- 1Department of Neurosurgery, Affiliated Nanjing Brain Hospital, Nanjing Medical University, Nanjing, China
- 2Department of Neurosurgery, The Suqian Clinical College of Xuzhou Medical University, Suqian, China
- 3Division of Life Sciences and Medicine, University of Science and Technology of China, Hefei, China
- 4Department of Neurosurgery, Affiliated Hospital of Xuzhou Medical University, Xuzhou, China
- 5Department of Neuro-Psychiatric Institute, Affiliated Nanjing Brain Hospital, Nanjing Medical University, Nanjing, China
Surgical resection and postoperative radiotherapy remained the most common therapeutic modalities for malignant tumors. However, tumor recurrence after receiving such combination is difficult to be avoided because of high invasiveness and radiation resistance of cancer cells during long-term therapy. Hydrogels, as novel local drug delivery systems, presented excellent biocompatibility, high drug loading capacity and sustained drug release property. Compared with conventional drug formulations, hydrogels are able to be administered intraoperatively and directly release the entrapped therapeutic agents to the unresectable tumor sites. Therefore, hydrogel-based local drug delivery systems have their unique advantages especially in sensitizing postoperative radiotherapy. In this context, classification and biological properties of hydrogels were firstly introduced. Then, recent progress and application of hydrogels for postoperative radiotherapy were summarized. Finally, the prospects and challenges of hydrogels in postoperative radiotherapy were discussed.
1 Introduction
Malignant tumors, as the most complicated and challenging diseases, has received considerable attention all over the world (1). By now, the combination treatment of surgical resection and postoperative radiotherapy is still one of the most effective strategy, and is widely used for many kinds of tumors (2, 3). However, malignant tumor cells grow infiltratively and interweave with surrounding healthy tissues, which make it difficult to be completely resected. More importantly, the therapeutic effect of postoperative radiotherapy is limited by the radiation resistance of residual tumor cells (4, 5). These above reasons make the tumor easy to relapse, and the curative effect often dissatisfies us.
Numerous attempts (such as high-dose radiation, utilization of radiosensitizers, chemoradiotherapy and immunoradiotherapy) have been made to address the pitfalls of surgery and postoperative radiotherapy (6–9). Unfortunately, it has been proved that high-dose radiation can cause irreversible damages to the peritumoral normal tissues and further cause various adverse side effect in patients (10). Traditional radiosensitizers or chemotherapeutics could not effectively penetrate into tumor tissues, which limits their radiosensitization effects after systemic administration. In addition, the systemic toxicity, gastrointestinal side effects, myelosuppression and some other side effects can also be caused by such systemic administration (11, 12). Immunoradiotherapy has attracted great research interests in recent years. Tumor-associated antigens (TAAs) can be released from radiation-induced apoptotic cancer cells, which further cause antitumor immune responses (13). But immune-related adverse events (irAEs), which produced by an overactive immune response against healthy organs, can seriously affect the therapeutic efficacy of immunoradiotherapy (14, 15). The nonspecific distribution of conventional antitumor drugs inhibits the effectiveness of postoperative radiotherapy, and the repeated systemic administration make antitumor therapy more complicated. As a result, local drug delivery systems, especially hydrogels, have been explored to address these constraints, with the potential to simultaneously improve antitumor efficacy and minimize systemic side effects.
With the fast development of material engineering and molecular biology, hydrogel has opened up new avenues for the treatment of cancer. Hydrogel refers to a three-dimensional network gel constituted with hydrophilic polymers, which has good permeability and excellent biocompatibility. It can be directly applied to the pathological tissue and has a wide range of applications prospects in the field of biological medicine (16–22). Hydrogel can be injected or sprayed into postoperative cavities, in situ gel and circumvent the biological barrier, such as blood brain barrier (23). Before reaching the tumor cells, the agents can be protected by hydrogel from being affected by severe surroundings. And the plasma half-life can be prolonged (24). To optimize therapy effectiveness and minimize systemic drug distribution, hydrogel can be designed to delivery drug to specific cells or tissues (25). In addition, the unique porous structure of the hydrogel can optimize drug sustained release properties. The controlled release properties can also be obtained by utilizing an elaborated stimuli-responsive hydrogel delivery system (26). The strategy of coadministration of different pharmaceuticals can similarly be achieved by hydrogel delivery systems, which can overcome drug resistance and boost therapeutic efficacy (27). From the above information, we can see that the hydrogel-based drug delivery systems have their unique advantages in postoperative radiotherapy. Traditional drugs formulations (such as radioisotopes, radiosensitizers, chemotherapeutic agents or immunomodulators) were recorded by encapsulating into hydrogels and combing with postoperative radiotherapy to inhibit tumor recurrence (Figure 1). This local drug delivery method can avoid the nonspecific distribution of traditional drugs, sensitize radiotherapy and achieve the combination of multiple treatment modalities.
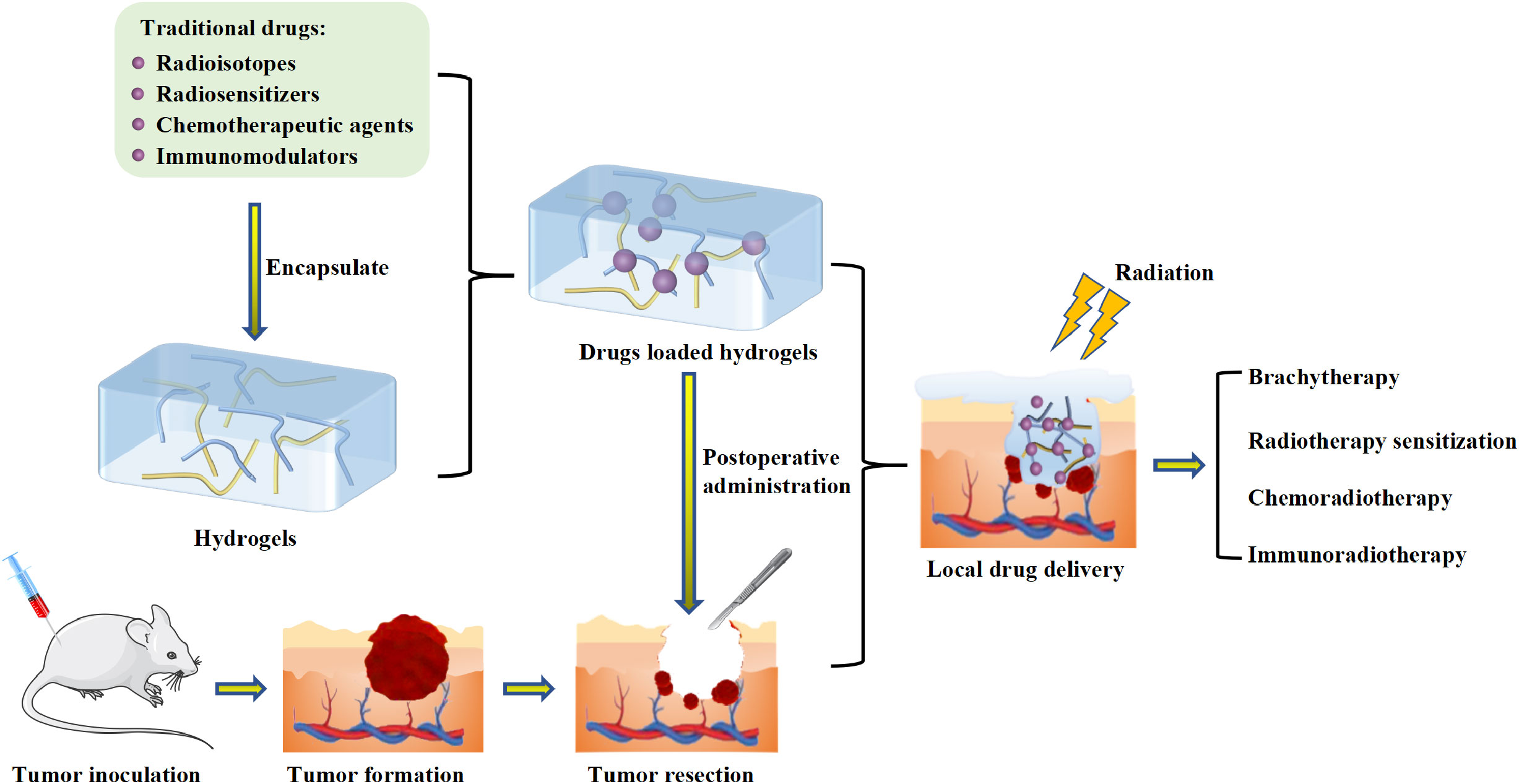
Figure 1 Schematic illumination of the application of hydrogel-based local drug delivery systems for postoperative radiotherapy.
In this review, we first introduced the classification and biological characteristics of hydrogels. Next, the applications of hydrogel-based local drug delivery systems for postoperative radiotherapy were further systematically investigated. Lastly, the prospects and challenges of hydrogel-based local drug delivery systems in postoperative radiotherapy were discussed.
