring (Supplementary Figure S8).
Discussion
To the best of our knowledge, strain M52 is the first arsenite-oxidizing betaproteobacterium which possesses the arx genes. It was isolated from a microbial mat of a hot spring which contained approximately 3 mg/L of total arsenic (Kubota et al., 2010; Ospino et al., 2018). From the same mat, partial arxA gene sequences were detected with culture-independent methods (Ospino et al., 2018). The arxA gene of strain M52 was not detected in that analysis, suggesting that culture-based approaches are still effective to explore diversity of arsenite-oxidizing bacteria with arx genes. Although strain M52 lacks aio genes in the genome, it oxidized arsenite under microaerophilic and nitrate-reducing conditions. Some genomic features of the strain are consistent with these observations, as the genome harbors genes for respiration with oxygen and nitrate. The two types of terminal oxidases encoded in the genome are both high-affinity terminal oxidases characterized by low Km values for oxygen (Morris and Schmidt, 2013), suggesting that the strain M52 is adapted to a low-oxygen environment. The concentration of oxygen in the air may be too high for this organism, and it might have suppressed arsenite oxidaion activity (Figure 2B). As another organism which oxidizes arsenite under microoxic conditions, Hydrogenobaculum strain H55 was reported in a previous study (Donahoe-Christiansen et al., 2004). In constrast to strain M52, strain H55 has genes for AIO (Clingenpeel et al., 2009).
The arx gene cluster of strain M52 had the same set of genes as those found in A. ehrlichii MLHE-1 and Ectothiorhodospira sp. PHS-1, with some small differences in positions of the genes. The comparative analysis showed that the majority of the genes in the arx gene cluster are conserved in more than half of the genomes (Figure 4). Especially, the arxABC genes encoding the putative functional elements of the enzyme are highly conserved. For other enzymes involved in arsenic metabolism, AIO arsenite oxidase and ARR arsenate reductase, the aioBA and arrAB genes encode the constituent subunits, and they are well-conserved in the genomes (Van Lis et al., 2013; Andres and Bertin, 2016). In contrast, the arxC gene homologous in the arr and aio gene cluster is not well-conserved (Slyemi and Bonnefoy, 2012; Grimaldi et al., 2013; Andres and Bertin, 2016). The arxB2 gene, predicted to encode a type of ferredoxin protein, appears to be specific to the arx gene cluster, since no homologous genes have been described for AIO or ARR. A previous study demonstrated the expression of the arxB2 gene in Ectothiorhodospira sp. BSL-9 (Hernandez-Maldonado et al., 2017). Additionally, transcripts were detected in the southern basin of Mono Lake (Edwardson and Hollibaugh, 2017). However, the role of arxB2 in the arsenite oxidation remain unclear. The arxD gene was also highly conserved and predicted to encode a TorD-like protein required to introduce the cofactor into the enzyme (Genest et al., 2009). The occurrence of homologous genes in the aio or arr gene cluster (aioD and arrD) is variable as well (Van Lis et al., 2013; Andres and Bertin, 2016). The arxXSR genes are absent from the arx gene cluster of the genomes from members of the order Oceanospirillales (Figure 4). Similar findings were reported in previous studies on the gene clusters of aio and arr, which indicated that the presence of aioXSR and arrXSR is variable among taxonomic groups (Slyemi and Bonnefoy, 2012; Van Lis et al., 2013; Badilla et al., 2018). The aioXSR genes regulate the expression of the aioAB genes in some arsenite-oxidizing strains (Kashyap et al., 2006; Koechler et al., 2010; Sardiwal et al., 2010; Liu et al., 2012; Li et al., 2013). A bacterium which lacks the aioXSR module, Halomonas sp. HAL1, seems to employ another two-component system to regulate expression of aioAB (Chen et al., 2015). Thus far, the involvement of the arxXSR genes in regulation of the expression of arxAB has not been demonstrated.
