- 1Division of Neonatology, Department of Pediatrics, Leiden University Medical Center, Leiden, Netherlands
- 2The Ritchie Centre, Hudson Institute of Medical Research, Melbourne, VIC, Australia
- 3Department of Obstetrics and Gynecology, Monash University, Melbourne, VIC, Australia
- 4Monash Newborn, Monash Medical Centre, Melbourne, VIC, Australia
Objective: Continuous positive airway pressures (CPAP) used to assist preterm infants at birth are limited to 4–8 cmH2O due to concerns that high-CPAP may cause pulmonary overexpansion and adversely affect the cardiovascular system. We investigated the effects of high-CPAP on pulmonary (PBF) and cerebral (CBF) blood flows and jugular vein pressure (JVP) after birth in preterm lambs.
Methods: Preterm lambs instrumented with flow probes and catheters were delivered at 133/146 days gestation. Lambs received low-CPAP (LCPAP: 5 cmH2O), high-CPAP (HCPAP: 15 cmH2O) or dynamic HCPAP (15 decreasing to 8 cmH2O at ~2 cmH2O/min) for up to 30 min after birth.
Results: Mean PBF was lower in the LCPAP [median (Q1–Q3); 202 (48–277) mL/min, p = 0.002] compared to HCPAP [315 (221–365) mL/min] and dynamic HCPAP [327 (269–376) mL/min] lambs. CBF was similar in LCPAP [65 (37–78) mL/min], HCPAP [73 (41–106) mL/min], and dynamic HCPAP [66 (52–81) mL/min, p = 0.174] lambs. JVP was similar at CPAPs of 5 [8.0 (5.1–12.4) mmHg], 8 [9.4 (5.3–13.4) mmHg], and 15 cmH2O [8.6 (6.9–10.5) mmHg, p = 0.909]. Heart rate was lower in the LCPAP [134 (101–174) bpm; p = 0.028] compared to the HCPAP [173 (139–205)] and dynamic HCPAP [188 (161–207) bpm] groups. Ventilation or additional caffeine was required in 5/6 LCPAP, 1/6 HCPAP, and 5/7 dynamic HCPAP lambs (p = 0.082), whereas 3/6 LCPAP, but no HCPAP lambs required intubation (p = 0.041), and 1/6 LCPAP, but no HCPAP lambs developed a pneumothorax (p = 0.632).
Conclusion: High-CPAP did not impede the increase in PBF at birth and supported preterm lambs without affecting CBF and JVP.
Introduction
Respiratory support for preterm infants at birth has shifted from intubation and mechanical ventilation toward non-invasive strategies (1–4). However, when applied non-invasively, intermittent positive pressure ventilation (iPPV) is unable to ventilate the lung if the larynx is closed, which is known to occur in the fetus and newborn during apnea (5–8). As such, attention has now focused on stimulating and supporting spontaneous breathing at birth using continuous positive airway pressure (CPAP) (9–11). This has highlighted a knowledge gap regarding how CPAP should be applied in the delivery room. Currently, 4–8 cmH2O of CPAP is widely adopted, but this has been extrapolated from strategies used in the neonatal intensive care unit as there is little scientific evidence to support this pressure range at birth (12, 13).
To optimize CPAP support for preterm infants at birth, the underlying physiology of the infant needs to be considered as it transitions to newborn life. During pregnancy, the airways are liquid-filled and pulmonary blood flow (PBF) is low and so at birth, the airways must be cleared of liquid to allow the entry of air and PBF must increase to facilitate the onset of pulmonary gas exchange (14–18). Lung aeration triggers a decrease in pulmonary vascular resistance (PVR) and increase in PBF (19, 20), which is critical for the maintenance of cardiac output after birth, as PBF must take over the role of providing preload for the left ventricle following cord clamping (17).
As transpulmonary pressures generated by inspiration drive lung aeration after birth, increasing this pressure gradient could assist spontaneously breathing newborns aerate their lungs (18, 21, 22). This can be achieved by applying CPAP, but the optimal CPAP strategy is unknown. As airway resistance is initially high, due to the high viscosity of airway liquid (23, 24), theoretically, a high-CPAP will help overcome the initial high resistance of the liquid-filled airways. However, as the lungs aerate the resistance reduces and so the required CPAP level may also reduce (18, 22–25). Thus, a CPAP strategy that starts high and then decreases could reflect a changing physiological role for CPAP at birth. Initially CPAP may assist with lung aeration, but as the lung aerates and liquid is replaced by air, the role of CPAP could change to preventing liquid re-entry and alveolar collapse (18, 22–25). However, high airway pressures have been associated with pulmonary overexpansion, pneumothoraxes, reductions in cardiac output and PBF as well as elevated central venous pressures in (preterm) infants (26–28), adults (29–32), and animals (33–36).
Indeed, while high positive end-expiratory pressures (PEEP) enhance lung aeration and oxygenation and can reduce intubations and surfactant requirements in preterm infants (37), they also reduce PBF and increase the risk of pneumothoraxes in intubated and mechanically ventilated animals (38–42). As the application of CPAP during spontaneous breathing and PEEP during mechanical ventilation may have different effects on lung physiology, it is important to understand how high-CPAP levels affect lung physiology during spontaneous breathing. Recently, a retrospective study across two hospitals has compared two different CPAP levels (5–8 cmH2O vs. 12–35 cmH2O) in preterm infants. While they found no differences in oxygenation levels or heart rates, high-CPAP was found to reduce the use of supplemental oxygen at the expense of a higher pneumothorax rate (43). The latter may result from pulmonary overexpansion, but similar oxygenation levels and heart rates indicate that the cardiovascular system was not compromised. Although high intra-thoracic pressures are known to reduce venous return, increase central venous pressure, reduce cardiac output and reduce PBF, the impact of high-CPAP levels during spontaneous breathing on cardiovascular function is unknown (35, 38, 41, 44, 45).
The aim of this study was to determine the effect of high-CPAP levels, applied immediately after birth, on PBF, cerebral blood flow (CBF) and jugular venous pressure (JVP) and to determine whether a decrease in CPAP after stabilization can avoid these adverse effects. We hypothesized that high-CPAP during transition will reduce PBF and CBF and increase JVP, whereas high-CPAP levels that are reduced as the lung aerates will avoid these adverse effects.
Materials and Methods
Ethics Statement
Study procedures were performed in accordance with the National Health and Medical Research Council of Australia guidelines for care and use of experimental animals and were approved by Monash University “MMCA” Ethics committee. All research staff exposed to the sheep were vaccinated against Q fever (Q-Vax CSL, Australia).
Pre-experimental Surgical Preparation
At 129–130 days gestation, ewes (Border-Leicester) were anesthetized for fetal instrumentation. Anesthesia was induced with sodium thiopental (1 g in 20 mL, Pentothal IV; Jurox, NSW, Australia) and maintained, following endotracheal intubation, with inhaled isoflurane (≈2–5%; Isoflow, Abbott Laboratories, IL, US) in air/oxygen as previously described (46). Following exteriorization of the fetus (Merino X Border-Leicester), ultrasonic flow probes (Transonic Systems, Ithaca, NY, US) were placed around the left carotid artery (size 3) and left pulmonary artery (size 4), whereas polyvinyl catheters (0.86 mm ID, Dural Plastics, Sydney, NSW, Australia; 20 G × 1.16 peripheral venous catheter (1.1 mm × 30 mm), BD InsyteTM, Franklin Lakes, NJ, US) were inserted into the left brachial artery and right jugular vein. A silastic tube (0.078 and 0.125 mm ID, Down Corning, US) was placed in the upper trachea, and a sterile, saline-filled intrapleural balloon catheter (2.6 mm ID, Dural Plastics, Sydney, NSW, Australia) was placed in the intrapleural cavity. A transdermal fentanyl patch (75 μg/h; Janssen Cilag, Belgium) was used for post-operative analgesia and antibiotics (Cefazolin; AFT Pharmaceuticals, Auckland, New Zealand) were administered to the ewe (1 g), fetus (100 mg), and amniotic sac (400 mg) on the day of surgery, and daily for 2 days post-operatively.
Delivery and Support
At 132–133 days gestation (equivalent to ~28–30 weeks gestation age in humans), lambs were delivered by cesarean section under spinal anesthesia, as previously described (47). Ewes were initially sedated with intravenous propofol (1 mg/kg; Claris Lifesciences Limited, Gujarat, India) while spinal anesthesia was induced (lignocaine 2%, 0.1 mL/kg, Pfizer, NY, US), following which sedation continued with intravenous midazolam (1.0 mg/kg/h at 20–25 mL/h, Alphapharm, NSW, Australia); local anesthetic (xylocaine 10%, AstraZeneca, Cambridge, UK) was applied to the incision site.
Just prior to full delivery, a transcutaneous pulse oximeter (Radical 7, Masimo, CA, US) was attached to the right forelimb of the lamb to measure peripheral arterial oxygen saturation (SpO2) and a Near Infrared Spectroscopy (NIRS) optode (Casmed Foresight, CAS Medical Systems Inc., Branford, CT, US) was placed over the left frontal cortex. Blood gas measurements were corrected to core body temperature measured using a rectal temperature probe (ADInstruments, NSW, Australia). Catheters and probes were connected to a PowerLab (ADInstruments, NSW, Australia) to enable continuous recording of physiological data throughout the study.
