- 1Department of Obstetrics and Gynecology, Gynecologic Oncology Division, University of Virginia, Charlottesville, VA, United States
- 2Department of Pathology, University of Virginia, Charlottesville, VA, United States
- 3Department of Biomedical Engineering & Mechanics, Fralin Biomedical Research Institute, Virginia Polytechnic Institute and State University, Roanoke, VA, United States
- 4Department of Biomedical Engineering, University of Virginia, Charlottesville, VA, United States
- 5Department of Microbiology, Immunology, and Cancer Biology, University of Virginia, Charlottesville, VA, United States
- 6Department of Chemistry, University of Virginia, Charlottesville, VA, United States
- 7Department of Genetics & Genome Sciences, Lerner Research Institute, Cleveland, OH, United States
- 8Department of Pathology and Laboratory Medicine, Brown University, Providence, RI, United States
- 9Department of Pathology and Laboratory Medicine, Women & Infants Hospital of Rhode Island, Providence, RI, United States
- 10Department of Hematology and Oncology, University of Virginia, Charlottesville, VA, United States
Chemotherapy has been used to inhibit cancer growth for decades, but emerging evidence shows it can affect the tumor stroma, unintentionally promoting cancer malignancy. After treatment of primary tumors, remaining drugs drain via lymphatics. Though all drugs interact with the lymphatics, we know little of their impact on them. Here, we show a previously unknown effect of platinums, a widely used class of chemotherapeutics, to directly induce systemic lymphangiogenesis and activation. These changes are dose-dependent, long-lasting, and occur in healthy and cancerous tissue in multiple mouse models of breast cancer. We found similar effects in human ovarian and breast cancer patients whose treatment regimens included platinums. Carboplatin treatment of healthy mice prior to mammary tumor inoculation increased cancer metastasis as compared to no pre-treatment. These platinum-induced phenomena could be blocked by VEGFR3 inhibition. These findings have implications for cancer patients receiving platinums and may support the inclusion of anti-VEGFR3 therapy into treatment regimens or differential design of treatment regimens to alter these potential effects.
Summary
Platinum chemotherapy induces VEGFR3-dependent lymphangiogenesis, priming tissues for metastasis of breast cancer. Inhibition of VEGFR3 via antibody blockade can reverse these effects.
Introduction
Over 650,000 cancer patients receive chemotherapy in the United States every year, with platinums, taxanes, and anthracyclines representing the most common classes of drugs (1). Unfortunately, many of these patients suffer recurrence. In breast cancer, the second most common cause of cancer death in women in the US, mortality results from metastasis rather than primary tumor growth (2). Ovarian cancer is similarly deadly due to dissemination of tumor rather than initial growth (3). Despite the centrality of metastasis to patient outcomes, it remains unclear why tumors that appear to be controlled or even cleared by initial chemotherapy later recur.
Emerging evidence shows that chemotherapy can have off-target effects that may alter the tissue microenvironment in cancer-promoting ways. Chemotherapy can enrich for cancer stem cell populations (4), promote invasiveness (5), and induce epithelial-to-mesenchymal transition (EMT) to encourage drug resistance (6). Stromal cells such as fibroblasts and macrophages also take up chemotherapies resulting in cellular activation and increased cytokine secretion that can contribute to tumor progression (7, 8). Thus, chemotherapy has been linked to worsening disease via multiple mechanisms, and an understanding of how this occurs is essential to better treatment planning.
The majority of cancers show lymphangiogenesis in the peritumoral spaces of the tissue stroma. Chemotherapeutic drugs enter the tumor via blood vasculature and drain through the stroma into these peritumoral lymphatics. Similarly, tumor cells can metastasize from carcinomas via the lymphatics and downstream lymph nodes (9). Thus, the lymphatics represent a point of access for both tumor cells and chemotherapies to the rest of the body. Enlargement, sprouting, and proliferation of lymphatic vessels at both the primary tumor site (10, 11) and metastatic sites (12) are associated with increased cancer growth, metastasis, and poor prognosis (13). Increased lymphangiogenesis in tumors due to induction of vascular endothelial growth factor C (VEGF-C) promotes metastatic spread in melanoma, breast carcinoma, and colorectal cancer (13–15). Treatment of lymphangiogenic tumors with inhibitors of VEGF Receptor 3 (VEGFR3) to specifically attenuate proliferation and activation of lymphatic endothelial cells (LECs) inhibits lymph node and systemic metastasis in multiple models of cancer (16, 17).
Although chemotherapies pass through lymphatics, thus interacting with these gatekeeping vessels, little information is available on how chemotherapies affect them (16, 17). Taxanes have been shown to induce lymphangiogenesis in mice (17), and can promote metastatic spread of tumor cells. While taxanes are routinely incorporated in clinical regimens, they only represent one of multiple classes of chemotherapeutic drugs used in the treatment of cancer. Here we examine how the other major classes of chemotherapy impact the lymphatics, with an emphasis on platinum chemotherapy, which has not been independently studied in the context of lymphangiogenesis, though some studies have suggested potential non-lymphangiogenic effects at low doses (18).
Platinum chemotherapy is a common standard of care for multiple cancers and has shown efficacy in reducing primary disease in patients with breast or ovarian cancer (19, 20). However, overall survival and long term outcomes are not always affected by inclusion of platinum agents in treatment regimens, especially in subsets of patients with TNBC or recurrent ovarian cancers (20, 21). Though platinum resistance is a major contributor to cancer recurrence, other promoters of metastasis and tumor regrowth have the potential to impact disease.
Here, we investigate one contributor to metastatic spread and disease recurrence, lymphangiogenesis, after platinum therapy in cancerous and normal tissues in multiple contexts, including patients, murine models, in vitro, and ex vivo systems. Specifically, we examine the impact of platinum agents on lymphatic endothelial cells in normal tissues, outside of the context of cancer, to understand how these agents may be priming or altering the tissue microenvironment. We hypothesized that these changes may contribute to worsened disease outcomes due to metastatic spread, with a focus on breast cancer.
Results
Lymphatic Endothelial Cells Respond to Platinum Chemotherapy
To assess if chemotherapeutics directly affects lymphatic endothelial cells, we treated monolayers of human LECs with low doses of docetaxel, doxorubicin, or carboplatin. Breakdown of LEC junctions is associated with vessel permeability, enhancing opportunities for tumor cells to intravasate (22). We observed dramatic morphological changes to LECs that included large gaps between cells in previously confluent monolayers after treatment with carboplatin (Figures 1A, B), as well as other platinum agents, cisplatin and oxaliplatin (Figures S1A, B), but not with doxorubicin or docetaxel (Figures S1C, D). 95% of lymphatic endothelial cells exhibited junctional gaps with platinum treatment, compared to only 15% with vehicle treatment (Figure 1C). Additionally, the severity of this junctional breakdown increased substantially, with platinum-treated LECs displaying junctional gaps nearly 7 times the size of vehicle-treated control LECs (Figure 1D). Cellular adhesion molecules on LECs facilitate immune cell trafficking but are hijacked by tumor cells entering lymphatic vessels (23). LEC monolayers treated with carboplatin displayed higher numbers of ICAM1+ cells in hotspot regions compared to vehicle (Figures 1E, F).
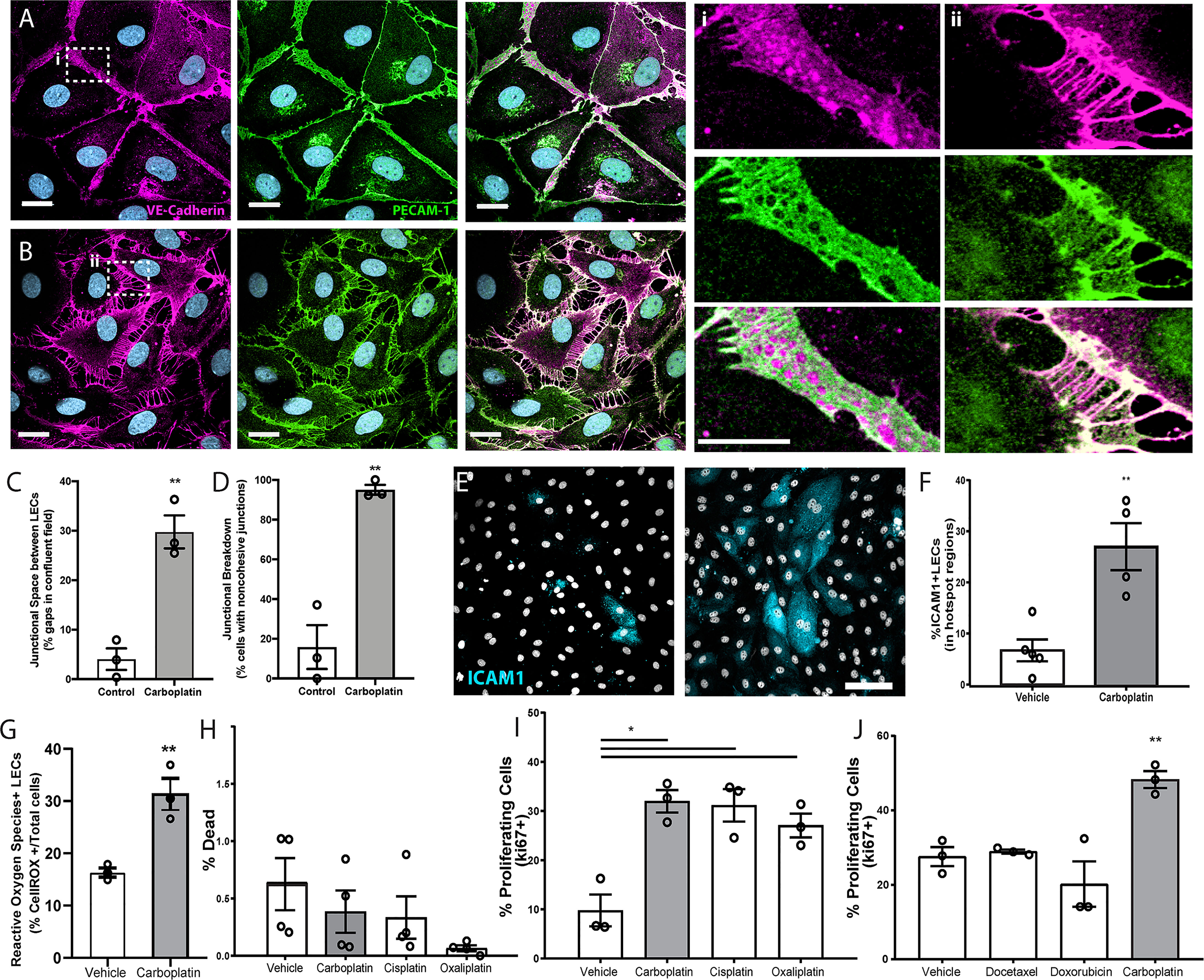
Figure 1 Lymphatic endothelial cell (LEC) monolayers are activated by carboplatin. (A) Vehicle-treated human LECs in monolayer culture; VE-cadherin (magenta) and PECAM-1 (green) with DAPI (gray-blue) for nuclei (scale bar=25µm) with (i) high magnification image cell-cell junction. (B) Human LEC monolayer treated with 1µM carboplatin with (ii) high magnification image of cell-cell junction. (C) Size of gaps between cells within a single field of view; listed as percentage of the field that is comprised of intracellular gap space (n=3/group). (D) Percentage of cells within fields of view that display non-cohesive junctions (n=3/group). (E) Representative images of LECs nuclei (DAPI, gray) and ICAM1 (cyan). Scale bar=250µm. (F) Percent ICAM1+ LECs in hotspot regions (n=5/group). (G) Percent ROS+ LECs per field (n=3/group). (H) Percent dead cells per field after 48h of platinum agent (1µM) assessed by amine-based fluorescent reactive dye (n=4/group). (I) Percent proliferating cells per field by KI67 positivity after 6h in culture with platinum agents (1 µM) (n=3/group). (J) Percent proliferating cells per field by Ki67 positivity after 6h in culture with chemotherapeutic agents (1 µM) (n=3/group). Each data point/n represents one independent experiment (i.e. biological replicate). *p < 0.05, **p < 0.01.
Platinum chemotherapy induces cellular stress and can lead to production of reactive oxygen species (ROS). Further, ROS has been shown to disrupt tight junctions in endothelial cells and activate ICAM-1 and other cell adhesion molecules (24, 25). Therefore, we examined ROS production in LECs treated with or without platinum. Carboplatin led to a significant 2-fold increase in the number of ROS+ LECs compared to control (Figure 1G). ROS can have a dualistic role in cell signaling. In some contexts, ROS can trigger insurmountable oxidative stress that leads to cell death. Conversely, in other contexts, ROS can promote cell survival and even proliferation. To understand the potential implications of heightened ROS in LECs after chemotherapy, we chose to examine both cell death and proliferation in LECs treated with carboplatin. Interestingly, platinum treatment (24h) did not diminish viability (Figure 1H); in fact, it induced a 3-fold increase in LEC proliferation, a necessary precursor for in vivo lymphangiogenesis (Figures 1I, S1E–G). Platinums were the only class of chemotherapy tested that induced significant proliferation in LECs (Figure 1I). Together, these data suggest that platinum chemotherapy acts directly on LECs to induce phenotypes indicative of elements of lymphangiogenesis.
