- 1Université de Lorraine, CNRS, LIEC, Nancy, France
- 2Université de Lorraine, INRAE, LSE, Nancy, France
Phytoextraction using hyperaccumulating plants is a method for the remediation of soils contaminated with trace elements (TEs). As a strategy for improvement, the concept of fungal-assisted phytoextraction has emerged in the last decade. However, the role played by fungal endophytes of hyperaccumulating plants in phytoextraction is poorly studied. Here, fungal endophytes isolated from calamine or non-metalliferous populations of the Cd/Zn hyperaccumulator Noccaea caerulescens were tested for their growth promotion abilities affecting the host plant. Plants were inoculated with seven different isolates and grown for 2 months in trace element (TE)-contaminated soil. The outcomes of the interactions between N. caerulescens and its native strains ranged from neutral to beneficial. Among the strains, Alternaria thlaspis and Metapochonia rubescens, respectively, isolated from the roots of a non-metallicolous and a calamine population of N. caerulescens, respectively, exhibited the most promising abilities to enhance the Zn phytoextraction potential of N. caerulescens related to a significant increase of the plant biomass. These strains significantly increased the root elemental composition, particularly in the case of K, P, and S, suggesting an improvement of the plant nutrition. Results obtained in this study provide new insights into the relevance of microbial-assisted phytoextraction approaches in the case of hyperaccumulating plants.
Introduction
Among the different phytoremediation approaches applied, phytoextraction using hyperaccumulating plants is a method for the remediation of soils contaminated with trace elements (TE) (Robinson et al., 1998; Zhao et al., 2003; McGrath et al., 2006). Among the TE targeted for phytoextraction applications, cadmium (Cd) and zinc (Zn) are of particular interest, as they are found at elevated levels in soils mainly due to anthropic activities such as mining, smelting, the use of domestic and industrial wastes or the burning of fossil fuels, causing serious environmental problems worldwide (Alloway, 2013; Su et al., 2014). Cadmium is considered one of the most toxic non-essential elements for plants (Clemens and Ma, 2016), and Zn has phytotoxic effects when present in excess in plants (Kabata-Pendias, 2010). Among the various hyperaccumulators, Noccaea caerulescens (J. & C. Presl) F. K. Mey (Brassicaceae) is one of the species with the highest ability to tolerate and accumulate Cd and Zn (Lombi et al., 2000; McGrath et al., 2006). In situ, N. caerulescens can concentrate up to 2,890 μg Cd g–1 and 53,450 μg Zn g–1 in dry shoots (Reeves et al., 2001); however, significant variations in metal accumulation depend on the populations and edaphic type (Gonneau et al., 2014; Sterckeman et al., 2017). Among all the known populations, the calamine Ganges ecotype is the most studied due to its high capacity to accumulate Cd and its tolerance to Cd and Zn (Assunção et al., 2003; Gonneau et al., 2014).
Recent studies aimed to optimize the phytoextraction efficiency of N. caerulescens by maximizing both the biomass production and metal concentration in plants. According to Escarré et al. (2013), shoot concentrations tend to be restricted to a physiological maximum, limiting the possibility of improving this factor. There is, however, high potential for improvement in the biomass factor in the case of N. caerulescens (Sterckeman et al., 2017). Various levers have been investigated to increase biomass production, such as the selection of high-growth rate cultivars (Sterckeman et al., 2017, 2019) and the development of adequate cultural practices (Maxted et al., 2007; Jacobs et al., 2018a,b; Rees et al., 2020), including the addition of biological amendments. Indeed, we previously demonstrated that several DSE strains isolated from poplar roots collected from TE-contaminated sites were able to colonize the roots of N. caerulescens, with plant responses ranging from neutral to beneficial (Yung et al., 2021b). We also identified two promising fungal strains for the fungal-assisted phytoextraction of Cd and Zn with N. caerulescens. However, the diversity of the fungal endophytome of Zn- and Cd-adapted N. caerulescens and the effect of potentially plant-growth promoting (PGP) native fungal strains on the phytoextraction potential of the plant have not yet been investigated.
Over the last decade, the concept of microbial-assisted phytoremediation (MAP) has developed. When applied to phytoextraction, it consists of inoculating metal-(hyper)accumulating plants with plant-associated microorganisms to enhance metal recovery rates (Thijs et al., 2016; Benizri et al., 2021). MAP is based on the ability of PGP microorganisms such as PGP rhizobacteria (Hansda et al., 2014), endophytic bacteria (Ma et al., 2016), arbuscular mycorrhizal fungi (Ciadamidaro et al., 2017; Miransari, 2017; Berthelot et al., 2018), ectomycorrhizal fungi (Ciadamidaro et al., 2017; Phanthavongsa et al., 2017; Gil-Martínez et al., 2018), and endophytic fungi (Lacercat-Didier et al., 2016; Berthelot et al., 2017, 2018; Deng and Cao, 2017) to enhance plant development, health, tolerance to abiotic stress and resistance to phytopathogens (Kong and Glick, 2017; Thijs et al., 2016; Otero-Blanca et al., 2018; Durand et al., 2021). Additionally, root-associated microorganisms are able to increase the mobility of TEs in the soil, suggesting that their presence is essential for plants to reach their full phytoextraction potential (Lebeau et al., 2008; Sessitsch et al., 2013). In this context, hyperaccumulating plants and associated microorganisms could be considered together as plant-microbiome superorganisms (Bosch and McFall-Ngai, 2011), in which the microbial component acts as a dynamic system, increasing the adaptability of the plant-microbiome superorganism under environmental pressure (Durand et al., 2021). Indeed, in the case of TE-enriched soils, the host plant can promote metal-tolerant beneficial microorganisms from the enormous and diverse pool of microorganisms present in the bulk soil (Becerra-Castro et al., 2012; Álvarez-López et al., 2016; Yung et al., 2021a). Detecting and isolating these preferentially selected microorganisms represents a preliminary step of MAP.
The diversity of potentially PGP fungi and bacteria associated with N. caerulescens has been poorly investigated (Visioli et al., 2015a). Bacterial endophytes of N. caerulescens were mainly studied utilizing cultivation-dependent methods (Aboudrar et al., 2007; Visioli et al., 2014). Recently, 16S DNA profiling has been used to investigate root-associated bacterial communities (Visioli et al., 2019) and endophytic bacterial communities associated with seeds (Durand et al., 2021) of N. caerulescens. Several promising strains of metal-resistant or PGP endophytic bacteria have already been isolated (Visioli et al., 2014; Fones et al., 2016; Burges et al., 2017), and their potential to improve biomass production and metal accumulation has been tested in planta (Visioli et al., 2015b; Burges et al., 2017). Like most plants belonging to the Brassicaceae family, N. caerulescens is a recognized non-mycorrhizal plant, suggesting that endophytic fungi may be the dominant group of symbiotic fungi inhabiting its roots. However, its fungal endophytome has been poorly studied thus far. The first data about the cultivable fungal diversity associated with the roots and leaves of N. caerulescens grown on Ni-enriched soils were recently reported (Ważny et al., 2021). Among the 13 taxa isolated, most of the strains showed a positive effect on the production of plant biomass and on Ni accumulation in roots and/or shoots. Additionally, Ważny et al. (2021) reported that shoots accumulated more Ni when the plants were inoculated with a strain of Phomopsis columnaris isolated from N. caerulescens, highlighting the importance of selecting indigenous strains. However, indigenous fungal strains have not been tested for their effect on Cd/Zn phytoextraction.
By examining fungal endophytes isolated from calamine or non-metalliferous populations of N. caerulescens, this study therefore aimed to (i) characterize some of their PGP abilities and their influence on the mobility of TE through in vitro tests, (ii) assess their capacity to colonize the roots of the hyperaccumulating plant N. caerulescens, (iii) study their effect on plant growth, leaf pigment contents and mineral nutrient status, and (iv) determine their impact on the accumulation and phytoextraction of Cd and Zn.
Materials and Methods
Fungal Endophyte Isolation and Identification
Isolation of fungal endophytes from the roots and leaves of 58 individuals of N. caerulescens from seven populations (around eight plants per population) collected in two regions of France (Grand Est and Occitanie) was performed, with plants from each region corresponding to a genetic subunit (Gonneau et al., 2017). The full description of the cultivable fungal endophytome of N. caerulescens will be described elsewhere (unpublished results). Once collected, plant parts were carefully washed with tap water, surface-sterilized by immersion in 30% (v/v) H2O2 for 30 s and finally rinsed three times with sterile distilled water. For each plant, 15 root segments of 1 cm and 15 leaf fragments of 1 cm2 were placed on malt extract agar (MEA, malt extract: 12 g/L and agar: 15 g/L, pH 5.5) at 24°C in the dark. Ampicillin and chloramphenicol (100 μg/ml) were also added to the medium to avoid bacterial development. After 15 days of growth, fungal mycelium growing out of the plant tissues was isolated and subcultured in new MEA plates for 3 weeks.
For this experiment, we considered seven strains that were molecularly identified. Fungal DNA was first extracted (100 μL) using a REDextract-N-AmpTM Plant PCR kit (Sigma-Aldrich, Saint-Quentin Fallavier, France) according to the manufacturer’s instructions. The internal transcribed spacer (ITS) region was then amplified using ITS1 and ITS4 primers (White et al., 1990). Twenty microliters of a mixture containing 0.1 μM of each primer, 1 μL of the fungal extract, 8.2 μl of water and 10 μl of REDExtract-N-Amp PCR ready mix was used. The following PCR program was used: 3 min denaturation at 94°C, followed by 40 cycles of 94°C for 30 s, 55°C for 30 s and 72°C for 75 s and 10 min final extension at 72°C. The DNA quality and quantity were assessed by agarose gel electrophoresis using Molecular Imager® Gel DocTM XR (Bio-Rad, United States). Fungal amplicons were then sequenced using the Sanger method (Eurofins, Germany). The obtained ITS sequences were used to retrieve similar sequences from GenBank using the NCBI BLASTn program.
Indole Acetic Acid Production
The quantities of indole acetic acid (IAA) produced by the seven strains were determined by inoculating 40 mL of potato dextrose broth (PDB, pH 5.2) supplemented or not with 0.2% (m/v) tryptophan with six discs of mycelium of 5 mm in diameter (n = 3). The fungal cultures were incubated at 24°C for 10 days with constant shaking at 150 rpm. They were subsequently centrifuged at 10,000 g for 6 min to separate the supernatant from the fungal biomass. The mycelium was dried at 60°C for 2 days and weighed using a precision balance (Mettler AE 163 analytical). One hundred μL of the supernatant was mixed with 400 μL of Salkowski’s reagent, which is a solution comprising 0.5 M FeCl3 and 35% perchloric acid (Platt and Thimann, 1956). The production of IAA was evidenced by the development of a pink color after a 30 min incubation in the dark. A semi-quantitative measure of the quantity of produced IAA was obtained by measuring the absorbance of the solutions supplemented with tryptophan at a wavelength of 530 nm, subtracting the absorbance of the solution without tryptophan and comparing it to a standard curve of IAA. The semi-quantitative concentrations of IAA were expressed per gram of mycelium (μg/g).
Zinc and Cadmium Mobilization From the Soil
The ability of the seven fungal strains to mobilize Cd and Zn from the soil was estimated using the fungal extracts from the previous culture made in PDB without tryptophan (n = 3). The supernatants were filtered at 0.2 μm, and the pH of the filtrates was measured. Four milliliters of the filtrates were rotary shaken for 2 h at room temperature with 0.8 g of sterilized and dried Cd/Zn-contaminated soil used for the inoculation assay. Controls consisted of 0.8 g aliquots of the same soil incubated with 4 ml of fresh PDB with the pH adjusted to 3.4, 3.9, or 4.2 (pH range of the fungal extracts) or without adjustment (pH 5.2, corresponding to the original pH of the medium used for the fungal cultures). After centrifugation (7,000 rpm, 5 min), filtration at 0.45 μm and acidification at 5% with HNO3, the concentrations of Zn and Cd in the filtrates were determined by inductively coupled plasma optical emission spectrometry (ICP-OES, iCap 600, Thermo Fischer Scientific, Pittsburgh, PA, United States). Cadmium and Zn concentrations were expressed per g of mycelium. The ability to mobilize Zn and Cd from contaminated soil was estimated by measuring the difference in Cd and Zn concentrations (μg/g of soil per g of mycelium) between soil mixed with fungal extract and soil mixed with pH 5.2 PDB.
