Endogenous and Exogenous Stem/Progenitor Cells in the Lung and Their Role in the Pathogenesis and Treatment of Pediatric Lung Disease
- 1Program of Physiology & Experimental Medicine, The Hospital for Sick Children, Toronto, ON, Canada
- 2Department of Physiology, University of Toronto, Toronto, ON, Canada
- 3Department of Pediatrics, University of California San Diego, San Diego, CA, USA
The lung is a complex organ with a vast surface area whose main function is to release cellular waste to be exhaled and to replenish the supply of oxygen to the tissues of the body. The conduction of air from the external environment is not without risks, and the lung contains many specialized epithelial cell subtypes that are protecting the lung from foreign material and injury. Specialized cell subtypes are produced during lung development in the fetus as well as postnatally and injury to them due to genetic disease, premature birth, or postnatal environmental injury may lead to devastating disease. Chronic diseases, such as bronchopulmonary dysplasia, cystic fibrosis, and pulmonary arterial hypertension, contribute significantly to morbidity and mortality worldwide, yet successful interventions are often limited. Stem/progenitor cells have emerged as a potentially new preventative or therapeutic option. They are generally defined by the ability to undergo self-renewal and give rise to more differentiated cells. They are important in the early development of embryonic structures and organ differentiation in utero. Postnatally, they function in continued growth, maintenance, and regeneration. Clinically, the immunomodulatory properties of some classes of stem/progenitor cells avoid the major obstacle of immunological rejection seen in organ transplantation and other cell therapies. This review highlights some known human progenitor/stem cells and the most recent advances in stem cell therapies both in vivo and in vitro to prevent and treat pediatric lung disease.
Lung Development
The lung develops through five stages in the human (Figure 1), and a multitude of genes and transcription factors are involved in the mediation of each phase. In the embryonic phase, endoderm, specifically anterior endoderm, gives rise to the lung, which begins with the formation of a groove in the ventral lower pharynx, which then buds to form the true lung primordium. (1). The interaction between the epithelium and mesenchyme is critical in this early stage (2). Septation of the tracheoesophageal tube separates the respiratory tree from the gastrointestinal tract. Transcription factors are important in this process and are activated early in lung progenitor cells (3).
In the pseudoglandular stage, branching morphogenesis of the epithelium into the surrounding mesenchyme dominates and forms the entire air-conducting bronchial tree up to the terminal bronchioli. During this period, the developing epithelium begins to secrete fluid into the budding airways, which is important for the further growth of the primordial lung (4). Branching occurs within a preprogrammed set of rules and is governed by the balance of attraction and inhibition of FGF10 from the mesenchyme along with multiple other regulators (2). Embryonic stem/progenitor cell functions have been uncovered in the pseudoglandular stage, such as a multipotent progenitor population expressing the inhibitor of differentiation 2 (Id2) in the mouse (5). This transcriptional regulator is very strongly expressed in distal epithelial tips of the branching sites that are thought to give rise to both proximal conducting airway and distal alveolar epithelial cell types. Another lung progenitor cell, this time from the mesoderm, namely, cardiopulmonary mesoderm progenitor (CPP) cells, arises from the cardiopulmonary mesenchyme and gives rise to cardiomyocytes, endocardium, pulmonary vascular, and airway smooth muscle cells (6). Interestingly, CPP cells do not rely on parallel epithelial lung development for their growth as previously thought.
The canalicular stage comprises the branching of the respiratory portion of the lung from the terminal bronchioli. These air spaces form an acinus comprising respiratory bronchioles and the alveolar ducts. In this stage, the capillaries invade the mesenchyme and begin to surround the acini. Type I and II pneumocytes appear, proliferate, and surfactant begins to be secreted. It is late in this stage when a preterm newborn has the capacity to survive with assisted ventilation in the extra uterine environment.
At approximately 25 weeks, whole clusters of sacs extend from the terminal bronchiole, defining the saccular stage. These saccules are coated with type I and II pneumocytes, and they are divided by primary septa, which are thick and contain two layers of capillaries. The interstitial space or matrix becomes rich with a variety of cell types as well as collagen and elastic fibers. Bipotential alveolar progenitors in the mouse develop into ATII and alveolar type I (ATI) cells but the signals and timing are still unknown as whether there is an equivalent progenitor in the human (7).
The final stage beginning in the late third trimester is defined by the alveolarization of the lung. Secondary septa begin to form, and the basement membrane of the capillary endothelium and the saccular epithelium merge to form a thin barrier. A large number of small protrusions form along the primary septa, becoming larger and subdivide the sacculi into smaller subunits, the alveoli, which are delimited by secondary septa (8). This phenomenon continues well into extra uterine life.
Vascular development of the lung consists of vasculogenesis (de novo formation of the vascular plexus from mesodermal progenitor cells) and angiogenesis (sprouting of endothelial cells from pre-existing vessels to form new tubes) (9). Endothelial cells of the newly formed tubes recruit pericytes, which wrap around endothelial tubes and induce stabilization and maturation (10). Tight regulation of this interaction is required for normal vascular development, and disruption of this process may lead postnatal lung disease.
Finally, an integral part of the developing lung is the extracellular matrix (ECM). This is a complex network of components that have a structural, biochemical, and mechanical function. In lung development, the ECM serves as a scaffold that directs cell migration and differentiation, becoming more complex with development (11). Any alterations in the structure of the ECM whether through premature developmental arrest or injury will greatly alter the function of the lung.
This complicated process of lung development has been well studied in animal models, and many of the genetic and molecular factors of the major stages, as well as some stem cell populations have been defined with respect to human lung development, although much is still unknown (12).
Lung Disease Resulting from Disrupted Lung Development
Bronchopulmonary dysplasia is the sequela of preterm birth. The hallmark of this disease is alveolar growth arrest and abnormal lung vascular growth (13). This alveolar growth arrest is both a product of the disruption of secondary septation formation as well as a matrix that has not been fully formed. The formation of the pulmonary capillaries destined to appose the alveoli is also stunted. The etiology is multifactorial including infection, hyperoxia, and volutrauma superimposed on an immature lung with decreased defenses (14). At a cellular level, these abnormalities are presumed to be due to defective elastogenesis and ECM remodeling (15), altered alveolar epithelial–mesenchymal interactions, and impaired development of lung microvasculature (16). These changes may have an effect on the intrinsic stem/progenitor cell populations, making it difficult to repair the fragile young lung.