The incongruency between the phylogeny of ARX and RPs suggested that horizontal gene transfer events have affected the evolution of the ARX and arsenite-oxidizing bacteria (Supplementary Figure S3). In other arsenic-related enzymes, the involvement of horizontal gene transfer in their evolutionary history has been reported as well (Andres and Bertin, 2016). In the genomes harboring arx genes, some features suggestive of gene transfer were identified. Some of the arx gene clusters were located in mobile genetic elements such as plasmids, as in Sulfuricella denitrificans skB26 (Watanabe et al., 2014) and Halomonas sp. A3H3 (NCBI Reference Sequence: NZ_HG423344.1) (Koechler et al., 2013), and in a genomic island in Azoarcus sp. CIB (Martin-Moldes et al., 2015). In strain M52, a gene encoding transposase (92% identity to the IS110 family transposase of Thiomonas sp.) was identified upstream of the arxRSX.
In this study, ARX were grouped into three groups based on phylogenetic analysis of ArxAB (Figure 6). The three groups have some specific characteristics, as follows. Strains with ARX in group 1 are all haloalkaliphiles and belong to the orders Chromatiale and Oceanospirillales. The phylogenetic analysis of previously reported data reconfirmed that the partial arxA sequences clustered with group 1 were all detected in environments with high pH and salinity. The sole exception was Desulfotomaculum sp. TC-1 obtained by PCR amplification (Wu et al., 2017). This strain exhibits optimum growth at pH 6.8 and belongs to the phylum Firmicutes, although arx genes identified in this study were only in the genomes of Proteobacteria. More detailed analysis of this organism is necessary to obtain the full sequences of the arx genes. Ectothiorhodospira sp. PHS-1 is haloalkaliphile belonging to the order Chromatiale, but its ARX was classified into group 2. Group 2 seemed to be the most heterogeneous group, because the genes encoding this type of ARX were identified in seven genomes belonging to four classes in the phylum Proteobacteria. These genomes included those of phototrophic haloalkaliphiles, heterotrophs growing in low-salt medium, and MAGs obtained from freshwater environments. Partial sequences of arxA, related to group 2, were detected in a freshwater lake and an alkaline salt lake. The former is Lake Mizugaki, from which some OTUs were obtained in this study, and the latter is Mono Lake, where close relatives of Ectothiorhodospira sp. PHS-1 were detected in previous studies (Figure 8). In contrast to these groups, ARX of group 3 was not identified in the genome of phototrophic organisms. The isolated strains with group 3 ARX are all sulfur-oxidizing chemolithoautotrophs. The MAGs with this type of ARX were obtained from a CO2-driven geyser (Probst et al., 2017) and groundwater sample (Anantharaman et al., 2016), suggesting that corresponding organisms do not depend on light. These findings also suggest that the ARX of group 3 is specific for low-salt environments. This idea is supported by phylogenetic analysis of the partial arxA gene sequences. Among the previously reported partial sequences, those from Hot Creek riverbed sediment were clustered with group 3 (Supplementary Figure S8). This sample is characterized by low salt concentrations in comparison to the other samples analyzed in the previous studies. The other partial sequences of group 3 were obtained in this study. Group 3 is characterized by a phylogenetic relationship that is highly congruent with the phylogeny of ArxD (Supplementary Figure S4).
The long insertion in ArxA was identified only in ARX of group 3 and encoded in the genomes of Betaproteobacteria and Gammaproteobacteria. The clone library analysis in this this study expanded knowledge about the diversity of ArxA with insertions (Supplementary Figure S8). It is important to point out that the primers used for this analysis were designed based on a limited number of group 3 ArxA sequences related to each other, and failed to amplify the arxA gene of strain M52 and J5B (Supplementary Figure S7). Therefore, the results have been affected by PCR bias and close relatives of strain M52 might be missed in the analysis. However, application of these primers resulted in the unexpected detection of arxA without insertions. One of the samples used in this analysis was the isolation source of strain M52, and the other one was used in a previous study to detect the arrA gene (Watanabe et al., 2017). In that study, arsenate respiration by Sulfuritalea in the deep water of Lake Mizugaki was suggested (Watanabe et al., 2017). In another sample of deep water obtained from the same lake in a different year, partial arxA gene sequences were detected with another primer pair (Ospino et al., 2018). It is plausible that anaerobic bacteria detected with forms of arxA and arrA are driving the arsenate cycle in the anoxic layer of water in this lake. In contrast to ARX of group 1 and group 2, involvement of the group 3 ARX in arsenite oxidation has not been demonstrated. Strain M52 is the first organism for which the presence of the genes for group 3 ARX and arsenite oxidation were both demonstrated (Figures 2, 3). The absence of the aio gene cluster in the genome of strain M52 suggests that the arx genes are responsible for its arsenite oxidation. However, further experimental evidence is required to confirm arsenite oxidation by group 3 ARX. Strain M52 also has a long insertion in ArxA and an unusual gene arrangement in the arx gene cluster characterized by the unique position of arxB2 (Figure 4). The role of these elements in arsenite oxidation by strain M52 will be important subject of further studies.
