Short Term Usage of Omega-3 Polyunsaturated Fatty Acids Ameliorate Lipopolysaccharide-Induced Inflammatory Response and Oxidative Stress in the Neonatal Rat Hippocampal Tissue
- 1Henan Key Laboratory of Neurorestoratology, Department of Neonatology, The First Affiliated Hospital of Xinxiang Medical University, Weihui, China
- 2The First Affiliated Hospital of Wenzhou Medical University, Zhejiang, Wenzhou, China
- 3The Second Affiliated Hospital of Wenzhou Medical University, Zhejiang, Wenzhou, China
Objective: To investigate the effect of omega-3 polyunsaturated fatty acids (ω-3 PUFAs) on lipopolysaccharide (LPS)-induced inflammatory response and oxidative stress in neonatal rat brain.
Methods: Ninety-six 3-day-old Sprague Dawley rats were divided into four groups: control (saline/saline), LPS/ω-3, LPS/ω-6, and LPS/saline (n = 24/group). All rats, except those in the control group, were intraperitoneally challenged once with LPS (0.6 mg/kg) and were treated with ω-3 PUFAs, ω-6 PUFAs, or saline at 15 mL/kg for 1 or 5 consecutive days beginning on the day of LPS-challenge. Rats in the control group underwent the same procedures and received saline (vehicle). After 1 or 5 days of treatment, 12 rats from each group were sacrificed and their hippocampuses were collected. The expression of inflammation-related genes as well as the levels of oxidative stress markers in hippocampal tissues were determined.
Results: After 1 or 5 days of treatment, the expression of toll-like receptor 4 and multiple proinflammatory cytokines were significantly decreased in the LPS/ω-3 group compared with those in the LPS/saline group. The activities of superoxide dismutase and glutathione (GSH) were significantly elevated, whereas amounts of malondialdehyde and oxidized glutathione (GSSG) and the ratio of GSSG/GSH were remarkably lowered in the LPS/ω-3 group compared with those in the LPS/saline group after 1 day of treatment. Opposite effects were observed in the LPS/ω-6 group.
Conclusion: ω-3 PUFAs may protect rat brain tissue against LPS-induced inflammatory response and oxidative stress.
Introduction
Preterm birth, a leading cause of neonatal mortality, remains an important public health challenge worldwide. Approximately 15 million infants are born preterm each year, accounting for 11% of all pregnancies (1, 2). It is estimated that one-third of preterm birth survivors suffer from long-term neurological disabilities (3), such as palsy, mental retardation, epilepsy, which are majorly attributed to neonatal infection-induced cerebral injury (4). The most common form of brain injury in premature birth survivors is white matter injury (WMI), which is defined by degeneration of preoligodendrocytes (5). It is well-established that preoligodendrocytes are particularly vulnerable to oxidative stress and inflammation, which are the two major mechanisms underlying the injury and death of preoligodendrocytes (3, 6). Thus, targeting oxidative stress and inflammation, in conjunction with antimicrobial agents, is an important adjuvant strategy that could be employed to prevent or ameliorate WMI in neonatal infections (7–9).
Lipopolysaccharide (LPS), a major cell wall component of Gram-negative bacteria, has been widely used to model various brain diseases in rodents (10–13). As an inflammation inducer (14), LPS initiates inflammation through binding to toll-like receptor 4 (TLR4), which induces translocation of nuclear factor kappa B (NF-κB) into nuclei, leading to the release of various proinflammatory cytokines, including tumor necrosis factor-alpha (TNF-α), interleukin-1β (IL-1β), and IL-6 (15–17). In addition, LPS has been shown to induce oxidative damage in various diseases (18, 19). In causing brain disorders, LPS can stimulate reactive oxygen species (ROS) formation, resulting in significant alteration in the levels of superoxide dismutase (SOD), malondialdehyde (MDA), glutathione (GSH), and oxidized glutathione (GSSG) in the brain (13, 19). Previous studies have indicated that a single dose of systemic LPS is adequate to induce the production of inflammatory cytokines and alter the levels of oxidative stress markers in the prefrontal cortex and hippocampus of murine models (20–22).
Unlike saturated and monounsaturated fatty acids that can be synthesized in the liver, omega-3 (ω-3) and omega-6 (ω-6) polyunsaturated fatty acids (PUFAs) are considered essential FAs in the human diet derived, respectively, from alpha-linolenic acid (ALA, 18:3 ω-3) and linoleic acid (LA, 18:2 ω-6) (23). Both of them are routinely used as a part of intravenous nutrition in preterm infants to meet their nutritional needs (24). ω-3 PUFAs consisting of eicosapentaenoic acid (EPA) and docosahexaenoic acid (DHA) are primarily derived from fish oil. Dietary supplementation of ω-3 PUFAs has been found to exert anti-inflammatory effects against a number of inflammatory diseases, such as osteoarthritis and inflammatory bowel disease (25, 26). A previous study showed that ω-3 PUFAs can reduce the secretion of pulmonary inflammatory factors (TNF-α, IL-1β, and IL-6) in rats with acute lung injury through suppressing the TLR4/NF-κB signaling pathway. By contrast, ω-6 PUFAs, richer in the Western diet, comprise (conjugated) LA, gamma-linolenic acid, and arachidonic acid (ARA) (23). ARA is a precursor to a number of potent pro-inflammatory mediators including well described prostaglandins and leukotrienes (23). It is generally believed that ω-3 PUFAs lead to the production of anti-inflammatory eicosanoids, decosanoid neuroprotectins, and resolvins while the longer ω-6 fatty acid ARA tends to the generation of proinflammatory eicosanoids (leukotriene, prostaglandin and thromboxane) (27).
However, little is known about the effect of ω-3 PUFAs in infection-induced inflammation and oxidative stress in neonatal brain (28–30). In the present study, we examined and compared the effect of ω-3 PUFAs on LPS-induced inflammation and oxidative stress in the hippocampal tissue of 3-day-old neonatal rats (31). The expression of the TLR4/NF-κB signaling and downstream proinflammatory cytokines as well as the amounts of oxidative stress markers were measured in the hippocampal tissue. Our findings provided new insights into the potential benefit of ω-3 PUFAs in preventing and alleviating infection-triggered brain injury in preterm infants.