2 The classification and biocompatibility of hydrogels
2.1 Classifications of hydrogels
According to different standards, hydrogels are divided into different classifications (Table 1).
a) Based on the original materials, it can be divided into natural hydrogels and synthetic hydrogels (28). The former includes alginates hydrogel, chitosan hydrogel, collagen hydrogel, hyaluronic acid hydrogel, etc (35–37). The latter is synthetized by a variety of polymers, including polyethylene glycol (PEG), poly glycolic acid (PGA) and poly lactic-co-glycolic acid (PLGA) (38–40).
b) According to the cross-linking junction types, hydrogels can be divided into physical cross-linked hydrogels and chemical cross-linked hydrogels. On the molecular level, noncovalent bonding interactions lead to physically cross-linked hydrogels. Although the connections are typically fleeting, they are enough to make hydrogels insoluble in aqueous media. Irreversible covalent cross-linking interaction produces chemically cross-linked hydrogels. Linear or branching polymers directly come into contact with one another by chemical cross-linking reaction, which leads to extremely high mechanical strength (29, 30).
c) In terms of network charge, three groups of hydrogels are categorized: anionic, cationic and neutral hydrogels. The whole network’s charge is determined by the polymer’s charge (31, 32).
d) In accordance with the polymeric compositions, hydrogels are divided into three classes: (I) Homopolymeric hydrogels (composed of a single monomer species). (II) Copolymeric hydrogels (obtained from two or more monomer species, at least one being hydrophilic). (III) Multipolymeric hydrogels (made up of two independent and cross-linked chains of polymers) (33).
e) Hydrogels can also be generally divided into conventional and intelligent hydrogels. Conventional hydrogels refer to hydrogels that are not sensitive to environment, such as temperature or pH, etc. Conventional hydrogels have a few fundamental properties. The unique porous architectures of conventional hydrogels can facilitate molecular penetration. The hard chain architecture can resist dissolution, and the flexible architecture can make conventional hydrogels extendable and collapsible (34). Intelligent hydrogels, also named as stimuli-responsive hydrogels, have the capacity to alter self-physical characteristics (swelling ability, mechanical properties, molecular diffusion, etc). It can response to a variety of environmental stimuli, such as temperature, light, pH, enzyme, electric fields, and some other biological factors (41–45). In the tumor microenvironment, stimuli-responsive hydrogels can modify its own rheological behavior (46). By encapsulating pharmaceuticals in unique response hydrogel, the medication release could be better controlled. For example, pH-responsive hydrogels can control the drug release in the acidic tumor microenvironment (47).
2.2 Biocompatibility of hydrogels
Biocompatibility is the biological property that a material can tolerate the action of various systems of the host, while the material can still remain relatively stable and not be rejected or destroyed. Excellent biocompatibility is a prerequisite for hydrogels to be widely used in biomedical fields (48). Polysaccharide is a well-known raw material of hydrogel. Zhao et al. detected its cytotoxicity in vivo and found that it did not produce acute toxic reaction to the blood system (49). Zhang et al. investigated the cytotoxicity of fibrin hydrogel to DC 2.4 cells and C57BL/6 mice. The in vitro data showed that the cell survival rate was more than 90% after coincubation with fibrin hydrogel for 24 h, and the in vivo H&E staining assay did not show obvious inflammatory response (50). Liu et al. evaluated the cytotoxicity of polymer HA-DEG/UPy hydrogel on 3T3 fibroblasts. The experimental results showed that HA-DEG/UPy hydrogel had almost no toxicity to 3T3 fibroblasts cells and the cell viability remained almost 100% (51). The high biocompatibility of hydrogel effectively avoids the generation of systemic toxicity, it can be safely applied in the treatment of diseases.
3 The application of hydrogel-based local drug delivery systems in postoperative radiotherapy
Hydrogels have the capacity to encapsulate various drugs through blending them together in precursor solution, which are then converted into three-dimensional mesh structure gel. After in vivo administration, the therapeutic substances encapsulated in hydrogels localized in the target area and the drug delivery systems sustained the drug release for an extended time (52, 53). Hydrogel based local drug delivery systems have been explored to encapsulate traditional drug (such as radioisotopes, radiosensitizers, chemotherapeutic agents, and immunotherapy agents), which can enhance the therapeutic efficacy of postoperative radiotherapy.
3.1 Hydrogels for the local delivery of radioisotopes in postoperative radiotherapy
Brachytherapy, also known as internal radiotherapy, is a kind of radiotherapy that deliver sealed radiation source to tumor tissue or post-operative tumor cavity. This therapeutic modality has been applied to the treatment of a variety of tumors (54, 55). If administered systemically, the non-specific distribution of radioisotopes have harmful effects on the normal organs of patients. Hydrogel drug delivery systems can directly transfer radioisotopes to the tumor area. It can avoid the non-specific distribution of radioisotopes and have received great attention. Meng et al. designed a CuS/131I-PEGDA/AIPH hydrogel, in which copper sulfide responsed to the near-infrared laser to raise the temperature of the tumor-bearing region. When the AIPH thermal initiator was activated, the polymer matrix PEGDA began to gel and 131I (Iodine-131) was effectively preserved in the tumor cavity. The in-situ gelation of PEGDA triggered by NIR could prolong the retention time of 131I in tumor cavity and prevent it from leaking into adjacent normal tissues. What’s more, hyperthermia, induced by NIR responsive photothermal hydrogels, could boost blood circulation and further alleviated the tumor hypoxia microenvironment. As a result, the radiation sensitivity of tumor cells could be improved (56).
Apart from 131I, the hydrogel-based delivery systems could also deliver some other radioisotopes, such as 125I (Iodine-125) and 188Re (Rhenium-188). 125I implantation had been demonstrated to be an effective approach to eradicate tumor cells, and it only cause minor damage to adjacent normal cells (57). Wu et al. constructed an injectable near-infrared induced polymerized hydrogel (125I-GPA), which could continuously release 125I-GNR-RGDY into tumor tissues. It had been successfully applied to the surgical resection model of breast cancer (22). In this experiment, hyperthermia induced by near-infrared radiation could eradicate potentially pathogenic bacteria and was helpful to prevent postoperative wound infection. Besides, the RGD peptide modification on the surface of 125I-GNR-RGDY could target tumor cells and achieve more accurate brachytherapy (Figure 2). The recurrence time of different treatment groups demonstrated that the combination treatment of 125I-GPA + NIR suppress tumor growth more effectively than GAP + NIR. And no tumor recurrence was observed in 125I-GPA + NIR treated group.
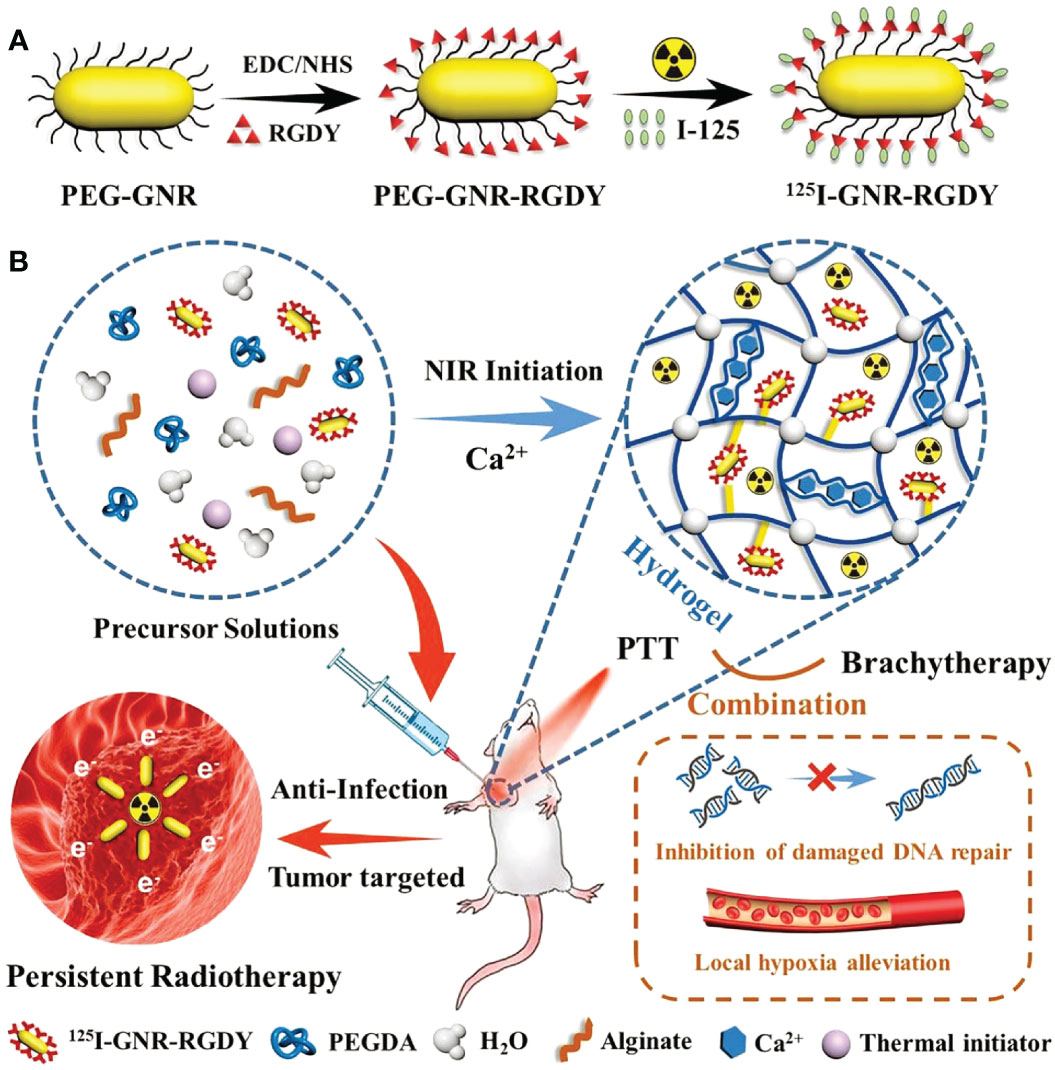
Figure 2 (A) 125I-GNR-RGDY and (B) nanocomposite double-network GPA hydrogel and their theranostic application for inhibition of postoperative breast cancer recurrence and wound infection through synergistic brachytherapy and photothermal therapy (22).
188Re is an another radionuclide with β-ray that has been frequently applied in radioisotope therapy (58, 59). Shi et al. encapsulated the radiopharmaceutical 188Re-EL in a temperature-sensitive hydrogel to construct a hybrid radioactive thermosensitive hydrogel system (188Re-EL/hydrogel), which synergistically potentiated the therapeutic efficacy of 188Re-EL on hepatocellular carcinoma (Figure 3) (60).