Phosphate and Zinc Solubilization Activity
The ability of the seven fungal strains to solubilize phosphate and Zn was determined by inoculating the strains onto solid Pachlewski medium [2.3 g/L C4H12N2O6, 0.5 g/l KCl, 1 g/L MgSO4, 7H2O, 5 g/L maltose, 20 g/L glucose, 10 μL/L thiamine HCl (1 g/L) and 100 μL/L Kanieltra solution, pH 5.5] supplemented with 12.5 g/L Ca3(PO4)2, 3 g/L ZnO, 5.5 g/L ZnCO3 or 5.25 g/L Zn3(PO4)2 (n = 4). Agar plates were covered with a sterile cellophane membrane and inoculated with 1 cm2 agar mycelial plugs. After 7 days of culture at 24°C, the diameters of both the colonies and the solubilization halos were measured. Finally, the solubilization index was calculated as the “diameter of the solubilization halo” over the “diameter of the colony” ratio.
Siderophore Production
The siderophore production of the seven fungal strains was tested on chrome azurol S agar plates (n = 4). Fungal strains were grown on plates half-filled with Fe-free M9 medium (3 g/L C4H12N2O6, 1 g/L NH4Cl, 6 g/L Na2HPO4, 0.5 g/L NaCl, and 4 g/L glucose). After 12 days of growth at 24°C, fungal colonies were removed together with the cellophane membranes, and the plates were filled with a CAS-blue agar overlay. The overlay medium (pH 6.8) was made of (per L) chrome azurol S (CAS) 60.5 mg, hexadecyltrimetyl ammonium bromide (HDTMA) 72.9 mg, piperazine-1,4-bis(2-ethanesulfonic acid) (PIPES) 30.24 g, 20 mL of FeCl3 (10 mM) prepared in HCl (100 mM) and agarose (0.9%, w/v) (Pérez-Miranda et al., 2007). A change in color from blue to orange observed in the overlaid medium evidenced the production of siderophores. The siderophore index was calculated as the “diameter of the orange halo” over the “diameter of the colony” ratio.
Experimental Design of the Pot Experiment
A pot experiment was conducted with sandy-loamy soil taken from the top horizon of agricultural soil located at Chenevières (Grand Est, France), which was previously spiked with Cd, Pb, Cu, and Zn and used for the cultivation of N. caerulescens as part of previous studies (Sterckeman et al., 2017, 2019). At the beginning of the present study, the Cd and Zn concentrations were 3.6 and 649 mg/kg, respectively. Some physico-chemical parameters of the soil are provided in Supplementary Table 1. The seeds of N. caerulescens originated from a metallicolous population from a mining site located in Ganges [Occitanie, France, Gonneau et al. (2014)]. The seeds were sown in a sterilized mix (v/v) of compost (75%) and sand (25%) and placed in a growth chamber. Seven weeks after germination, plants of similar developmental stages were selected for the pot experiment.
In the present study, we tested seven fungal endophytic strains (DBF60, DBF79, DBF81, DBF107, DBF108, DBF129, and DBF159) originating from the collection of strains isolated from N. caerulescens. They were selected based on their potential as PGP fungi, according to their description in the literature. The production of inoculum and the soil inoculation procedure are fully described in Yung et al. (2021b). As a control treatment (CTRL), we mixed one aliquot of soil with perlite containing fungus-free agar plugs. Five replicates were considered for each fungal treatment, and four were considered for the mock-inoculated treatment. Seedlings were transplanted into 40 plastic pots (8 treatments × 5 replicates) of 500 mL volume (8.6 cm in diameter) previously loaded with 400 g of the inoculated soils. The pots were then placed in a growth chamber with 16 h of light at 22°C and 8 h of darkness at 18°C, 70% relative humidity and a photon flux density of 200 μmol photons m–2 s–1 in the PAR range. Water was supplied every 2 or 3 days to 80% of the field capacity.
Estimation of NBI and Pigment Indices
Chlorophyl, flavonoid and anthocyanin contents in leaves of N. caerulescens and the nitrogen balance index (NBI) were measured using a dual-excitation fluorimeter DUALEX® SCIENTIFIC+ (Force-A, Orsay, France). Full details about the analytical method are available in Yung et al. (2021b). These measurements were carried out on five mature leaves per plant at the end of the experiment.
Plant Harvesting, Biomass Determination and Analysis of Trace and Major Elements
After 8 weeks of growth, roots and shoots were harvested and separated. Shoots were thoroughly washed with tap water and rinsed with deionized water. Soil was removed from the roots first by washing them with tap water and then by immersing them in 37.6 mM tetrasodium diphosphate for 16 h. The plant samples were then dried at 70°C for 48 h and weighed. Dried samples (0.5 g) were ground and digested according to the protocol detailed in Yung et al. (2021b). Trace and major elements were analyzed by ICP-OES. Control samples from N. caerulescens with known compositions (according to internal analyses carried out by INRA-USRAVE, Villenave d’Ornon, France), as well as a certified solution (EU-H-2, SCP Science, Courtaboeuf, France), were included in all analyses as quality controls.
Estimation of the Rate of Fungal Colonization of Plant Roots
To estimate the colonization rate of plant roots by the inoculated endophytes, root segments were labeled with 20 μg/mL WGA-AF®488 (Invitrogen, France) (Berthelot et al., 2016) and observed by fluorescence microscopy. Labeled roots were cut into 10 mm segments (20 per plant) that were assessed separately using a microscope. The rate of root colonization (semi-quantitative) by fungal strains was estimated by assessing the frequency of microsclerotia and typical intracellular hyphae.
Statistical Analyses
Statistical analyses were performed with R software v.3.5.1 (R Development Core Team, 2015). All statistical tests were considered significant at P ≤ 0.05. The mean values of the variables obtained from the in vitro tests of the inoculated strains were compared using Tukey’s HSD or the Kruskal–Wallis post hoc test to classify the strains according to their PGP abilities and their capacity to modify the mobility of Cd and/or Zn in the soil.
A multiple-factor analysis (MFA, “FactomineR” package) was used to evaluate the influence of the seven tested strains on (i) plant shoot and root biomass production, (ii) leaf pigments and NBI, and (iii) the concentrations of trace and major elements in roots and shoots. The latter variables and the amounts of extracted Cd and Zn (concentration of Cd/Zn in leaves × biomass) were then analyzed by comparing the CTRL treatment with the seven fungal treatments using an analysis of variance (ANOVA) followed by a Dunnett test in the “multcomp” package. The adequacy of the data from each measurement with respect to the ANOVA models was verified by examining the residuals for independence (Durbin-Watson test, “car” package), normality (Shapiro test) and homogeneity (Levene test). If the latter assumptions were not validated after “sqrt” or “log” transformation of the data, a Wilcoxon pairwise test with a Holm correction of the p-value was used.
Results
Taxonomic and Functional Description of the Fungal Isolates
Among the collection of endophytic strains isolated from the parts of N. caerulescens, the seven strains studied in the present study were isolated from populations originating from two calamine stations (Ganges and Montdardier; Occitanie, France) and two non-metallicolous stations located in Croix des Moinats (Grand Est, France) and Baraquette (Occitanie, France) (Gonneau, 2014). DBF79, DBF81 and DBF129 were isolated from leaves, while DBF60, DBF107, DBF108 and DBF159 were isolated from roots. The molecular identification enabled assignment, with sequence identities of 99–100% (Table 1), of the following affiliations. DBF60 (Metapochonia rubescens), DBF81 (Trichoderma harzianum) and DBF159 (Microdochium bolleyi) belong to Sordariomycetes, DBF79 (Alternaria thlaspis), DBF107 (Cladosporium sp.), and DBF129 (Cladosporium cladosporioides) belong to Dothideomycetes, and DBF108 (Phialophora mustea) belongs to Eurotiomycetes.
The isolates were further characterized for their ability to promote plant growth through in vitro tests. To determine the ability of the isolates to assist Fe uptake by plants, siderophore production using the CAS-blue agar assay was carried out. The color change of agar from blue to orange was only observed in the case of DBF60, indicating that this strain was the only one able to produce siderophores (Table 2). The ability of the tested strains to produce IAA was determined through a colorimetric assay. The seven isolates had the ability to produce IAA with concentrations ranging from 1.0 to 4.1 μg/g DW of mycelium (Table 2). DBF81 produced the lowest IAA quantities. Tricalcium phosphate and various forms of Zn supplemented in Pachlewski medium were used to test the ability of the isolates to solubilize insoluble phosphate and Zn. All isolates had the ability to solubilize tricalcium phosphate (Table 2). DBF60, DBF81, and DBF159 were also able to solubilize Zn3(PO4)2. Moreover, DBF60 and DBF81 had the ability to solubilize ZnCO3 and ZnO (Table 2).
The ability of the isolates to mobilize metals was further tested by measuring the concentrations of Zn and Cd mobilized from contaminated soil by the filtrates of the fungal cultures. All strains had the ability to increase the mobility of Zn and Cd from the soil when compared to the control medium (pH 5.2; no fungus cultivated; P < 0.05). DBF81, DBF107, and DBF129 had the highest ability to mobilize both Zn and Cd (Table 3). Filtrates obtained from the culture of DBF81, DBF107, and DBF129 had pH values spanning from 3.3 to 3.6. The filtrates of the other strains were less acidic (pH ≥ 3.9) (Supplementary Figure 1). A highly significant negative correlation (r = −0.90; P < 0.001) between the pH and Cd concentrations mobilized by the fungal filtrates was found (Supplementary Figure 1). The same finding was observed for Zn (r = 0.91; P < 0.001; Supplementary Figure 1). However, according to the slopes of regression lines drawn for fungal extracts and for control media (Supplementary Figure 1), fungal extracts, particularly the DBF60, DBF81, and DBF129 extracts, mobilized more Cd and Zn compared to the control media adjusted to equivalent pH values.
Ability of the Endophytic Isolates to Colonize the Roots of Noccaea caerulescens
After inoculation with the seven DSE strains (DBF60, DBF79, DBF81, DBF107, DBF108, DBF129, and DBF159), typical structures of fungal endophytes in labeled roots were observed and quantified. We detected thin extracellular hyphae located on the root surface and within root tissues for all endophyte-inoculated treatments. Notably, twisting septate hyphae located within the cortical cells of roots were also found (Figure 1D). The root surface of DBF60-inoculated plants was highly colonized by fungal hyphae (Figure 1B), although intracellular hyphae associated with conidiospores were also detected (Figure 1E). In the case of DBF79 and DBF107, hyphae were often parallel to the vascular tissues (Figure 1F). For most of the inoculated roots and particularly for DBF79- and DBF129-inoculated plants, we detected a high number of intracellular conidiospores (Figure 2C). Contracted hyphae forming intracellular structures such as microsclerotia were observed in DBF108-inoculated roots (Figure 1D). However, the success of root colonization by the endophytes depended on the strain (Figure 1A). With a mean colonization rate <5%, DBF81, DBF107, and DBF129 poorly developed within the roots of N. caerulescens. DBF79- and DBF60-inoculated plants had mean colonization rates of 9.3 and 17.0%, respectively, corresponding to intermediate values. Conversely, DBF159 and DBF108 highly colonized the roots of N. caerulescens, with mean colonization rates of 38.6 and 67.8%, respectively.
