- 1Department of Radiation Oncology, University Medical Center Utrecht, Utrecht, Netherlands
- 2Princess Máxima Center for Pediatric Oncology, Utrecht, Netherlands
- 3Department of Radiation Oncology, City of Hope National Medical Center and Beckman Research Institute, Duarte, CA, United States
- 4Alfred Health Radiation Oncology, The Alfred Hospital, Melbourne, VIC, Australia
- 5Department of Radiation Oncology, University Hospital, Ludwig Maximilian University of Munich, Munich, Germany
- 6Department of Radiation Oncology, Fondazione IRCCS Policlinico San Matteo and University of Pavia, Pavia, Italy
- 7Division of Biostatistics and Bioinformatics, Department of Epidemiology and Public Health, University of Maryland School of Medicine, Baltimore, MD, United States
- 8Stem Cell Transplantation Unit, Clinica Paediatrica Università degli Studi di Milano Bicocca, Monza, Italy
- 9Department of Oncology, Rigshospitalet, University of Copenhagen, Copenhagen, Denmark
Total body irradiation (TBI) has been a pivotal component of the conditioning regimen for allogeneic myeloablative haematopoietic stem cell transplantation (HSCT) in very-high-risk acute lymphoblastic leukaemia (ALL) for decades, especially in children and young adults. The myeloablative conditioning regimen has two aims: (1) to eradicate leukaemic cells, and (2) to prevent rejection of the graft through suppression of the recipient's immune system. Radiotherapy has the advantage of achieving an adequate dose effect in sanctuary sites and in areas with poor blood supply. However, radiotherapy is subject to radiobiological trade-offs between ALL cell destruction, immune and haematopoietic stem cell survival, and various adverse effects in normal tissue. To diminish toxicity, a shift from single-fraction to fractionated TBI has taken place. However, HSCT and TBI are still associated with multiple late sequelae, leaving room for improvement. This review discusses the past developments of TBI and considerations for dose, fractionation and dose-rate, as well as issues regarding TBI setup performance, limitations and possibilities for improvement. TBI is typically delivered using conventional irradiation techniques and centres have locally developed heterogeneous treatment methods and ways to achieve reduced doses in several organs. There are, however, limitations in options to shield organs at risk without compromising the anti-leukaemic and immunosuppressive effects of conventional TBI. Technological improvements in radiotherapy planning and delivery with highly conformal TBI or total marrow irradiation (TMI), and total marrow and lymphoid irradiation (TMLI) have opened the way to investigate the potential reduction of radiotherapy-related toxicities without jeopardising efficacy. The demonstration of the superiority of TBI compared with chemotherapy-only conditioning regimens for event-free and overall survival in the randomised For Omitting Radiation Under Majority age (FORUM) trial in children with high-risk ALL makes exploration of the optimal use of TBI delivery mandatory. Standardisation and comprehensive reporting of conventional TBI techniques as well as cooperation between radiotherapy centres may help to increase the ratio between treatment outcomes and toxicity, and future studies must determine potential added benefit of innovative conformal techniques to ultimately improve quality of life for paediatric ALL patients receiving TBI-conditioned HSCT.
Introduction
Since the 1970s total body irradiation (TBI) is considered to be a cornerstone of myeloablative conditioning for haematopoietic stem cell transplantation (HSCT) in children. It has been used in combination with chemotherapy as conditioning regimen both in autologous and allogeneic HSCT for malignant and non-malignant diseases (1). However, it gradually became clear that HSCT survivors suffered from various late adverse effects, many of which related to TBI (2–6). As HSCT strategies improved and evolved over time, and reduction of late sequelae was warranted, chemotherapy-only conditioning schedules (chemoconditioning) became the mainstay for most indications; the use of myeloablative TBI was limited mainly to patients with high-risk haematologic malignancies in the allogeneic setting (7–10). For most paediatric acute myeloblastic leukaemia (AML) HSCT indications, chemoconditioning gained preference over TBI-based conditioning (11–14). In children with very-high-risk acute lymphoblastic leukaemia (ALL), studies consistently showed superior survival outcomes of TBI-based conditioning (15–19).
The aspiration to reduce acute and long-term effects after HSCT—especially in developing children—has motivated radiation oncologists to seek out improvements in TBI performance. For many years, myeloablative TBI was mostly given as a single fraction of up to 10 Gy combined with cyclophosphamide (20, 21). Gradually, studies showed decreased toxicities and equal or improved survival with fractionated TBI (22–24), and this has become the standard. However, institutions have developed site-specific TBI setups and techniques, making practises heterogeneous (25–28). With technological advances, general radiation treatments have evolved into highly conformal intensity-modulated techniques delivering high doses to treatment volumes while increasingly sparing the surrounding tissues. For TBI, however, most centres still use two-dimensional (2D) conventional techniques with opposing beams that capture the entire body while shielding certain organs at risk (OAR) (27, 28) (Figure 1). This technique tends to deliver heterogeneous doses throughout the body while shielding also blocks bone marrow compartments. Several centres have introduced highly conformal techniques that offer better dose homogeneity while allowing more options to spare OAR, albeit with higher dose rates than classical setups (29–31). More targeted radiotherapy strategies such as total marrow irradiation (TMI), total lymphoid irradiation (TLI), and total marrow and lymphoid irradiation (TMLI) allow dose escalation to the bone marrow and/or lymphoid volumes of high-risk ALL patients while reducing doses in the remainder of the body. Clinical studies to establish the role of TMLI in HSCT-conditioning are ongoing (32).
