Novel Strategies to Reduce Pulmonary Hypertension in Infants With Bronchopulmonary Dysplasia
- Department of Pediatrics, Section of Neonatology, Baylor College of Medicine, Texas Children's Hospital, Houston, TX, United States
Bronchopulmonary dysplasia (BPD) is a developmental lung disorder of preterm infants primarily caused by the failure of host defense mechanisms to prevent tissue injury and facilitate repair. This disorder is the most common complication of premature birth, and its incidence remains unchanged over the past few decades. Additionally, BPD increases long-term cardiopulmonary and neurodevelopmental morbidities of preterm infants. Pulmonary hypertension (PH) is a common morbidity of BPD. Importantly, the presence of PH increases both the short- and long-term morbidities and mortality in BPD infants. Further, there are no curative therapies for this complex disease. Besides providing an overview of the pathogenesis and diagnosis of PH associated with BPD, we have attempted to comprehensively review and summarize the current literature on the interventions to prevent and/or mitigate BPD and PH in preclinical studies. Our goal was to provide insight into the therapies that have a high translational potential to meaningfully manage BPD patients with PH.
Introduction
Bronchopulmonary dysplasia (BPD), the most common infantile chronic lung disease in the US, is still one of the most challenging complications of preterm infants. The histopathological hallmarks of this disease are alveolar simplification and dysmorphic pulmonary vascularization (1, 2). The definition of BPD continues to be very challenging and evolving as a result of the ongoing advances in the respiratory management of preterm infants (3–10). With increasing survival of extremely preterm infants, the incidence of this disease remains high (11–14). Further, BPD poses a major clinical, social, and economic problem (15, 16). Infants with this disease have long-term pulmonary, cardiovascular, and neurodevelopmental abnormalities (17–21). The hospitalization cost of BPD infants is twice that of non-BPD infants in the 1st year of life (16). Pulmonary hypertension (PH) is a common morbidity of BPD. The pooled prevalence rates of PH in mild, moderate, and severe BPD are 6, 12, and 39%, respectively (22). Importantly, the presence of PH increases both the short- and long-term morbidities and mortality in BPD infants (22–26). In this review, we provide a summary of the literature on the pathogenesis of BPD and PH and the potential strategies to prevent and manage this disease complex.
Methods for Literature Search
We searched PubMed and MEDLINE databases through the time period 1990 to present. The following mesh terms without any language restrictions were included in our search: “Bronchopulmonary Dysplasia” AND “Pulmonary Hypertension” OR “Bronchopulmonary Dysplasia” AND “Pulmonary Hypertension” AND “Pathogenesis” OR “Bronchopulmonary Dysplasia” AND “Pulmonary Hypertension” AND “Diagnosis” OR “Bronchopulmonary Dysplasia” AND “Pulmonary Hypertension” AND “Treatment OR Therapy OR Management,” OR “Bronchopulmonary Dysplasia” AND “Pulmonary Hypertension” AND “Novel Therapeutic Strategies” OR (“Bronchopulmonary Dysplasia” AND “Pulmonary Hypertension” OR Lung Injury”) AND (“Preclinical Studies” OR “Animals”). The two authors (AE-S and BS) independently evaluated the titles and abstracts of the studies for eligibility for inclusion in this review. We obtained the full-text version for assessment and resolved any differences by discussion.
Pathogenesis
The pathophysiology of PH in BPD is complex and is characterized by decreased pulmonary blood vessel density, endothelial cell dysfunction, and increased remodeling and altered vasoreactivity of resistance pulmonary arteries (blood vessels with a diameter of <150 μm) (27). The pathogenesis of this disease complex is multifactorial, and the pathogenic factors can be prenatal, natal, or postnatal in origin. The majority of these risk factors are common for both BPD and BPD with PH (25). Here, we attempt to briefly summarize the factors that predominantly predispose infants to develop BPD and PH. Placental abnormalities, especially those associated with hypo- or under-perfusion, increase the risk of BPD and PH (28, 29). Placental abnormalities are associated with decreased levels of proangiogenic and increased levels of anti-angiogenic molecules (30–32), which can inhibit angiogenesis and cause endothelial cell dysfunction at birth. Not surprisingly, infants born to mothers with such placental vascular anomalies are at high risk of BPD and PH because of the well-established association between the structural and functional abnormalities of the lung vasculature and the development of BPD (33). Fetal growth restriction, regardless of its severity, is a significant antenatal risk factor for the development of PH in BPD infants, whereas maternal hypertensive disorders without fetal growth restriction do not increase the risk of BPD and PH (34). Other prenatal risk factors for BPD and PH include genetic abnormalities, oligohydramnios, and maternal diabetes (35–42). Natal risk factors include preterm birth, low birthweight, and low 5-min Apgar scores (35, 39). Postnatal risk factors for this disease complex include oxidative stress, mechanical ventilation, infection, and hemodynamic overcirculation (42, 43).
Diagnosis and Evaluation
Cardiac catheterization remains the gold standard for the evaluation and diagnosis of PH (25). Catheterization is particularly helpful in detecting response to vasodilating agents and oxygen therapy (44). It is also helpful in characterizing pathologies such as pulmonary stenosis and aortopulmonary collaterals, in addition to left ventricular dysfunction (25). However, catheterization is invasive, requires general anesthesia, and carries several risks, especially in small infants. Therefore, the procedure should be considered wisely in infants with BPD and PH (44).
Echocardiography is the most widely used tool for the evaluation of pulmonary artery pressure because it is non-invasive and easily accessible. However, the optimal time to screen BPD infants for PH by echocardiography is uncertain. Considerable heterogeneity in the timing to screen for PH exists in neonatal intensive care units across the world. The consensus is to screen infants with moderate and severe BPD at 36 weeks postmenstrual age or infants requiring significant respiratory support with recurrent hypoxemic episodes at any age (45, 46). To obtain meaningful information, the echocardiography should evaluate and quantify the structural and pulmonary venous abnormalities; shunts; ventricular size, hypertrophy, and function in both systole and diastole; interventricular septal position in systole and diastole; and tricuspid and pulmonary regurgitation jet velocities (TRJV). Further, the systemic blood pressure values should be documented during the echocardiography screen (45). The TRJV is commonly used to estimate PH by echocardiography using a modified Bernoulli equation (47). A limitation of the TRJV measurement is that it is only detected in 61% of infants with BPD and PH, and its absence does not exclude PH (44, 48). Evaluation of other parameters like interventricular septum flattening and right ventricular hypertrophy or dilatation can be subjective and inaccurate. In patients with PH, it is recommended to evaluate the right and left ventricular function. The left ventricular eccentricity index measurements can be used to identify BPD infants suffering from PH. As the pulmonary pressure rises, it causes right ventricular overload and septal distortion. The left ventricular eccentricity index quantifies the septal distortion at end-systole and end-diastole and is reported to be significantly higher in patients with PH and BPD than those with BPD alone (49). Right ventricle (RV) systolic function can also be assessed by quantifying the tricuspid annular plane systolic excursion (TAPSE) (50). The TAPSE correlates directly with the gestational age (GA) and increases with advancing GA (51). In BPD infants with PH, the TAPSE is significantly decreased (52, 53). Pulmonary artery acceleration time (PAAT) is a reliable measure of RV afterload and, therefore, the pulmonary arterial pressure in infants and children (54). The PAAT and PAAT/RV ejection time are decreased in BPD infants with PH (55, 56). For detection of certain pathologies that are not identified by conventional methods, tissue Doppler and speckle tracking echocardiography have been suggested (57). Specifically, speckle tracking echocardiography is used in infants and children with PH to identify RV longitudinal strain, a parameter of RV systolic function. The strain measures the degree of myocardial deformation over time and is directly proportional to the myocardial contractility and ventricular systolic function (58). RV longitudinal strain is decreased in BPD infants with PH (59). It is also important to interrogate the pulmonary veins by Doppler echocardiography in BPD infants who have PH because pulmonary vein stenosis (PVS) is increasingly recognized in these infants (60, 61). Further, PVS develops over time, occurs more frequently in infants with necrotizing enterocolitis, and increases the mortality rate (62, 63).