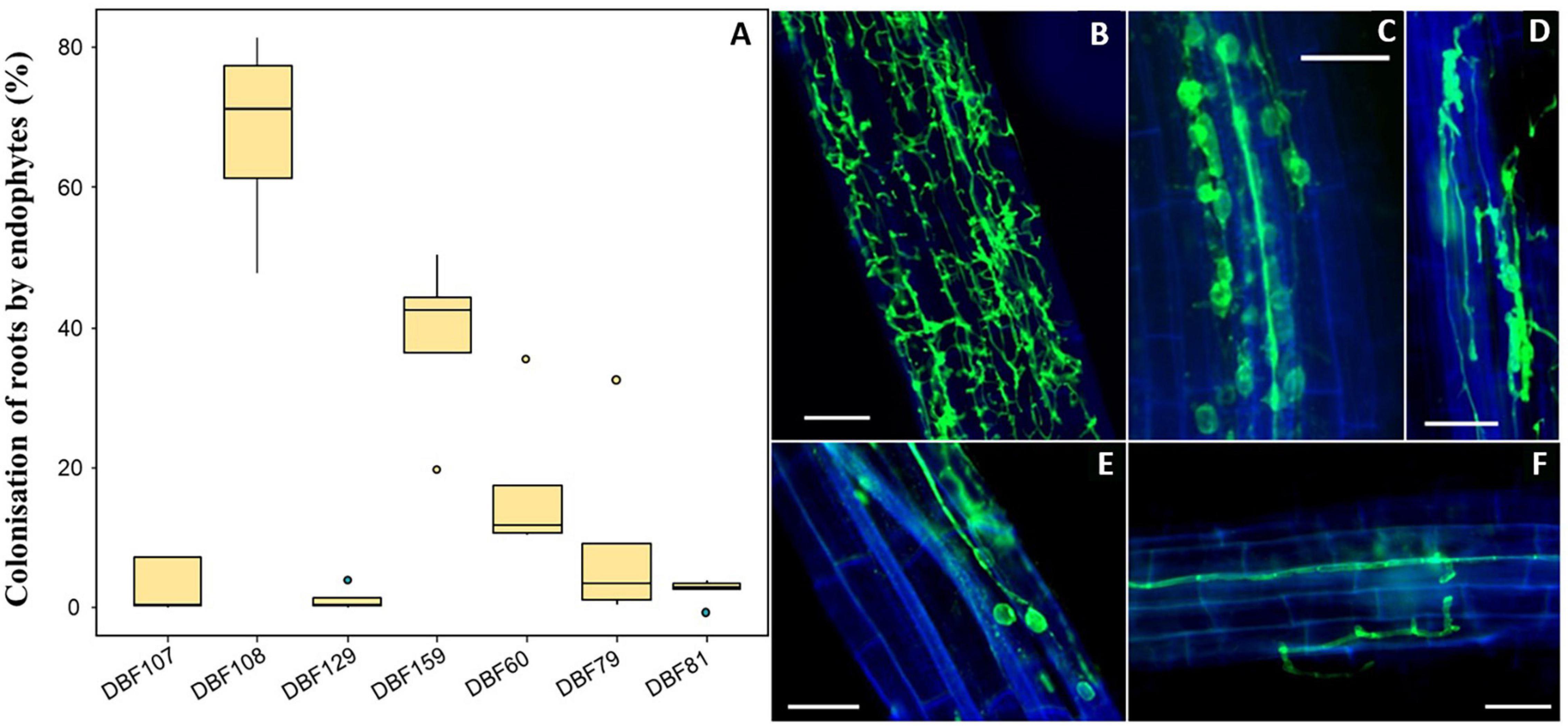
Figure 1. Root colonization of N. caerulescens inoculated by the endophytic isolates. (A) Level of fungal colonization for each strain (n = 5) based on the fluorescence microscopy observation of fungal structures within roots after labeling with WGA-AF488. Fungal structures were absent in mock-inoculated plants. (B) Intracellular colonization of the entire roots by DBF60. (C) Intracellular hyphae associated with conidiospores in DBF107-inoculated plants. (D) Contracted hyphae forming microsclerotia in DBF108-inoculated plants. (E) Intracellular hyphae associated with conidiospores in DBF60-inoculated plants. (F) Intracellular hyphae parallel to the vascular tissues and following the wall of cortical cells. Scales bars = 50 μm.
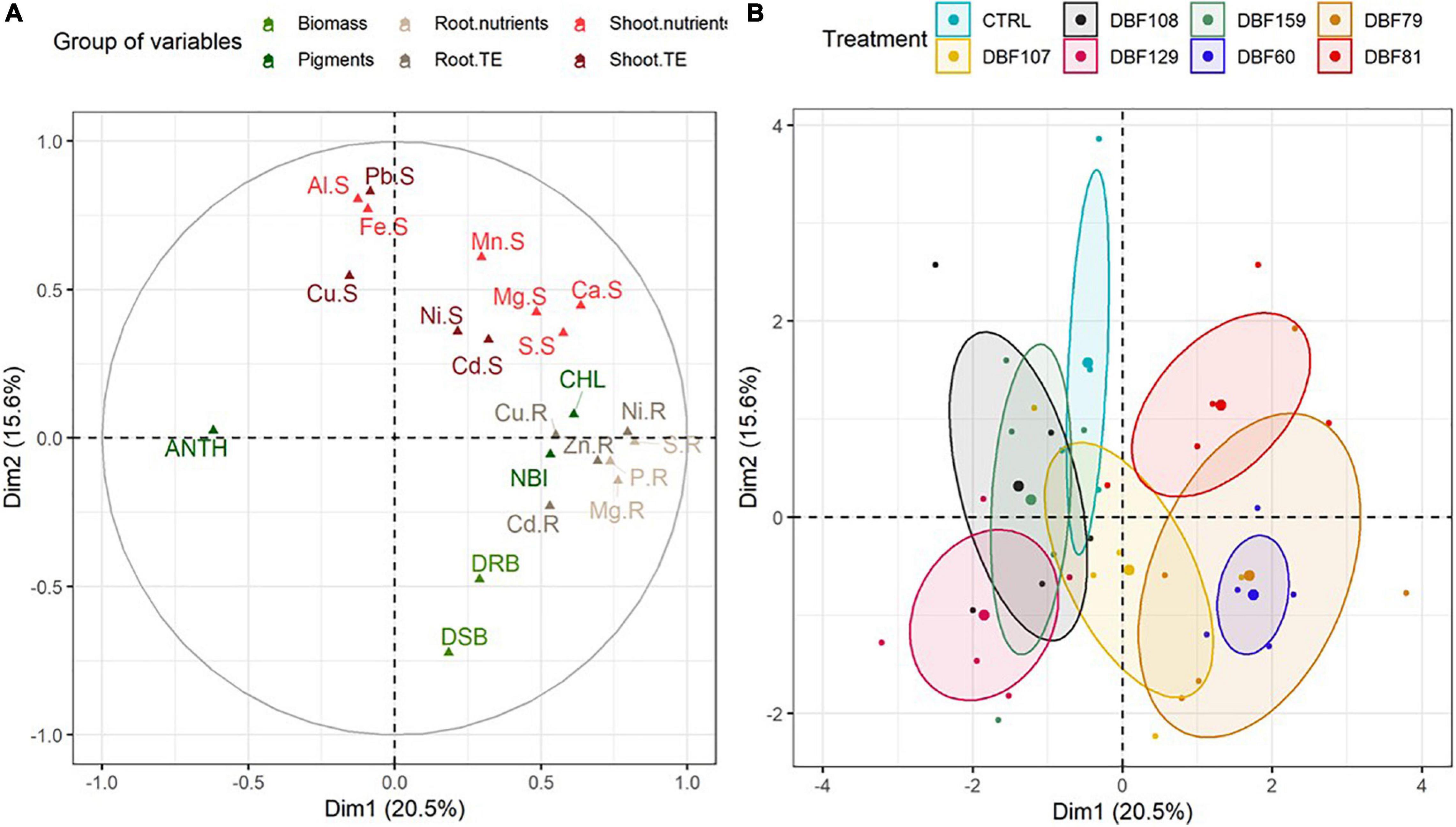
Figure 2. Multiple factor analysis included plant biomass [dry shoot biomass (DSB) and dry root biomass (DRB)], concentration of trace and major elements in roots (“.R”) and shoots (“.S”), and pigment index [anthocyanin (“ANT”), chlorophyll (“CHL”) and flavonoid (“FLA”)] variables for N. caerulescens grown in metal-contaminated soil and inoculated with seven endophytes. (A) Projections of element vectors on the two dimensions. (B) Projection of the groups [plants inoculated with the fungal strains and mock-inoculated (CTRL)], with confidence ellipses.
Influence of Fungal Inoculation on the Biomass of Noccaea caerulescens
As suggested by the MFA, the contribution of variables related to growth parameters [dry shoot biomass (DSB) and dry root biomass (DRB)] to the variability was quite high (Figure 2A). At the end of cultivation, the mean biomass of mock-inoculated N. caerulescens was 61 mg DW for roots and 206 mg DW for shoots, while endophyte-inoculated treatments ranged from 87 to 124 mg DW for roots and from 215 to 385 mg DW for shoots (Figure 3).
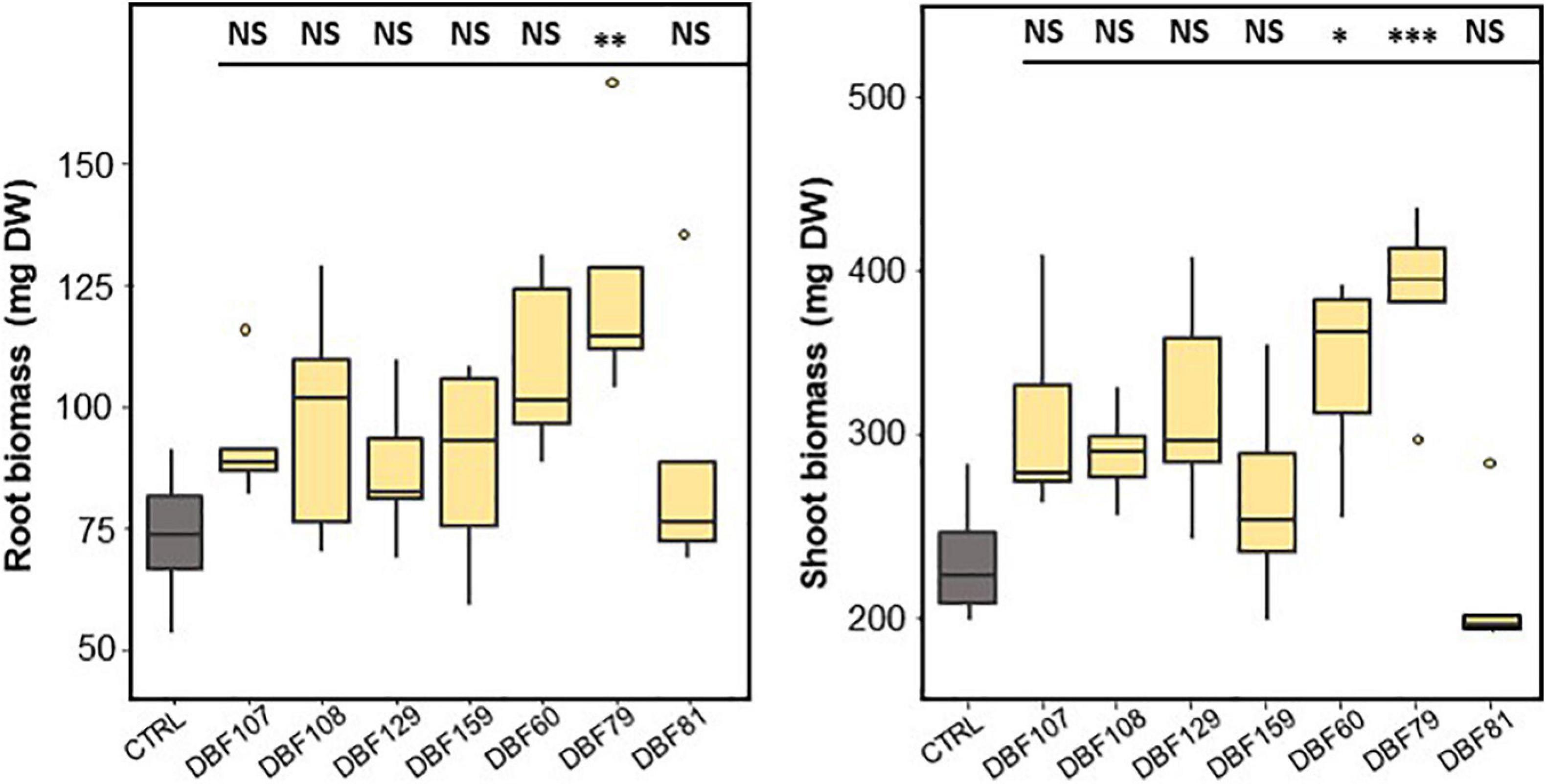
Figure 3. Effect of endophyte inoculation on the root (left) and shoot (right) biomass production of N. caerulescens. Plants were grown for 2 months on metal-contaminated soil and were either inoculated with a fungal strain (n = 5) or mock-inoculated (CTRL, n = 4). Significant differences (ANOVA, Dunnett test) between the CTRL condition and the fungal treatments are represented with the following legend: ∗P < 0.05; ∗∗P < 0.01; ∗∗∗P < 0.001.