Materials and Methods
Animals
A total of 96 neonatal (3-day-old) Sprague Dawley (SD) rats were provided by and reared in the Center of Laboratory Animal Science at Xinxiang Medical university (Xinxiang, Henan, China). Rats maintained at 25°C under a 12-h light/12-h dark cycle were randomly divided into four groups: control (saline/saline), LPS/saline, LPS/ω-3, and LPS/ω-6 (n = 24/group). All rats, except those in the control group, were intraperitoneally (i.p.) injected with 0.6 mg/kg LPS (Escherichia coli serotype O111:B4, Sigma-Aldrich, USA) to induce inflammation and oxidative stress as previously described (17). The rats were either treated with sterile saline, 10% ω-3 PUFAs (100 mL contains: highly refined fish oil 10.0 g, EPA 1.25–2.82 g, DHA 1.44–3.09 g, myristic acid 0.1–0.6 g, palmitic acid 0.25–1.0 g, palmitoleic acid 0.3–0.9 g, stearic acid 0.05–0.2 g, oleic acid 0.6–1.3 g, linoleic acid 0.1–0.7 g, linolenic acid ≤ 0.2 g, octadecatetraenoic acid 0.05–0.4 g, eicosaenoic acid 0.05–0.3 g, ARA 0.1–0.4 g, docosaenoic acid ≤ 0.15 g, docosapentaenoic acid 0.15–0.45 g, dl-α-Tocopherol 0.015–0.0296 g), or 20% ω-6 PUFAs [Sigma-Aldrich, USA, 100 mL contains: soy bean oil 10 g (content of essential fatty acids: Linoleic acid 4.38–5.86 g; α-Linolenic acid 0.45–1.1 g); Glycerol 2.5 g; Phospholipids from egg 0.6 g] via i.p. injection at 15 mL/kg/day for 1 or 5 consecutive days, beginning on the day of LPS-challenge. Rats in the control group underwent the same procedure and received the same volume of saline as vehicle (29). Twelve rats in each group were chosen randomly and sacrificed at 1 or 5 days after treatment, respectively. The hippocampal tissues were immediately collected, weighed, and stored at −80°C until use. This study was approved by and all animal procedures were conducted in accordance with the Animal Care and Use Committee of Health Guide for the Care and Use of Laboratory Animals.
Polymerase Chain Reaction (RT-PCR)
Total RNA was extracted from the hippocampal tissue using TRIzol reagent (Invitrogen, Carlsbad, CA, USA) according to the manufacturer's instructions. One μg of total RNA was converted to cDNA using a reverse transcription kit (Promega, Madison, WI, USA, No. A3500) following the manufacturer's instructions. PCR amplification was carried out using Taq DNA polymerase (Takara, Tokyo, Japan) in a thermal cycler (580BR, Bio-Rad, Hercules, CA, USA). The primers (Table 1) were designed and synthesized by Sangon Biological Engineering Technology & Services Co., Ltd. (Shanghai, China) based on sequences from GenBank. Each reaction started at 95°C for 5 min, amplified with 35 cycles of 30 s at 94°C, 30 s at the annealing temperature, and 60 s at 72°C, and ended with 10 min of extension at 72°C. The annealing temperatures for TLR4, NF-κB, TNF-α, IL-1β, IL-6, and GAPDH (β-actin) were 55, 61, 60, 65.5, 60, and 51°C, respectively. Then, 7.5 μL of each PCR product was subject to electrophoresis on a 1% agarose gel, and the density of each band was in a double-blinded manner analyzed on a gel image analysis system. The mRNA level of TLR4, NF-κB, TNF-α, IL-1β, or IL-6 was respectively normalized and determined based on the following density relative to the β-actin mRNA.
Western Blot Analysis
Protein lysates were obtained from hippocampal tissue using a protein extract kit (Active Motif, Tokyo, Japan) according to the manufacturer's protocols. Thirty micrograms proteins were separated on 7.5% SDS-PAGE gel, and then transferred onto 0.45 μm PVDF membranes (Bio-Rad). The membranes were blocked with 5% nonfat milk in Tris-buffered saline containing 0.1% Tween 20 (TBST) for 2 h and then incubated overnight at 4°C with rabbit polyclonal antibody against mouse TLR4, NF-κB, TNF-α, IL-1β, or IL-6 (Cell Signaling Technology, USA). After washing with TBST 3 times at room temperature for 10 min each, the membranes were incubated with a horseradish peroxidase (HRP)-conjugated secondary antibody (1:2,000, Cell Signaling Technology) for 1.5 h. After 3 washes with TBST, immunoreactive bands were visualized with an electrochemiluminescence reagent (AmerControl, Uppsala, Sweden). GAPDH (Cell Signaling Technology, USA) were used as protein controls to normalize protein expression levels. Densitometric quantification of the protein bands was performed using Image Lab software (Bio-Rad).
Biochemical Measurements
The amounts/enzymatic activity of SOD, MDA, GSSG, and GSH were determined using commercial kits (Jiancheng Bioengineering Institute, Nanjing, China), respectively; GSH and GSSG levels were also measured using additional kits from Cayman Chemical, USA.
Statistical Analysis
Data were expressed as the mean ± standard deviation and analyzed using SPSS 20.0 for Windows (IBM, Armonk, NY, USA). Statistical analysis was carried out using one-way analysis of variance for each time point. Comparisons among groups were conducted using Tukey-Kramer, and verified by using Bonferroni post-hoc test with P-values expressed in the following results. A value of P < 0.05 was considered significant.
Results
ω-3 PUFAs Downregulate the Expression of Inflammation-Related Genes and Proteins in Neonatal Rat Hippocampal Tissues
To determine whether ω-3 plays a role in the modulation of inflammation induced by LPS, we determined the expression of the TLR4/NF-κB signaling and downstream proinflammatory cytokines in hippocampal tissues of rats. As shown in Figure 1, LPS challenge unanimously and significantly increased the mRNA levels of TLR4 (A), NF-κB (B), TNF-α (C), IL-1β (D), and IL-6 (E) compared with the control group at both timepoints (day 1 or day 5 after treatment). Importantly, the mRNA levels of these inflammation-related genes were markedly decreased in the LPS/ω-3 group when compared with the LPS/saline group. By contrast, opposite effects were observed in the LPS/ω-6 group (Figures 1A–E). Similar trends were found in the protein levels of these genes (Figures 2A–G).
