- 1Division of Pharmacology, Utrecht Institute of Pharmaceutical Sciences, Faculty of Science, Utrecht University, Utrecht, Netherlands
- 2Pediatric Immunology, Laboratory of Translational Immunology, The Wilhelmina Children's Hospital, University Medical Center, Utrecht, Netherlands
- 3Department of Immunology and Department of Human Milk Research & Analytical Science, Danone Nutricia Research, Utrecht, Netherlands
One of the well-known features of human milk, is the capacity to protect against the risk and impact of neonatal infections, as well as to influence the onset of allergic and metabolic disease manifestations. The major objective of this review is to provide a detailed overview regarding the role of human milk, more specifically the diversity in human milk oligosaccharides (HMOS), on early life immune development. Novel insights in immune modulatory effects of HMOS obtained by in vitro as well as in vivo studies, adds to the understanding on how early life nutrition may impact immune development. Extensive description and analysis of single HMOS contributing to the diversity within the composition provided during breastfeeding will be discussed with specific emphasis on immune development and the susceptibility to neonatal and childhood infections.
The Protective Effect of Breastfeeding Against Infections
It has been long noted that breastfeeding protects newborns against infections. Infant formula has been developed over many decades into adequate nutrition for those infants who cannot receive human milk. However, even modern infant formulas lack many components tailor made by each mother for the immune imprinting of her baby, such as specific antibodies (based on the immunologic history of the mother) and human milk oligosaccharides (HMOS) (based on the mother's specific genetic makeup regarding e.g., Lewis (Le) blood group and secretor (Se) status). Exclusive breastfeeding until the age of 4 months followed by partial breastfeeding is associated with a reduction in respiratory and gastrointestinal infectious diseases (1). For example, infants admitted to the hospital with Respiratory Syncytial Virus infection (RSV) are less likely to have been breastfed (2, 3). Similarly, infants who are not exclusively breastfed at 6–8 weeks of age, have a higher risk of hospitalization in early life in relation to a wide range of common infections (2). On the contrary, specific prebiotic oligosaccharides [like short chain galacto- and long chain fructo-oligosaccharides (scGOS/lcFOS)] are already added in a 9:1 ratio to plain infant formula and have been shown to reduce the development of atopic eczema and allergies as well as reduce the impact of pediatric infections (4, 5). Therefore, further optimization of infant nutrition when breastfeeding is unavailable is a principal factor required to further support immune development in early life.
The protective effect of human milk is postulated to be achieved by several mechanisms including the provision of pathogen specific maternal antibodies. This will provide the infant with pathogen specific protection during the first months of life, in which infant's own B cell development has not reached its full potential. Specifically, within the first years of life, the B cell repertoire matures upon encounter of pathogens, eventually providing a full range of protection against the recurrent pathogens. The immunoglobulins in human milk possess a broad range of pathogen specificity, which mirrors the maternal antigenic state. In addition, the concentration of soluble IgA (sIgA) are remarkably high and variable, and correlate to levels of IgG and IgM detected within different regions (6). At birth (for example via vaginal delivery as well as during breastfeeding) the neonate encounters a large variety of microorganisms which are determinant in the establishment of the microbiome in adult-life (7, 8). The initial colonization follows successive steps and is altered through the first year of life by diverse factors including genetic as well as environmental factors such as, introduction of oral feeding. Human milk was shown to stimulate healthy intestinal microbial diversity which includes colonization of several Bifidobacteria and Lactobaccillus species, which in turn, will result in the development of a balanced metabolic response (8, 9). In addition, human milk provides direct support to further development of the immune system in the neonate (10, 11).
The immune system of the neonates needs to adapt and respond to diverse stimuli encountered in early life. Immune homeostasis is determined by the cross-talk between exposure to the mucosal surfaces encompassing cross talk between epithelial cells and underlying immune cells (12). Human milk contains diverse factors like HMOS, milk epidermal growth factor or vitamin A, which contribute to the development of the neonatal mucosa and thus, to the promotion of the neonatal immune system by counterbalancing the deficiencies in early life. These variations are designated as poor IgA production, defective antimicrobial peptide secretion, lack of epithelial chemokine secretion as well as increased permeability among others (6, 10, 13).
Beyond functional components in human milk also the intestinal microbiota can help to further develop these aspects of the mucosal immune system (2). This emphasizes the relevance of a healthy intestinal microbiome diversity for adequate immune development in the first years of life (9). Next to the various classes of pathogen specific immunoglobulins in human milk, the presence of antimicrobial molecules, including specific non-digestible free carbohydrate structures and other molecules like glycoconjugates in breast milk have been shown to bind to pathogens (14). The nutrient source in early life, in particular the non-digestible human milk oligosaccharides (HMOS) in case of breastfeeding are of importance for healthy neonatal microbial colonization (7, 8, 12), immune development (15–19), as well as B cell development (20). This review aims to reveal the current state of knowledge regarding the immunomodulatory properties of HMOS and its unique complexity with differences in short chain as well as long chain structures. Recently some of these structures have become available via manufacturing procedures, and it might be considered to apply these in future generations of infant milk formula (21).
Diversity of Human Milk Oligosaccharide Composition
HMOS are the third most abundant class of biomolecules found in human milk after lactose and lipids, reaching between 5 and 20 g/L in mature human milk (22). Up to 1% of HMOS are absorbed in the gastrointestinal tract and found available in systemic circulation (23). This diversity and abundance is unique in humans and not seen in other mammals (24). The concentration of total HMOS is subjected to variations dependent on lactational stage (25), maternal nutrition (26), genetic predisposition (27) or even geographic localization and socioeconomic environment of milk donors (28). Although HMOS are composed out of only 5 different monosaccharides, the structural complexity of HMOS encountered in human milk is unique (21). The monosaccharides which are used as building blocks for HMOS are glucose (Glc), galactose (Gal), N-Acetyl-Glucosamine (GlcNAc), fucose (Fuc), and sialic acid (Neu5Ac). These single monosaccharides are conjugated via several linkage types (i.e., glycosidic bonds). With only a few exceptions, HMOS structures do follow a strict building plan (Figure 1). Each HMOS structure starts with a lactose unit “Gal (β1-4) Glc” which results from formation of a β1-4 glycosidic linkage between galactose and glucose catalyzed by the lactose synthase protein complex (30). Several tri-saccharides can be synthesized by appending either galactose or fucose to the reducing or non-reducing end of the lactose residue, which is performed through galactosyl- or fucosyl-transferase activity. Resulting components are e.g., 3′-galactosyllactose (Gal(β1-3)Gal(β1-4)Glc), 4′-galactosyllactose (Gal(β1-4)Gal(β1-4)Glc), 6′-galactosyllactose (Gal(β1-6)Gal(β1-4)Glc), 2′-FL (Fuc(α 1-2)Gal(β1-4)Glc), and 3-fucosyllactose (3-FL) (Gal(β1-4)[Fucα1-3]Glc). If sialic acids are connected to the non-reducing end of lactose via sialyl-transferases, 3′-sialyllactose (3′-SL; Neu5Ac (α 2-3)Gal(β1-4)Glc) and 6′-sialyllactose (6′-SL) (Neu5Ac(α 2-6)Gal(β1-4)Glc) are formed. Further elongation of lactose via the free 3-OH group of galactose can occur by addition of Gal (β1-x)GlcNAc units of either type I (Gal (β1-3)GlcNAc, Lacto-N-biose) or type II (Gal (β1-4)GlcNAc, N-Acetyllactosamine). Up to now, 19 different human milk oligosaccharide core structures have been described. These core structures may be linear or branched and can be further decorated with fucoses or sialic acid residues. Which indicates a myriad of different HMOS structures produced in the human mammary gland. The cellular localization of HMOS synthesis in the mammary gland epithelium is believed to be the Golgi apparatus.