Magnetic resonance imaging (MRI) can be a useful non-invasive imaging modality to characterize BPD and its associated complications, including PH (64). With advancements in MRI technology over the years, the scanning time and the need for sedation during imaging have been substantially decreased, making it a promising imaging modality for infants with BPD. While MRI has been used to evaluate PH in adults, its use in neonates and infants remains under investigation. Cardiac MRI is a three-dimensional imaging study that accurately quantifies the architectural details of the cardiac chambers, including their respective volumes, mass, and transvalvular flow (65). Recently, Critser et al. investigated whether cardiac MRI would predict BPD severity and identify the infants with BPD who required therapy for PH (26). The investigators used MRI to measure several parameters of cardiac structure and function, including the main pulmonary artery-to-aorta diameter (PA/AO) ratio, left ventricular eccentricity index (MR-EI), left and right ventricle end-systolic volumes, right ventricular end-diastolic volume, ejection fraction, and the cardiac index. Further, they used measurements obtained by clinically indicated echocardiography studies and compared the MRI and echocardiographic measurements with the following outcomes: BPD severity, length of hospital stay (LOS), duration of respiratory support, the need for respiratory support at discharge, and the need for PH therapy. The investigators observed that increased PA/AO ratio, MR-EI, and echocardiography-EI correlated with the predetermined clinical outcomes; however, there was no correlation between pulmonary arterial blood flow and the BPD outcomes. After controlling for the confounding variables, MR-EI predicted the LOS and duration of respiratory support, whereas the PA/AO ratio and MR-EI predicted the need for PH therapy both during hospitalization and at discharge. Despite the study limitations, the findings suggest that cardiac MRI may be useful to manage BPD infants with PH.
Serum brain natriuretic peptide (BNP) and its prohormone N-terminal-proBNP are released by the cardiomyocytes in response to neurohormonal factors (66) and are used for the diagnosis and monitoring of infants with PH in the context of BPD (67–69). Asymmetric dimethylarginine, endostatin, and angiopoietin are the other molecules that are abnormally expressed in BPD patients with PH (37, 70).
Importantly, infants with BPD and PH should be evaluated for comorbidities that could initiate and potentiate the pulmonary vascular disease. These morbidities include aspiration, gastroesophageal reflux disease, structural airway disease (vocal cord paralysis, subglottic stenosis, tracheomalacia, bronchomalacia, and airway hyperreactivity), PVS, left ventricle (LV) dysfunction, and aortopulmonary collaterals (45).
Management
Multidisciplinary care provided by a team of neonatologists, cardiologists, PH specialists, nursing staff, respiratory therapists, pharmacists, dieticians, occupational, and developmental specialists should be the norm for the comprehensive management of BPD infants with PH.
General management principles include strategies to avoid intermittent or chronic increases in the pulmonary vascular resistance PVR), treat comorbidities (45), provide optimal nutrition to allow the lungs to repair and recover from the chronic injury (7), and promote developmental support care to improve the neurodevelopmental outcomes (71). Maintaining oxygen saturation (SpO2) between 92 and 95% (72), avoiding hypercapnia, and preventing lung over- or under-inflation are the general approaches to decrease the PVR (73).
Data related to the dose, route of administration, efficacy, and safety of specific pulmonary vasodilators in BPD patients with PH are limited and continue to evolve. Increased pulmonary vascular pressures and RV dysfunction that persist after treating the comorbidities and excluding LV dysfunction and PVS are indications to initiate specific pulmonary vasodilator therapy in BPD infants (45). Inhaled nitric oxide (iNO) is commonly used to treat PH associated with BPD; however, the only FDA-approved indication of this medication in neonates is to treat infants >34 weeks' gestation with hypoxic respiratory failure secondary to PH (25, 74). Therapy with iNO selectively vasodilates the pulmonary vasculature, decreases PVR, and improves oxygenation in BPD infants who have PH (75, 76). There is limited data regarding the use of phosphodiesterase inhibitors (sildenafil and milrinone), endothelin receptor antagonist (bosentan), and prostacyclins and their analogs for the management of PH in BPD infants (25, 45).
Novel Experimental Therapies
This section includes data from preclinical studies that have modeled human BPD with PH, quantified the PH phenotype, and demonstrated the effects of the intervention on lung development and PH (Table 1).
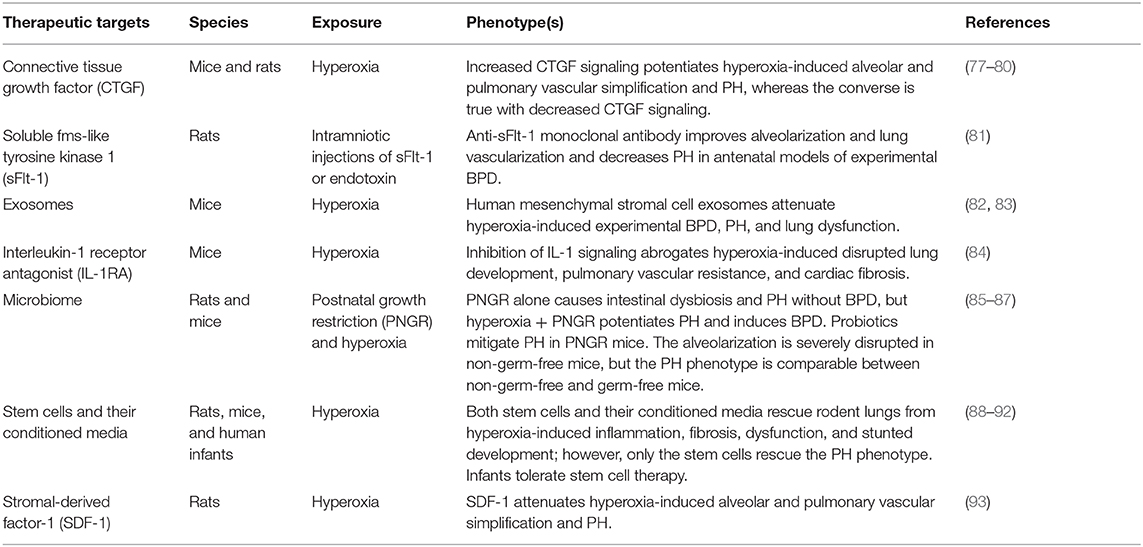
Table 1. Overview of the novel therapeutic interventions in experimental bronchopulmonary dysplasia and pulmonary hypertension.
Anti-connective Tissue Growth Factor Antibody
Connective tissue growth factor (CTGF) is a transforming growth factor beta target gene that regulates cell proliferation, adhesion and migration, extracellular matrix deposition, angiogenesis, and development of the skeletal system (94–97). Aberrant expression of CTGF is associated with tissue fibrosis and remodeling (98, 99). Further, the expression of this growth factor is increased in animal models and infants with BPD (77, 100, 101). Mechanistic preclinical studies have consistently demonstrated that CTGF disrupts postnatal lung development and potentiates experimental BPD with PH (78–80). Conversely, the administration of a neutralizing CTGF antibody to rats mitigates hyperoxia-induced alveolar and pulmonary vascular simplification as well as PH (77).
Anti-soluble fms-Like Tyrosine Kinase 1 Antibody
Soluble fms-like tyrosine kinase 1 (sFlt-1) is a soluble vascular endothelial growth factor (VEGF) receptor-1 that exerts anti-angiogenic effects by acting as an endogenous VEGF inhibitor (102–104). Elevated levels of this receptor increase the risk and severity of BPD in both animals (81) and humans (105). Using a rigorous approach, Wallace et al. (81) demonstrated that both prophylactic and rescue therapies with an anti-sFlt-1 monoclonal antibody in rats are effective in increasing alveolarization and lung vascularization and decreasing right ventricular hypertrophy in two prenatal models of BPD. Importantly, these investigators modeled preeclampsia and chorioamnionitis, the two major antenatal determinants of BPD in infants. Further, their molecule of interest was targeted to a pathway that has been well-established to play a role in BPD pathogenesis. Therefore, targeting sFlt-1 has a high translational potential in infants with BPD and PH who have increased expression of sFlt-1.
Exosomes
Exosome, a subtype of extracellular vesicle, is a plasma membrane-enclosed signaling vector secreted via the endosomal pathway. Their enriched bioactive cargo, including small non-coding RNAs, free fatty acids, surface antigens, and protein, allows them to be one of the most effective mediators of cell signaling (106–108). The number and miRNA signatures of these exosomes are altered in BPD infants (109), indicating that these vesicles may play a pathogenic role and can be targeted to develop therapies. Two recent preclinical studies strongly suggest that exosomes can be an effective therapy for BPD infants with PH. In a murine model of hyperoxia-induced BPD and PH, Willis and colleagues (82) elegantly demonstrated that exosomes, purified from the mesenchymal stromal cells of both human bone marrow and umbilical cord Wharton's jelly, attenuated pulmonary vascular remodeling, PH, and lung fibrosis and improved lung development and function in mice exposed to hyperoxia. Similarly, Chaubey et al. (83) demonstrated that umbilical cord-derived exosomes ameliorate hyperoxia-induced lung inflammation, alveolar simplification, and PH in neonatal mice. Although recent advances in processes such as isolation, purification, and characterization of the exosomes have increased our understanding of these vesicles in health and disease, the advances are still at an infancy stage, and there is a need for improvement and standardization of these processes before we can definitely conclude on the detrimental and beneficial effects of exosomal therapy (110).