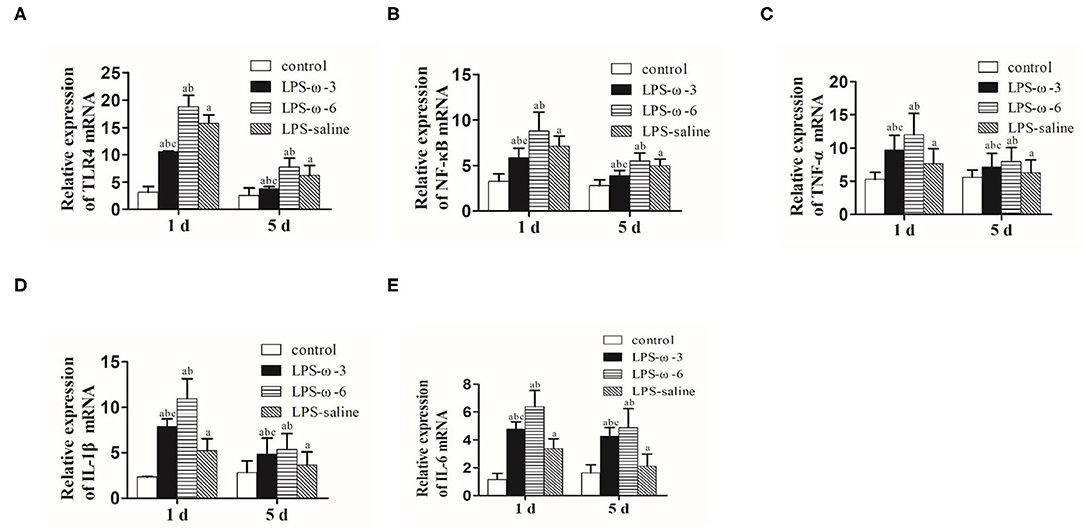
Figure 1. mRNA expression of inflammation-related genes. PCR was performed to determine the mRNA expression of TLR4 (A), NF-κB (B), TNF-α (C), IL-1β (D), and IL-6 (E) in the hippocampus of neonatal rats. Data are expressed as mean ± standard deviation (SD). aP < 0.05 vs. the control group, bP < 0.05 vs. the LPS group, cP < 0.05 vs. the ω-6 group; n = 12. LPS, lipopolysaccharide; NF-κB, nuclear factor kappa B; TNF-α, tumor necrosis factor-α; IL-1β, interleukin-1β; IL-6, interleukin-6.
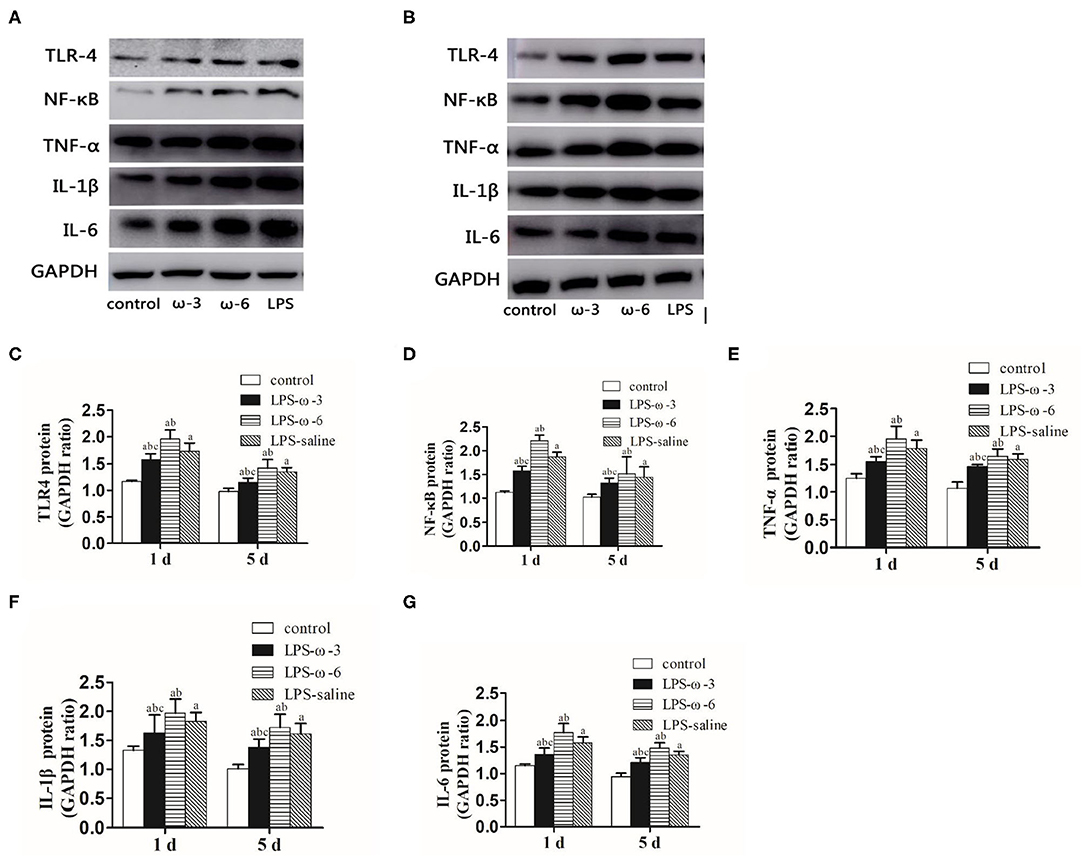
Figure 2. Protein expression of inflammation-related genes. (A) Western blot results showing the TLR4, NF-κB, TNF-α, IL-1β, and IL-6 proteins in the hippocampus in neonatal rats from the four groups 1 day after the intraperitoneal injection of drugs. (B) Western blot results showing the TLR4, NF-κB, TNF-α, IL-1β, and IL-6 proteins in the hippocampus in neonatal rats from the four groups 5 days after the intraperitoneal injection of drugs. Western blot analysis was performed to determine the protein expression of TLR4 (C), NF-κB (D), TNF-α (E), IL-1β (F), and IL-6 (G) protein expression in the hippocampus in neonatal rats from the different groups. The data points represent the mean ± SD; n = 12 (aP < 0.05 compared with the control group; bP < 0.05 compared with the LPS group; cP < 0.05 compared with the ω-6 group).
ω-3 PUFAs Reduce the Alterations of Oxidative Stress Markers in Neonatal Rat Hippocampal Tissues
To explore the effect of ω-3 PUFAs on LPS-induced oxidative stress, we measured the levels of oxidative stress markers in neonatal rat hippocampal tissues. As shown in Figure 3, ω-3 treatment significantly elevated the enzymatic activities of SOD (A) and GSH (C) but remarkably reduced the amounts of MDA (B) and GSSG (D) as well as the ratio of GSSG/GSH (E) compared to the LPS/saline group after 1 day of treatment. Opposite effects were observed in the LPS-ω-6 group at this timepoint (Figures 3A–E). However, no significant change of oxidative stress markers was found after 5 days of treatment.