DBF79-inoculated plants showed a significant effect on biomass production by increasing the mean root (P < 0.01) and shoot biomass (P < 0.001) by 69 and 65%, respectively, compared to those of the mock-inoculated plants (Figure 3). DBF60 also increased the biomass production of N. caerulescens by 48% for roots (not significant) and 47% for shoots (P < 0.05) compared to the CTRL treatment. Plants inoculated with DBF107, DBF108, DBF129, and DBF159 tended to produce more root and shoot biomass; however, the substantial inter-sample variability did not allow us to confirm this result statistically (Figure 3). This tendency was not found for plants inoculated with DBF81.
Influence of Endophytes on the Leaf Pigments and Mineral Nutrition of Noccaea caerulescens
The chlorophyll content in the leaves of DBF60-inoculated plants was significantly increased compared to that in the mock-inoculated plants (P < 0.01), while the anthocyanin content was reduced (P < 0.05) (Figure 4). The other endophytic strains had no significant influence on the content of leaf pigments or NBI (Figure 4). However, when compared to the CTRL treatment, inoculation with the six other strains tended to slightly decrease the anthocyanin index of leaves, while chlorophyll, flavonoid and NBI indices did not show any particular trend (Figure 4).
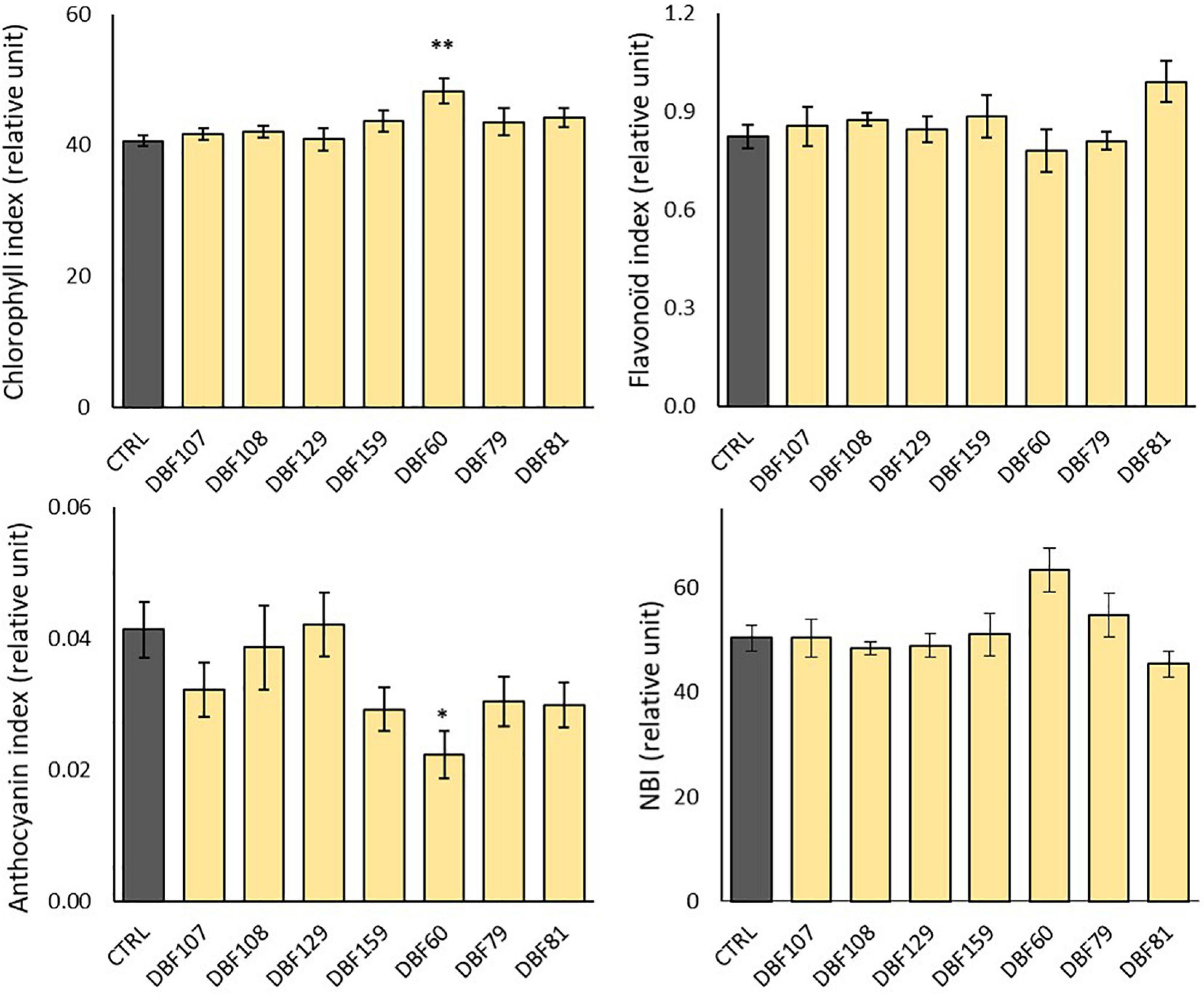
Figure 4. Effect of endophyte inoculation on the leaf pigment contents and nitrogen balance index (NBI) of N. caerulescens. Plants were grown for 2 months on metal-contaminated soil and were either inoculated with a fungal endophyte strain or mock-inoculated (CTRL). Data are the means ± SE (n = 5 for the fungal treatments and n = 4 for the mock-inoculated treatment). Significant differences (ANOVA, Dunnett or Wilcoxon pairwise test) between the CTRL condition and the fungal treatments are represented with the following legend: ∗P < 0.05; ∗∗P < 0.01.
All strains influenced the mineral nutrition of N. caerulescens when inoculated, as they induced significant effects on the elemental composition of roots and shoots, although the effects were more pronounced in roots (Supplementary Table 2). According to the MFA, the groups of variables related to the root nutrient and TE status and shoot pigment content had the highest contributions to the variability for dimension one. Dimension two was mostly explained by the groups of variables related to the shoot nutrients and TE status and biomass production (Figure 2A and Supplementary Figure 2). The cluster corresponding to plants inoculated with DBF60, DBF79, DBF81, and DBF107 differed from those of the other treatments due to its different nutritional status (Figure 2B). The inoculation of N. caerulescens with DBF60, DBF79, and DBF81 increased the root concentrations of Ca (+86, +149, and +199%, respectively; P < 0.01), K (+401, +429, and +656%, respectively, P < 0.01), Mg (+48, +69, and +102%, respectively, P < 0.05), P (+302, +329, and +391%, respectively, P < 0.01) and S (+143, +164, and +223%, respectively, P < 0.01) compared to the CTRL (Figure 5). Among these three strains, only DBF60 had a significant influence on the elemental composition of leaves by increasing K concentrations by 32% and slightly decreasing Al,
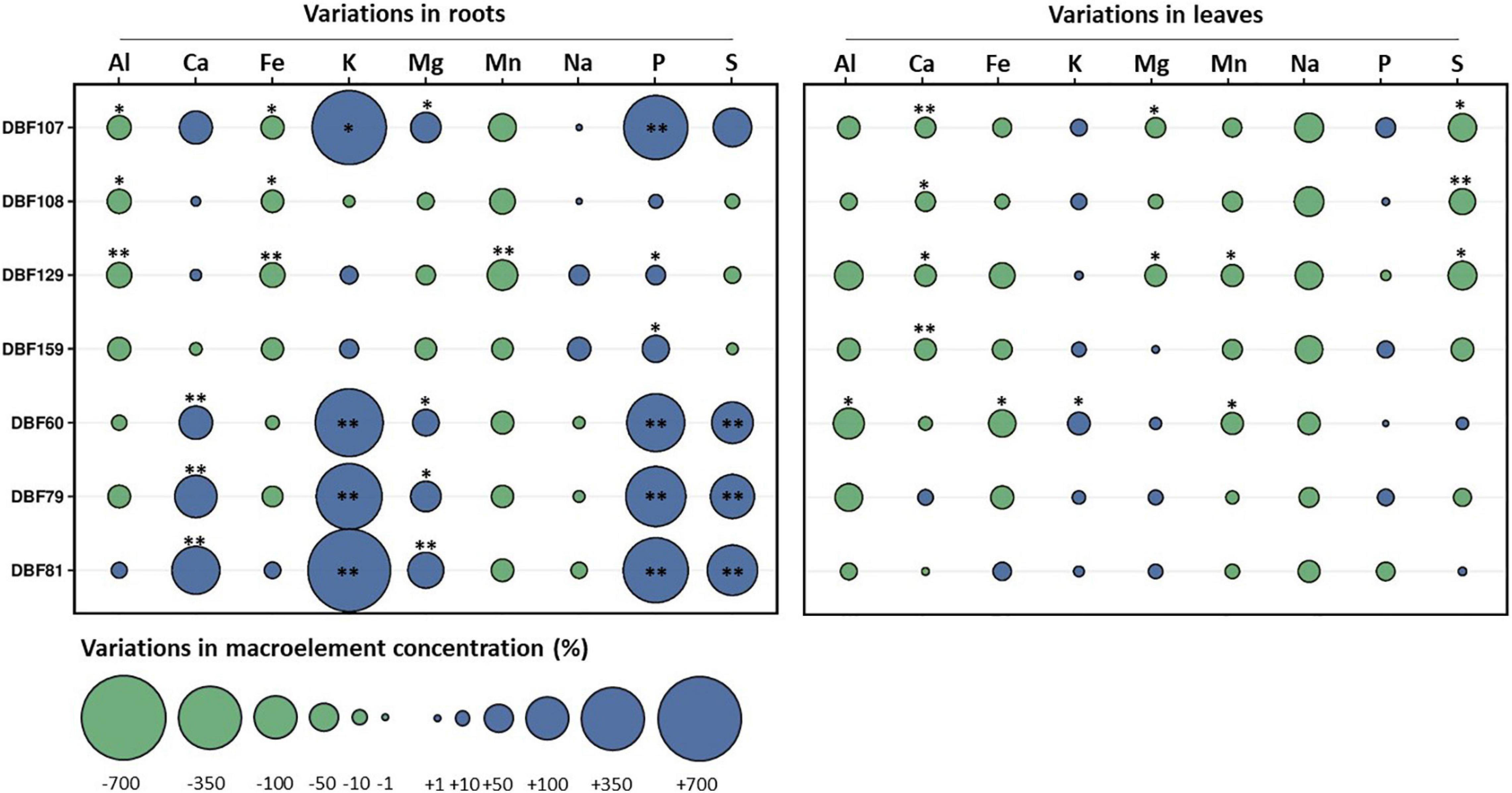
Figure 5. Effect of fungal endophytes on the elemental composition of roots and leaves of N. caerulescens. Data represent the variations (%) of the concentrations of macroelements in roots or leaves of endophyte-inoculated plants in comparison to those of the mock-inoculated plants. Asterisks denote significant differences (ANOVA, Dunnett test) between a given fungal treatment and the control condition with the following legend: ∗∗P < 0.01; ∗P < 0.05.
Fe and Mn concentrations (P < 0.05) (Figure 5). DBF107 had a significant influence on the root concentrations of K (+518%, P < 0.05), Mg (+68%, P < 0.05), and P (+378%, P < 0.01) and on the leaf concentrations of Ca (−25%, P < 0.01), Mg (−23%, P < 0.05), and S (−55%, P < 0.05) (Figure 5). Inoculation of N. caerulescens with DBF108, DBF129, and DBF159 had only slight effects on the nutrient concentrations in leaves and roots (Figure 5).
Influence of Fungal Strains on the Phytoextraction Potential of Noccaea caerulescens
Globally, the mean Cd concentrations in N. caerulescens were 22.3 mg/kg DW for roots and 23.3 mg/kg DW for leaves, which corresponds to values sixfold higher than the concentrations found in the soil. The mean Zn concentration of N. caerulescens roots was approximately twofold lower than the mean soil concentration, while leaves accumulated 1,662.7 mg Zn/kg DW, corresponding to 2.6-fold the mean soil concentration.