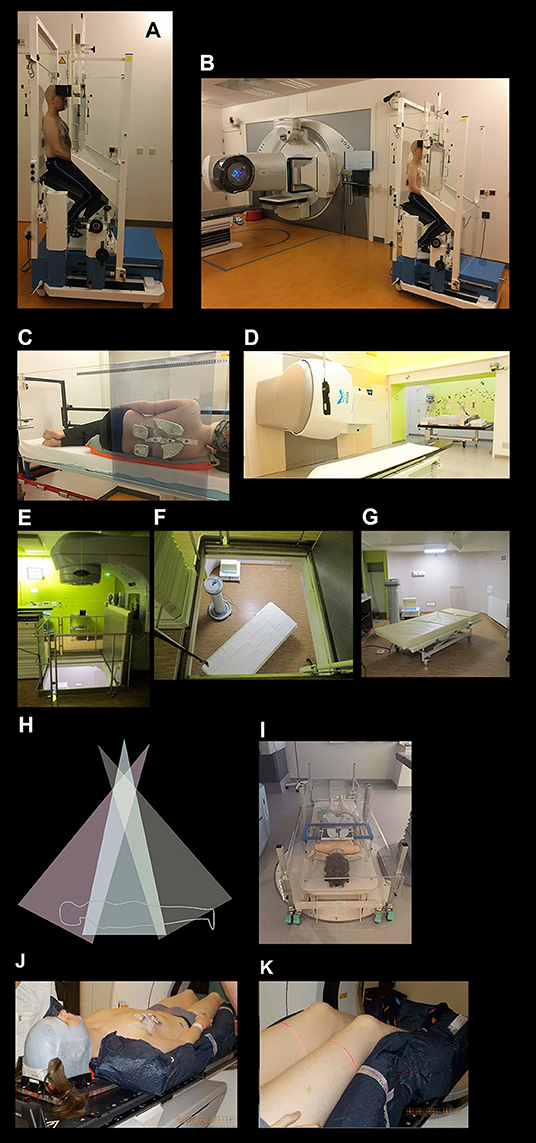
Figure 1. Total body irradiation setup examples. (A,B) A patient in an institution-developed TBI “chair” setup for opposed anterior/posterior (AP-PA) dose delivery with acrylic beam spoilers in front of and behind the patient; the chair is rotated 180° halfway through each fraction; shielding of lungs, kidneys, and lenses is performed with individually moulded cerrobend blocks. (C) A patient in an institution-developed TBI “bed” setup for AP-PA dose delivery in the lateral decubitus position, with beam spoilers; the patient is rotated 180° halfway through each fraction; shielding of lungs, kidneys, and lenses is performed with individually moulded cerrobend blocks. (D) A patient in an institution-developed TBI “bed” setup for lateral dose delivery in the supine position, with beam spoilers; the bed is rotated 180° halfway through each fraction and there is shielding of lungs. (E–G) An institution-developed TBI “bed” setup for AP-PA dose delivery where the linear accelerator gantry is positioned one floor above the patient, and the patient is rotated from the supine to prone position halfway through a fraction. (H,I) A sweeping-beam TBI “bed” setup for AP-PA dose delivery where the linear accelerator gantry is positioned ±2 m above the patient and sweeps stepwise in an arc over the entire body, delivering the dose in multiple static (up to 20) positions, thereby increasing dose homogeneity; the patient is rotated from the supine to prone position halfway through a fraction; beam spoilers cover the patient, with individually moulded lung blocks placed below the spoiler. (J,K) A patient in a highly conformal isocentric technique treatment position (e.g., VMAT TBI, TomoTherapy TBI, TMI, or TMLI) lying supine in a body-length vacuum bag and open head mask for secure positioning during treatment; as the gantry rotates around sequential isocentres in the body and table translations take place. TMI, total marrow irradiation; TMLI, total marrow and lymphoid irradiation; VMAT, volumetric-modulated arc therapy. Images (E–G) courtesy of S. Supiot, Institut de Cancérologie de l'Ouest, Nantes St. Herblain, France. Images (H,I) courtesy of L. Sim, Radiation Oncology Princess Alexandra Raymond Terrace, Brisbane, Queensland, Australia.
Since the superiority of including TBI in conditioning regimens prior to HSCT for very-high-risk ALL paediatric patients has been reinforced by the results of the For Omitting Radiation Under Majority age (FORUM) trial (19), it is timely to review TBI application and rationale for these patients and to gauge future directions.
Studies of TBI-Based Conditioning for all
TBI has been the most frequently applied myeloablative conditioning for HSCT in patients with ALL. Most centres now avoid TBI in children below the age of 3 years because of increased side effects especially on the young developing brain. Prior to the FORUM trial, there was remaining debate over whether non-inferior conditioning in children, adolescents, or young adults could be achieved without TBI (33).
Davies et al. compared HSCT outcomes in children with ALL transplanted from human leukocyte antigen (HLA)-identical siblings who received cyclophosphamide plus TBI conditioning (n = 451) vs. those who received oral busulfan plus cyclophosphamide conditioning (n = 176) (16). The 3-year probability of overall survival (OS) was 55% [95% confidence interval (CI) 50–60%] with TBI and cyclophosphamide and 40% (95% CI 32–48%) with busulfan and cyclophosphamide (p = 0.003), with a higher risk of treatment failure (relapse or death) in the busulfan group [relative risk (RR) 1.39; p = 0.017].
