- 1School of Biomedical Sciences, The University of Western Australia, Perth, WA, Australia
- 2Wal-yan Respiratory Research Center, Telethon Kids Institute, The University of Western Australia, Crawley, WA, Australia
- 3Department of Respiratory Medicine, Sir Charles Gairdner Hospital, Perth, WA, Australia
- 4Institute for Respiratory Health, School of Medicine, The University of Western Australia, Perth, WA, Australia
- 5The Marshall Center for Infectious Diseases Research and Training, School of Biomedical Sciences, The University of Western Australia, Perth, WA, Australia
- 6Department of Respiratory and Sleep Medicine, Perth Children’s Hospital, Perth, WA, Australia
- 7Center for Cell Therapy and Regenerative Medicine, School of Medicine and Pharmacology, The University of Western Australia and Harry Perkins Institute of Medical Research, Perth, WA, Australia
- 8Occupation and the Environment, School of Public Health, Curtin University, Perth, WA, Australia
- 9Murdoch Children’s Research Institute, Melbourne, VIC, Australia
- 10Department of Pediatrics, University of Melbourne, Melbourne, VIC, Australia
Individuals with cystic fibrosis (CF) are given antimicrobials as prophylaxis against bacterial lung infection, which contributes to the growing emergence of multidrug resistant (MDR) pathogens isolated. Pathogens such as Pseudomonas aeruginosa that are commonly isolated from individuals with CF are armed with an arsenal of protective and virulence mechanisms, complicating eradication and treatment strategies. While translation of phage therapy into standard care for CF has been explored, challenges such as the lack of an appropriate animal model demonstrating safety in vivo exist. In this review, we have discussed and provided some insights in the use of primary airway epithelial cells to represent the mucoenvironment of the CF lungs to demonstrate safety and efficacy of phage therapy. The combination of phage therapy and antimicrobials is gaining attention and has the potential to delay the onset of MDR infections. It is evident that efforts to translate phage therapy into standard clinical practice have gained traction in the past 5 years. Ultimately, collaboration, transparency in data publications and standardized policies are needed for clinical translation.
Introduction
Cystic Fibrosis (CF) is a life-limiting genetic disease caused by mutations to the Cystic Fibrosis Transmembrane Regulator (CFTR) gene. There are 2,000 variant mutations in the CFTR gene, however, more than 70% of people with CF carry the p.Phe508del mutation. Currently there are six classes of mutations, each with varying degrees of disruption to CFTR protein production and function (Pettit and Fellner, 2014; Lopes-Pacheco, 2016; Veit et al., 2016), correlating to varying severity of disease phenotype. The genetic defect leads to impaired water and electrolyte traffic of airway epithelial cells (AECs), tenacious airway surface liquid, impaired mucociliary clearance mucus build-up in the lungs of those afflicted due to the inability of AECs to allow chloride ions to pass into the airway surface liquid (Crawford et al., 1991; Haq et al., 2016). This leads to airway obstruction, accelerated lung function decline, reduced quality of life, and ultimately premature lung failure and death. With defective mucociliary clearance, accumulation of mucus in the airway of CF lungs occurs, creating an ideal microenvironment for the growth and persistence of bacteria. The inability to clear these bacteria allows opportunistic pathogens to establish a niche within the environment, ensuring their survival (Moreau-Marquis et al., 2008; Bjarnsholt et al., 2009; Ramsay et al., 2016).
With an increasing lifespan of CF patients due to currently available therapies and surveillance programs, isolation of multidrug resistant (MDR) bacteria from the respiratory tract has also increased. Pseudomonas aeruginosa (P. aeruginosa) remains by far the most common airway pathogen particularly amongst adults with CF. The CF Foundation has recently reported ∼17.9% of P. aeruginosa isolated from CF individuals in North America were MDR (Cystic Fibrosis Foundation, 2019). An earlier study conducted across CF centers in Australia found that 31 and 35% of P. aeruginosa isolated from a pediatric and an adult cohort, respectively, were also MDR (Smith et al., 2016). It is known that the lungs of CF individuals are prone to bacterial colonization, particularly by P. aeruginosa. Once colonized, the bacteria are impossible to eradicate and individuals undergo long-term antimicrobial regimes to treat and prophylactically control the rate of infection. The increasing rates of MDR infections are attributed to the prolonged use of antimicrobial drugs for both treatment and prophylaxis, and consequent gain of resistance genes and selection of hypermutator isolates (Kidd et al., 2013, 2018). Cross infection of MDR P. aeruginosa strains due to less stringent infection control measures had also contributed to the rapid rise in MDR rates over the past two decades (Salunkhe et al., 2005; Johansen et al., 2008; Fothergill et al., 2012; Parkins et al., 2014). Treatments for MDR pathogens are accompanied with a caveat; cumulative antibiotic burden leads to development of drug allergy and toxicity (Levison and Levison, 2009; Kalghatgi et al., 2013; Gao et al., 2017). Progressive limitation in antimicrobial treatment in CF, particularly in the aging population given the emergence of MDR organisms, antimicrobial allergy and toxicity are associated with lengthier hospitalizations, increased rates of re-admittance, and extensive treatment regimens (Baumann et al., 2003; Sansgiry et al., 2012).
The issue of MDR is now so widespread that World Health Organization (WHO) has declared the issue of antimicrobial resistance a global crisis (Shrivastava et al., 2017). The discovery pipeline into new classes of antimicrobials is also slow since it is relatively unprofitable, and innovations are unable to keep up with emerging resistance. To address this, alternative treatment methods must be explored. Treatment strategies targeting bacterial virulence and resistance have been studied extensively. Anti-virulence compounds such as quorum sensing inhibitors (Müh et al., 2006; Brackman et al., 2011; O’Loughlin et al., 2013; Soukarieh et al., 2020) and iron chelation (Moreau-Marquis et al., 2009; Parquet et al., 2018) have been found to be successful in inhibiting biofilm formation, reducing pathogenicity and increasing susceptibility to traditional antimicrobials. Strategies targeting resistance have included investigating efflux pump inhibitors (Sabatini et al., 2013; Shriram et al., 2018), anti-sense oligomers (Geller et al., 2013; Sawyer et al., 2013), immunotherapy (Feigman et al., 2018), host defense peptides (Overhage et al., 2008; de la Fuente-Núñez et al., 2015) and bacteriophages. Many of these strategies are still in exploration and validation phases and are still some way off from translating to standard clinical care practice. The vital need for a swift translation of alternative therapy into clinical use has identified bacteriophage (phage) therapy as one of the top candidates due to its successful use in humans when approved on compassionate grounds. Phage treatments are also cheaper due to shorter treatment periods, exhibit little or no toxicity, and are more effective than current antimicrobial strategies (Alemayehu et al., 2012; Agarwal et al., 2018; Oliveira et al., 2020).
Bacteriophages and Their Therapeutic Applications to Date
Bacteriophages are viruses found ubiquitously on Earth. They are able to undergo two life cycles: lytic (lyse the bacterial host in the process of replication) or lysogenic (integrate into the genome of bacterial host). Thus, when considering these for therapeutic application, selection should primarily be limited to the use of lytic phages in order to minimize the possibility of virulence or resistance genes transfer. They were first described and observed to display lytic activity in the early 1910s by microbiologists Frederick W. Twort and Felix d’Herelle (Twort, 1914; d’Herelle, 1917) but despite their initial success, they were soon overshadowed by the introduction of antibiotics (Clokie et al., 2011). Antibiotics were considered a cheap and safe way to treat bacterial infections and their assessment was typically accompanied with well documented research that demonstrated their beneficial effects. Although resistance against penicillin emerged almost immediately after its introduction, discovery of new antibiotics maintained their primary use in the Western world. With restricted access, eastern bloc countries progressed phage therapy through to its clinical inauguration. Entities including the Eliava Institute of Bacteriophages, Microbiology and Virology (EIBMV) in Georgia and the Phage Therapy Unit at the Hirszfeld Institute of Immunology and Experimental Therapy in Poland are still operational and patients are provided personalized phage therapy for chronic infections (d’Herelle, 1931; Häusler, 2006; Kutateladze and Adamia, 2008; Chanishvili, 2012, 2016; Kutateladze, 2015; Górski et al., 2018).
In the West, hesitancy in translating to a human treatment pipeline has been primarily due to the lack of published scientific reports and rigorous safety data. However, human phage therapy has gained significant traction in the last 5 years and has been tested clinically (Figure 1). One study used phage therapy to treat a patient who had been infected with MDR Acinetobacter baumanii (A. baumanii) (Schooley et al., 2017) (Figure 1). Multiple rounds of antibiotic treatments had initially failed to control the infection which had spread beyond the abdominal cavity. In vitro experiments were initially conducted to determine phage specificity and efficacy against the infective strain and approval for clinical use was then sought from the Food and Drug Administration (FDA). This was granted on compassionate grounds and treatment resulted in the patient’s full recovery (Schooley et al., 2017). Since this pioneering study, compassionate ground use of phage therapy has been successfully used to treat patients with distinct MDR infections (Chan et al., 2018; Dedrick et al., 2019). A recent Australian study has highlighted the translatable potential of phage therapy into clinical applications to treat multiple patients suffering from severe Staphylococcus aureus (S. aureus) bacteremia (Petrovic Fabijan et al., 2020b). While this specific study supported phage therapy for disseminated bacterial infection, what is less clear is whether it is applicable for patients suffering from chronic soft tissue infection caused by biofilm-producing bacteria.