The umbilical cord was immediately clamped before lambs were moved to a warmed bed (CosyCot, Fisher and Paykel, Auckland, New Zealand) and were given naloxone (0.01 mg/kg, Juno Therapeutics, WA, US) and anexate (0.1 mg/mL, Flumazenil, Apotex, Toronto, Canada) to counteract any maternally administered fentanyl and midazolam that had crossed the placenta before cord clamping. Also, caffeine (20 mg/kg, Sigma-Aldrich, St. Louis, MO, US) and doxapram (5 mg/kg, Bayer, Leverkusen, Germany) were given to stimulate spontaneous breathing immediately after birth. CPAP was provided by a ventilator in CPAP mode (Babylog 8000+, Drager, GA Lübeck, Germany) using warmed and humidified gases (MR850 Heated Humidifier, Fisher & Paykel, Auckland, New Zealand) and applied using bi-nasal prongs especially designed for preterm sheep. Respiratory support was commenced with CPAP and a fraction of inspired oxygen (FiO2) of 0.3. If the lamb showed visible gastric distension, a tube was placed briefly in the esophagus to remove air. The tube was removed after the gastric distension diminished.
If lambs became apneic (defined as an absence of breathing for 4–5 s) or had a decreasing cerebral or peripheral oxygenation, tactile stimulation (rubbing fingers along the lambs spine, limbs, and body) was applied, FiO2 was stepwise increased (0.3–0.5–1.0) to target a SpO2 of 90–95% and if this failed rescue interventions were given [additional caffeine bolus (20 mg/kg) and/or iPPV (PEEP 8, PIP 35 cmH2O 1:1 inflation: deflation time)].
CPAP Intervention
Lambs received various CPAP levels to (I) compare CPAP-strategies and (II) to explore the effect of changes in CPAP level. The experimental design is detailed below and presented in Figure 1.
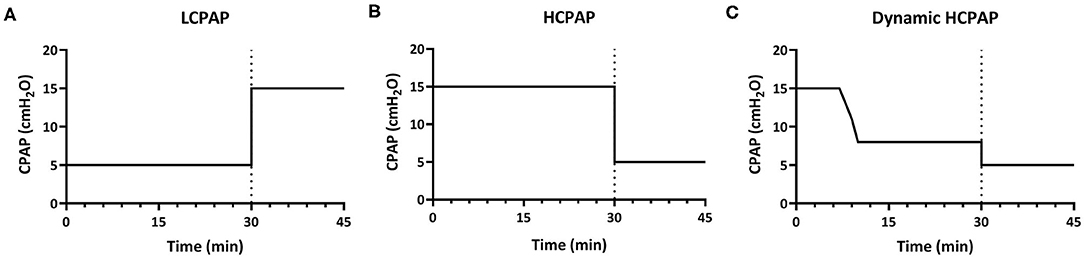
Figure 1. Experimental design. In part 1, lambs were divided into three groups and for 30 min received either low-CPAP [5 cmH2O; LCPAP (A)], high-CPAP [15 cmH2O; HCPAP (B)], or high-CPAP that was gradually reduced after reaching set physiological parameters [decreasing from 15 to 8 cmH2O; dynamic HCPAP (C)]. After 30 min (indicated by dotted line), CPAP was either increased (to 15 cmH2O) in the LCPAP group or reduced (to 5 cmH2O) in both the HCPAP and dynamic HCPAP groups for a further 15 min (part II).
Part I: Comparing CPAP-strategies (0–30 min)
Lambs were randomly allocated to a low-CPAP (control group: LCPAP, 5 cmH2O) or two high-CPAP groups that used different strategies (intervention groups). A CPAP of 5 cmH2O was used as the control group as it is widely used in clinical practice and it is well-established that an end-expiratory pressure is required to help aerate the lung of preterm neonates (12, 13). Lambs allocated to the intervention groups received high-CPAP (HCPAP, 15 cmH2O) or an initially high-CPAP that was decreased (dynamic HCPAP, 15–8 cmH2O). In the dynamic HCPAP group, CPAP was reduced when PBF was >300 mL/min, SaO2 >90%, FiO2 ≤ 0.7 and the lamb was spontaneously breathing. At this time, CPAP was decreased in a stepwise fashion at a rate of ~2 cmH2O per minute to 8 cmH2O, which is the upper limit of the recommended CPAP range in the ANZCOR guidelines (48).
Part II: Exploring the Effect of CPAP Changes (30–45 min)
In lambs that completed the first 30 min of the experiment, CPAP levels were either increased or decreased and the study continued for a further 15 min to explore the effect of CPAP changes on lung physiology after aeration. In the LCPAP group, CPAP was immediately increased from 5 to 15 cmH2O, whereas in the HCPAP group, CPAP was immediately decreased from 15 to 5 cmH2O and in the dynamic HCPAP group, CPAP was immediately decreased from 8 to 5 cmH2O.
Lambs received a lethal dose of sodium pentobarbitone (100 mg/kg intravenously, Virbac Pty Ltd., Peakhurst, Australia) after completing study duration (30 + 15 min) or earlier due to reaching an ethical endpoint, where it was assessed that intubation was considered necessary. Intubation was deemed necessary if lambs were hypoxic and did not initiate breathing, despite the use of tactile stimulation, 100% oxygen and the rescue interventions detailed above.
Outcome Measures
Physiological Data Measurement and Collection
PBF and CBF were measured using a flow meter (Transonics systems; Ithaca, NY, US), whereas brachial arterial (BAP), intrapleural, upper tracheal (UTP), and jugular venous pressures (JVP) were measured with pressure transducers (BP Transducer/Cable kit, ADInstruments, New South Wales Australia) and FiO2 was measured with a MX300-I Oxygen sensor (Teledyne Analytical instruments, CA, US). Physiological data was recorded continuously using LabChart 8 (ADInstruments, New South Wales Australia) and arterial blood gas status was measured every 3 min (ABL90 flex and ABL800 analyzer, Radiometer Medical ApS, Brønshøj, Denmark).
For physiological data, a mean was calculated over a 10 s epoch every 3 min, heart rate was derived from PBF and alveolar to arterial differences in PO2 (AaDO2) was calculated as [(713*FiO2) -(PaCO2/0.8) -PaO2]. To assess CBF stability, a mean CBF was calculated over each 15 s epochs and the CBF variability was calculated by measuring the coefficient of variation of mean CBF values throughout the experiment; this was calculated as [standard deviation (CBF)/average (CBF)*100]. Breathing rate (60/duration period*number of breaths) and inter-breath variability [standard deviation (duration breath)/average (duration breath)*100] were calculated in 30 s epochs. Expirations that involved distinct increases in intrapleural pressure above baseline were considered to be active expirations that involve the use of expiratory muscles.
Statistical Analysis
Data were analyzed using SPSS Statistics version 24.0 (IBM Software, Chicago, Illinois, US, 2018). Baseline characteristics were compared using Kruskal-Wallis test, Pearson Chi-Square test or Fishers exact test. Raw data are presented as median (Q1–Q3) or n (%). Statistical significance was accepted when p < 0.05.
CPAP strategies were compared over time using linear mixed-effect regression models, accounting for the relation between multiple measurements of the same lamb with a first-order autoregressive covariance structure on residuals. Group, time and group*time interaction were included in the model as fixed factors and random effects. To ensure that the estimated value of the parameters remained between 0–100% and 21–100%, logit transformations were used on the SaO2 (Ln(X/(1-X))) and FiO2 (Ln(79/100*(X-0.21)/(1–79/100*(X-0.21)))). The data included in the analysis were obtained either throughout the study duration, including during iPPV, or until reaching the ethical endpoint of intubation. Group p-values were used to compare means between groups. Post-hoc analyzes were performed using the False Discovery Rate. Data are presented as the median (Q1–Q3) of the group effect in the first 30 min after birth.
As rescue interventions were allowed, a comparison between groups did not reflect the effect of CPAP alone. To clarify the impact of iPPV and additional caffeine treatments on the results, the effect of successful interventions was explored using Wilcoxon Signed-Rank Test. Interventions were deemed successful if lambs continued spontaneously breathing on CPAP after iPPV or if breathing improved after the additional caffeine administration.
JVP was compared between CPAP levels using a Kruskal-Wallis Test. UTP and CPAP pressures were compared with a Wilcoxon Signed-Rank Test. The effect of a change in CPAP pressure was analyzed using Friedman's test as missing values were completed using the mean value of the group.
Results
Lambs allocated to the LCPAP (n = 6), HCPAP (n = 6), or dynamic HCPAP (n = 7) groups showed no differences in baseline characteristics at birth (Table 1). In the dynamic HCPAP group, CPAP was reduced after a median (Q1–Q3) of 13.4 (8.4–20.3) min after birth, when PBF was 339 (288–423) mL/min and SaO2 was 91 (86–96) %, while supported by a FiO2 of 0.59 (0.29–0.68).