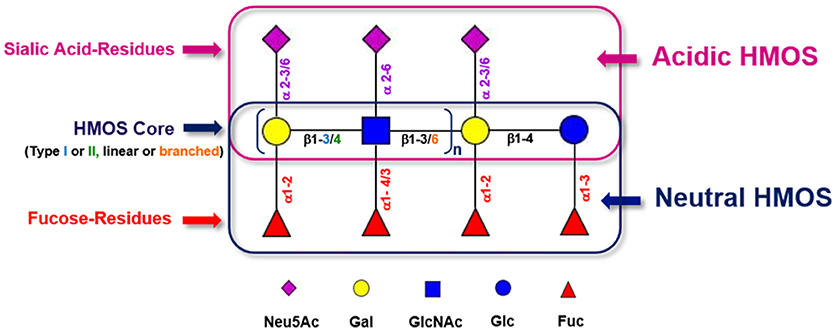
Figure 1. Generic building scheme of HMOS. Lactose and Type I (Gal (β1-3)GlcNAc-R) or Type II (Gal (β1-4)GlcNAc-R) HMOS core structures can be further extended linearly by adding additional Gal-GlcNAc building blocks to terminal Galactoses via β1-3 glycosidic linkages or via β1-6 glycosidic linkages. In the latter case, branching of the HMOS structure occurs. The (elongated) HMOS core structures can be further decorated with Fucoses (Fuc) and/or Sialic Acid (Neu5Ac) residues following distinct rules. Symbolic representation of monosaccharides according to CFG guidelines (29).
Among other early life factors, the individual maternal genetic disposition has a huge influence on the HMOS profile of human milk. More specifically, the individual expression pattern of Lewis (Le) and Secretor (Se) gene alleles codes for different fucosyl-transferases (FUTs), as shown in Table 1 The activity of these FUTs can lead to fucosylation of lactose and various other human milk core structures as indicated.

Table 1. Relationship between maternal genotype and exemplified Le- or Se- related major HMOS expected to be present in milks of respective milk types.
An active Se gen codes for FUT2 which transfers fucose via an α 1-2 glycosidic linkage. Prominent HMOS resulting from FUT2 activity are e.g., 2′-FL and LNFP I. Glycans like LNFP I which are carrying the reducing terminus Fuc (α1-2)Gal (β1-3)GlcNAc belong to the group of Led or H type 1 antigens. H type antigens link the Le/Se system with the blood group ABH system (31). In contrast, an active Le gene codes for FUT3 which in turn enables fucosylation via either α1-3 or α1-4 glycosidic linkage. FUT3 related structures are e.g., LNFP II and LNFP III. LNFP III is also an example for an Lewisx (Lex) structural motif, whereas LNFP II represents a Lewisa (Lea) epitope. Lea epitopes are characterized by the carbohydrate sequence Gal (β1-3)[Fuc (α1-4)]GlcNAc-R. Lex-antigens contain type II structures with the following residue: Gal (β1-4)[Fuc (α1-3)]GlcNAc-R. If both, Se and Le genes are active, fucosylated HMOS structures bearing either one, two or all the possible types of fucosylation (i.e., via α 1-2, α 1-3, and α 1-4 glycosidic linkages) can occur. Lacto-N-difucohexaose I (LNDFH I) which also resembles a Lewisb epitope with the monosaccharide motif Fuc (α1-2)Gal (β1-3)[Fuc (α1-4)]GlcNAc-R, is a known metabolite of joined FUT2 and FUT3 activity. It is noteworthy to mention that also other, Le/Se-system independent fucosyl-transferases may contribute to formation of α 1-3-fucosylated HMOS such as 3′-FL or LNFP V.
The complexity and relative abundance of different HMOS contained in human milk can for instance be characterized by size exclusion chromatography (SEC) and coupled refractive index detection (RI). A resulting SEC-RI trace is shown in Figure 2. Even more detailed information about complexity and individual monosaccharide compositions of HMOS could be derived by a subsequent MALDI-MS (33) analysis of individual SEC HMOS fractions. The acidic sub-fraction adds a further dimension to the overall variety of HMOS. The total number of neutral and acidic HMOS structures based on the MALDI-MS analyses of total human milk carbohydrate SEC-fractions is estimated to exceed the number of 1,000 different structures (24).
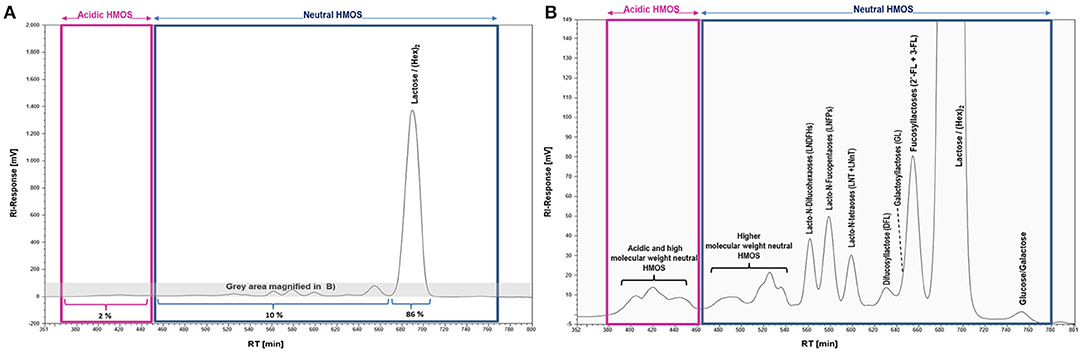
Figure 2. SEC-RI profiles of the total HMOS and mineral fraction from pooled human milk; (A) Full SEC-RI Profile, 86% of compounds detected by RI consist of Lactose/(Hex)2, 10% of other neutral HMOS and 2% of acidic HMOS; (B) Magnified section of (A) zooming into acidic and neutral HMOS; HM sampling, pooling, isolation of the total HMOS fraction and SEC-RI analysis have been performed as described earlier (32).