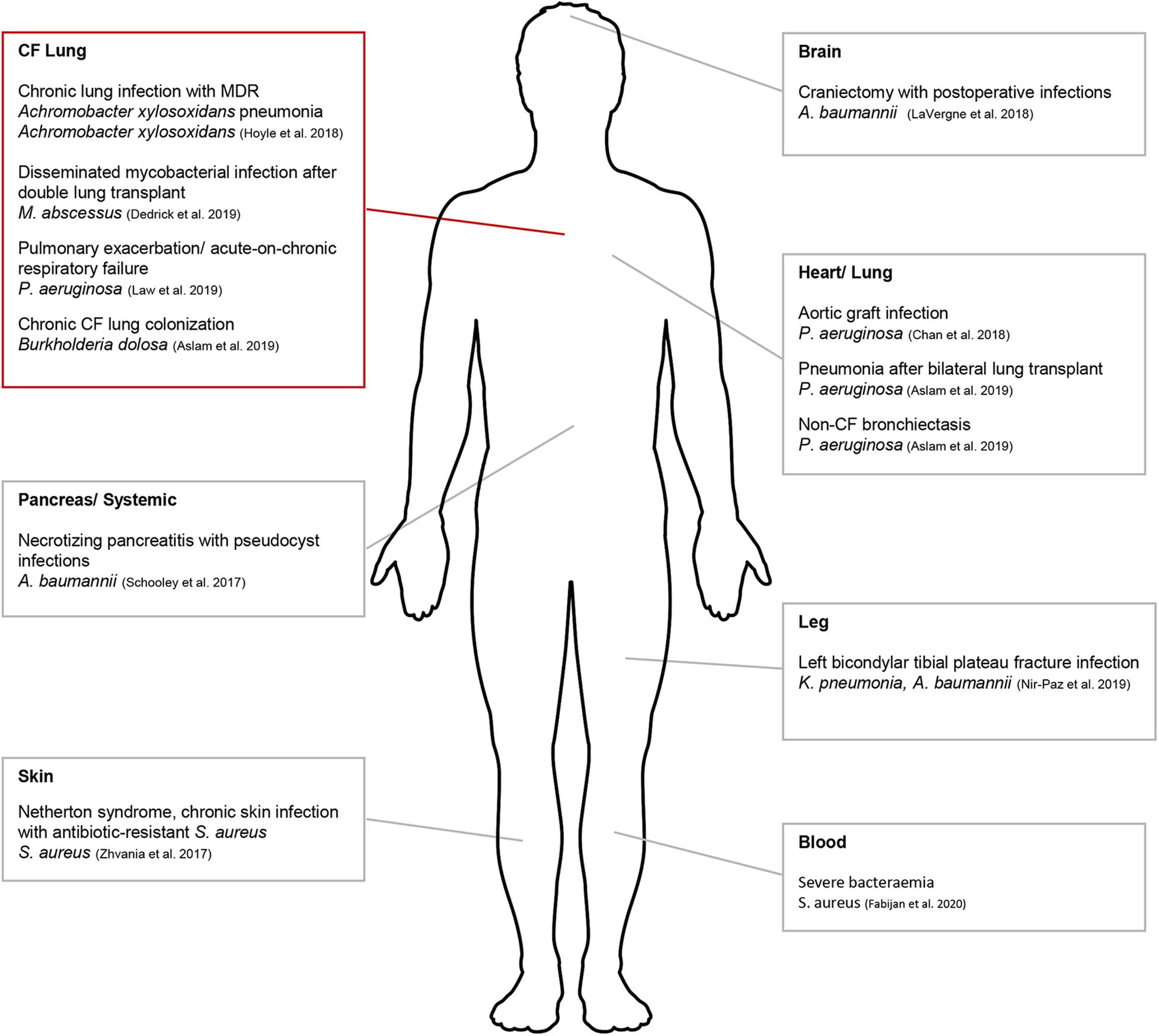
Figure 1. Schematic diagram indicating areas where phage therapy had been applied clinically for compassionate use. Compassionate use in CF denoted by red box. This figure was created using Servier Medical Art templates, which are licensed under a Creative Commons Attribution 3.0 Unported License; https://smart.servier.com.
The Potential of Phage Therapy in CF
In order to more accurately assess how phage therapy is currently advancing, we conducted an initial review of articles in NCBI using the article title search term “Bacteriophage.” We selectively excluded all published abstracts and still identified >12,000 published articles in this area. When “Therapy” was included in the article title search term, 141 published articles were found, including 46 review papers. The remaining 95 original research papers were then screened and found to report on research performed in the medical (83) or veterinary sphere (12). Collectively, these findings imply that despite an enormous amount of research being carried out in the discovery and characterization of phages, the field has struggled to translate results into standard clinical care. Although successful use of phage therapy in CF has been reported, this has been approved on compassionate grounds and its translation into standard clinical care still requires additional rigorous, systematic and detailed exploration (Supplementary Table 1).
While P. aeruginosa, S. aureus, and Burkholderia cepacia complex (B. cepacia complex) are commonly isolated pathogens from individuals with CF, Mycobacterium and other infections are emerging. One ideal therapeutic target is Mycobacterium abscessus (Mab), an emerging pathogen isolated from the lungs of individuals with CF (Bernut et al., 2019). Mab is able to form biofilms (Qvist et al., 2015; Fennelly et al., 2016) and is intrinsically resistant to many antitubercular drugs, requiring prolong usage of at least three antimicrobial drugs for up to 2 years (Floto et al., 2016). While there has been clinical evidence correlating Mab infections with declining lung function, whether the bacterium is the causative agent is unknown (Sanguinetti et al., 2001; Esther et al., 2010; Qvist et al., 2016). The first reported compassionate use of phage therapy in CF was for a 15-year old patient with disseminated Mab infection at sites other than the lung following bilateral lung transplant (Dedrick et al., 2019; Figure 1). Despite additional antimicrobials used, Mab had infected other areas of the body apart from the surgical site. A cocktail of three phages was used and were bioengineered from mutant derivatives that displayed optimum lysis of Mab isolated directly from the patient. A single topical application was followed by intravenous therapy for ∼32 weeks. Upon completion, lung function had improved from 50% FEV1, immediately following transplant up to 80–90% FEV1. The significance of this study lies in the outlined pipeline needed to ensure phage therapy translation including appropriate in vitro safety and efficacy studies.
The second reported compassionate use of phage therapy in CF was for a 26-year old patient who presented with severe acute-on-chronic respiratory failure resulting in mechanical ventilation (Law et al., 2019). The patient was infected with two strains of MDR P. aeruginosa and received multiple courses of high dose antibiotics which then triggered kidney failure. Approval for AP-PA01, a cocktail of four bacteriophages produced by AmpliPhi Biosciences Corporation (now Armata Pharmaceuticals) was granted and phages were administered intravenously. After 8-weeks of treatment, the patient was successfully cleared of P. aeruginosa colonization without side effect, was ambulatory and stable enough to be once again placed on a lung transplantation waitlist. Other pathogens targeted by phage therapy in CF have included Achromobacter xylosoxidans and Burkholderia dolosa (Figure 1), due to increasing incidences of multidrug resistance (Kalish et al., 2006; Jeukens et al., 2017). Phage therapy has also been applied for compassionate use at various sites other than the lung (non-CF associated) suggesting that a number of other CF-associated pathogens including S. aureus, A. baumanii, and Klebsiella pneumoniae may also be clinically targeted using phage therapy (Figure 1). Emerging CF pathogens such as Stenotrophomonas maltophilia, are also of concern due to their increasing incidence of isolation (Millar et al., 2009; Razvi et al., 2009; Emerson et al., 2010) and multidrug resistance (Gajdács and Urbán, 2019; Gröschel et al., 2020). Since their infectivity and transmission mechanisms are still to be elucidated (Stanojevic et al., 2013; Gröschel et al., 2020) which would typically direct targeted treatment regimens, phage therapy has been postulated as a potential therapeutic option currently (Chang et al., 2005; Peters et al., 2015, 2017).
There have also been attempts to assess phage therapy as part of potential standard clinical care, however, rarely have results of performed clinical trials been published. It is critical to report this information in order to inform the trial process and guide future study designs. An example identified through a screen of registered trials on ClinicalTrials.gov is “MUCOPHAGES” (NCT01818206). Although identified as completed, there is no available information on formulation used, length of phage exposure, and importantly what bacteria were being targeted. Closed findings only act to hinder progress and there needs to be greater transparency if we are to pipeline this therapy into standard clinical care.
Another challenge is that most published studies on the efficacy of phage therapy on CF-derived pathogens have been performed using pathogens in planktonic state. However, bacterial pathogens often exist in biofilm state within the CF airways. Such significant differences between the in vitro experiment model and in vivo CF airway conditions render the translation of benchside result to bedside questionable. Biofilms are made up by a pure population or a consortium of microorganisms, creating a unique bacterial lifestyle and niche habitat, enhancing protection against antimicrobials, and able to establish in conditions with a flow of liquid and withstand shear force, forming classic tower-like structures (Flemming and Wingender, 2010; Flemming et al., 2016). Thus, phages with effective biofilm dispersal capabilities are highly desirable. Current evaluation of phage activity on biofilm is usually performed on abiotic surfaces which typically do not account for biological flow strength, nor the properties of the tissues where typically biofilms reside, namely (in the case of CF) the airway epithelium. Flow-cell systems and fluorescence microscopy are considered the “gold standard” to observe spatiotemporal changes of biofilm heterogeneity in real-time (Crusz et al., 2012; Tolker-Nielsen and Sternberg, 2014; Haagensen et al., 2015). The use of flow-cell also maintains biofilm’s viability while the hydrodyanamic movement removes planktonic bacteria and eliminates a confounding factor in the measurement of phage therapy efficacy on the biofilms. Thus, having a biological relevant model of CF would assist in our understanding not only of biofilm formation but also of bacterial evasion of the host innate and adaptive immune responses.