Part I: Comparing CPAP Strategies
During the first 30 min after birth, mean (p = 0.002) and end-diastolic (p = 0.016) PBF [median (Q1–Q3) over 30 min] were significantly lower in the LCPAP group compared to the HCPAP and dynamic HCPAP groups (Figures 2A,B and Table 2). There was no difference in CBF (p = 0.166) or CBF variability (p = 0.218) between the LCPAP, HCPAP and dynamic HCPAP groups over the 30 min period after birth (Figure 2C and Table 2). BAP (p = 0.037) was lower in the LCPAP group compared to the HCPAP and dynamic HCPAP group (Figure 2D and Table 2). Heart rates were lower (p = 0.028) in the LCPAP group compared to the dynamic HCPAP group but were not different to the HCPAP group (Figure 2E and Table 2).
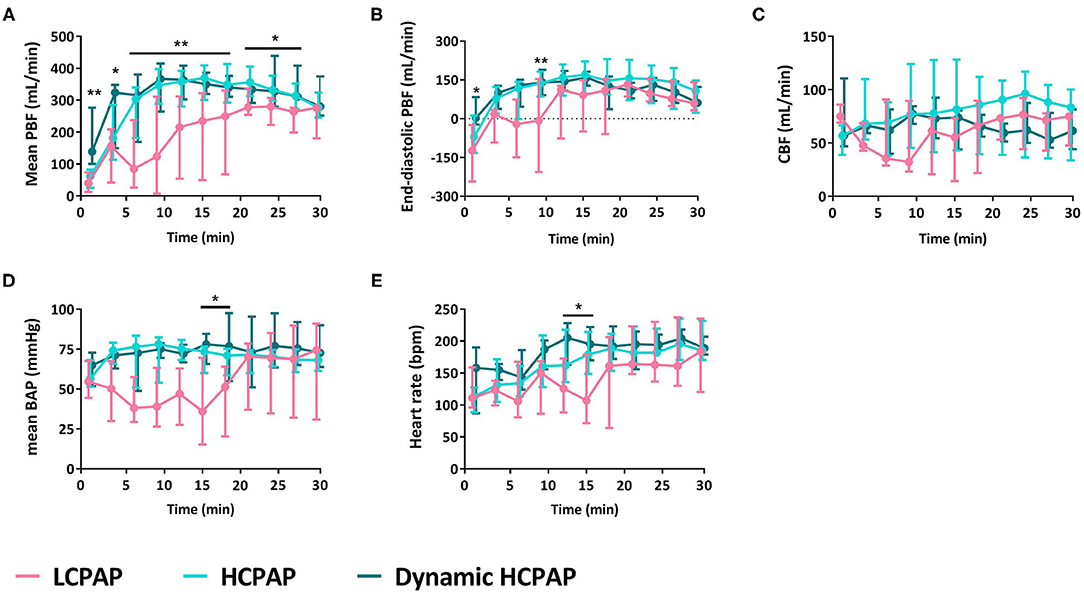
Figure 2. Cardiovascular outcomes. Median (Q1–Q3) of mean (A) and end-diastolic (B) pulmonary blood flow (PBF), cerebral blood flow (CBF, C), mean brachial artery pressure (BAP, D), and heart rate (E) in lambs receiving LCPAP vs. HCPAP vs. dynamic HCPAP in the first 30 min after birth (t0 = cord clamping). All parameters were compared over time using linear mixed-effect regression models. One (*) or two asterisks (**) indicate significant difference between the LCPAP vs. (dynamic) HCPAP or LCPAP vs. both HCPAP groups, respectively.
Breathing rates were lower in the LCPAP group (p = 0.009) compared to the HCPAP and dynamic HCPAP groups, whereas inter-breath variability was not different between groups (p = 0.412, Figure 3A and Table 2). The need for rescue interventions was statistically similar (p = 0.082) between groups, although only 1/6 (17%) HCPAP treated lambs received rescue treatments, whereas 5/6 (83%) LCPAP, and 5/7 (71%) dynamic HCPAP lambs received rescue interventions (Figure 3B and Table 2). Nevertheless, more LCPAP lambs [3/6 (50%)] reached the point at which intubation was required, compared with [0/6 (0%)] HCPAP and [0/7 (0%)] dynamic HCPAP lambs (p = 0.041). During post-mortem examination, 1/6 (17%), 0/6 (0%), and 0/7 (0%) lambs of the LCPAP, HCPAP, and dynamic HCPAP group, respectively, had developed a pneumothorax (p = 0.632).
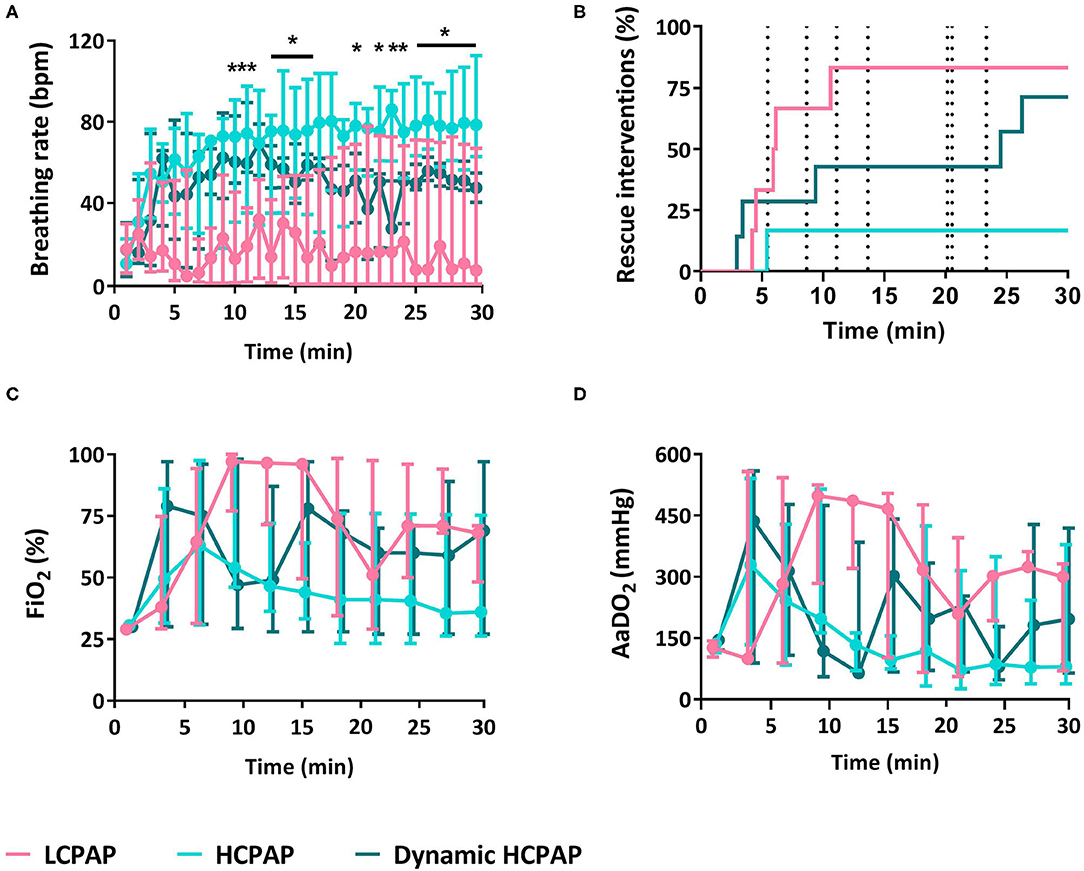
Figure 3. Breathing and support. Median (Q1–Q3) breathing rate (A), rescue interventions (B), fraction of inspired oxygen (FiO2, C), and alveolar to arterial differences in PO2 (AaDO2, D) in lambs receiving LCPAP vs. HCPAP vs. dynamic HCPAP in the first 30 min after birth (time 0 = cord clamping). All parameters were compared over time using linear mixed-effect regression models. One (*) or two asterisks (**) indicate significant difference between the LCPAP vs. (dynamic) HCPAP or LCPAP vs. both HCPAP groups, respectively. Dotted vertical lines (B) indicates the point of CPAP change in the dynamic HCPAP group.
There were no differences in FiO2 (p = 0.286), AaDO2 (p = 0.241), SaO2 (p = 0.273), PaCO2 (p = 0.303), pH (p = 0.097), and glucose (p = 0.435) between groups (Figure 4 and Table 2). Lactate levels tended (p = 0.050) to be greater in the LCPAP group and base excess was lower (p = 0.044) in the LCPAP group, compared to the HCPAP group (Figures 3C,D, 4 and Table 2).