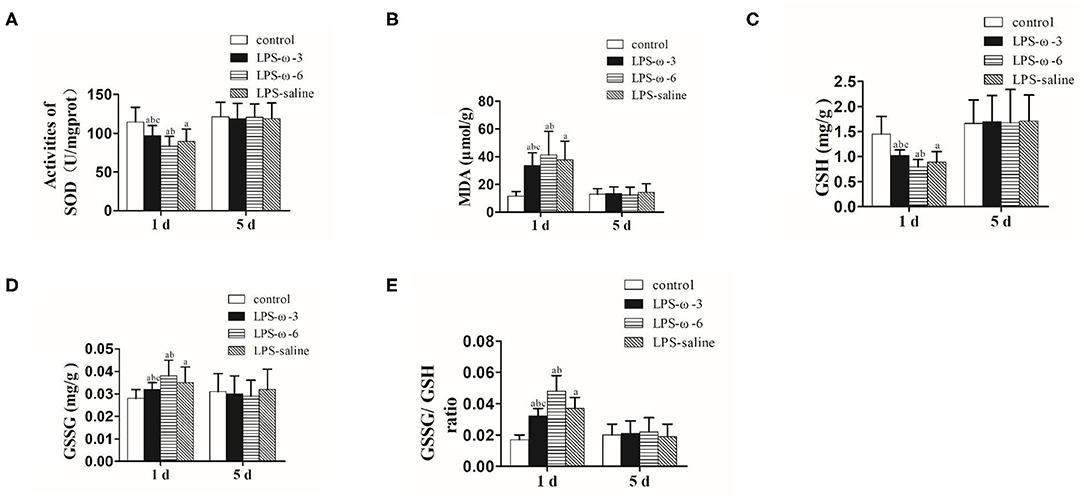
Figure 3. The amounts of SOD (A), MDA (B), GSH (C), GSSG (D), and GSSG/GSH (E) in the hippocampal tissues from the different groups. The data points represent the mean ± SD; n = 12 (aP < 0.05 compared with the control group; bP < 0.05 compared with the LPS group; cP < 0.05 compared with the ω-6 group). SOD, superoxide dismutase; MDA, malondialdehyde; GSH, glutathione; GSSG, oxidized glutathione.
Discussion
The present study aimed to evaluate the neuroprotective effect of ω-3 PUFAs on LPS-induced inflammation and oxidative damage in the hippocampus of neonatal rats. We found that TLR4/NF-κB-mediated expression of proinflammatory cytokines and the level of oxidative damage in the hippocampus were significantly enhanced in response to systemic LPS administration. Treatment with ω-3 PUFAs improved all these parameters in the hippocampus, suggesting that ω-3 PUFAs may protect neonatal brain against infection-induced inflammation and oxidative stress. Viewing that DHA and EPA have shown neuroprotection against brain injury (32, 33), memory impairment (34, 35) and motor malfunction (36) in ischemic rats and mice, our next goal is to decipher the contribution of the major components of ω-3 PUFAs to neuroprotection in our LPS-challenge rat model.
In the present study, we used a single i.p. injection with LPS to trigger activation of inflammatory signaling and oxidative stress in the brain of neonatal rats. Our results showed that all the expression of TLR-4, NF-κB, and downstream proinflammatory factors, including TNF-α, IL-1β, and IL-6, was significantly upregulated in the hippocampal tissues in the LPS/saline group compared with the control group beginning on the day of LPS-challenge and continuing for 5 days. On day 1 or day 5 after LPS application, the TLR4, NF-κB, TNF-α, IL-1β, and IL-6 mRNA expression levels were significantly higher in the LPS group, the ω-6 group and the ω-3 group than in the control group (P < 0.05). The mRNA levels of TLR4, NF-κB, TNF-α, IL-1β and IL-6 in the ω-3 group were lower than those in the ω-6 group on days 1 and 5 (all P < 0.05). In contrast, the TLR4, NF-κB, TNF-α, IL-1β, and IL-6 mRNA expression levels in hippocampal tissues were higher in the ω-6 group than in the LPS group on days 1 and 5 (P < 0.05). These data suggest that ω-3 PUFAs, but not ω-6 PUFAs, may inhibit LPS-induced inflammatory response in neonatal rat brain.
Similarly, the activities of SOD and GSH were significantly decreased, whereas the amounts of MDA and GSSG were noticeably elevated in the LPS/saline group compared with the control group on the same day of LPS treatment. These results suggest that single systemic LPS administration is adequate to induce short-lasting inflammation response and oxidative stress in the neonatal rat brain, which is consistent with previous reports (20–22, 37–42). The activities of SOD and GSH in the hippocampal tissues were significantly higher in the ω-3 group than in the ω-6 and LPS groups on day 1 (both P < 0.05). Further, the amounts of MDA and GSSG in the hippocampus in the ω-3 group were significantly lower than those in the ω-6 and LPS groups on day 1 (both P < 0.05). In addition, the ratios of GSSG to GSH in the hippocampal tissues were significantly lower in the ω-3 group than in the ω-6 and LPS groups on day 1 (both P < 0.05). However, on day 5, the activity of SOD and GSH and the amounts of MDA and GSSG in the hippocampal tissues from the four groups were not significantly different (P > 0.05). These results suggest that ω-3 PUFAs may reduce oxidative stress induced by LPS in neonatal rat brain.
Both ω-3 and ω-6 PUFAs are essential FAs in the human diet (24). Accumulating evidence has demonstrated that ω-3 PUFAs are beneficial in a multitude of diseases, including autoimmune disorders, inflammatory diseases, and heart disease (43–47). The anti-inflammatory properties of ω-3 PUFAs are well-characterized in chronic inflammation-associated disorders, such as obesity, rheumatoid arthritis, coronary heart disease, and Crohn's disease (48–50). A diet rich in ω-6 PUFAs produce proinflammatory eicosanoids, whereas ω-3 PUFAs are able to decrease the production of proinflammatory cytokines and eicosanoids in various tissues and cells (51). Consistently, in this study, we observed that ω-6 PUFAs treatment further enhanced the expression of the TLR4/NF-κB signaling and downstream inflammation mediators induced by LPS. By contrast, ω-3 PUFAs exhibited anti-inflammatory effects based on their ability to inhibit the TLR4/NF-κB signaling, thereby decreasing the production of proinflammatory cytokines. Because proinflammatory cytokines, such as TNF-α and IL-1β, exert toxic effects on brain tissues through activating microglia and astrocytes and promoting accumulation of neutrophils, monocytes and lymphocytes (52, 53), our results suggest that ω-6 PUFAs may exacerbate, whereas ω-3 PUFAs may improve LPS-induced neuronal damage in newborn rats (31, 54, 55), However, we did not directly show the pathological alterations occurred in the rat brain (56) in this study, which should be addressed in the future.