Based on the Le/Se status of the mother and specifically the related fucosylated HMOS structures found in the respective human milks, milk group systems of 4 different milk types have been defined (27). Therefore, it is possible to determine individual human milk types by probing presence of specific fucosylated HMOS like 2′-FL, DFL, LNFP I, LNFP II, LNDFH I, and LNDFH II with suited analytical means. An overview of the relationship between maternal Le and Se genotype and some major HMOS structures present in the respective milk types is given in Table 1. A recent review has summarized most of the qualitative and quantitative approaches to characterize the diversity of HMOS structures present within human milk (21).
Biological Functions of the Different HMOS
The presence of the unique diversity of HMOS, suggests different biological functions and mechanisms by which they may influence the infant's microbiome and immune maturation and their susceptibility to infections as summarized below and shown in Figure 3.
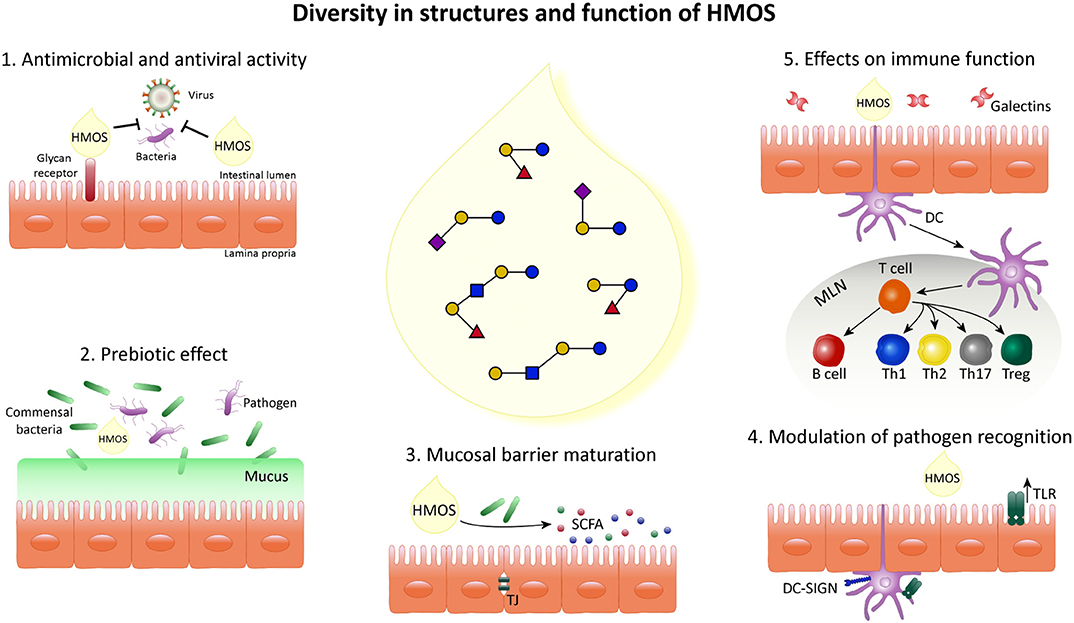
Figure 3. Schematic overview indicating the diversity in structure and function of HMOS. HMOS are composed of a complex mixture of oligosaccharides. This diversity of structures results in various roles in the epithelial cell layer, surrounding mucosa and immune system composing the digestive tract of breastfed infants. (1) HMOS have shown antimicrobial and antiviral effects by binding to virus bacteria, toxins and/or eukaryotes reaching the mucosal surfaces as well as by direct binding to epithelial surface receptors and blocking the access of pathogens. Thus, avoiding their replication and subsequent infection. (2) Commensal bacteria, illustrated as Bifidobacteria, metabolize HMOS and thus, their growth is promoted while pathogens less able to metabolize HMOS will experience growth suppression. (3) HMOS and Short Chain Fatty Acid (SCFA), metabolites of HMOS, were shown to influence intestinal epithelial cell (IEC) maturation by promoting differentiation while suppressing proliferation as well as tight junction development, required for proper intestinal barrier function. (4) Expression of receptors involved in pathogen recognition, such as TLR as well as their signal transduction was increased after HMOS exposure which in turn impacts the immune homeostasis. (5) DC in close proximity to the intestinal epithelial barrier are involved in the immunomodulatory effects described for HMOS. DCs exposed to HMOS play a role in the DC/T cell interaction leading to T cell differentiation and/or T cell/B cell interaction which may occur in secondary lymphoid organs, depicted as mesenteric lymph nodes (MLN), subsequently promoting immune homeostasis.
The topics exemplified in Figure 3 are further substantiated point by point in the following section.
Antimicrobial and Antiviral Effects of HMOS
HMOS play a role in the prevention of infections in breastfed infants by direct blockage of viral and bacterial cellular pathogens and toxins infection by mimicking cell entry receptors (34–36). The first mechanisms by which HMOS may exert their anti-infective properties are through the inhibition of virus binding to the host cells by mimicking viral receptors and/or by blocking virus entry into the cell, as well as intracellularly, by blocking viral replication. The anti-infective potential of HMOS has been demonstrated for both neutral as well as acidic HMOS, for example different strains of Norovirus have affinity for specific HMOS structures (35, 37). In addition, both sialylated and fucosylated milk oligosaccharides reduced the infectivity of rotavirus (38). Interestingly, HMOS with multiple Lex epitopes were shown to inhibit HIV-1 transfer to CD4+ T lymphocytes more efficiently than other HMOS structures (39). HMOS may also block microbial pathogen entry, since HMOS from pooled human milk were shown to significantly reduce Escherichia coli attachment to cultured epithelial cells (40). Likewise, it has been shown in vitro that LNT, or its fucosylated derivative LNFPI, both can inhibit the growth of Group B Streptococci (41). Moreover, the presence of 3′-FL within the complex mixture of HMOS structures has been inversely correlated with Group-B Streptococci abundancy in infants (42). In addition, α(1-2)-fucosylated HMOS like 2′-FL, or LNDFH I may reduce of early life diarrhea incidence and severity, via their ability to block specific diarrhea inducing pathogens (43).