Gene set enrichment analysis of microarray data of LECs treated with the same doses of carboplatin reinforced these phenotypic changes, showing upregulation of pathways associated with proliferation, survival, and neovascularization (Table S1). Carboplatin treatment showed enrichment of the pro-survival and proliferation pathways MAPK, JAK-STAT, PI3K/AKT, RAS, and HIF1-α signaling in LECs and increased expression of genes such as MTOR, INOS, ANGPT1, CMYC, PI3K, and others. Reverse phase protein array (RPPA) and pathway analysis indicated similar pathway upregulation, pointing to signaling via VEGFR, FGFR, and EGFR families (Table S2). Other enriched pathways included those governing cellular adhesion molecules, GAP junctions, and chemokine signaling, all important in LEC activation. Pathway enrichment analysis of the top upregulated microRNAs (9 in total, >2-fold increase in expression) showed similar pathway activation (Table S3).
Carboplatin Induces Dose-Dependent and Sustained Lymphangiogenesis in Healthy Murine, Rat, and Human Tissues
Building on these in vitro data, we tested lymphangiogenesis in physiologically relevant models. First, we used ex vivo rat mesentery tissue (26) to analyze impact of treatment on lymphatics within intact vascular networks (Figure 2A). Carboplatin significantly increased sprouting of lymphatic vessels by 3.5-fold (Figures 2B–D) but had no detectable effect on blood vessels (Figures S2A–C).
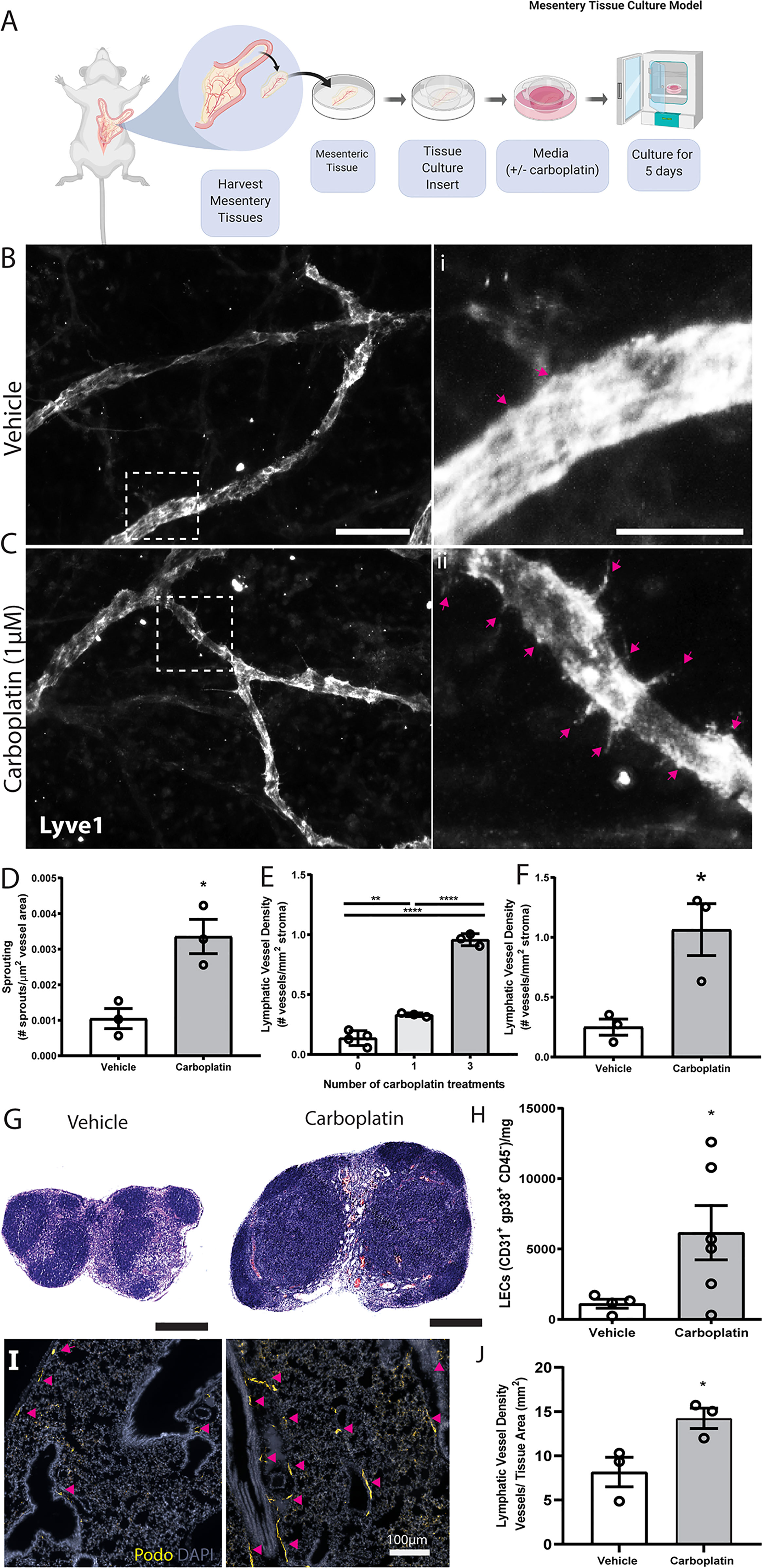
Figure 2 Carboplatin induces lymphangiogenesis in healthy tissues (A) Schematic of rat mesentery culture model. (B) Vehicle-treated lymphatic vessels from mesentery cultures stained with LYVE-1 (grey). (i) High magnification image of boxed area in (B). (C) Carboplatin-treated lymphatic vessels from mesentery cultures stained with LYVE-1 (grey). (ii) High magnification image of boxed area in (C) Scale bar=100µm. (D) Number of sprouts per lymphatic vessel area (n=3/group). (E) Lymphatic vessel density (podoplanin+ vessels per mm2 stroma) in whole mammary fat pads of healthy mice treated with systemic carboplatin (8 mg/kg/dose) or vehicle by IV(n=3-4/group). (F) Lymphatic vessel density measured in mammary fat pads of healthy mice 2 months after treatment with 3 doses of carboplatin or vehicle, (n=3/group). (G) Lymph nodes from healthy, tumor-naïve mice treated with vehicle and stained H&E. (H) LEC number in vehicle-treated and carboplatin-treated lymph nodes in vivo (n=6/group). (I) Representative images of lungs from mice treated with 3 doses of vehicle (left) and carboplatin (right). Podoplanin+ lymphatic vessels noted by arrowheads. (J) Lymphatic vessel density in stromal tissue of lungs of mice pre-treated with carboplatin (n=3/group). *p < 0.05, **p < 0.01, ****p < 0.001. Each data point represents one mouse.
The importance of lymphatics in mammary carcinoma progression is well-established. We treated healthy, non-tumor-bearing female mice with 0-3 doses of carboplatin (8 mg/kg/dose) to examine lymphangiogenesis in naïve mammary fat pads (MFP) (Figure 2E), histologically quantifying lymphatic vessel density (LVD), area, and perimeter (Figures S3A, B). Treatment doses were selected to balance a dose-dense treatment strategy with potential toxicities in mice and represent a mid-range overall treatment dose compared to other preclinical studies. Carboplatin treatment resulted in significant dose-dependent increases in LVD in the MFP stroma of both Balb/c and SCID mice (Figure 2E and Figures S3C–G), but no significant increase in vessel area or perimeter (Figures S3H, I). LVD remained elevated 8 weeks after final treatment with carboplatin comparable to that at day 3 (Figure 2F and Figures S4A, B).
The observed lymphangiogenic effect of carboplatin on healthy mesentery suggests its lymphangiogenic effect is not restricted to mammary tissue. As lymph nodes are a frequent site of cancer spread away from the primary tumor, we also examined lymph node lymphangiogenesis in healthy mice treated with carboplatin chemotherapy. Carboplatin treatment resulted in larger inguinal LNs (Figure 2G) and showed a significant increase in LEC number (Figure 2H and Figures S4C, D).
In addition to lymph nodes, we were also curious to examine the effect of platinum on lungs. Lungs are one of the most common metastatic sites in cancer. Metastatic tumor cells can induce lymphangiogenesis in metastasis-bearing lung tissue, which can then exacerbate the growth of these metastatic lesions and promote further spread to other organs (27). We examined whether a systemically-administered platinum agent could also induce lung lymphangiogenesis, even without metastatic tumor cells present in the lung tissue. Indeed, platinum treatment of healthy mice stimulated lung lymphangiogenesis, leading to a nearly 2-fold increase in lung LVD after carboplatin (Figures 2I, J).
Chemotherapy Regimens That Include Platinum Agents Are Associated With Higher LVD in Patients
We next sought to replicate this phenomenon in platinum-treated normal human tissues. However, acquisition of normal (non-cancerous) tissues that underwent chemotherapy is limited. Ultimately, we chose to quantify lymphatics in omental tissues for the following reasons: (1) platinum chemotherapy is standard of care in high-grade serous ovarian cancer (HGSOC), along with cytoreductive surgery; (2) because omentum is a frequent site of HGSOC metastasis, it is routinely removed during surgery and therefore could be acquired for research purposes; and (3) lymphatics can be readily quantified in these tissues. We analyzed lymphatics in histologically normal omentum from patients treated with neoadjuvant carboplatin and docetaxel chemotherapy prior to surgery (Table S4). All omentum samples were pathologist-identified as uninvolved and ostensibly healthy. Histologically normal omentum treated with carboplatin had 12-fold higher LVD compared to that of untreated patients (Figures 3A, B). Though we could not feasibly procure patient tissues treated with only platinum due to carbotaxol standard of care, our previous findings in animal models have demonstrated that taxanes require tumor to promote lymphangiogenesis (17). Thus, the higher LVD observed here are likely attributable to carboplatin, concordant with our results in vitro and in rodents.
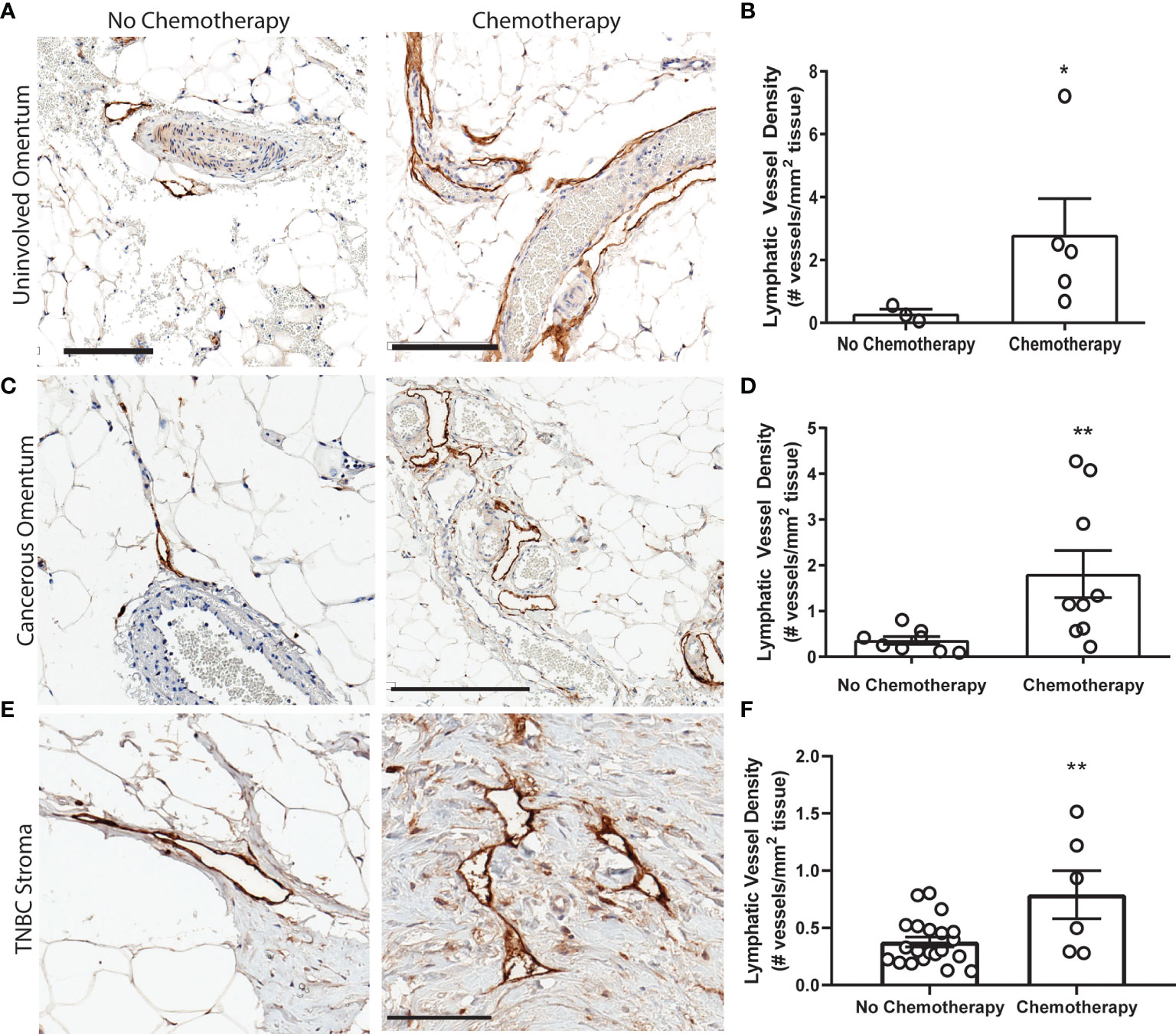
Figure 3 Platinum chemotherapy is associated with higher LVD in human cancer patients (A) Representative images of lymphatic vessels in histologically benign omentum from patients treated with no chemotherapy (left) or neoadjuvant carboplatin combination chemotherapy (right) (see Table S4) with podoplanin (brown) and hematoxylin (blue). (B) Quantified lymphatic vessel density in patient samples. Scale bar = 200 µms. N=8 patients. (C) Representative images of tissues from ovarian cancer patients treated with no chemotherapy (left) or neoadjuvant carboplatin and paclitaxel (right) in cancerous omentum with podoplanin(brown) and hematoxylin(blue). (D) Quantified lymphatic vessel density (N= 17). (E) Representative images of tissues from triple negative breast cancer patients treated with no chemotherapy (left) or neoadjuvant platinum and taxane (right) with podoplanin(brown) and hematoxylin(blue). (F) Quantified lymphatic vessel density (N=27) *p < 0.05, **p < 0.01, scale bar = 200µm. Each data point represents one patient.