Author Contributions
MO, HK, and MF designed the study. MO and HK performed experiments and wrote the manuscript. MO conducted data analysis. All authors contributed to manuscript revision, and approved the submitted version.
Funding
This study was supported by JSPS KAKENHI (Grant No. 15K07209).
Conflict of Interest Statement
The authors declare that the research was conducted in the absence of any commercial or financial relationships that could be construed as a potential conflict of interest.
Acknowledgments
We thank Arisa Shinohara, Tomohiro Watanabe, and Tomoya Iwata for their assistance.
Supplementary Material
The Supplementary Material for this article can be found online at: https://www.frontiersin.org/articles/10.3389/fmicb.2019.01210/full#supplementary-material
Footnotes
References
Anantharaman, K., Brown, C. T., Hug, L. A., Sharon, I., Castelle, C. J., Probst, A. J., et al. (2016). Thousands of microbial genomes shed light on interconnected biogeochemical processes in an aquifer system. Nat. Commun. 7, 1–11. doi: 10.1038/ncomms13219
Andres, J., and Bertin, P. N. (2016). The microbial genomics of arsenic. FEMS Microbiol. Rev. 39:fuv050. doi: 10.1093/femsre/fuv050
Anisimova, M., and Gascuel, O. (2006). Approximate likelihood-ratio test for branches: a fast, accurate, and powerful alternative. Syst. Biol. 55, 539–552. doi: 10.1080/10635150600755453
Badilla, C., Osborne, T. H., Cole, A., Watson, C., Djordjevic, S., and Santini, J. M. (2018). A new family of periplasmic-binding proteins that sense arsenic oxyanions. Sci. Rep. 8, 1–12. doi: 10.1038/s41598-018-24591-w
Bendtsen, J. D., Nielsen, H., Widdick, D., Palmer, T., and Brunak, S. (2005). Prediction of twin-arginine signal peptides. BMC Bioinform. 6:167. doi: 10.1186/1471-2105-6-167
Buchfink, B., Xie, C., and Huson, D. H. (2014). Fast and sensitive protein alignment using DIAMOND. Nat. Methods 12, 59–60. doi: 10.1038/nmeth.3176
Challacombe, J. F., Majid, S., Deole, R., Brettin, T. S., Bruce, D., Delano, S. F., et al. (2013). Complete genome sequence of Halorhodospira halophila SL1. Stand. Genomic Sci. 8, 206–214. doi: 10.4056/sigs.3677284
Chen, F., Cao, Y., Wei, S., Li, Y., Li, X., Wang, Q., et al. (2015). Regulation of arsenite oxidation by the phosphate two-component system PhoBR in Halomonas sp. HAL1. Front. Microbiol. 6:923. doi: 10.3389/fmicb.2015.00923
Clingenpeel, S. R., D’Imperio, S., Oduro, H., Druschel, G. K., and McDermott, T. R. (2009). Cloning and in situ expression etudies of the Hydrogenobaculum arsenite oxidase genes. Appl. Environ. Microbiol. 75, 3362–3365. doi: 10.1128/AEM.00336-339
Connon, S. A., Koski, A. K., Neal, A. L., Wood, S. A., and Magnuson, T. S. (2008). Ecophysiology and geochemistry of microbial arsenic oxidation within a high arsenic, circumneutral hot spring system of the Alvord Desert. FEMS Microbiol. Ecol. 64, 117–128. doi: 10.1111/j.1574-6941.2008.00456.x
Dereeper, A., Guignon, V., Blanc, G., Audic, S., Buffet, S., Chevenet, F., et al. (2008). Phylogeny.fr: robust phylogenetic analysis for the non-specialist. Nucleic Acids Res. 36, 465–469. doi: 10.1093/nar/gkn180
Donahoe-Christiansen, J., D’Imperio, S., Jackson, C. R., Inskeep, W. P., and McDermott, T. R. (2004). Arsenite-oxidizing Hydrogenobaculum strain isolated from an acid-sulfate-chloride geothermal spring in Yellowstone National Park. Appl. Environ. Microbiol. 70, 1865–1868. doi: 10.1128/AEM.70.3.1865-1868.2004
Drozdetskiy, A., Cole, C., Procter, J., and Barton, G. J. (2015). JPred4: a protein secondary structure prediction server. Nucleic Acids Res. 43, W389–W394. doi: 10.1093/nar/gkv332