Interleukin-1 Receptor Antagonist
Interleukin-1 (IL-1) is a cytokine that is implicated in the patho genesis of many acute and chronic inflammatory diseases. Not surprisingly, elevated levels of this cytokine are associated with increased BPD incidence in infants (111–113). It is also one of the few cytokines that have been directly implicated in the pathogenesis of experimental BPD (114–118). Bui et al. (84) recently demonstrated for the first time that IL-1 receptor antagonist (IL-1Ra) decreases both the short- and long-term adverse effects of neonatal hyperoxia on pulmonary vasculature in mice. Using elegant and robust methods, they showed that IL-1Ra improves pulmonary vascular density and alveolarization and decreases pulmonary vascular resistance and cardiac fibrosis. These observations indicate that IL-1Ra attenuates murine BPD and PH. The antagonist was also recently shown to be safe and effective in adult patients with PH and right ventricular failure (119), emphasizing the translational potential of this compound for BPD infants with PH.
Microbiome
Dysbiosis, or a disruption in the balance between the structure of complex microbial communities on or inside the body, plays a major role in the pathogenesis of several inflammatory diseases (120). We now know that the human respiratory tract microbial colonization begins in utero (121, 122) or shortly after birth (123, 124). Chorioamnionitis, antibiotic exposure, mode of delivery, method of feeding, and bowel colonization can decrease bacterial diversity and increase pathogenic microbial colonization in the lungs (125), increasing the risk of lung inflammation and BPD. Two recent preclinical studies highlight the role of microbiota in the pathogenesis of BPD and PH. Postnatal growth restriction (PNGR) causes PH without disrupted lung development in neonatal rats (85). However, when these growth-restricted rats are exposed to hyperoxia, they also develop alveolar simplification and have a severe PH phenotype. Further, Wedgwood et al. showed that PNGR, but not hyperoxia, independently alters intestinal microbiota in the same model, and mitigation of this intestinal dysbiosis with a probiotic alleviates the PH in neonatal PNGR mice exposed to normoxia or hyperoxia (86). To elucidate the pathogenic role of microbiota in BPD, Dolma et al. (87) exposed germ-free (GF) and non-germ-free (NGF) mice to 21% FiO2 (normoxia) or 85% FiO2 (hyperoxia) for up to postnatal day 14, which is a well-established murine model of experimental BPD. At baseline, lung development was comparable between GF and NGF mice. However, hyperoxia-induced interruption in lung development was significantly worse in NGF than in GF mice, indicating that pathogenic bacteria can worsen experimental lung injury. Interestingly, the severity of PH was similar in hyperoxia-exposed GF and NGF mice, suggesting that microbiota may not play a major role in the pathogenesis of hyperoxia-induced PH. The above two studies indicate the need for further robust studies to identify the select population of BPD infants with PH who may benefit from therapies targeting the microbiota.
Stem Cells and Their Conditioned Media
There has been an increased focus on stem cell biology for discovering therapies for BPD and PH because the very biological effects of stem cells such as self-renewal and differentiation into specialized cell types (126) make them ideal candidates to promote organ development and repair. Further, stem cell biologists have focused mainly on and used mesenchymal stem cells (MSCs) in experimental lung injury due to reasons such as the feasibility of isolation and the pleiotropic effects of these cells (127). Other types of stem cells used for intervention in experimental BPD include endothelial colony forming cells, endothelial progenitor cells, bone marrow-derived angiogenic cells, mononuclear and cord blood CD34+ cells, human amniotic fluid stem and epithelial cells, bone marrow derived ckit+ cells, and human-induced pluripotent stem cells (128). The major source of MSCs used in experimental models of BPD has been the bone marrow, while the predominant route of administration of the cells in these models has been the intratracheal route (88). Systematic analyses of 25 preclinical studies on MSC therapy in BPD (88) indicated that MSCs improved lung alveolarization regardless of the source and route of administration. The cell therapy also decreased lung inflammation, apoptosis, fibrosis, pulmonary vascular remodeling, and pulmonary hypertension and improved lung function (compliance, elastance, total lung capacity, and inspiratory capacity). Therapy with the conditioned media from these MSCs was also effective in improving alveolarization and lung vascularization and in reducing lung inflammation and fibrosis. Interestingly, the systematic review indicated that, unlike MSCs, their conditioned media do not improve the PH (88). A similar conclusion was drawn from another systematic review of preclinical studies that used MSC-conditioned media for various lung diseases (129). Only two studies (89, 90) on experimental BPD met the criteria for inclusion in these analyses. The anti-inflammatory effects of the conditioned media were comparable to MSCs. Further, the conditioned media also improved alveolarization. Recently, Moreira et al. for the first time, demonstrated the safety and efficacy of intranasal human umbilical cord-derived MSCs in experimental BPD (91). The first trial of stem cell therapy for BPD infants was conducted by Lim et al. from Australia. These investigators demonstrated that allogenic amniotic cells could safely be administered intravenously to preterm infants with BPD (n = 6) (92). A follow-up of these infants (n = 5) for 2 years indicated that there were no long-term adverse consequences of amniotic cell therapy (130). Similarly, Powell et al. showed that MSCs from human umbilical cord blood were well-tolerated without any adverse effects in extremely low-birth-weight infants (n = 12) when administered by the intratracheal route (131). Based on all these studies and systematic reviews, MSCs or their conditioned media are excellent therapeutic candidates for BPD infants.
Stromal-Derived Factor-1
Stromal-derived factor-1 (SDF-1) is a chemokine that signals through the receptors, chemokine receptors 4 and 7, and modulates cell migration, proliferation, and angiogenesis, the biological processes that are crucial for organ development and repair (132–137). In neonatal rats, Guerra et al. (93) recently showed that SDF-1 is expressed in both lung epithelial and endothelial cells, and hyperoxia decreases the expression of this chemokine. Further, using a non-viral form of gene therapy, they elegantly demonstrated that SDF-1 delivery to the lungs improves angiogenesis and alveolarization, decreases pulmonary vascular remodeling and inflammation, and attenuates experimental BPD and PH induced by hyperoxia. Importantly, this non-viral form of gene therapy is proven to be safe and effective in humans (138) and, therefore, has a high translational potential to target therapies to the diseased lung while avoiding off-target effects.
Conclusions
BPD with PH remains a significant short- and long-term morbidity of preterm infants that lacks specific therapies. Robust mechanistic preclinical studies done in the past two decades have identified several therapeutic targets and interventions that have a high translational potential to meaningfully manage infants with this disabling disease complex. Most of these are rodent studies, and their strengths include: 1) a comparable lung developmental stage and disease phenotype with preterm infants; 2) a reproducible disease phenotype with exposure to well-known insults of lung injury; 3) conducive to establishing a direct cause–effect relationship because of the feasibility of gene manipulation in these animals; 4) feasibility of obtaining a sufficient number of animals to test the hypothesis accurately; 5) timeliness with rapid turnover; 6) ease of animal husbandry; and 7) cost-effectiveness. However, there are certain disadvantages with such studies. For instance, despite the anatomic similarities between the murine lungs and preterm human lungs, there are functional differences. Murine lungs are surfactant-sufficient and do not have respiratory disease at basal conditions, whereas preterm infants are surfactant-deficient and have respiratory failure at birth. Further, the smaller size of the rodents poses technical challenges and precludes one from simulating an identical clinical scenario of a preterm infant who is at high risk of developing BPD and PH. For example, it is practically impossible to deliver positive pressure ventilation through continuous positive airway pressure (NCPAP) and mechanical ventilation or to administer intravenous parental nutrition to these small animals. Therefore, there is a need to validate these interventions and therapies in large animals such as lambs and non-human primates before designing clinical trials. The larger body and organ dimensions of these animals make it relatively easy to subject them to all the interventions of a preterm infant and accurately model human BPD and PH.
Author Contributions
AE-S and BS participated in the conception and design, performed the literature search, analyzed and interpreted the data, drafted, revised, and approved the final version of the submitted manuscript.
Funding
This work was supported by the National Institutes of Health grants HL139594 to BS.
Conflict of Interest
The authors declare that the research was conducted in the absence of any commercial or financial relationships that could be construed as a potential conflict of interest.