In addition to our analysis of pathologically normal tissues, we quantified LVD in cancerous omental tissue from 17 ovarian cancer patients with or without carbotaxol chemotherapy prior to surgery (Figure 3C and Table S5). We again detected a significantly higher LVD in patients that received neoadjuvant platinums (Figure 3D). In addition to gynecologic malignancies, platinums are routinely used in the clinical management of triple negative breast cancer (TNBC) often as a neoadjuvant treatment prior to surgery. Similarly, in primary tumor stromal tissue from 27 TNBC patients (Figure 3E and Table S6), there was a significantly higher LVD in patients treated with platinums prior to surgical resection (Figure 3F).
Platinum Chemotherapy Induces Lymphangiogenesis in Breast Tumor Stroma
To experimentally investigate causal effects of platinum on tumor lymphangiogenesis, we employed a series of preclinical breast cancer models: orthotopic 4T1 syngeneic tumors (immune-competent); orthotopic MDAMB231 xenograft (immune-compromised); or the inducible autochthonous mammary tumor model, L-Stop-L-K-KRasG12Dp53flx/flx-L-Stop-L-Myristoylated p110α-GFP (immune-competent), which more closely mimics the malignant transformation and progression in humans (28). We chose breast cancer as a model for these studies because breast cancer preferentially metastasizes via lymphatics (9), unlike ovarian cancers that can spread through multiple mechanisms. Mice received carboplatin when tumors were palpable and were treated in the same dosing and schedule as previously discussed. There were no differences in tumor size observed (Figure S5A). Histological analysis (Figures 4A–D and Figures S5B, C) showed significantly increased LVD after three treatments of carboplatin (Figure 4E). Unlike in naïve MFP, lymphatic vessel enlargement was present, with lymphatic vessel area and perimeter significantly increased in immunocompetent mice (Figures 4F and S5D).
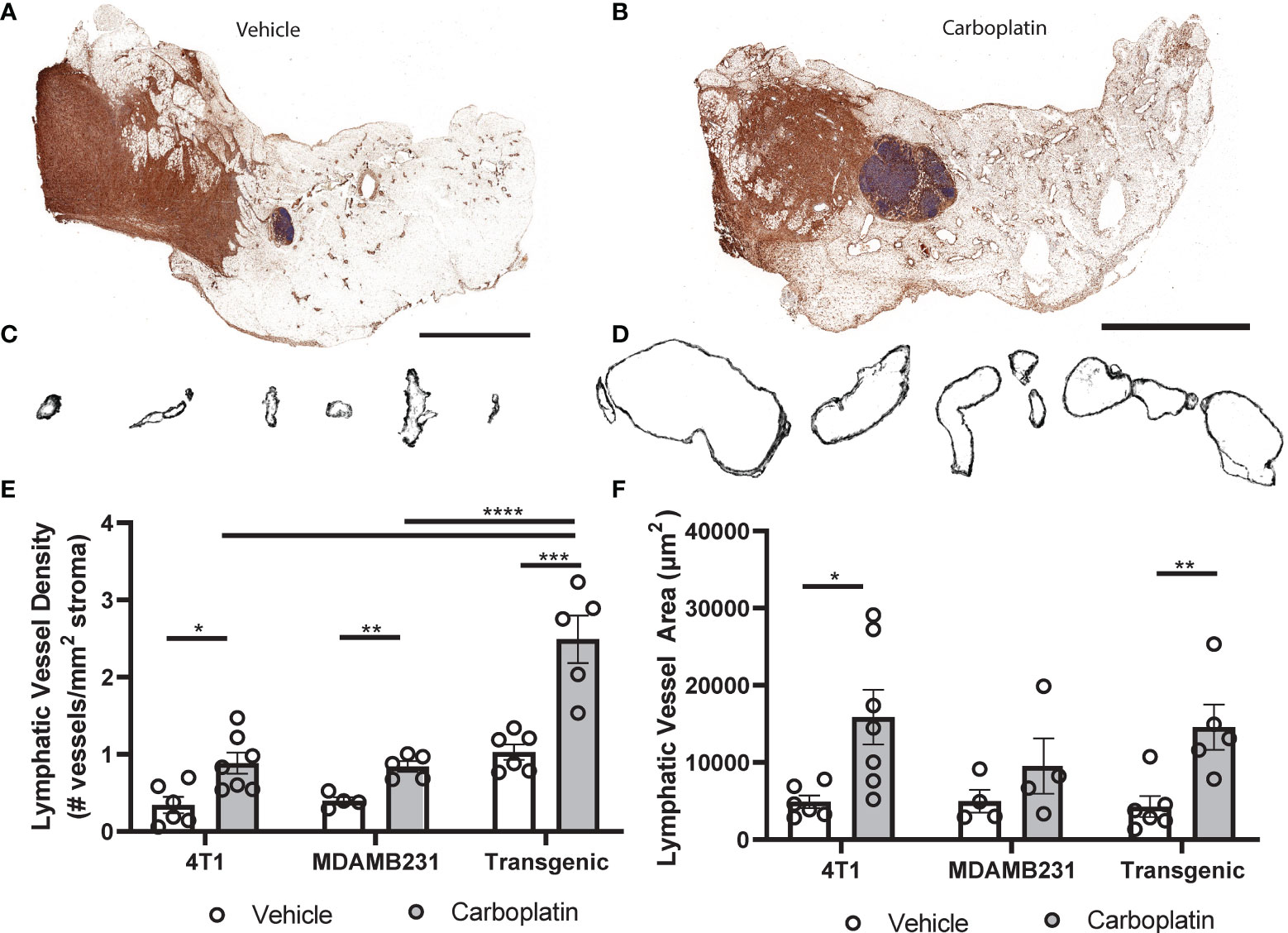
Figure 4 Platinum agents induce lymphangiogenesis in the tumor stroma (A) Representative tumor-bearing mammary fat pad with podoplanin (brown) and hematoxylin (blue) from vehicle-treated transgenic KrasG120Dp53fl/flp110amyr mice. (B) Representative whole tumor-bearing mammary fat pad from carboplatin-treated transgenic mice. (C) Representative individual lymphatic vessel cross-sections from (A). (D) Isolated lymphatic vessels from (B). (E) Lymphatic vessel density (lymphatic vessels per stromal area) in tumor-bearing mammary fat pads in 3 orthotopic mouse models of breast cancer (syngeneic 4T1 N = 12, transgenic KrasG120Dp53fl/flp110amyr N = 11, and xenografted MDA-MB-231 N = 9). (F) Average area of individual lymphatic vessels (µm2) in mouse models of breast cancer as described previously. *p < 0.05, **p < 0.01, ***p < 0.001, ****p < 0.0001, each data point represents one mouse.
Systemic Carboplatin Pre-Treatment Leads to Increased Lymph Node and Lung Metastasis in Murine Breast Cancer
Enlargement and remodeling of lymphatic vessels is known to increase tumor cell dissemination (11, 13, 29), with LVD and tumor spread correlating in murine and human cancers (30–32). Using a tissue engineered model of the breast cancer microenvironment, we also saw that addition of carboplatin in the presence of lymphatic endothelial cells would increase tumor cell invasion towards lymphatics (Figures S6A, B). We hypothesized that priming of healthy tissues with carboplatin creates a hospitable niche that will later promote tumor cell invasion and metastasis once the cancer disease process has started.
We treated healthy, tumor-naïve mice with systemic carboplatin to induce lymphangiogenesis (Figure 5A), followed by orthotopic implantation of 4T1 tumor cells 1 week later. Since carboplatin was allowed to clear completely prior to cancer initiation, tumors were never exposed to the drug and thus there was no significant difference in tumor size at endpoints, as expected (Figure S6C). As we previously observed that platinum increases LEC numbers in the lymph node and lung, we assessed metastasis to those organs, which are common sites of cancer spread. Tumor cell metastasis to the tumor-draining inguinal lymph node (TDLN), considered a first step in tumor cell dissemination (9), significantly increased in platinum pre-treated TDLNs compared to control (Figures 5B–D). These data suggest that the lymphatics activated and remodeled by platinums are functionally capable of promoting metastasis. We then evaluated whether platinum-priming could contribute to distant metastases, e.g. to lung. 21 days after implantation, 100% of carboplatin pre-treated mice showed gross lung metastases, compared to 50% of the vehicle. Microscopic examination of lungs showed significantly increased numbers of foci (Figures 5E, F) that were significantly larger with pretreatment (Figure 5G). Therefore, we find that systemic pre-treatment with carboplatin increases LN and lung metastasis.