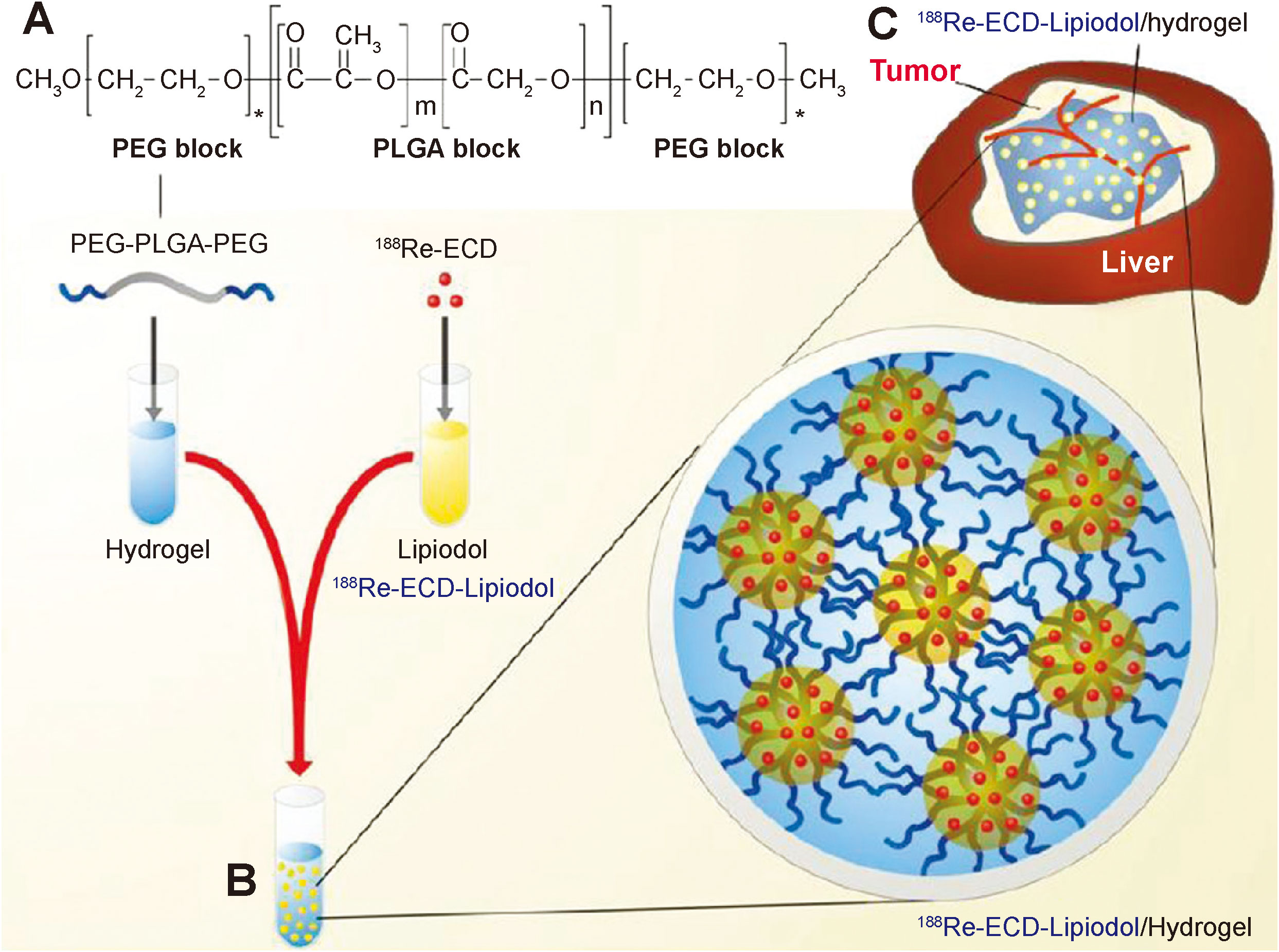
Figure 3 PEG-PLGA-PEG chemical structure, preparation of 188Re-ECD-Lipiodol/hydrogel, and hepatoma animal model treatment. (A) Chemical structure of PEG-PLGA-PEG. (B) Optical images of 188Re-ECD-Lipiodol/hydrogel, prepared by mixing PEG-PLGA-PEG (30% w/w) and 188Re-ECDLipiodol in a 1:1 ratio. (C) 188Re-ECD-Lipiodol/hydrogel was administered to N1-S1 hepatoma-bearing rats via intratumoral injection (60).
Overall, encapsulating radionuclides into hydrogels exhibit obvious advantages in treating tumors. Compared with conventional radioisotope implantation, hydrogel can prolong the retention time of radionuclides in tumors, which is conducive to improving the effectiveness of internal radiotherapy. At the same time, hydrogel can effectively prevent the implanted radioisotopes from migrating to adjacent normal cells and reduce the adverse reactions of radionuclides.
3.2 Hydrogels for the local delivery of radiosensitizers in postoperative radiotherapy
Ionizing radiation, which includes high-energy X-rays, gamma rays, heavy ions, as well as electrons, is used in radiotherapy to directly produce DNA damage, or indirectly induce cell death by stimulating the generation of massive numbers of toxic reactive oxygen species (ROS) (61). However, abnormal vasculature and insufficient blood flow together contribute to hypoxia in solid tumors, which significantly reduces the effectiveness of radiotherapy and leads to radiation resistance (62). Besides, radiotherapy has the characteristic that significantly suppresses tumor growth in a dose-dependent manner, and its effectiveness is typically restricted by the maximum radiation dose which can be applied to the tumor area without causing serious damage to surrounding tissues (63). Therefore, radiosensitizers (such as nitroimidazoles, cisplatin, metal-based nanoparticles and so on) have been developed to minimize peripheral tissue lesion while maintaining sufficient ionization damage to tumors (64, 65). Hydrogels, as local drug carriers, can be injected into surgical cavities and overcome the limitation of physiological barriers for traditional drug delivery. It has been applied to the administration of various radiosensitizers to tumor areas, generating a synergistic radiosensitive effect (66).
Glioblastoma (GBM), the most common primary malignant brain tumors, have a median survival of only 12-15 months (67). The radiation tolerance of glioma cells limits the therapeutic effect of radiotherapy. Worse still, the presence of blood brain barrier (BBB) makes traditional anticancer agents ineffective, resulting in unexpected GBM recurrence and poor prognosis for patients (68, 69). To overcome this limitation, Liang et al. reported a thermal-sensitive hydrogel encapsulating carboplatin that can be injected into the surgical cavity, circumventing BBB and delivering radiosensitizer to improve the postoperative radiotherapy of GBM (Figure 4) (70). In this research, carboplatin was used as radiosensitizer to prevent radiation-induced DNA damage from being repaired, making radiation therapy more sensitive. This research found that intratumorally administered hydrogels have the advantages of lowering systemic toxicity, bypassing the BBB and simplifying the drug delivery frequency. It is indicated that the injection of hydrogel systems containing smart radiosensitizers into the surgical cavity, combined with ionizing radiation, is a promising treatment strategy for glioblastoma.
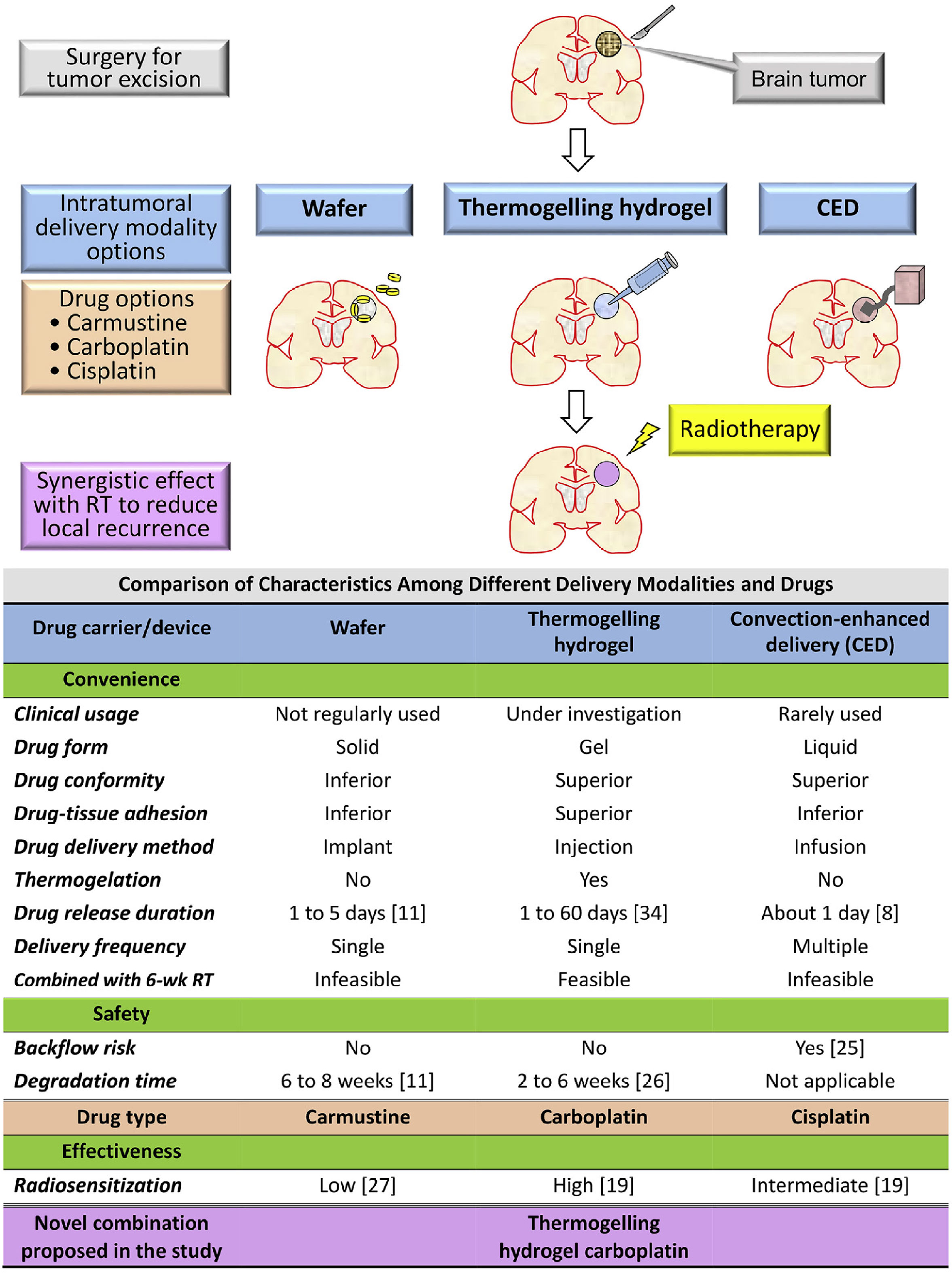
Figure 4 Rationale and purpose of this study: To compare the characteristics of intratumoral delivery modalities and drugs for malignant gliomas and propose a novel combination to satisfy the unmet clinical need of convenience, effectiveness, and safety. RT, radiotherapy; wk, week (70).