As previously observed for macroelements, the group comprising DBF107, DBF60, DBF79 and DBF81 tended to have higher concentrations of Cd and Zn in roots of N. caerulescens when inoculated, while DBF108, DBF129, and DBF159 showed no effect (Figure 6A). The concentrations of Cd in roots increased by 102% for DBF107-inoculated plants (P < 0.01), by 38% for DBF60-inoculated plants (not significant) and by 57% for DBF79-inoculated plants (not significant) compared to the mock-inoculated plants (Figure 6A). The latter endophytic strains as well as DBF81 also tended to increase Zn concentrations in roots from 43 to 56% compared with the CTRL treatment, although not significantly (Figure 6A). None of the tested strains significantly increased the TE concentrations in the leaves of N. caerulescens (Supplementary Table 3).
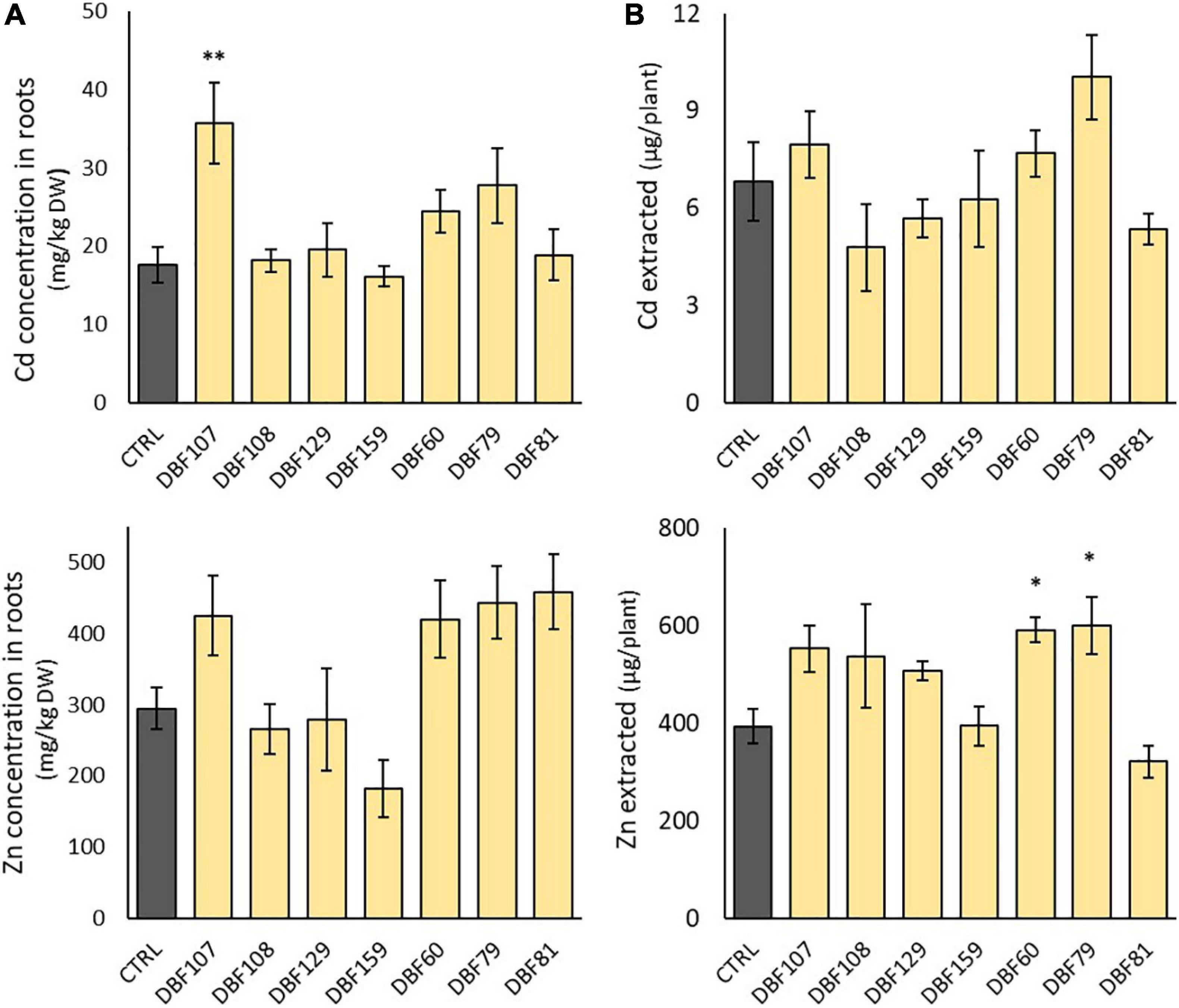
Figure 6. Effect of endophytes on Cd and Zn concentrations in the roots of N. caerulescens (A) and on the amount of Cd and Zn extracted by leaves of N. caerulescens (B). Plants were grown for 2 months on metal-contaminated soil and were either inoculated with an endophytic strain or mock-inoculated (CTRL). Data are the means ± SE (n = 5 for the fungal treatments and n = 4 for the mock-inoculated treatment). Significant differences (ANOVA, Dunnett test) between the CTRL condition and the fungal treatments are represented with the following legend: ∗P < 0.05; ∗∗P < 0.01.
However, when focusing on the amount of Zn extracted from the soil by each plant (leaf biomass × Zn concentrations in leaves), DBF60- and DBF79-inoculated plants extracted +50 and +53% of Zn from the soil, respectively, compared with the mock-inoculated plants (P < 0.05) (Figure 6B). With the amount of Cd extracted by inoculated plants ranging from 4.8 to 10.0 μg/plant vs. 6.8 μg/plant for mock-inoculated plants, we did not detect any significant influence of the strains on the potential of N. caerulescens for Cd phytoextraction (Figure 6B).
Discussion
Noccaea caerulescens Hosts Several Taxa of Recognized PGP Fungal Endophytes
Noccaea caerulescens has been recently shown to be a potential candidate for fungal-assisted phytoextraction, as plant responses are beneficial when associated with certain host (isolated from the same species) (Ważny et al., 2021) and non-host (Yung et al., 2021b) endophytic strains under TE exposure. In the present study, we tested seven fungal endophytes isolated from non-metallicolous and calamine populations of N. caerulescens based on their taxonomy. Among the panel of isolated taxa, we selected seven taxa that have previously demonstrated promising direct PGP abilities (i.e., improvement of plant nutrition, growth, and/or stress alleviation) or indirect positive impacts by acting as biocontrol agents. We selected two strains belonging to Cladosporium sp. (DBF107) and C. cladosporioides (DBF129). Ważny et al. (2021) also isolated a strain of C. cladosporioides from the root of N. caerulescens that significantly increased plant biomass and stimulated the uptake and accumulation of Ni in both roots and shoots compared to non-inoculated controls. Phialophora mustea (DBF108) is a DSE with recognized PGP abilities, including the improvement of metal tolerance for the plant (Berthelot et al., 2017; Zhu et al., 2018). A strain of P. mustea isolated from poplar roots has already been proposed as a promising candidate to enhance the phytoextraction potential of N. caerulescens (Yung et al., 2021b). Alternaria thlaspis (syn: Embellisia thlaspis; DBF79) was described after isolation from the roots of N. caerulescens growing in soil with high levels of Zn and Pb (David et al., 2000). This species was also recently isolated from roots and leaves of calamine and serpentine N. caerulescens and tested for its PGP abilities on N. caerulescens (Ważny et al., 2021). Microdochium bolleyi (syn: Idriella bolleyi; DBF159) is a well-known species of DSE that has been extensively isolated from roots and shoots of several cereals and grasses (Gadd, 1981; David et al., 2016; Rothen et al., 2018). Although, it is sometimes considered as a pathogen, it is also considered non-pathogenic in some cases (Kirk and Deacon, 1987), with potential implications for organic matter mineralization, plant nutrition (Mandyam et al., 2010), and biocontrol agents (Jadubansa et al., 1994). Indeed, Shadmani et al. (2021) recently demonstrated that M. Bolleyi could be exploited to improve the potential of barley for the remediation of Cd-contaminated sites. Trichoderma harzanium (DBF81) is a well-studied fungus used as a biocontrol agent in agriculture against plant pathogens and nematodes (Lorito et al., 2010). Some strains are able to induce plant defenses (Yedidia et al., 1999) and stimulate plant growth and development (Yedidia et al., 2001; Hermosa et al., 2012) by establishing a molecular dialog with the roots (Mendoza-Mendoza et al., 2018). These beneficial effects of T. harzanium have been mainly demonstrated on horticultural plants. Recently, Poveda et al. (2019) showed that T. harzianum favors the access of arbuscular mycorrhizal fungi to non-host Brassicaceae roots, providing new perspectives for agronomy. Metapochonia rubescens was mainly studied for its application as a biocontrol agent as a facultative parasite of major plant-parasitic nematodes (Moosavi and Zare, 2020).
Fungal Endophytes Differentially Colonized the Roots of Noccaea caerulescens
While the method used for estimating the rate of fungal colonization is semi-quantitative, it enabled to monitor the success of the inoculations, by confirming the presence of fungi associated with plant roots and providing an estimation of the extent to which the colonization occurred (Ayliffe et al., 2013). Although all of the selected strains here were indigenous and belonged to taxa that have been described as potential auxiliary endophytic fungi in other contexts, under metallic stress conditions, the success of their association (i.e., ability to colonize roots and positive host plant response) with N. caerulescens was variable. Although DBF79, DBF81, and DBF129 were isolated from the leaves of N. caerulescens in the present study, these taxa are not strict phyllospheric fungi. When reinoculated with N. caerulescens by mixing the inocula with the soil, these strains indeed colonized the plant roots, although with a low rate of colonization. According to the literature, A. thlaspi (DBF79) and C. cladosporioides (DBF129) have already been isolated from N. caerulescens roots (David et al., 2000; Ważny et al., 2021), whereas T. harzanium (DBF81) is a well-known soil-borne filamentous fungus (Lorito et al., 2010), suggesting its probable presence in the rhizosphere. Fungal structural characteristics of endophytic fungi, including twisting hyphae, have also been observed for all of the other strains.
Three Endophytic Strains Had a Neutral Effect on Noccaea caerulescens
An endophytic relationship, which can vary from parasitism to mutualism (Freeman and Rodriguez, 1993; Mandyam and Jumpponen, 2005; Newsham, 2011; Mayerhofer et al., 2013), has been demonstrated to be context-dependent, with determining factors such as environmental conditions (Álvarez-Loayza et al., 2011), the composition of the plant-associated microbial community (Junker et al., 2012) and the plant genotype (Wheeler et al., 2019). Long-term endophyte-host interactions should be considered a basis for mutual interactions, with both sides constantly shaping the organization and structure of the other, resulting in a beneficial relation (Saikkonen et al., 2004). This plant-microbiome superorganism, which is also called a holobiont, is subjected to evolutionary forces that may result in the coevolution of host symbiont interactions (Rosenberg and Zilber-Rosenberg, 2018), where microorganisms could be considered a genetic extension of the host plant genome, favoring hologenome plasticity and thus plant adaptability to its environment (Durand et al., 2021). The success of inoculation could be mainly dependent on the host-symbiont combination at the species level (Fernando and Currah, 1996; Newsham, 2011; Mayerhofer et al., 2013; Berthelot et al., 2016), but in some cases, the plant population-fungal strain level appeared to be important (Ważny et al., 2021).
In the present study, the effects of reinoculating N. caerulescens with their associated endophytes were neutral to beneficial, depending on the strain. The lack of effect of DBF129 could be related to its low ability to colonize the roots of inoculated plants. The fact that DBF159 and DBF108 were highly abundant within the cortical cells of N. caerulescens roots and that they have been identified as potential PGP taxa elsewhere (Mandyam et al., 2010; Yung et al., 2021b), while having no effect on plant growth and/or nutrition in the present study, confirms the somewhat variable outcomes of host-endophyte interactions. Interestingly, we showed in our previous study that the non-host strain of P. mustea (Pr27, isolated from poplar roots) had a positive effect on the mineral nutrition of N. caerulescens (Yung et al., 2021b), while the indigenous strain DBF108 belonging to the same species had no effect in the present study. These results do not support assumptions in favor of the necessity of long-term adaptation of the plant and fungal endophytes resulting in mutualism.