Abbreviations
BPD, bronchopulmonary dysplasia; BNP, brain natriuretic peptide; CTGF, connective tissue growth factor; GA, gestational age; GF, germ-free; IL, interleukin; iNO, inhaled nitric oxide; LV, left ventricle; MSCs, mesenchymal stem cells; NGF, non-germ-free; PAAT, pulmonary artery acceleration time; PH, pulmonary hypertension; PNGR, postnatal growth restriction; PVR, pulmonary vascular resistance; PVS, pulmonary vein stenosis; RV, right ventricle; SDF-1, stromal-derived factor-1; sFlt-1, soluble fms-like tyrosine kinase 1; TAPSE, tricuspid annular plane systolic excursion; TRJV, tricuspid regurgitation jet velocity; VEGF, vascular endothelial growth factor
References
1. Husain AN, Siddiqui NH, Stocker JT. Pathology of arrested acinar development in postsurfactant bronchopulmonary dysplasia. Hum Pathol. (1998) 29:710–7. doi: 10.1016/S0046-8177(98)90280-5
2. Coalson JJ. Pathology of new bronchopulmonary dysplasia. Semin Neonatol. (2003) 8:73–81. doi: 10.1016/S1084-2756(02)00193-8
3. Shennan AT, Dunn MS, Ohlsson A, Lennox K, Hoskins EM. Abnormal pulmonary outcomes in premature infants: prediction from oxygen requirement in the neonatal period. Pediatrics. (1988) 82:527–32.
4. Jobe AH, Bancalari E. Bronchopulmonary dysplasia. Am J Respir Crit Care Med. (2001) 163:1723–9. doi: 10.1164/ajrccm.163.7.2011060
5. Walsh MC, Wilson-Costello D, Zadell A, Newman N, Fanaroff A. Safety, reliability, and validity of a physiologic definition of bronchopulmonary dysplasia. J Perinatol. (2003) 23:451–6. doi: 10.1038/sj.jp.7210963
6. Poindexter BB, Feng R, Schmidt B, Aschner JL, Ballard RA, Hamvas A, et al. Comparisons and limitations of current definitions of bronchopulmonary dysplasia for the prematurity and respiratory outcomes program. Ann Am Thorac Soc. (2015) 12:1822–30. doi: 10.1513/AnnalsATS.201504-218OC
7. Abman SH, Collaco JM, Shepherd EG, Keszler M, Cuevas-Guaman M, Welty SE, et al. Interdisciplinary care of children with severe bronchopulmonary dysplasia. J Pediatr. (2017) 181:12–28 e11. doi: 10.1016/j.jpeds.2016.10.082
8. Hines D, Modi N, Lee SK, Isayama T, Sjors G, Gagliardi L, et al. Scoping review shows wide variation in the definitions of bronchopulmonary dysplasia in preterm infants and calls for a consensus. Acta Paediatr. (2017) 106:366–74. doi: 10.1111/apa.13672
9. Higgins RD, Jobe AH, Koso-Thomas M, Bancalari E, Viscardi RM, Hartert TV, et al. Bronchopulmonary dysplasia: executive summary of a workshop. J Pediatr. (2018) 197:300–8. doi: 10.1016/j.jpeds.2018.01.043
10. Jensen EA, Dysart K, Gantz MG, Mcdonald S, Bamat NA, Keszler M, et al. The diagnosis of bronchopulmonary dysplasia in very preterm infants. An evidence-based approach. Am J Respir Crit Care Med. (2019) 200:751–9. doi: 10.1164/rccm.201812-2348OC
11. Day CL, Ryan RM. Bronchopulmonary dysplasia: new becomes old again! Pediatr Res. (2017) 81:210–3. doi: 10.1038/pr.2016.201
12. Lui K, Lee SK, Kusuda S, Adams M, Vento M, Reichman B, et al. Trends in outcomes for neonates born very preterm and very low birth weight in 11 high-income countries. J Pediatr. (2019) 215:32–40 e14. doi: 10.1016/j.jpeds.2019.08.020
13. Starr MC, Boohaker L, Eldredge LC, Menon S, Griffin R, Mayock DE, et al. Acute kidney injury and bronchopulmonary dysplasia in premature neonates born less than 32 weeks' gestation. Am J Perinatol. (2019) 37:341–8. doi: 10.1055/s-0039-3400311
14. Mcevoy CT, Jain L, Schmidt B, Abman S, Bancalari E, Aschner JL. Bronchopulmonary dysplasia: NHLBI workshop on the primary prevention of chronic lung diseases. Ann Am Thorac Soc. (2014) 11(Suppl.3):S146–53. doi: 10.1513/AnnalsATS.201312-424LD
15. Principi N, Di Pietro GM, Esposito S. Bronchopulmonary dysplasia: clinical aspects and preventive and therapeutic strategies. J Transl Med. (2018) 16:36. doi: 10.1186/s12967-018-1417-7
16. Lapcharoensap W, Bennett MV, Xu X, Lee HC, Dukhovny D. Hospitalization costs associated with bronchopulmonary dysplasia in the first year of life. J Perinatol. (2019). doi: 10.1038/s41372-019-0548-x
17. Islam JY, Keller RL, Aschner JL, Hartert TV, Moore PE. Understanding the short- and long-term respiratory outcomes of prematurity and bronchopulmonary dysplasia. Am J Respir Crit Care Med. (2015) 192:134–56. doi: 10.1164/rccm.201412-2142PP
18. Simpson SJ, Hall GL, Wilson AC. Lung function following very preterm birth in the era of “new” bronchopulmonary dysplasia. Respirology. (2015) 20:535–40. doi: 10.1111/resp.12503
19. Gou X, Yang L, Pan L, Xiao D. Association between bronchopulmonary dysplasia and cerebral palsy in children: a meta-analysis. BMJ Open. (2018) 8:e020735. doi: 10.1136/bmjopen-2017-020735
20. Simpson SJ, Turkovic L, Wilson AC, Verheggen M, Logie KM, Pillow JJ, et al. Lung function trajectories throughout childhood in survivors of very preterm birth: a longitudinal cohort study. Lancet Child Adolesc Health. (2018) 2:350–9. doi: 10.1016/S2352-4642(18)30064-6
21. Twilhaar ES, Wade RM, De Kieviet JF, Van Goudoever JB, Van Elburg RM, Oosterlaan J. Cognitive outcomes of children born extremely or very preterm since the 1990s and associated risk factors: a meta-analysis and meta-regression. JAMA Pediatr. (2018) 172:361–7. doi: 10.1001/jamapediatrics.2017.5323
22. Arjaans S, Zwart EaH, Ploegstra MJ, Bos AF, Kooi EMW, et al. Identification of gaps in the current knowledge on pulmonary hypertension in extremely preterm infants: a systematic review and meta-analysis. Paediatr Perinat Epidemiol. (2018) 32:258–67. doi: 10.1111/ppe.12444
23. Slaughter JL, Pakrashi T, Jones DE, South AP, Shah TA. Echocardiographic detection of pulmonary hypertension in extremely low birth weight infants with bronchopulmonary dysplasia requiring prolonged positive pressure ventilation. J Perinatol. (2011) 31:635–40. doi: 10.1038/jp.2010.213
24. Nakanishi H, Uchiyama A, Kusuda S. Impact of pulmonary hypertension on neurodevelopmental outcome in preterm infants with bronchopulmonary dysplasia: a cohort study. J Perinatol. (2016) 36:890–6. doi: 10.1038/jp.2016.108
25. Berkelhamer SK, Mestan KK, Steinhorn R. An update on the diagnosis and management of bronchopulmonary dysplasia (BPD)-associated pulmonary hypertension. Semin Perinatol. (2018) 42:432–43. doi: 10.1053/j.semperi.2018.09.005
26. Critser PJ, Higano NS, Tkach JA, Olson ES, Spielberg DR, Kingma PS, et al. Cardiac MRI evaluation of neonatal bronchopulmonary dysplasia associated pulmonary hypertension. Am J Respir Crit Care Med. (2020) 201:73–82. doi: 10.1164/rccm.201904-0826OC
27. Mourani PM, Abman SH. Pulmonary hypertension and vascular abnormalities in bronchopulmonary dysplasia. Clin Perinatol. (2015) 42:839–55. doi: 10.1016/j.clp.2015.08.010
28. Mestan KK, Check J, Minturn L, Yallapragada S, Farrow KN, Liu X, et al. Placental pathologic changes of maternal vascular underperfusion in bronchopulmonary dysplasia and pulmonary hypertension. Placenta. (2014) 35:570–4. doi: 10.1016/j.placenta.2014.05.003