In addition, macrophages and other immune cells generate excessive ROS upon exposure to LPS, resulting in decreased antioxidant capacity, overactive lipid peroxidation, and a disrupted redox balance (57). Premature brain is particularly vulnerable to redox imbalance (58, 59). To evaluate the effect of ω-3 PUFAs on LPS-induced oxidative damage in neonatal rat brain, we determined the amount of multiple oxidative stress markers in the hippocampus. The activities of SOD and GSH, which are important compounds for free radical removal, represent the state of the antioxidant system. On the other hand, products of oxidation processes, such as MDA and GSSG, are indirect indicators of the severity of oxidative damage (60). In this study, we observed that the SOD and GSH expression in the hippocampus of newborn rats were significantly decreased, whereas the amount of MDA and GSSG and the ratio of GSSG/GSH were remarkably increased in the LPS/ω-3 group compared with the LPS-saline group on the same day of LPS-challenge, suggesting that ω-3 PUFAs may reduce the damage caused by oxidative stress products in brain tissue. Opposite effects were observed in the LPS/ω-6 group, suggesting that ω-6 PUFAs may aggravate infection-induced neuronal injury. Although significant changes in inflammation-related genes lasted for at least 5 days after LPS-challenge, no significant difference was observed in the oxidative markers on the fifth day after LPS application. This finding suggest that inflammation and oxidative damage are not entirely correlated in the context of LPS, which requires further investigation.
Although the interaction between inflammation (their lipid mediator derivatives) and PUFAs is complex and poorly understood, EPA and DHA in ω-3 PUFAs are generally regarded to be anti-inflammatory and neuroprotective, to promote resolution of inflammation and to decrease pain in inflammatory conditions (36). By contrast, ω-6 PUFAs, including ARA, produce not only pro-inflammatory eicosanoids, but also lipid mediators that play an important role in inflammation resolution (61).
Hormesis, as elegantly explained in reviews (62), is featured with a biphasic dose response pattern (i.e., low dose stimulation and high dose inhibition). Although ω-3 PUFAs is extensively studied (44) and some showed a dose-dependent effect (63), it is so far not investigated in a specific hormetic approach as for Ginkgo biloba extract components (64) and some polyphenols (65, 66). Future study should be designed to explore its hermetic potential. Although ω-3 PUFAs modulated the transcription and translation of some vitagenes (67–69) in our animal model, such as SOD and GSSG/GSH, its antioxidant role in humans remains to be seriously tested.
Some of the drawbacks of the current study are that only one dose of each PUFAs and one age group of rats were used, one cannot possibly see any dosage effect required to observe hormesis (62) and link age with LPS susceptibility and PUFAs treatment, and only hippocampal tissues were collected, limiting opportunity to observe the systemic effect of LPS and PUFAs. Indeed, systemic LPS challenge could affect organs (kidney, lung, liver, and brain) to a different degree via different molecular mechanisms (70), and age is a critical factor to skew cytokine production pattern upon LPS treatment (71). Future work preferably should use a more elegant and sophisticated strategy to expand the current study.
Conclusion
In conclusion, ω-3 PUFA supplementation may have neuroprotective effects against LPS-induced inflammation and oxidative damage in neonatal rats through downregulating the expression of TLR4/NF-κB-mediated proinflammatory cytokines and reducing oxidative stress, respectively. This finding may provide ω-3 PUFAs as potential therapeutic agents in protecting or alleviating infection-induced neonatal brain injury in preterm infants.
Data Availability Statement
The datasets presented in this study can be found in online repositories. The names of the repository/repositories and accession number(s) can be found in the article/Supplementary Material.
Ethics Statement
The animal study was reviewed and approved by Wenzhou Medical University, wydw2019-0501.
Author Contributions
All authors listed have made a substantial, direct and intellectual contribution to the work and approved it for publication.
Conflict of Interest
The authors declare that the research was conducted in the absence of any commercial or financial relationships that could be construed as a potential conflict of interest.
Acknowledgments
This work was supported by the Henan Key Laboratory of Neurorestoratology (HNSJXF-2018-009).