Idiopathic pulmonary arterial hypertension (IPAH) is a rare disorder of unknown etiology clinically defined by raised pulmonary artery pressures involving pathological changes in precapillary pulmonary artery. Although there has been significant progress to improve the morbidity and mortality of this disease, it remains a serious condition, which is extremely challenging to manage (17).
These diseases have been a focus of human stem cell treatment and will be highlighted below.
Stem/Progenitor Cells in Homeostasis/Injury Repair
Although stem cells/progenitors are important in the development of the lung, they also play an important role in lung regeneration and repair. Human tissue regeneration via native or recruited stem cell populations involves several mechanisms, which are regionally distinct and dependent on the type of injury (18). Constantly renewing organs, such as the hematopoietic system, have stem cells that are unspecialized, have a low rate of division, and are located in specialized “niches” (19). The lung is an organ that is slow to regenerate but can initiate rapid repair after injury. It is postulated that there are niches in the lung that house quiescent progenitor cells that have the potential to self-renew and generate progeny to regenerate the lung epithelium specific to that location (Figure 2) (20). In the proximal airway, located in the gland duct and on the surface in the intercartilaginous zone, are basal cells, which are multipotent stem cells in the tracheobronchial region that can both self-renew and give rise to ciliated and secretory lineages during postnatal growth, homeostasis, and repair following damage to the epithelium (21). Non-ciliated secretory Clara cells (or Club cells) and variant Clara cells, which are located at the bronchiolar–alveolar duct junction and associated with the neuroepithelial body, give rise to ciliated and secretory cells (22, 23). A small number of cells in the bronchioalveolar duct junction (BADJ) co-express SCGB1A1 (a Clara cell marker) and Surfactant protein C (SFTPC), a protein that is expressed by ATII cells in the alveoli. It has been proposed that these “dual positive” cells are bronchioalveolar stem cells or BASCs (24), which apparently give rise to bronchiolar and alveolar cell types in culture. In the peribronchial region, basal cells appear during repair and proliferate in the distal lung, giving rise to functional alveoli (25).
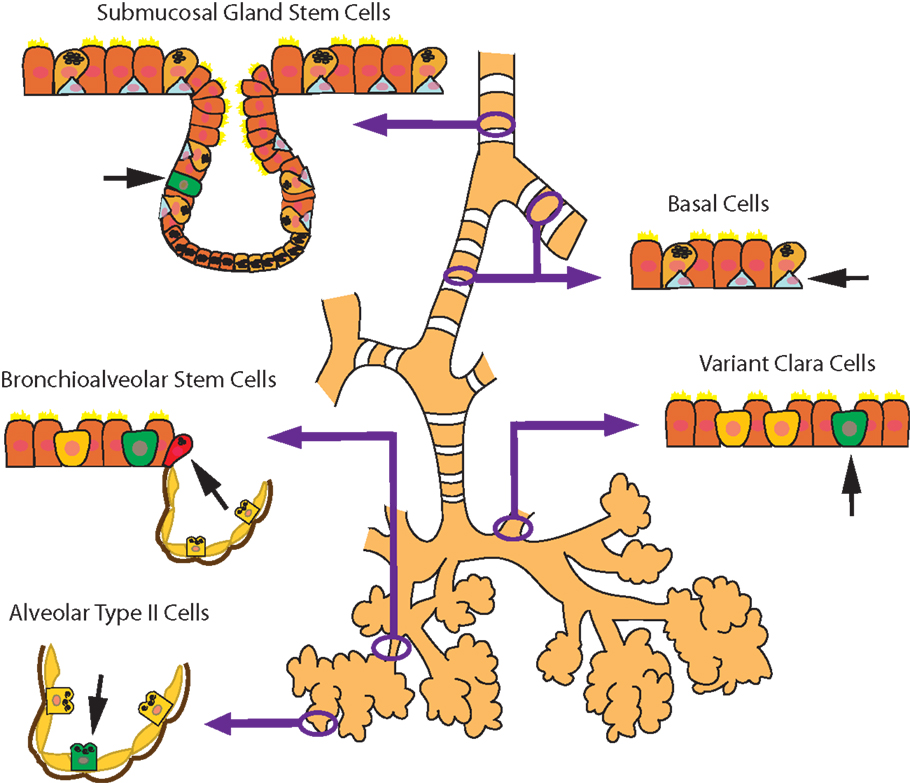
Figure 2. Schematic diagram of the microenvironmental niches that may contain lung stem/progenitor cells.
Finally, ATII cells in the alveoli give rise to ATI cells although the rate of conversion depends on whether the cells are in steady state (slow rate) or during repair after injury (fast rate) (18, 26). Although more is known of the stem cell niches and cell populations in the mouse lung, there have been some advances in the human lung as well. Investigators have discovered c-Kit+ cells in human lungs that are undifferentiated, self-renewing, clonigenic, and multipotent in vitro. C-kit is a transmembrane tyrosine kinase receptor for stem cell factor (SCF) and has a diverse range of biological functions including cell proliferation, differentiation, migration, and survival (27). Injection of the c-kit+ cells into a mouse model of focal lung injury regenerates lung components as diverse as bronchioles, alveoli, smooth muscle, and pulmonary vessels (28). This is the first evidence of a “lung stem cell” that can regenerate both endodermal and mesenchymal cell lineages, although these findings have been disputed (29). It has been challenging to define the properties of lung stem/progenitor cells due to the lungs complexity and diversity of cell types as well as the slow turnover of the respiratory epithelium. The regenerative capacity of many of these cells is also not solely determined by their intrinsic potential. The microenvironment of their specific niche, including the ECM, accessory cells, and many signaling factors, are also important regulators (30, 31) and warrant further investigation.