A retrospective European Society for Blood and Marrow Transplantation (EBMT) study assessed the role of TBI in patients aged 2–18 years who were transplanted for ALL in remission with a bone marrow or peripheral blood graft from a compatible donor, and compared patients who had received TBI-based myeloablative conditioning (n = 1,336) with patients who had been transplanted after chemoconditioning (n = 210) between 2000 and 2012 (18). An inferior outcome was reported after chemoconditioning for patients with ALL in second complete remission (CR2), with a 1.75-fold higher risk of death, 1.86-fold higher risk of any failure and a 1.9-fold higher risk of relapse compared with those receiving TBI-conditioning. Conversely, no difference could be detected for those transplanted in first complete remission (CR1). Nevertheless, as TBI was the standard regimen, a selection bias could have affected regimen allocation, with patients who had experienced severe toxicities and infections prior to HSCT being more likely to being allocated chemoconditioning. Furthermore, logistical issues could have limited timely access to fractionated TBI. Similar results were reported when cord blood units were used as the stem cell source, with TBI being associated with a lower risk of relapse than chemoconditioning (34). Within the Centre for International Blood and Marrow Transplant Research (CIBMTR), attempts to decrease the risk of relapse by intensifying the conditioning of 12 Gy TBI and cyclophosphamide—which included increasing the TBI dose to 13.2–14 Gy and/or adding a second chemotherapeutic agent—were not effective (35).
In the recent international, multicentre, randomised FORUM trial in high-risk ALL patients aged 4–21 years at HSCT, 2-year OS was 91% following conditioning with fractionated 12 Gy TBI and etoposide (n = 212) compared with 75% following chemoconditioning (a combination of fludarabine, thiotepa, and either treosulfan of busulfan; n = 201; p < 0.0001); the 2-year cumulative incidence of relapse and treatment-related mortality were 33 vs. 12% (p < 0.0001) and 9 vs. 2% (p = 0.0269), respectively (19). The median follow-up at interim analysis was relatively short (2.1 years), but the advantage of TBI was striking throughout all subgroups and randomisation was discontinued, as the stopping rule was reached. Whether longer follow-up and associated insights regarding late sequelae will lead to reassessment of the benefit of TBI remains a question.
Efforts to provide equal outcomes with reduced TBI doses, adapted radiotherapy target volumes or the exclusion of TBI are ongoing. For now, however, based on the results of the FORUM study, TBI (12 Gy in six fractions, given twice per day) is the standard of care for ALL patients ≥4 years old who are eligible for HSCT and have no absolute contraindication to radiotherapy.
The Importance of Minimal Residual Disease
Minimal residual disease (MRD) consists of a small number of leukaemia cells in the bone marrow detectable by flow cytometry, real-time quantitative polymerase chain reaction (RT-qPCR) or next-generation sequencing (NGS) below a level that can be detected morphologically. MRD is recognised as the strongest independent prognostic factor for disease relapse and survival in frontline and relapse ALL treatment, as well as in the transplant setting (36, 37). Most current protocols stratify patients according to response to treatment, including MRD, which, besides guiding treatment decisions, maintains its predictive value (37–39).
The predictive role of pre- and post-HSCT MRD invariably stands throughout ALL patient groups (38), despite the fact that MRD data are mainly used in real time to modulate immunosuppression tapering and/or discontinuation, possibly associated with the use of cell therapy (donor lymphocyte infusion, cytokine-induced killer cells) (40), or targeted therapy (blinatumomab, chimeric antigen receptor T cells) in the attempt to reduce the relapse risk (41–44). The effectiveness of such immunomodulation cannot be assessed.
It has been suggested that the decision regarding the conditioning regimen could be based on MRD, as defined by means of next generation sequencing (NGS) (45). Such an approach is evaluated in the ongoing prospective study which performs a non-TBI based conditioning regimen in patients ≤25 years old diagnosed with B-cell acute ALL who are pre-HSCT NGS MRD negative (NCT03509961) (46).
The role of the MRD level prior to and after HSCT in children and adolescents is discussed in depth in the publication by Merli et al. (47) in this supplement of Frontiers in Paediatrics.
Immunosuppressive and ANTI-Leukaemic Effects of TBI
The rationale for inclusion of TBI in the conditioning regimen before HSCT for ALL is two-fold: 1) to eradicate leukaemic cells, and 2) to prevent rejection of allogeneic engraftment through ablation of the recipient's immune system. Radiotherapy targets leukaemic cells in the entire body, including in sanctuary sites where chemotherapy delivery is hampered by perfusion, diffusion and blood-barrier effects. Optimising the immunosuppressive effect of fractionated TBI schedules while sparing normal tissue from injury where possible requires consideration of the combination of total dose, dose rate, fraction size, and overall treatment time. Advances in the clinical radiobiology of TBI inferred from data originating from trials or retrospective data sets have been limited, in contrast to what is the case for many solid malignancies and the associated OAR. This is, in part, explained by the large variability in patient and treatment characteristics within and between studies, as well as by the difficulties in obtaining reliable patient-level dosimetry for tissues and OAR from TBI. All of these difficulties are compounded by the fact that many institutional TBI protocols included numerous temporal adjustments to planning and delivery as well as to the dose-time-fractionation regimens used, which further hamper direct comparisons of disease control and toxicity between series. The lack of consistency in practise patterns, dosimetry and reporting of TBI doses among institutions is documented in the recent surveys of practise patterns of paediatric TBI from the European Society for Paediatric Oncology (SIOPE) and Children's Oncology Group (COG) (27, 28). As a result of these obstacles, much of the radiobiological rationale for current TBI regimens is derived from in vitro or experimental animal studies, many dating back to the 1970s and 1980s, and only supported qualitatively by clinical data.