References
1. Howson CP, Kinney MV, McDougall L, Lawn JE. Born too soon: preterm birth matters. Reprod Health. (2013) 10(Suppl. 1):S1. doi: 10.1186/1742-4755-10-S1-S1
2. Blencowe H, Cousens S, Oestergaard MZ, Chou D, Moller AB, Narwal R, et al. National, regional, and worldwide estimates of preterm birth rates in the year 2010 with time trends since 1990 for selected countries: a systematic analysis and implications. Lancet. (2012) 379:2162–72. doi: 10.1016/S0140-6736(12)60820-4
3. Lawn JE, Cousens S, Zupan J. 4 million neonatal deaths: when? Where? Why? Lancet. (2005) 365:891–900. doi: 10.1016/S0140-6736(05)71048-5
4. Strunk T, Inder T, Wang X, Burgner D, Mallard C, Levy O. Infection-induced inflammation and cerebral injury in preterm infants. Lancet Infect Dis. (2014) 14:751–62. doi: 10.1016/S1473-3099(14)70710-8
5. Back SA. White matter injury in the preterm infant: pathology and mechanisms. Acta Neuropathol. (2017) 134:331–49. doi: 10.1007/s00401-017-1718-6
6. Marlow N, Wolke D, Bracewell MA, Samara M. Neurologic and developmental disability at six years of age after extremely preterm birth. N Engl J Med. (2005) 352:9–19. doi: 10.1056/NEJMoa041367
7. Congle Z. Understanding of encephalopathy of prematurity. J Clin Pediatr. (2015) 201–4. doi: 10.3969/j.issn.1000-3606.2015.03.001
8. Volpe JJ. Postnatal sepsis, necrotizing entercolitis, and the critical role of systemic inflammation in white matter injury in premature infants. J Pediatr. (2008) 153:160–3. doi: 10.1016/j.jpeds.2008.04.057
9. Dean JM, Shi Z, Fleiss B, Gunn KC, Groenendaal F, van Bel F, et al. A critical review of models of perinatal infection. Dev Neurosci. (2015) 37:289–304. doi: 10.1159/000370309
10. Medeiros IU, Ruzza C, Asth L, Guerrini R, Romao PR, Gavioli EC, et al. Blockade of nociceptin/orphanin FQ receptor signaling reverses LPS-induced depressive-like behavior in mice. Peptides. (2015) 72:95–103. doi: 10.1016/j.peptides.2015.05.006
11. Zhu F, Zhang L, Ding YQ, Zhao J, Zheng Y. Neonatal intrahippocampal injection of lipopolysaccharide induces deficits in social behavior and prepulse inhibition and microglial activation in rats: Implication for a new schizophrenia animal model. Brain Behav Immun. (2014) 38:166–74. doi: 10.1016/j.bbi.2014.01.017
12. Kirsten TB, Queiroz-Hazarbassanov N, Bernardi MM, Felicio LF. Prenatal zinc prevents communication impairments and BDNF disturbance in a rat model of autism induced by prenatal lipopolysaccharide exposure. Life Sci. (2015) 130:12–7. doi: 10.1016/j.lfs.2015.02.027
13. Sharma N, Nehru B. Characterization of the lipopolysaccharide induced model of Parkinson's disease: Role of oxidative stress and neuroinflammation. Neurochem Int. (2015) 87:92–105. doi: 10.1016/j.neuint.2015.06.004
14. Ramachandran G. Gram-positive and gram-negative bacterial toxins in sepsis: a brief review. Virulence. (2014) 5:213–8. doi: 10.4161/viru.27024
15. Yao L, Kan EM, Lu J, Hao A, Dheen ST, Kaur C, et al. Toll-like receptor 4 mediates microglial activation and production of inflammatory mediators in neonatal rat brain following hypoxia: role of TLR4 in hypoxic microglia. J Neuroinflammation. (2013) 10:23. doi: 10.1186/1742-2094-10-23
16. Yang S, Li R, Qu X, Tang L, Ge G, Fang W, et al. Fosinoprilat alleviates lipopolysaccharide (LPS)-induced inflammation by inhibiting TLR4/NF-kappaB signaling in monocytes. Cell Immunol. (2013) 284:182–6. doi: 10.1016/j.cellimm.2013.06.009
17. Gorina R, Font-Nieves M, Marquez-Kisinousky L, Santalucia T, Planas AM. Astrocyte TLR4 activation induces a proinflammatory environment through the interplay between MyD88-dependent NFkappaB signaling, MAPK, and Jak1/Stat1 pathways. Glia. (2011) 59:242–55. doi: 10.1002/glia.21094
18. Casedas G, Bennett AC, Gonzalez-Burgos E, Gomez-Serranillos MP, Lopez V, Smith C. Polyphenol-associated oxidative stress and inflammation in a model of LPS-induced inflammation in glial cells: do we know enough for responsible compounding? Inflammopharmacology. (2018) 27(Suppl. 5):189–97. doi: 10.1007/s10787-018-0549-y
19. Noworyta-Sokolowska K, Gorska A, Golembiowska K. LPS-induced oxidative stress and inflammatory reaction in the rat striatum. Pharmacol Rep. (2013) 65:863–9. doi: 10.1016/S1734-1140(13)71067-3
20. Sulakhiya K, Kumar P, Jangra A, Dwivedi S, Hazarika NK, Baruah CC, et al. Honokiol abrogates lipopolysaccharide-induced depressive like behavior by impeding neuroinflammation and oxido-nitrosative stress in mice. Eur J Pharmacol. (2014) 744:124–31. doi: 10.1016/j.ejphar.2014.09.049
21. Ho YH, Lin YT, Wu CW, Chao YM, Chang AY, Chan JY. Peripheral inflammation increases seizure susceptibility via the induction of neuroinflammation and oxidative stress in the hippocampus. J Biomed Sci. (2015) 22:46. doi: 10.1186/s12929-015-0157-8
22. Shukla R, Tyagi E, Kumar R. Protective effect of COX and NOS inhibitors on LPS induced oxidative stress in rat. Ann Neurosci. (2008) 15:6–10. doi: 10.5214/ans.0972.7531.2008.150102
23. Silva AR, Moraes BPT, Gonçalves-de-Albuquerque CF. Mediterranean diet: lipids, inflammation, and malaria infection. Int J Mol Sci. (2020) 21:4489. doi: 10.3390/ijms21124489
24. Salama GS, Kaabneh MA, Almasaeed MN, Alquran M. Intravenous lipids for preterm infants: a review. Clin Med Insights Pediatr. (2015) 9:25–36. doi: 10.4137/CMPed.S21161
25. Zainal Z, Longman AJ, Hurst S, Duggan K, Caterson B, Hughes CE, et al. Relative efficacies of omega-3 polyunsaturated fatty acids in reducing expression of key proteins in a model system for studying osteoarthritis. Osteoarthr Cartil. (2009) 17:896–905. doi: 10.1016/j.joca.2008.12.009
26. Simopoulos AP. Omega-3 fatty acids in inflammation and autoimmune diseases. J Am Coll Nutr. (2002) 21:495–505. doi: 10.1080/07315724.2002.10719248
27. Lewis MD, Bailes J. Neuroprotection for the warrior: dietary supplementation with omega-3 fatty acids. Mil Med. (2011) 176:1120–7. doi: 10.7205/MILMED-D-10-00466
28. Graham EM, Holcroft CJ, Rai KK, Donohue PK, Allen MC. Neonatal cerebral white matter injury in preterm infants is associated with culture positive infections and only rarely with metabolic acidosis. Am J Obstet Gynecol. (2004) 191:1305–10. doi: 10.1016/j.ajog.2004.06.058