Oxygen, acknowledged as the definitive hypoxic cell radiosensitizer, has been widely used to enhance radiotherapeutic efficacy (71, 72). Yang et al. constructed an oxygen-enriched thermosensitive hydrogel that steadily provided exogenous oxygen playing as a potent radiosensitization role in increasing the radiosensitivity of tumor cells (16). As shown in Figure 5, exogenous oxygen sustained released from O2@PFOB@Gel and induced reoxygenation of hypoxic tumor, successfully eliminating the hypoxia-related radiation resistance and significantly suppressing the tumor growth.
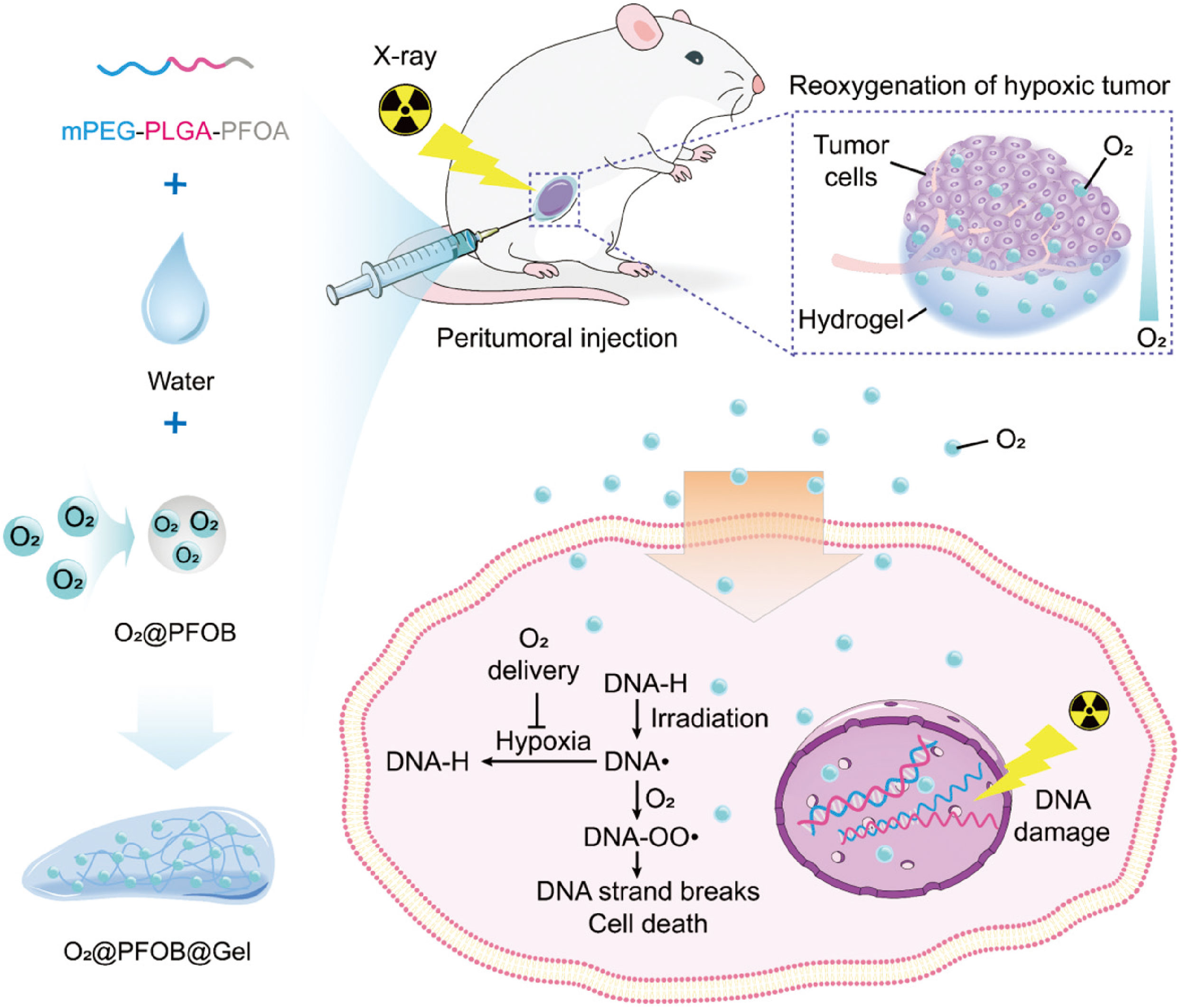
Figure 5 Schematic representation of the preparation of an oxygen-enriched thermosensitive composite hydrogel (O2@PFOB@Gel) and its radiosensitizing effect on tumor. The thermosensitive composite hydrogel undergoing a thermoreversible sol–gel transition is made up of a PFOA-modified mPEG–PLGA diblock copolymer and PFOB. After peritumoral injection of the O2@PFOB@Gel system, the hypoxic tumor microenvironment gains great alleviation via the sustained release of oxygen. As the released oxygen reacts with a damaged segment of DNA strand that is induced by X-ray exposure, the repair of DNA strand breaks is inhibited, resulting in more cell death. Consequently, a radiosensitizing effect is achieved (16).
Sunitinib, as a small molecule multikinase inhibitor targeting VEGF receptors, has powerful antiangiogenic properties and has been proposed as a radiosensitier (73, 74). Fu et al. introduced a matrix metalloproteinase (MMP) -responsive hydrogel loaded with sunitinib nanoparticles (NS-MRH) to improve radiosensitivity and prevent local breast cancer recurrence (75). By being injected into the postoperative cavity and continuously releasing sunitinib, the constructed hydrogel system in this study successfully sensitized radiation and inhibited tumor recurrence.
3.3 Hydrogels for the local delivery of chemotherapeutic agents in postoperative radiotherapy
Postoperative concurrent chemoradiotherapy, which involves the simultaneous administration of chemotherapeutics and radiotherapy following surgery, has become the standard treatment for various solid tumors including lung cancers, esophageal cancer, gastrointestinal malignancies, as well as brain tumors. During the treatment of radiotherapy, normal tissues are also subjected to radiation exposure. When the radiation dose exceeds the maximum tolerance level of normal tissues, radioactive necrosis will occur (76). Traditional chemotherapeutic agents have been proven to sensitize radiotherapy in solid tumors, but their nonspecific tissue distribution usually causes severe damages to normal tissues and organs (77).
Because of the unique characteristics of intraoperative administration, sustained drug release and high drug loading, hydrogel has been widely employed in postoperative chemoradiotherapy (78, 79). Doxorubicin (DOX), as a broad-spectrum anticancer drug, can effectively inhibit the synthesis of RNA and DNA to eliminate tumor cells. But the toxic side effects of doxorubicin, such as myelosuppression and myocardial toxicity, seriously limit its use in the clinic (80, 81). In order to minimize DOX toxicity, intelligence hydrogels have been employed. Huang et al. designed a thermos-responsive hydrogel (PEG-PLGA-PEG) for the co-delivery 131I as a radioactive source and DOX/PECT micelles as a chemotherapeutic to achieve combined chemoradiotherapy (Figure 6) (82). This hydrogel delivery system was confirmed that it enhanced the synergistic therapeutic efficacy of chemoradiotherapy and alleviated the toxic side effects of DOX. Another study utilizing DOX as the major sensitization ingredient was presented by Peng and his coworkers. 188Re-Tin and liposomal DOX were encapsulated in a thermoresponsive hydrogel (PCL-PEG-PCL) to maximize the 188Re therapeutic efficacy for hepatocellular carcinoma (83).