Phage Therapy: CF Experimental Models and What Is Missing
While lung disease is the major cause of morbidity and mortality in CF, there are currently no effective models that mimic the CF lung infection pathology (Table 1). Despite different animal models developed to study CF, the data generated often are still limited by the models. The CF porcine model successfully mimics several CF manifestations including meconium ileus (MI), pancreatic deficiency and subsequent gastrointestinal tract obstruction with similar airway epithelia and submucosal gland (SMG) activities at birth to humans (Rogers et al., 2008). However, the model is not commonly used due to severe disease pathology and difficulties in maintaining longevity of the pigs due to MI. High animal husbandry costs also make the model unfeasible for most laboratories to utilize. CF murine and rodent models have also been explored to mimic the pathophysiological states of CF in vivo. The CF mouse model was developed shortly after the discovery of the CFTR gene (Snouwaert et al., 1992) where it successfully mimicked chronic lung infection with mucoid strains of P. aeruginosa (Coleman et al., 2003). However, differences between the abundance and distribution of airway epithelial cell (AEC) types make the model less translatable to the human CF airway (Plopper et al., 1983; Grubb and Boucher, 1999). In comparison, rats have more developed SMGs and their implication in CF lung pathology is well studied and linked with disease progression in humans (Smolich et al., 1978; Jayaraman et al., 2001; Wine, 2004; Tuggle et al., 2014). Mucus plugging, one of the characteristics of CF lung disease has been observed in the lungs of a Cftr–/– rat model developed by Birket et al. (2018). This is an essential requirement when studying mucociliary transport and bacterial colonization (Birket et al., 2018). Chronic infections are also difficult to establish in these models and typically use bacterial cells embedded into agar beads before lung installation (Bragonzi et al., 2009; Facchini et al., 2014; Kukavica-Ibrulj et al., 2014; Kukavica-Ibrulj and Levesque, 2015; Bayes et al., 2016; Cigana et al., 2016, 2020). Results are also hard to interpret since it is difficult to distinguish induced immune response initiated by bacteria such as P. aeruginosa and the presence of a foreign body (agar beads). Finally, the Cftr–/– ferret model resulted due to the high similarity in the anatomy and biology of its lungs with those of humans (Sun et al., 2008). The CF ferret model mimics the human condition in its susceptibility to lung infection, and lung function decline as the main cause of mortality (Sun et al., 2010, 2014). Despite this model being the best to test therapeutics against lung infection and inflammation, progress in this field remains slow as inconsistencies in severity of lung disease confound the ability to understand the impact of disease progression and efficacy of therapeutics.
Cell Cultures and the Role They Play in Phage Therapy Research
An alternative to the use of animal models is the use of AEC cultures. Universal cell lines such as H441 (lung epithelial cells) (Hermanns et al., 2004) and HeLa (cervical carcinoma cells) (Scherer et al., 1953; Lucey et al., 2009) have been utilized in a wide variety of research ranging from cancer to infection models. Immortalized CF cell lines such as CFBE45o – (CF bronchial epithelial cells) (Gruenert et al., 2004) and CuFi (Zabner et al., 2003) have also been instrumental in different studies including the development and efficacy testing of therapies, both genetic and pharmacological. AEC lines have also been valuable in high-throughput screening of potential drugs. However, submerged monolayer cultures of CF AEC lines are unable to mimic the microenvironment of a CF lung, which is characterized by mucus production and persistent inflammation status. Another limitation of CF AEC lines is the inability to test for interactions and efficacy of phage therapy when used in conjunction with CFTR modulators that target specific mutations in the CFTR gene. However, the use of primary AECs obtained from patients would be able to overcome this limitation. Although CF AEC lines can be grown at air-liquid interface (ALI), there have been inconsistencies in barrier integrity, as well as their capacity to form the multiple cell layers that comprise the airway lining architecture. Furthermore, it is unknown whether they also produce multiple cell types typically including goblet cells (Hermanns et al., 2004; Ren et al., 2016).
Fully differentiated primary AECs have been described extensively in the literature and serve as the most representative in vitro model of the airway (Randell et al., 2011; Martinovich et al., 2017). Although AEC ALI cultures may not mimic the biological environment perfectly, they demonstrate features of the airway, with polarization of progenitor cells into ciliated, basal, undifferentiated columnar and secretory cells. Tight junctions are also well developed in 3D ALI cultures (Crystal et al., 2008; Choi et al., 2016; Looi et al., 2016, 2018). A concerted effort by the Australian Respiratory Early Surveillance Team for Cystic Fibrosis (AREST CF) to biobank AECs collected from children with CF longitudinally has created a valuable repository that is readily accessible (Sutanto et al., 2011; Garratt et al., 2017). With prospective sampling, an in vitro AEC ALI model could be built in the laboratory to screen for and test efficacies of phages against clinical isolates from CF patients, accounting for immunological responses arising from the application of phages. Currently, there are no appropriate biofilm models grown on AECs described in the literature at the time of writing this review (2020). While most research on medical biofilms has been carried out on abiotic surfaces (Alemayehu et al., 2012; Tinoco et al., 2016) or submerged monolayers of AEC (Trend et al., 2018), these are not reflective biological representations of the complex airway epithelium where biofilms establish. Despite advances in cell culture methodologies, recapitulating the entire human lungs remains a challenge. The human lung is a complex organ with more than 59 cell types located in different anatomical locations (Travaglini et al., 2019) and compounding this further, is the complex genotypic and molecular mechanisms within and between these cells. However, a laboratory model is still essential for airway phage therapy application and AEC ALI cultures still remain the most relevant model that mimics key features of the airway epithelium. Recent developments in 3D printing of organs is rapidly evolving and may be able to add further complexity whilst remaining accurate in its recapitulation of the human lung (Grigoryan et al., 2019; Shrestha et al., 2019).
Delivery of prepared phages could also be tested on AEC ALI cultures to capture a more comprehensive pre and post application reaction of the host mammalian cells (Buckley et al., 2018). While topical and/or intravenous applications of delivery have been effective, they are not ideal for pulmonary infections, particularly in pediatrics. Compliance toward the therapy is an important factor that would ensure optimum efficiency of released phages in the lungs against the pathogens. Therefore, inhalation of phages would be the most suitable for pulmonary infections (Sahota et al., 2015; Malik et al., 2017; Wallin et al., 2019). Currently, studies measuring the stability and efficacy of both liquid (Carrigy et al., 2017) and dry powder formulations (Chang et al., 2017, 2020) of nebulized phages have been conducted and show potential in ensuring the dispersion into lower airway of the lungs. Further work is needed to fully elucidate the best formulation and delivery methodologies before phage therapy can be implemented as part of standard clinical care.
Phages and Antimicrobials: Safety and the Partnership Potential
Safety and side effects of phage therapy remain the greatest concerns in the translation to clinical care. To date, there have been no known adverse effects or mild effects that failed to resolve by the end of the treatment reported from the application of phages (Vandenheuvel et al., 2015; Supplementary Table 1). Although there have been clinical trials that had been terminated, this was due to a lack of significant improvement (Sarker et al., 2016) or insufficient efficacy (Jault et al., 2019). While animal models do not fully mimic the human’s immunological response, Trend et al. (2018) have demonstrated that the application of P. aeruginosa-specific phages on primary AECs did not elicit an immunological response (Trend et al., 2018). Furthermore, although Żaczek and colleagues reported that anti-staphylococcal phages applied orally or locally did induce a humoral response in some patients, there was no increase in inflammatory markers or reduction in effectiveness of phages (Żaczek et al., 2016). Safety and tolerability have also been demonstrated by two recent studies using phage therapy to treat S. aureus chronic rhinosinusitis (Ooi et al., 2019) and severe sepsis (Petrovic Fabijan et al., 2020b), respectively. In cases of phage therapy directed against P. aeruginosa respiratory infections, no adverse phage therapy-related effects have yet been identified (Aslam et al., 2019; Law et al., 2019). Nevertheless, interpretation of potential safety and side effects of phage therapy has been limited by the lack of published results.
Currently, approval for phage therapy in CF has been granted on compassionate grounds. However, translation of phage therapy to standard clinical care would most likely target a larger population of patients infected with antibiotic susceptible pathogens. Those on prophylaxis and prolonged treatment regimes would benefit the most since P. aeruginosa isolated from early CF lungs of children have been found to be more similar to environmental strains and susceptible to antimicrobial treatments (Burns et al., 2001; Jelsbak et al., 2007; Marvig et al., 2015). A combination of phage therapy may act synergistically with antimicrobials to potentiate the reduction or delay of MDR infection occurrence (Knezevic and Aleksic Sabo, 2019; Petrovic Fabijan et al., 2020a). Phage therapy in combination with suboptimal concentrations of antibiotics has shown potential in eradicating infection more efficiently, lowering the risk of adverse effects from long-term usage of antibiotics (Kirby, 2012; Knezevic et al., 2013; Kamal and Dennis, 2015). A study by Chan et al. (2016) demonstrated that the application of φOMKO1 was able to restore antibiotic sensitivity in P. aeruginosa, which reinforces the potential of phages against drug-resistance (Chan et al., 2016). A further benefit of joint use of antibiotics and phage therapy may be a reduction in the development of bacterial resistance to phages, as even when phage mixtures (cocktails) are employed (Wright et al., 2019), gain of phage resistance can occur throughout the length of therapy (Schooley et al., 2017). Further examples of this approach have recently been reviewed by Tagliaferri and colleagues (Tagliaferri et al., 2019). The potential of phage therapy when used in combination with antimicrobials is yet to be fully exploited and future detailed exploration in this area is warranted.
Phage Therapy: Future Prospects
The future of phage therapy is not necessarily to replace current therapies, rather there is potential for clinical applications to supplement and provide an alternative treatment for infections. With a predicted shift into personalized phage therapy in the immediate future, research in this area is likely to grow at an exponential rate (Pirnay, 2020). However, the full potential of phage therapy can only be achieved when there is transparency and a willingness to share knowledge as well as resources. Ideally, phage libraries should be freely available through a network of collaboration, and information on preparation and delivery methods for phages meant for clinical usage should be well documented. Phage formulation and delivery are also critical considerations in order to direct activity to targeted areas and maximize efficacy. In fact, use of phage therapy already appears to be coordinated in various countries according to national regulations, and by major public health institutes such as Therapeutic Goods Administration (TGA) (Australia) (Donovan, 2017; Lin et al., 2019), Food and Drug Authority (FDA) (United States of America) (Jarow et al., 2017; Puthumana et al., 2018) and the European Medicines Agency (EMA) (Europe) (Balasubramanian et al., 2016; Debarbieux et al., 2016). Importantly, a universal code of ethics should be established and regulatory bodies reach a consensus on the exchange of information, usage of phages as treatment and reporting of treatment outcomes (Furfaro et al., 2018; Borysowski et al., 2019). Due to the critical nature of the rise of MDR, increasing the urgency for phage therapy to be implemented as standard care, alternative therapies to be translated into clinical applications need to be expedited. A concerted effort with both local and global partners could see phage therapy being translated into standard care in the next 5 years.