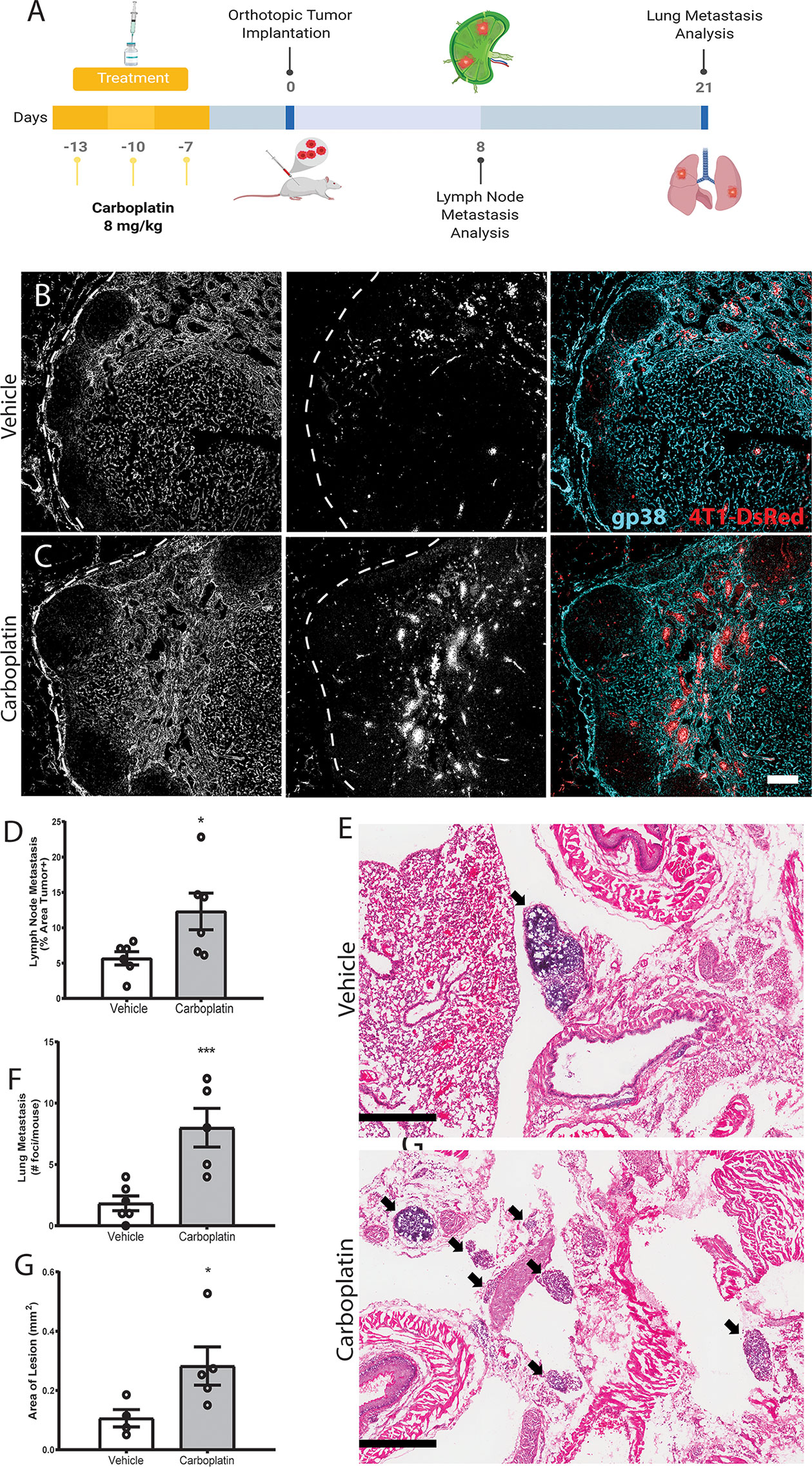
Figure 5 Systemic pre-treatment with carboplatin increases metastatic spread of 4T1 breast tumors Systemic pre-treatment with carboplatin increases metastatic spread of 4T1 breast tumors (A) Experimental timeline for carboplatin pretreatment (8 mg/kg x 3 or vehicle) followed by 4T1 tumor implant and subsequent tissue harvest. n=6/group (B) Representative images of 4T1 cells (red) in vehicle pretreated tumor-draining inguinal lymph nodes (TDLN) counterstained with podoplanin (cyan). (C) Representative images from carboplatin pretreated TDLNs. Scale bar=100µm. (D) Percentage of TDLN area covered by tumor cells as assessed by image thresholding in ImageJ. (E) Representative images of lung metastases at endpoint by H&E in vehicle (top) and carboplatin (bottom) -primed mice, denoted by arrowheads. Scale bar = 500 µm. (F) Number of metastatic foci in lung per mouse. (G) Area of macroscopic metastatic lesions in lung. *p < 0.05, ***p < 0.005 as analyzed by individual t test. Each data point represents one mouse.
VEGFR3 Blockade Mitigates Carboplatin-Induced Lymphangiogenesis and Metastasis
VEGFR3 blockade reduces metastatic spread and lymphangiogenesis in a number of tumor models, as the VEGFC : VEGFR3 signaling pathway is one of the quintessential drivers of LEC proliferation and lymphatic expansion (11). Based on the literature implicating VEGFR3 in metastatic spread in murine breast cancer models, its specificity for lymphatics, as well as the potential connection to VEGFR3 as seen in our gene set enrichment analysis (Figure 1J), we hypothesized that blockade of VEGFR3 would abrogate the effects of carboplatin on lymphatics. Thus, we examined outcomes in the presence of anti-VEGFR3 inhibition.
We used MAZ51 (3-(4-Dimethylamino-naphthalen-1-ylmethylene)-1,3-dyhydro-indol-2-one), a specific small molecule inhibitor of VEGFR3, on monolayers of LECs in vitro. MAZ51 had little effect on LEC proliferation alone, but significantly suppressed carboplatin-induced LEC proliferation (Figure 6A) and junctional disruption (Figure S7A) to resemble untreated LECs (Figure 1). Combination treatment with carboplatin and MAZ51 only modestly increased LEC death from less than 1% to ~3% (Figures S7B, C).
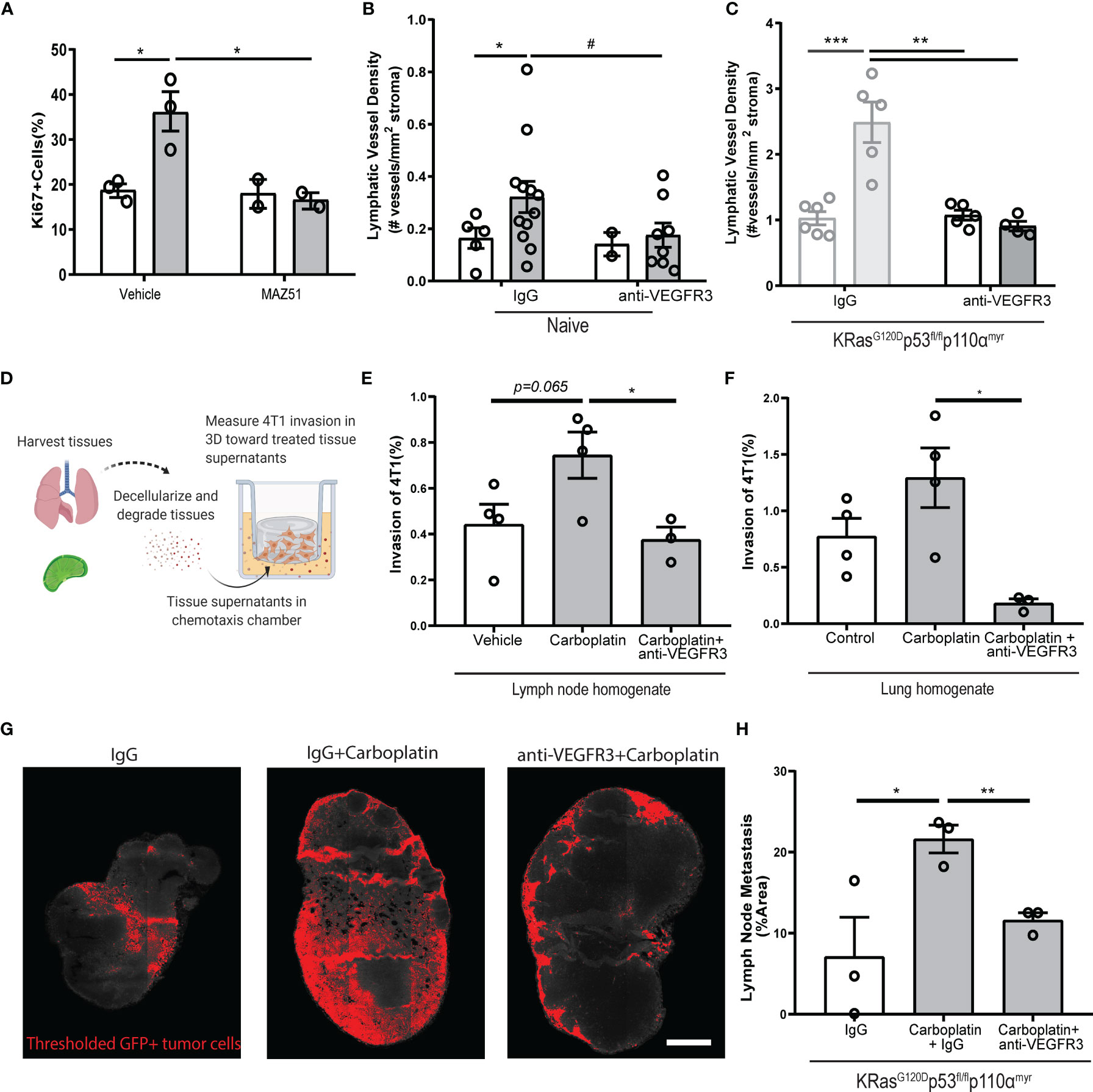
Figure 6 Blockade of VEGFR3 inhibits carboplatin-induced lymphangiogenesis and metastasis (A) Percent proliferating cells per field by Ki67+ staining of human LEC monolayers treated in vitro with carboplatin (1 µM), VEGFR3 inhibitor MAZ51 (1 µM), or both for 6h (n=2-3/group; data points represent independent experiments). (B) Lymphatic vessel density in naïve, tumor-free fat pads from mice treated with vehicle/carboplatin and anti-VEGFR3/IgG (N=27; one data point per mouse). (C) Lymphatic vessel density (lymphatic vessels per stromal area) in tumor-bearing fat pad of KRasG120Dp53fl/flp110αmyr mice treated vehicle/carboplatin and anti-VEGFR3/IgG. Grayed bars represent data presented in previous figure but included here as point of reference. (N=21; one data point per mouse). (D) Experimental schematic of chemotaxis assay using digested and decellularized in vivo-treated tissues. Healthy, tumor-free mice were treated in vivo with carboplatin (8 mg/kg x 3 or vehicle) and/or anti-VEGFR3 antibody (or control IgG antibody). Lymph nodes and lungs were harvested 3 days following final treatment, digested and decellularized, and used in a 3D in vitro chemotaxis assay for 4T1 cells. (E, F) Invasion of 4T1 tumor cells towards digested and decellularized lymph node (E) and lung (F) from treated mice (n=3-4/group; each data point represents extract from one mouse, as average of 3 independent in vitro experiments). (G) Representative thresholded images and (H) quantification GFP+ tumor cells in whole inguinal lymph nodes from KRasG120Dp53fl/flp110αmyr mice treated with vehicle/carboplatin and anti-VEGFR3/IgG (n=3/group; one data point per mouse). #p < 0.1, *p < 0.05, **p < 0.01, ***p < 0.001.
Inhibition of VEGFR3 fully attenuated LEC proliferation caused by platinums. We speculated that platinum-induced lymphangiogenesis in tumor-free tissues was also dependent on VEGFR3 signaling. Indeed, we observed the same trend in the mammary fat pads of mice treated with a clinically relevant VEGFR3 blocking antibody, where VEGFR3 inhibition reduced LVD to baseline levels (Figure 6B). In tumor-bearing tissue from our breast cancer animal models, we could reverse carboplatin-mediated increases in LVD in both 4T1 (Figure S8A) and KRasG120Dp53fl/flp110αmyr (Figure 6C) tumor-bearing fat pads. Similarly, blockade significantly reduced tumor-associated vessel area (Figures S8B, D) and perimeter (Figures S8C, E). Importantly, anti-VEGFR3 co-treatment fully reversed the platinum-induced lymphangiogenesis in murine lungs of healthy mice treated with platinums in vivo (Figure S8F). These data suggest the changes to lymphatics by platinum chemotherapy is mediated by VEGFR3 and its inhibition can reverse these phenomena.
With platinums, we observed increased activation (Figure 1) and proliferation (Figures 1, 2) of lymphatics in otherwise healthy tissues throughout the body. The lymphatic endothelium secretes a number of chemotactic factors that can promote pre-metastatic niche formation and subsequent tumor cell invasion. To understand whether factors secreted by lymphatics in the tissue milieu could enhance tumor cell chemotaxis, we treated healthy, tumor-free mice with carboplatin and anti-VEGFR3 antibody in vivo and harvested their lymph nodes and lungs three days after the final treatment (Figures 6D–F). These tissues were digested and decellularized (Figure 6D); their supernatants were then used in a chemotaxis chamber to measure invasion of murine breast cancer cells through a 3D matrix. Tumor cells invaded more to carboplatin-treated lymph node (Figure 6E) and lung (Figure 6F) digested and decellularized tissues. Further, tissue supernatants from mice that were also treated with anti-VEGFR3 antibody in vivo showed significantly attenuated platinum-induced invasion of 4T1 cells toward both tissue homogenates. These data indicate that the activating effects of platinum on lymphatics that promote tumor cell invasion are mediated through VEGFR3.
Ultimately, metastasis reduction in vivo is desired. While carboplatin treatment increased lymph node metastasis by nearly 3-fold (Figures 6G, H), alternating carboplatin with anti-VEGFR3 therapy (Figures S8G, H) resulted in reversion of metastatic spread induced by carboplatin in KRasG120Dp53fl/flp110αmyr mice (Figures 6G, H). Taken together, these data indicate that carboplatin-associated lymphangiogenesis occurs via a VEGFR3-dependent mechanism. VEGFR3 inhibition could directly revert the phenotypic effects of carboplatin seen in vitro, ex vivo, and in vivo, thereby explaining its therapeutic success.