29. Yallapragada SG, Mestan KK, Palac H, Porta N, Gotteiner N, Hamvas A, et al. Placental villous vascularity is decreased in premature infants with bronchopulmonary dysplasia-associated pulmonary hypertension. Pediatr Dev Pathol. (2016) 19:101–7. doi: 10.2350/15-05-1646-OA.1
30. Su EJ, Xin H, Yin P, Dyson M, Coon J, Farrow KN, et al. Impaired fetoplacental angiogenesis in growth-restricted fetuses with abnormal umbilical artery doppler velocimetry is mediated by aryl hydrocarbon receptor nuclear translocator (ARNT). J Clin Endocrinol Metab. (2015) 100:E30–40. doi: 10.1210/jc.2014-2385
31. Korzeniewski SJ, Romero R, Chaiworapongsa T, Chaemsaithong P, Kim CJ, Kim YM, et al. Maternal plasma angiogenic index-1 (placental growth factor/soluble vascular endothelial growth factor receptor-1) is a biomarker for the burden of placental lesions consistent with uteroplacental underperfusion: a longitudinal case-cohort study. Am J Obstet Gynecol. (2016) 214:629 e621–9 e617. doi: 10.1016/j.ajog.2015.11.015
32. Mestan KK, Gotteiner N, Porta N, Grobman W, Su EJ, Ernst LM. Cord blood biomarkers of placental maternal vascular underperfusion predict bronchopulmonary dysplasia-associated pulmonary hypertension. J Pediatr. (2017) 185:33–41. doi: 10.1016/j.jpeds.2017.01.015
33. Abman SH. Bronchopulmonary dysplasia: “a vascular hypothesis.” Am J Respir Crit Care Med. (2001) 164:1755–6. doi: 10.1164/ajrccm.164.10.2109111c
34. Check J, Gotteiner N, Liu X, Su E, Porta N, Steinhorn R, et al. Fetal growth restriction and pulmonary hypertension in premature infants with bronchopulmonary dysplasia. J Perinatol. (2013) 33:553–7. doi: 10.1038/jp.2012.164
35. Kim DH, Kim HS, Choi CW, Kim EK, Kim BI, Choi JH. Risk factors for pulmonary artery hypertension in preterm infants with moderate or severe bronchopulmonary dysplasia. Neonatology. (2012) 101:40–6. doi: 10.1159/000327891
36. Trittmann JK, Nelin LD, Zmuda EJ, Gastier-Foster JM, Chen B, Backes CH, et al. Arginase I gene single-nucleotide polymorphism is associated with decreased risk of pulmonary hypertension in bronchopulmonary dysplasia. Acta Paediatr. (2014) 103:e439–443. doi: 10.1111/apa.12717
37. Trittmann JK, Peterson E, Rogers LK, Chen B, Backes CH, Klebanoff MA, et al. Plasma asymmetric dimethylarginine levels are increased in neonates with bronchopulmonary dysplasia-associated pulmonary hypertension. J Pediatr. (2015) 166:230–3. doi: 10.1016/j.jpeds.2014.09.004
38. Trittmann JK, Gastier-Foster JM, Zmuda EJ, Frick J, Rogers LK, Vieland VJ, et al. A single nucleotide polymorphism in the dimethylarginine dimethylaminohydrolase gene is associated with lower risk of pulmonary hypertension in bronchopulmonary dysplasia. Acta Paediatr. (2016) 105:e170–175. doi: 10.1111/apa.13296
39. Trittmann JK, Bartenschlag A, Zmuda EJ, Frick J, Stewart WCL, Nelin LD. Using clinical and genetic data to predict pulmonary hypertension in bronchopulmonary dysplasia. Acta Paediatr. (2018) 107:2158–64. doi: 10.1111/apa.14600
40. Chen LL, Zmuda EJ, Talavera MM, Frick J, Brock GN, Liu Y, et al. Dual-specificity phosphatase (DUSP) genetic variants predict pulmonary hypertension in patients with bronchopulmonary dysplasia. Pediatr Res. (2019) 87:81–7. doi: 10.1038/s41390-019-0502-9
41. Guler E, Narin N, Pamukcu O, Taheri S, Tufan E, Guler Y, et al. Can microsomal RNA be a biomarker in pulmonary hypertension secondary to bronchopulmonary dysplasia? J Matern Fetal Neonatal Med. (2019) 7:1–6. doi: 10.1080/14767058.2019.1638107
42. Sheth S, Goto L, Bhandari V, Abraham B, Mowes A. Factors associated with development of early and late pulmonary hypertension in preterm infants with bronchopulmonary dysplasia. J Perinatol. (2019) 40:138–48. doi: 10.1038/s41372-019-0549-9
43. Baker CD, Abman SH, Mourani PM. Pulmonary hypertension in preterm infants with bronchopulmonary dysplasia. Pediatr Allergy Immunol Pulmonol. (2014) 27:8–16. doi: 10.1089/ped.2013.0323
44. Altit G, Dancea A, Renaud C, Perreault T, Lands LC, Sant'anna G. Pathophysiology, screening and diagnosis of pulmonary hypertension in infants with bronchopulmonary dysplasia–a review of the literature. Paediatr Respir Rev. (2017) 23:16–26. doi: 10.1016/j.prrv.2016.11.002
45. Krishnan U, Feinstein JA, Adatia I, Austin ED, Mullen MP, Hopper RK, et al. Evaluation and management of pulmonary hypertension in children with bronchopulmonary dysplasia. J Pediatr. (2017) 188:24–34 e21. doi: 10.1016/j.jpeds.2017.05.029
46. Vayalthrikkovil S, Vorhies E, Stritzke A, Bashir RA, Mohammad K, Kamaluddeen M, et al. Prospective study of pulmonary hypertension in preterm infants with bronchopulmonary dysplasia. Pediatr Pulmonol. (2019) 54:171–8. doi: 10.1002/ppul.24211
47. Berger M, Haimowitz A, Van Tosh A, Berdoff RL, Goldberg E. Quantitative assessment of pulmonary hypertension in patients with tricuspid regurgitation using continuous wave Doppler ultrasound. J Am Coll Cardiol. (1985) 6:359–65. doi: 10.1016/S0735-1097(85)80172-8
48. Mourani PM, Sontag MK, Younoszai A, Ivy DD, Abman SH. Clinical utility of echocardiography for the diagnosis and management of pulmonary vascular disease in young children with chronic lung disease. Pediatrics. (2008) 121:317–25. doi: 10.1542/peds.2007-1583
49. Mccrary AW, Malowitz JR, Hornick CP, Hill KD, Cotten CM, Tatum GH, et al. Differences in eccentricity index and systolic-diastolic ratio in extremely low-birth-weight infants with bronchopulmonary dysplasia at risk of pulmonary hypertension. Am J Perinatol. (2016) 33:57–62. doi: 10.1055/s-0035-1556757
50. Zakaria D, Sachdeva R, Gossett JM, Tang X, O'connor MJ. Tricuspid annular plane systolic excursion is reduced in infants with pulmonary hypertension. Echocardiography. (2015) 32:834–8. doi: 10.1111/echo.12797
51. Koestenberger M, Nagel B, Ravekes W, Urlesberger B, Raith W, Avian A, et al. Systolic right ventricular function in preterm and term neonates: reference values of the tricuspid annular plane systolic excursion (TAPSE) in 258 patients and calculation of Z-score values. Neonatology. (2011) 100:85–92. doi: 10.1159/000322006
52. Seo YH, Choi HJ. Clinical utility of echocardiography for early and late pulmonary hypertension in preterm infants: relation with bronchopulmonary dysplasia. J Cardiovasc Ultrasound. (2017) 25:124–30. doi: 10.4250/jcu.2017.25.4.124
53. Altit G, Bhombal S, Feinstein J, Hopper RK, Tacy TA. Diminished right ventricular function at diagnosis of pulmonary hypertension is associated with mortality in bronchopulmonary dysplasia. Pulm Circ. (2019) 9:2045894019878598. doi: 10.1177/2045894019878598
54. Levy PT, Patel MD, Groh G, Choudhry S, Murphy J, Holland MR, et al. Pulmonary artery acceleration time provides a reliable estimate of invasive pulmonary hemodynamics in children. J Am Soc Echocardiogr. (2016) 29:1056–65. doi: 10.1016/j.echo.2016.08.013
55. Gill AB, Weindling AM. Pulmonary artery pressure changes in the very low birthweight infant developing chronic lung disease. Arch Dis Child. (1993) 68:303–7. doi: 10.1136/adc.68.3_Spec_No.303