Various stem/progenitor cells that function in lung repair reside in other areas of the body and are recruited in times of injury and inflammation. Cells from the bone marrow, blood, adipose tissue, placenta, and umbilical cord have been shown to structurally engraft in the airway (32, 33) as well as the pulmonary vasculature. Another potential mechanism is that bone marrow-derived cells are recruited to the lung upon injury and exert their regenerative effects via a paracrine function (34, 35). One population is the mesenchymal stromal cells (MSCs). These cells are multipotent and have a diverse but restricted ability to differentiate to a specific lineage. They appear to function in a paracrine manner with minimal engraftment, interact with the innate and adaptive immune systems (36, 37), and aid in lung repair and regeneration via secretion of cytokines and growth factors to restore alveolar epithelial and endothelial permeability (38–41). Chang et al. (42) used a hyperoxia rat model of BPD to determine the best administration route of human cord blood-derived MSCs. They exposed rat pups to 95% oxygen from birth and at day 5 delivered the MSCs either intratracheally or intraperitoneally. They showed that the intratracheally transplanted MSCs were better in preventing alveolar growth arrest and alleviating fibrotic changes in the lungs of oxygen challenged rat pups than the intraperitoneally administered cells. These finding have been corroborated by Zhang et al. (43) using a similar hyperoxic BPD model. They treated the rat pups with bone marrow-derived MSCs 7 days after the hyperoxic insult and saw a decrease in alveolar apoptosis. They concluded that the MSC’s protective function was due to stimulation of mediators that participated in tissue repair. Thebaud’s group (34), using the same BPD rat model, showed that human umbilical cord-derived MSCs partially prevented and rescued lung function and structure, although cell engraftment was low. Postulating a paracrine effect of MSCs, they derived MSC conditioned media, which after infusion into the hyperoxia exposed animal, showed similar therapeutic benefits as the cells themselves. They also looked at the lungs of these rats 6 months after the intervention and showed a persistent improvement in lung capacity and lung structure. Kourembanas’ group evaluated the pulmonary abnormalities in BPD. They found that a single dose of MSC conditioned media in hyperoxia-exposed newborn mice reversed the hyperoxia-induced parenchymal fibrosis, partially reversed alveolar injury, normalized lung function defined as airway resistance and dynamic lung compliance, fully reversed the moderate right ventricular hypertrophy, and attenuated peripheral pulmonary artery muscularization associated with hyperoxia-induced BPD (44). There are many investigators studying the biology of MSCs on lung injury, and for a full review of animal models of BPD and therapeutic use of MSCs please, see the review by O’Reilly et al. (45).
Endothelial progenitor cells (EPCs) come in two flavors: “early” EPCs, which have hematopoietic surface markers, secrete pro-angiogenic factors, and have limited differentiation ability; “late” EPCs [or endothelial colony-forming cells (ECFCs)] that have no hematopoietic surface markers, do not secrete pro-angiogenic factors, make endothelioid tubes in vitro, grow late in culture, and are important in replacing damaged endothelium (46). These cells exert their therapeutic effects via direct differentiation and engraftment into the vasculature of the lung and secretion of factors that mobilize endothelial and progenitor cells. Kung et al. (47) seeded adult peripheral blood ECFCs onto acellular human skin and transplanted the celluarized skin scaffolds into immunocompromised mice. They formed functional human endothelial cell vessels, which had anastomosed with the circulation of the mouse. Shepherd et al. (48) compared umbilical cord blood-derived ECFCs, adult peripheral blood-derived ECFCs, or human umbilical vein endothelial cells (HUVECs) in a similar model. The umbilical cord blood-derived ECFCs exhibited a greater human vessel density than the other ECFCs. These studies show that ECFCs represent a promising source for vascular regeneration.
Amniotic fluid stem cells (AFSCs) have been used since the late 90s in animal models to study their function in a variety of organ systems. Human AFSCs are fetal-associated cells that are multipotent and can differentiate into all germ layers and can be easily and ethically obtained from amniocentesis specimens. Intratracheal injection of human AFSCs into a rabbit model of congenital diaphragmatic hernia showed improved lung density and function (49). Carraro et al. (33) injected human AFSCs locally to the murine distal lung and saw integration into the epithelium and expression of the early lung marker NKX2-1. After oxidative injury, the injected cells expressed both NKX2-1 and SFPTC (ATII cell marker). These same investigators deduced that the hAFSCs attached within the ATII wound and expedited repair through the secretion of cytokines. The damaged milieu also allowed healing through the differentiation of these cells into distal alveolar epithelium (50). Although no clinical trials using hAFSCs in lung disease have been done, they show promising future use.
Human amnion epithelial cells (hAECs) are found in the lining of the placenta and are able to develop into all the germ layers, possess regenerative and anti-inflammatory properties, and display low immunogenicity. Many animal models of lung injury have been used to study the effects of these cells. Hodges et al. (51) looked at three groups of lambs, and evaluated their lung injury after being ventilated in utero alone or with intravenous and intratracheal administration of hAECs at 110 days of gestation. The lambs were replaced into the womb, and after a week, removed and evaluated. The investigators found that the stem cells mitigated ventilation-induced lung injury, engrafted onto the lung and differentiated into ATI and ATII cells. Vosodoganes et al. (52) showed in an intrauterine LPS-induced model of lung inflammation in fetal sheep that hAECs significantly attenuated the fetal pulmonary inflammatory response after being administered intravenously, but that they did not improve lung structure. Other investigators studying the anti-inflammatory effect of hAECs on bleomycin-treated mice confirmed that hAEC’s attenuated the inflammatory response and improved lung function (53, 54). Murphy et al. (55) found that hAECs formed three-dimensional structures, expressed the CFTR gene and protein after culture in Small Airway Growth Medium (SAGM) and possessed functional iodide/chloride [I(−)/Cl(−)] ion channels. This showed that hAECs may be a new source for the development of a cellular therapy for cystic fibrosis.
These cells have not yet been used in clinical trials due to the lack of large scale production of clearly defined amnion epithelial cells.
Clinical Application of Stem/Progenitor Cells in Pediatric Lung Disease
Animal models have been important in elucidating many of the potential repair mechanisms of a variety of stem/progenitor cells but, even without knowing all underlying mechanisms or risks, their clinical application for use in BPD and other pediatric lung diseases has exploded.