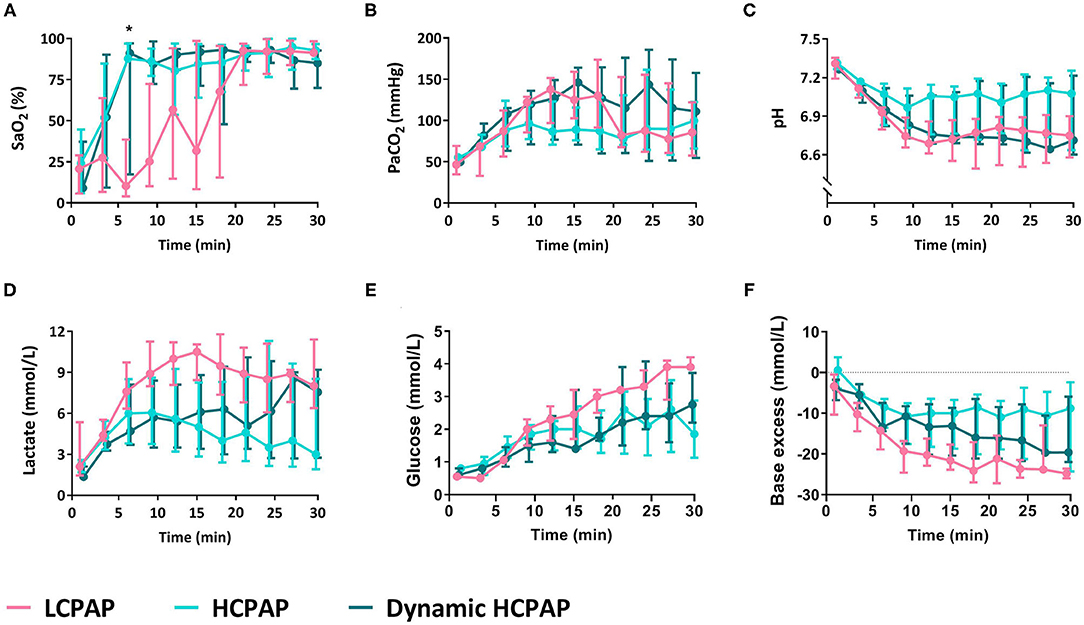
Figure 4. Blood gas parameters. Median (Q1–Q3) percentage of oxygen saturation of arterial blood (SaO2, A), partial pressure of carbon dioxide in arterial blood (PaCO2, B), pH (C), lactate (D), glucose (E), and base excess (F) in lambs receiving LCPAP vs. HCPAP vs. dynamic HCPAP in the first 30 min after birth (time 0 = cord clamping). All parameters were compared over time using linear mixed-effect regression models. One asterisk (*) indicates the significant difference between the LCPAP vs. HCPAP group.
Baseline JVP levels were measured for each CPAP level over the first 30 min after birth, to avoid pressure changes associated with movement and breathing. Baseline JVP levels were similar at CPAP levels of 5 cmH2O [8.0 (5.1–12.4)], 8 cmH2O [9.4 (5.3–13.4)], and 15 cmH2O [8.6 (6.9–10.5)] and were also similar during iPPV 5.4 [(4.9–9.4) mmHg, p = 0.571]. However, JVP levels were increased, by an average of 3.8 (1.6–8.3) mmHg, when lambs were actively exhaling or utilizing expiratory braking maneuvers (indicated by airway pressures above CPAP). These activities resulted in maximum JVPs of 12.3 (5.9–17.3) mmHg (Figure 5A).
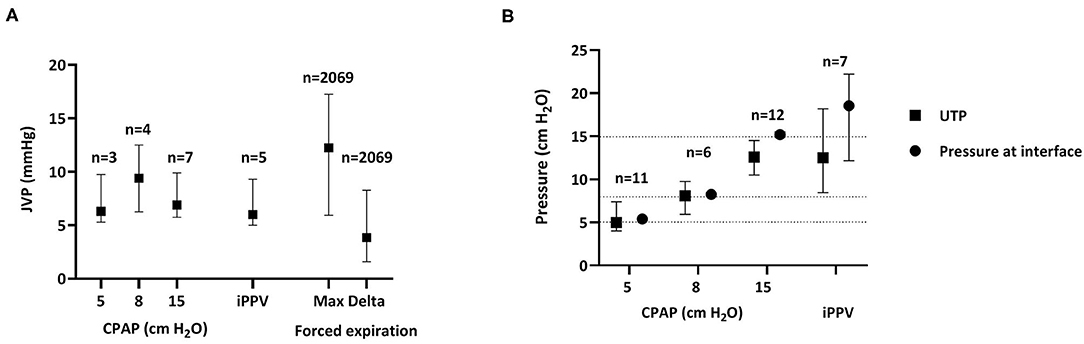
Figure 5. Jugular venous pressure and upper tracheal pressure. (A) Jugular venous pressures [JVP; median (Q1–Q3)] measured during the first 30 min after birth at different CPAP levels and during iPPV as well as the maximum (Max) pressure achieved and the change in pressure (Delta) associated with body movements or forced expirations; n = the number of animals during CPAP or iPPV, whereas n = the number of breaths with active forced expiration. JVPs were compared between groups using Kruskal-Wallis Tests. (B) Pressures [median (Q1–Q3)] measured simultaneously in the upper trachea (UTP, filled squares) and CPAP interface (nasal prongs, filled circles) during CPAP and iPPV throughout the entire experiment (30 + 15 min) n = animal number. Pressures were compared using Wilcoxon Signed-Rank Tests. Dotted horizontal line indicates 5, 8, and 15 cmH2O CPAP.
Baseline UTP levels were measured for each CPAP level throughout both phases of the experiment (0–45 min) to avoid pressure changes associated with movement and breathing. When a CPAP of 5 cmH2O was given, pressures measured at the CPAP interface (nasal prongs) were similar to those measured in the upper trachea, below the larynx [CPAP vs. UTP, 5 cmH2O: 5.4 (5.1–5.6) vs. 5.0 (4.0–7.4), p = 0.953]. Similarly, at a CPAP of 8 cmH2O the pressures measure at the CPAP interface [8.3 (8.1–8.5) cmH2O] were similar to UTPs [8.1 (6.0–9.8) cmH2O; p = 0.715]. However, when 15 cmH2O of CPAP was applied, UTP was lower than the pressure applied at the CPAP interface [12.6 (10.5–14.5) vs. 15.2 (14.9–15.5) cmH2O, respectively, p = 0.013]. Similarly, during iPPV, mean airway pressures measured in the UT were significantly less than that measured at the CPAP interface [12.5 (8.5–18.2) vs. 18.6 (12.2–22.2), respectively, p = 0.028; Figure 5].
Rescue Interventions
In the first 30 min after birth, 11 lambs received 9 additional dosages of caffeine and/or 13 periods of iPPV via the nasal prongs. Lambs were ventilated with an inflation rate of 50 (30–50) bpm and inter-breath variability of 11 (8–40) % for a duration of 3:08 (2:27–9:22) min (Table 3).
On 5/9 (56%) occasions, caffeine improved breathing and increased mean PBF. On the 10/13 (77%) occasions that iPPV was deemed successful, the first inflation was transmitted to the lungs after 4 (2–29) s and increased breathing rate, mean PBF, heart rate, SaO2, and AaDO2 (Table 3). In 3/13 (23%) animals, ventilation did not reach the lungs and intubation was required.
Part II: Changing CPAP Levels
Reducing CPAP from 15 to 8 or from 15 to 5 cmH2O, increased the FiO2 requirement, without affecting mean PBF, CBF or any of the blood gas parameters (Figure 6 and Table 4). Increasing CPAP from 5 to 15 cmH2O, reduced the FiO2 requirement and resulted in a small (~10%) but statistically significant decrease in mean PBF that took ~5 min to manifest (Figure 6). Otherwise, increasing CPAP levels had no effect on CBF, breathing rate, PaCO2, and SaO2 (Table 4).