Dose-Fractionation Biology of Leukaemic and Haematopoietic Cells
In vitro radiosensitivity estimates have historically been quantified using the D0 value: the dose required to reduce the surviving cell fraction to 37% on the log-linear part of the dose vs. cell-survival curve. Normal haematopoietic cells (mainly lymphocytes in most studies) have D0 values between 0.5 and 1.4 Gy, indicating overall high radiosensitivity (48–50). D0 values for peripheral blood cells in vivo tend to be somewhat higher than in vitro values. Studies in animals suggest that there is a small subpopulation of haematopoietic stem cells with higher radioresistance than the overall population (51). In a clinical study, Shank et al. studied peripheral blood cell survival kinetics during hyperfractionated TBI (13.2 Gy in 11 fractions of 1.2 Gy, given three times a day) given before cyclophosphamide as HSCT conditioning in 14 children in remission for ALL and found a D0 range of 3.7–5.4 Gy for peripheral blood lymphocytes, without a shoulder in the survival curve (see below), and a D0 of 10 Gy for granulocytes (52). Absolute nucleated cell concentration in the bone marrow had dropped to 7–44% of base levels only on the last TBI-day, while marrow myeloid elements decreased continuously. Myeloablative TBI has a prolonged effect on bone marrow recovery, with a 30% decreased marrow cellularity even at 1 year post-HSCT (53).
Leukaemic cell populations have an overall high radiosensitivity with median D0 values of 0.74 Gy, usually with a minimal or absent shoulder in the survival curve (54). Specific leukaemic cell types, however, show a wide range of in vitro radiosensitivities: wider than that of normal haematopoietic cells (55–59). In a study of 74 children with ALL, B-lineage ALL types proved to be more radioresistant than T-lineage ALL types (60). Monzen et al. performed mRNA expression analysis on a model of radioresistant acute promyelocytic leukaemia cells and found that specific changes in intracellular genetic network profiles were associated with radioresistance in their AML cell line (61).
Fractionation sensitivity, i.e., the total dose adjustment required to maintain a given level of biological effect after changing the dose per fraction or the dose rate, is generally quantified using the α/β value of the linear quadratic (LQ) model (62). Higher values of α/β indicate less sensitivity to dose per fraction/dose rate. Historically, this was quantified by the “shoulder” of the in vitro dose–survival curve: a large shoulder indicates large fractionation sensitivity, corresponding to a low α/β value in the LQ model.
Early studies of haematopoietic cells generally showed small shoulders of in vitro dose–survival curves, suggesting a limited effect of dose-fractionation (63). In vitro studies on ALL cells retrieved from 74 children found that, contrary to previous notions, about a third of B- and T-lineage ALL cell clonogens display a shoulder in the survival curve and possess sublethal radiation damage repair capacity, which is most relevant during fractionated radiotherapy (64). Uckun et al. (64) estimated α/β values ranging between 0 and 101 Gy, and two-thirds of progenitor cells from 34 evaluated cases had an α/β value <5 Gy, indicating a substantial effect of dose per fraction.
A large dose per fraction and/or increased dose rate of TBI will counter the recovery of leukaemic cells between fractions (65, 66) but, obviously, this should be balanced against the potential sparing of normal tissue effects from low fraction sizes/low dose rate. Wheldon and Barrett devised a mathematical model for leukaemic cell kill based on 27 fractionated TBI schedules that are iso-effective for interstitial pneumonitis (IP) risk and applied this to a hypothetical patient population with diverse leukaemic intrinsic radiosensitivities (67). They surmised that many of the current TBI schedules have a similar propensity for leukaemia cure in unselected patient populations. Ideally, a patient's individual leukaemic cell radiosensitivity should be known to select their optimal TBI schedule. However, this would only generate a modest improvement in general cure probability and would benefit mainly outliers with relatively low leukaemic radiosensitivity (67). As research into cellular radiobiology predictive assays generally has failed to impact clinical radiotherapy in other indications, it seems unlikely that in vitro cellular assays of the individual radiosensitivity of haematopoietic volumes and leukaemic cell types in a patient before beginning TBI-based conditioning prior to HSCT would be a valuable translational addition to future studies regarding ALL HSCT.
As genotyping increasingly becomes a part of the routine clinical work-up of patients with leukaemia, it is conceivable that putative links between genotypes and the effect of TBI will be discovered in the coming years. Genomics, in particular germ-line single nucleotide polymorphisms, have been studied in 10,000s of radiotherapy patients as a potential cause of inter-individual variability in early and late toxicity after radiotherapy (68). Initial reports were encouraging. However, a large UK validation study in patients with prostate or breast cancer with 2-year clinical assessment of late radiation adverse effects showed that the early literature was dominated by false-positive findings (69). More recently, there is emerging evidence from large studies that sequence alterations may affect adverse events after radiotherapy. Somatic sequence alterations in leukaemic cells could also, in theory, affect the therapeutic effect of TBI. So far, except for a few rare genetic disorders, there are currently no generally accepted and validated genotypes that affect radiotherapy prescriptions in other radiotherapy indications (70).
Clinical Data on TBI Dose-Fractionation Response
In the 1950s, the discovery that stem cell transplantation could counteract acute mortality from the depletion of blood-forming tissues after TBI injury triggered many studies into the application of HSCT against haematologic malignancies and immunodeficiency diseases in particular (71–73). Experiments in mice showed that extremely high lethal TBI doses of 20–50 Gy or higher were needed to sterilise advanced leukaemia in the body (74), and that the graft-versus-leukaemia effect of infused stem cells was therefore essential for cure when lower TBI doses were applied. The first clinical allogeneic HSCTs were performed with TBI-only conditioning and were largely a disappointment because of disease recurrence, non-engraftment, graft-versus-host-disease- (GvHD) and treatment-related death (75). When up to 10 Gy single-fraction TBI was combined with cyclophosphamide, and immunosuppressive and peri-transplantation care evolved, more patients with acute leukaemia survived (20, 24, 76).