Prebiotic Effect of HMOS
Development of selective bacterial strains is subjected to their capacity to metabolize HMOS (44). The role of microbial modulation i.e., the prebiotic capacity of specific HMOS structures have in addition been subject of extensive studies. More specifically, secretor positivity of mothers, hence expressing FUT2 and therefore able to produce α(1-2)-glycosidic-fucosylated HMOS, have been shown to affect the gut bifidobacterial communities of breastfed infants (45). Bifidobacteria and Bacteroides species are known to metabolize HMOS with high efficiency in contrast to other bacterial species such as E. coli, Clostridia, Eubacteria, Enterococci (44). This appears strain specific and selective for specific HMOS structure (44, 46, 47). For example, Bifidobacteria exhibited strong growth stimulation while expansion of Clostridium perfringens and E. coli were suppressed within cultures using specific HMOS (like 2′-FL, 3-FL, and LDFT), whereas Enterobacteria could not grow on 2′-FL or 6′-SL cultures (48). In addition, utilization of fucosylated type human milk oligosaccharides by isolated human gut microbes was shown (49). These data indicate selective and specific prebiotic capacities of different functional HMOS structures, showing growth of commensal bacteria such as Bifidobacteria at the expense of pathogens, as shown in Figure 3. Hence beyond directly blocking viral and bacterial entrance to the host also these prebiotic capacities of HMOS may help to reduce the susceptibility to infection of the host.
Mucosal Barrier Maturation by HMOS
HMOS interact with glycans present in the surface of intestinal epithelial cells (IEC) or with dendritic cells (DC) which protrude to the gut lumen from lamina propria. This results in direct support of epithelial barrier maturation or an indirect effect on barrier integrity via modulation of the microbiota and consequent short chain fatty acid (SCFA) production (50). In this regard, beyond blocking pathogen invasion, HMOS may also promote mucosal barrier maturation by increasing the differentiation of IECs. Indeed, synthetic HMOS or HMOS isolated from human milk were shown to promote differentiation and reduce proliferation of various IEC cultures (HT-29 and Caco-2). Similarly, expression of mucosal maturation factors was promoted in fetal intestine cultures after exposure to HMOS isolated from colostrum. These findings suggest that some specific HMOS may be able to promote gut maturation and contribute to epithelial barrier integrity in the gastrointestinal tract of neonates (18, 50, 51).
Modulation of Pathogen Recognition by HMOS
Receptors involved in the recognition of microbes such as toll-like receptors (TLR) are suggested to be modulated by HMOS. Subsequently the response of the host cell to pathogens is altered (17, 37). In vitro studies to elucidate the receptors involved in HMOS effects have been performed mostly in cells isolated from adult individuals which might not translate directly to the neonatal situation. Specific HMOS structures have been postulated to modulate bacterial and viral signaling on epithelial cells and/or DC (19). For instance, 2′-FL modulates CD14 expression in human enterocytes, thereby attenuating LPS-induced inflammation in vitro (17). On the contrary, HMOS such as sialyllactoses, human galactosyllactoses and/or LNFP III may be ligands for toll like receptors (TLR). For example, TLR-3 signaling seems specifically inhibited by human milk 3′-galactosylactose (52). Moreover, it has been shown that the addition of human milk as well as HMOS interacts directly with DCs, through DC-SIGN, Siglecs and related glycan-binding proteins which are also essential in immune regulation (53–55). DCs are key in directing the adaptive immune response toward effective immunity identification and clearance pathogens. Alpha-fucosylated HMOS (2′-FL and 3-FL) showed specific binding to DC-SIGN (54). Effects of scGOS/lcFOS were suggested to be mediated by TLR-4 (56). Similarly, TLR-4 as well as TLR-3 have also been related to modulate the effects of HMOS. 3′-FL, 2′-FL were able to modulate TLR-3 and elicit an anti-inflammatory effect, while exposure to 2′-FL inhibited inflammation through TLR-4 (52). More specifically it has clearly been shown that the addition of scGOS/lcFOS ameliorates the microbial composition reducing the presence of clinically relevant pathogens (57). Selectins were also suggested as possible receptors for binding of HMOS due to their ability to block P-selectin (58). Several receptors are hypothesized to be involved in the recognition of HMOS. The diversity of HMOS structures present in human milk might determine HMOS-glycan receptor binding. HMOS target TLRs and C-type lectins which are vital in pathogen recognition, immune modulation and essential during development of the immune system in early life. Therefore, HMOS may contribute to the development of a balanced and effective immune response, hereby providing protection toward infections.
Effect on Immune System Development by HMOS
Specific HMOS, such as 2′-FL, 3′-SL, 6′-SL, and LNT have been detected within the intestine as well as in systemic circulation of breastfed infants (23, 59, 60). Increasing evidence collected during the past two decades suggesting a role of HMOS directly on immune cells. Despite all efforts, the effects described remain rather incomplete (19). Nevertheless, it is suggested that HMOS play a role in supporting the developing mucosal and systemic immune system (13, 16). HMOS derived from human colostrum can modulate intrinsic expression of inflammatory markers associated with cell trafficking and modulate signaling pathways related to maturation of lymphoid tissue and influence cytokine and chemokine networks that regulate Th1/Th2 lymphocyte balance. The anti-inflammatory effect of for instance 2′-FL is known. 2′-FL from pooled human milk showed the ability to dampen pro-inflammatory mediator IL-8 release from T84 IEC line after type 1 pili E. coli infection (17). Similarly, reduced IL-8 expression was measured in fetal intestinal human tissue when exposed to 3′-, 4- and 6′-galactosyllactoses from human milk colostrum (17, 52). 2′-FL was shown to inhibit the inflammatory mediators secreted after TNFα induced in vitro, possibly through the inhibition of NF-κB activation (61). Furthermore, in vitro data demonstrate 2′-FL and LNFP I to be able to reduce monocyte activation and to modulate the release of IFNγ, IL-12, and IL-10 (62).
In addition, specific prebiotic oligosaccharides have been demonstrated to be immune modulatory (63–65). Immunomodulatory effects have been demonstrated for 2′-FL, suggesting an additional function of specific oligosaccharides (66–68). However, if these effects also relate to improved infection susceptibility in infants remains to be established. From the in vitro based human milk immune cell interaction studies some specific anti-inflammatory effects have been identified.
Galectins are another class of lectins involved in the regulation of immune and inflammatory processes (55). Interestingly, HMOS are reported to bind to various recombinant human galectins like hGal-1, -3, -4, -7, -8, and -9 in a very structure dependent and selective way. Human milk glycans with terminal type I sequences (Galβ1-3 GlcNAc) preferentially bind to hGal-7, whereas hGal-2 did not bind to human milk glyco-types but to a human blood group A Type 2 determinant (55). Beyond serving as a glycan receptor, galectins can also be secreted as soluble mediators and affect immune function. In this regard, IEC derived galectin-9 was increased after exposure of IEC to a mixture of scGOS/lcFOS in combination with a TLR-9 ligand in an in vitro co-culture model of IEC and activated immune cells (69). Galectin-9 played a key role in enhancing IFNγ and IL-10 production by immune cells underlying the IEC in this model (55). Further research will reveal the specific role of galectins in immunomodulation after exposure to HMOS, as well as their similarities with the immunomodulatory properties seen by scGOS/lcFOS. However, it is important to realize that an efficient immune response remains to be mounted against the intruding pathogen. Providing efficient protection, in most cases, will go hand in hand with the induction of inflammation. If an anti-inflammatory response is beneficial in relation to the protection against pathogens, will be pathogen and host specific, and can only be elucidated in vivo.