Mesenchymal stromal cells can be obtained from multiple tissues of the body in adults as well as children and large supplies are known to come from the products of pregnancy including cord blood, placenta, and amnion. Cord blood MSCs are generating a lot of interest since they can be obtained without ethical constrictions, can be easily harvested, and are superior in their healing capabilities than adult bone marrow cells (56). Their widespread use in clinical trials has also been due to their immunomodulatory behavior. MSCs express low levels of major histocompatibility complex (MHC) class I molecules and no MHC class II molecules, allowing them to be poorly immunogenic. They also do not express costimulatory molecules involved in the activation of T cell for transplant rejection (57). All these characteristics have been delineated in both adult and fetal MSCs and make them strong candidates for cellular therapies. Clinically, MSCs have been used successfully in many disease processes, but in the lung, a phase II trial for moderate to severe COPD (58) showed no therapeutic effect, although it was not powered for clinical efficacy. In patients that received the MSCs, there were no changes from baseline except a decrease in CRP, a marker of inflammation, and there were no harmful side effects that differed between the two groups. In preterm infants with BPD, a phase I trial in Korea evaluated the safety and the efficacy of human umbilical cord blood-derived-mesenchymal stromal cell (hUCB) treatment in premature infants with BPD. Intratracheal MSC transplantation was performed in nine preterm infants, with a mean gestational age of 25 weeks. BPD severity was lower in the transplant recipients, and rates of other adverse outcomes did not differ between the comparison group and transplant recipients. They concluded that intratracheal transplantation of allogeneic hUCB-derived MSCs in preterm infants was safe and feasible, and warranted a larger and controlled phase II study (59). Other pulmonary diseases in which these cells are currently being investigated clinically are asthma, idiopathic pulmonary fibrosis (IPF), and bronchiolitis obliterans syndrome (BOS) (60). The barriers of clinically using MSCs include not knowing the safest and efficacious route, optimal dose, and the incomplete understanding of mechanism of action. These cells are also heterogeneous, and a well-defined clinically validated product is not available.
Angiogenesis is crucial for normal postnatal alveolar development (61). EPCs and ECFCs are circulating peripheral cells that travel to ischemic sites and augment angiogenesis via paracrine effects (62, 63). Experimentally, a depletion of EPCs in the blood, bone marrow, and lungs of neonatal mice was detected after exposing mice to hyperoxia, but there was a twofold increase of EPCs in the lungs of adult mice exposed to hyperoxia, suggesting the migration of these cells to the lung for repair (64). This was not consistent in human babies (65). Thirty-six preterm neonates at risk of lung injury were evaluated for serum levels of EPCs, and it was found that levels of EPCs did not affect the risk of developing BPD. Follow-up blood draws at 36 weeks postmenstrual weeks showed that the levels of the EPCs were preserved after delivery. Another group of investigators studied ECFCs in the cord blood of 98 preterm babies for the proportion of circulating cells at birth and up to a week after, using flow cytometry (63). They found that ECFCs in cord blood were lower in infants who later developed BPD. This was felt to contribute to the vascular immaturity seen in this disease.
Human trials have been performed to examine whether exogenously transplanted EPCs to patients with vascular lung disease are beneficial. Zhu et al. (66) used autologous EPC transplantation in children with IPAH. Thirteen children received IV autologous EPCs and after 12 weeks, showed improvements in exercise capacity and pulmonary hemodynamics. Although this was just a pilot study, the clinical use of EPCs in childhood IPAH seems safe and feasible. A Canadian phase I study using EPCs transfected with endothelial nitric oxide synthase in seven patients has recently been completed (NCT00469027), and the final results are pending. Clinical use is hampered by the lack of large-scale production of clearly defined EPCs and ECFCs, and their method of action is even less understood than MSCs.
Table 1 summarizes the clinical applications of the various cell types in pediatric lung disease.
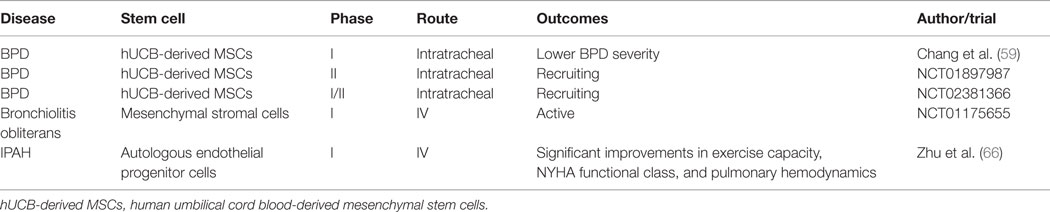
Table 1. Pediatric clinical trials using a variety of stem/progenitor cells for the treatment of pulmonary diseases.
In Vitro Stem Cell-Derived Lung Cells
Human embryonic stem cells (hESC) and induced pluripotent stem cells (hiPSCs) are pluripotent cells that can be differentiated into any tissue in the body. They are naturally derived from human embryos (hESC) (67) or derived from differentiated tissues such as skin or blood after transfection with a specific set of transcription factors (hiPSCs) (68). Both cell types are easy to maintain in culture and can be produced in large quantities for clinical application. By recapitulating lung development in culture, these cells can be coaxed into differentiating into the vast array of epithelia subtypes although lung generation from stem cells has lagged behind other tissue types (12). There are many protocols for differentiating stem cells into lung cells, but the induction of definitive endoderm from the stem cell is mostly achieved with a high concentration of activin A, a known signaling molecule in early lung development. Anterior foregut endoderm is then derived using a combination of small molecules and cytokines (69). These cells are then exposed to a variety of cytokines in order to reach a lung progenitor phenotype, expressing the transcription factor NKX2-1, the first transcription factor signaling the appearance of lung progenitor cells (70). Longmire et al. (71) used Nkx2-1–GFP reporter mouse ESCs to sort out the Nkx2-1-positive cells, and after treating them in culture, there was expression of both proximal and distal lung cell markers. Multiple researchers have then exposed human lung progenitor cells to a cocktail of exogenous signals in a variety of culture conditions and were able to show markers expressing basal, ciliated, and mucus cells (72), distal ATI and ATII (70), mature ciliated epithelium using airway liquid interface (ALI) culture (73), and three-dimensional spheroids expressing multiple lung subtype markers, both proximal and distal (74). Ghaedi et al. (75) went a step further and seeded SPC+ ATII cells derived from hiPSCs onto a decelluralized lung matrix onto which they adhered and proliferated. The Otts lab recently reported the regeneration of functional pulmonary vasculature by repopulating the vascular compartment of decellularized rat and human lung scaffolds with human cells (76).