However, the acute and late effects of single-fraction TBI, especially for developing children, became an issue of worry. Peters et al. argued that the therapeutic ratio of the radiosensitive normal tissues vs. the immunosuppressive and anti-leukaemic effects of TBI could be improved by decreasing the single-fraction dose rate (which meant an irradiation lasting up to >10 h for patients) or by dose fractionation (77). The latter was confirmed in a randomised trial (78).
Many different fractionation schedules began to be used (79) and it was difficult to evaluate differences in efficacy because of the multifactorial influence of treatment effects, GvHD and toxicities in cohorts of patients with various diseases and age groups (67). Fractionated doses <9–10 Gy would result in non-engraftment and disease relapse (80, 81). In many instances, lung toxicity was found to be the dose-limiting factor at 2-Gy fractionated 16 Gy TBI (82); it was also diagnosed more frequently after single-fraction TBI than after fractionated TBI in leukaemia patients (83–86). For children, other significant TBI effects such as growth inhibition or cataract formation were reduced by TBI fractionation (23, 87). One fractionation schedule that was applied early on was 12 Gy in six fractions given over 3 or 6 days. To optimise the therapeutic ratio, twice-daily fractionation of doses between 1.5 and 2 Gy to doses ≥12 Gy was estimated to be optimal, while more hyperfractionated schedules with three to four fractions daily seem to have worse anti-leukaemic/immunosuppressive effect as well as being impractical in terms of delivery within working hours while giving healthy tissues the aspired 6-hour recovery period between fractions (54, 88–90). Giving 12 Gy TBI in once-daily fractions of 4 Gy increased acute effects such as mucositis (91, 92). A randomised dose-escalation study comparing 12 Gy TBI over 6 days with 15.75 Gy TBI over 7 days displayed a decreased relapse rate after high-dose TBI but increased rate of non-relapse mortality (NRM), ultimately resulting in equal probabilities of survival (93, 94). In a single-centre ALL HSCT cohort, 12 Gy in six fractions over 3 days was deemed the optimal TBI schedule regarding GvHD occurrence and overall prognosis after variations in TBI dose, dose rate and technical setting had been applied during a span of 12 years (95). Another centre—comparing 10 Gy with 12 and 13.2 Gy TBI given over 3 days—concluded that 10 Gy gave the highest 5-year OS benefit (96). One publication compared 16 TBI studies regarding fractionation and dose rate, the combination of which was recalculated into the biologically effective dose (BED) for leukaemic cells and several OAR (e.g., 6 times 2 Gy with a dose rate 0.16 Gy/min gives a BEDleukaemia of 14.2 Gy and BEDlens of 42.8 Gy) (97). A high BEDleukaemia in the fractionated schedules significantly reduced relapse incidence and increased OS. Shielding for lungs, kidneys and lenses was advised to BEDs ≤15, ≤17, and ≤40–45 Gy, respectively.
Hard conclusions regarding TBI fractionation for ALL specifically are difficult to draw from these studies as they cover different patient and disease categories as well as temporal changes in overall and HSCT-specific treatment protocols. The FORUM trial delivered a conditioning of etoposide combined with TBI as a 6 times 2 Gy TBI schedule given over 3 days and lung shielding at 10 Gy (19). For the moment, fractionated TBI schedules giving doses of 12–14 Gy with lung shielding have been adopted as optimal schedules in ALL HSCT by many paediatric radiation oncology centres (27, 28). Nonetheless, continuous reassessment of TBI optimization is needed as pre-HSCT factors improve and new combinations of chemotherapy with lower doses of TBI are researched (98, 99).
TBI Dose Rate
The biologic radiotherapy effect of TBI on cells and tissues depends on their inherent radiosensitivity, the micro-environment, total dose, fractionation, overall treatment time, dose rate, dose homogeneity, TBI setup, patient and disease characteristics, and other therapies. TBI with an extended source-surface distance (SSD) setup is institution specific, precluding normalisation of TBI dose and dose rate (100). Published works may report dose rate at the prescription point of a patient's midplane, in the lung or in air. Reported values may represent measured or calculated data, and measurement and calculation methods can differ between centres. These differences must be considered when comparing and interpreting published data. In older studies, TBI was often delivered with cobalt teletherapy and source decay exposed the analysed cohorts to varying dose rates through time (81). In modern extended SSD TBI, the dose rate is chiefly determined by the SSD (through the inverse square law) and the linear accelerator dose rate.
In the 1970s, the most commonly used TBI schedule was 8–10 Gy given at a low dose rate over several hours, to balance treatment effect against toxicities (101). Fractionated TBI was recommended to improve the therapeutic ratio. For leukaemic cell kill and allogeneic engraftment success, fractionated TBI with a higher dose rate is preferable to a lower dose rate (77, 89). In preclinical studies, increased dose rates during TBI improved allogeneic engraftment (102–104). In clinical studies, dose rates of ≤0.04 Gy/min showed increased leukaemia relapses in patients given TBI doses of 8.4–12.5 Gy in 3 days (81). Bone marrow displays a marginally increased sensitivity for fractionation with 1.2- and 2-Gy fractions, and little effect of higher dose rates of 0.8 Gy/min when compared with 0.05 Gy/min in single-fraction TBI (105). At dose rates >0.3 Gy/min, no extra effect for haematopoietic cell damage is expected (106).