Human Milk Oligosaccharide Impact in vivo
It is the unique complexity of human milk oligosaccharides which leads to the speculation that these abundantly available structures in human milk play a key role in providing protection against infections in neonates. From the limited in vivo studies, we know that specific HMOS structures can reduce the interaction of specific pathogens like Salmonella, Shigella, Vibrio cholerae, E. coli, Polioviruses, Rotavirus and Respiratory Syncytial virus (RSV) with the host (11, 70). Within some studies, levels of 2′-FL, lacto-N-difucohexaose (LNDFH I), (α 2-linked fucosyloligosaccharide) and ratios between 2-linked and 3-/4-linked oligosaccharide were associated, with presence of specific pathogens like E. coli, Campylobacter and Norovirus (35, 43). Interestingly, the provision of secretory type related complex mixtures of HMOS, have been associated with a direct protection against specific infections (71). Fucosyltransferase 2 non-secretor and low secretor status seems to associate with severe outcomes in premature infants. Meaning that within this study a low secretor phenotype was associated with the onset of NEC, and non-secretor genotype was associated with gram negative sepsis (71). In addition, it has been suggested that FUT2, the regulator of Lewis and ABO(H) antigens in the intestinal mucosa, could be a host genotypic feature affecting susceptibility to ETEC infection (72). Several intervention studies have reported the functional benefit of adding prebiotic oligosaccharides to infant formula. More specifically, specific prebiotic oligosaccharides have been shown to ameliorate the development of allergies as well as reduce the impact of pediatric infections (4, 5, 73–75). In this regard, the immune modulating effect that seems to decrease the risk on developing atopy and allergy, also may lower the infection risk in neonates, which is suggestive for basic immune modulation early in life. The clinical consequences of specific individual HMOS structures however, remain to be further elucidated (76). The first clinical safety studies are now reported on the use of specific HMOS combinations i.e., 2′-FL and scGOS (60) or the combination of two single oligosaccharides 2′-FL and LNnT (68, 77). Although growth and 2′-FL uptake were similar between formula receiving infants and as seen in breastfed infants, the possible functional benefits regarding immune development and/or infection susceptibility related to a single oligosaccharide are however not extractable from these studies. Therefore, the identification and understanding of protective elements in human breast milk decreasing infant's susceptibility to infection remains limited (75).
In conclusion, components of breast milk (including HMOS) play a key role in the development of the neonatal immune system by preventing pathogen replication, promoting healthy microbial diversity, inducing maturation of intestinal mucosa and by modulation of immune cells as well as pathogen recognition receptors. Currently, there is little understanding about the role of the diverse HMOS structures in optimally inducing microbiome and immune development and consequently how they may provide protection against infections. Therefore, we postulate that HMOS are involved in regulation of mucosal immune and barrier function in multiple ways, although the specific mechanisms remain poorly understood and may be a compilation of the biological functions of individual structures and their interactions. Further investigation into the components of breast milk and their roles in providing protection to infants is required, irrespective of the mechanism by which specific HMOS structures can provide protection toward certain pathogens.
Author Contributions
VA-M, AvS, and MM have written the review. LW, JG, and BvL supervised the program. BS made specific contributions to the program with regard to human milk and in particular functional oligosaccharides. All authors listed have approved it for publication.
Conflict of Interest Statement
JG is head of the Division of Pharmacology, Utrecht Institute for Pharmaceutical Sciences, Faculty of Science at the Utrecht University and partly employed by Nutricia Research. BvL is leading a strategic alliance between University Medical Centre Utrecht/Wilhelmina Children's Hospital and Nutricia Research. BS, MM, and BvL are employed by Nutricia Research.
The remaining authors declare that the research was conducted in the absence of any commercial or financial relationships that could be construed as a potential conflict of interest.
Abbreviations
2′-FL, 2′-Fucosyllactose; 3′-SL, 3′-Sialyllactose; 6′-SL, 6′-Sialyllactose; APC, Antigen-presenting cells; DC, Dendritic cell; DC-SIGN, Dendritic Cell-Specific Intercellular adhesion molecule-3-Grabbing Non-integrin; FoxP3, Forkhead box protein 3; FUT 2, Fucosyltransferase 2; HMOS, Human Milk Oligosaccharides; lcFOS, Long Chain Fructo-oligosaccharides; MHC-I (II), Major Histocompatibility Complex Class I (II) molecules; SCFAs, Short Chain Fatty Acids; scGOS, Short Chain Galacto-oligosaccharides; tDC, tolerogenic dendritic cells; Th, T-helper cell; TJ, Tight-Junction; TLRs, Toll-Like Receptors; Tregs, Regulatory T cells.