Author Contributions
RN and AK conceived the review topic and focus. RN conducted literature review and drafted the manuscript. AK, AT, BC, and SS contributed to the structure and content, provided critical review and approved the final version to be published. All authors contributed to the article and approved the submitted version.
Funding
RN is supported the Australian Government Research Training Program Scholarship, The University of Western Australia & Graduate Women (WA) Research Scholarship, CFWA Golf Classic Scholarship and Wesfarmers Center for Vaccines and Infectious Diseases Ph.D. Top Up Scholarship. AK is a Rothwell Family Fellow. SS is a NHMRC Practitioner-Fellow.
Conflict of Interest
The authors declare that the research was conducted in the absence of any commercial or financial relationships that could be construed as a potential conflict of interest.
Acknowledgments
The authors thank the subjects and families for their generous contributions to the AREST CF program. The full membership of the Australian Respiratory Early Surveillance Team for Cystic Fibrosis (AREST CF) is available at www.arestcf.org.
Supplementary Material
The Supplementary Material for this article can be found online at: https://www.frontiersin.org/articles/10.3389/fmicb.2020.593988/full#supplementary-material
References
Agarwal, R., Johnson, C. T., Imhoff, B. R., Donlan, R. M., McCarty, N. A., and García, A. J. (2018). Inhaled bacteriophage-loaded polymeric microparticles ameliorate acute lung infections. Nat. Biomed. Eng. 2, 841–849. doi: 10.1038/s41551-018-0263-5
Alemayehu, D., Casey, P. G., McAuliffe, O., Guinane, C. M., Martin, J. G., Shanahan, F., et al. (2012). Bacteriophages φMR299-2 and φNH-4 can eliminate Pseudomonas aeruginosa in the murine lung and on cystic fibrosis lung airway cells. MBio 3:e00029-12. doi: 10.1128/mBio.00029-12
Aslam, S., Courtwright, A. M., Koval, C., Lehman, S. M., Morales, S., Furr, C. L. L., et al. (2019). Early clinical experience of bacteriophage therapy in 3 lung transplant recipients. Am. J. Transplant. 19, 2631–2639. doi: 10.1111/ajt.15503
Balasubramanian, G., Morampudi, S., Chhabra, P., Gowda, A., and Zomorodi, B. (2016). An overview of compassionate use programs in the European union member states. Intractable Rare Dis. Res. 5, 244–254. doi: 10.5582/irdr.2016.01054
Baumann, U., Stocklossa, C., Greiner, W., von der Schulenburg, J. M. G., and von der Hardt, H. (2003). Cost of care and clinical condition in paediatric cystic fibrosis patients. J. Cyst. Fibros. 2, 84–90. doi: 10.1016/S1569-1993(03)00024-9
Bayes, H. K., Ritchie, N., Irvine, S., and Evans, T. J. (2016). A murine model of early Pseudomonas aeruginosa lung disease with transition to chronic infection. Sci. Rep. 6:35838. doi: 10.1038/srep35838
Bernut, A., Dupont, C., Ogryzko, N. V., Neyret, A., Herrmann, J.-L., Floto, R. A., et al. (2019). CFTR protects against Mycobacterium abscessus infection by fine-tuning host oxidative defenses. Cell Rep. 26, 1828–1840.e4. doi: 10.1016/j.celrep.2019.01.071
Birket, S. E., Davis, J. M., Fernandez, C. M., Tuggle, K. L., Oden, A. M., Chu, K. K., et al. (2018). Development of an airway mucus defect in the cystic fibrosis rat. JCI Insight 3:e97199. doi: 10.1172/jci.insight.97199
Bjarnsholt, T., Jensen, P. Ø, Fiandaca, M. J., Pedersen, J., Hansen, C. R., Andersen, C. B., et al. (2009). Pseudomonas aeruginosa biofilms in the respiratory tract of cystic fibrosis patients. Pediatr. Pulmonol. 44, 547–558. doi: 10.1002/ppul.21011
Borysowski, J., Ehni, H., and Górski, A. (2019). Ethics codes and use of new and innovative drugs. Br. J. Clin. Pharmacol. 85, 501–507. doi: 10.1111/bcp.13833
Brackman, G., Cos, P., Maes, L., Nelis, H. J., and Coenye, T. (2011). Quorum sensing inhibitors increase the susceptibility of bacterial biofilms to antibiotics in vitro and in vivo. Antimicrob. Agents Chemother. 55, 2655–2661. doi: 10.1128/AAC.00045-11
Bragonzi, A., Paroni, M., Nonis, A., Cramer, N., Montanari, S., Rejman, J., et al. (2009). Pseudomonas aeruginosa microevolution during cystic fibrosis lung infection establishes clones with adapted virulence. Am. J. Respir. Crit. Care Med. 180, 138–145. doi: 10.1164/rccm.200812-1943OC
Bragonzi, A., Worlitzsch, D., Pier, G. B., Timpert, P., Ulrich, M., Hentzer, M., et al. (2005). Nonmucoid Pseudomonas aeruginosa expresses alginate in the lungs of patients with cystic fibrosis and in a mouse model. J. Infect. Dis. 192, 410–419. doi: 10.1086/431516
Buckley, A. G., Looi, K., Iosifidis, T., Ling, K.-M., Sutanto, E. N., Martinovich, K. M., et al. (2018). Visualisation of multiple tight junctional complexes in human airway epithelial cells. Biol. Proced. Online 20:3. doi: 10.1186/s12575-018-0070-0
Burns, J. L., Gibson, R. L., McNamara, S., Yim, D., Emerson, J., Rosenfeld, M., et al. (2001). Longitudinal assessment of Pseudomonas aeruginosa in young children with cystic fibrosis. J. Infect. Dis. 183, 444–452. doi: 10.1086/318075
Cafora, M., Deflorian, G., Forti, F., Ferrari, L., Binelli, G., Briani, F., et al. (2019). Phage therapy against Pseudomonas aeruginosa infections in a cystic fibrosis zebrafish model. Sci. Rep. 9:1527. doi: 10.1038/s41598-018-37636-x
Carrigy, N. B., Chang, R. Y., Leung, S. S. Y., Harrison, M., Petrova, Z., Pope, W. H., et al. (2017). Anti-tuberculosis bacteriophage D29 delivery with a vibrating mesh nebulizer, jet nebulizer, and soft mist inhaler. Pharm. Res. 34, 2084–2096. doi: 10.1007/s11095-017-2213-4
Chan, B. K., Sistrom, M., Wertz, J. E., Kortright, K. E., Narayan, D., and Turner, P. E. (2016). Phage selection restores antibiotic sensitivity in MDR Pseudomonas aeruginosa. Sci. Rep. 6:26717. doi: 10.1038/srep26717
Chan, B. K., Turner, P. E., Kim, S., Mojibian, H. R., Elefteriades, J. A., and Narayan, D. (2018). Phage treatment of an aortic graft infected with Pseudomonas aeruginosa. Evol. Med. Public Heal. 2018, 60–66. doi: 10.1093/emph/eoy005
Chang, H.-C., Chen, C.-R., Lin, J.-W., Shen, G.-H., Chang, K.-M., Tseng, Y.-H., et al. (2005). Isolation and characterization of novel giant Stenotrophomonas maltophilia phage φSMA5. Appl. Environ. Microbiol. 71, 1387–1393. doi: 10.1128/AEM.71.3.1387-1393.2005
Chang, R. Y., Wong, J., Mathai, A., Morales, S., Kutter, E., Britton, W., et al. (2017). Production of highly stable spray dried phage formulations for treatment of Pseudomonas aeruginosa lung infection. Eur. J. Pharm. Biopharm. 121, 1–13. doi: 10.1016/j.ejpb.2017.09.002
Chang, R. Y. K., Kwok, P. C. L., Khanal, D., Morales, S., Kutter, E., Li, J., et al. (2020). Inhalable bacteriophage powders: glass transition temperature and bioactivity stabilization. Bioeng. Transl. Med. 5:e10159. doi: 10.1002/btm2.10159
Chanishvili, N. (2012). “Phage therapy—history from twort and d’Herelle through soviet experience to current approaches,” in Advances in Virus Research, Vol. 83, eds M. Ł́obocka and W. Szybalski (Amsterdam: Elsevier), 3–40. doi: 10.1016/B978-0-12-394438-2.00001-3
Chanishvili, N. (2016). Bacteriophages as therapeutic and prophylactic means: summary of the soviet and post soviet experiences. Curr. Drug Deliv. 13, 309–323. doi: 10.2174/156720181303160520193946
Choi, J., Iich, E., and Lee, J.-H. (2016). Organogenesis of adult lung in a dish: differentiation, disease and therapy. Dev. Biol. 420, 278–286. doi: 10.1016/j.ydbio.2016.10.002
Cigana, C., Lorè, N. I., Riva, C., De Fino, I., Spagnuolo, L., Sipione, B., et al. (2016). Tracking the immunopathological response to Pseudomonas aeruginosa during respiratory infections. Sci. Rep. 6:21465. doi: 10.1038/srep21465
Cigana, C., Ranucci, S., Rossi, A., De Fino, I., Melessike, M., and Bragonzi, A. (2020). Antibiotic efficacy varies based on the infection model and treatment regimen for Pseudomonas aeruginosa. Eur. Respir. J. 55:1802456. doi: 10.1183/13993003.02456-2018
Clokie, M. R. J., Millard, A. D., Letarov, A. V., and Heaphy, S. (2011). Phages in nature. Bacteriophage 1, 31–45. doi: 10.4161/bact.1.1.14942
Coleman, F. T., Mueschenborn, S., Meluleni, G., Ray, C., Carey, V. J., Vargas, S. O., et al. (2003). Hypersusceptibility of cystic fibrosis mice to chronic Pseudomonas aeruginosa oropharyngeal colonization and lung infection. Proc. Natl. Acad. Sci. U.S.A. 100, 1949–1954. doi: 10.1073/pnas.0437901100
Colledge, W. H., Abella, B. S., Southern, K. W., Ratcliff, R., Jiang, C., Cheng, S. H., et al. (1995). Generation and characterization of a ΔF508 cystic fibrosis mouse model. Nat. Genet. 10, 445–452. doi: 10.1038/ng0895-445
Cooper, D. K., Ezzelarab, M., Hara, H., and Ayares, D. (2008). Recent advances in pig-to-human organ and cell transplantation. Expert Opin. Biol. Ther. 8, 1–4. doi: 10.1517/14712598.8.1.1
Crawford, I., Maloney, P. C., Zeitlin, P. L., Guggino, W. B., Hyde, S. C., Turley, H., et al. (1991). Immunocytochemical localization of the cystic fibrosis gene product CFTR. Proc. Natl. Acad. Sci. U.S.A. 88, 9262–9266. doi: 10.1073/pnas.88.20.9262
Crusz, S. A., Popat, R., Rybtke, M. T., Cámara, M., Givskov, M., Tolker-Nielsen, T., et al. (2012). Bursting the bubble on bacterial biofilms: a flow cell methodology. Biofouling 28, 835–842. doi: 10.1080/08927014.2012.716044
Crystal, R. G., Randell, S. H., Engelhardt, J. F., Voynow, J., and Sunday, M. E. (2008). Airway epithelial cells: current concepts and challenges. Proc. Am. Thorac. Soc. 5, 772–777. doi: 10.1513/pats.200805-041HR
Cystic Fibrosis Foundation (2019). 2018 Patient Registry Annual Data Report. Bethesda, MD: Cystic Fibrosis Foundation.