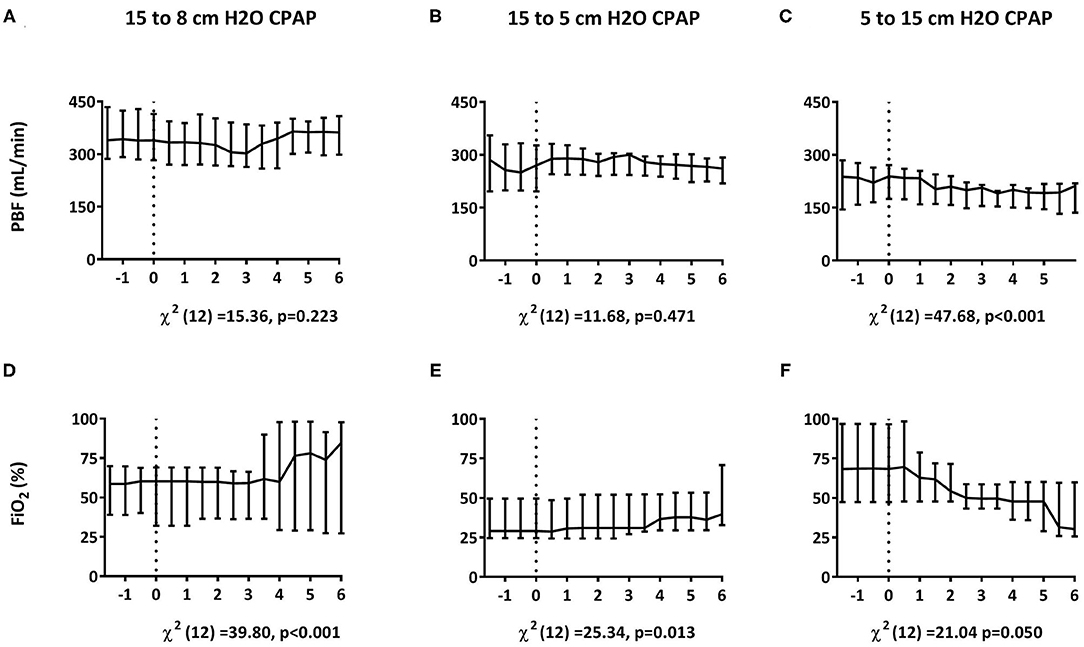
Figure 6. Effect of changing CPAP levels. Changes in pulmonary blood flow [PBF; A–C; median (Q1–Q3)] and fraction of inspired oxygen [FiO2; D–F; median (Q1–Q3)] measured in response to changes in CPAP levels from 15 to 8 cmH2O within 30 min after birth (A,D) and from 15 to 5 cmH2O (B,E) or 5 to 15 cmH2O (C,F) at 30 min after birth. Parameters were compared over time using Friedman's tests. t = 0 indicates the start of CPAP change.
Discussion
This is the first study to investigate the effect of high-CPAP, applied non-invasively, on cardiorespiratory function at birth in spontaneously breathing animals. Contrary to our hypothesis, high-CPAP did not impede the normal increase in PBF at birth, nor reduce CBF or increase JVP in spontaneously breathing preterm sheep immediately after birth. Instead, high-CPAP resulted in higher PBF, heart rate and BAP compared to low-CPAP, while showing no effect on CBF, CBF variability or the incidence of pneumothoraces. The increased mean and end-diastolic PBF also indicates that HCPAP lambs sooner achieved a lower PVR compared to the LCPAP lambs, that was substantially lower than the systemic venous resistance. While CPAP levels had little impact on JVP, large fluctuations in JVP were observed in all groups and were associated with active expirations that involved the use of expiratory muscles (mostly abdominal). In addition, lambs receiving high-CPAP had a higher breathing rates, tended to receive fewer rescue interventions and required significantly fewer intubations. Overall, high-CPAP was more successful in supporting preterm lambs throughout the neonatal transition at birth. After stabilization, reducing the CPAP level from 15 to 8 cmH2O resulted in a higher FiO2 requirement, while increasing the CPAP level from 5 to 15 cmH2O reduced the FiO2 requirement, but also caused a small reduction in PBF. Thus, we found no evidence to indicate that the use of high-CPAP immediately after birth causes pulmonary overexpansion (as indicated by a reduction in PBF) or compromises the cardiovascular system. As high-CPAP did not affect CBF, its stability or JVP, compared to low-CPAP, we found no evidence that suggests that high-CPAP may increase the risk of IVH.
Our results are in marked contrast with previous findings showing that end-expiratory pressures above 8 cmH2O cause pulmonary overexpansion and reduce PBF in intubated and mechanically ventilated animals (38–41). Increasing PEEP during iPPV is thought to decrease venous return to the right atrium and increase PVR by increasing alveolar pressures above alveolar capillary pressures, causing capillary closure and a reduction in PBF (39). While these studies were the best available evidence for predicting how high-pressure support affected cardiovascular function at birth, the different PBF responses between studies highlights that the two respiratory support modes are distinctly different. The primary differences being that; (i) superimposing sub-atmospheric intrathoracic pressures (due to spontaneous breathing) on a background of high-CPAP, produces different intrathoracic pressure gradients than intubation and iPPV with PEEP (Figure 7); and (ii) intubation and mechanical ventilation removes the role of the larynx, whereas CPAP and spontaneous breathing includes it.
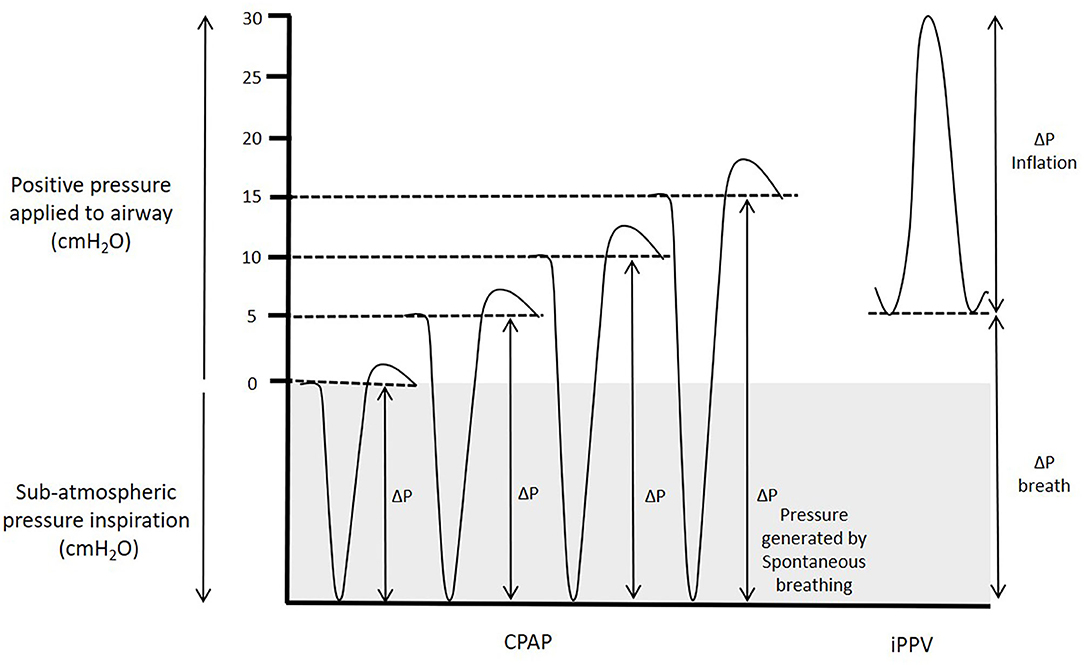
Figure 7. Pressure generated during CPAP and iPPV. Transpulmonary pressures (ΔP) are the primary factor that drives lung aeration after birth. During spontaneous breathing, thoracic expansion generates sub-atmospheric intrathoracic pressures, which when combined with CPAP increase the effective transpulmonary pressure applied to the lung. While intrathoracic pressures must transiently increase above the CPAP level to effect expiration, the CPAP level is effectively the highest external pressure applied to the airways and the mean intrathoracic airway pressure is considerably lower than the CPAP level. In contrast, during iPPV, PEEP is the lower limit of the applied pressure to the airways and the transpulmonary pressure that drives airway liquid clearance results from pressure increases above PEEP resulting in a mean airway pressure considerably higher than the PEEP.
The differential effects of spontaneous breathing and iPPV on PBF are well-established in adult physiology and the ability of individual breaths to increase PBF has been shown directly in fetal sheep (49) and indirectly in newborn infants (50). When infants breathe spontaneously during CPAP, the intrathoracic pressure phasically decreases below the CPAP level. As such, the CPAP level is effectively the highest external pressure applied to the airways, although pressures in the lower airways must briefly increase above the CPAP level to effect expiration. Nevertheless, the mean airway pressure is lower than the CPAP level measured at the mouth opening. However, during iPPV with PEEP, the PEEP level is the lower limit of the externally applied pressure and pressures phasically increase above this with each inflation (Figure 7). As such, the mean airway pressure is higher than the PEEP level and is substantially higher than occurs with a similar CPAP level during spontaneous breathing. Indeed, we found that during iPPV, lambs had a mean airway pressure of 18.6 cmH2O, despite using a PEEP of 5 cmH2O.
As adduction of the larynx can seal the airways and prevent air from enter or leaving the lungs (6–8), it can regulate the pressure in the lower airways and protect the lungs from high pressures and hyperinflation. It also allows pressurization of the airways and thorax during active expiration, as occurs during a Valsalva maneuver, for example. This is consistent with the concept that preterm newborns commonly use expiratory breaking maneuvers, either generated by post-inspiratory diaphragmatic contractions and/or laryngeal closure to help defend end-expiratory gas volumes during expiration (22, 51). We found that, when giving 15 cmH2O of CPAP or iPPV, only a proportion (~75%) of the pressure applied at the nasal prongs was transmitted through the larynx into the upper trachea. In contrast, at lower CPAP levels (at 5 and 8 cmH2O), all of the pressure applied at the nasal prongs was transmitted through the larynx into the upper trachea. Thus, it is possible that the larynx was closing to seal the airways near the end of expiration during high-CPAP, but remained open throughout the respiratory cycle at lower CPAP pressures. This may explain why studies in intubated preterm lambs observed higher pneumothorax rates when using PEEP ≤ 15 cmH2O (38, 41), while a recent study (37) found that the use of similar pressures applied non-invasively during iPPV in preterm infants did not increase the risk of adverse events.