References
1. Duijts L, Jaddoe VW, Hofman A, Moll HA. Prolonged and exclusive breastfeeding reduces the risk of infectious diseases in infancy. Pediatrics (2010) 126:e18–25. doi: 10.1542/peds.2008-3256
2. Ajetunmobi OM, Whyte B, Chalmers J, Tappin DM, Wolfson L, Fleming M, et al. Breastfeeding is associated with reduced childhood hospitalization: evidence from a Scottish Birth Cohort (1997-2009). J Pediatr. (2015) 166:620–5 e4. doi: 10.1016/j.jpeds.2014.11.013
3. Shi T, Balsells E, Wastnedge E, Singleton R, Rasmussen ZA, Zar HJ, et al. Risk factors for respiratory syncytial virus associated with acute lower respiratory infection in children under five years: systematic review and meta-analysis. J Glob Health (2015) 5:020416. doi: 10.7189/jogh.05.020416
4. Moro G, Arslanoglu S, Stahl B, Jelinek J, Wahn U, Boehm G. A mixture of prebiotic oligosaccharides reduces the incidence of atopic dermatitis during the first six months of age. Arch Dis Child. (2006) 91:814–9. doi: 10.1136/adc.2006.098251
5. Arslanoglu S, Moro GE, Boehm G. Early supplementation of prebiotic oligosaccharides protects formula-fed infants against infections during the first 6 months of life. J Nutr. (2007) 137:2420–4. doi: 10.1093/jn/137.11.2420
6. Ruiz L, Espinosa-Martos I, Garcia-Carral C, Manzano S, McGuire MK, Meehan CL, et al. What's normal? Immune profiling of human milk from healthy women living in different geographical and socioeconomic settings. Front Immunol. (2017) 8:696. doi: 10.3389/fimmu.2017.00696
7. Martin R, Nauta AJ, Ben Amor K, Knippels LM, Knol J, Garssen J. Early life: gut microbiota and immune development in infancy. Benef Microbes. (2010) 1:367–82. doi: 10.3920/BM2010.0027
8. Walker WA, Iyengar RS. Breast milk, microbiota, and intestinal immune homeostasis. Pediatr Res. (2015) 77:220–8. doi: 10.1038/pr.2014.160
9. Lloyd-Price J, Abu-Ali G, Huttenhower C. The healthy human microbiome. Genome Med. (2016) 8:51. doi: 10.1186/s13073-016-0307-y
10. Torow N, Marsland BJ, Hornef MW, Gollwitzer ES. Neonatal mucosal immunology. Mucosal Immunol. (2017) 10:5–17. doi: 10.1038/mi.2016.81
11. Turfkruyer M, Verhasselt V. Breast milk and its impact on maturation of the neonatal immune system. Curr Opin Infect Dis. (2015) 28:199–206. doi: 10.1097/QCO.0000000000000165
12. Renz H, Holt PG, Inouye M, Logan AC, Prescott SL, Sly PD. An exposome perspective: early-life events and immune development in a changing world. J Allergy Clin Immunol. (2017) 140:24–40. doi: 10.1016/j.jaci.2017.05.015
13. Hanson LA, Korotkova M, Lundin S, Haversen L, Silfverdal SA, Mattsby-Baltzer I, et al. The transfer of immunity from mother to child. Ann N Y Acad Sci. (2003) 987:199–206. doi: 10.1111/j.1749-6632.2003.tb06049.x
14. Peterson R, Cheah WY, Grinyer J, Packer N. Glycoconjugates in human milk: protecting infants from disease. Glycobiology (2013) 23:1425–38. doi: 10.1093/glycob/cwt072
15. Gollwitzer ES, Marsland BJ. Impact of early-life exposures on immune maturation and susceptibility to disease. Trends Immunol. (2015) 36:684–96. doi: 10.1016/j.it.2015.09.009
16. Newburg DS, Walker WA. Protection of the neonate by the innate immune system of developing gut and of human milk. Pediatr Res. (2007) 61:2–8. doi: 10.1203/01.pdr.0000250274.68571.18
17. He Y, Liu S, Kling DE, Leone S, Lawlor NT, Huang Y, et al. The human milk oligosaccharide 2'-fucosyllactose modulates CD14 expression in human enterocytes, thereby attenuating LPS-induced inflammation. Gut (2016) 65:33–46. doi: 10.1136/gutjnl-2014-307544
18. He Y, Liu S, Leone S, Newburg DS. Human colostrum oligosaccharides modulate major immunologic pathways of immature human intestine. Mucosal Immunol. (2014) 7:1326–39. doi: 10.1038/mi.2014.20
19. Kulinich A, Liu L. Human milk oligosaccharides: the role in the fine-tuning of innate immune responses. Carbohydr Res. (2016) 432:62–70. doi: 10.1016/j.carres.2016.07.009
20. Xiao L, Leusink-Muis T, Kettelarij N, van Ark I, Blijenberg B, Hesen NA, et al. Human milk oligosaccharide 2'-fucosyllactose improves innate and adaptive immunity in an influenza-specific murine vaccination model. Front Immunol. (2018) 9:452. doi: 10.3389/fimmu.2018.00452
21. Thurl S, Munzert M, Boehm G, Matthews C, Stahl B. Systematic review of the concentrations of oligosaccharides in human milk. Nutr Rev. (2017) 75:920–33. doi: 10.1093/nutrit/nux044
22. Urashima T, Asakuma S, Leo F, Fukuda K, Messer M, Oftedal OT. The predominance of type I oligosaccharides is a feature specific to human breast milk. Adv Nutr. (2012) 3:473S−82S. doi: 10.3945/an.111.001412
23. Goehring KC, Kennedy AD, Prieto PA, Buck RH. Direct evidence for the presence of human milk oligosaccharides in the circulation of breastfed infants. PLoS ONE (2014) 9:e101692. doi: 10.1371/journal.pone.0101692
24. Boehm G, Stahl B. Oligosaccharides from milk. J Nutr. (2007) 137(3 Suppl 2):847S–9S. doi: 10.1093/jn/137.3.847S
25. Davidson B, Meinzen-Derr JK, Wagner CL, Newburg DS, Morrow AL. Fucosylated oligosaccharides in human milk in relation to gestational age and stage of lactation. Adv Exp Med Biol. (2004) 554:427–30. doi: 10.1007/978-1-4757-4242-8_56
26. Charbonneau MR, O'Donnell D, Blanton LV, Totten SM, Davis JC, Barratt MJ, et al. Sialylated milk oligosaccharides promote microbiota-dependent growth in models of infant undernutrition. Cell (2016) 164:859–71. doi: 10.1016/j.cell.2016.01.024
27. Thurl S, Henker J, Siegel M, Tovar K, Sawatzki G. Detection of four human milk groups with respect to Lewis blood group dependent oligosaccharides. Glycoconj J. (1997) 14:795–9. doi: 10.1023/A:1018529703106
28. McGuire MK, Meehan CL, McGuire MA, Williams JE, Foster J, Sellen DW, et al. What's normal? Oligosaccharide concentrations and profiles in milk produced by healthy women vary geographically. Am J Clin Nutr. (2017) 105:1086–1100. doi: 10.3945/ajcn.116.139980
29. Varki A, Cummings RD, Aebi M, Packer NH, Seeberger PH, Esko JD, et al. Symbol nomenclature for graphical representations of glycans. Glycobiology (2015) 25:1323–4. doi: 10.1093/glycob/cwv091
30. Kuhn NJ, Carrick DT, Wilde CJ. Lactose synthesis: the possibilities of regulation. J Dairy Sci. (1980) 63:328–36. doi: 10.3168/jds.S0022-0302(80)82934-1
31. Oriol R, Le Pendu J, Mollicone R. Genetics of ABO, H, Lewis, X and related antigens. Vox Sang. (1986) 51:161–71. doi: 10.1111/j.1423-0410.1986.tb01946.x
32. Finke B, Mank M, Daniel H, Stahl B. Offline coupling of low-pressure anion-exchange chromatography with MALDI-MS to determine the elution order of human milk oligosaccharides. Anal Biochem. (2000) 284:256–65. doi: 10.1006/abio.2000.4680
33. Stahl B, Thurl S, Zeng JR, Karas M, Hillenkamp F, Steup M, et al. Oligosaccharides from human-milk as revealed by matrix-assisted laser-desorption ionization mass-spectrometry. Anal Biochem. (1994) 223:218–26. doi: 10.1006/abio.1994.1577