Edgar, R. C. (2004). MUSCLE: multiple sequence alignment with high accuracy and high throughput. Nucleic Acids Res. 32, 1792–1797. doi: 10.1093/nar/gkh340
Edwardson, C. F., and Hollibaugh, J. T. (2017). Metatranscriptomic analysis of prokaryotic communities active in sulfur and arsenic cycling in Mono Lake, California, USA. ISME J. 11, 2195–2208. doi: 10.1038/ismej.2017.80
Finn, R. D., Bateman, A., Clements, J., Coggill, P., Eberhardt, R. Y., Eddy, S. R., et al. (2014). Pfam: the protein families database. Nucleic Acids Res. 42, 222–230. doi: 10.1093/nar/gkt1223
Gascuel, O. (1997). BIONJ: an improved version of the NJ algorithm based on a simple model of sequence data. Mol. Biol. Evol. 14, 685–695. doi: 10.1093/oxfordjournals.molbev.a025808
Genest, O., Méjean, V., and Iobbi-Nivol, C. (2009). Multiple roles of TorD-like chaperones in the biogenesis of molybdoenzymes. FEMS Microbiol. Lett. 297, 1–9. doi: 10.1111/j.1574-6968.2009.01660.x
Glasser, N. R., Oyala, P. H., Osborne, T. H., Santini, J. M., and Newman, D. K. (2018). Structural and mechanistic analysis of the arsenate respiratory reductase provides insight into environmental arsenic transformations. Proc. Natl. Acad. Sci. U.S.A. 115, E8614–E8623. doi: 10.1073/pnas.1807984115
Grimaldi, S., Schoepp-cothenet, B., Ceccaldi, P., Guigliarelli, B., and Magalon, A. (2013). The prokaryotic Mo / W- bis PGD enzymes family: a catalytic workhorse in bioenergetic. Biochim. Biophys. Acta 1827, 1048–1085. doi: 10.1016/j.bbabio.2013.01.011
Guindon, S., Dufayard, J. F., Lefort, V., Anisimova, M., Hordijk, W., and Gascuel, O. (2010). New algorithms and methods to estimate maximum-likelihood phylogenies: assessing the performance of PhyML 3.0. Syst. Biol. 59, 307–321. doi: 10.1093/sysbio/syq010
Hamamura, N., Itai, T., Liu, Y., Reysenbach, A. L., Damdinsuren, N., and Inskeep, W. P. (2014). Identification of anaerobic arsenite-oxidizing and arsenate-reducing bacteria associated with an alkaline saline lake in Khovsgol, Mongolia. Environ. Microbiol. Rep. 6, 476–482. doi: 10.1111/1758-2229.12144
Hernandez-Maldonado, J., Sanchez-Sedillo, B., Stoneburner, B., Boren, A., Miller, L., Mccann, S., et al. (2017). The genetic basis of anoxygenic photosynthetic arsenite oxidation. Environ. Microbiol. 19, 130–141. doi: 10.1111/1462-2920.13509
Hernandez-Maldonado, J., Stoneburner, B., Boren, A., Miller, L., Rosen, M., Oremland, R. S., et al. (2016). Genome sequence of the photoarsenotrophic bacterium Ectothiorhodospira sp. strain BSL-9, isolated from a hypersaline alkaline arsenic-rich extreme environment. Genome Announc. 4, 9–10. doi: 10.1128/genomeA.01139-1116
Hoeft McCann, S., Boren, A., Hernandez-Maldonado, J., Stoneburner, B., Saltikov, C., Stolz, J., et al. (2017). Arsenite as an electron donor for anoxygenic photosynthesis: description of three strains of Ectothiorhodospira from Mono Lake, California and Big Soda Lake, Nevada. Life 7, 1–14. doi: 10.3390/life7010001
Hyatt, D., Chen, G. L., LoCascio, P. F., Land, M. L., Larimer, F. W., and Hauser, L. J. (2010). Prodigal: prokaryotic gene recognition and translation initiation site identification. BMC Bioinform. 11:119. doi: 10.1186/1471-2105-11-119
Kashyap, D. R., Botero, L. M., Franck, W. L., Daniel, J., Mcdermott, T. R., and Hassett, D. J. (2006). Complex regulation of arsenite oxidation in Agrobacterium tumefaciens. J. Bacteriol. 188, 1081–1088. doi: 10.1128/JB.188.3.1081
Katoh, K., Rozewicki, J., and Yamada, K. D. (2017). MAFFT online service: multiple sequence alignment, interactive sequence choice and visualization. Brief. Bioinform. [Epub ahead of print].