Discussion
Here we see that platinum agents, and most specifically carboplatin, increase lymphatic expansion and proliferation across a number of models. The phenotypic changes we observed with platinums in vitro were indicative of changes in vivo. Though the pathway we ultimately targeted to reduce the phenotype, VEGFR3, is non-novel for lymphangiogenesis, the connection to carboplatin (and to a lesser degree, platinum agents in general), is. Accordingly, we observed increases in LEC numbers and LVD in vitro, ex vivo, in vivo, and in patient tissues with platinum agents. It is counterintuitive that chemotherapies would induce expansion of a cellular population and promote tumor progression; however, in fibroblasts, induction of stress responses by DNA-damaging chemotherapies can result in similar activation and compensatory proliferation (33, 34). Platinums have been shown to increase ROS (35), and ROS can lead to enhanced proliferation, survival, and inflammatory pathways (36), all of which we observed in our LECs after treatment. Interestingly, ROS, of which we see an increase, can induce oxidation of growth factor receptors and downstream phosphatases to enhance growth factor receptor signaling (37). Therefore, though we know that VEGFR3 blockade can reduce the pro-lymphangiogenic effects that we see, the full mechanism requires further exploration to determine the link between platinum agents, ROS, and lymphangiogenesis across physiological contexts.
In addition to observing platinum-induced lymphangiogenesis within mammary tissues, driving lymphatic metastasis from the primary tumor site, we also observed VEGFR3-dependent increases in LVD in the lymph nodes and lungs. Increased LVD at metastatic sites, such as the lung [in breast cancer (27)] and the liver [in colorectal cancer (31)], is also correlated with poorer prognosis. Previous studies have shown that lymphatic remodeling and lymphangiogenesis at these distant sites can exacerbate growth of metastatic colonies within the lung and promote further spread to other organs. To date, lymphangiogenesis in distant organs has been attributed to either (1) the presence of metastatic tumor cells already seeded within that organ (27), or (2) tumor-derived pro-lymphangiogenic factors secreted by the primary tumor that act on organ-residing LECs (12). Interestingly, after organ-residing LECs are activated through this mechanism, these distant LECs have been shown to secrete pro-chemotactic molecules that can recruit breast cancer cells into lymphatics, as well as VEGF that can promote lymph node angiogenesis and vascular permeability in the lungs to encourage metastatic extravasation and colonization (12). Here, we observed a previously undescribed phenomenon through which the lymphangiogenic effects of platinum occur in the lymph nodes and lungs in the absence of lung metastases (or any cancer, for that matter). Yet, regardless of the stimulus for distant lymphangiogenesis, it is conceivable that the biological implications of increased and activated LECs at these sites would be similar to what has been previously reported.
Platinum-induced lymphangiogenesis occurred in multiple tissues, such as mammary tissues, lymph nodes, lungs, and connective tissues, and was sustained long-term. Permanent increases and remodeling to lymphatics not only at the primary tumor site but also in distant tissues may prime these tissues by encouraging formation of the pre-metastatic niche, creating more potential escape routes for disseminated tumor cells. In fact, this is the case in cancers such as colorectal and breast cancer, where increased LVD in the liver and lungs, respectively, has been correlated to increased instance of metastasis in those tissues (38); indeed, we observed increased LVD in the lungs of platinum-treated mice that was VEGFR3-dependent. This is in direct contrast to lymphatic effects seen with other chemotherapeutic agents like taxanes that require the presence of a tumor to enact these changes and occur only in the local tumor microenvironment (16, 17). Differences in these mechanisms may arise from the inherent differences in drug mechanism of action between taxanes, which are not DNA damaging, and platinums, which act directly on DNA. As patients are exposed to chemotherapies on a systemic level, platinum-induced lymphangiogenesis could conceivably occur in distant tissues posing an increased risk for lymphatic metastasis to these other sites.
All therapies, anti-cancer or otherwise, eventually drain via our lymphatic system, but the majority of research has focused on the impacts of drugs on the blood vasculature, often ignoring the impact on this endothelium (39). To our knowledge, this is the first study that characterizes the effects of chemotherapy on lymphatics in healthy, cancer-free in vitro, ex vivo, and in vivo models. Indeed, more studies are emerging that suggest chemotherapy may paradoxically counteract its own efficacy through action on either the tumor cells or its associated stroma. In this study, we examined the physiological implications of platinum-induced lymphangiogenesis in the context of metastasis, illustrating potential deleterious off-target effects of a commonly used therapy. However, some off-target effects of chemotherapy can also be desirable. We did not investigate the impact of platinum-induced lymphangiogenesis on tumor immunity, which may offer positive benefits by increased immune cell migration to the tumor and metastatic sites. Platinums and other chemotherapies can generate neoantigens and activate T cells for enhanced immunological response (40, 41). Activated lymphatics can assist in immune cell trafficking to the tumor site. Through this lens, it is possible that the presence of increased tolerogenic LECs and normalized vasculature after VEGFR3 therapy could interfere with those benefits (42). Further investigation is needed into the physiologic implications of these lymphovascular changes in patients. Certainly, platinum chemotherapy has demonstrated potent cytoreductive efficacy over decades of clinical use. In TNBC, platinum agents are successful in aiding in disease remission, which has been shown across multiple clinical studies (19, 43). In metastatic disease, preliminary analyses indicate that there may be a moderate survival benefit (44). However, there are still subsets of patients who are refractory to platinum agents, especially in ovarian cancer, and thus, may be at risk for secondary effects of the therapy (45). Thus, clinical decision-making cannot be truly informed until more studies examine how these agents affect normal tissues in patients receiving platinums.
Our studies describe a previously unknown pro-lymphangiogenic action of platinum chemotherapeutics. In vivo, we selected a dose of carboplatin that would not affect tumor growth and thus reduce confounding effects of tumor size and toxicity with lymphangiogenesis, as tumor size is correlated with changes to tumor stroma including lymphatics (46, 47). In our pre-treatment animal models, we found that this led to enhanced metastasis. Use of higher doses of platinum agents, or other chemotherapies, in mouse models have shown mixed effects on metastatic spread and tumor growth (48–50). In 4T1, others have seen that with a single high dose (100 mg/kg) delivered during tumor growth, there was decreased metastasis (51). In the context of this study, we do not know if use of higher tumor-growth reducing doses would lead to similar alterations in lymphangiogenesis. However, the distribution of drugs to and within tumors is highly heterogeneous and as such, there are likely lymphatics that receive a range of therapies over different amounts of time, which may result in differential pro- or anti-lymphangiogenic effects. Other therapeutics, such as doxorubicin, delivered to lymphatics result in reduced lymphatic flows through reduced function of smooth muscle cells (52). We did not specifically examine the function of the platinum-treated lymphatics here, though this would be an interesting future study to determine effects on not only metastatic spread, but also lymphedema, which is a common clinical sequelae of treatment and can be enhanced with lymphangiogenesis (53–55). Ultimately, we see across multiple dosing schemes in multiple contexts (in vitro, ex vivo, in vivo, and clinic) indications of lymphangiogenesis in healthy and diseased tissues with platinum agents.
Platinum-induced lymphatic proliferation and expansion were dependent on VEGFR3 and supplementing chemotherapeutic regimens with anti-VEGFR3 was sufficient to prevent them. VEGFR3 inhibition has been suggested as a potential anti-metastatic treatment option in human cancers since the discovery of this pathway as a leading driver of lymphangiogenesis. It has shown successful anti-metastatic benefits in multiple murine models of cancer (10, 29, 56–59) but had mixed results in early phase clinical trial. Our data begin to suggest it may be most advantageous not as a single agent but instead in combination with a platinum chemotherapy as a preventative measure against countertherapeutic VEGFR3-dependent lymphangiogenesis in early-stage cancers that have yet to infiltrate into the lymphatics, though more in-depth pre-clinical testing of this pairing is warranted to fully understand its clinical potential.
We believe that these findings highlight our incomplete understanding of chemotherapeutic action in the tissue stroma. Our data here suggest that informed pairing of chemotherapy with targeted therapies to the tumor microenvironment may improve overall efficacy of these valuable treatments. Specifically, we believe our findings may renew interest in the clinical potential of anti-VEGFR3 therapy, such as IMC-3C5 (60), which is well-tolerated, yet stalled in clinical trials, and may show promising results when used in combination with specific chemotherapy within the proper disease context.
Materials and Methods
Cell Culture
Human lymphatic endothelial cells (HMVEC-dLy, Lonza) were cultured in Endothelial Cell Growth Medium (EBM-2 basal media, Lonza) supplemented with recommended growth supplement kit (EGM-2MV BulletKit, Lonza). Mouse mammary carcinoma cell line 4T1-luc-red (generously given by the Cross laboratory at University of Virginia) originated from ATCC and were acquired from Perkin-Elmer (BW124087V) after lentiviral transduction of Red-FLuc luciferase gene. 4T1 cells were cultured in RPMI medium supplemented with 10% FBS. MDA-MB-231 were acquired from the ATCC and cultured in Dulbecco’s modified Eagle’s medium (DMEM, Gibco) and supplemented with 10% fetal bovine serum (FBS). All cell lines were grown sterilely in humidified atmosphere of 5% CO2 and 95% oxygen at 37°C. All cell lines were confirmed to be mycoplasma free by PCR testing conducted in the Cell Culture Core Facility at the University of Virginia. All experiments were completed afterwards.
In Vitro Drug Treatment, Immunocytochemistry, and Live/Dead Assays
LECs were cultured on glass coverslips in complete media as described above; the LEC monolayer was treated with 1 μM carboplatin, cisplatin, oxaliplatin, docetaxel, doxorubicin, MAZ51 (61), or appropriate solvent control (referred to as ‘vehicle’) for 6 hours (phenotypic studies) or 48 hours (live/dead analysis). After treatment, coverslips were fixed with 4% paraformaldehyde (PFA) for 30 minutes at room temperature and underwent immunofluorescent staining with Ki67 to assess proliferation (Millipore, Cat. #AB9260); ICAM1 to assess cellular adhesion molecule expression (Abcam, Cat. #AB2213); VE-Cadherin (Abcam, Cat. #AB33168) and CD31 (R&D systems, Cat. #AF806) to assess cellular junctions. For live/dead analysis, amine-based fixable live/dead solutions (Life Technologies, Cat. #L23101) were added to cell media of living LECs after 48 hours of drug treatment and five random images were taken of each well; technical replicates were averaged to yield one biological replicate. Each quantification was performed with a minimum of 3 biological replicates.
CellROX Reactive Oxygen Species (ROS) Assay
Relative ROS generation was measured using CellROX Green Reagent (Thermo Fisher, Cat. # C10444). This is a DNA-binding probe that fluoresces bright green upon oxidation by ROS. The assay was conducted as recommended by the supplier. In brief, LECs were cultured in complete media as described above; the LEC monolayer was treated with 1 μM carboplatin, MAZ51 (50), 100 uM Menadione K3 (Sigma, Cat.#47775) as a positive inducer of ROS (62), 1mM N-Acetylcysteine (Sigma, Cat.# A9165) as a negative control per manufacturer’s recommendation, or appropriate solvent control (referred to as ‘vehicle’) for 6 hours. After treatment, cells were washed with PBS and incubated with 2.5 μM CellROX Green Reagent and 1.62 uM Hoescht 33342 in the dark for 30 minutes at 37°C. Afterwards samples were washed once with PBS before measuring intracellular levels of ROS with a Zeiss Axio Observer fluorescent microscope. Five random images were taken of each well using the DAPI and FITC filter. Quantification was done using ImageJ, to count the number of cellROX green positive cells with signal present in both the nucleus and mitochondria being considered. To find CellROX+ percent of cells, number of CellROX+ cells was divided by total nuclei per area. For CellROX ROS analysis, means taken from each treatment triplicate were considered a biological replicate for statistical analysis.
Microarray and Gene Set Enrichment Analysis
Human LECs were treated in vitro with 1 µM carboplatin for 4 hours. Cells were lysed with RLT buffer and RNA was isolated using the QIAGEN RNeasy Kit (Cat #74104). Microarray was performed by the UVA DNA Sciences core using the Human Affymetrix GeneChip Array ([HuGene-2_1-st] Affymetrix Human Gene 2.1 ST Array). Gene lists were generated from microarray data corresponding to genes >1.25 LogFC and <0.75 logFC were used in downstream analyses. Differential targets identified were further subjected to gene set enrichment analysis performed using the ShinyGO enrichment tool using the STRING api. The STRING database (Search Tool for the Retrieval of Interacting Genes/Proteins) is a tool for looking at functional associations between different proteins (63). Protein-protein interactions are mapped across several curated databases and functional association is mapped in interaction networks. Gene interactions are then mapped to the KEGG pathway annotations with the adjusted p values representing pathway enrichment analysis from KEGG Release 86.1. MicroRNAs upregulated by >2 LogFC after treatment were subjected to enrichment analysis by DIANA mirPath v.3 from KEGG pathways significantly enriched with the union of target genes (64).