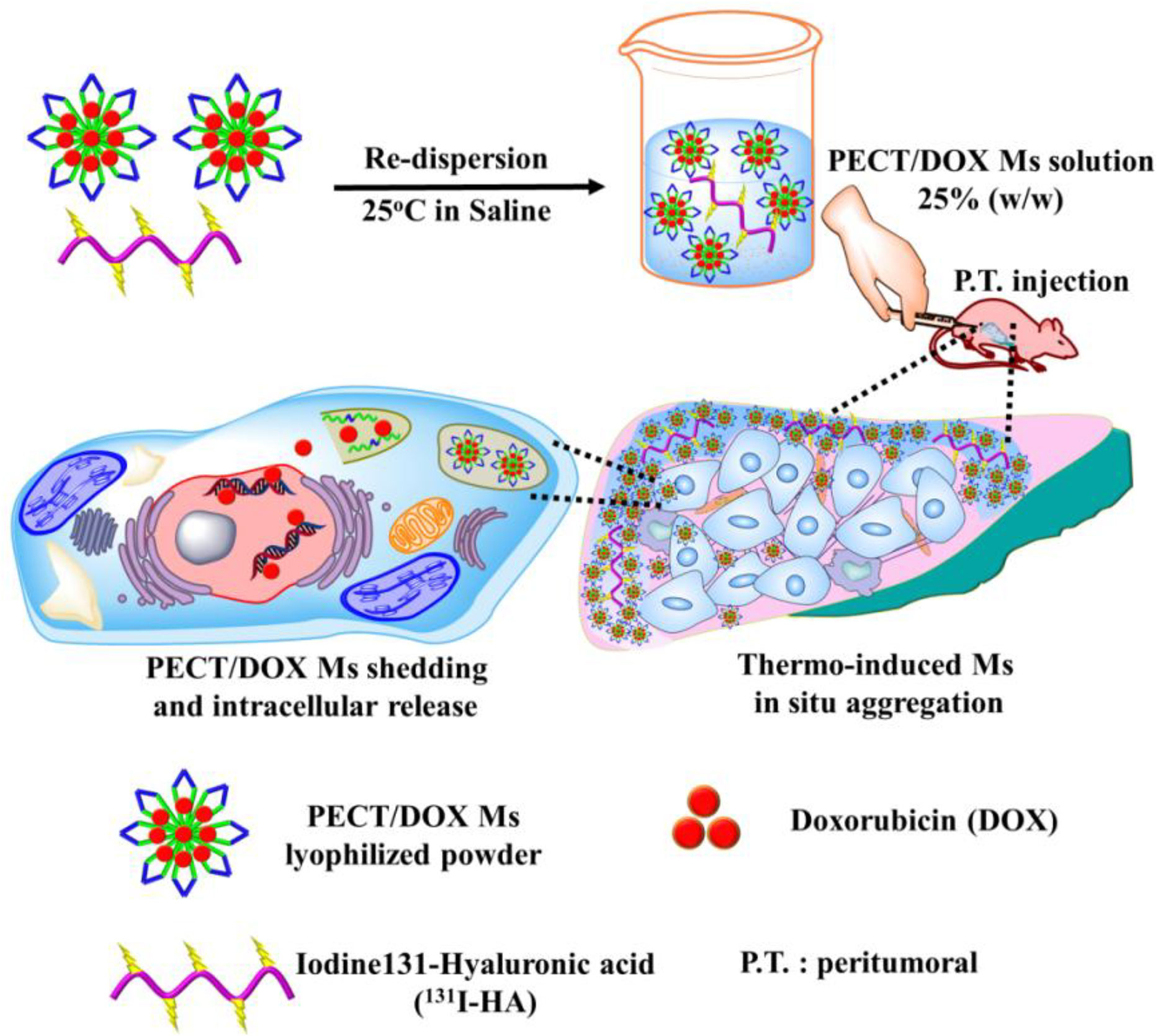
Figure 6 The schematic diagram of the formation of PECT/DOX MHg as the nanodrug and radionuclide reservoir and its following action model of in situ chemoradiotherapy (82).
3.4 Hydrogels for the local delivery of immunomodulators in postoperative radiotherapy
It has been confirmed that radiation can enhance antitumor immune responses by inducing immunogenic cell death (ICD) (84–86). Danger associated molecular patterns (DAMPs) released by ICD promote the maturation of antigen-presenting cells (APCs), the infiltration of cytotoxic T lymphocytes (CTLs) and the release of associated cytokines (87, 88). CTLs could cooperate with cytokines such as interferon-γ(IFN-γ) and tumor necrosis factor-α (TNF-α) to reverse the immunosuppressive tumor microenvironment into an immunogenic phenotype, increasing immunotherapy responses of tumor cells (89, 90). However, the increased sensitivity of immunotherapy in post-radiation therapy is only to a certain extent and radiotherapy alone could not effectively suppress distant tumor growth (91). To enhance the immune efficacy of radiation and mitigate the systemic toxic side effects of immunotherapy drugs, hydrogels were used to deliver immunomodulators after injection into the tumor cavity and obtain combination effect with radiotherapy for various tumor treatment.
Sun et al. developed the ADU-AAV-PD1@Gel, a ROS-responsive hydrogel for localized radioimmunotherapy in the glioblastoma resection mouse model (92). As shown in Figure 7, STING agonist (ADU) was used to improve tumor immunogenicity and adeno-associated virus-based-PD1 (AAV-PD1) was utilized to secret therapeutic PD-1 proteins to block the interaction between PD-1 and PD-L1 for restoring immunotherapy. ADU and AAV-PD1 were encapsulated together in a ROS-responsive hydrogel and orthotopically injected into the postoperative glioma cavity, enhanced the immune response induced by radiotherapy. This combination treatment effectively inhibited the recurrence of glioma after operation and prolonged the survival time of the model mice.
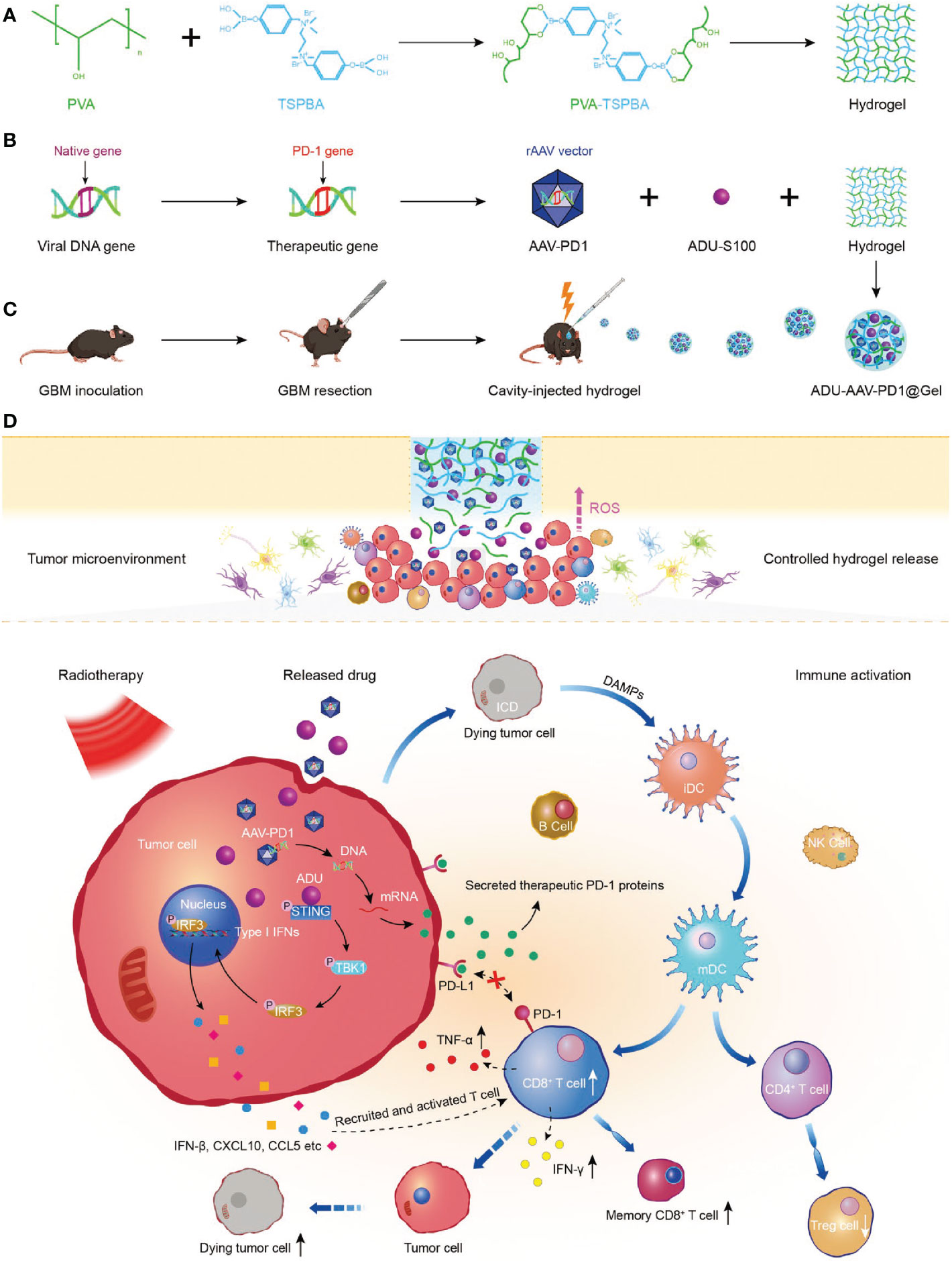
Figure 7 Preparation and mechanism of ADU-AAV-PD1@Gel. (A) Schematic illustration of the preparation of PVA-TSPBA hydrogel. (B) Flowchart of generation of the AAV-PD1 and formation of the ADU-AAV-PD1@Gel. (C) The ADU-AAV-PD1@Gel combined with RT treatment was performed in the GBM resection mice model. ADU-AAV-PD1@Gel was injected into the GBM resection cavity on day 11 after the GBM inoculation. The RT was performed on day 12, 13, and 14. (D) Mechanism of combining ADU-AAV-PD1@Gel with RT treatment for synergetic radioimmunotherapy of post-resection glioma (92).
Immune adjuvants have been shown to be highly effective in enhancing antitumor immune responses and promoting radiation-induced ICD (93–95). CpG oligodeoxynucleotide (CpG ODN) is a toll-like receptor 9 (TLR9) agonist, which is widely used as an immune adjuvant (96, 97). Liu et al. created an ATP-responsive sodium alginate hydrogel containing ATP-specific aptamers and CpG ODNs for local immunoradiotherapy (98). CpG ODNs were released in response to ATP secreted from dying cells after radiotherapy, and the released CpG had been confirmed to have the ability to boost the antitumor immunotherapy of ionizing radiation at low doses. Notably, when combined with systemic administration of PD-L1 antibody, the constructed smart hydrogel exhibited excellent ability to eliminate established tumors and inhibit distant tumor metastasis, the latter of which was made possible by long-term immune memory effect.
Taken together, these findings demonstrated that hydrogel delivery of immunomodulators can be directly injected into the operation cavity, successfully bypassing physiological barriers (such as BBB) and reducing the off target effects of immunostimulatory agents, enhancing radiation-induced immune responses, and having significant efficacy in suppressing local and distant tumors.
4 Conclusions and prospects
In situ hydrogel drug delivery system has received considerable concerns and numerous successful applications of hydrogel as anti-cancer local delivery carrier has been witnessed in recent decades. Ideal hydrogels should present biodegradable property, avoiding producing harmful substance during degradation, has high drug loading efficiency and minimize the negative effects of anti-tumor drugs. In this context, we systematically summarized the recent researches of hydrogel-based drug delivery systems in postoperative radiotherapy. Different types of anti-tumor drug agents, such as radioisotopes, radiosensitizers, chemotherapeutic molecules or immunomodulators can be encapsulated in hydrogels to achieve combined therapeutic effect for postoperative radiotherapy, which has been proven to effectively sensitize radiation therapy and successfully suppress tumor growth and recurrence.