The clinical application of these stem cell-derived lung cells into injured lung tissue has many possibilities, including correcting the genetic mutations in patient specific cells, and eventually replenishing the injured lung with the corrected cells, without immunologic rejection, although this still has a long way to go. Safety, mode of delivery, efficiency, large scale production, and purity have to first be evaluated in animals successfully. One strategy that has been evaluated in the mouse and human respiratory system is decellularization using the intact acellular matrix of the lung as a base for fresh lung progenitors. Recently, Shojaie et al. (77) evaluated the role of the lung ECM in differentiating stem cell-derived definitive endoderm into mature airway epithelia. Clinically, a Swedish group decellularized an adult human donor trachea, which was then colonized by epithelial cells and mesenchymal stem cell-derived chondrocytes. This graft was then used to replace the recipient’s left main bronchus. At the 5-year follow-up, the tissue-engineered trachea was patent, well vascularized, completely recellularized with respiratory epithelium, and had normal ciliary function and mucus clearance. No stem cell-related teratoma formed, and no anti-donor antibodies developed (78). Another group, led by Di Coppi, treated a pediatric patient with congenital tracheal stenosis with a decellularised cadaveric donor tracheal scaffold and seeded it with bone marrow mesenchymal stem cells. The graft revascularized within 1 week after surgery, the patient had a normal chest CT scan and ventilation–perfusion scan after 18 months after surgery, and at 2-year follow-up, he had a functional airway (79).
Once the derivation of mature and functional lung epithelial cells that mirror their in vivo counterparts is possible and the best route and both short- and long-term safety are guaranteed, these patient-specific derived lung cells can potentially be used clinically to populate severely injured lung tissue in various pediatric diseases, without the immunologic and ethical burden of lung transplantation.
Conclusion
In conclusion, although the field of stem cell lung biology is expanding rapidly and animal models of various pediatric lung diseases are providing insight to other molecular mechanisms of lung injury, these diseases remain a chronic burden on the child despite symptomatic therapy. Evidence has shown that damage to endogenous stem cells may be contributing to the risk and etiology of the disease, and exogenously administered stem cells may offer new possibilities in preventing or curing these diseases. Continued work in stem cell biology, lung development, and the underlying disruption of normal development will add to our knowledge, and when applied clinically, will provide us with successful protocols to finally prevent or treat these diseases.
Author Contributions
Conception and design, writing, review, and/or revision of the manuscript: SL and MP. Study supervision: MP.
Conflict of Interest Statement
The authors declare that the research was conducted in the absence of any commercial or financial relationships that could be construed as a potential conflict of interest.
Funding
This work was supported by an operating grant (FND-143309) from Canadian Institutes of Health Research.
References
1. Cardoso WV, Williams MC. Basic mechanisms of lung development: Eighth Woods Hole Conference on lung cell biology 2000. Am J Respir Cell Mol Biol (2001) 25(2):137–40. doi: 10.1165/ajrcmb.25.2.4537
2. Cardoso WV. Molecular regulation of lung development. Annu Rev Physiol (2001) 63:471–94. doi:10.1146/annurev.physiol.63.1.471
3. Herriges M, Morrisey EE. Lung development: orchestrating the generation and regeneration of a complex organ. Development (2014) 141(3):502–13. doi:10.1242/dev.098186
4. Hooper SB, Harding R. Fetal lung liquid: a major determinant of the growth and functional development of the fetal lung. Clin Exp Pharmacol Physiol (1995) 22(4):235–47. doi:10.1111/j.1440-1681.1995.tb01988.x
5. Rawlins EL, Clark CP, Xue Y, Hogan BL. The Id2+ distal tip lung epithelium contains individual multipotent embryonic progenitor cells. Development (2009) 136(22):3741–5. doi:10.1242/dev.037317
6. Peng T, Tian Y, Boogerd CJ, Lu MM, Kadzik RS, Stewart KM, et al. Coordination of heart and lung co-development by a multipotent cardiopulmonary progenitor. Nature (2013) 500(7464):589–92. doi:10.1038/nature12358
7. Desai TJ, Brownfield DG, Krasnow MA. Alveolar progenitor and stem cells in lung development, renewal and cancer. Nature (2014) 507(7491):190–4. doi:10.1038/nature12930
8. Wert SE. Normal and abnormal structural development of the lung. In: Polin RA, Fox WW, Abman SH, editors. Fetal and Neonatal Physiology. Philadelphia, PA: Saunders (2004). p. 783–801.
10. Carmeliet P. Angiogenesis in life, disease and medicine. Nature (2005) 438(7070):932–6. doi:10.1038/nature04478
11. Ozbek S, Balasubramanian PG, Chiquet-Ehrismann R, Tucker RP, Adams JC. The evolution of extracellular matrix. Mol Biol Cell (2010) 21(24):4300–5. doi:10.1091/mbc.E10-03-0251
12. Morrisey EE, Hogan BL. Preparing for the first breath: genetic and cellular mechanisms in lung development. Dev Cell (2010) 18(1):8–23. doi:10.1016/j.devcel.2009.12.010
13. Alphonse RS, Rajabali S, Thebaud B. Lung injury in preterm neonates: the role and therapeutic potential of stem cells. Antioxid Redox Signal (2012) 17(7):1013–40. doi:10.1089/ars.2011.4267