In both the fetus and newborn, apnea or unstable breathing (varying in depth and rate), causes the larynx to close and only open during a breath (5–8). In contrast, during regular stable breathing, the larynx remains mostly open, but can briefly close to effect expiratory braking. However, lambs supported with 15 cmH2O were breathing continuously in a regular stable pattern and, as such we would expect the larynx to be mostly open. Nevertheless, glottic closure during expiration could be part of complicated breathing maneuvers induced by high-CPAP levels. Indeed, we noted that active expirations were common in these lambs, which likely reflects a physiological response to the increase in pressure that may also have included expiratory braking maneuvers during the expiratory phase of the breathing cycle (51).
It is also pertinent to note that in this study, the high-CPAP was applied from birth, when the lungs are initially liquid filled. In most previous studies, the lungs were aerated before the effect of high-PEEP levels on PBF was examined. This is consistent with our finding that increasing CPAP levels from 5 to 15 cmH2O at 30 min after birth caused a small (~10%) decrease in PBF, although this was substantially less than the decrease (~40%) observed when PEEP was increased from 4 to 12 cmH2O during iPPV at 20 min after birth (39). Nevertheless, the timing for when high-CPAP levels are applied to the airways may influence whether or not high pressures adversely affect PBF. Indeed, a sustained inflation (to 35 cmH2O) for up to 1 min does not adversely affect PBF when applied during lung aeration (52). Why this should occur is unclear, but it is possible that the initial stimulus for the increase in PBF overwhelms the adverse effect of increased alveolar pressure (52). It has recently been shown that a neural reflex, activated in response to liquid leaving the airways and entering lung tissue, triggers a global increase in PBF, which overrides all other influences such as oxygenation (19, 53). Thus, both the type and timing of application to the airways appear to determine how high airway pressures influence the cardiovascular system at birth.
At birth, pulmonary gas exchange is dependent on the available surface area (i.e., how much of the lung is aerated) and the partial pressure gradient for the respiratory gases, which for oxygen is largely determined by the FiO2. When the surface area is small, a large partial pressure gradient for oxygen is required for adequate oxygen exchange, whereas with increasing lung aeration, the surface area increases and so the required partial pressure gradient decreases. This finding is consistent with previous studies that have shown high-PEEP levels increase lung aeration and oxygenation and lowers the oxygen requirement (38, 39, 42, 43, 54). While we found no statistically significant differences between groups in FiO2, SaO2, or AaDO2, there was a clear trend toward a higher AaDO2 and FiO2 requirement and lower SaO2 in the low-CPAP group. The absence of a significant difference likely results from the large variability associated with application of rescue therapies (iPPV and additional caffeine) and differences in physical stimulation levels, which are difficult to standardize. Nevertheless, the close relation between CPAP level and FiO2 requirement was clearly indicated by changing CPAP levels. To maintain a similar SaO2, decreasing CPAP levels increased the FiO2 requirement, whereas increasing CPAP levels reduced the FiO2 requirement. This is consistent with the concept that higher CPAP levels increases the available area for gas exchange. Whilst supplemental oxygen stimulates spontaneous breathing and improves oxygenation, as too much oxygen can cause harm (through hyperoxia) it is important to limit the use of high O2 concentrations (10, 55, 56). Our results, and those of our previous study (30), indicate that this can be achieved through the use of high-CPAP, which increases lung aeration, improves gas exchange, and reduces the FiO2 requirement. Future studies are required to confirm the role of high-CPAP on improving lung aeration, and to investigate the interaction between CPAP level and FiO2, to find the optimal support strategy that can be tested in preterm infants at birth.
Contrary to our hypothesis, the dynamic high-CPAP strategy was not superior to high-CPAP. Indeed, our findings indicate that the dynamic high-CPAP group may have benefitted by remaining at the high CPAP level for longer and delaying the decrease. Whilst these lambs continued to have a high PBF and SaO2 following the reduction in CPAP, they needed a higher FiO2 to maintain their oxygenation levels and most probably their level of breathing activity. As preterm infants often only require a maximum of 8 cmH2O CPAP in the neonatal ward, it is necessary to decrease the CPAP level at some stage, but the questions of when and upon what indication, remains unknown. Clearly, the strategy of dynamic high-CPAP strategy needs further investigation, to identify the desirable moment for reducing the CPAP level.
Our model of spontaneously breathing preterm animals over time introduces some bias into the study because it incorporates strategies to stimulate lambs to breath. As a result, differential use of rescue interventions between groups effected the results by improving study outcomes in groups with higher rates of rescue interventions. As the interventions were required more frequently in the low-CPAP and dynamic high-CPAP group, mostly after reducing the CPAP level, the differences between these groups and the high-CPAP group were potentially reduced. The early drop-out of animals in the low-CPAP group, which occurred after they had reached the ethical endpoint where intubation was required, likely further reduced the difference between the groups. As such, high-CPAP levels likely benefitted these preterm sheep to a greater degree than we were able to demonstrate.
It is unclear why lambs in all groups experienced high pCO2 and low pH levels, although it is likely to be mostly metabolic in origin as indicated by the high lactate and large negative base excess levels. Indeed, at 15–30 min, lambs in the HCPAP group had a high SaO2 (>90%) while receiving a relatively low FiO2 (~30%), but still had high PCO2 levels. As the solubility and exchange potential for CO2 is almost 30 times greater than O2, a problem with CO2 exchange in the absence of a problem with O2 exchange is unlikely and so the high PCO2 is most likely secondary to the metabolic acidosis. As lambs were not hypoxic, the metabolic acidosis is unlikely to have resulted from anaerobic glucose metabolism. It could have resulted from activation of the sympathetic nervous system due to birth related stress or to a cold stimulus as the lambs were not sedated. Indeed, while we measured core body temperatures and applied external heat as required, activation of non-shivering thermogenesis may have contributed to maintaining core body temperature. Furthermore, as caffeine is a non-specific phosphodiesterase inhibitor (57, 58), it may have enhanced these cAMP mediated responses. This is consistent with the finding that HCPAP lambs received less caffeine and tended to have higher pH and lower PCO2 levels. While acidosis and high PCO2 levels can adversely affect the cardiovascular system and reduce PBF, we found no evidence for this as all lambs, that completed the 30 min experimental period, achieved high PBFs with significant left-to-right shunting despite many of them had a low pH; the latter is indicative of a low pulmonary vascular resistance.
In summary, high-CPAP levels resulted in PBF levels that were markedly higher than those seen with low-CPAP levels when applied from birth, and successfully supported preterm lambs throughout the neonatal transition after birth. We did not find any indication that high-CPAP caused pulmonary overexpansion, compromised the cardiovascular system or increased risk factors for IVH when given directly at birth. However, at 30 min after birth, while increasing CPAP levels reduced the FiO2 requirement, it also caused a small reduction in PBF. This indicates that the timing after birth, and the type of positive pressure respiratory support applied, determines whether high airway pressures adversely affect the cardiovascular system at birth.
Data Availability Statement
The datasets supporting the conclusions of this article will be made available by the authors upon request to the corresponding author.
Ethics Statement
Study procedures were performed in accordance with the National Health and Medical Research Council of Australia guidelines for care and use of experimental animals and were approved by Monash University MMCA Ethics committee. All research staff exposed to the sheep were vaccinated against Q fever (Q-Vax CSL, Australia).
Author Contributions
TM, KC, KR, AP, and SH made substantial contributions to conception and design of the study. TM, KC, KR, JD, AD, AM, VZ, GP, CR, and SH performed the experiments and obtained the data. TM, SH, and AP were responsible for data analysis and interpretation and drafted the first version of the manuscript. All authors provided feedback and approved the final version of the manuscript. All authors included in this paper fulfill the criteria of authorship.
Funding
This research was supported by the National Health and Medical Research council (NHRMC) Program Grant (606789), and the Victorian Government's Operational Infrastructure Support Program. SH recipients of the NHMRC Research Fellowships (APP1058537), AP of a NWO innovational research incentives scheme (VIDI 91716428), CR and GP of Investigator Grants (APP1175634 and APP1173731), and AD of a German Research Foundation (AD: DFG 1909/2-1).
Conflict of Interest
The authors declare that the research was conducted in the absence of any commercial or financial relationships that could be construed as a potential conflict of interest.
Acknowledgments
We would like to thank Dr. Ilias Nitsos and Dalibor Stanojkovic for their technical assistance.
Abbreviations
AaDO2, alveolar to arterial differences in PO2; BAP, Brachial Artery Pressure; CBF, Carotid Blood Flow; CPAP, Continuous Positive Airway Pressure; FiO2, Fraction of inspired Oxygen; HCPAP, High-CPAP; iPPV, intermittent Positive Pressure Ventilation; JVP, Jugular Vein Pressure; LCPAP, Low-CPAP; UTP, Upper Tracheal Pressure; PaCO2, Partial Pressure of Carbon Dioxide in arterial blood; PBF, pulmonary blood flow; PEEP, Positive End-Expiratory Pressure; SaO2, percentage of oxygen saturation of arterial blood.