Although many advances have been achieved in the application of hydrogels for postoperative radiotherapy, there are still some challenges to be considered. From the perspective of clinical translation, hydrogels still could not completely replace the existing adjuvant treatment methods. The design of existent hydrogel drug delivery system is too complex, which is a great challenge to the quality control of products. Hydrogels showed controlled local drug release, but it was difficult to accurately control the drug release behavior per unit time. Therefore, simplifying the preparation procedure of hydrogels, improving the accuracy of drug release and release rate will hopefully further improve the application prospect of hydrogels in postoperative radiotherapy.
Author contributions
YX and HL designed the manuscript. YX and CY were responsible for the writing of the abstract and introduction of the manuscript. ML and TG were responsible for the writing of the second part of the manuscript. CC and KY were responsible for the writing of the third part of the manuscript. YX and XT were responsible for writing the fourth part of the manuscript. HX, XT and HL revised the manuscript and gave final approval of the version to be published. All authors contributed to the article and approved the submitted version.
Funding
This work was supported by grants from the National Natural Science Foundation of China (Grant No. 81902535, 81972350), the clinical medical special Program of Science and Technology Project of Jiangsu Province (BE2019734) and the Postgraduate Research & Practice Innovation Program of Jiangsu Province (Grant No. JX22013904).
Conflict of interest
The authors declare that the research was conducted in the absence of any commercial or financial relationships that could be construed as a potential conflict of interest.
Publisher’s note
All claims expressed in this article are solely those of the authors and do not necessarily represent those of their affiliated organizations, or those of the publisher, the editors and the reviewers. Any product that may be evaluated in this article, or claim that may be made by its manufacturer, is not guaranteed or endorsed by the publisher.
References
1. Siegel RL, Miller KD, Fuchs HE, Jemal A. Cancer statistics, 2022. CA Cancer J Clin (2022) 72(1):7–33. doi: 10.3322/caac.21708
2. Verry C, Dufort S, Lemasson B, Grand S, Pietras J, Tropres I, et al. Targeting brain metastases with ultrasmall theranostic nanoparticles, a first-in-human trial from an MRI perspective. Sci Adv (2020) 6(29):eaay5279. doi: 10.1126/sciadv.aay5279
3. Lin SH, Zhang J, Giri U, Stephan C, Sobieski M, Zhong L, et al. A high content clonogenic survival drug screen identifies mek inhibitors as potent radiation sensitizers for KRAS mutant non-small-cell lung cancer. J Thorac Oncol (2014) 9(7):965–73. doi: 10.1097/JTO.0000000000000199
4. Zhang Z, Kuang G, Zong S, Liu S, Xiao H, Chen X, et al. Sandwich-like Fibers/Sponge composite combining chemotherapy and hemostasis for efficient postoperative prevention of tumor recurrence and metastasis. Adv Mater (2018) 30(49):e1803217. doi: 10.1002/adma.201803217
5. Mirrahimi M, Khateri M, Beik J, Ghoreishi FS, Dezfuli AS, Ghaznavi H, et al. Enhancement of chemoradiation by co-incorporation of gold nanoparticles and cisplatin into alginate hydrogel. J BioMed Mater Res B Appl Biomater (2019) 107(8):2658–63. doi: 10.1002/jbm.b.34356
6. De Ruysscher D, Faivre-Finn C, Le Pechoux C, Peeters S, Belderbos J. High-dose re-irradiation following radical radiotherapy for non-small-cell lung cancer. Lancet Oncol (2014) 15(13):e620–e4. doi: 10.1016/S1470-2045(14)70345-6
7. Gong L, Zhang Y, Liu C, Zhang M, Han S. Application of radiosensitizers in cancer radiotherapy. Int J Nanomedicine (2021) 16:1083–102. doi: 10.2147/IJN.S290438
8. Jiang W, Li Q, Zhu Z, Wang Q, Dou J, Zhao Y, et al. Cancer chemoradiotherapy duo: Nano-enabled targeting of DNA lesion formation and DNA damage response. ACS Appl Mater Interfaces (2018) 10(42):35734–44. doi: 10.1021/acsami.8b10901
9. Yu WD, Sun G, Li J, Xu J, Wang X. Mechanisms and therapeutic potentials of cancer immunotherapy in combination with radiotherapy and/or chemotherapy. Cancer Lett (2019) 452:66–70. doi: 10.1016/j.canlet.2019.02.048
10. Hillman GG, Singh-Gupta V, Lonardo F, Hoogstra DJ, Abernathy LM, Yunker CK, et al. Radioprotection of lung tissue by soy isoflavones. J Thorac Oncol (2013) 8(11):1356–64. doi: 10.1097/JTO.0b013e3182a4713e
11. Bouwman P, Jonkers J. The effects of deregulated DNA damage signalling on cancer chemotherapy response and resistance. Nat Rev Cancer (2012) 12(9):587–98. doi: 10.1038/nrc3342
12. Perez-Herrero E, Fernandez-Medarde A. Advanced targeted therapies in cancer: Drug nanocarriers, the future of chemotherapy. Eur J Pharm Biopharm (2015) 93:52–79. doi: 10.1016/j.ejpb.2015.03.018
13. Song G, Cheng L, Chao Y, Yang K, Liu Z. Emerging nanotechnology and advanced materials for cancer radiation therapy. Adv Mater (2017) 29(32). doi: 10.1002/adma.201700996
14. Ramos-Casals M, Brahmer JR, Callahan MK, Flores-Chavez A, Keegan N, Khamashta MA, et al. Immune-related adverse events of checkpoint inhibitors. Nat Rev Dis Primers (2020) 6(1):38. doi: 10.1038/s41572-020-0160-6
15. Postow MA, Sidlow R, Hellmann MD. Immune-related adverse events associated with immune checkpoint blockade. N Engl J Med (2018) 378(2):158–68. doi: 10.1056/NEJMra1703481
16. Yang X, Wang Y, Mao T, Wang Y, Liu R, Yu L, et al. An oxygen-enriched thermosensitive hydrogel for the relief of a hypoxic tumor microenvironment and enhancement of radiotherapy. Biomater Sci (2021) 9(22):7471–82. doi: 10.1039/d1bm01280k
17. Shi Y, Li D, He C, Chen X. Design of an injectable polypeptide hydrogel depot containing the immune checkpoint blocker anti-PD-L1 and doxorubicin to enhance antitumor combination therapy. Macromol Biosci (2021) 21(6):e2100049. doi: 10.1002/mabi.202100049
18. Hu Q, Li H, Archibong E, Chen Q, Ruan H, Ahn S, et al. Inhibition of post-surgery tumour recurrence via a hydrogel releasing CAR-T cells and anti-PDL1-conjugated platelets. Nat BioMed Eng (2021) 5(9):1038–47. doi: 10.1038/s41551-021-00712-1
19. Zhou L, Pi W, Hao M, Li Y, An H, Li Q, et al. An injectable and biodegradable nano-photothermal DNA hydrogel enhances penetration and efficacy of tumor therapy. Biomater Sci (2021) 9(14):4904–21. doi: 10.1039/d1bm00568e
20. Lu Y, Wu C, Yang Y, Chen X, Ge F, Wang J, et al. Inhibition of tumor recurrence and metastasis via a surgical tumor-derived personalized hydrogel vaccine. Biomater Sci (2022) 10(5):1352–63. doi: 10.1039/d1bm01596f
21. Luo FQ, Xu W, Zhang JY, Liu R, Huang YC, Xiao C, et al. An injectable nanocomposite hydrogel improves tumor penetration and cancer treatment efficacy. Acta Biomater (2022) 147:235–44. doi: 10.1016/j.actbio.2022.05.042
22. Wu Y, Yao Y, Zhang J, Gui H, Liu J, Liu J. Tumor-targeted injectable double-network hydrogel for prevention of breast cancer recurrence and wound infection via synergistic photothermal and brachytherapy. Adv Sci (Weinh) (2022) e2200681. doi: 10.1002/advs.202200681
23. Chen Q, Wang C, Zhang X, Chen G, Hu Q, Li H, et al. In situ sprayed bioresponsive immunotherapeutic gel for post-surgical cancer treatment. Nat Nanotechnol (2019) 14(1):89–97. doi: 10.1038/s41565-018-0319-4
24. Correa S, Grosskopf AK, Lopez Hernandez H, Chan D, Yu AC, Stapleton LM, et al. Translational applications of hydrogels. Chem Rev (2021) 121(18):11385–57. doi: 10.1021/acs.chemrev.0c01177
25. Narayanaswamy R, Torchilin VP. Hydrogels and their applications in targeted drug delivery. Molecules (2019) 24(3):603. doi: 10.3390/molecules24030603
26. Xu Z, Tang E, Zhao H. An environmentally sensitive silk Fibroin/Chitosan hydrogel and its drug release behaviors. Polymers (Basel) (2019) 11(12):1980. doi: 10.3390/polym11121980
27. Yu S, He C, Chen X. Injectable hydrogels as unique platforms for local chemotherapeutics-based combination antitumor therapy. Macromol Biosci (2018) 18(12):e1800240. doi: 10.1002/mabi.201800240
28. Kim JK, Kim HJ, Chung JY, Lee JH, Young SB, Kim YH. Natural and synthetic biomaterials for controlled drug delivery. Arch Pharm Res (2014) 37(1):60–8. doi: 10.1007/s12272-013-0280-6