Four Endophytic Strains Highly Enhanced the Mineral Nutrition of Noccaea caerulescens
The most pronounced effects of the isolates, particularly those of DBF60, DBF79, DBF81, and DBF107, concerned the root elemental concentrations of Ca, Mg, K, P, and S, with a fivefold increase in some cases. Our study indicates a good potential of the DBF60 and DBF81 strains to solubilize inorganically bound phosphate, which is consistent with the improvement of the P concentration in plant roots. T. harzanium (DBF81) is known to be involved in mineral solubilization (Altomare et al., 1999; Rawat and Tewari, 2011; Tallapragada and Gudimi, 2011; Bader et al., 2020) through various mechanisms, including solubilization via acidification, redox reactions, chelation and hydrolysis (Li et al., 2015). According to several authors, the main mechanism by which fungal endophytes solubilize phosphate and other minerals is the production of organic acids that lowers the pH of the soil, consequently causing the solubilization of insoluble minerals (Chhabra and Dowling, 2017; Adhikari and Pandey, 2019; Mehta et al., 2019). All of the tested fungal strains, except DBF79, reduced the pH of their media. Therefore, improvement of the mineral nutrition of DBF79-inoculated plants is unlikely due to the sole mechanism of acidification. The rhizophagy, in which fungi can be the prey of roots and serve as nourishing factors to improve plant nutrition and growth, would be a possible hypothesis explaining the improvement of the root elemental composition of DBF79-inoculated plants in the absence of strain-mediated acidification (Paungfoo-Lonhienne et al., 2010; White et al., 2018). However, we cannot rule out an important acidification of DBF79 in the rhizosphere. To examine this hypothesis, further experiments are needed to locally measure the pH in the rhizosphere of both non-inoculated and inoculated plants.
DBF60 and DBF79 Are Two Promising Fungal Isolates for Improving the Zn Phytoextraction Potential of Noccaea caerulescens
In the case of DBF60- and DBF79-inoculated plants, the improvement of mineral nutrition was concomitant with an increase in plant biomass production of the same order of magnitude as those detected for similar inoculation assays using plants exposed to TE (Khan et al., 2017; Rozpądek et al., 2018; Sharma et al., 2019; Ważny et al., 2021). According to the literature, such tripartite relationships (i.e., fungal colonization vs. nutrient content vs. plant biomass) have not been reported in many studies (Berthelot et al., 2019). Our results for A. thlaspis (DBF79) confirmed that this species could promote the growth of N. caerulescens, while this is the first report of direct PGP abilities of M. rubescens (DBF60). The comparable ability of the seven endophytes to produce IAA suggests that this phytohormone, which is well known to improve root development and enhance root exudation and mineral uptake (Fu et al., 2015), alone cannot explain the higher increase in biomass for DBF60- and DBF79-inoculated plants. However, the correlation between IAA synthesis and growth promotion in soil-based systems is scarce (Nieto-Jacobo et al., 2017) and deserves further attention in the future. In addition to the improvement of plant mineral nutrition, DBF60 also induced changes in the metabolism of N. caerulescens by increasing chlorophyll and decreasing anthocyanin contents, suggesting an enhancement of primary metabolism at the cost of secondary metabolism. The promotion of secondary metabolism through the production of anthocyanins is commonly observed when plants face biotic or abiotic stressors (Landi et al., 2015). In our context, the decrease in the anthocyanin concentration in favor of chlorophyll indicates that DBF60 could help plants alleviate metallic stress.
Due to their ability to increase the aboveground biomass of N. caerulescens and their tendency to increase Zn concentrations in leaves (statistically not significant), DBF60 and DBF79 enhanced the Zn phytoextraction potential (+ 50%) of N. caerulescens. This improvement was slightly better than that induced by the non-host Pr27 strain (amount of Zn extracted increased by 30%) (Yung et al., 2021b).
The mechanism leading to the increase in TE in a plant could be related to the capacity of endophytes to improve TE bioavailability through the release of metal chelating agents (e.g., siderophores, biosurfactants or organic acids), acidification of soils and redox activity (Deng and Cao, 2017; Domka et al., 2019). The results from our assay revealed that the quantity of Cd and Zn mobilized from the soil by the fungal extracts of DBF60 and DBF79 was the lowest. These results suggest that the ability of fungi to mobilize TE from the soil is not representative of the mechanisms occurring in the case of plant-fungus interactions. According to the results obtained from in vitro tests, the enhancement of plant nutrition and Zn phytoextraction mediated by DBF60 is partly associated with its ability to (i) solubilize various forms of insoluble Zn and P, (ii) acidify its environment, and (iii) produce siderophores. However, the effect of DBF79 on plant nutrition and Zn phytoextraction has not been corroborated by in vitro tests, confirming the necessity to adopt in planta approaches to better understand the solubilization level mediated by fungal endophytes.
The selected fungal endophytes were tested for their ability to improve the TE phytoextraction of N. caerulescens in sterilized soil, limiting competition with native microflora and consequently mitigating the risk of inoculation failure. It would be interesting to conduct a similar study comparing host and non-host PGP strains within the same system using non-sterilized soil to investigate the potential of these strains to tolerate competition with indigenous microflora.
Conclusion
In conclusion, we demonstrated that N. caerulescens responses to fungal inoculation using native strains ranged from neutral to beneficial. Despite the seven tested strains had PGP abilities according to the in vitro tests, only two of them exhibited relevant effects to improve the phytoextraction potential of N. caerulescens when reinoculated. The improvement in plant elemental nutrition mediated by M. rubescens (DBF60) and A. thlaspis (DBF79) was significant and led to an increase in plant biomass and consequently to a higher amount of Zn extracted. These fungal endophytes could represent potential candidates for field applications using hyperaccumulating plants, with benefits for microbial-assisted phytoextraction and agromining.
Data Availability Statement
The datasets presented in this study can be found in online repositories. The names of the repository/repositories and accession number(s) can be found in the article/Supplementary Material.
Author Contributions
CS and DB designed the study. LY, CS, AA-B, and DB performed the material preparation, data collection, and analysis. LY, CS, and DB wrote the manuscript. All authors read and approved the final manuscript.
Funding
This work was supported by the Endoextract project funded by the Pôle Scientifique OTELo and by the French PIA project “Lorraine Université d’Excellence” Deepsurf, reference ANR-15-IDEX-04-LUE.
Conflict of Interest
The authors declare that the research was conducted in the absence of any commercial or financial relationships that could be construed as a potential conflict of interest.
Acknowledgments
The authors would like to thank Christine Friry and Romain Goudon for their technical support in microscopic examinations and ICP-AES analyses, respectively. This work is included in the scientific program of the GISFI research consortium (Groupement d’Intérêt Scientifique sur les Friches Industrielles – http://www.gisfi.univ-lorraine.fr).
Supplementary Material
The Supplementary Material for this article can be found online at: https://www.frontiersin.org/articles/10.3389/fmicb.2021.689367/full#supplementary-material
References
Aboudrar, W., Schwartz, C., Morel, J.-L., and Boularbah, A. (2007). Soil microbial diversity as affected by the rhizosphere of the hyperaccumulator Thlaspi caerulescens under natural conditions. Int. J. Phytoremed. 9, 41–52. doi: 10.1080/15226510601139417
Adhikari, P., and Pandey, A. (2019). Phosphate solubilization potential of endophytic fungi isolated from Taxus wallichiana Zucc. roots. Rhizosphere 9, 2–9. doi: 10.1016/j.rhisph.2018.11.002
Alloway, B. J. (2013). “Sources of heavy metals and metalloids in soils,” in Heavy Metals in Soils: Trace Metals and Metalloids in Soils and their Bioavailability Environmental Pollution, ed. B. J. Alloway (Dordrecht: Springer), 11–50.
Altomare, C., Norvell, W. A., Björkman, T., and Harman, G. E. (1999). Solubilization of phosphates and micronutrients by the plant-growth-promoting and biocontrol fungus Trichoderma harzianum Rifai 1295-22. Appl. Environ. Microbiol. 65, 2926–2933. doi: 10.1128/AEM.65.7.2926-2933.1999
Álvarez-Loayza, P. Jr., White, J. F., Torres, M. S., Balslev, H., Kristiansen, T., Svenning, J.-C., et al. (2011). Light converts endosymbiotic fungus to pathogen, influencing seedling survival and niche-space filling of a common tropical tree, Iriartea deltoidea. PLoS One 6:e16386. doi: 10.1371/journal.pone.0016386
Álvarez-López, V., Prieto-Fernández, Á, Becerra-Castro, C., Monterroso, C., and Kidd, P. S. (2016). Rhizobacterial communities associated with the flora of three serpentine outcrops of the Iberian Peninsula. Plant Soil 403, 233–252. doi: 10.1007/s11104-015-2632-0
Assunção, A. G. L., Bookum, W. M., Nelissen, H. J. M., Vooijs, R., Schat, H., and Ernst, W. H. O. (2003). Differential metal-specific tolerance and accumulation patterns among Thlaspi caerulescens populations originating from different soil types. New Phytol. 159, 411–419. doi: 10.1046/j.1469-8137.2003.00819.x
Ayliffe, M., Periyannan, S. K., Feechan, A., Dry, I., Schumann, U., Wang, M.-B., et al. (2013). A simple method for comparing fungal biomass in infected plant tissues. Mol. Plant-Microbe Interact. 26, 658–667. doi: 10.1094/MPMI-12-12-0291-R
Bader, A. N., Salerno, G. L., Covacevich, F., and Consolo, V. F. (2020). Native Trichoderma harzianum strains from Argentina produce indole-3 acetic acid and phosphorus solubilization, promote growth and control wilt disease on tomato (Solanum lycopersicum L.). J. King Saud Univ. Sci. 32, 867–873. doi: 10.1016/j.jksus.2019.04.002
Becerra-Castro, C., Monterroso, C., Prieto-Fernández, A., Rodríguez-Lamas, L., Loureiro-Viñas, M., Acea, M. J., et al. (2012). Pseudometallophytes colonising Pb/Zn mine tailings: a description of the plant–microorganism–rhizosphere soil system and isolation of metal-tolerant bacteria. J. Hazard. Mater. 21, 350–359. doi: 10.1016/j.jhazmat.2012.03.039
Benizri, E., Lopez, S., Durand, A., and Kidd, P. S. (2021). “Diversity and role of endophytic and rhizosphere microbes associated with hyperaccumulator plants during metal accumulation,” in Agromining: Farming for Metals: Extracting Unconventional Resources Using Plants Mineral Resource Reviews, eds A. van der Ent, A. J. M. Baker, G. Echevarria, M.-O. Simonnot, and J. L. Morel (Cham: Springer International Publishing), 239–279.
Berthelot, C., Blaudez, D., Beguiristain, T., Chalot, M., and Leyval, C. (2018). Co-inoculation of Lolium perenne with Funneliformis mosseae and the dark septate endophyte Cadophora sp. in a trace element-polluted soil. Mycorrhiza 28, 301–314. doi: 10.1007/s00572-018-0826-z
Berthelot, C., Blaudez, D., and Leyval, C. (2017). Differential growth promotion of poplar and birch inoculated with three dark septate endophytes in two trace element-contaminated soils. Int. J. Phytoremediat. 19, 1118–1125. doi: 10.1080/15226514.2017.1328392
Berthelot, C., Chalot, M., Leyval, C., and Blaudez, D. (2019). “From darkness to light: emergence of the mysterious dark septate endophytes in plant growth promotion and stress alleviation,” in Endophytes for a Growing World, eds T. R. Hodkinson, F. M. Doohan, and M. J. Saunders (Cambridge: Cambridge University Press), 143–164.