Reverse-Phase Protein Array and Pathway Analysis
Human LECs were treated in vitro with 1 µM carboplatin for 6 hours. Cells were washed and lysed using RIPA buffer with protease and phosphatase inhibitors. RPPA was performed by the MD Anderson Functional Proteomics Core Facility as previously published (65). To assess which proteins reacted to the treatment, ratios of brightness were calculated, and the distribution was assessed. Outlier proteins, for which the ratio was more than 2 standard deviations from the mean of the ratio distribution, were taken to be most affected by the treatment. Pathway enrichment analysis for these proteins was performed using ConsensusPathDB (66) online tool and pathways enriched with statistical significance of unadjusted p-value of 0.01 were retained for further analysis.
Harvest, Treatment, and Whole Mounting of Ex Vivo Rat Mesentery Tissues
Mesentery tissues were harvested from rats as previously described by Azimi, et al. (26, 67) (Figure 2A). Briefly, mesenteric tissue was harvested from the small intestine of an adult Wistar rat and transferred into a culture dish. Tissues were arranged on permeable membranes of cell crown inserts and cultured in MEM with 20% FBS and 1% penicillin/streptomycin for five days as previously described with 1 μM carboplatin or vehicle control. Tissues were then removed from inserts, grossed, mounted to slides, and fixed with methanol to undergo immunofluorescent staining with PECAM-1/CD-31 (BD Biosciences, Cat. #555026) for blood vessels and LYVE-1 (AngioBio Co., Cat. #11-034) for lymphatic vessels. Images were taken of a minimum of five random areas of vessel remodeling for each well and quantified by counting the number of sprouts normalized to vessel area in each field.
In Vivo Animal Study Design
All methods were performed in accordance with relevant guidelines and regulations and approved by the University of Virginia Institutional Animal Care and Use Committee.
Studies in Healthy, Tumor-Naive Animals
6-week-old female Balb/c mice were treated via tail vein injection of carboplatin unless otherwise noted. Mice underwent three total treatments with 8 mg/kg carboplatin or vehicle control (saline); each treatment was three days apart and mice were euthanized CO2 inhalation three days following the final treatment. Naïve mammary fat pads and axillary lymph nodes were harvested; fat pads were post-fixed in 4% PFA for 24 hours, dehydrated, paraffin-embedded, and sectioned at 7-micron thickness to undergo immunohistrochemical staining (see Immunohistochemistry). Lungs were harvested, post-fixed in 4% PFA followed by 30% sucrose and embedded in OCT and sectioned at 12-micron thickness. For lymph node LEC analysis, carboplatin or vehicle equivalent was injected directly into the inguinal fat pad at a dose of 5 mg/kg three times over one week. Inguinal lymph nodes were harvested three days after final treatment. Lymph nodes were digested [dissociation as previously described (68)], and total LEC counts quantified (see Flow Cytometry).
Studies in 4T1 Breast Cancer Model
4T1 mouse mammary carcinoma cells were cultured as described above. 4T1 cells were suspended in 3.3 mg/ml growth-factor reduced basement membrane extract (Cultrex) in phosphate-buffered saline (PBS) and orthotopically injected in a subareolar fashion into the fourth mammary fat pad of female balb/c mice. For most experiments, 50,000 4T1 cells were injected and mice treated with either 1 or 3 doses of 8 mg/kg carboplatin or vehicle by tail vein injection staggered by one day with anti-VEGFR3 antibody (100 μg per injection x 3 total injections, I.P., eBioscience (now ThermoFisher), Control IgG: rat monoclonal IgG2a kappa Isotype Control, Cat.#16-4321-85; Anti-VEGFR3: rat monoclonal IgG2a kappa to mouse VEGFR3 (AFL4): Cat. #16-5988-85) or IgG control antibody after tumors were just palpable. Mice were euthanized by CO2 inhalation once tumors reached desired endpoints as assessed by caliper measurement. Tumor-bearing and contralateral naïve fat pads containing inguinal lymph nodes were harvested and post-fixed (24 h for naïve tissues, 48 h for tumors) in 4% PFA and processed for histology as described above; tumor-draining axillary lymph nodes were dissociated for flow cytometric analysis for LEC number.
For pre-treatment experiments, naïve mice were treated with three rounds of carboplatin as previously described and drug was allowed one week to clear (69). 10,000 4T1 cells were then injected as described above and allowed to grow until desired size endpoints were reached. Tumor-bearing fat pads were harvested and processed as described above. Lungs were removed, washed in saline, and fixed in 4% PFA for 48 h where they were then embedded in OCT and cryosectioned to undergo histological staining to examine metastasis.
Studies in Transgenic Breast Cancer Model
L-Stop-L-KRasG12Dp53flx/flxL-Stop-L-Myristoylated p110α−GFP+ mice on a C57BL/6 background were generously provided by Melanie Rutkowski, University of Virginia. Mammary tumors were initiated by intraductal injection of adenovirus-Cre in these mice as previously described (28). Tumor growth was tracked via weekly caliper measurements. Once tumor growth became palpable, mice were randomized into groups with normalization of tumor size across groups, followed by the initiation of treatment. Animals were treated with 3 doses of IV carboplatin (8 mg/kg) or vehicle staggered by one day with 3 doses of anti-VEGFR3 antibody (100 μg) or IgG control as described above once tumors were palpable. Mice were euthanized by CO2 inhalation when largest tumors reached 2 cm in any direction; tumor-bearing and contralateral naïve fat pads were collected for histology as described above.
Studies in Human Xenograft Breast Cancer Model
Human MDA-MB-231 breast cancer cells were cultured as described above. 1x106 cells were suspended in 3.3 mg/ml growth-factor reduced matrigel in phosphate-buffered saline (PBS) and orthotopically injected in a subareolar fashion into the fourth mammary fat pad of immunocompromised female mice (NOD.CB17-Prkdcscid/J). Once tumors were palpable, carboplatin was administered in three injections as described above. Mice were euthanized by CO2 inhalation one week following final treatment; tumor-bearing and contralateral naïve fat pads were processed for histology as described above.
Human Tissue Sample Acquisition
Remnant, to be discarded, surgical resections (not needed for diagnostic purposes) of omental metastatic ovarian cancer and patient-matched normal omentum and benign pelvic mass omentum for immunohistochemical staining with podoplanin were collected into a tissue and data bank by waiver of consent and approved by the University of Virginia Institutional Review Board for Health Sciences Research. The UVA Biorepository and Tissue Research Facility procured remnant samples, including all breast cancer specimens and the majority of omental specimens, under this protocol from UVA Pathology for fixed and embedded specimens in paraffin. De-identified tissues and associated clinical data were pulled from this tissue bank and used in experiments approved by UVa IRB-HSR.
Immunohistochemistry
Tumor-bearing mammary fat pads and lung tissues were dissected from mice and post-fixed in 4% PFA for 48 hours at 4°C; naïve fat pads underwent 24 hours of fixation. Fat pads were transferred to 70% ethanol for 24 hours, dehydrated, and paraffin-embedded. Tissues were sectioned at 7 μm thickness. Sections were stained with anti-podoplanin antibody (1 μg/ml, R&D Systems, Cat. # AF3244) followed by ImmPRESS HRP anti-goat IgG peroxidase/SG peroxidase detection (Vector Labs, Cat. # PI-9500-1) and nuclear counter-staining with hematoxylin (Vector Labs) was performed. Human samples were processed and stained similarly. Slides were scanned at 20X on an Aperio Scanscope. For quantification of lymphatic vessel size, a custom interactive MATLAB (MathWorks) program utilizing the Image Processing Toolbox (MathWorks) was designed to identify and analyze lymphatic vessels. First, IHC images were binarized using an intensity threshold capable of isolating vessels with high specificity. Next, a flood-fill operation was used to uniformly fill the vessel area. These regions were then extracted for analysis. Vessels not captured or incompletely captured by the automated procedure were identified by user-drawn regions of interest (n=5/cohort, minimum of 20-30 representative vessels/cohort). Centroid coordinates, perimeter, and area was computed for each vessel. For lymphatic vessel density, all lymphatic (podoplanin+) vessels in the mammary fat pad were counted and vessel number was normalized to size of stromal area for each mouse to assess lymphatic vessel density as lymphatic vessel #/mm2 stroma. Intratumoral lymphatic vessels were rare and not included in these analyses in animal models. For lymphatic metastasis, sections were stained with anti-RFP antibody (5 μg/ml, Thermo Fisher, RFP Tag Monoclonal Antibody RF5R, Cat. # MA5-15257) and whole node confocal scans were used to quantify percent metastatic area of total node via image thresholding in ImageJ. Lungs were cryopreserved and embedded in OCT following fixation, cryosectioned, and stained with hematoxylin and eosin (H&E) to detect metastasis. Macroscopic metastatic lesions were counted by eye, whereas whole 20X scans of lung tissue were taken to detect micrometastatic lesions. Area of macroscopic lesions were measured in ImageJ.
3D In Vitro Co-Culture Model
A detailed description and full protocol of this 3D in vitro co-culture model of the human breast tumor microenvironment has been published previously (70). Briefly, 10,000 LECs were allowed to adhere and grow on the underside of 8 μm pore size 96-well tissue culture inserts (Corning). After 48 h, 50 μl of a Rat Tail Collagen I (Corning)/basement membrane extract (Trevigen) (0.18 mg/ml Collagen, 0.5 mg/ml BME) containing Cell Tracker dye (ThermoFisher). Labeled human mammary fibroblasts (100,000 HMF/ml) and human breast cancer cells (660,000 TNBC cells/ml) was placed atop the inserts. After gelation, media was added to the bottom compartment and flow was applied through the top compartment overnight (~ 1 μm/s; 16-18 h), after which point carboplatin was applied via flow to the top compartment for 24 h and then flushed from the system with basal media. 48 h after drug application, gels were removed, dissociated using Liberase TM (Roche), and processed for flow cytometry. Inserts were processed for invasion analysis as described (17, 70). All experimental conditions were run as triplicate samples in individual inserts.
Invasion Assays
Tissue Engineered 3D Model of Human Breast Tumor Microenvironment
After gel was removed, tissue culture inserts were washed briefly in phosphate-buffered saline (PBS) and fixed with 4% paraformaldehyde. Inserts were stained with DAPI and visualized by fluorescence microscopy. Cancer cells (DAPI + Cell Tracker Deep Red+) were counted in five individual fields per well. Percent cancer cell invasion was calculated as previously described. Three technical replicates were averaged for each experimental run to give a single biological replicate value for statistical analysis.
Tissue Homogenates From Treated Mice
Tissues from mice treated with 3 doses of carboplatin (as described above) were perfused with saline and dissected out. These tissues were degraded using 0.1 mg/ml Liberase TM for 30 minutes at 37C and then centrifuged down. The supernatant was removed and snap frozen until use. These samples were analyzed using BCA assay (Pierce) to determine total protein content. For invasion assays, 100,000 4T1 cells were resuspended in a 1.8 mg/ml collagen I (rat tail collagen, Corning), 0.2 mg/ml basement membrane extract (reduced growth factor, Trevigen) matrix which were loaded into a 96-well tissue culture insert plate (Corning). 25 µg of supernatants were placed in the lower chamber and cells invaded for 18h before gels were removed. Membranes were fixed and stained with DAPI, and cells quantified to calculate total percent invasion based on total cells counted/total cells seeded.
Flow Cytometry on In Vivo Tissues
Cells from in vivo digested lymph nodes and lungs were dissociated as previously described and stained with live/dead reactive dye, anti-mouse CD45 PerCP-Cy5.5 (eBioscience, Cat. # 45-0451-82), anti-mouse CD31 FITC (eBioscience, Cat. # 11-0311-82), and anti-mouse gp38 PE-Cy7 (eBioscience, Cat. # 25-5381-82). Flow cytometry samples were processed using the Millipore Guava easyCyte 8HT Flow Cytometer and analyzed using InCyte software for total LEC counts per mg as well as percentage of LECs. Gating was done first on live cells, followed by CD45- populations. This subpopulation was gated for gp38 and CD31 with the following populations: CD31+gp38+ (LECs); CD31+gp38-(blood endothelial cells), and CD31-gp38+(fibroblastic reticular cells).
Statistical Analysis
All data are presented as mean ± standard error of the mean (SEM). One-way or two-way ANOVA followed by Tukey’s multiple comparison test was used for statistical analysis of unmatched groups. Two group comparisons of normally distributed data as assessed by QQ plots were performed using unpaired t tests (with Welch’s correction if standard deviations were unequal), while comparisons of non-normal data were performed using Mann-Whitney U tests. Statistical analyses were run using Graphpad Prism software. p<0.05 is considered statistically significant. All in vitro assays were performed with a minimum of three biological replicates unless otherwise noted, murine study numbers are noted in legends and by individual graphed data points. Graphs were generated using Graphpad Prism software and are shown with mean +/- standard error.
Figure Generation
Figures were generated using Adobe Creative Suite (Photoshop and Illustrator). Schematics were generated using BioRender with license to JMM.