Darnell, M. E. R., Plant, E. P., Watanabe, H., Byrum, R., St. Claire, M., Ward, J. M., et al. (2007). Severe acute respiratory syndrome coronavirus infection in vaccinated ferrets. J. Infect. Dis. 196, 1329–1338. doi: 10.1086/522431
de la Fuente-Núñez, C., Reffuveille, F., Mansour, S. C., Reckseidler-Zenteno, S. L., Hernández, D., Brackman, G., et al. (2015). D-enantiomeric peptides that eradicate wild-type and multidrug-resistant biofilms and protect against lethal Pseudomonas aeruginosa infections. Chem. Biol. 22, 196–205. doi: 10.1016/j.chembiol.2015.01.002
Debarbieux, L., Pirnay, J.-P., Verbeken, G., De Vos, D., Merabishvili, M., Huys, I., et al. (2016). A bacteriophage journey at the European Medicines Agency. FEMS Microbiol. Lett. 363:fnv225. doi: 10.1093/femsle/fnv225
Dedrick, R. M., Guerrero-Bustamante, C. A., Garlena, R. A., Russell, D. A., Ford, K., Harris, K., et al. (2019). Engineered bacteriophages for treatment of a patient with a disseminated drug-resistant Mycobacterium abscessus. Nat. Med. 25, 730–733. doi: 10.1038/s41591-019-0437-z
d’Herelle, F. (1917). Sur un microbe invisible antagoniste des bacteries dysenteriques. Compt. Rend. Acad. Sci. 165, 373–375.
d’Herelle, F. (1931). Bacteriophage as a treatment in acute medical and surgical infections. Bull. N. Y. Acad. Med. 7, 329–348.
Donovan, P. (2017). Access to unregistered drugs in Australia. Aust. Prescr. 40, 194–196. doi: 10.18773/austprescr.2017.062
Emerson, J., McNamara, S., Buccat, A. M., Worrell, K., and Burns, J. L. (2010). Changes in cystic fibrosis sputum microbiology in the United States between 1995 and 2008. Pediatr. Pulmonol. 45, 363–370. doi: 10.1002/ppul.21198
Engelhardt, J. F., Yankaskas, J. R., Ernst, S. A., Yang, Y., Marino, C. R., Boucher, R. C., et al. (1992). Submucosal glands are the predominant site of CFTR expression in the human bronchus. Nat. Genet. 2, 240–248. doi: 10.1038/ng1192-240
Esther, C. R., Esserman, D. A., Gilligan, P., Kerr, A., and Noone, P. G. (2010). Chronic Mycobacterium abscessus infection and lung function decline in cystic fibrosis. J. Cyst. Fibros. 9, 117–123. doi: 10.1016/j.jcf.2009.12.001
Facchini, M., De Fino, I., Riva, C., and Bragonzi, A. (2014). Long term chronic Pseudomonas aeruginosa airway infection in mice. J. Vis. Exp. 85:51019. doi: 10.3791/51019
Feigman, M. S., Kim, S., Pidgeon, S. E., Yu, Y., Ongwae, G. M., Patel, D. S., et al. (2018). Synthetic immunotherapeutics against gram-negative pathogens. Cell Chem. Biol. 25, 1185–1194.e5. doi: 10.1016/j.chembiol.2018.05.019
Fennelly, K. P., Ojano-Dirain, C., Yang, Q., Liu, L., Lu, L., Progulske-Fox, A., et al. (2016). Biofilm formation by Mycobacterium abscessus in a lung cavity. Am. J. Respir. Crit. Care Med. 193, 692–693. doi: 10.1164/rccm.201508-1586IM
Flemming, H.-C., and Wingender, J. (2010). The biofilm matrix. Nat. Rev. Microbiol. 8, 623–633. doi: 10.1038/nrmicro2415
Flemming, H.-C., Wingender, J., Szewzyk, U., Steinberg, P., Rice, S. A., and Kjelleberg, S. (2016). Biofilms: an emergent form of bacterial life. Nat. Rev. Microbiol. 14, 563–575. doi: 10.1038/nrmicro.2016.94
Floto, R. A., Olivier, K. N., Saiman, L., Daley, C. L., Herrmann, J.-L., Nick, J. A., et al. (2016). US Cystic Fibrosis Foundation and European Cystic Fibrosis Society consensus recommendations for the management of non-tuberculous mycobacteria in individuals with cystic fibrosis. Thorax 71, i1–i22. doi: 10.1136/thoraxjnl-2015-207360
Fothergill, J. L., Walshaw, M. J., and Winstanley, C. (2012). Transmissible strains of Pseudomonas aeruginosa in cystic fibrosis lung infections. Eur. Respir. J. 40, 227–238. doi: 10.1183/09031936.00204411
Furfaro, L. L., Payne, M. S., and Chang, B. J. (2018). Bacteriophage therapy: clinical trials and regulatory hurdles. Front. Cell. Infect. Microbiol. 8:376. doi: 10.3389/fcimb.2018.00376
Gajdács, M., and Urbán, E. (2019). Prevalence and antibiotic resistance of Stenotrophomonas maltophilia in respiratory tract samples: a 10-year epidemiological snapshot. Heal. Serv. Res. Manag. Epidemiol. 6:233339281987077. doi: 10.1177/2333392819870774
Gao, Z., Chen, Y., and Guan, M.-X. (2017). Mitochondrial DNA mutations associated with aminoglycoside induced ototoxicity. J. Otol. 12, 1–8. doi: 10.1016/j.joto.2017.02.001
Garratt, L. W., Kicic, A., Robertson, C., Ranganathan, S., Sly, P. D., and Stick, S. M. (2017). The AREST CF experience in biobanking — More than just tissues, tubes and time. J. Cyst. Fibros. 16, 622–627. doi: 10.1016/j.jcf.2017.08.003
Geller, B. L., Marshall-Batty, K., Schnell, F. J., McKnight, M. M., Iversen, P. L., and Greenberg, D. E. (2013). Gene-silencing antisense oligomers inhibit acinetobacter growth in vitro and in vivo. J. Infect. Dis. 208, 1553–1560. doi: 10.1093/infdis/jit460
Górski, A., Międzybrodzki, R., Ł́obocka, M., Głowacka-Rutkowska, A., Bednarek, A., Borysowski, J., et al. (2018). Phage therapy: what have we learned? Viruses 10:288. doi: 10.3390/v10060288
Grigoryan, B., Paulsen, S. J., Corbett, D. C., Sazer, D. W., Fortin, C. L., Zaita, A. J., et al. (2019). Multivascular networks and functional intravascular topologies within biocompatible hydrogels. Science 364, 458–464. doi: 10.1126/science.aav9750
Gröschel, M. I., Meehan, C. J., Barilar, I., Diricks, M., Gonzaga, A., Steglich, M., et al. (2020). The phylogenetic landscape and nosocomial spread of the multidrug-resistant opportunist Stenotrophomonas maltophilia. Nat. Commun. 11:2044. doi: 10.1038/s41467-020-15123-0
Grubb, B. R., and Boucher, R. C. (1999). Pathophysiology of gene-targeted mouse models for cystic fibrosis. Physiol. Rev. 79, S193–S214. doi: 10.1152/physrev.1999.79.1.S193
Gruenert, D. C., Willems, M., Cassiman, J. J., and Frizzell, R. A. (2004). Established cell lines used in cystic fibrosis research. J. Cyst. Fibros. 3, 191–196. doi: 10.1016/j.jcf.2004.05.040
Haagensen, J. A. J., Verotta, D., Huang, L., Spormann, A., and Yang, K. (2015). New in vitro model to study the effect of human simulated antibiotic concentrations on bacterial biofilms. Antimicrob. Agents Chemother. 59, 4074–4081. doi: 10.1128/AAC.05037-14
Haq, I. J., Gray, M. A., Garnett, J. P., Ward, C., and Brodlie, M. (2016). Airway surface liquid homeostasis in cystic fibrosis: pathophysiology and therapeutic targets. Thorax 71, 284–287. doi: 10.1136/thoraxjnl-2015-207588
Hermanns, M. I., Unger, R. E., Kehe, K., Peters, K., and Kirkpatrick, C. J. (2004). Lung epithelial cell lines in coculture with human pulmonary microvascular endothelial cells: development of an alveolo-capillary barrier in vitro. Lab. Investig. 84, 736–752. doi: 10.1038/labinvest.3700081
Hikke Van Doorninck, J., French, P. J., Verbeek, E., Peters, R. H. P. C., Morreau1, H., Bijman, J., et al. (1995). A mouse model for the cystic fibrosis AF508 mutation. EMBO J. 14, 4403–4411.