Koechler, S., Cleiss-Arnold, J., Proux, C., Sismeiro, O., Dillies, M.-A., Goulhen-Chollet, F., et al. (2010). Multiple controls affect arsenite oxidase gene expression in Herminiimonas arsenicoxydans. BMC Microbiol. 10:53. doi: 10.1186/1471-2180-10-53
Koechler, S., Plewniak, F., Barbe, V., Battaglia-Brunet, F., Jost, B., Joulian, C., et al. (2013). Genome sequence of Halomonas sp. strain A3H3, isolated from arsenic-rich marine sediments. Genome Announc. 1:e00819-13. doi: 10.1128/genomeA.00819-813
Kojima, H., Watanabe, M., and Fukui, M. (2017). Sulfuritortus calidifontis gen. nov., sp. nov., a sulfur oxidizer isolated from a hot spring microbial mat. Int. J. Syst. Evol. Microbiol. 67, 1355–1358. doi: 10.1099/ijsem.0.001813
Kubota, Y., Sakakibara, M., and Sano, S. (2010). Arsenic cocentration of hot spring and river waters at Jyozankei hot spring area in Sapporo city, western Hokkaido, Japan. Mem. Fac. Sci. Ehime Univ. 16, 7–13.
Kwok, S. C., and Hodges, R. S. (2004). Stabilizing and destabilizing clusters in the hydrophobic core of long two-stranded α-helical coiled-coils. J. Biol. Chem. 279, 21576–21588. doi: 10.1074/jbc.M401074200
Lefort, V., Desper, R., and Gascuel, O. (2015). FastME 2. 0: a comprehensive, accurate, and fast distance-based phylogeny inference program. Mol. Biol. Evol. 32, 2798–2800. doi: 10.1093/molbev/msv150
Li, H., Li, M., Huang, Y., Rensing, C., and Wang, G. (2013). In silico analysis of bacterial arsenic islands reveals remarkable synteny and functional relatedness between arsenate and phosphate. Front. Microbiol. 4:347. doi: 10.3389/fmicb.2013.00347
Liu, G., Liu, M., Kim, E. H., Maaty, W. S., Bothner, B., Lei, B., et al. (2012). A periplasmic arsenite-binding protein involved in regulating arsenite oxidation. Environ. Microbiol. 14, 1624–1634. doi: 10.1111/j.1462-2920.2011.02672.x
Lupas, A. (1997). Predicting coiled-coil regions in proteins. Curr. Opin. Struct. Biol. 7, 388–393. doi: 10.1016/S0959-440X(97)80056-80055
Martin-Moldes, Z., Zamarro, M. T., del Cerro, C., Valencia, A., Gomez, M. J., Arcas, A., et al. (2015). Whole-genome analysis of Azoarcus sp. strain CIB provides genetic insights to its different lifestyles and predicts novel metabolic features. Syst. Appl. Microbiol. 38, 462–471. doi: 10.1016/j.syapm.2015.07.002
Monera, O. D., Hodges, R. S., Zhou, N. E., Lavigne, P., and Kay, C. M. (2002). Formation of parallel and antiparallel coiled-coils controlled by the relative positions of alanine residues in the hydrophobic core. J. Biol. Chem. 271, 3995–4001. doi: 10.1074/jbc.271.8.3995
Morris, R. L., and Schmidt, T. M. (2013). Shallow breathing: bacterial life at low O2. Nat. Rev. Microbiol. 11, 205–212. doi: 10.1038/nrmicro2970
Oremland, R. S., Hoeft, S. E., Santini, J. M., Bano, N., Hollibaugh, R. A., and Hollibaugh, J. T. (2002). Anaerobic oxidation of arsenite in Mono Lake water and by a facultative, arsenite-oxidizing chemoautotroph, strain MLHE-1. Appl. Environ. Microbiol. 68, 4795–4802. doi: 10.1128/AEM.68.10.4795
Oremland, R. S., Miller, L. G., Colbertson, C. W., Robinson, S. W., Smith, R. L., Lovley, D., et al. (1993). “Aspects of the biogeochemistry of methane in Mono Lake and the Mono Basin of California,” in Biogeochemistry of Global Change, ed. R. S. Oremland (Boston, MA: Springer), 704–741. doi: 10.1007/978-1-4615-2812-8