Berthelot, C., Leyval, C., Foulon, J., Chalot, M., and Blaudez, D. (2016). Plant growth promotion, metabolite production and metal tolerance of dark septate endophytes isolated from metal-polluted poplar phytomanagement sites. FEMS Microbiol. Ecol. 92:fiw144. doi: 10.1093/femsec/fiw144
Bosch, T. C. G., and McFall-Ngai, M. J. (2011). Metaorganisms as the new frontier. Zoology 114, 185–190. doi: 10.1016/j.zool.2011.04.001
Burges, A., Epelde, L., Blanco, F., Becerril, J. M., and Garbisu, C. (2017). Ecosystem services and plant physiological status during endophyte-assisted phytoremediation of metal contaminated soil. Sci. Total Environ. 584–585, 329–338. doi: 10.1016/j.scitotenv.2016.12.146
Chhabra, S., and Dowling, D. N. (2017). “Endophyte-promoted nutrient acquisition: phosphorus and iron,” in Functional Importance of the Plant Microbiome: Implications for Agriculture, Forestry and Bioenergy, ed. S. L. Doty (Cham: Springer International Publishing), 21–42. doi: 10.1007/978-3-319-65897-1_3
Ciadamidaro, L., Girardclos, O., Bert, V., Zappelini, C., Yung, L., Foulon, J., et al. (2017). Poplar biomass production at phytomanagement sites is significantly enhanced by mycorrhizal inoculation. Environ. Exp. Bot. 139, 48–56. doi: 10.1016/j.envexpbot.2017.04.004
Clemens, S., and Ma, J. F. (2016). Toxic heavy metal and metalloid accumulation in crop plants and foods. Annu. Rev. Plant Biol. 67, 489–512. doi: 10.1146/annurev-arplant-043015-112301
David, A. S., Haridas, S., LaButti, K., Lim, J., Lipzen, A., Wang, M., et al. (2016). Draft genome sequence of Microdochium bolleyi, a dark septate fungal endophyte of beach grass. Genome Announc. 4:e00270-16. doi: 10.1128/genomeA.00270-16
David, J. C., Coles, K., Fisher, J., and Moss, S. T. (2000). A new species of Embellisia from soil with high levels of heavy metals. Mycoscience 41, 533–537. doi: 10.1007/BF02460917
Deng, Z., and Cao, L. (2017). Fungal endophytes and their interactions with plants in phytoremediation: a review. Chemosphere 168, 1100–1106. doi: 10.1016/j.chemosphere.2016.10.097
Domka, A. M., Rozpaądek, P., and Turnau, K. (2019). Are fungal endophytes merely mycorrhizal copycats? The role of fungal endophytes in the adaptation of plants to metal toxicity. Front. Microbiol. 10:371. doi: 10.3389/fmicb.2019.00371
Durand, A., Leglize, P., and Benizri, E. (2021). Are endophytes essential partners for plants and what are the prospects for metal phytoremediation? Plant Soil. 460, 1–30. doi: 10.1007/s11104-020-04820-w
Escarré, J., Lefèbvre, C., Frérot, H., Mahieu, S., and Noret, N. (2013). Metal concentration and metal mass of metallicolous, non metallicolous and serpentine Noccaea caerulescens populations, cultivated in different growth media. Plant Soil 370, 197–221. doi: 10.1007/s11104-013-1618-z
Fernando, A. A., and Currah, R. S. (1996). A comparative study of the effects of the root endophytes Leptodontidium orchidicola and Phialocephala fortinii (Fungi Imperfecti) on the growth of some subalpine plants in culture. Can. J. Bot. 74, 1071–1078. doi: 10.1139/b96-131
Fones, H. N., McCurrach, H., Mithani, A., Smith, J. A. C., and Preston, G. M. (2016). Local adaptation is associated with zinc tolerance in Pseudomonas endophytes of the metal-hyperaccumulator plant Noccaea caerulescens. Proc. R. Soc. B Biol. Sci. 283:20160648. doi: 10.1098/rspb.2016.0648
Freeman, S., and Rodriguez, R. J. (1993). Genetic conversion of a fungal plant pathogen to a nonpathogenic, endophytic mutualist. Science 260, 75–78. doi: 10.1126/science.260.5104.75
Fu, S.-F., Wei, J.-Y., Chen, H.-W., Liu, Y.-Y., Lu, H.-Y., and Chou, J.-Y. (2015). Indole-3-acetic acid: a widespread physiological code in interactions of fungi with other organisms. Plant Signal. Behav. 10:e1048052. doi: 10.1080/15592324.2015.1048052
Gadd, G. M. (1981). Preliminary studies on Microdochium bolleyi with special reference to colonization of barley. Trans. Br. Mycol. Soc. 76, 397–403. doi: 10.1016/S0007-1536(81)80065-4
Gil-Martínez, M., López-García, Á, Domínguez, M. T., Navarro-Fernández, C. M., Kjøller, R., Tibbett, M., et al. (2018). Ectomycorrhizal fungal communities and their functional traits mediate plant–soil interactions in trace element contaminated soils. Front. Plant Sci. 9:1682. doi: 10.3389/fpls.2018.01682
Gonneau, C. (2014). Distribution, Écologie et Évolution de L’hyperaccumulation des Éléments en Traces par Noccaea caerulescens. Ph.D. Thesis. Metz: Université de Lorraine.
Gonneau, C., Genevois, N., Frérot, H., Sirguey, C., and Sterckeman, T. (2014). Variation of trace metal accumulation, major nutrient uptake and growth parameters and their correlations in 22 populations of Noccaea caerulescens. Plant Soil 384, 271–287. doi: 10.1007/s11104-014-2208-4
Gonneau, C., Noret, N., Godé, C., Frérot, H., Sirguey, C., Sterckeman, T., et al. (2017). Demographic history of the trace metal hyperaccumulator Noccaea caerulescens (J. Presl and C. Presl) F. K. Mey. in Western Europe. Mol. Ecol. 26, 904–922. doi: 10.1111/mec.13942
Hansda, A., Kumar, V., Anshumali, A., and Usmani, Z. (2014). Phytoremediation of Heavy Metals Contaminated Soil Using Plant Growth Promoting Rhizobacteria (PGPR): A Current Perspective. Available online at: http://updatepublishing.com/journal/index.php/rrst/article/view/1183 (accessed on 17 April 2020)
Hermosa, R., Viterbo, A., Chet, I., and Monte, E. (2012). Plant-beneficial effects of Trichoderma and of its genes. Microbiology 158, 17–25. doi: 10.1099/mic.0.052274-0
Jacobs, A., De Brabandere, L., Drouet, T., Sterckeman, T., and Noret, N. (2018a). Phytoextraction of Cd and Zn with Noccaea caerulescens for urban soil remediation: influence of nitrogen fertilization and planting density. Ecol. Eng. 116, 178–187. doi: 10.1016/j.ecoleng.2018.03.007
Jacobs, A., Drouet, T., and Noret, N. (2018b). Field evaluation of cultural cycles for improved cadmium and zinc phytoextraction with Noccaea caerulescens. Plant Soil 430, 381–394. doi: 10.1007/s11104-018-3734-2
Jadubansa, P., Lethbridge, G., and Bushell, M. (1994). Physiology of production of viable biomass and spore inoculum for the biocontrol agent Idriella (Microdochium) bolleyi. Enzyme Microb. Technol. 16, 24–28. doi: 10.1016/0141-0229(94)90105-8
Junker, C., Draeger, S., and Schulz, B. (2012). A fine line – endophytes or pathogens in Arabidopsis thaliana. Fungal Ecol. 5, 657–662. doi: 10.1016/j.funeco.2012.05.002
Khan, A. R., Ullah, I., Waqas, M., Park, G.-S., Khan, A. L., Hong, S.-J., et al. (2017). Host plant growth promotion and cadmium detoxification in Solanum nigrum, mediated by endophytic fungi. Ecotoxicol. Environ. Saf. 136, 180–188. doi: 10.1016/j.ecoenv.2016.03.014
Kirk, J. J., and Deacon, J. W. (1987). Control of the take-all fungus by Microdochium bolleyi, and interactions involving M. bolleyi, Phialophora graminicola and Periconia macrospinosa on cereal roots. Plant Soil 98, 231–237. doi: 10.1007/BF02374826
Kong, Z., and Glick, B. R. (2017). “Chapter Two - The role of plant growth-promoting bacteria in metal phytoremediation,” in Advances in Microbial Physiology, ed. R. K. Poole (Cambridge: Academic Press), 97–132. doi: 10.1016/bs.ampbs.2017.04.001
Lacercat-Didier, L., Berthelot, C., Foulon, J., Errard, A., Martino, E., Chalot, M., et al. (2016). New mutualistic fungal endophytes isolated from poplar roots display high metal tolerance. Mycorrhiza 26, 657–671. doi: 10.1007/s00572-016-0699-y
Landi, M., Tattini, M., and Gould, K. S. (2015). Multiple functional roles of anthocyanins in plant-environment interactions. Environ. Exp. Bot. 119, 4–17. doi: 10.1016/j.envexpbot.2015.05.012
Lebeau, T., Braud, A., and Jézéquel, K. (2008). Performance of bioaugmentation-assisted phytoextraction applied to metal contaminated soils: a review. Environ. Pollut. 153, 497–522. doi: 10.1016/j.envpol.2007.09.015
Li, R.-X., Cai, F., Pang, G., Shen, Q.-R., Li, R., and Chen, W. (2015). Solubilisation of phosphate and micronutrients by Trichoderma harzianum and its relationship with the promotion of tomato plant growth. PLoS One 10:e0130081. doi: 10.1371/journal.pone.0130081
Lombi, E., Zhao, F. J., Dunham, S. J., and McGRATH, S. P. (2000). Cadmium accumulation in populations of Thlaspi caerulescens and Thlaspi goesingense. New Phytol. 145, 11–20. doi: 10.1046/j.1469-8137.2000.00560.x
Lorito, M., Woo, S. L., Harman, G. E., and Monte, E. (2010). Translational research on Trichoderma: from ’Omics to the field. Annu. Rev. Phytopathol. 48, 395–417. doi: 10.1146/annurev-phyto-073009-114314
Ma, Y., Rajkumar, M., Zhang, C., and Freitas, H. (2016). Beneficial role of bacterial endophytes in heavy metal phytoremediation. J. Environ. Manage. 174, 14–25. doi: 10.1016/j.jenvman.2016.02.047
Mandyam, K., and Jumpponen, A. (2005). Seeking the elusive function of the root-colonising dark septate endophytic fungi. Stud. Mycol. 53, 173–189. doi: 10.3114/sim.53.1.173
Mandyam, K., Loughin, T., and Jumpponen, A. (2010). Isolation and morphological and metabolic characterization of common endophytes in annually burned tallgrass prairie. Mycologia 102, 813–821. doi: 10.3852/09-212
Maxted, A. P., Black, C. R., West, H. M., Crout, N. M. J., Mcgrath, S. P., and Young, S. D. (2007). Phytoextraction of cadmium and zinc by Salix from soil historically amended with sewage sludge. Plant Soil 290, 157–172. doi: 10.1007/s11104-006-9149-5
Mayerhofer, M. S., Kernaghan, G., and Harper, K. A. (2013). The effects of fungal root endophytes on plant growth: a meta-analysis. Mycorrhiza 23, 119–128. doi: 10.1007/s00572-012-0456-9
McGrath, S. P., Lombi, E., Gray, C. W., Caille, N., Dunham, S. J., and Zhao, F. J. (2006). Field evaluation of Cd and Zn phytoextraction potential by the hyperaccumulators Thlaspi caerulescens and Arabidopsis halleri. Environ. Pollut. 141, 115–125. doi: 10.1016/j.envpol.2005.08.022
Mehta, P., Sharma, R., Putatunda, C., and Walia, A. (2019). “Endophytic fungi: role in phosphate solubilization,” in Advances in Endophytic Fungal Research: Present Status and Future Challenges Fungal Biology, ed. B. P. Singh (Cham: Springer International Publishing), 183–209.
Mendoza-Mendoza, A., Zaid, R., Lawry, R., Hermosa, R., Monte, E., Horwitz, B. A., et al. (2018). Molecular dialogues between Trichoderma and roots: role of the fungal secretome. Fungal Biol. Rev. 32, 62–85. doi: 10.1016/j.fbr.2017.12.001
Miransari, M. (2017). “Arbuscular mycorrhizal fungi and heavy metal tolerance in plants,” in Arbuscular Mycorrhizas and Stress Tolerance of Plants, ed. Q.-S. Wu (Singapore: Springer Singapore), 147–161.
Moosavi, M. R., and Zare, R. (2020). “Fungi as biological control agents of plant-parasitic nematodes,” in Plant Defence: Biological Control Progress in Biological Control, eds J.-M. Mérillon and K. G. Ramawat (Cham: Springer International Publishing), 333–384.