Data Availability Statement
The original contributions presented in the study are included in the article/Supplementary Material. Further inquiries can be directed to the corresponding author.
Ethics Statement
The studies involving human participants were reviewed and approved by University of Virginia Institutional Review Board. The patients/participants provided their written informed consent to participate in this study. The animal study was reviewed and approved by University of Virginia Animal Care and Use Committee.
Author Contributions
Conceptualization: AH and JM. Investigation: AH, MA, FA, MB, SE, MP, CR, DL, RB, RCC, KT, JM. Formal analysis: AH, SE, DL, RC, JS, and ST. Data curation: DL, CP, PD, AMi, and CL. Project administration: AH and JM. Resources: SP-C, CL, RP, MR, CP and JM. Software: AMa, RC, ST, and JS. Validation: SE, MA, FA, CP, AH, JM. Visualization: AH, RC, SE, and JM. Writing of original manuscript: AH and JM. Review and editing of manuscript: JM, AH, SE, MR, RP, and all authors. All authors contributed to the article and approved the submitted version.
Funding
Funding provided to JMM from the Kincaid Foundation and the University of Virginia Cancer Center. Funding to CL from the Marty Whitlow Fund. Funding to SMP from NIH R21EY028868. Funding provided to MRR by a Susan G Komen Career Catalyst award CCR17483602 and ACS-IRG-17-097-31 and from the University of Virginia Cancer Center and support from NCI Cancer Center Support grant P30CA44570 as startup funds. Funding to ARH from NIHGMS T32GM0087155, funding to MJP from NCI T32CA009109, and funding to MB and CBR from NIAID T32AI007496.
Conflict of Interest
The authors declare that the research was conducted in the absence of any commercial or financial relationships that could be construed as a potential conflict of interest.
Publisher’s Note
All claims expressed in this article are solely those of the authors and do not necessarily represent those of their affiliated organizations, or those of the publisher, the editors and the reviewers. Any product that may be evaluated in this article, or claim that may be made by its manufacturer, is not guaranteed or endorsed by the publisher.
Acknowledgments
The authors would like to acknowledge Janet Cross, Scott Verbridge, J. Brandon Dixon, and Joshua Hooks for useful discussion. They’d also like to acknowledge Sharon Michelhaugh and Jennifer Hammel for help with reviews.
Supplementary Material
The Supplementary Material for this article can be found online at: https://www.frontiersin.org/articles/10.3389/fonc.2022.801764/full#supplementary-material
References
1. Chabner BA, Roberts TG. Chemotherapy and the War on Cancer. Nat Rev Cancer (2005) 5:65–72. doi: 10.1038/nrc1529
2. Scully O, Bay B, Yip G, Yu Y. Breast Cancer Metastasis. Cancer Genomics Proteomics (2012) 9:311–20. doi: 10.1103/PhysRevLett.81.5457
3. Weidle UH, Birzele F, Kollmorgen G, Rueger R. Mechanisms and Targets Involved in Dissemination of Ovarian Cancer. Cancer Genomics Proteomics (2016) 13:407–23. doi: 10.21873/cgp.20004
4. Lu H, Chen I, Shimoda LA, Tran L, Zhang H, Semenza Correspondence GL, et al. Chemotherapy-Induced Ca 2+ Release Stimulates Breast Cancer Stem Cell Enrichment. Cell Rep (2017) 18(8):1946–57. doi: 10.1016/j.celrep.2017.02.001
5. Volk-Draper L, Hall K, Griggs C, Rajput S, Kohio P, DeNardo D, et al. Paclitaxel Therapy Promotes Breast Cancer Metastasis in a TLR4-Dependent Manner. Cancer Res (2014) 74:5421–34. doi: 10.1158/0008-5472.CAN-14-0067
6. Shah PP, Dupre TV, Siskind LJ, Beverly LJ. Common Cytotoxic Chemotherapeutics Induce Epithelial- Mesenchymal Transition (EMT) Downstream of ER Stress. Oncotarget (2017) 8:22625–39. doi: 10.18632/oncotarget.15150
7. Correia AL, Bissell MJ. The Tumor Microenvironment Is a Dominant Force in Multidrug Resistance. Drug Resistance Updates (2012) 15:39–49. doi: 10.1016/j.drup.2012.01.006
8. Nakasone ES, Askautrud HA, Kees T, Park JH, Plaks V, Ewald AJ, et al. Imaging Tumor-Stroma Interactions During Chemotherapy Reveals Contributions of the Microenvironment to Resistance. Cancer Cell (2012) 21:488–503. doi: 10.1016/j.ccr.2012.02.017
9. Wong SY, Hynes RO. Lymphatic or Hematogenous Dissemination: How Does a Metastatic Tumor Cell Decide? Cell Cycle (2006) 5:812–7. doi: 10.4161/cc.5.8.2646
10. Lin J, Lalani AS, Harding TC, Gonzalez M, Wu WW, Luan B, et al. Inhibition of Lymphogenous Metastasis Using Adeno-Associated Virus-Mediated Gene Transfer of a Soluble VEGFR-3 Decoy Receptor. Cancer Res (2005) 65:6901–9. doi: 10.1158/0008-5472.CAN-05-0408
11. Stacker SA, Williams SP, Karnezis T, Shayan R, Fox SB, Achen MG. Lymphangiogenesis and Lymphatic Vessel Remodelling in Cancer. Nat Rev Cancer (2014) 14:159–72. doi: 10.1038/nrc3677
12. Lee E, Fertig EJ, Jin K, Sukumar S, Pandey NB, Popel AS. Breast Cancer Cells Condition Lymphatic Endothelial Cells Within Pre-Metastatic Niches to Promote Metastasis. Nat Commun (2014) 5:1–16. doi: 10.1038/ncomms5715
13. Christiansen A, Detmar M. Lymphangiogenesis and Cancer. Genes Cancer (2011) 2:1146–58. doi: 10.1177/1947601911423028
14. Su JL, Yen CJ, Chen PS, Chuang SE, Hong CC, Kuo IH, et al. The Role of the VEGF-C/VEGFR-3 Axis in Cancer Progression. Br J Cancer (2007) 96:541–5. doi: 10.1038/sj.bjc.6603487
15. Zong S, Li H, Shi Q, Liu S, Li W, Hou F. Prognostic Significance of VEGF-C Immunohistochemical Expression in Colorectal Cancer: A Meta-Analysis ☆. (2016). doi: 10.1016/j.cca.2016.04.037
16. Alishekevitz D, Gingis-Velitski S, Kaidar-Person O, Gutter-Kapon L, Scherer SD, Raviv Z, et al. Macrophage-Induced Lymphangiogenesis and Metastasis Following Paclitaxel Chemotherapy Is Regulated by VEGFR3. Cell Rep (2016) 17:1344–56. doi: 10.1016/j.celrep.2016.09.083
17. Harris AR, Perez MJ, Munson JM. Docetaxel Facilitates Lymphatic-Tumor Crosstalk to Promote Lymphangiogenesis and Cancer Progression. BMC Cancer (2018), 1–16. doi: 10.1186/s12885-018-4619-8
18. Zhu J-S, Xu Z-P, Chen J-L, Zhang Q, Zhang Q, Sun Q. Effects of NM-3 on Lymphatic Vessel Density and Vascular Endothelial Growth Factor of Colon Cancer in Orthotopic Implantation Model of a Severe Combined Immune Deficiency Mice. Gastroenterol Insights (2010) 2:45–8. doi: 10.4081/gi.2010.e12
19. Silver DP, Richardson AL, Eklund AC, Wang ZC, Szallasi Z, Li Q, et al. Efficacy of Neoadjuvant Cisplatin in Triple-Negative Breast Cancer. J Clin Oncol (2010) 28:1145–53. doi: 10.1200/JCO.2009.22.4725
20. Sikov WM, Berry DA, Perou CM, Singh B, Cirrincione CT, Tolaney SM, et al. Impact of the Addition of Carboplatin and/or Bevacizumab To Neoadjuvant Once-Per-Week Paclitaxel Followed by Dose-Dense Doxorubicin and Cyclophosphamide on Pathologic Complete Response Rates in Stage II to III Triple-Negative Breast Cancer: CALGB 40603 (Alliance). J Clin Oncol (2015) 33(1):13–21. doi: 10.1200/JCO.2014.57.0572
21. Paoletti X, Lewsley L-A, Daniele G, Cook A, Yanaihara N, Tinker A, et al. Assessment of Progression-Free Survival as a Surrogate End Point of Overall Survival in First-Line Treatment of Ovarian Cancer: A Systematic Review and Meta-Analysis. JAMA Network Open (2020) 3:e1918939. doi: 10.1001/jamanetworkopen.2019.18939
22. Alitalo A, Detmar M. Interaction of Tumor Cells and Lymphatic Vessels in Cancer Progression. Oncogene (2011) 31:4499–508. doi: 10.1038/onc.2011.602
23. Miteva DO, Rutkowski JM, Dixon JB, Kilarski W, Shields JD, Swartz MA. Transmural Flow Modulates Cell and Fluid Transport Functions of Lymphatic Endothelium. Circ Res (2010) 106:920–31. doi: 10.1161/CIRCRESAHA.109.207274
24. Kim KA, Jung JH, Kang IG, Choi YS, Kim ST. ROS Is Involved in Disruption of Tight Junctions of Human Nasal Epithelial Cells Induced by HRV16. Laryngoscope (2018) 128:E393–401. doi: 10.1002/lary.27510
25. Kim SR, Bae YH, Bae SK, Choi KS, Yoon KH, Koo TH, et al. Visfatin Enhances ICAM-1 and VCAM-1 Expression Through ROS-Dependent NF-κb Activation in Endothelial Cells. Biochim Biophys Acta - Mol Cell Res (2008) 1783:886–95. doi: 10.1016/j.bbamcr.2008.01.004
26. Azimi MS, Motherwell JM, Murfee WL. An Ex Vivo Method for Time-Lapse Imaging of Cultured Rat Mesenteric Microvascular Networks. (2018). doi: 10.3791/55183
27. Ma Q, Dieterich LC, Ikenberg K, Bachmann SB, Mangana J, Proulx ST, et al. Unexpected Contribution of Lymphatic Vessels to Promotion of Distant Metastatic Tumor Spread. Sci Adv (2018) 4:eaat4758. doi: 10.1126/sciadv.aat4758
28. Rutkowski MR, Allegrezza MJ, Svoronos N, Tesone AJ, Stephen TL, Perales-Puchalt A, et al. Initiation of Metastatic Breast Carcinoma by Targeting of the Ductal Epithelium With Adenovirus-Cre: A Novel Transgenic Mouse Model of Breast Cancer Video Link. J Vis Exp (2014) (85):51171. doi: 10.3791/51171
29. He Y, Rajantie I, Pajusola K, Jeltsch M, Holopainen T, Yla-Herttuala S, et al. Vascular Endothelial Cell Growth Factor Receptor 3-Mediated Activation of Lymphatic Endothelium Is Crucial for Tumor Cell Entry and Spread via Lymphatic Vessels. Cancer Res (2005) 65:4739–46. doi: 10.1158/0008-5472.CAN-04-4576
30. Wang J, Guo Y, Wang B, Bi J, Li K, Liang X, et al. Lymphatic Microvessel Density and Vascular Endothelial Growth Factor-C and -D as Prognostic Factors in Breast Cancer: A Systematic Review and Meta-Analysis of the Literature. Mol Biol Rep (2012) 39(12):11153–65. doi: 10.1007/s11033-012-2024-y
31. Muralidharan V, Nguyen L, Banting J, Christophi C. The Prognostic Significance of Lymphatics in Colorectal Liver Metastases. HPB Surg (2014) 2014:954604. doi: 10.1155/2014/954604
32. Ji R-C. Lymphatic Endothelial Cells, Tumor Lymphangiogenesis and Metastasis: New Insights Into Intratumoral and Peritumoral Lymphatics. (2006). doi: 10.1007/s10555-006-9026-y
33. Sun Y, Campisi J, Higano C, Beer TM, Porter P, Coleman I, et al. Treatment-Induced Damage to the Tumor Microenvironment Promotes Prostate Cancer Therapy Resistance Through WNT16B. Nat Med (2012) 18:1359–68. doi: 10.1038/nm.2890
34. Hsu H-S, Liu C-C, Lin J-H, Hsu T-W, Hsu J-W, Su K. Involvement of ER Stress, PI3K/ AKT Activation, and Lung Fibroblast Proliferation in Bleomycin-Induced Pulmonary Fibrosis. Sci Rep (2017) 7(1):14272. doi: 10.1038/s41598-017-14612-5