29. Williams JJ, Mayurasakorn K, Vannucci SJ, Mastropietro C, Bazan NG, Ten VS, et al. N-3 fatty acid rich triglyceride emulsions are neuroprotective after cerebral hypoxic-ischemic injury in neonatal mice. PLoS ONE. (2013) 8:e56233. doi: 10.1371/journal.pone.0056233
30. Song Y, You Q, Wang Q, Tang C. Effect of omega-3 fish oil fat emulsion on the expression of Toll-like receptor 4 and nuclear factor-κB in the neonatal rats hippocampus after hypoxic-ischemic. Chin J Appl Clin Pediatr. (2016) 31:923–6. doi: 10.3760/cma.j.issn.2095-428X.2016.12.013
31. Hagberg H, Peebles D, Mallard C. Models of white matter injury: comparison of infectious, hypoxic-ischemic, and excitotoxic insults. Ment Retard Dev Disabil Res Rev. (2002) 8:30–8. doi: 10.1002/mrdd.10007
32. Pan HC, Kao TK, Ou YC, Yang DY, Yen YJ, Wang CC, et al. Protective effect of docosahexaenoic acid against brain injury in ischemic rats. J Nutr Biochem. (2009) 20:715–25. doi: 10.1016/j.jnutbio.2008.06.014
33. Chang CY, Kuan YH, Li JR, Chen WY, Ou YC, Pan HC, et al. Docosahexaenoic acid reduces cellular inflammatory response following permanent focal cerebral ischemia in rats. J Nutr Biochem. (2013) 24:2127–37. doi: 10.1016/j.jnutbio.2013.08.004
34. Okabe N, Nakamura T, Toyoshima T, Miyamoto O, Lu F, Itano T. Eicosapentaenoic acid prevents memory impairment after ischemia by inhibiting inflammatory response and oxidative damage. J Stroke Cerebrovasc Dis. (2011) 20:188–95. doi: 10.1016/j.jstrokecerebrovasdis.2009.11.016
35. Ueda M, Inaba T, Nito C, Kamiya N, Katayama Y. Therapeutic impact of eicosapentaenoic acid on ischemic brain damage following transient focal cerebral ischemia in rats. Brain Res. (2013) 1519:95–104. doi: 10.1016/j.brainres.2013.04.046
36. Gonzalo-Gobernado R, Ayuso MI, Sansone L, José Bernal-Jiménez J, Ramos-Herrero VD, Sánchez-García E, et al. neuroprotective effects of diets containing olive oil and DHA/EPA in a mouse model of cerebral ischemia. Nutrients. (2019) 11:1109. doi: 10.3390/nu11051109
37. Stoll BJ, Hansen NI, Adams-Chapman I, Fanaroff AA, Hintz SR, Vohr B, et al. Neurodevelopmental and growth impairment among extremely low-birth-weight infants with neonatal infection. JAMA. (2004) 292:2357–65. doi: 10.1001/jama.292.19.2357
38. Fan LW, Tien LT, Zheng B, Pang Y, Lin RC, Simpson KL, et al. Dopaminergic neuronal injury in the adult rat brain following neonatal exposure to lipopolysaccharide and the silent neurotoxicity. Brain Behav Immun. (2011) 25:286–97. doi: 10.1016/j.bbi.2010.09.020
39. Mallard C, Wang X. Infection-induced vulnerability of perinatal brain injury. Neurol Res Int. (2012) 2012:102153. doi: 10.1155/2012/102153
40. Chau V, Brant R, Poskitt KJ, Tam EW, Synnes A, Miller SP. Postnatal infection is associated with widespread abnormalities of brain development in premature newborns. Pediatr Res. (2012) 71:274–9. doi: 10.1038/pr.2011.40
41. Korzeniewski SJ, Romero R, Cortez J, Pappas A, Schwartz AG, Kim CJ, et al. A “multi-hit” model of neonatal white matter injury: cumulative contributions of chronic placental inflammation, acute fetal inflammation and postnatal inflammatory events. J Perinat Med. (2014) 42:731–43. doi: 10.1515/jpm-2014-0250
42. Biran V, Verney C, Ferriero DM. Perinatal cerebellar injury in human and animal models. Neurol Res Int. (2012) 2012:858929. doi: 10.1155/2012/858929
43. De Caterina R, Madonna R, Massaro M. Effects of omega-3 fatty acids on cytokines and adhesion molecules. Curr Atheroscler Rep. (2004) 6:485–91. doi: 10.1007/s11883-004-0090-x
44. Griffin MD, Sanders TA, Davies IG, Morgan LM, Millward DJ, Lewis F, et al. Effects of altering the ratio of dietary n-6 to n-3 fatty acids on insulin sensitivity, lipoprotein size, and postprandial lipemia in men and postmenopausal women aged 45–70 y: the OPTILIP Study. Am J Clin Nutr. (2006) 84:1290–98. doi: 10.1093/ajcn/84.6.1290
45. Capanni M, Calella F, Biagini MR, Genise S, Raimondi L, Bedogni G, et al. Prolonged n-3 polyunsaturated fatty acid supplementation ameliorates hepatic steatosis in patients with non-alcoholic fatty liver disease: a pilot study. Aliment Pharmacol Ther. (2006) 23:1143–51. doi: 10.1111/j.1365-2036.2006.02885.x
46. Donadio JV, Bergstralh EJ, Offord KP, Holley KE, Spencer DC. Clinical and histopathologic associations with impaired renal function in IgA nephropathy. Mayo Nephrology Collaborative Group. Clin Nephrol. (1994) 41:65–71.
47. Goldberg RJ, Katz J. A meta-analysis of the analgesic effects of omega-3 polyunsaturated fatty acid supplementation for inflammatory joint pain. Pain. (2007) 129:210–23. doi: 10.1016/j.pain.2007.01.020
48. White PJ, Marette A. Is omega-3 key to unlocking inflammation in obesity? Diabetologia. (2006) 49:1999–2001. doi: 10.1007/s00125-006-0346-9
49. Calder PC. n-3 polyunsaturated fatty acids, inflammation, and inflammatory diseases. Am J Clin Nutr. (2006) 83:1505s–1519s. doi: 10.1093/ajcn/83.6.1505S
50. Kromhout D. Omega-3 fatty acids and coronary heart disease. The final verdict? Curr Opin Lipidol. (2012) 23:554–9. doi: 10.1097/MOL.0b013e328359515f
51. Lombardo YB, Chicco AG. Effects of dietary polyunsaturated n-3 fatty acids on dyslipidemia and insulin resistance in rodents and humans. A review. J Nutr Biochem. (2006) 17:1–13. doi: 10.1016/j.jnutbio.2005.08.002
52. Girard S, Kadhim H, Roy M, Lavoie K, Brochu ME, Larouche A, et al. Role of perinatal inflammation in cerebral palsy. Pediatr Neurol. (2009) 40:168–74. doi: 10.1016/j.pediatrneurol.2008.09.016
53. Fan LW, Tien LT, Zheng B, Pang Y, Rhodes PG, Cai Z. Interleukin-1beta-induced brain injury and neurobehavioral dysfunctions in juvenile rats can be attenuated by alpha-phenyl-n-tert-butyl-nitrone. Neuroscience. (2010) 168:240–52. doi: 10.1016/j.neuroscience.2010.03.024
54. Fa LX, Z FL B. Q D, D JJ. Effects of bacterial lipopolysaccharide on brain development of neonatal mice at different maturity levels. J Appl Clin Pediatr. (2012) 27:611–13, 616.