Hoffmann, N., Rasmussen, T. B., Jensen, P., Stub, C., Hentzer, M., Molin, S., et al. (2005). Novel mouse model of chronic Pseudomonas aeruginosa lung infection mimicking cystic fibrosis. Infect. Immun. 73, 2504–2514. doi: 10.1128/IAI.73.4.2504-2514.2005
Jarow, J. P., Lurie, P., Ikenberry, S. C., and Lemery, S. (2017). Overview of FDA’s expanded access program for investigational drugs. Ther. Innov. Regul. Sci. 51, 177–179. doi: 10.1177/2168479017694850
Jault, P., Leclerc, T., Jennes, S., Pirnay, J. P., Que, Y. A., Resch, G., et al. (2019). Efficacy and tolerability of a cocktail of bacteriophages to treat burn wounds infected by Pseudomonas aeruginosa (PhagoBurn): a randomised, controlled, double-blind phase 1/2 trial. Lancet Infect. Dis. 19, 35–45. doi: 10.1016/S1473-3099(18)30482-1
Jayaraman, S., Joo, N. S., Reitz, B., Wine, J. J., and Verkman, A. S. (2001). Submucosal gland secretions in airways from cystic fibrosis patients have normal [Na+] and pH but elevated viscosity. Proc. Natl. Acad. Sci. U.S.A. 98, 8119–8123. doi: 10.1073/pnas.131087598
Jelsbak, L., Johansen, H. K., Frost, A.-L., Thøgersen, R., Thomsen, L. E., Ciofu, O., et al. (2007). Molecular epidemiology and dynamics of Pseudomonas aeruginosa populations in lungs of cystic fibrosis patients. Infect. Immun. 75, 2214–2224. doi: 10.1128/IAI.01282-06
Jeukens, J., Freschi, L., Vincent, A. T., Emond-Rheault, J.-G., Kukavica-Ibrulj, I., Charette, S. J., et al. (2017). A pan-genomic approach to understand the basis of host adaptation in achromobacter. Genome Biol. Evol. 9, 1030–1046. doi: 10.1093/gbe/evx061
Johansen, H. K., Moskowitz, S. M., Ciofu, O., Pressler, T., and Høiby, N. (2008). Spread of colistin resistant non-mucoid Pseudomonas aeruginosa among chronically infected Danish cystic fibrosis patients. J. Cyst. Fibros. 7, 391–397. doi: 10.1016/j.jcf.2008.02.003
Kalghatgi, S., Spina, C. S., Costello, J. C., Liesa, M., Morones-Ramirez, J. R., Slomovic, S., et al. (2013). Bactericidal antibiotics induce mitochondrial dysfunction and oxidative damage in mammalian cells. Sci. Transl. Med. 5:192ra85. doi: 10.1126/scitranslmed.3006055
Kalish, L. A., Waltz, D. A., Dovey, M., Potter-Bynoe, G., McAdam, A. J., LiPuma, J. J., et al. (2006). Impact of Burkholderia dolosa on lung function and survival in cystic fibrosis. Am. J. Respir. Crit. Care Med. 173, 421–425. doi: 10.1164/rccm.200503-344OC
Kamal, F., and Dennis, J. J. (2015). Burkholderia cepacia complex phage-antibiotic synergy (PAS): antibiotics stimulate lytic phage activity. Appl. Environ. Microbiol. 81, 1132–1138. doi: 10.1128/AEM.02850-14
Kidd, T. J., Canton, R., Ekkelenkamp, M., Johansen, H. K., Gilligan, P., LiPuma, J. J., et al. (2018). Defining antimicrobial resistance in cystic fibrosis. J. Cyst. Fibros. 17, 696–704. doi: 10.1016/j.jcf.2018.08.014
Kidd, T. J., Ramsay, K. A., Hu, H., Marks, G. B., Wainwright, C. E., Bye, P. T., et al. (2013). Shared Pseudomonas aeruginosa genotypes are common in Australian cystic fibrosis centres. Eur. Respir. J. 41, 1091–1100. doi: 10.1183/09031936.00060512
Kirby, A. E. (2012). Synergistic action of gentamicin and bacteriophage in a continuous culture population of Staphylococcus aureus. PLoS One 7:e51017. doi: 10.1371/journal.pone.0051017
Knezevic, P., and Aleksic Sabo, V. (2019). “Combining bacteriophages with other antibacterial agents to combat bacteria,” in Phage Therapy: A Practical Approach, eds A. Górski, R. Międzybrodzki, and J. Borysowski (Cham: Springer International Publishing), 257–293. doi: 10.1007/978-3-030-26736-0_10
Knezevic, P., Curcin, S., Aleksic, V., Petrusic, M., and Vlaski, L. (2013). Phage-antibiotic synergism: a possible approach to combatting Pseudomonas aeruginosa. Res. Microbiol. 164, 55–60. doi: 10.1016/j.resmic.2012.08.008
Kukavica-Ibrulj, I., Facchini, M., Cigana, C., Levesque, R. C., and Bragonzi, A. (2014). Assessing Pseudomonas aeruginosa virulence and the host response using Murine models of acute and chronic lung infection. Methods Mol. Biol. 1149, 757–771. doi: 10.1007/978-1-4939-0473-0_58
Kukavica-Ibrulj, I., and Levesque, R. C. (2015). “Essential genes in the infection model of Pseudomonas aeruginosa-PCR-based signature-tagged mutagenesis,” in Methods in Molecular Biology, ed. L. Lu (New York, NY: Humana Press Inc.), 97–123. doi: 10.1007/978-1-4939-2398-4_7
Kutateladze, M. (2015). Experience of the Eliava institute in bacteriophage therapy. Virol. Sin. 30, 80–81. doi: 10.1007/s12250-014-3557-0
Kutateladze, M., and Adamia, R. (2008). Phage therapy experience at the Eliava Institute. Med. Mal. Infect. 38, 426–430. doi: 10.1016/j.medmal.2008.06.023
Längin, M., Mayr, T., Reichart, B., Michel, S., Buchholz, S., Guethoff, S., et al. (2018). Consistent success in life-supporting porcine cardiac xenotransplantation. Nature 564, 430–433. doi: 10.1038/s41586-018-0765-z
Law, N., Logan, C., Yung, G., Furr, C.-L. L., Lehman, S. M., Morales, S., et al. (2019). Successful adjunctive use of bacteriophage therapy for treatment of multidrug-resistant Pseudomonas aeruginosa infection in a cystic fibrosis patient. Infection 47, 665–668. doi: 10.1007/s15010-019-01319-0
Levison, M. E., and Levison, J. H. (2009). Pharmacokinetics and pharmacodynamics of antibacterial agents. Infect. Dis. Clin. North Am. 23, 791–815. doi: 10.1016/j.idc.2009.06.008
Lin, R. C. Y., Fabijan, A., Attwood, L., and Iredell, J. (2019). State of the regulatory affair: regulation of phage therapy in Australia. Capsid Tail 40
Looi, K., Buckley, A. G., Rigby, P. J., Garratt, L. W., Iosifidis, T., Zosky, G. R., et al. (2018). Effects of human rhinovirus on epithelial barrier integrity and function in children with asthma. Clin. Exp. Allergy 48, 513–524. doi: 10.1111/cea.13097
Looi, K., Troy, N. M., Garratt, L. W., Iosifidis, T., Bosco, A., Buckley, A. G., et al. (2016). Effect of human rhinovirus infection on airway epithelium tight junction protein disassembly and transepithelial permeability. Exp. Lung Res. 42, 380–395. doi: 10.1080/01902148.2016.1235237
Lopes-Pacheco, M. (2016). CFTR modulators: shedding light on precision medicine for cystic fibrosis. Front. Pharmacol. 7:275. doi: 10.3389/fphar.2016.00275
Lucey, B. P., Nelson-Rees, W. A., and Hutchins, G. M. (2009). Henrietta lacks, HeLa cells, and cell culture contamination. Arch. Pathol. Lab. Med. 133, 1463–1467. doi: 10.1043/1543-2165-133.9.1463
Malik, D. J., Sokolov, I. J., Vinner, G. K., Mancuso, F., Cinquerrui, S., Vladisavljevic, G. T., et al. (2017). Formulation, stabilisation and encapsulation of bacteriophage for phage therapy. Adv. Colloid Interface Sci. 249, 100–133. doi: 10.1016/j.cis.2017.05.014
Martinovich, K. M., Iosifidis, T., Buckley, A. G., Looi, K., Ling, K.-M., Sutanto, E. N., et al. (2017). Conditionally reprogrammed primary airway epithelial cells maintain morphology, lineage and disease specific functional characteristics. Sci. Rep. 7:17971. doi: 10.1038/s41598-017-17952-4
Marvig, R. L., Sommer, L. M., Molin, S., and Johansen, H. K. (2015). Convergent evolution and adaptation of Pseudomonas aeruginosa within patients with cystic fibrosis. Nat. Genet. 47, 57–64. doi: 10.1038/ng.3148
Meyerholz, D. K., Stoltz, D. A., Namati, E., Ramachandran, S., Pezzulo, A. A., Smith, A. R., et al. (2010). Loss of cystic fibrosis transmembrane conductance regulator function produces abnormalities in tracheal development in neonatal pigs and young children. Am. J. Respir. Crit. Care Med. 182, 1251–1261. doi: 10.1164/rccm.201004-0643OC
Millar, F. A., Simmonds, N. J., and Hodson, M. E. (2009). Trends in pathogens colonising the respiratory tract of adult patients with cystic fibrosis, 1985–2005. J. Cyst. Fibros. 8, 386–391. doi: 10.1016/j.jcf.2009.08.003
Moreau-Marquis, S., O’Toole, G. A., and Stanton, B. A. (2009). Tobramycin and FDA-approved iron chelators eliminate Pseudomonas aeruginosa biofilms on cystic fibrosis cells. Am. J. Respir. Cell Mol. Biol. 41, 305–313. doi: 10.1165/rcmb.2008-0299OC
Moreau-Marquis, S., Stanton, B. A., and O’Toole, G. A. (2008). Pseudomonas aeruginosa biofilm formation in the cystic fibrosis airway. Pulm. Pharmacol. Ther. 21, 595–599. doi: 10.1016/j.pupt.2007.12.001
Morissette, C., Skamene, E., and Gervais, F. (1995). Endobronchial inflammation following Pseudomonas aeruginosa infection in resistant and susceptible strains of mice. Infect. Immun. 63, 1718–1724. doi: 10.1128/iai.63.5.1718-1724.1995
Müh, U., Schuster, M., Heim, R., Singh, A., Olson, E. R., and Greenberg, E. P. (2006). Novel Pseudomonas aeruginosa quorum-sensing inhibitors identified in an ultra-high-throughput screen. Antimicrob. Agents Chemother. 50, 3674–3679. doi: 10.1128/AAC.00665-06
Oliveira, V. C., Bim, F. L., Monteiro, R. M., Macedo, A. P., Santos, E. S., Silva-Lovato, C. H., et al. (2020). Identification and characterization of new bacteriophages to control multidrug-resistant Pseudomonas aeruginosa biofilm on endotracheal tubes. Front. Microbiol. 11:2401. doi: 10.3389/fmicb.2020.580779