14. Baraldi E, Filippone M. Chronic lung disease after premature birth. N Engl J Med (2007) 357(19):1946–55. doi:10.1056/NEJMra067279
15. Bourbon J, Boucherat O, Chailley-Heu B, Delacourt C. Control mechanisms of lung alveolar development and their disorders in bronchopulmonary dysplasia. Pediatr Res (2005) 57(5 Pt 2):38R–46R. doi:10.1203/01.PDR.0000159630.35883.BE
16. Bhatt AJ, Pryhuber GS, Huyck H, Watkins RH, Metlay LA, Maniscalco WM. Disrupted pulmonary vasculature and decreased vascular endothelial growth factor, Flt-1, and TIE-2 in human infants dying with bronchopulmonary dysplasia. Am J Respir Crit Care Med (2001) 164(10 Pt 1):1971–80. doi:10.1164/ajrccm.164.10.2101140
17. Rosenzweig EB, Barst RJ. Idiopathic pulmonary arterial hypertension in children. Curr Opin Pediatr (2005) 17(3):372–80. doi:10.1097/01.mop.0000163356.51027.c1
18. Chapman HA, Li X, Alexander JP, Brumwell A, Lorizio W, Tan K, et al. Integrin alpha6beta4 identifies an adult distal lung epithelial population with regenerative potential in mice. J Clin Invest (2011) 121(7):2855–62. doi:10.1172/JCI59981
19. Fuchs E, Tumbar T, Guasch G. Socializing with the neighbors: stem cells and their niche. Cell (2004) 116(6):769–78. doi:10.1016/S0092-8674(04)00255-7
20. Stripp BR. Hierarchical organization of lung progenitor cells: is there an adult lung tissue stem cell? Proc Am Thorac Soc (2008) 5(6):695–8. doi:10.1513/pats.200801-011AW
21. Rock JR, Randell SH, Hogan BL. Airway basal stem cells: a perspective on their roles in epithelial homeostasis and remodeling. Dis Model Mech (2010) 3(9–10):545–56. doi:10.1242/dmm.006031
22. Rawlins EL, Okubo T, Xue Y, Brass DM, Auten RL, Hasegawa H, et al. The role of Scgb1a1+ Clara cells in the long-term maintenance and repair of lung airway, but not alveolar, epithelium. Cell Stem Cell (2009) 4(6):525–34. doi:10.1016/j.stem.2009.04.002
23. Hong KU, Reynolds SD, Giangreco A, Hurley CM, Stripp BR. Clara cell secretory protein-expressing cells of the airway neuroepithelial body microenvironment include a label-retaining subset and are critical for epithelial renewal after progenitor cell depletion. Am J Respir Cell Mol Biol (2001) 24(6):671–81. doi:10.1165/ajrcmb.24.6.4498
24. Kim CF, Jackson EL, Woolfenden AE, Lawrence S, Babar I, Vogel S, et al. Identification of bronchioalveolar stem cells in normal lung and lung cancer. Cell (2005) 121(6):823–35. doi:10.1016/j.cell.2005.03.032
25. Kumar PA, Hu Y, Yamamoto Y, Hoe NB, Wei TS, Mu D, et al. Distal airway stem cells yield alveoli in vitro and during lung regeneration following H1N1 influenza infection. Cell (2011) 147(3):525–38. doi:10.1016/j.cell.2011.10.001
26. Barkauskas CE, Cronce MJ, Rackley CR, Bowie EJ, Keene DR, Stripp BR, et al. Type 2 alveolar cells are stem cells in adult lung. J Clin Invest (2013) 123(7):3025–36. doi:10.1172/JCI68782
27. Suzuki T, Suzuki S, Fujino N, Ota C, Yamada M, Suzuki T, et al. c-Kit immunoexpression delineates a putative endothelial progenitor cell population in developing human lungs. Am J Physiol Lung Cell Mol Physiol (2014) 306(9):L855–65. doi:10.1152/ajplung.00211.2013
28. Kajstura J, Rota M, Hall SR, Hosoda T, D’Amario D, Sanada F, et al. Evidence for human lung stem cells. N Engl J Med (2011) 364(19):1795–806. doi:10.1056/NEJMoa1101324
29. Stripp B. Lung stem cells: looking beyond the hype. Nat Med (2011) 17(7):788–9. doi:10.1038/nm0711-788
30. Watkins DN, Berman DM, Burkholder SG, Wang B, Beachy PA, Baylin SB. Hedgehog signalling within airway epithelial progenitors and in small-cell lung cancer. Nature (2003) 422(6929):313–7. doi:10.1038/nature01493
31. Gjorevski N, Nelson CM. Bidirectional extracellular matrix signaling during tissue morphogenesis. Cytokine Growth Factor Rev (2009) 20(5–6):459–65. doi:10.1016/j.cytogfr.2009.10.013
32. Sueblinvong V, Loi R, Eisenhauer PL, Bernstein IM, Suratt BT, Spees JL, et al. Derivation of lung epithelium from human cord blood-derived mesenchymal stem cells. Am J Respir Crit Care Med (2008) 177(7):701–11. doi:10.1164/rccm.200706-859OC
33. Carraro G, Perin L, Sedrakyan S, Giuliani S, Tiozzo C, Lee J, et al. Human amniotic fluid stem cells can integrate and differentiate into epithelial lung lineages. Stem Cells (2008) 26(11):2902–11. doi:10.1634/stemcells.2008-0090
34. Pierro M, Ionescu L, Montemurro T, Vadivel A, Weissmann G, Oudit G, et al. Short-term, long-term and paracrine effect of human umbilical cord-derived stem cells in lung injury prevention and repair in experimental bronchopulmonary dysplasia. Thorax (2013) 68(5):475–84. doi:10.1136/thoraxjnl-2012-202323
35. Ionescu L, Byrne RN, van Haaften T, Vadivel A, Alphonse RS, Rey-Parra GJ, et al. Stem cell conditioned medium improves acute lung injury in mice: in vivo evidence for stem cell paracrine action. Am J Physiol Lung Cell Mol Physiol (2012) 303(11):L967–77. doi:10.1152/ajplung.00144.2011
36. Sueblinvong V, Weiss DJ. Cell therapy approaches for lung diseases: current status. Curr Opin Pharmacol (2009) 9(3):268–73. doi:10.1016/j.coph.2009.03.002
37. Griffin MD, Ritter T, Mahon BP. Immunological aspects of allogeneic mesenchymal stem cell therapies. Hum Gene Ther (2010) 21(12):1641–55. doi:10.1089/hum.2010.156
38. Wong AP, Keating A, Lu WY, Duchesneau P, Wang X, Sacher A, et al. Identification of a bone marrow-derived epithelial-like population capable of repopulating injured mouse airway epithelium. J Clin Invest (2009) 119(2):336–48. doi:10.1172/JCI36882