34. Iskarpatyoti JA, Morse EA, McClung RP, Ikizler M, Wetzel JD, Contractor N, et al. Serotype-specific differences in inhibition of reovirus infectivity by human-milk glycans are determined by viral attachment protein sigma1. Virology (2012) 433:489–97. doi: 10.1016/j.virol.2012.08.036
35. Shang J, Piskarev VE, Xia M, Huang P, Jiang X, Likhosherstov LM, et al. Identifying human milk glycans that inhibit norovirus binding using surface plasmon resonance. Glycobiology (2013) 23:1491–8. doi: 10.1093/glycob/cwt077
36. Pandey RP, Kim DH, Woo J, Song J, Jang SH, Kim JB, et al. Broad-spectrum neutralization of avian influenza viruses by sialylated human milk oligosaccharides: in vivo assessment of 3'-sialyllactose against H9N2 in chickens. Sci Rep. (2018) 8:2563. doi: 10.1038/s41598-018-20955-4
37. Weichert S, Koromyslova A, Singh BK, Hansman S, Jennewein S, Schroten H, et al. Structural basis for norovirus inhibition by human milk oligosaccharides. J Virol. (2016) 90:4843–8. doi: 10.1128/JVI.03223-15
38. Laucirica DR, Triantis V, Schoemaker R, Estes MK, Ramani S. Milk oligosaccharides inhibit human rotavirus infectivity in MA104 cells. J Nutr. (2017) 147:1709–14. doi: 10.3945/jn.116.246090
39. Naarding MA, Ludwig IS, Groot F, Berkhout B, Geijtenbeek TB, Pollakis G, et al. Lewis X component in human milk binds DC-SIGN and inhibits HIV-1 transfer to CD4+ T lymphocytes. J Clin Invest. (2005) 115:3256–64. doi: 10.1172/JCI25105
40. Manthey CF, Autran CA, Eckmann L, Bode L. Human milk oligosaccharides protect against enteropathogenic Escherichia coli attachment in vitro and EPEC colonization in suckling mice. J Pediatr Gastroenterol Nutr. (2014) 58:165–8. doi: 10.1097/MPG.0000000000000172
41. Lin AE, Autran CA, Szyszka A, Escajadillo T, Huang M, Godula K, et al. Human milk oligosaccharides inhibit growth of group B Streptococcus. J Biol Chem. (2017) 292:11243–9. doi: 10.1074/jbc.M117.789974
42. Andreas NJ, Al-Khalidi A, Jaiteh M, Clarke E, Hyde MJ, Modi N, et al. Role of human milk oligosaccharides in Group B Streptococcus colonisation. Clin Transl Immunol. (2016) 5:e99. doi: 10.1038/cti.2016.43
43. Newburg DS, Ruiz-Palacios GM, Morrow AL. Human milk glycans protect infants against enteric pathogens. Annu Rev Nutr. (2005) 25:37–58. doi: 10.1146/annurev.nutr.25.050304.092553
44. Marcobal A, Barboza M, Froehlich JW, Block DE, German JB, Lebrilla CB, et al. Consumption of human milk oligosaccharides by gut-related microbes. J Agric Food Chem. (2010) 58:5334–40. doi: 10.1021/jf9044205
45. Lewis ZT, Totten SM, Smilowitz JT, Popovic M, Parker E, Lemay DG, et al. Maternal fucosyltransferase 2 status affects the gut bifidobacterial communities of breastfed infants. Microbiome (2015) 3:13. doi: 10.1186/s40168-015-0071-z
46. Sela DA, Li Y, Lerno L, Wu S, Marcobal AM, German JB, et al. An infant-associated bacterial commensal utilizes breast milk sialyloligosaccharides. J Biol Chem. (2011) 286:11909–18. doi: 10.1074/jbc.M110.193359
47. Asakuma S, Hatakeyama E, Urashima T, Yoshida E, Katayama T, Yamamoto K, et al. Physiology of consumption of human milk oligosaccharides by infant gut-associated bifidobacteria. J Biol Chem. (2011) 286:34583–92. doi: 10.1074/jbc.M111.248138
48. Hoeflinger JL, Davis SR, Chow J, Miller MJ. In vitro impact of human milk oligosaccharides on Enterobacteriaceae growth. J Agric Food Chem. (2015) 63:3295–302. doi: 10.1021/jf505721p
49. Yu ZT, Chen C, Kling DE, Liu B, McCoy JM, Merighi M, et al. The principal fucosylated oligosaccharides of human milk exhibit prebiotic properties on cultured infant microbiota. Glycobiology (2013) 23:169–77. doi: 10.1093/glycob/cws138
50. Holscher HD, Bode L, Tappenden KA. Human milk oligosaccharides influence intestinal epithelial cell maturation in vitro. J Pediatr Gastroenterol Nutr. (2017) 64:296–301. doi: 10.1097/MPG.0000000000001274
51. Kuntz S, Kunz C, Rudloff S. Oligosaccharides from human milk induce growth arrest via G2/M by influencing growth-related cell cycle genes in intestinal epithelial cells. Br J Nutr. (2009) 101:1306–15. doi: 10.1017/S0007114508079622
52. He Y, Lawlor NT, Newburg DS. Human milk components modulate toll-like receptor-mediated inflammation. Adv Nutr. (2016) 7:102–11. doi: 10.3945/an.115.010090
53. Stowell SR, Arthur CM, McBride R, Berger O, Razi N, Heimburg-Molinaro J, et al. Microbial glycan microarrays define key features of host-microbial interactions. Nat Chem Biol. (2014) 10:470–6. doi: 10.1038/nchembio.1525
54. Noll AJ, Yu Y, Lasanajak Y, Duska-McEwen G, Buck RH, Smith DF, et al. Human DC-SIGN binds specific human milk glycans. Biochem J. (2016) 473:1343–53. doi: 10.1042/BCJ20160046
55. Noll AJ, Gourdine JP, Yu Y, Lasanajak Y, Smith DF, Cummings RD. Galectins are human milk glycan receptors. Glycobiology (2016) 26:655–69. doi: 10.1093/glycob/cww002
56. Lehmann S, Hiller J, van Bergenhenegouwen J, Knippels LM, Garssen J, Traidl-Hoffmann C. In vitro evidence for immune-modulatory properties of non-digestible oligosaccharides: direct effect on human monocyte derived dendritic cells. PLoS ONE (2015) 10:e0132304. doi: 10.1371/journal.pone.0132304
57. Knol J, Boehm G, Lidestri M, Negretti F, Jelinek J, Agosti M, et al. Increase of faecal bifidobacteria due to dietary oligosaccharides induces a reduction of clinically relevant pathogen germs in the faeces of formula-fed preterm infants. Acta Paediatr Suppl. (2005) 94:31–3. doi: 10.1080/08035320510043529
58. Schumacher G, Bendas G, Stahl B, Beermann C. Human milk oligosaccharides affect P-selectin binding capacities: in vitro investigation. Nutrition (2006) 22:620–7. doi: 10.1016/j.nut.2005.12.009
59. Dotz V, Rudloff S, Blank D, Lochnit G, Geyer R, Kunz C. 13C-labeled oligosaccharides in breastfed infants' urine: individual-, structure- and time-dependent differences in the excretion. Glycobiology (2014) 24:185–94. doi: 10.1093/glycob/cwt099
60. Marriage BJ, Buck RH, Goehring KC, Oliver JS, Williams JA. Infants fed a lower calorie formula with 2'FL show growth and 2'FL uptake like breast-fed infants. J Pediatr Gastroenterol Nutr. (2015) 61:649–58. doi: 10.1097/MPG.0000000000000889
61. Newburg DS, Ko JS, Leone S, Nanthakumar NN. Human milk oligosaccharides and synthetic galactosyloligosaccharides contain 3'-, 4-, and 6'-galactosyllactose and attenuate inflammation in human T84, NCM-460, and H4 cells and intestinal tissue ex vivo. J Nutr. (2016) 146:358–67. doi: 10.3945/jn.115.220749
62. Sotgiu S, Arru G, Fois ML, Sanna A, Musumeci M, Rosati G, et al. Immunomodulation of fucosyl-lactose and lacto-N-fucopentaose on mononuclear cells from multiple sclerosis and healthy subjects. Int J Biomed Sci. (2006) 2:114–20.