55. Dammann O, Leviton A. Inflammatory brain damage in preterm newborns—dry numbers, wet lab, and causal inferences. Early Hum Dev. (2004) 79:1–15. doi: 10.1016/j.earlhumdev.2004.04.009
56. Berman DR, Mozurkewich E, Liu Y, Shangguan Y, Barks JD, Silverstein FS. Docosahexaenoic acid augments hypothermic neuroprotection in a neonatal rat asphyxia model. Neonatology. (2013) 104:71–8. doi: 10.1159/000351011
57. Dal-Pizzol F, Ritter C, Cassol OJ Jr, Rezin GT, Petronilho F, Zugno AI, et al. Oxidative mechanisms of brain dysfunction during sepsis. Neurochem Res. (2010) 35:1–12. doi: 10.1007/s11064-009-0043-4
58. Khan JY, Black SM. Developmental changes in murine brain antioxidant enzymes. Pediatr Res. (2003) 54:77–82. doi: 10.1203/01.PDR.0000065736.69214.20
59. Blomgren K, Hagberg H. Free radicals, mitochondria, and hypoxia-ischemia in the developing brain. Free Radic Biol Med. (2006) 40:388–97. doi: 10.1016/j.freeradbiomed.2005.08.040
60. Montuschi P, Barnes PJ, Roberts LJ II. Isoprostanes: markers and mediators of oxidative stress. FASEB J. (2004) 18:1791–800. doi: 10.1096/fj.04-2330rev
61. Innes JK, Calder PC. Omega-6 fatty acids and inflammation. Prostaglandins Leukot Essent Fatty Acids. (2018) 132:41–8. doi: 10.1016/j.plefa.2018.03.004
62. Calabrese V, Santoro A, Trovato Salinaro A, Modafferi S, Scuto M, Albouchi F, et al. Hormetic approaches to the treatment of Parkinson's disease: perspectives and possibilities. J Neurosci Res. (2018) 96:1641–62. doi: 10.1002/jnr.24244
63. DiNicolantonio JJ, Meier P, O'Keefe JH. Omega-3 polyunsaturated fatty acids for the prevention of cardiovascular disease: do formulation, dosage & comparator matter? Mo Med. (2013) 110:495–8.
64. Calabrese EJ, Calabrese V, Tsatsakis A, Giordano JJ. Hormesis and Ginkgo biloba (GB): numerous biological effects of GB are mediated via hormesis. Ageing Res Rev. (2020) 101019. doi: 10.1016/j.arr.2020.101019
65. Leri M, Scuto M, Ontario ML, Calabrese V, Calabrese EJ, Bucciantini M, et al. Healthy effects of plant polyphenols: molecular mechanisms. Int J Mol Sci. (2020) 21:1250. doi: 10.3390/ijms21041250
66. Di Rosa G, Brunetti G, Scuto M, et al. Healthspan enhancement by olive polyphenols in C. elegans wild type and Parkinson's models. Int J Mol Sci. (2020) 21:3893 doi: 10.3390/ijms21113893
67. V Cornelius C, Dinkova-Kostova AT, Calabrese EJ, Mattson MP. Cellular stress responses, the hormesis paradigm, and vitagenes: novel targets for therapeutic intervention in neurodegenerative disorders. Antioxid Redox Signal. (2010) 13:1763–811. doi: 10.1089/ars.2009.3074
68. Peters V, Calabrese V, Forsberg E, Volk N, Fleming T, Baelde H, et al. Protective actions of anserine under diabetic conditions. Int J Mol Sci. (2018) 19:2751. doi: 10.3390/ijms19092751
69. Cornelius C, Trovato Salinaro A, Scuto M, Fronte V, Cambria MT, Pennisi M, et al. Cellular stress response, sirtuins and UCP proteins in Alzheimer disease: role of vitagenes. Immun Ageing. (2013) 10:41. doi: 10.1186/1742-4933-10-41
70. Proniewski B, Kij A, Sitek B, Kelley EE, Chlopicki S. Multiorgan development of oxidative and nitrosative stress in LPS-induced endotoxemia in C57Bl/6 mice: DHE-based in vivo approach. Oxid Med Cell Longev. (2019) 2019:7838406. doi: 10.1155/2019/7838406
71. Belderbos ME, van Bleek GM, Levy O, Blanken MO, Houben ML, Schuijff L, et al. Skewed pattern of Toll-like receptor 4-mediated cytokine production in human neonatal blood: low LPS-induced IL-12p70 and high IL-10 persist throughout the first month of life. Clin Immunol. (2009) 133:228–37. doi: 10.1016/j.clim.2009.07.003
Keywords: omega-3 polyunsaturated fatty acids, lipopolysaccharide, proinflammatory cytokines, oxidative stress, neonatal rat brain
Citation: Shi J, wang W, Sang G, Xi H, Sun Y, Lu C, Ye H and Huang L (2020) Short Term Usage of Omega-3 Polyunsaturated Fatty Acids Ameliorate Lipopolysaccharide-Induced Inflammatory Response and Oxidative Stress in the Neonatal Rat Hippocampal Tissue. Front. Nutr. 7:572363. doi: 10.3389/fnut.2020.572363
Received: 14 June 2020; Accepted: 12 October 2020;
Published: 17 November 2020.
Edited by:
Vittorio Calabrese, University of Catania, ItalyReviewed by:
Ludmila Okruhlicova, Slovak Academy of Sciences (SAS), SlovakiaHercules Rezende Freitas, UC Davis, United States
Copyright © 2020 Shi, wang, Sang, Xi, Sun, Lu, Ye and Huang. This is an open-access article distributed under the terms of the Creative Commons Attribution License (CC BY). The use, distribution or reproduction in other forums is permitted, provided the original author(s) and the copyright owner(s) are credited and that the original publication in this journal is cited, in accordance with accepted academic practice. No use, distribution or reproduction is permitted which does not comply with these terms.
*Correspondence: Hezhen Ye, yehezhen@163.com; Limi Huang, hlm0201262@126.com