O’Loughlin, C. T., Miller, L. C., Siryaporn, A., Drescher, K., Semmelhack, M. F., and Bassler, B. L. (2013). A quorum-sensing inhibitor blocks Pseudomonas aeruginosa virulence and biofilm formation. Proc. Natl. Acad. Sci. U.S.A. 110, 17981–17986. doi: 10.1073/pnas.1316981110
Ooi, M. L., Drilling, A. J., Morales, S., Fong, S., Moraitis, S., MacIas-Valle, L., et al. (2019). Safety and tolerability of bacteriophage therapy for chronic rhinosinusitis due to Staphylococcus aureus. JAMA Otolaryngol. Head Neck Surg. 145, 723–729. doi: 10.1001/jamaoto.2019.1191
Ostedgaard, L. S., Meyerholz, D. K., Chen, J.-H., Pezzulo, A. A., Karp, P. H., Rokhlina, T., et al. (2011). The F508 mutation causes CFTR misprocessing and cystic fibrosis-like disease in pigs. Sci. Transl. Med. 3:74ra24. doi: 10.1126/scitranslmed.3001868
Overhage, J., Campisano, A., Bains, M., Torfs, E. C. W., Rehm, B. H. A., and Hancock, R. E. W. (2008). Human host defense peptide LL-37 prevents bacterial biofilm formation. Infect. Immun. 76, 4176–4182. doi: 10.1128/IAI.00318-08
Parkins, M. D., Glezerson, B. A., Sibley, C. D., Sibley, K. A., Duong, J., Purighalla, S., et al. (2014). Twenty-five-year outbreak of Pseudomonas aeruginosa infecting individuals with cystic fibrosis: identification of the prairie epidemic strain. J. Clin. Microbiol. 52, 1127–1135. doi: 10.1128/JCM.03218-13
Parquet, M., del, C., Savage, K. A., Allan, D. S., Davidson, R. J., and Holbein, B. E. (2018). Novel iron-chelator DIBI inhibits Staphylococcus aureus growth, suppresses experimental MRSA infection in mice and enhances the activities of diverse antibiotics in vitro. Front. Microbiol. 9:1811. doi: 10.3389/fmicb.2018.01811
Peters, D. L., Lynch, K. H., Stothard, P., and Dennis, J. J. (2015). The isolation and characterization of two Stenotrophomonas maltophilia bacteriophages capable of cross-taxonomic order infectivity. BMC Genomics 16:664. doi: 10.1186/s12864-015-1848-y
Peters, D. L., Stothard, P., and Dennis, J. J. (2017). The isolation and characterization of Stenotrophomonas maltophilia T4-like bacteriophage DLP6. PLoS One 12:e0173341. doi: 10.1371/journal.pone.0173341
Petrovic Fabijan, A., Khalid, A., Maddocks, S., Ho, J., Gilbey, T., Sandaradura, I., et al. (2020a). Phage therapy for severe bacterial infections: a narrative review. Med. J. Aust. 212, 279–285. doi: 10.5694/mja2.50355
Petrovic Fabijan, A., Lin, R. C. Y., Ho, J., Maddocks, S., Ben Zakour, N. L., and Iredell, J. R. (2020b). Safety of bacteriophage therapy in severe Staphylococcus aureus infection. Nat. Microbiol. 5, 465–472. doi: 10.1038/s41564-019-0634-z
Pettit, R. S., and Fellner, C. (2014). CFTR modulators for the treatment of cystic fibrosis. P T 39, 500–511.
Pirnay, J.-P. (2020). Phage therapy in the year 2035. Front. Microbiol. 11:1171. doi: 10.3389/fmicb.2020.01171
Plopper, C. G., Mariassy, A. T., Wilson, D. W., Alley, J. L., Nishio, S. J., and Nettesheim, P. (1983). Comparison of nonciliated tracheal epithelial cells in six mammalian species: ultrastructure and population densities. Exp. Lung Res. 5, 281–294. doi: 10.3109/01902148309061521
Puthumana, J., Miller, J. E., Kim, J., and Ross, J. S. (2018). Availability of investigational medicines through the US food and drug administration’s expanded access and compassionate use programs. JAMA Netw. open 1:e180283. doi: 10.1001/jamanetworkopen.2018.0283
Qvist, T., Eickhardt, S., Kragh, K. N., Andersen, C. B., Iversen, M., Høiby, N., et al. (2015). Chronic pulmonary disease with Mycobacterium abscessus complex is a biofilm infection. Eur. Respir. J. 46, 1823–1826. doi: 10.1183/13993003.01102-2015
Qvist, T., Taylor-Robinson, D., Waldmann, E., Olesen, H. V., Hansen, C. R., Mathiesen, I. H., et al. (2016). Comparing the harmful effects of nontuberculous mycobacteria and Gram negative bacteria on lung function in patients with cystic fibrosis. J. Cyst. Fibros. 15, 380–385. doi: 10.1016/j.jcf.2015.09.007
Ramsay, K., Stockwell, R., Bell, S., and Kidd, T. (2016). Infection in cystic fibrosis: impact of the environment and climate. Expert Rev. Respir. Med. 10, 505–519. doi: 10.1586/17476348.2016.1162715
Randell, S. H., Fulcher, M. L., O’Neal, W., and Olsen, J. C. (2011). Primary epithelial cell models for cystic fibrosis research. Methods Mol. Biol. 742, 285–310. doi: 10.1007/978-1-61779-120-8_18
Razvi, S., Quittell, L., Sewall, A., Quinton, H., Marshall, B., and Saiman, L. (2009). Respiratory microbiology of patients with cystic fibrosis in the United States, 1995 to 2005. Chest 136, 1554–1560. doi: 10.1378/chest.09-0132
Ren, H., Birch, N. P., and Suresh, V. (2016). An optimised human cell culture model for alveolar epithelial transport. PLoS One 11:e0165225. doi: 10.1371/journal.pone.0165225
Rogers, C. S., Stoltz, D. A., Meyerholz, D. K., Ostedgaard, L. S., Rokhlina, T., Taft, P. J., et al. (2008). Disruption of the CFTR gene produces a model of cystic fibrosis in newborn pigs. Science 321, 1837–1841. doi: 10.1126/science.1163600
Sabatini, S., Gosetto, F., Iraci, N., Barreca, M. L., Massari, S., Sancineto, L., et al. (2013). Re-evolution of the 2-phenylquinolines: ligand-based design, synthesis, and biological evaluation of a potent new class of Staphylococcus aureus NorA efflux pump inhibitors to combat antimicrobial resistance. J. Med. Chem. 56, 4975–4989. doi: 10.1021/jm400262a
Sahota, J. S., Smith, C. M., Radhakrishnan, P., Winstanley, C., Goderdzishvili, M., Chanishvili, N., et al. (2015). Bacteriophage delivery by nebulization and efficacy against phenotypically diverse Pseudomonas aeruginosa from cystic fibrosis patients. J. Aerosol Med. Pulm. Drug Deliv. 28, 353–360. doi: 10.1089/jamp.2014.1172
Salunkhe, P., Smart, C. H. M., Morgan, J. A. W., Panagea, S., Walshaw, M. J., Hart, C. A., et al. (2005). A cystic fibrosis epidemic strain of Pseudomonas aeruginosa displays enhanced virulence and antimicrobial resistance. J. Bacteriol. 187, 4908–4920. doi: 10.1128/JB.187.14.4908-4920.2005
Sanguinetti, M., Ardito, F., Fiscarelli, E., La Sorda, M., D’Argenio, P., Ricciotti, G., et al. (2001). Fatal pulmonary infection due to multidrug-resistant mycobacterium abscessus in a patient with cystic fibrosis. J. Clin. Microbiol. 39, 816–819. doi: 10.1128/JCM.39.2.816-819.2001
Sansgiry, S. S., Joish, V. N., Boklage, S., Goyal, R. K., Chopra, P., and Sethi, S. (2012). Economic burden of Pseudomonas aeruginosa infection in patients with cystic fibrosis. J. Med. Econ. 15, 219–224. doi: 10.3111/13696998.2011.638954
Sarker, S. A., Sultana, S., Reuteler, G., Moine, D., Descombes, P., Charton, F., et al. (2016). Oral phage therapy of acute bacterial diarrhea with two coliphage preparations: a randomized trial in children from Bangladesh. EBioMedicine 4, 124–137. doi: 10.1016/j.ebiom.2015.12.023
Sawyer, A. J., Wesolowski, D., Gandotra, N., Stojadinovic, A., Izadjoo, M., Altman, S., et al. (2013). A peptide-morpholino oligomer conjugate targeting Staphylococcus aureus gyrA mRNA improves healing in an infected mouse cutaneous wound model. Int. J. Pharm. 453, 651–655. doi: 10.1016/j.ijpharm.2013.05.041
Scherer, W. F., Syverton, J. T., and Gey, G. O. (1953). Studies on the propagation in vitro of poliomyelitis viruses: IV. Viral multiplication in a stable strain of human malignant epithelial cells (strain hela) derived from an epidermoid carcinoma of the cervix. J. Exp. Med. 97, 695–710. doi: 10.1084/jem.97.5.695
Schooley, R. T., Biswas, B., Gill, J. J., Hernandez-Morales, A., Lancaster, J., Lessor, L., et al. (2017). Development and use of personalized bacteriophage-based therapeutic cocktails to treat a patient with a disseminated resistant Acinetobacter baumannii infection. Antimicrob. Agents Chemother. 61:e00954-17. doi: 10.1128/AAC.00954-17
Sehgal, A., Presente, A., and Engelhardt, J. F. (1996). Developmental expression patterns of CFTR in ferret tracheal surface airway and submucosal gland epithelia. Am. J. Respir. Cell Mol. Biol. 15, 122–131. doi: 10.1165/ajrcmb.15.1.8679216
Shrestha, J., Ghadiri, M., Shanmugavel, M., Razavi Bazaz, S., Vasilescu, S., Ding, L., et al. (2019). A rapidly prototyped lung-on-a-chip model using 3D-printed molds. Organs Chip 1:100001. doi: 10.1016/j.ooc.2020.100001
Shriram, V., Khare, T., Bhagwat, R., Shukla, R., and Kumar, V. (2018). Inhibiting bacterial drug efflux pumps via phyto-therapeutics to combat threatening antimicrobial resistance. Front. Microbiol. 9:2990. doi: 10.3389/fmicb.2018.02990
Shrivastava, S., Shrivastava, P., and Ramasamy, J. (2017). World health organization releases global priority list of antibiotic-resistant bacteria to guide research, discovery, and development of new antibiotics. J. Med. Soc. 32, 76–77. doi: 10.4103/jms.jms_25_17
Smith, D. J., Ramsay, K. A., Yerkovich, S. T., Reid, D. W., Wainwright, C. E., Grimwood, K., et al. (2016). Pseudomonas aeruginosa antibiotic resistance in Australian cystic fibrosis centres. Respirology 21, 329–337. doi: 10.1111/resp.12714
Smolich, J. J., Stratford, B. F., Maloney, J. E., and Ritchie, B. C. (1978). New features in the development of the submucosal gland of the respiratory tract. J. Anat. 127, 223–238.