39. Lee JW, Fang X, Gupta N, Serikov V, Matthay MA. Allogeneic human mesenchymal stem cells for treatment of E. coli endotoxin-induced acute lung injury in the ex vivo perfused human lung. Proc Natl Acad Sci U S A (2009) 106(38):16357–62. doi:10.1073/pnas.0907996106
40. Danchuk S, Ylostalo JH, Hossain F, Sorge R, Ramsey A, Bonvillain RW, et al. Human multipotent stromal cells attenuate lipopolysaccharide-induced acute lung injury in mice via secretion of tumor necrosis factor-alpha-induced protein 6. Stem Cell Res Ther (2011) 2(3):27. doi:10.1186/scrt68
41. Bustos ML, Mura M, Marcus P, Hwang D, Ludkovski O, Wong AP, et al. Bone marrow cells expressing clara cell secretory protein increase epithelial repair after ablation of pulmonary clara cells. Mol Ther (2013) 21(6):1251–8. doi:10.1038/mt.2013.53
42. Chang YS, Oh W, Choi SJ, Sung DK, Kim SY, Choi EY, et al. Human umbilical cord blood-derived mesenchymal stem cells attenuate hyperoxia-induced lung injury in neonatal rats. Cell Transplant (2009) 18(8):869–86. doi:10.3727/096368909X471189
43. Zhang H, Fang J, Wu Y, Mai Y, Lai W, Su H. Mesenchymal stem cells protect against neonatal rat hyperoxic lung injury. Expert Opin Biol Ther (2013) 13(6):817–29. doi:10.1517/14712598.2013.778969
44. Hansmann G, Fernandez-Gonzalez A, Aslam M, Vitali SH, Martin T, Mitsialis SA, et al. Mesenchymal stem cell-mediated reversal of bronchopulmonary dysplasia and associated pulmonary hypertension. Pulm Circ (2012) 2(2):170–81. doi:10.4103/2045-8932.97603
45. O’Reilly M, Thebaud B. Animal models of bronchopulmonary dysplasia. The term rat models. Am J Physiol Lung Cell Mol Physiol (2014) 307(12):L948–58. doi:10.1152/ajplung.00160.2014
46. Rehman J, Li J, Orschell CM, March KL. Peripheral blood “endothelial progenitor cells” are derived from monocyte/macrophages and secrete angiogenic growth factors. Circulation (2003) 107(8):1164–9. doi:10.1161/01.CIR.0000058702.69484.A0
47. Kung EF, Wang F, Schechner JS. In vivo perfusion of human skin substitutes with microvessels formed by adult circulating endothelial progenitor cells. Dermatol Surg (2008) 34(2):137–46. doi:10.1111/j.1524-4725.2007.34030.x
48. Shepherd BR, Enis DR, Wang F, Suarez Y, Pober JS, Schechner JS. Vascularization and engraftment of a human skin substitute using circulating progenitor cell-derived endothelial cells. FASEB J (2006) 20(10):1739–41. doi:10.1096/fj.05-5682fje
49. DeKoninck P, Toelen J, Roubliova X, Carter S, Pozzobon M, Russo FM, et al. The use of human amniotic fluid stem cells as an adjunct to promote pulmonary development in a rabbit model for congenital diaphragmatic hernia. Prenat Diagn (2015) 35(9):833–40. doi:10.1002/pd.4621
50. Buckley S, Shi W, Carraro G, Sedrakyan S, Da Sacco S, Driscoll BA, et al. The milieu of damaged alveolar epithelial type 2 cells stimulates alveolar wound repair by endogenous and exogenous progenitors. Am J Respir Cell Mol Biol (2011) 45(6):1212–21. doi:10.1165/rcmb.2010-0325OC
51. Hodges RJ, Jenkin G, Hooper SB, Allison B, Lim R, Dickinson H, et al. Human amnion epithelial cells reduce ventilation-induced preterm lung injury in fetal sheep. Am J Obstet Gynecol (2012) 206(5):e8–15. doi:10.1016/j.ajog.2012.02.038
52. Vosdoganes P, Hodges RJ, Lim R, Westover AJ, Acharya RY, Wallace EM, et al. Human amnion epithelial cells as a treatment for inflammation-induced fetal lung injury in sheep. Am J Obstet Gynecol (2011) 205(2):e26–33. doi:10.1016/j.ajog.2011.03.054
53. Moodley Y, Ilancheran S, Samuel C, Vaghjiani V, Atienza D, Williams ED, et al. Human amnion epithelial cell transplantation abrogates lung fibrosis and augments repair. Am J Respir Crit Care Med (2010) 182(5):643–51. doi:10.1164/rccm.201001-0014OC
54. Murphy S, Lim R, Dickinson H, Acharya R, Rosli S, Jenkin G, et al. Human amnion epithelial cells prevent bleomycin-induced lung injury and preserve lung function. Cell Transplant (2011) 20(6):909–23. doi:10.3727/096368910X543385
55. Murphy SV, Lim R, Heraud P, Cholewa M, Le Gros M, de Jonge MD, et al. Human amnion epithelial cells induced to express functional cystic fibrosis transmembrane conductance regulator. PLoS One (2012) 7(9):e46533. doi:10.1371/journal.pone.0046533
56. Yannarelli G, Dayan V, Pacienza N, Lee CJ, Medin J, Keating A. Human umbilical cord perivascular cells exhibit enhanced cardiomyocyte reprogramming and cardiac function after experimental acute myocardial infarction. Cell Transplant (2013) 22(9):1651–66. doi:10.3727/096368912X657675
57. Barry FP, Murphy JM, English K, Mahon BP. Immunogenicity of adult mesenchymal stem cells: lessons from the fetal allograft. Stem Cells Dev (2005) 14(3):252–65. doi:10.1089/scd.2005.14.252
58. Weiss DJ, Casaburi R, Flannery R, LeRoux-Williams M, Tashkin DP. A placebo-controlled, randomized trial of mesenchymal stem cells in COPD. Chest (2013) 143(6):1590–8. doi:10.1378/chest.12-2094
59. Chang YS, Ahn SY, Yoo HS, Sung SI, Choi SJ, Oh WI, et al. Mesenchymal stem cells for bronchopulmonary dysplasia: phase 1 dose-escalation clinical trial. J Pediatr (2014) 164(5):966.e–72.e. doi:10.1016/j.jpeds.2013.12.011
60. Mobius MA, Thebaud B. Stem cells and their mediators – next generation therapy for bronchopulmonary dysplasia. Front Med (2015) 2:50. doi:10.3389/fmed.2015.00050