Oremland, R. S., Saltikov, C. W., Wolfe-Simon, F., and Stolz, J. F. (2009). Arsenic in the evolution of earth and extraterrestrial ecosystems. Geomicrobiol. J. 26, 522–536. doi: 10.1080/01490450903102525
Ospino, M. C., Kojima, H., Watanabe, T., Iwata, T., and Fukui, M. (2018). Diversity of anaerobic arsenite-oxidizing bacteria in low-salt environments analyzed with a newly developed PCR-based method. Limnology 19, 177–183. doi: 10.1007/s10201-018-0539-531
Overbeek, R., Olson, R., Pusch, G. D., Olsen, G. J., Davis, J. J., Disz, T., et al. (2014). The SEED and the rapid annotation of microbial genomes using subsystems technology (RAST). Nucleic Acids Res. 42, 206–214. doi: 10.1093/nar/gkt1226
Probst, A. J., Castelle, C. J., Singh, A., Brown, C. T., Anantharaman, K., Sharon, I., et al. (2017). Genomic resolution of a cold subsurface aquifer community provides metabolic insights for novel microbes adapted to high CO2 concentrations. Environ. Microbiol. 19, 459–474. doi: 10.1111/1462-2920.13362
Sardiwal, S., Santini, J. M., Osborne, T. H., and Djordjevic, S. (2010). Characterization of a two-component signal transduction system that controls arsenite oxidation in the chemolithoautotroph NT-26. FEMS Microbiol. Lett. 313, 20–28. doi: 10.1111/j.1574-6968.2010.02121.x
Schloss, P. D., Westcott, S. L., Ryabin, T., Hall, J. R., Hartmann, M., Hollister, E. B., et al. (2009). Introducing mothur: open-source, platform-independent, community-supported software for describing and comparing microbial communities. Appl. Environ. Microbiol. 75, 7537–7541. doi: 10.1128/AEM.01541-1549
Slyemi, D., and Bonnefoy, V. (2012). How prokaryotes deal with arsenic. Environ. Microbiol. Rep. 4, 571–586. doi: 10.1111/j.1758-2229.2011.00300.x
Surkont, J., and Pereira-Leal, J. B. (2015). Evolutionary patterns in coiled-coils. Genome Biol. Evol. 7, 545–556. doi: 10.1093/gbe/evv007
Talavera, G., and Castresana, J. (2007). Improvement of phylogenies after removing divergent and ambiguously aligned blocks from protein sequence alignments. Syst. Biol. 56, 564–577. doi: 10.1080/10635150701472164
Tamura, K., Stecher, G., Peterson, D., Filipski, A., and Kumar, S. (2013). MEGA6: molecular evolutionary genetics analysis version 6.0. Mol. Biol. Evol. 30, 2725–2729. doi: 10.1093/molbev/mst197
Truebestein, L., and Leonard, T. A. (2016). Coiled-coils: the long and short of it. BioEssays 38, 903–916. doi: 10.1002/bies.201600062
Umezawa, K., Watanabe, T., Miura, A., Kojima, H., and Fukui, M. (2016). The complete genome sequences of sulfur-oxidizing Gammaproteobacteria Sulfurifustis variabilis skN76Tand Sulfuricaulis limicola HA5T. Stand. Genomic Sci. 11:71. doi: 10.1186/s40793-016-0196-0
Van Lis, R., Nitschke, W., Duval, S., and Schoepp-Cothenet, B. (2013). Arsenics as bioenergetic substrates. Biochim. Biophys. Acta Bioenerg. 1827, 176–188. doi: 10.1016/j.bbabio.2012.08.007