Newsham, K. K. (2011). A meta-analysis of plant responses to dark septate root endophytes. New Phytol. 190, 783–793. doi: 10.1111/j.1469-8137.2010.03611.x
Nieto-Jacobo, M. F., Steyaert, J. M., Salazar-Badillo, F. B., Nguyen, D. V., Rostás, M., Braithwaite, M., et al. (2017). Environmental growth conditions of Trichoderma spp. Affects indole acetic acid derivatives, volatile organic compounds, and plant growth promotion. Front. Plant Sci. 8:102. doi: 10.3389/fpls.2017.00102
Otero-Blanca, A., Folch-Mallol, J. L., Lira-Ruan, V., del Rayo Sánchez Carbente, M., and Batista-García, R. A. (2018). “Phytoremediation and fungi: an underexplored binomial,” in Approaches in Bioremediation: The New Era of Environmental Microbiology and Nanobiotechnology Nanotechnology in the Life Sciences, eds R. Prasad and E. Aranda (Cham: Springer International Publishing), 79–95.
Paungfoo-Lonhienne, C., Rentsch, D., Robatzek, S., Webb, R. I., Sagulenko, E., Näsholm, T., et al. (2010). Turning the table: plants consume microbes as a source of nutrients. PLoS One 5:e11915. doi: 10.1371/journal.pone.0011915
Pérez-Miranda, S., Cabirol, N., George-Téllez, R., Zamudio-Rivera, L. S., and Fernández, F. J. (2007). O-CAS, a fast and universal method for siderophore detection. J. Microbiol. Methods 70, 127–131. doi: 10.1016/j.mimet.2007.03.023
Phanthavongsa, P., Chalot, M., Papin, A., Lacercat-Didier, L., Roy, S., Blaudez, D., et al. (2017). Effect of mycorrhizal inoculation on metal accumulation by poplar leaves at phytomanaged sites. Environ. Exp. Bot. 143, 72–81. doi: 10.1016/j.envexpbot.2017.08.012
Platt, R. S., and Thimann, K. V. (1956). Interference in salkowski assay of indoleacetic acid. Science 123, 105–106. doi: 10.1126/science.123.3186.105-a
Poveda, J., Hermosa, R., Monte, E., and Nicolás, C. (2019). Trichoderma harzianum favours the access of arbuscular mycorrhizal fungi to non-host Brassicaceae roots and increases plant productivity. Sci. Rep. 9, 1–11. doi: 10.1038/s41598-019-48269-z
R Development Core Team (2015). R: A Language and Environment for Statistical Computing. Vienna: R Foundation for Statistical Computing.
Rawat, R., and Tewari, L. (2011). Effect of abiotic stress on phosphate solubilization by biocontrol fungus Trichoderma sp. Curr. Microbiol. 62, 1521–1526. doi: 10.1007/s00284-011-9888-2
Rees, F., Sterckeman, T., and Morel, J. L. (2020). Biochar-assisted phytoextraction of Cd and Zn by Noccaea caerulescens on a contaminated soil: a four-year lysimeter study. Sci. Total Environ. 707:135654. doi: 10.1016/j.scitotenv.2019.135654
Reeves, R. D., Schwartz, C., Morel, J. L., and Edmondson, J. (2001). Distribution and metal-accumulating behavior of Thlaspi caerulescens and associated metallophytes in France. Int. J. Phytoremediat. 3, 145–172. doi: 10.1080/15226510108500054
Robinson, B. H., Leblanc, M., Petit, D., Brooks, R. R., Kirkman, J. H., and Gregg, P. E. H. (1998). The potential of Thlaspi caerulescens for phytoremediation of contaminated soils. Plant Soil 203, 47–56. doi: 10.1023/A:1004328816645
Rosenberg, E., and Zilber-Rosenberg, I. (2018). The hologenome concept of evolution after 10 years. Microbiome 6:78. doi: 10.1186/s40168-018-0457-9
Rothen, C. P., Miranda, M. V., Fracchia, S., Godeas, A. M., and Rodriguez, M. A. (2018). Microdochium bolleyi (Ascomycota: Xylariales): physiological characterization and structural features of its association with wheat. Bol. Soc. Argent. Bot. 53, 169–182.
Rozpądek, P., Domka, A., Ważny, R., Nosek, M., Jędrzejczyk, R., Tokarz, K., et al. (2018). How does the endophytic fungus Mucor sp. improve Arabidopsis arenosa vegetation in the degraded environment of a mine dump? Environ. Exp. Bot. 147, 31–42. doi: 10.1016/j.envexpbot.2017.11.009
Saikkonen, K., Wäli, P., Helander, M., and Faeth, S. H. (2004). Evolution of endophyte–plant symbioses. Trends Plant Sci. 9, 275–280. doi: 10.1016/j.tplants.2004.04.005
Sessitsch, A., Kuffner, M., Kidd, P., Vangronsveld, J., Wenzel, W. W., Fallmann, K., et al. (2013). The role of plant-associated bacteria in the mobilization and phytoextraction of trace elements in contaminated soils. Soil Biol. Biochem. 60, 182–194. doi: 10.1016/j.soilbio.2013.01.012
Shadmani, L., Jamali, S., and Fatemi, A. (2021). Effects of root endophytic fungus, Microdochium bolleyi on cadmium uptake, translocation and tolerance by Hordeum vulgare L. Biologia 76, 711–719. doi: 10.2478/s11756-020-00598-5
Sharma, V. K., Li, X., Wu, G., Bai, W., Parmar, S., and White, J. F. Jr., et al. (2019). Endophytic community of Pb-Zn hyperaccumulator Arabis alpina and its role in host plants metal tolerance. Plant Soil 437, 397–411. doi: 10.1007/s11104-019-03988-0
Sterckeman, T., Cazes, Y., Gonneau, C., and Sirguey, C. (2017). Phenotyping 60 populations of Noccaea caerulescens provides a broader knowledge of variation in traits of interest for phytoextraction. Plant Soil 418, 523–540. doi: 10.1007/s11104-017-3311-0
Sterckeman, T., Cazes, Y., and Sirguey, C. (2019). Breeding the hyperaccumulator Noccaea caerulescens for trace metal phytoextraction: first results of a pure-line selection. Int. J. Phytoremediat. 21, 448–455. doi: 10.1080/15226514.2018.1537250
Su, C., Jiang, L., and Zhang, W. (2014). A Review on Heavy Metal Contamination in the Soil Worldwide: Situation, Impact and Remediation Techniques. Available online at: https://agris.fao.org/agris-search/search.do?recordID=CN2014200043 (accessed on 10 March 2021).
Tallapragada, P., and Gudimi, M. (2011). Phosphate solubility and biocontrol activity of Trichoderma harzianum. Turk. J. Biol. 35, 593–600.
Thijs, S., Sillen, W., Rineau, F., Weyens, N., and Vangronsveld, J. (2016). Towards an enhanced understanding of plant–microbiome interactions to improve phytoremediation: engineering the metaorganism. Front. Microbiol. 7:341. doi: 10.3389/fmicb.2016.00341
Visioli, G., D’Egidio, S., and Sanangelantoni, A. M. (2015a). The bacterial rhizobiome of hyperaccumulators: future perspectives based on omics analysis and advanced microscopy. Front. Plant Sci. 5:752. doi: 10.3389/fpls.2014.00752
Visioli, G., D’Egidio, S., Vamerali, T., Mattarozzi, M., and Sanangelantoni, A. M. (2014). Culturable endophytic bacteria enhance Ni translocation in the hyperaccumulator Noccaea caerulescens. Chemosphere 117, 538–544. doi: 10.1016/j.chemosphere.2014.09.014
Visioli, G., Sanangelantoni, A. M., Conti, F. D., Bonati, B., Gardi, C., and Menta, C. (2019). Above and belowground biodiversity in adjacent and distinct serpentine soils. Appl. Soil Ecol. 133, 98–103. doi: 10.1016/j.apsoil.2018.09.013
Visioli, G., Vamerali, T., Mattarozzi, M., Dramis, L., and Sanangelantoni, A. M. (2015b). Combined endophytic inoculants enhance nickel phytoextraction from serpentine soil in the hyperaccumulator Noccaea caerulescens. Front. Plant Sci. 6:638. doi: 10.3389/fpls.2015.00638
Ważny, R., Rozpądek, P., Domka, A., Jędrzejczyk, R. J., Nosek, M., Hubalewska-Mazgaj, M., et al. (2021). The effect of endophytic fungi on growth and nickel accumulation in Noccaea hyperaccumulators. Sci. Total Environ. 768:144666. doi: 10.1016/j.scitotenv.2020.144666
Wheeler, D. L., Dung, J. K. S., and Johnson, D. A. (2019). From pathogen to endophyte: an endophytic population of Verticillium dahliae evolved from a sympatric pathogenic population. New Phytol. 222, 497–510. doi: 10.1111/nph.15567
White, J. F., Kingsley, K. L., Verma, S. K., and Kowalski, K. P. (2018). Rhizophagy cycle: an oxidative process in plants for nutrient extraction from symbiotic microbes. Microorganisms 6: 95. doi: 10.3390/microorganisms6030095
White, T. J., Bruns, T., Lee, S., and Taylor, J. (1990). Amplification and direct sequencing of fungal ribosomal RNA genes for phylogenetics. PCR Protoc. Guide Methods Appl. 18, 315–322.
Yedidia, I., Benhamou, N., and Chet, I. (1999). Induction of defense responses in cucumber plants (Cucumis sativus L.) by the biocontrol agent Trichoderma harzianum. Appl. Environ. Microbiol. 65, 1061–1070. doi: 10.1128/AEM.65.3.1061-1070.1999
Yedidia, I., Srivastva, A. K., Kapulnik, Y., and Chet, I. (2001). Effect of Trichoderma harzianum on microelement concentrations and increased growth of cucumber plants. Plant Soil 235, 235–242. doi: 10.1023/A:1011990013955
Yung, L., Bertheau, C., Tafforeau, F., Zappelini, C., Valot, B., Maillard, F., et al. (2021a). Partial overlap of fungal communities associated with nettle and poplar roots when co-occurring at a trace metal contaminated site. Sci. Total Environ. 782:146692. doi: 10.1016/j.scitotenv.2021.146692
Yung, L., Blaudez, D., Maurice, N., Azou-Barré, A., and Sirguey, C. (2021b). Dark septate endophytes isolated from non-hyperaccumulator plants can increase phytoextraction of Cd and Zn by the hyperaccumulator Noccaea caerulescens. Environ. Sci. Pollut. Res. 28, 1–14. doi: 10.1007/s11356-020-11793-x
Zhao, F. J., Lombi, E., and McGrath, S. P. (2003). Assessing the potential for zinc and cadmium phytoremediation with the hyperaccumulator Thlaspi caerulescens. Plant Soil 249, 37–43. doi: 10.1023/A:1022530217289
Keywords: hyperaccumulator, inoculation, plant-fungus interaction, plant growth promotion, trace elements
Citation: Yung L, Sirguey C, Azou-Barré A and Blaudez D (2021) Natural Fungal Endophytes From Noccaea caerulescens Mediate Neutral to Positive Effects on Plant Biomass, Mineral Nutrition and Zn Phytoextraction. Front. Microbiol. 12:689367. doi: 10.3389/fmicb.2021.689367
Received: 31 March 2021; Accepted: 03 June 2021;
Published: 06 July 2021.
Edited by:
Markus Puschenreiter, University of Natural Resources and Life Sciences Vienna, AustriaReviewed by:
Piotr Rozpądek, Jagiellonian University, PolandLonghua Wu, Institute of Soil Science, Chinese Academy of Sciences, China
Rafael Clemente, Department of Soil and Water Conservation and Organic Waste Management, CEBAS-CSIC, Spain
Copyright © 2021 Yung, Sirguey, Azou-Barré and Blaudez. This is an open-access article distributed under the terms of the Creative Commons Attribution License (CC BY). The use, distribution or reproduction in other forums is permitted, provided the original author(s) and the copyright owner(s) are credited and that the original publication in this journal is cited, in accordance with accepted academic practice. No use, distribution or reproduction is permitted which does not comply with these terms.
*Correspondence: Damien Blaudez, ZGFtaWVuLmJsYXVkZXpAdW5pdi1sb3JyYWluZS5mcg==