56. Patel MD, Breatnach CR, James AT, Choudhry S, Mcnamara PJ, Jain A, et al. Echocardiographic assessment of right ventricular afterload in preterm infants: maturational patterns of pulmonary artery acceleration time over the first year of age and implications for pulmonary hypertension. J Am Soc Echocardiogr. (2019) 32:884–94 e884. doi: 10.1016/j.echo.2019.03.015
57. Singh GK, Levy PT, Holland MR, Hamvas A. Novel methods for assessment of right heart structure and function in pulmonary hypertension. Clin Perinatol. (2012) 39:685–701. doi: 10.1016/j.clp.2012.06.002
58. Jamal F, Bergerot C, Argaud L, Loufouat J, Ovize M. Longitudinal strain quantitates regional right ventricular contractile function. Am J Physiol Heart Circ Physiol. (2003) 285:H2842–7. doi: 10.1152/ajpheart.00218.2003
59. Kwon HW, Kim HS, An HS, Kwon BS, Kim GB, Shin SH, et al. Long-Term outcomes of pulmonary hypertension in preterm infants with bronchopulmonary dysplasia. Neonatology. (2016) 110:181–9. doi: 10.1159/000445476
60. Drossner DM, Kim DW, Maher KO, Mahle WT. Pulmonary vein stenosis: prematurity and associated conditions. Pediatrics. (2008) 122:e656–61. doi: 10.1542/peds.2008-0075
61. Del Cerro MJ, Sabate Rotes A, Carton A, Deiros L, Bret M, Cordeiro M, et al. Pulmonary hypertension in bronchopulmonary dysplasia: clinical findings, cardiovascular anomalies and outcomes. Pediatr Pulmonol. (2014) 49:49–59. doi: 10.1002/ppul.22797
62. Heching HJ, Turner M, Farkouh-Karoleski C, Krishnan U. Pulmonary vein stenosis and necrotising enterocolitis: is there a possible link with necrotising enterocolitis? Arch Dis Child Fetal Neonatal Ed. (2014) 99:F282–5. doi: 10.1136/archdischild-2013-304740
63. Mahgoub L, Kaddoura T, Kameny AR, Lopez Ortego P, Vanderlaan RD, Kakadekar A, et al. Pulmonary vein stenosis of ex-premature infants with pulmonary hypertension and bronchopulmonary dysplasia, epidemiology, and survival from a multicenter cohort. Pediatr Pulmonol. (2017) 52:1063–70. doi: 10.1002/ppul.23679
64. Walkup LL, Woods JC. Newer imaging techniques for bronchopulmonary dysplasia. Clin Perinatol. (2015) 42:871–87. doi: 10.1016/j.clp.2015.08.012
65. Marrone G, Mamone G, Luca A, Vitulo P, Bertani A, Pilato M, et al. The role of 1.5T cardiac MRI in the diagnosis, prognosis and management of pulmonary arterial hypertension. Int J Cardiovasc Imaging. (2010) 26:665–81. doi: 10.1007/s10554-010-9623-2
66. Warwick G, Thomas PS, Yates DH. Biomarkers in pulmonary hypertension. Eur Respir J. (2008) 32:503–12. doi: 10.1183/09031936.00160307
67. Kim GB. Pulmonary hypertension in infants with bronchopulmonary dysplasia. Korean J Pediatr. (2010) 53:688–93. doi: 10.3345/kjp.2010.53.6.688
68. Berkelhamer SK, Mestan KK, Steinhorn RH. Pulmonary hypertension in bronchopulmonary dysplasia. Semin Perinatol. (2013) 37:124–31. doi: 10.1053/j.semperi.2013.01.009
69. Montgomery AM, Bazzy-Asaad A, Asnes JD, Bizzarro MJ, Ehrenkranz RA, Weismann CG. Biochemical screening for pulmonary hypertension in preterm infants with bronchopulmonary dysplasia. Neonatology. (2016) 109:190–4. doi: 10.1159/000442043
70. Kim DH, Kim HS. Serial changes of serum endostatin and angiopoietin-1 levels in preterm infants with severe bronchopulmonary dysplasia and subsequent pulmonary artery hypertension. Neonatology. (2014) 106:55–61. doi: 10.1159/000358374
71. Choi EK, Shin SH, Kim EK, Kim HS. Developmental outcomes of preterm infants with bronchopulmonary dysplasia-associated pulmonary hypertension at 18–24 months of corrected age. BMC Pediatr. (2019) 19:26. doi: 10.1186/s12887-019-1400-3
72. Abman SH, Hansmann G, Archer SL, Ivy DD, Adatia I, Chung WK, et al. Pediatric pulmonary hypertension: guidelines from the American Heart Association and American Thoracic Society. Circulation. (2015) 132:2037–99. doi: 10.1161/CIR.0000000000000329
73. Hansmann G, Koestenberger M, Alastalo TP, Apitz C, Austin ED, Bonnet D, et al. 2019 updated consensus statement on the diagnosis and treatment of pediatric pulmonary hypertension: the European Pediatric Pulmonary Vascular Disease Network (EPPVDN), endorsed by AEPC, ESPR and ISHLT. J Heart Lung Transplant. (2019) 38:879–901. doi: 10.1016/j.healun.2019.06.022
74. Philips JB 3rd. Past, current and future perspectives of the use of inhaled NO as a primary therapeutic for the newborn. Nitric Oxide. (2020) 94:92–4. doi: 10.1016/j.niox.2019.11.001
75. Banks BA, Seri I, Ischiropoulos H, Merrill J, Rychik J, Ballard RA. Changes in oxygenation with inhaled nitric oxide in severe bronchopulmonary dysplasia. Pediatrics. (1999) 103:610–8. doi: 10.1542/peds.103.3.610
76. Mourani PM, Ivy DD, Gao D, Abman SH. Pulmonary vascular effects of inhaled nitric oxide and oxygen tension in bronchopulmonary dysplasia. Am J Respir Crit Care Med. (2004) 170:1006–13. doi: 10.1164/rccm.200310-1483OC
77. Alapati D, Rong M, Chen S, Hehre D, Rodriguez MM, Lipson KE, et al. Connective tissue growth factor antibody therapy attenuates hyperoxia-induced lung injury in neonatal rats. Am J Respir Cell Mol Biol. (2011) 45:1169–77. doi: 10.1165/rcmb.2011-0023OC
78. Wu S, Platteau A, Chen S, Mcnamara G, Whitsett J, Bancalari E. Conditional overexpression of connective tissue growth factor disrupts postnatal lung development. Am J Respir Cell Mol Biol. (2010) 42:552–63. doi: 10.1165/rcmb.2009-0068OC
79. Chen S, Rong M, Platteau A, Hehre D, Smith H, Ruiz P, et al. CTGF disrupts alveolarization and induces pulmonary hypertension in neonatal mice: implication in the pathogenesis of severe bronchopulmonary dysplasia. Am J Physiol Lung Cell Mol Physiol. (2011) 300:L330–40. doi: 10.1152/ajplung.00270.2010
80. Rong M, Chen S, Zambrano R, Duncan MR, Grotendorst G, Wu S. Inhibition of beta-catenin signaling protects against CTGF-induced alveolar and vascular pathology in neonatal mouse lung. Pediatr Res. (2016) 80:136–44. doi: 10.1038/pr.2016.52
81. Wallace B, Peisl A, Seedorf G, Nowlin T, Kim C, Bosco J, et al. Anti-sFlt-1 therapy preserves lung alveolar and vascular growth in antenatal models of bronchopulmonary dysplasia. Am J Respir Crit Care Med. (2018) 197:776–87. doi: 10.1164/rccm.201707-1371OC
82. Willis GR, Fernandez-Gonzalez A, Anastas J, Vitali SH, Liu X, Ericsson M, et al. Mesenchymal stromal cell exosomes ameliorate experimental bronchopulmonary dysplasia and restore lung function through macrophage immunomodulation. Am J Respir Crit Care Med. (2018) 197:104–16. doi: 10.1164/rccm.201705-0925OC
83. Chaubey S, Thueson S, Ponnalagu D, Alam MA, Gheorghe CP, Aghai Z, et al. Early gestational mesenchymal stem cell secretome attenuates experimental bronchopulmonary dysplasia in part via exosome-associated factor TSG-6. Stem Cell Res Ther. (2018) 9:173. doi: 10.1186/s13287-018-0903-4
84. Bui CB, Kolodziej M, Lamanna E, Elgass K, Sehgal A, Rudloff I, et al. Interleukin-1 receptor antagonist protects newborn mice against pulmonary hypertension. Front Immunol. (2019) 10:1480. doi: 10.3389/fimmu.2019.01480