61. Thébaud B, Ladha F, Michelakis ED, Sawicka M, Thurston G, Eaton F, et al. Vascular endothelial growth factor gene therapy increases survival, promotes lung angiogenesis, and prevents alveolar damage in hyperoxia-induced lung injury: evidence that angiogenesis participates in alveolarization. Circulation (2005) 112(16):2477–86. doi:10.1161/CIRCULATIONAHA.105.541524
62. Basile DP, Yoder MC. Circulating and tissue resident endothelial progenitor cells. J Cell Physiol (2014) 229(1):10–6. doi:10.1002/jcp.24423
63. Borghesi A, Massa M, Campanelli R, Bollani L, Tzialla C, Figar TA, et al. Circulating endothelial progenitor cells in preterm infants with bronchopulmonary dysplasia. Am J Respir Crit Care Med (2009) 180(6):540–6. doi:10.1164/rccm.200812-1949OC
64. Balasubramaniam V, Mervis CF, Maxey AM, Markham NE, Abman SH. Hyperoxia reduces bone marrow, circulating, and lung endothelial progenitor cells in the developing lung: implications for the pathogenesis of bronchopulmonary dysplasia. Am J Physiol Lung Cell Mol Physiol (2007) 292(5):L1073–84. doi:10.1152/ajplung.00347.2006
65. Paviotti G, Fadini GP, Boscaro E, Agostini C, Avogaro A, Chiandetti L, et al. Endothelial progenitor cells, bronchopulmonary dysplasia and other short-term outcomes of extremely preterm birth. Early Hum Dev (2011) 87(7):461–5. doi:10.1016/j.earlhumdev.2011.03.011
66. Zhu JH, Wang XX, Zhang FR, Shang YP, Tao QM, Zhu JH, et al. Safety and efficacy of autologous endothelial progenitor cells transplantation in children with idiopathic pulmonary arterial hypertension: open-label pilot study. Pediatr Transplant (2008) 12(6):650–5. doi:10.1111/j.1399-3046.2007.00863.x
67. Shand J, Berg J, Bogue C; Committee for Pediatric Research; Committee on Bioethics. Human embryonic stem cell (hESC) and human embryo research. Pediatrics (2012) 130(5):972–7. doi:10.1542/peds.2012-2482
68. Takahashi K, Tanabe K, Ohnuki M, Narita M, Ichisaka T, Tomoda K, et al. Induction of pluripotent stem cells from adult human fibroblasts by defined factors. Cell (2007) 131(5):861–72. doi:10.1016/j.cell.2007.11.019
69. Green MD, Chen A, Nostro MC, d’Souza SL, Schaniel C, Lemischka IR, et al. Generation of anterior foregut endoderm from human embryonic and induced pluripotent stem cells. Nat Biotechnol (2011) 29(3):267–72. doi:10.1038/nbt.1788
70. Huang SX, Islam MN, O’Neill J, Hu Z, Yang YG, Chen YW, et al. Efficient generation of lung and airway epithelial cells from human pluripotent stem cells. Nat Biotechnol (2014) 32(1):84–91. doi:10.1038/nbt.2754
71. Longmire TA, Ikonomou L, Hawkins F, Christodoulou C, Cao Y, Jean JC, et al. Efficient derivation of purified lung and thyroid progenitors from embryonic stem cells. Cell Stem Cell (2012) 10(4):398–411. doi:10.1016/j.stem.2012.01.019
72. Wong AP, Bear CE, Chin S, Pasceri P, Thompson TO, Huan LJ, et al. Directed differentiation of human pluripotent stem cells into mature airway epithelia expressing functional CFTR protein. Nat Biotechnol (2012) 30(9):876–82. doi:10.1038/nbt.2328
73. Firth AL, Dargitz CT, Qualls SJ, Menon T, Wright R, Singer O, et al. Generation of multiciliated cells in functional airway epithelia from human induced pluripotent stem cells. Proc Natl Acad Sci U S A (2014) 111(17):E1723–30. doi:10.1073/pnas.1403470111
74. Gotoh S, Ito I, Nagasaki T, Yamamoto Y, Konishi S, Korogi Y, et al. Generation of alveolar epithelial spheroids via isolated progenitor cells from human pluripotent stem cells. Stem Cell Reports (2014) 3(3):394–403. doi:10.1016/j.stemcr.2014.07.005
75. Ghaedi M, Calle EA, Mendez JJ, Gard AL, Balestrini J, Booth A, et al. Human iPS cell-derived alveolar epithelium repopulates lung extracellular matrix. J Clin Invest (2013) 123(11):4950–62. doi:10.1172/JCI68793
76. Ren X, Moser PT, Gilpin SE, Okamoto T, Wu T, Tapias LF, et al. Engineering pulmonary vasculature in decellularized rat and human lungs. Nat Biotechnol (2015) 33(10):1097–102. doi:10.1038/nbt.3354
77. Shojaie S, Ermini L, Ackerley C, Wang J, Chin S, Yeganeh B, et al. Acellular lung scaffolds direct differentiation of endoderm to functional airway epithelial cells: requirement of matrix-bound HS proteoglycans. Stem Cell Reports (2015) 4(3):419–30. doi:10.1016/j.stemcr.2015.01.004
78. Gonfiotti A, Jaus MO, Barale D, Baiguera S, Comin C, Lavorini F, et al. The first tissue-engineered airway transplantation: 5-year follow-up results. Lancet (2014) 383(9913):238–44. doi:10.1016/S0140-6736(13)62033-4
Keywords: stem cells, lung, developmental biology, therapeutics, lung diseases
Citation: Leibel S and Post M (2016) Endogenous and Exogenous Stem/Progenitor Cells in the Lung and Their Role in the Pathogenesis and Treatment of Pediatric Lung Disease. Front. Pediatr. 4:36. doi: 10.3389/fped.2016.00036
Received: 28 January 2016; Accepted: 31 March 2016;
Published: 14 April 2016
Edited by:
Mary E. Sunday, Duke University Medical Center, USAReviewed by:
Sule Cataltepe, Brigham and Women’s Hospital and Harvard Medical School, USAMatthew K. Lee, University of Southern California, USA
Bernadette Marie Levesque, Boston University, USA
Copyright: © 2016 Leibel and Post. This is an open-access article distributed under the terms of the Creative Commons Attribution License (CC BY). The use, distribution or reproduction in other forums is permitted, provided the original author(s) or licensor are credited and that the original publication in this journal is cited, in accordance with accepted academic practice. No use, distribution or reproduction is permitted which does not comply with these terms.
*Correspondence: Martin Post, martin.post@sickkids.ca