35. Kleih M, Böpple K, Dong M, Gaißler A, Heine S, Olayioye MA, et al. Direct Impact of Cisplatin on Mitochondria Induces ROS Production That Dictates Cell Fate of Ovarian Cancer Cells. Cell Death Dis (2019) 10:1–12. doi: 10.1038/s41419-019-2081-4
36. Reczek CR, Chandel NS. The Two Faces of Reactive Oxygen Species in Cancer. Annu Rev Cancer Biol (2017) 1:79–98. doi: 10.1146/annurev-cancerbio-041916-065808
37. Weng M-S, Chang J-H, Hung W-Y, Yang Y-C, Chien M-H. The Interplay of Reactive Oxygen Species and the Epidermal Growth Factor Receptor in Tumor Progression and Drug Resistance. J Exp Clin Cancer Res (2018) 37(1):61. doi: 10.1186/s13046-018-0728-0
38. Fatehi M, Hunt C, Ma R, Toyota BD. Persistent Disparities in Survival for Patients With Glioblastoma. World Neurosurgery (2018) 120:e511–6. doi: 10.1016/j.wneu.2018.08.114
39. Dewhirst MW, Secomb TW. Transport of Drugs From Blood Vessels to Tumour Tissue. Nat Rev Cancer (2017) 17:738–50. doi: 10.1038/nrc.2017.93
40. Bernal-Estévez D, Sánchez R, Tejada RE, Parra-López C. Chemotherapy and Radiation Therapy Elicits Tumor Specific T Cell Responses in a Breast Cancer Patient. BMC Cancer (2016) 16:591. doi: 10.1186/s12885-016-2625-2
41. O’Donnell T, Christie EL, Ahuja A, Buros J, Aksoy BA, Bowtell DDL, et al. Chemotherapy Weakly Contributes to Predicted Neoantigen Expression in Ovarian Cancer. BMC Cancer (2018) 18:87. doi: 10.1186/s12885-017-3825-0
42. Hato SV, Khong A, Jolanda I, De Vries M, Lesterhuis WJ. Molecular Pathways: The Immunogenic Effects of Platinum- Based Chemotherapeutics CME Staff Planners’ Disclosures Learning Objectives. Clin Cancer Res (2014) 20:2831–7. doi: 10.1158/1078-0432.CCR-13-3141
43. Kern P, Kalisch A, Kolberg H-C, Kimmig R, Otterbach F, Minckwitz Gv, et al. Neoadjuvant, Anthracycline-Free Chemotherapy With Carboplatin and Docetaxel in Triple-Negative, Early-Stage Breast Cancer: A Multicentric Analysis of Feasibility and Rates of Pathologic Complete Response. CHE (2013) 59:387–94. doi: 10.1159/000362756
44. Egger SJ, Willson ML, Morgan J, Walker HS, Carrick S, Ghersi D, et al. Platinum-Containing Regimens for Metastatic Breast Cancer. Cochrane Database Systematic Rev (2017) 6(6):CD003374. doi: 10.1002/14651858.CD003374.pub4
45. Davis A, Tinker AV, Friedlander M. “Platinum Resistant” Ovarian Cancer: What Is It, Who to Treat and How to Measure Benefit? Gynecol Oncol (2014) 133:624–31. doi: 10.1016/j.ygyno.2014.02.038
46. Widodo I, Ferronika P, Harijadi A, Triningsih FE, Utoro T. Soeripto Null. Clinicopathological Significance of Lymphangiogenesis and Tumor Lymphovascular Invasion in Indonesian Breast Cancers. Asian Pac J Cancer Prev (2013) 14:997–1001. doi: 10.7314/apjcp.2013.14.2.997
47. Zhang Y, Liu Y, Shen D, Zhang H, Huang H, Li S, et al. Detection and Prognostic Value of Intratumoral and Peritumoral Lymphangiogenesis in Colorectal Cancer. Trans Cancer Res (2017) 7(1):14272. doi: 10.21037/tcr-20-1038
48. Brodeur MN, Simeone K, Leclerc-Deslauniers K, Fleury H, Carmona E, Provencher DM, et al. Carboplatin Response in Preclinical Models for Ovarian Cancer: Comparison of 2D Monolayers, Spheroids, Ex Vivo Tumors and In Vivo Models. Sci Rep (2021) 11:18183. doi: 10.1038/s41598-021-97434-w
49. Zhu X, Xu J, Cai H, Lang J. Carboplatin and Programmed Death-Ligand 1 Blockade Synergistically Produce a Similar Antitumor Effect to Carboplatin Alone in Murine ID8 Ovarian Cancer Model. J Obstetrics Gynaecol Res (2018) 44:303–11. doi: 10.1111/jog.13521
50. Etienne MC, Leger F, Pivot X, Chatelut E, Bensadoun RJ, Guardiola E, et al. Pharmacokinetics of Low-Dose Carboplatin and Applicability of a Method of Calculation for Estimating Individual Drug Clearance. Ann Oncol (2003) 14:643–7. doi: 10.1093/annonc/mdg162
51. Souza CMde, Gamba C de O, Campos CBde, Lopes MTP, Ferreira MAND, Andrade SP, et al. Carboplatin Delays Mammary Cancer 4T1 Growth in Mice. Pathol - Res Pract (2013) 209:24–9. doi: 10.1016/j.prp.2012.10.003
52. Stolarz AJ, Sarimollaoglu M, Marecki JC, Fletcher TW, Galanzha EI, Rhee SW, et al. Doxorubicin Activates Ryanodine Receptors in Rat Lymphatic Muscle Cells to Attenuate Rhythmic Contractions and Lymph Flow. J Pharmacol Exp Ther (2019) 371:278–89. doi: 10.1124/jpet.119.257592
53. Ayre K, Parker C. Lymphedema After Treatment of Breast Cancer: A Comprehensive Review. J Unexplored Med Data (2019) 4:5. doi: 10.20517/2572-8180.2019.02
54. Biglia N, Zanfagnin V, Daniele A, Robba E, Bounous VE. Lower Body Lymphedema in Patients With Gynecologic Cancer. Anticancer Res (2017) 37:4005–15. doi: 10.21873/anticanres.11785
55. Ogata F, Fujiu K, Matsumoto S, Nakayama Y, Shibata M, Oike Y, et al. Excess Lymphangiogenesis Cooperatively Induced by Macrophages and CD4+ T Cells Drives the Pathogenesis of Lymphedema. J Invest Dermatol (2016) 136:706–14. doi: 10.1016/j.jid.2015.12.001
56. He Y, Kozaki K-I, Karpanen T, Koshikawa K, Yla-Herttuala S, Takahashi T, et al. Suppression of Tumor Lymphangiogenesis and Lymph Node Metastasis by Blocking Vascular Endothelial Growth Factor Receptor 3 Signaling. J Natl Cancer Inst (2002) 94(11):819–25. doi: 10.1093/jnci/94.11.819
57. Sini P, Samarzija I, Baffert F, Littlewood-Evans A, Schnell C, Theuer A, et al. Inhibition of Multiple Vascular Endothelial Growth Factor Receptors (VEGFR) Blocks Lymph Node Metastases But Inhibition of VEGFR-2 Is Sufficient to Sensitize Tumor Cells to Platinum-Based Chemotherapeutics. Cancer Res (2008) 68:1581–92. doi: 10.1158/0008-5472.CAN-06-4685
58. Krishnan J, Kirkin V, Steffen A, Hegen M, Weih D, Tomarev S, et al. Differential In Vivo and In Vitro Expression of Vascular Endothelial Growth Factor (VEGF)-C and VEGF-D in Tumors and Its Relationship to Lymphatic Metastasis in Immunocompetent Rats. Cancer Res (2003) 63:713–22.
59. Karpanen T, Egeblad M, Karkkainen MJ, Kubo H, Ylä-Herttuala S, Jäättelä M, et al. Vascular Endothelial Growth Factor C Promotes Tumor Lymphangiogenesis and Intralymphatic Tumor Growth. Cancer Res (2001) 61:1786–90.
60. Saif MW, Knost JA, Chiorean EG, Kambhampati SRP, Yu D, Pytowski B, et al. Phase 1 Study of the Anti-Vascular Endothelial Growth Factor Receptor 3 Monoclonal Antibody LY3022856/IMC-3C5 in Patients With Advanced and Refractory Solid Tumors and Advanced Colorectal Cancer. Cancer Chemother Pharmacol (2016) 78:815–24. doi: 10.1007/s00280-016-3134-3
61. Kirkin V, Thiele W, Baumann P, Mazitschek R, Rohde K, Fellbrich G, et al. MAZ51, an Indolinone That Inhibits Endothelial Cell and Tumor Cell Growth In Vitro, Suppresses Tumor Growth In Vivo. Int J Cancer (2004) 112:986–93. doi: 10.1002/ijc.20509
62. Morris CJ, Kameny RJ, Boehme J, Gong W, He Y, Zhu T, et al. KLF2-Mediated Disruption of PPAR-γ Signaling in Lymphatic Endothelial Cells Exposed to Chronically Increased Pulmonary Lymph Flow. Am J Physiology-Heart Circulatory Physiol (2018) 315:H173–81. doi: 10.1152/ajpheart.00635.2017
63. Szklarczyk D, Franceschini A, Wyder S, Forslund K, Heller D, Huerta-Cepas J, et al. STRING V10: Protein-Protein Interaction Networks, Integrated Over the Tree of Life. Nucleic Acids Res (2015) 43:D447–52. doi: 10.1093/nar/gku1003
64. Vlachos IS, Zagganas K, Paraskevopoulou MD, Georgakilas G, Karagkouni D, Vergoulis T, et al. DIANA-Mirpath V3.0: Deciphering microRNA Function With Experimental Support. Nucleic Acids Res (2015) 43:W460. doi: 10.1093/NAR/GKV403
65. Tibes R, Qiu Y, Lu Y, Hennessy B, Andreeff M, Mills GB, et al. Reverse Phase Protein Array: Validation of a Novel Proteomic Technology and Utility for Analysis of Primary Leukemia Specimens and Hematopoietic Stem Cells. Mol Cancer Ther (2006) 5:2512–21. doi: 10.1158/1535-7163.MCT-06-0334
66. Kamburov A, Wierling C, Lehrach H, Herwig R. ConsensusPathDB–a Database for Integrating Human Functional Interaction Networks. Nucleic Acids Res (2009) 37:D623–8. doi: 10.1093/nar/gkn698
67. Azimi MS, Lacey M, Mondal D, Murfee WL. An Ex Vivo Tissue Culture Model for Anti-Angiogenic Drug Testing. Methods Mol Biol (2016) 1464:85–95. doi: 10.1007/978-1-4939-3999-2_8
68. Broggi MAS, Schmaler M, Lagarde N, Rossi SW. Isolation of Murine Lymph Node Stromal Cells. J Vis Exp (2014) (90):e51803. doi: 10.3791/51803
69. Siddik ZH, Newell DR, Boxall FE, Harrap KR. The Comparative Pharmacokinetics of Carboplatin and Cisplatin in Mice and Rats. Biochem Pherntacoogy (1987) 36. doi: 10.1016/0006-2952(87)90490-4
Keywords: platinum, chemotherapy, lymphangiogenesis, metastasis, breast cancer, ovarian cancer, lymphatic endothelial cells, anti-VEGFR3 therapy
Citation: Harris AR, Esparza S, Azimi MS, Cornelison R, Azar FN, Llaneza DC, Belanger M, Mathew A, Tkachenko S, Perez MJ, Rosean CB, Bostic RR, Cornelison RC, Tate KM, Peirce-Cottler SM, Paquette C, Mills A, Landen CN, Saucerman J, Dillon PM, Pompano RR, Rutkowski MA and Munson JM (2022) Platinum Chemotherapy Induces Lymphangiogenesis in Cancerous and Healthy Tissues That Can be Prevented With Adjuvant Anti-VEGFR3 Therapy. Front. Oncol. 12:801764. doi: 10.3389/fonc.2022.801764
Received: 25 October 2021; Accepted: 31 January 2022;
Published: 17 March 2022.
Edited by:
Angela Toss, University of Modena and Reggio Emilia, ItalyReviewed by:
Lasse Dahl Ejby Jensen, Linköping University, SwedenMonika Ehnman, Karolinska Institutet (KI), Sweden
Copyright © 2022 Harris, Esparza, Azimi, Cornelison, Azar, Llaneza, Belanger, Mathew, Tkachenko, Perez, Rosean, Bostic, Cornelison, Tate, Peirce-Cottler, Paquette, Mills, Landen, Saucerman, Dillon, Pompano, Rutkowski and Munson. This is an open-access article distributed under the terms of the Creative Commons Attribution License (CC BY). The use, distribution or reproduction in other forums is permitted, provided the original author(s) and the copyright owner(s) are credited and that the original publication in this journal is cited, in accordance with accepted academic practice. No use, distribution or reproduction is permitted which does not comply with these terms.
*Correspondence: Jennifer M. Munson, Sm00a3RAdnQuZWR1