85. Wedgwood S, Warford C, Agvateesiri SC, Thai P, Berkelhamer SK, Perez M, et al. Postnatal growth restriction augments oxygen-induced pulmonary hypertension in a neonatal rat model of bronchopulmonary dysplasia. Pediatr Res. (2016) 80:894–902. doi: 10.1038/pr.2016.164
86. Wedgwood S, Warford C, Agvatisiri SR, Thai PN, Chiamvimonvat N, Kalanetra KM, et al. The developing gut-lung axis: postnatal growth restriction, intestinal dysbiosis, and pulmonary hypertension in a rodent model. Pediatr Res. (2019) 87:472–9. doi: 10.1038/s41390-019-0578-2
87. Dolma K, Freeman AE, Rezonzew G, Payne GA, Xu X, Jilling T, et al. Effects of hyperoxia on alveolar and pulmonary vascular development in germ free mice. Am J Physiol Lung Cell Mol Physiol. (2019) 318:L421–8. doi: 10.1152/ajplung.00316.2019
88. Augustine S, Avey MT, Harrison B, Locke T, Ghannad M, Moher D, et al. Mesenchymal stromal cell therapy in bronchopulmonary dysplasia: systematic review and meta-analysis of preclinical studies. Stem Cells Transl Med. (2017) 6:2079–93. doi: 10.1002/sctm.17-0126
89. Aslam M, Baveja R, Liang OD, Fernandez-Gonzalez A, Lee C, Mitsialis SA, et al. Bone marrow stromal cells attenuate lung injury in a murine model of neonatal chronic lung disease. Am J Respir Crit Care Med. (2009) 180:1122–30. doi: 10.1164/rccm.200902-0242OC
90. Sutsko RP, Young KC, Ribeiro A, Torres E, Rodriguez M, Hehre D, et al. Long-term reparative effects of mesenchymal stem cell therapy following neonatal hyperoxia-induced lung injury. Pediatr Res. (2013) 73:46–53. doi: 10.1038/pr.2012.152
91. Moreira A, Winter C, Joy J, Winter L, Jones M, Noronha M, et al. Intranasal delivery of human umbilical cord Wharton's jelly mesenchymal stromal cells restores lung alveolarization and vascularization in experimental bronchopulmonary dysplasia. Stem Cells Transl Med. (2019) 9:221–34. doi: 10.1002/sctm.18-0273
92. Lim R, Malhotra A, Tan J, Chan ST, Lau S, Zhu D, et al. First-in-human administration of allogeneic amnion cells in premature infants with bronchopulmonary dysplasia: a safety study. Stem Cells Transl Med. (2018) 7:628–35. doi: 10.1002/sctm.18-0079
93. Guerra K, Bryan C, Dapaah-Siakwan F, Sammour I, Drummond S, Zambrano R, et al. Intra-tracheal administration of a naked plasmid expressing stromal derived factor-1 improves lung structure in rodents with experimental bronchopulmonary dysplasia. Respir Res. (2019) 20:255. doi: 10.1186/s12931-019-1224-6
94. Ivkovic S, Yoon BS, Popoff SN, Safadi FF, Libuda DE, Stephenson RC, et al. Connective tissue growth factor coordinates chondrogenesis and angiogenesis during skeletal development. Development. (2003) 130:2779–91. doi: 10.1242/dev.00505
95. Aguiar DP, De Farias GC, De Sousa EB, De Mattos Coelho-Aguiar J, Lobo JC, Casado PL, et al. New strategy to control cell migration and metastasis regulated by CCN2/CTGF. Cancer Cell Int. (2014) 14:61. doi: 10.1186/1475-2867-14-61
96. Cheong ML, Lai TH, Wu WB. Connective tissue growth factor mediates transforming growth factor beta-induced collagen expression in human endometrial stromal cells. PLoS ONE. (2019) 14:e0210765. doi: 10.1371/journal.pone.0210765
97. Wang X, Cui H, Wu S. CTGF: a potential therapeutic target for Bronchopulmonary dysplasia. Eur J Pharmacol. (2019) 860:172588. doi: 10.1016/j.ejphar.2019.172588
98. Lipson KE, Wong C, Teng Y, Spong S. CTGF is a central mediator of tissue remodeling and fibrosis and its inhibition can reverse the process of fibrosis. Fibrogenesis Tissue Repair. (2012) 5:S24. doi: 10.1186/1755-1536-5-S1-S24
99. Yang Z, Sun Z, Liu H, Ren Y, Shao D, Zhang W, et al. Connective tissue growth factor stimulates the proliferation, migration and differentiation of lung fibroblasts during paraquat-induced pulmonary fibrosis. Mol Med Rep. (2015) 12:1091–7. doi: 10.3892/mmr.2015.3537
100. Chen CM, Wang LF, Chou HC, Lang YD, Lai YP. Up-regulation of connective tissue growth factor in hyperoxia-induced lung fibrosis. Pediatr Res. (2007) 62:128–33. doi: 10.1203/PDR.0b013e3180987202
101. Wu S, Capasso L, Lessa A, Peng J, Kasisomayajula K, Rodriguez M, et al. High tidal volume ventilation activates Smad2 and upregulates expression of connective tissue growth factor in newborn rat lung. Pediatr Res. (2008) 63:245–50. doi: 10.1203/PDR.0b013e318163a8cc
102. Kendall RL, Wang G, Thomas KA. Identification of a natural soluble form of the vascular endothelial growth factor receptor, FLT-1, and its heterodimerization with KDR. Biochem Biophys Res Commun. (1996) 226:324–8. doi: 10.1006/bbrc.1996.1355
103. Goldman CK, Kendall RL, Cabrera G, Soroceanu L, Heike Y, Gillespie GY, et al. Paracrine expression of a native soluble vascular endothelial growth factor receptor inhibits tumor growth, metastasis, and mortality rate. Proc Natl Acad Sci USA. (1998) 95:8795–800. doi: 10.1073/pnas.95.15.8795
104. Ye C, Feng C, Wang S, Wang KZ, Huang N, Liu X, et al. sFlt-1 gene therapy of follicular thyroid carcinoma. Endocrinology. (2004) 145:817–22. doi: 10.1210/en.2003-1106
105. Hasan J, Beharry KD, Valencia AM, Strauss A, Modanlou HD. Soluble vascular endothelial growth factor receptor 1 in tracheal aspirate fluid of preterm neonates at birth may be predictive of bronchopulmonary dysplasia/chronic lung disease. Pediatrics. (2009) 123:1541–7. doi: 10.1542/peds.2008-1670
106. Thery C. Exosomes: secreted vesicles and intercellular communications. F1000 Biol Rep. (2011) 3:15. doi: 10.3410/B3-15
107. Kowal J, Tkach M, Thery C. Biogenesis and secretion of exosomes. Curr Opin Cell Biol. (2014) 29:116–25. doi: 10.1016/j.ceb.2014.05.004
108. Van Der Pol E, Boing AN, Harrison P, Sturk A, Nieuwland R. Classification, functions, and clinical relevance of extracellular vesicles. Pharmacol Rev. (2012) 64:676–705. doi: 10.1124/pr.112.005983
109. Lal CV, Olave N, Travers C, Rezonzew G, Dolma K, Simpson A, et al. Exosomal microRNA predicts and protects against severe bronchopulmonary dysplasia in extremely premature infants. JCI Insight. (2018) 3:e93994. doi: 10.1172/jci.insight.93994
110. Willis GR, Fernandez-Gonzalez A, Reis M, Mitsialis SA, Kourembanas S. Macrophage immunomodulation: the gatekeeper for mesenchymal stem cell derived-exosomes in pulmonary arterial hypertension? Int J Mol Sci. (2018) 19:2534. doi: 10.3390/ijms19092534
111. Cayabyab RG, Jones CA, Kwong KY, Hendershott C, Lecart C, Minoo P, et al. Interleukin-1beta in the bronchoalveolar lavage fluid of premature neonates: a marker for maternal chorioamnionitis and predictor of adverse neonatal outcome. J Matern Fetal Neonatal Med. (2003) 14:205–11. doi: 10.1080/jmf.14.3.205.211
112. Bose CL, Dammann CE, Laughon MM. Bronchopulmonary dysplasia and inflammatory biomarkers in the premature neonate. Arch Dis Child Fetal Neonatal Ed. (2008) 93:F455–461. doi: 10.1136/adc.2007.121327
113. Ambalavanan N, Carlo WA, D'angio CT, Mcdonald SA, Das A, Schendel D, et al. Cytokines associated with bronchopulmonary dysplasia or death in extremely low birth weight infants. Pediatrics. (2009) 123:1132–41. doi: 10.1542/peds.2008-0526