29. Lu L, Yuan S, Wang J, Shen Y, Deng S, Xie L, et al. The formation mechanism of hydrogels. Curr Stem Cell Res Ther (2018) 13(7):490–96. doi: 10.2174/1574888X12666170612102706
30. Bashir S, Hina M, Iqbal J, Rajpar AH, Mujtaba MA, Alghamdi NA, et al. Fundamental concepts of hydrogels: Synthesis, properties, and their applications. Polymers (Basel) (2020) 12(11):2702. doi: 10.3390/polym12112702
31. Ahmad Z, Salman S, Khan SA, Amin A, Rahman ZU, Al-Ghamdi YO, et al. Versatility of hydrogels: From synthetic strategies, classification, and properties to biomedical applications. Gels (2022) 8(3):167. doi: 10.3390/gels8030167
32. Ahmed EM. Hydrogel: Preparation, characterization, and applications: A review. J Adv Res (2015) 6(2):105–21. doi: 10.1016/j.jare.2013.07.006
33. Chyzy A, Tomczykowa M, Plonska-Brzezinska ME. Hydrogels as potential nano-, micro- and macro-scale systems for controlled drug delivery. Materials (Basel) (2020) 13(1):188. doi: 10.3390/ma13010188
34. Tan B, Huang L, Wu Y, Liao J. Advances and trends of hydrogel therapy platform in localized tumor treatment: A review. J BioMed Mater Res A (2021) 109(4):404–25. doi: 10.1002/jbm.a.37062
35. Grijalvo S, Nieto-Diaz M, Maza RM, Eritja R, Diaz DD. Alginate hydrogels as scaffolds and delivery systems to repair the damaged spinal cord. Biotechnol J (2019) 14(12):e1900275. doi: 10.1002/biot.201900275
36. Zhang C, Yang X, Hu W, Han X, Fan L, Tao S. Preparation and characterization of carboxymethyl chitosan/collagen peptide/oxidized konjac composite hydrogel. Int J Biol Macromol (2020) 149:31–40. doi: 10.1016/j.ijbiomac.2020.01.127
37. Tan S, Yamashita A, Gao SJ, Kurisawa M. Hyaluronic acid hydrogels with defined crosslink density for the efficient enrichment of breast cancer stem cells. Acta Biomater (2019) 94:320–29. doi: 10.1016/j.actbio.2019.05.040
38. Livingston MK, Morgan MM, Daly WT, Murphy WL, Johnson BP, Beebe DJ, et al. Evaluation of PEG-based hydrogel influence on estrogen receptor driven responses in MCF7 breast cancer cells. ACS Biomater Sci Eng (2019) 5(11):6089–98. doi: 10.1021/acsbiomaterials.9b00480
39. Liu WC, Wang HY, Lee TH, Chung RJ. Gamma-poly glutamate/gelatin composite hydrogels crosslinked by proanthocyanidins for wound healing. Mater Sci Eng C Mater Biol Appl (2019) 101:630–9. doi: 10.1016/j.msec.2019.04.018
40. Yang Z, Liu J, Lu Y. Doxorubicin and CDCUR inclusion complex coloaded in thermosensitive hydrogel PLGAPEGPLGA localized administration for osteosarcoma. Int J Oncol (2020) 57(2):433–44. doi: 10.3892/ijo.2020.5067
41. Pishavar E, Khosravi F, Naserifar M, Rezvani Ghomi E, Luo H, Zavan B, et al. Multifunctional and self-healable intelligent hydrogels for cancer drug delivery and promoting tissue regeneration. In Vivo. Polymers (Basel) (2021) 13(16):2680. doi: 10.3390/polym13162680
42. Kasinski A, Zielinska-Pisklak M, Oledzka E, Sobczak M. Smart hydrogels - synthetic stimuli-responsive antitumor drug release systems. Int J Nanomedicine (2020) 15:4541–72. doi: 10.2147/IJN.S248987
43. Zhang Y, Huang Y. Rational design of smart hydrogels for biomedical applications. Front Chem (2020) 8:615665. doi: 10.3389/fchem.2020.615665
44. Shriky B, Kelly A, Isreb M, Babenko M, Mahmoudi N, Rogers S, et al. Pluronic F127 thermosensitive injectable smart hydrogels for controlled drug delivery system development. J Colloid Interface Sci (2020) 565:119–30. doi: 10.1016/j.jcis.2019.12.096
45. Shu G, Zhu W, Jiang Y, Li X, Pan J, Zhang X, et al. Persistent luminescence immune hydrogel for photodynamic-immunotherapy of tumors In vivo. Advanced Funct Materials (2021) 31(36). doi: 10.1002/adfm.202104472
46. Andrade F, Roca-Melendres MM, Duran-Lara EF, Rafael D, Schwartz S Jr. Stimuli-responsive hydrogels for cancer treatment: The role of pH, light, ionic strength and magnetic field. Cancers (Basel) (2021) 13(5):1164. doi: 10.3390/cancers13051164
47. Fan DY, Tian Y, Liu ZJ. Injectable hydrogels for localized cancer therapy. Front Chem (2019) 7:675. doi: 10.3389/fchem.2019.00675
48. Naahidi S, Jafari M, Logan M, Wang Y, Yuan Y, Bae H, et al. Biocompatibility of hydrogel-based scaffolds for tissue engineering applications. Biotechnol Adv (2017) 35(5):530–44. doi: 10.1016/j.biotechadv.2017.05.006
49. Yang Z, Han B, Fu D, Liu W. Acute toxicity of high dosage carboxymethyl chitosan and its effect on the blood parameters in rats. J Mater Sci Mater Med (2012) 23(2):457–62. doi: 10.1007/s10856-011-4467-4
50. Zhang L, Zhou J, Hu L, Han X, Zou X, Chen Q, et al. In situ formed fibrin scaffold with cyclophosphamide to synergize with immune checkpoint blockade for inhibition of cancer recurrence after surgery. Advanced Funct Materials (2019) 30(7). doi: 10.1002/adfm.201906922
51. Liu M, Cao Z, Zhang R, Chen Y, Yang X. Injectable supramolecular hydrogel for locoregional immune checkpoint blockade and enhanced cancer chemo-immunotherapy. ACS Appl Mater Interfaces (2021) 13(29):33874–84. doi: 10.1021/acsami.1c08285
52. Ma H, He C, Chen X. Injectable hydrogels as local depots at tumor sites for antitumor immunotherapy and immune-based combination therapy. Macromol Biosci (2021) 21(6):e2100039. doi: 10.1002/mabi.202100039
53. Li J, Mooney DJ. Designing hydrogels for controlled drug delivery. Nat Rev Mater (2016) 1(12):16071. doi: 10.1038/natrevmats.2016.71
54. Schaal JL, Li X, Mastria E, Bhattacharyya J, Zalutsky MR, Chilkoti A, et al. Injectable polypeptide micelles that form radiation crosslinked hydrogels in situ for intratumoral radiotherapy. J Control Release (2016) 228:58–66. doi: 10.1016/j.jconrel.2016.02.040
55. Cunha JAM, Flynn R, Belanger C, Callaghan C, Kim Y, Jia X, et al. Brachytherapy future directions. Semin Radiat Oncol (2020) 30(1):94–106. doi: 10.1016/j.semradonc.2019.09.001
56. Meng Z, Chao Y, Zhou X, Liang C, Liu J, Zhang R, et al. Near-Infrared-Triggered in situ gelation system for repeatedly enhanced photothermal brachytherapy with a single dose. ACS Nano (2018) 12(9):9412–22. doi: 10.1021/acsnano.8b04544
57. Hu Y, Chi C, Wang S, Wang L, Liang P, Liu F, et al. A comparative study of clinical intervention and interventional photothermal therapy for pancreatic cancer. Adv Mater (2017) 29(33). doi: 10.1002/adma.201700448
58. Tanderup K, Menard C, Polgar C, Lindegaard JC, Kirisits C, Potter R. Advancements in brachytherapy. Adv Drug Delivery Rev (2017) 109:15–25. doi: 10.1016/j.addr.2016.09.002
59. Shih YH, Peng CL, Weng MF, Chiang PF, Luo TY, Lin XZ. Evaluation efficacy of rhenium-188-Loaded micro-particles for radiotherapy in a mouse model of hepatocellular carcinoma. Mol Pharm (2019) 16(3):1083–91. doi: 10.1021/acs.molpharmaceut.8b01083
60. Shih YH, Lin XZ, Yeh CH, Peng CL, Shieh MJ, Lin WJ, et al. Preparation and therapeutic evaluation of (188)Re-thermogelling emulsion in rat model of hepatocellular carcinoma. Int J Nanomedicine (2014) 9:4191–201. doi: 10.2147/IJN.S66346
61. Chen M, Wang Z, Suo W, Bao Z, Quan H. Injectable hydrogel for synergetic low dose radiotherapy, chemodynamic therapy and photothermal therapy. Front Bioeng Biotechnol (2021) 9:757428. doi: 10.3389/fbioe.2021.757428
62. Chafe SC, Vizeacoumar FS, Venkateswaran G, Nemirovsky O, Awrey S, Brown WS, et al. Genome-wide synthetic lethal screen unveils novel CAIX-NFS1/xCT axis as a targetable vulnerability in hypoxic solid tumors. Sci Adv (2021) 7(35):eabj0364. doi: 10.1126/sciadv.abj0364
63. Thariat J, Hannoun-Levi JM, Sun Myint A, Vuong T, Gerard JP. Past, present, and future of radiotherapy for the benefit of patients. Nat Rev Clin Oncol (2013) 10(1):52–60. doi: 10.1038/nrclinonc.2012.203
64. Xie Y, Han Y, Zhang X, Ma H, Li L, Yu R, et al. Application of new radiosensitizer based on nano-biotechnology in the treatment of glioma. Front Oncol (2021) 11:633827. doi: 10.3389/fonc.2021.633827
65. Ni K, Lan G, Chan C, Quigley B, Lu K, Aung T, et al. Nanoscale metal-organic frameworks enhance radiotherapy to potentiate checkpoint blockade immunotherapy. Nat Commun (2018) 9(1):2351. doi: 10.1038/s41467-018-04703-w
66. Norouzi M, Nazari B, Miller DW. Injectable hydrogel-based drug delivery systems for local cancer therapy. Drug Discovery Today (2016) 21(11):1835–49. doi: 10.1016/j.drudis.2016.07.006