63. Schijf MA, Kerperien J, Bastiaans J, Szklany K, Meerding J, Hofman G, et al. Alterations in regulatory T cells induced by specific oligosaccharides improve vaccine responsiveness in mice. PLoS ONE (2013) 8:e75148. doi: 10.1371/journal.pone.0075148
64. Schijf MA, Kruijsen D, Bastiaans J, Coenjaerts FE, Garssen J, van Bleek GM, et al. Specific dietary oligosaccharides increase Th1 responses in a mouse respiratory syncytial virus infection model. J Virol. (2012) 86:11472–82. doi: 10.1128/JVI.06708-11
65. van't Land B, Schijf M, van Esch BC, van Bergenhenegouwen J, Bastiaans J, Schouten B, et al. Regulatory T-cells have a prominent role in the immune modulated vaccine response by specific oligosaccharides. Vaccine (2010) 28:5711–7. doi: 10.1016/j.vaccine.2010.06.046
66. Xiao L, Van't Land B, Engen PA, Naqib A, Green SJ, Nato A, et al. Human milk oligosaccharides protect against the development of autoimmune diabetes in NOD-mice. Sci Rep. (2018) 8:3829. doi: 10.1038/s41598-018-22052-y
67. Koning N, Kessen SF, Van Der Voorn JP, Appelmelk BJ, Jeurink PV, Knippels LM, et al. Human milk blocks DC-SIGN-pathogen interaction via MUC1. Front Immunol. (2015) 6:112. doi: 10.3389/fimmu.2015.00112
68. Goehring KC, Marriage BJ, Oliver JS, Wilder JA, Barrett EG, Buck RH. Similar to those who are breastfed, infants fed a formula containing 2'-fucosyllactose have lower inflammatory cytokines in a randomized controlled trial. J Nutr. (2016) 146:2559–66. doi: 10.3945/jn.116.236919
69. de Kivit S, Kraneveld AD, Knippels LM, van Kooyk Y, Garssen J, Willemsen LE. Intestinal epithelium-derived galectin-9 is involved in the immunomodulating effects of nondigestible oligosaccharides. J Innate Immun. (2013) 5:625–38. doi: 10.1159/000350515
70. Goldman AS. The immune system in human milk and the developing infant. Breastfeed Med. (2007) 2:195–204. doi: 10.1089/bfm.2007.0024
71. Morrow AL, Meinzen-Derr J, Huang P, Schibler KR, Cahill T, Keddache M, et al. Fucosyltransferase 2 non-secretor and low secretor status predicts severe outcomes in premature infants. J Pediatr. (2011) 158:745–51. doi: 10.1016/j.jpeds.2010.10.043
72. Mottram L, Wiklund G, Larson G, Qadri F, Svennerholm AM. FUT2 non-secretor status is associated with altered susceptibility to symptomatic enterotoxigenic Escherichia coli infection in Bangladeshis. Sci Rep. (2017) 7:10649. doi: 10.1038/s41598-017-10854-5
73. Arslanoglu S, Moro GE, Boehm G, Wienz F, Stahl B, Bertino E. Early neutral prebiotic oligosaccharide supplementation reduces the incidence of some allergic manifestations in the first 5 years of life. J Biol Regul Homeost Agents (2012) 26(3 Suppl):49–59.
74. Gruber C, van Stuijvenberg M, Mosca F, Moro G, Chirico G, Braegger CP, et al. Reduced occurrence of early atopic dermatitis because of immunoactive prebiotics among low-atopy-risk infants. J Allergy Clin Immunol. (2010) 126:791–7. doi: 10.1016/j.jaci.2010.07.022
75. Ranucci G, Buccigrossi V, Borgia E, Piacentini D, Visentin F, Cantarutti L, et al. Galacto-oligosaccharide/polidextrose enriched formula protects against respiratory infections in infants at high risk of atopy: a randomized clinical trial. Nutrients (2018) 10:E286. doi: 10.3390/nu10030286
76. Munblit D, Peroni DG, Boix-Amoros A, Hsu PS, Van't Land B, Gay MCL, et al. Human milk and allergic diseases: an unsolved puzzle. Nutrients. (2017) 9:E894. doi: 10.3390/nu9080894
Keywords: human milk oligosaccharides, mucosal immunity, tolerogenic dendritic cells, infections, early life nutrition
Citation: Ayechu-Muruzabal V, van Stigt AH, Mank M, Willemsen LEM, Stahl B, Garssen J and van't Land B (2018) Diversity of Human Milk Oligosaccharides and Effects on Early Life Immune Development. Front. Pediatr. 6:239. doi: 10.3389/fped.2018.00239
Received: 30 April 2018; Accepted: 07 August 2018;
Published: 10 September 2018.
Edited by:
Valerie Verhasselt, University of Western Australia, AustraliaReviewed by:
Kirsty Le Doare, Imperial College London, United KingdomAntonio Condino-Neto, Universidade de São Paulo, Brazil
Copyright © 2018 Ayechu-Muruzabal, van Stigt, Mank, Willemsen, Stahl, Garssen and van't Land. This is an open-access article distributed under the terms of the Creative Commons Attribution License (CC BY). The use, distribution or reproduction in other forums is permitted, provided the original author(s) and the copyright owner(s) are credited and that the original publication in this journal is cited, in accordance with accepted academic practice. No use, distribution or reproduction is permitted which does not comply with these terms.
*Correspondence: Belinda van't Land, Yi52YW50bGFuZEB1bWN1dHJlY2h0Lm5s
†These authors have contributed equally to this work