Multiple studies have explored the effect of TBI dose rate on toxicity. In preclinical studies exploring single-fraction TBI, dose rate changes in a lower dose rate range had a much greater influence on toxicity occurrence in late responding tissues (especially the lung, kidney and liver), than dose rate changes in the higher dose rate range (101, 106). For late non-hematopoietic tissue effects, this resulted in e.g., an iso-effective dose factor of ±2.4 for a dose rate of 0.02 Gy/min, ±1.5 for a dose rate of 0.1 Gy/min, and ±1.0 for a dose rate range of 1 to >10 Gy/min. Experiments in mice indicate that average dose rate may be more relevant for lung tissue toxicity than instantaneous dose rate (107). At midplane dose rates ≤0.15 Gy/min, fractionation of total dose had a greater sparing effect on late-responding tissues than reduction of dose rate (23, 106). High dose rates of 0.75 Gy/min induced more gastrointestinal damage in dogs after TBI than dose rates down to 0.021 Gy/min, but this effect could be compensated for by fractionation (108). In dogs given autologous HSCT, acute TBI tolerance doses measured as 50% mortality at 7 days were comparable between single-fraction and fractionated TBI (2 Gy three times daily) at exposure rates of 0.02–0.1 Gy/min, but fractionation benefit occurred at a dose rate of 0.2 Gy/min, with tolerance doses of 10.56 Gy (95% CI 9.39–11.74) vs. 13.2 Gy (95% CI 11.36–15.05), respectively (109). In mice, low dose rates of 0.05 Gy/min as compared with 0.8 Gy/min, had a highly protective effect on late lethality in single dose TBI, but this effect diminished or disappeared when TBI was given in 1.2- or 2-Gy fractions (105). These studies exemplify that influence of dose rate on toxicity induction diminishes through fractionation, that fractionation increases tolerance of normal tissues, that dose rate changes in the lower dose rate range (e.g., <0.15 Gy/min) influence late toxicity effects more than dose rate changes in the higher dose rate range (e.g., >0.3 Gy/min), and that average dose rate may be more relevant for biological effect correlation than instantaneous dose rate.
In a BED calculation of 16 clinical studies, it was demonstrated that different dose rates at ≤0.15 Gy/min for fractionated schedules do not induce large BED differences for leukaemic cells and OAR, in contrast to single-fraction schedules (97). Most clinical research into dose rate effects has focused on lung toxicity. In 202 acute leukaemia patients, 8 times 1.65 Gy fractionated TBI given at dose rates of >0.15 Gy/min induced significantly more IP and worse OS than dose rates of ≤0.15 Gy/min when lungs were only shielded by the arms in a bilateral beam setup (IP incidence: 29 vs. 10%, respectively, p < 0.01; 1-year OS: 60 vs. 76%, respectively, p = 0.01) (110). In studies using fractionated conventional TBI, the impact of dose rates up to 0.15 Gy/min becomes negligible for IP development, as long as the registered lung dose does not exceed 8–9 Gy (111–114). At dose rates of 0.15–0.21 Gy/min, IP risk increased with increasing dose rates in studies with lung shielding of 10–12 Gy for TBI schedules of 12 Gy in 6–8 fractions (115, 116). In a meta-analysis including TBI lung dose rates of 0.03–0.41 Gy/min, dose rate was not significantly associated with IP (117). A high dose rate affects late renal damage inasmuch as it can increase BED to levels above tolerance doses, generating the need for kidney shielding (118–120). Cataract development is related to dose rate, with increasing cataract risk at increasing dose rates between 0.02 and 0.56 Gy/min (121, 122). Although not repeated in all publications, clinical studies show that for dose rate ranges of e.g., 0.04–0.4 Gy/min in a conventional SSD TBI setup, increasing the dose rate increases risk of late toxicities in lungs, kidneys and lenses even for fractionated schedules, generating a need for adequate organ shielding.
Momentarily disregarding the numerous influential variables and inconsistent reports regarding the issue of dose rate, dose rates between 0.04 and 0.15 Gy/min seem to be the most frequently reported option for extended-SSD, fractionated conventional TBI schedules in paediatric ALL patients, albeit with appropriate OAR shielding. Regarding immunosuppressive and anti-leukaemic cell effect, the higher end of this spectrum may be preferable. For more staunch multicentre conclusions, we need comparable schedules, uniform specifications, and complete reporting of all relevant parameters including applied dose rates.
Patient comfort is a factor as well. Delivery of 2-Gy fractions at 0.04 Gy/min requires 50 min beam-on time and motionless patient positioning, which would mean more indications for multiple sedations of >1 h in children.
Improved dose homogeneity and specific OAR dose reduction can be achieved with highly conformal TBI techniques. This, along with fractionation, may allow for more favourable toxicity profiles even with a high instantaneous dose rate. Low dose rates are preserved with an image-guided intensity-modulated radiotherapy (IMRT) technique at extended SSD, deriving midplane dose rates of 0.14–0.19 Gy/min (123). First experiences with this technique show encouraging results for outcome and lung/kidney toxicity, with a 15% dose reduction at these organs (124). With highly conformal source-to-axis distance techniques such as TomoTherapy (a device combining a helical computed tomography (CT) scanner and a linear accelerator) and volumetric-modulated arc therapy (VMAT—rotational IMRT delivered on a standard linear accelerator), instantaneous dose rates are inherently higher (e.g., 0.2 to >10 Gy/min) and are variable during treatment (125–127). The first experiences with TomoTherapy and VMAT TBI (with overall instantaneous dose rates of ±13 Gy/min and ±0.31 Gy/min and instantaneous dose rates around the lung of ±8.4 and ±0.11 Gy/min, respectively), showed promising results in 197 children with regard to outcome and toxicity profiles (128). Centres can opt for a decrease of monitor unit output at the level of e.g., the lungs or pelvis to achieve average dose rates of even <0.06 Gy/min if desired (125, 126). Fractionated TMLI, with greater sparing of dose-limiting OAR, may provide a means to preserve immunogenic and anti-leukaemic effects while conveying highly acceptable toxicity profiles with high instantaneous dose rate (32, 129).