114. Bry K, Whitsett JA, Lappalainen U. IL-1beta disrupts postnatal lung morphogenesis in the mouse. Am J Respir Cell Mol Biol. (2007) 36:32–42. doi: 10.1165/rcmb.2006-0116OC
115. Nold MF, Mangan NE, Rudloff I, Cho SX, Shariatian N, Samarasinghe TD, et al. Interleukin-1 receptor antagonist prevents murine bronchopulmonary dysplasia induced by perinatal inflammation and hyperoxia. Proc Natl Acad Sci USA. (2013) 110:14384–9. doi: 10.1073/pnas.1306859110
116. Liao J, Kapadia VS, Brown LS, Cheong N, Longoria C, Mija D, et al. The NLRP3 inflammasome is critically involved in the development of bronchopulmonary dysplasia. Nat Commun. (2015) 6:8977. doi: 10.1038/ncomms9977
117. Wang J, Bao L, Yu B, Liu Z, Han W, Deng C, et al. Interleukin-1beta promotes epithelial-derived alveolar elastogenesis via alphavbeta6 integrin-dependent TGF-beta activation. Cell Physiol Biochem. (2015) 36:2198–216. doi: 10.1159/000430185
118. Royce SG, Nold MF, Bui C, Donovan C, Lam M, Lamanna E, et al. Airway remodeling and hyperreactivity in a model of bronchopulmonary dysplasia and their modulation by IL-1 receptor antagonist. Am J Respir Cell Mol Biol. (2016) 55:858–68. doi: 10.1165/rcmb.2016-0031OC
119. Trankle CR, Canada JM, Kadariya D, Markley R, De Chazal HM, Pinson J, et al. IL-1 blockade reduces inflammation in pulmonary arterial hypertension and right ventricular failure: a single-arm, open-label, phase IB/II pilot study. Am J Respir Crit Care Med. (2019) 199:381–4. doi: 10.1164/rccm.201809-1631LE
120. Petersen C, Round JL. Defining dysbiosis and its influence on host immunity and disease. Cell Microbiol. (2014) 16:1024–33. doi: 10.1111/cmi.12308
121. Jimenez E, Fernandez L, Marin ML, Martin R, Odriozola JM, Nueno-Palop C, et al. Isolation of commensal bacteria from umbilical cord blood of healthy neonates born by cesarean section. Curr Microbiol. (2005) 51:270–4. doi: 10.1007/s00284-005-0020-3
122. Hansen R, Scott KP, Khan S, Martin JC, Berry SH, Stevenson M, et al. First-pass meconium samples from healthy term vaginally-delivered neonates: an analysis of the microbiota. PLoS ONE. (2015) 10:e0133320. doi: 10.1371/journal.pone.0133320
123. Lohmann P, Luna RA, Hollister EB, Devaraj S, Mistretta TA, Welty SE, et al. The airway microbiome of intubated premature infants: characteristics and changes that predict the development of bronchopulmonary dysplasia. Pediatr Res. (2014) 76:294–301. doi: 10.1038/pr.2014.85
124. Lal CV, Travers C, Aghai ZH, Eipers P, Jilling T, Halloran B, et al. The airway microbiome at birth. Sci Rep. (2016) 6:31023. doi: 10.1038/srep31023
125. Gallacher DJ, Kotecha S. Respiratory microbiome of new-born infants. Front Pediatr. (2016) 4:10. doi: 10.3389/fped.2016.00010
126. Slack JM. Origin of stem cells in organogenesis. Science. (2008) 322:1498–501. doi: 10.1126/science.1162782
127. Caplan AI, Correa D. The MSC: an injury drugstore. Cell Stem Cell. (2011) 9:11–5. doi: 10.1016/j.stem.2011.06.008
128. Augustine S, Cheng W, Avey MT, Chan ML, Lingappa SMC, Hutton B, et al. Are all stem cells equal? Systematic review, evidence map, and meta-analyses of preclinical stem cell-based therapies for bronchopulmonary dysplasia: concise review. Stem Cells Transl Med. (2019) 9:158–68. doi: 10.1002/sctm.19-0193
129. Emukah C, Dittmar E, Naqvi R, Martinez J, Corral A, Moreira A, et al. Mesenchymal stromal cell conditioned media for lung disease: a systematic review and meta-analysis of preclinical studies. Respir Res. (2019) 20:239. doi: 10.1186/s12931-019-1212-x
130. Malhotra A, Lim R, Mockler JC, Wallace EM. Two-year outcomes of infants enrolled in the first-in-human study of amnion cells for bronchopulmonary dysplasia. Stem Cells Transl Med. (2019) 9:289–94. doi: 10.1002/sctm.19-0251
131. Powell SB, Silvestri JM. Safety of intratracheal administration of human umbilical cord blood derived mesenchymal stromal cells in extremely low birth weight preterm infants. J Pediatr. (2019) 210:209–13 e202. doi: 10.1016/j.jpeds.2019.02.029
132. Askari AT, Unzek S, Popovic ZB, Goldman CK, Forudi F, Kiedrowski M, et al. Effect of stromal-cell-derived factor 1 on stem-cell homing and tissue regeneration in ischaemic cardiomyopathy. Lancet. (2003) 362:697–703. doi: 10.1016/S0140-6736(03)14232-8
133. Kucia M, Jankowski K, Reca R, Wysoczynski M, Bandura L, Allendorf DJ, et al. CXCR4-SDF-1 signalling, locomotion, chemotaxis and adhesion. J Mol Histol. (2004) 35:233–45. doi: 10.1023/B:HIJO.0000032355.66152.b8
134. Mavier P, Martin N, Couchie D, Preaux AM, Laperche Y, Zafrani ES. Expression of stromal cell-derived factor-1 and of its receptor CXCR4 in liver regeneration from oval cells in rat. Am J Pathol. (2004) 165:1969–77. doi: 10.1016/S0002-9440(10)63248-8
135. Chen WC, Tzeng YS, Li H, Tien WS, Tsai YC. Lung defects in neonatal and adult stromal-derived factor-1 conditional knockout mice. Cell Tissue Res. (2010) 342:75–85. doi: 10.1007/s00441-010-1035-z
136. Hunger C, Odemis V, Engele J. Expression and function of the SDF-1 chemokine receptors CXCR4 and CXCR7 during mouse limb muscle development and regeneration. Exp Cell Res. (2012) 318:2178–90. doi: 10.1016/j.yexcr.2012.06.020
137. Oliver JA, Maarouf O, Cheema FH, Liu C, Zhang QY, Kraus C, et al. SDF-1 activates papillary label-retaining cells during kidney repair from injury. Am J Physiol Renal Physiol. (2012) 302:F1362–73. doi: 10.1152/ajprenal.00202.2011
138. Chung ES, Miller L, Patel AN, Anderson RD, Mendelsohn FO, Traverse J, et al. Changes in ventricular remodelling and clinical status during the year following a single administration of stromal cell-derived factor-1 non-viral gene therapy in chronic ischaemic heart failure patients: the STOP-HF randomized Phase II trial. Eur Heart J. (2015) 36:2228–38. doi: 10.1093/eurheartj/ehv254
Keywords: bronchopulmonary dysplasia, pulmonary hypertension, pathogenesis, diagnosis, novel therapeutic strategies, preclinical studies
Citation: El-Saie A and Shivanna B (2020) Novel Strategies to Reduce Pulmonary Hypertension in Infants With Bronchopulmonary Dysplasia. Front. Pediatr. 8:201. doi: 10.3389/fped.2020.00201
Received: 12 December 2019; Accepted: 02 April 2020;
Published: 08 May 2020.
Edited by:
Karen Cecile Young, University of Miami, United StatesReviewed by:
Ulrich Herbert Thome, Leipzig University, GermanyTrent E. Tipple, University of Oklahoma Health Sciences Center, United States
Copyright © 2020 El-Saie and Shivanna. This is an open-access article distributed under the terms of the Creative Commons Attribution License (CC BY). The use, distribution or reproduction in other forums is permitted, provided the original author(s) and the copyright owner(s) are credited and that the original publication in this journal is cited, in accordance with accepted academic practice. No use, distribution or reproduction is permitted which does not comply with these terms.
*Correspondence: Binoy Shivanna, shivanna@bcm.edu