67. Hill L, Bruns J, Zustiak SP. Hydrogel matrix presence and composition influence drug responses of encapsulated glioblastoma spheroids. Acta Biomater (2021) 132:437–47. doi: 10.1016/j.actbio.2021.05.005
68. Da Ros M, De Gregorio V, Iorio AL, Giunti L, Guidi M, de Martino M, et al. Glioblastoma chemoresistance: The double play by microenvironment and blood-brain barrier. Int J Mol Sci (2018) 19(10):2879. doi: 10.3390/ijms19102879
69. Tang M, Rich JN, Chen S. Biomaterials and 3D bioprinting strategies to model glioblastoma and the blood-brain barrier. Adv Mater (2021) 33(5):e2004776. doi: 10.1002/adma.202004776
70. Liang HT, Lai XS, Wei MF, Lu SH, Wen WF, Kuo SH, et al. Intratumoral injection of thermogelling and sustained-release carboplatin-loaded hydrogel simplifies the administration and remains the synergistic effect with radiotherapy for mice gliomas. Biomaterials (2018) 151:38–52. doi: 10.1016/j.biomaterials.2017.10.015
71. Wardman P. Chemical radiosensitizers for use in radiotherapy. Clin Oncol (R Coll Radiol) (2007) 19(6):397–417. doi: 10.1016/j.clon.2007.03.010
72. Song X, Feng L, Liang C, Yang K, Liu Z. Ultrasound triggered tumor oxygenation with oxygen-shuttle nanoperfluorocarbon to overcome hypoxia-associated resistance in cancer therapies. Nano Lett (2016) 16(10):6145–53. doi: 10.1021/acs.nanolett.6b02365
73. Affolter A, Samosny G, Heimes AS, Schneider J, Weichert W, Stenzinger A, et al. Multikinase inhibitors sorafenib and sunitinib as radiosensitizers in head and neck cancer cell lines. Head Neck. (2017) 39(4):623–32. doi: 10.1002/hed.24557
74. Colin Brooks TS, Bridges K, Mason K, Kuban D, Mathew P, Meyn R. Preclinical evaluation of sunitinib, a multi-tyrosine kinase inhibitor, as a radiosensitizer for human prostate cancer. Radiat Oncol (2012) 7:154. doi: 10.1186/1748-717X-7-154
75. Fu Z, Li H, Xue P, Yu H, Yang S, Tao C, et al. Implantable bioresponsive hydrogel prevents local recurrence of breast cancer by enhancing radiosensitivity. Front Bioeng Biotechnol (2022) 10:881544. doi: 10.3389/fbioe.2022.881544
76. Stupp R, Hegi ME, Mason WP, van den Bent MJ, Taphoorn MJB, Janzer RC, et al. Eff ects of radiotherapy with concomitant and adjuvant temozolomide versus radiotherapy alone on survival in glioblastoma in a randomised phase III study: 5-year analysis of the EORTC-NCIC trial. Lancet Oncol (2009) 10(5):459–66. doi: 10.1016/S1470-2045(09)70025-7
77. Kumar S, Bajaj A. Advances in self-assembled injectable hydrogels for cancer therapy. Biomater Sci (2020) 8(8):2055–73. doi: 10.1039/d0bm00146e
78. Tian R, Chen J, Niu R. The development of low-molecular weight hydrogels for applications in cancer therapy. Nanoscale (2014) 6(7):3474–82. doi: 10.1039/c3nr05414d
79. Trombino S, Servidio C, Curcio F, Cassano R. Strategies for hyaluronic acid-based hydrogel design in drug delivery. Pharmaceutics (2019) 11(8):407. doi: 10.3390/pharmaceutics11080407
80. Tang L, Jiang W, Wu L, Yu X, He Z, Shan W, et al. TPGS2000-DOX prodrug micelles for improving breast cancer therapy. Int J Nanomedicine (2021) 16:7875–90. doi: 10.2147/IJN.S335405
81. Bartlett JJ, Trivedi PC, Pulinilkunnil T. Autophagic dysregulation in doxorubicin cardiomyopathy. J Mol Cell Cardiol (2017) 104:1–8. doi: 10.1016/j.yjmcc.2017.01.007
82. Huang P, Zhang Y, Wang W, Zhou J, Sun Y, Liu J, et al. Co-Delivery of doxorubicin and (131)I by thermosensitive micellar-hydrogel for enhanced in situ synergetic chemoradiotherapy. J Control Release (2015) 220:456–64. doi: 10.1016/j.jconrel.2015.11.007
83. Peng CL, Shih YH, Liang KS, Chiang PF, Yeh CH, Tang IC, et al. Development of in situ forming thermosensitive hydrogel for radiotherapy combined with chemotherapy in a mouse model of hepatocellular carcinoma. Mol Pharm (2013) 10(5):1854–64. doi: 10.1021/mp3006424
84. Ahmed A, Tait SWG. Targeting immunogenic cell death in cancer. Mol Oncol (2020) 14(12):2994–3006. doi: 10.1002/1878-0261.12851
85. Galluzzi L, Vitale I, Warren S, Adjemian S, Agostinis P, Martinez AB, et al. Consensus guidelines for the definition, detection and interpretation of immunogenic cell death. J Immunother Cancer (2020) 8(1):e000337. doi: 10.1136/jitc-2019-000337
86. Zhu M, Yang M, Zhang J, Yin Y, Fan X, Zhang Y, et al. Immunogenic cell death induction by ionizing radiation. Front Immunol (2021) 12:705361. doi: 10.3389/fimmu.2021.705361
87. Guan X, Sun L, Shen Y, Jin F, Bo X, Zhu C, et al. Nanoparticle-enhanced radiotherapy synergizes with PD-L1 blockade to limit post-surgical cancer recurrence and metastasis. Nat Commun (2022) 13(1):2834. doi: 10.1038/s41467-022-30543-w
88. Decraene B, Yang Y, De Smet F, Garg AD, Agostinis P, De Vleeschouwer S. Immunogenic cell death and its therapeutic or prognostic potential in high-grade glioma. Genes Immun (2022) 23(1):1–11. doi: 10.1038/s41435-021-00161-5
89. Kroemer G, Galluzzi L, Kepp O, Zitvogel L. Immunogenic cell death in cancer therapy. Annu Rev Immunol (2013) 31:51–72. doi: 10.1146/annurev-immunol-032712-100008
90. Li Z, Lai X, Fu S, Ren L, Cai H, Zhang H, et al. Immunogenic cell death activates the tumor immune microenvironment to boost the immunotherapy efficiency. Adv Sci (Weinh) (2022) 9(22):e2201734. doi: 10.1002/advs.202201734
91. Vanpouille-Box C, Formenti SC, Demaria S. Toward precision radiotherapy for use with immune checkpoint blockers. Clin Cancer Res (2018) 24(2):259–65. doi: 10.1158/1078-0432.CCR-16-0037
92. Sun S, Gu W, Wu H, Zhao Q, Qian S, Xiao H, et al. Immunostimulant In situ hydrogel improves synergetic radioimmunotherapy of malignant glioblastoma relapse post-resection. Advanced Funct Materials (2022) 2205038. doi: 10.1002/adfm.202205038
93. den Brok MH, Sutmuller RP, Nierkens S, Bennink EJ, Toonen LW, Figdor CG, et al. Synergy between in situ cryoablation and TLR9 stimulation results in a highly effective in vivo dendritic cell vaccine. Cancer Res (2006) 66(14):7285–92. doi: 10.1158/0008-5472.CAN-06-0206
94. Redondo P, del Olmo J, Lopez-Diaz de Cerio A, Inoges S, Marquina M, Melero I, et al. Imiquimod enhances the systemic immunity attained by local cryosurgery destruction of melanoma lesions. J Invest Dermatol (2007) 127(7):1673–80. doi: 10.1038/sj.jid.5700777
95. Chen Q, Chen J, Yang Z, Xu J, Xu L, Liang C, et al. Nanoparticle-enhanced radiotherapy to trigger robust cancer immunotherapy. Adv Mater (2019) 31(10):e1802228. doi: 10.1002/adma.201802228
96. Yazdani M, Gholizadeh Z, Nikpoor AR, Mohamadian Roshan N, Jaafari MR, Badiee A. Ex vivo dendritic cell-based (DC) vaccine pulsed with a low dose of liposomal antigen and CpG-ODN improved PD-1 blockade immunotherapy. Sci Rep (2021) 11(1):14661. doi: 10.1038/s41598-021-94250-0
97. Liu X, Feng Z, Wang C, Su Q, Song H, Zhang C, et al. Co-Localized delivery of nanomedicine and nanovaccine augments the postoperative cancer immunotherapy by amplifying T-cell responses. Biomaterials (2020) 230:119649. doi: 10.1016/j.biomaterials.2019.119649
Keywords: hydrogel, postoperative radiotherapy, radiation sensitization, local drug delivery, tumor recurrence
Citation: Xie Y, Liu M, Cai C, Ye C, Guo T, Yang K, Xiao H, Tang X and Liu H (2023) Recent progress of hydrogel-based local drug delivery systems for postoperative radiotherapy. Front. Oncol. 13:1027254. doi: 10.3389/fonc.2023.1027254
Received: 24 August 2022; Accepted: 02 February 2023;
Published: 13 February 2023.
Edited by:
Timothy James Kinsella, Brown University, United StatesReviewed by:
Amirhosein Kefayat, Isfahan University of Medical Sciences, IranDaoming Zhu, Southern Medical University, China
Copyright © 2023 Xie, Liu, Cai, Ye, Guo, Yang, Xiao, Tang and Liu. This is an open-access article distributed under the terms of the Creative Commons Attribution License (CC BY). The use, distribution or reproduction in other forums is permitted, provided the original author(s) and the copyright owner(s) are credited and that the original publication in this journal is cited, in accordance with accepted academic practice. No use, distribution or reproduction is permitted which does not comply with these terms.
*Correspondence: Hongyi Liu, hyliu18@njmu.edu.cn; Xianglong Tang, xltang0326@163.com; Hong Xiao, xiaohong63xx@163.com
†These authors have contributed equally to this work