TBI and Hypoxia
Hypoxia as a cause of radioresistance is a well-known problem in rapidly proliferating solid tumours which outgrow their blood supply. It has not gained much attention in leukaemia research. However, it turns out that the microenvironment in the deeper peri-sinusoidal bone marrow regions (where most of the long-term haematopoietic stem cells reside) is hypoxic, with O2 levels <10 mmHg (130–132). Moreover, leukaemic cells have been shown to be markedly hypoxic; hypoxia inducible factor-1α (HIF-1α), a molecular marker of hypoxia, was shown to be overexpressed in leukaemic cells in the bone marrow in paediatric patients with ALL (132, 133). Hypoxia induces chemoresistance and may play a role in the maintenance of MRD (134). The level of hypoxia in some leukaemic cells in the bone marrow is sufficient to cause hypoxic radioresistance. However, there are no data to support that this is a significant clinical problem, and, so far, no interventions directed at modifying the hypoxia of leukaemic cells have been proposed.
TBI and Radiotherapy Boost of Sanctuary Sites
The central nervous system (CNS) and the testes are protected by barriers that are difficult to penetrate by systemic treatment and have been shown to act as sanctuaries for leukaemic cells with a high risk of local recurrence. Including TBI in the conditioning regimen has the distinct advantage of reaching these sites with the planned treatment.
Radiotherapy can also deliver a higher dose to precisely defined volumes: a so-called boost. Adding a radiation boost to the sanctuary sites in order to reduce the recurrence risk was performed often in the past. However, the effectiveness of the systemic regimens has improved very significantly, making radiation boosts unnecessary in most cases (135–141).
The risk of CNS relapse after HSCT is very high in patients with residual CNS leukaemia after chemotherapy or in patients who develop a relapse involving the CNS. For these patients, additional CNS-directed radiotherapy is often considered (140, 142). Most often, whole brain radiotherapy has been applied to a cumulative cranial dose of 18–24 Gy (140). However, data indicate that craniospinal irradiation (CSI) may be more effective, which seems logical with leukaemic cells circulating in the cerebrospinal fluid. CSI is given to a cumulative dose of 18 in 2 Gy fractions (143). CNS-directed radiotherapy is given in the days immediately prior to TBI.
With modern systemic therapy for ALL, testicular relapses are rare. A boost is only considered for patients with a very high risk of testicular relapse, typically patients with residual disease after chemotherapy or who develop testicular recurrence. The scrotal content including both testes (or the contralateral testis after orchiectomy) is irradiated. If only the contralateral testicle with no evidence of disease is present, a single dose of 4 Gy is often given; however, if one or both testes are clinically involved, the cumulative dose (together with TBI) is 18–24 Gy given in 2 Gy fractions in the days immediately prior to TBI.
TBI Toxicity
Survivors after HSCT can develop morbidities in any organ system and have higher morbidity and mortality rates than those observed in the general population or in non-transplanted childhood cancer survivors (5, 6, 144–146). Very young children (aged below 3–4 years) are more prone to developing serious side effects from HSCT and TBI-based conditioning (4, 147, 148). Concerns are i.e., more negative effects on neurocognition, growth, endocrine and metabolic functioning and second malignancies, and many centres now avoid TBI in these young patients (27). Radiotherapy can cause toxicities depending on patient-, tissue-, disease-, treatment-, dose-, and location-related factors (149). Although many factors are at play in the establishment of HSCT sequelae, TBI-based conditioning causes more late effects than chemoconditioning (4, 5, 150). Within the French Leucémies de l'Enfant et l'Adolescent (LEA) cohort, at a median follow-up of 10.1 years, the 174 patients who received TBI reported more complications than the 66 patients conditioned with busulfan during the same time period (3.01 vs. 2.35, respectively, p = 0.03) (151).
Since late effects of HSCT will be explored in another review within the current Frontiers in Paediatrics supplement, this chapter will focus on fractionated TBI effects. Table 1 narrates several fractionated TBI-related sequelae. General observations from the literature are given as well as noteworthy specific articles. Consequences/recommendations for TBI, or consequences after TBI are remarked upon.
Setup and Planning for Conventional and Highly Conformal TBI Techniques
TBI practise worldwide remains varied, with radiotherapy centres typically developing site-specific setups and techniques (25–28, 79, 269, 270). Conventional TBI is mostly delivered using extended SSD techniques (79), where the radiation beam covers a patient's entire body, and delivers a relatively low dose rate in the patient as a consequence of linear accelerator dose rate adjustability and the inverse square dose reduction with distance (Figure 1). Other setups can be multiple parallel or adjacent beams, sweeping beams, a moving couch underneath a static beam, and field-in-field techniques (271–273).
Many large, open-field conventional techniques result in rather heterogeneous dose distributions, delivering between <80% to even >120% of prescribed doses (Figure 2B), although efforts are made to reduce heterogeneity to within 10%, according to guidelines (e.g., the American Association of Physicists in Medicine guidelines, Report No. 17) (274). The last decade has seen nascent implementation of highly conformal isocentric techniques (where the radiation gantry rotates around the patient on the treatment couch), with the intention to improve dose distribution homogeneity and to reduce the dose to OAR.