References
1. Morley CJ, Davis PG, Doyle LW, Brion LP, Hascoet JM, Carlin JB. Nasal CPAP or intubation at birth for very preterm infants. N Engl J Med. (2008) 358:700–8. doi: 10.1056/NEJMoa072788
2. Finer NN, Carlo WA, Walsh MC, Rich W, Gantz MG, Laptook AR, et al. Early CPAP versus surfactant in extremely preterm infants. N Engl J Med. (2010) 362:1970–9. doi: 10.1056/NEJMoa0911783
3. Trevisanuto D, Satariano I, Doglioni N, Criscoli G, Cavallin F, Gizzi C, et al. Changes over time in delivery room management of extremely low birth weight infants in Italy. Resuscitation. (2014) 85:1072–6. doi: 10.1016/j.resuscitation.2014.04.024
4. Brouwer E, Knol R, Vernooij ASN, van den Akker T, Vlasman PE, Klumper F, et al. Physiological-based cord clamping in preterm infants using a new purpose-built resuscitation table: a feasibility study. Arch Dis Child Fetal Neonatal Ed. (2019) 104:F396–402. doi: 10.1136/archdischild-2018-315483
5. Harding R, Bocking AD, Sigger JN. Upper airway resistances in fetal sheep: the influence of breathing activity. J Appl Physiol. (1986) 60:160–5.
6. Crawshaw JR, Kitchen MJ, Binder-Heschl C, Thio M, Wallace MJ, Kerr LT, et al. Laryngeal closure impedes non-invasive ventilation at birth. Arch Dis Child Fetal Neonatal Ed. (2018) 103:F112–9. doi: 10.1136/archdischild-2017-312681
7. Renolleau S, Letourneau P, Niyonsenga T, Praud JP, Gagne B. Thyroarytenoid muscle electrical activity during spontaneous apneas in preterm lambs. Am J Respir Crit Care Med. (1999) 159(5 Pt 1):1396–404. doi: 10.1164/ajrccm.159.5.9807088
8. Moreau-Bussiere F, Samson N, St-Hilaire M, Reix P, Lafond JR, Nsegbe E, et al. Laryngeal response to nasal ventilation in nonsedated newborn lambs. J Appl Physiol. (2007) 102:2149–57. doi: 10.1152/japplphysiol.00891.2006
9. Dekker J, Hooper SB, van Vonderen JJ, Witlox R, Lopriore E, Te Pas AB. Caffeine to improve breathing effort of preterm infants at birth: a randomized controlled trial. Pediatr Res. (2017) 82:290–6. doi: 10.1038/pr.2017.45
10. Dekker J, Martherus T, Lopriore E, Giera M, McGillick EV, Hutten J, et al. The effect of initial high vs. low FiO2 on breathing effort in preterm infants at birth: a randomized controlled trial. Front Pediatr. (2019) 7:504. doi: 10.3389/fped.2019.00504
11. Dekker J, Hooper SB, Martherus T, Cramer SJE, van Geloven N, Te Pas AB. Repetitive versus standard tactile stimulation of preterm infants at birth - a randomized controlled trial. Resuscitation. (2018) 127:37–43. doi: 10.1016/j.resuscitation.2018.03.030
12. Wyllie J, Bruinenberg J, Roehr CC, Rudiger M, Trevisanuto D, Urlesberger B. European resuscitation council guidelines for resuscitation 2015: section 7. Resuscitation and support of transition of babies at birth. Resuscitation. (2015) 95:249–63. doi: 10.1016/j.resuscitation.2015.07.029
13. Wyckoff MH, Aziz K, Escobedo MB, Kapadia VS, Kattwinkel J, Perlman JM, et al. Part 13: neonatal resuscitation: 2015 American heart association guidelines update for cardiopulmonary resuscitation and emergency cardiovascular care. Circulation. (2015) 132(18 Suppl. 2):S543–60. doi: 10.1161/cir.0000000000000267
14. Harding R, Hooper SB. Regulation of lung expansion and lung growth before birth. J Appl Physiol. (1996) 81:209–24. doi: 10.1152/jappl.1996.81.1.209
15. Hooper SB, Harding R. Fetal lung liquid: a major determinant of the growth and functional development of the fetal lung. Clin Exp Pharmacol Physiol. (1995) 22:235–47. doi: 10.1111/j.1440-1681.1995.tb01988.x
16. Rudolph AM. Fetal and neonatal pulmonary circulation. Annu Rev Physiol. (1979) 41:383–95. doi: 10.1146/annurev.ph.41.030179.002123
17. Hooper SB, Polglase GR, Roehr CC. Cardiopulmonary changes with aeration of the newborn lung. Paediatr Respir Rev. (2015) 16:147–50. doi: 10.1016/j.prrv.2015.03.003
18. Hooper SB, Kitchen MJ, Wallace MJ, Yagi N, Uesugi K, Morgan MJ, et al. Imaging lung aeration and lung liquid clearance at birth. FASEB J. (2007) 21:3329–37. doi: 10.1096/fj.07-8208com
19. Lang JA, Pearson JT, Binder-Heschl C, Wallace MJ, Siew ML, Kitchen MJ, et al. Vagal denervation inhibits the increase in pulmonary blood flow during partial lung aeration at birth. J Physiol. (2017) 595:1593–606. doi: 10.1113/JP273682
20. Lang JA, Pearson JT, te Pas AB, Wallace MJ, Siew ML, Kitchen MJ, et al. Ventilation/perfusion mismatch during lung aeration at birth. J Appl Physiol. (2014) 117:535–43. doi: 10.1152/japplphysiol.01358.2013
21. Hooper SB, Kitchen MJ, Siew ML, Lewis RA, Fouras A, te Pas AB, et al. Imaging lung aeration and lung liquid clearance at birth using phase contrast X-ray imaging. Clin Exp Pharmacol Physiol. (2009) 36:117–25. doi: 10.1111/j.1440-1681.2008.05109.x
22. Siew ML, Wallace MJ, Kitchen MJ, Lewis RA, Fouras A, Te Pas AB, et al. Inspiration regulates the rate and temporal pattern of lung liquid clearance and lung aeration at birth. J Appl Physiol. (2009) 106:1888–95. doi: 10.1152/japplphysiol.91526.2008
23. te Pas AB, Siew M, Wallace MJ, Kitchen MJ, Fouras A, Lewis RA, et al. Establishing functional residual capacity at birth: the effect of sustained inflation and positive end-expiratory pressure in a preterm rabbit model. Pediatr Res. (2009) 65:537–41. doi: 10.1203/PDR.0b013e31819da21b
24. te Pas AB, Siew M, Wallace MJ, Kitchen MJ, Fouras A, Lewis RA, et al. Effect of sustained inflation length on establishing functional residual capacity at birth in ventilated premature rabbits. Pediatr Res. (2009) 66:295–300. doi: 10.1203/PDR.0b013e3181b1bca4
25. Hooper SB, Te Pas AB, Kitchen MJ. Respiratory transition in the newborn: a three-phase process. Arch Dis Child Fetal Neonatal Ed. (2016) 101:F266–71. doi: 10.1136/archdischild-2013-305704
26. Hausdorf G, Hellwege HH. Influence of positive end-expiratory pressure on cardiac performance in premature infants: a Doppler-echocardiographic study. Crit Care Med. (1987) 15:661–4. doi: 10.1097/00003246-198707000-00007
27. Maayan C, Eyal F, Mandelberg A, Sapoznikov D, Lewis BS. Effect of mechanical ventilation and volume loading on left ventricular performance in premature infants with respiratory distress syndrome. Crit Care Med. (1986) 14:858–60. doi: 10.1097/00003246-198610000-00004
28. Trang TT, Tibballs J, Mercier JC, Beaufils F. Optimization of oxygen transport in mechanically ventilated newborns using oximetry and pulsed Doppler-derived cardiac output. Crit Care Med. (1988) 16:1094–7. doi: 10.1097/00003246-198811000-00002
29. Cassidy SS, Eschenbacher WL, Robertson CH Jr., Nixon JV, Blomqvist G, Johnson RL Jr. Cardiovascular effects of positive-pressure ventilation in normal subjects. J Appl Physiol Respir Environ Exerc Physiol. (1979) 47:453–61. doi: 10.1152/jappl.1979.47.2.453
30. Cournand A, Motley HL. Physiological studies of the effects of intermittent positive pressure breathing on cardiac output in man. Am J Physiol. (1948) 152:162–74. doi: 10.1152/ajplegacy.1947.152.1.162
31. Berendes E, Lippert G, Loick HM, Brussel T. Effects of positive end-expiratory pressure ventilation on splanchnic oxygenation in humans. J Cardiothorac Vasc Anesth. (1996) 10:598–602. doi: 10.1016/s1053-0770(96)80136-4
32. Eisner MD, Thompson BT, Schoenfeld D, Anzueto A, Matthay MA, Acute Respiratory Distress Syndrome Network. Airway pressures and early barotrauma in patients with acute lung injury and acute respiratory distress syndrome. Am J Respir Crit Care Med. (2002) 165:978–82. doi: 10.1164/ajrccm.165.7.2109059