Snouwaert, J. N., Brigman, K. K., Latour, A. M., Malouf, N. N., Boucher, R. C., Smithies, O., et al. (1992). An animal model for cystic fibrosis made by gene targeting. Science 257, 1083–1088. doi: 10.1126/science.257.5073.1083
Soukarieh, F., Liu, R., Romero, M., Roberston, S. N., Richardson, W., Lucanto, S., et al. (2020). Hit identification of new potent PqsR antagonists as inhibitors of quorum sensing in planktonic and biofilm grown Pseudomonas aeruginosa. Front. Chem. 8:204. doi: 10.3389/fchem.2020.00204
Stanojevic, S., Ratjen, F., Stephens, D., Lu, A., Yau, Y., Tullis, E., et al. (2013). Factors influencing the acquisition of Stenotrophomonas maltophilia infection in cystic fibrosis patients. J. Cyst. Fibros. 12, 575–583. doi: 10.1016/j.jcf.2013.05.009
Sun, X., Olivier, A. K., Liang, B., Yi, Y., Sui, H., Evans, T. I. A., et al. (2014). Lung phenotype of juvenile and adult cystic fibrosis transmembrane conductance regulator–knockout ferrets. Am. J. Respir. Cell Mol. Biol. 50, 502–512. doi: 10.1165/rcmb.2013-0261OC
Sun, X., Sui, H., Fisher, J. T., Yan, Z., Liu, X., Cho, H.-J., et al. (2010). Disease phenotype of a ferret CFTR-knockout model of cystic fibrosis. J. Clin. Invest. 120, 3149–3160. doi: 10.1172/JCI43052
Sun, X., Yan, Z., Yi, Y., Li, Z., Lei, D., Rogers, C. S., et al. (2008). Adeno-associated virus–targeted disruption of the CFTR gene in cloned ferrets. J. Clin. Invest. 118, 1578–1583. doi: 10.1172/JCI34599
Sutanto, E. N., Kicic, A., Foo, C. J., Stevens, P. T., Mullane, D., Knight, D. A., et al. (2011). Innate inflammatory responses of pediatric cystic fibrosis airway epithelial cells. Am. J. Respir. Cell Mol. Biol. 44, 761–767. doi: 10.1165/rcmb.2010-0368OC
Sykes, M., and Sachs, D. H. (2019). Transplanting organs from pigs to humans. Sci. Immunol. 4:eaau6298. doi: 10.1126/sciimmunol.aau6298
Tagliaferri, T. L., Jansen, M., and Horz, H. P. (2019). Fighting pathogenic bacteria on two fronts: phages and antibiotics as combined strategy. Front. Cell. Infect. Microbiol. 9:22. doi: 10.3389/fcimb.2019.00022
Tinoco, J. M., Buttaro, B., Zhang, H., Liss, N., Sassone, L., and Stevens, R. (2016). Effect of a genetically engineered bacteriophage on Enterococcus faecalis biofilms. Arch. Oral Biol. 71, 80–86. doi: 10.1016/j.archoralbio.2016.07.001
Tolker-Nielsen, T., and Sternberg, C. (2014). “Methods for studying biofilm formation: flow cells and confocal laser scanning microscopy,” in Pseudomonas Methods and Protocols. Methods in Molecular Biology, Vol. 1149, eds A. Filloux and J. L. Ramos (New York, NY: Humana Press), 615–629. doi: 10.1007/978-1-4939-0473-0_47
Travaglini, K. J., Nabhan, A. N., Penland, L., Sinha, R., Gillich, A., Sit, R. V., et al. (2019). A molecular cell atlas of the human lung from single cell RNA sequencing. bioRxiv [preprint] doi: 10.1101/742320bioRxiv:587
Trend, S., Chang, B. J., O’Dea, M., Stick, S. M., and Kicic, A. (2018). Use of a primary epithelial cell screening tool to investigate phage therapy in cystic fibrosis. Front. Pharmacol. 9:1330. doi: 10.3389/fphar.2018.01330
Tuggle, K. L., Birket, S. E., Cui, X., Hong, J., Warren, J., Reid, L., et al. (2014). Characterization of defects in ion transport and tissue development in cystic fibrosis transmembrane conductance regulator (CFTR)-knockout rats. PLoS One 9:e91253. doi: 10.1371/journal.pone.0091253
Twort, F. W. (1914). An investigation on the nature of ultra-microscopic viruses. Lancet 186, 1241–1243.
Vandenheuvel, D., Lavigne, R., and Brüssow, H. (2015). Bacteriophage therapy: advances in formulation strategies and human clinical trials. Annu. Rev. Virol. 2, 599–618. doi: 10.1146/annurev-virology-100114-054915
Veit, G., Avramescu, R. G., Chiang, A. N., Houck, S. A., Cai, Z., Peters, K. W., et al. (2016). From CFTR biology toward combinatorial pharmacotherapy: expanded classification of cystic fibrosis mutations. Mol. Biol. Cell 27, 424–433. doi: 10.1091/mbc.e14-04-0935
Wallin, M., Tang, P., Chang, R. Y. K., Yang, M., Finlay, W. H., and Chan, H.-K. (2019). Aerosol drug delivery to the lungs during nasal high flow therapy: an in vitro study. BMC Pulm. Med. 19:42. doi: 10.1186/s12890-019-0807-9
Wine, J. J. (2004). Submucosal glands and airway defense. Proc. Am. Thorac. Soc. 1, 47–53. doi: 10.1513/pats.2306015
Wright, R. C. T., Friman, V.-P., Smith, M. C. M., and Brockhurst, M. A. (2019). Resistance evolution against phage combinations depends on the timing and order of exposure. MBio 10:15. doi: 10.1128/mBio.01652-19
Zabner, J., Karp, P., Seiler, M., Phillips, S. L., Mitchell, C. J., Saavedra, M., et al. (2003). Development of cystic fibrosis and noncystic fibrosis airway cell lines. Am. J. Physiol. Cell. Mol. Physiol. 284, L844–L854. doi: 10.1152/ajplung.00355.2002
Żaczek, M., Ł́usiak-Szelachowska, M., Joñczyk-Matysiak, E., Weber-Dąbrowska, B., Międzybrodzki, R., Owczarek, B., et al. (2016). Antibody production in response to staphylococcal MS-1 phage cocktail in patients undergoing phage therapy. Front. Microbiol. 7:1681. doi: 10.3389/fmicb.2016.01681
Keywords: bacteriophage, cystic fibrosis, lung disease, alternative therapy, animal models, antimicrobials, biofilms, regulation
Citation: Ng RN, Tai AS, Chang BJ, Stick SM and Kicic A (2021) Overcoming Challenges to Make Bacteriophage Therapy Standard Clinical Treatment Practice for Cystic Fibrosis. Front. Microbiol. 11:593988. doi: 10.3389/fmicb.2020.593988
Received: 12 August 2020; Accepted: 08 December 2020;
Published: 11 January 2021.
Edited by:
Petar Knezevic, University of Novi Sad, SerbiaReviewed by:
Dinesh Sriramulu, Independent Researcher, Chennai, IndiaRoger C. Levesque, Laval University, Canada
Copyright © 2021 Ng, Tai, Chang, Stick and Kicic. This is an open-access article distributed under the terms of the Creative Commons Attribution License (CC BY). The use, distribution or reproduction in other forums is permitted, provided the original author(s) and the copyright owner(s) are credited and that the original publication in this journal is cited, in accordance with accepted academic practice. No use, distribution or reproduction is permitted which does not comply with these terms.
*Correspondence: Anthony Kicic, YW50aG9ueS5raWNpY0B0ZWxldGhvbmtpZHMub3JnLmF1