Watanabe, T., Kojima, H., and Fukui, M. (2014). Complete genomes of freshwater sulfur oxidizers Sulfuricella denitrificans skB26 and Sulfuritalea hydrogenivorans sk43H: genetic insights into the sulfur oxidation pathway of betaproteobacteria. Syst. Appl. Microbiol. 37, 387–395. doi: 10.1016/j.syapm.2014.05.010
Watanabe, T., Kojima, H., Umezawa, K., Hori, C., Takasuka, T. E., Kato, Y., et al. (2019). Genomes of neutrophilic sulfur-oxidizing chemolithoautotrophs representing 9 proteobacterial species from 8 genera. Front. Microbiol. 10:316. doi: 10.3389/fmicb.2019.00316
Watanabe, T., Miura, A., Iwata, T., Kojima, H., and Fukui, M. (2017). Dominance of Sulfuritalea species in nitrate-depleted water of a stratified freshwater lake and arsenate respiration ability within the genus. Environ. Microbiol. Rep. 9, 522–527. doi: 10.1111/1758-2229.12557
Widdel, F., and Bak, F. (1992). “Gram-negative mesotrophic sulfate-reducing bacteria,” in The Prokaryotes, eds A. Balows, H. G. Trüper, M. Dworkin, W. Harder, and K.-H. Schleifer (New York, NY: Springer), 3352–3378. doi: 10.1007/978-1-4757-2191-1_21
Wilkie, J. A., and Hering, J. G. (1998). Rapid oxidation of geothermal arsenic(III) in streamwaters of the eastern Sierra Nevada. Environ. Sci. Technol. 32, 657–662. doi: 10.1021/es970637r
Wu, G., Huang, L., Jiang, H., Peng, Y., Guo, W., Chen, Z., et al. (2017). Thioarsenate formation coupled with anaerobic arsenite oxidation by a sulfate-reducing bacterium isolated from a hot spring. Front. Microbiol. 8:1336. doi: 10.3389/fmicb.2017.01336
Yu, G., Smith, D. K., Zhu, H., Guan, Y., and Lam, T. T. Y. (2017). Ggtree: an R package for visualization and annotation of phylogenetic trees with their covariates and other associated data. Methods Ecol. Evol. 8, 28–36. doi: 10.1111/2041-210X.12628
Zargar, K., Conrad, A., Bernick, D. L., Lowe, T. M., Stolc, V., Hoeft, S., et al. (2012). ArxA, a new clade of arsenite oxidase within the DMSO reductase family of molybdenum oxidoreductases. Environ. Microbiol. 14, 1635–1645. doi: 10.1111/j.1462-2920.2012.02722.x
Keywords: arsenite oxidation, ARX, betaproteobacterium, arx gene cluster, freshwater, bacterial genomes
Citation: Ospino MC, Kojima H and Fukui M (2019) Arsenite Oxidation by a Newly Isolated Betaproteobacterium Possessing arx Genes and Diversity of the arx Gene Cluster in Bacterial Genomes. Front. Microbiol. 10:1210. doi: 10.3389/fmicb.2019.01210
Received: 18 January 2019; Accepted: 13 May 2019;
Published: 29 May 2019.
Edited by:
David Emerson, Bigelow Laboratory for Ocean Sciences, United StatesReviewed by:
Susan Childers, Colby College, United StatesRonald Oremland, United States Geological Survey, United States
Copyright © 2019 Ospino, Kojima and Fukui. This is an open-access article distributed under the terms of the Creative Commons Attribution License (CC BY). The use, distribution or reproduction in other forums is permitted, provided the original author(s) and the copyright owner(s) are credited and that the original publication in this journal is cited, in accordance with accepted academic practice. No use, distribution or reproduction is permitted which does not comply with these terms.
*Correspondence: Melody Cabrera Ospino, bWVsb2R5LmNhYnJlcmEub3NwaW5vQGdtYWlsLmNvbQ==; Hisaya Kojima, a29qaW1haEBwb3AubG93dGVtLmhva3VkYWkuYWMuanA=