33. Fuhrman BP, Everitt J, Lock JE. Cardiopulmonary effects of unilateral airway pressure changes in intact infant lambs. J Appl Physiol Respir Environ Exerc Physiol. (1984) 56:1439–48. doi: 10.1152/jappl.1984.56.5.1439
34. Fuhrman BP, Smith-Wright DL, Kulik TJ, Lock JE. Effects of static and fluctuating airway pressure on intact pulmonary circulation. J Appl Physiol. (1986) 60:114–22. doi: 10.1152/jappl.1986.60.1.114
35. Mirro R, Busija D, Green R, Leffler C. Relationship between mean airway pressure, cardiac output, and organ blood flow with normal and decreased respiratory compliance. J Pediatr. (1987) 111:101–6. doi: 10.1016/s0022-3476(87)80354-2
36. Cassidy SS, Robertson CH Jr., Pierce AK, Johnson RL Jr. Cardiovascular effects of positive end-expiratory pressure in dogs. J Appl Physiol Respir Environ Exerc Physiol. (1978) 44:743–50. doi: 10.1152/jappl.1978.44.5.743
37. Kanaan Z, Bloch-Queyrat C, Boubaya M, Levy V, Bolot P, Waszak P. Feasibility of combining two individualized lung recruitment maneuvers at birth for very low gestational age infants: a retrospective cohort study. BMC Pediatr. (2020) 20:144. doi: 10.1186/s12887-020-02055-3
38. Probyn ME, Hooper SB, Dargaville PA, McCallion N, Crossley K, Harding R, et al. Positive end expiratory pressure during resuscitation of premature lambs rapidly improves blood gases without adversely affecting arterial pressure. Pediatr Res. (2004) 56:198–204. doi: 10.1203/01.PDR.0000132752.94155.13
39. Polglase GR, Morley CJ, Crossley KJ, Dargaville P, Harding R, Morgan DL, et al. Positive end-expiratory pressure differentially alters pulmonary hemodynamics and oxygenation in ventilated, very premature lambs. J Appl Physiol. (2005) 99:1453–61. doi: 10.1152/japplphysiol.00055.2005
40. Polglase GR, Hooper SB, Gill AW, Allison BJ, McLean CJ, Nitsos I, et al. Cardiovascular and pulmonary consequences of airway recruitment in preterm lambs. J Appl Physiol. (2009) 106:1347–55. doi: 10.1152/japplphysiol.91445.2008
41. Crossley KJ, Morley CJ, Allison BJ, Polglase GR, Dargaville PA, Harding R, et al. Blood gases and pulmonary blood flow during resuscitation of very preterm lambs treated with antenatal betamethasone and/or Curosurf: effect of positive end-expiratory pressure. Pediatr Res. (2007) 62:37–42. doi: 10.1203/PDR.0b013e31806790ed
42. Kitchen MJ, Siew ML, Wallace MJ, Fouras A, Lewis RA, Yagi N, et al. Changes in positive end-expiratory pressure alter the distribution of ventilation within the lung immediately after birth in newborn rabbits. PLoS ONE. (2014) 9:e93391. doi: 10.1371/journal.pone.0093391
43. Martherus T, Oberthuer A, Dekker J, Kirchgaessner C, van Geloven N, Hooper SB, et al. Comparison of two respiratory support strategies for stabilization of very preterm infants at birth: a matched-pairs analysis. Front Pediatr. (2019) 7:3. doi: 10.3389/fped.2019.00003
44. Berglund JE, Halden E, Jakobson S, Landelius J. Echocardiographic analysis of cardiac function during high PEEP ventilation. Intensive Care Med. (1994) 20:174–80. doi: 10.1007/BF01704696
45. Abdel-Hady H, Matter M, Hammad A, El-Refaay A, Aly H. Hemodynamic changes during weaning from nasal continuous positive airway pressure. Pediatrics. (2008) 122:e1086–90. doi: 10.1542/peds.2008-1193
46. Kashyap AJ, Dekoninck PLJ, Rodgers KA, Thio M, McGillick EV, Amberg BJ, et al. Antenatal sildenafil treatment improves neonatal pulmonary hemodynamics and gas exchange in lambs with diaphragmatic hernia. Ultrasound Obstet Gynecol. (2019) 54:506–16. doi: 10.1002/uog.20415
47. Brouwer E, Te Pas AB, Polglase GR, McGillick EV, Bohringer S, Crossley KJ, et al. Effect of spontaneous breathing on umbilical venous blood flow and placental transfusion during delayed cord clamping in preterm lambs. Arch Dis Child Fetal Neonatal Ed. (2020) 105:26–32. doi: 10.1136/archdischild-2018-316044
48. Australian Resuscitation Council NZRC. ANZCOR Guideline 13.4 – Airway Management and Mask Ventilation of the Newborn Infant. (2016). Available online at: https://resus.org.au/guidelines/ (accessed August 13, 2020).
49. Polglase GR, Wallace MJ, Grant DA, Hooper SB. Influence of fetal breathing movements on pulmonary hemodynamics in fetal sheep. Pediatric Res. (2004) 56:932–8. doi: 10.1203/01.PDR.0000145254.66447.C0
50. van Vonderen JJ, Roest AA, Walther FJ, Blom NA, van Lith JM, Hooper SB, et al. The influence of crying on the ductus arteriosus shunt and left ventricular output at birth. Neonatology. (2014) 107:108–12. doi: 10.1159/000368880
51. te Pas AB, Davis PG, Kamlin CO, Dawson J, O'Donnell CP, Morley CJ. Spontaneous breathing patterns of very preterm infants treated with continuous positive airway pressure at birth. Pediatr Res. (2008) 64:281–5. doi: 10.1203/PDR.0b013e31817d9c35
52. Sobotka KS, Hooper SB, Allison BJ, Te Pas AB, Davis PG, Morley CJ, et al. An initial sustained inflation improves the respiratory and cardiovascular transition at birth in preterm lambs. Pediatr Res. (2011) 70:56–60. doi: 10.1203/PDR.0b013e31821d06a1
53. Lang JA, Pearson JT, Binder-Heschl C, Wallace MJ, Siew ML, Kitchen MJ, et al. Increase in pulmonary blood flow at birth: role of oxygen and lung aeration. J Physiol. (2016) 594:1389–98. doi: 10.1113/JP270926
54. Mulrooney N, Champion Z, Moss TJ, Nitsos I, Ikegami M, Jobe AH. Surfactant and physiologic responses of preterm lambs to continuous positive airway pressure. Am J Respir Crit Care Med. (2005) 171:488–93. doi: 10.1164/rccm.200406-774OC
55. Oei JL, Finer NN, Saugstad OD, Wright IM, Rabi Y, Tarnow-Mordi W, et al. Outcomes of oxygen saturation targeting during delivery room stabilisation of preterm infants. Arch Dis Child Fetal Neonatal Ed. (2017) 103:F1–9. doi: 10.1136/archdischild-2016-312366
56. van Henten TMA, Dekker J, Te Pas AB, Zivanovic S, Hooper SB, Roehr CC. Tactile stimulation in the delivery room: do we practice what we preach? Arch Dis Child Fetal Neonatal Ed. (2019) 104:F661–2. doi: 10.1136/archdischild-2018-316344
57. Beavo JA, Rogers NL, Crofford OB, Hardman JG, Sutherland EW, Newman EV. Effects of xanthine derivatives on lipolysis and on adenosine 3′,5′-monophosphate phosphodiesterase activity. Mol Pharmacol. (1970) 6:597–603.
Keywords: preterm, CPAP, pulmonary blood flow, cerebral blood flow, jugular venous pressure, birth
Citation: Martherus T, Crossley KJ, Rodgers KA, Dekker J, Demel A, Moxham AM, Zahra VA, Polglase GR, Roberts CT, te Pas AB and Hooper SB (2021) High-CPAP Does Not Impede Cardiovascular Changes at Birth in Preterm Sheep. Front. Pediatr. 8:584138. doi: 10.3389/fped.2020.584138
Received: 16 July 2020; Accepted: 22 December 2020;
Published: 22 January 2021.
Edited by:
Charles Christoph Roehr, University of Oxford, United KingdomReviewed by:
Mona Alotaibi, University of California, San Diego, United StatesFrans Walther, University of California, Los Angeles, United States
Copyright © 2021 Martherus, Crossley, Rodgers, Dekker, Demel, Moxham, Zahra, Polglase, Roberts, te Pas and Hooper. This is an open-access article distributed under the terms of the Creative Commons Attribution License (CC BY). The use, distribution or reproduction in other forums is permitted, provided the original author(s) and the copyright owner(s) are credited and that the original publication in this journal is cited, in accordance with accepted academic practice. No use, distribution or reproduction is permitted which does not comply with these terms.
*Correspondence: Tessa Martherus, dC5tYXJ0aGVydXNAbHVtYy5ubA==
†These authors have contributed equally to this work and share senior authorship