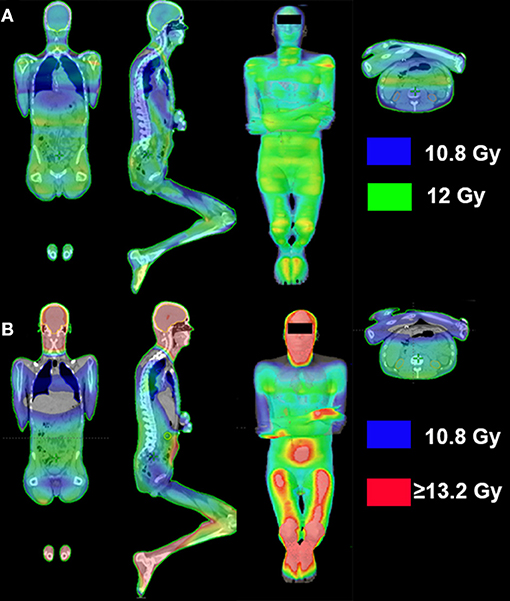
Figure 2. Conventional vs. SSD IMRT-planned total body irradiation dose distribution. (A) Computed tomography (CT)-planned, image-guided intensity-modulated radiotherapy (IMRT) dose distribution with lateral-beam setup at source-surface distance (SSD) (123); dose reductions were planned over lungs, and kidneys. (B) CT-planned two-dimensional conventional total body irradiation dose distribution with a lateral-beam setup, with lung dose reduction using lung blocks; the isowash-depicted dose range in the images represents 90% (10.8 Gy; blue) to ≥110% (≥13.2 Gy; red) of the prescribed dose.
Extended SSD Treatments
The Use of a Treatment Planning System
Several clinics deliver TBI plans calculated without the use of a treatment planning system (TPS) (25). These non-TPS based techniques may have remained unchanged for decades and do not allow for the determination of dose-volume histograms of the body or the OAR to be evaluated the way they would be in mainstream radiotherapy practise. Only large open-field TBI treatments should be calculated by these manual workflow methods. It is worth noting that dose estimates made without the use of a TPS may be quite inaccurate: this makes interpretation and comparison of older published outcomes such as dose-response relationships, dose rates, and normal tissue tolerances difficult.
If an isocentrically commissioned TPS is used at extended SSDs, the accuracy of the TPS must be verified for that specific geometry because the beam may have a different energy spectrum, resulting in a change in the depth-dose distributions and a larger component of in-room scatter to the patient dose (275). If verified under extended SSD conditions, an isocentric TPS can be used to calculate dose distributions from more complex techniques such as “step and shoot” IMRT (123, 155, 276) (Figure 2A) or extended SSD VMAT (277, 278).
Beam Angles, Spoilers, and Tissue Compensators
In extended SSD TBI, beams are typically delivered using an opposed anterior-posterior (AP-PA) technique, bilateral technique (Figure 2B), or combination of the two (271). Recent data showed that the use of a solely bilateral technique in children is disadvantageous since it results in higher lung doses and decreased survival (111).
Also in extended SSD TBI, a beam spoiler, usually a 1–2 cm thick acrylic screen that is placed in front of the patient, is typically used to counter the skin- and subcutaneous tissue-sparing effect of photon beams (279) (Figure 1). Depending on the protocol, tissue compensators that provide tissue-equivalent dose attenuation may be required to improve dose homogeneity across narrow body sections (155).
Lung Shielding
In TBI delivered with large open fields, the lung dose will be greater than the dose to the rest of the body because of the lower density of lung tissue (280). Shielding can be used to reduce the lung dose to the prescribed dose (280–282) or below the prescribed dose (159, 281, 283, 284) and may be achieved using metallic blocks (159, 284) or multi-leaf collimators with an IMRT setup (123). Unavoidably, lung shielding also reduces the dose to the target tissues surrounding the lungs, such as bone marrow in the ribs or mediastinal lymph nodes. The dose to these tissues may be increased by electron boost fields and mediastinal photon fields, respectively (285, 286).
Another issue when using a TPS is that it may not account for the scattered electrons from the non-shielded areas (287), which may increase the actual lung dose considerably. However, TPS algorithms have evolved. The differences between dose distributions calculated by the pencil beam and anisotropic analytical algorithms can be considerable (288), and thus discrepancies between measured doses and doses calculated with a pencil-beam algorithm may not be relevant to modern practise.
Shielding of Other Organs
While lung shielding for paediatric TBI delivery is common practise for many clinics (Figures 1C,I) (27, 28), shielding of other organs occurs infrequently. However, shielding should be considered for kidneys and lenses in children (Figure 1C). Dose-effect evaluation of 14 published cohorts produced a kidney BED tolerance threshold of 16 Gy (195). This report and others concluded that kidney shielding is required to avoid post-TBI CRD for almost all myeloablative regimens (192). Eye shielding for cataract reduction has been discussed in several papers (121, 122, 151, 160, 193). Eye shielding to BED <40 Gy reduces the risk of severe cataracts and increases latency time of cataract formation (195, 196).
Individual centres have conventional TBI setup protocols for shielding of the heart, liver (170) and even ovaries (289) but these measures are reported incidentally and no clear recommendation can be given. With highly conformal techniques, centres may choose to deliver reduced doses to multiple OAR, while the bone marrow/lymphoid target volume is adequately covered (31, 124).
Isocentric Highly Conformal TBI Techniques
Isocentrically delivered IMRT TBI requires the use of a TPS. It is a fundamentally different approach to extended SSD TBI because it uses a much higher dose rate and requires field junctioning. Examples of isocentric TBI techniques include TomoTherapy (29, 290–293) and VMAT (30, 125, 127, 294) (Figure 3). These isocentric techniques are seeing nascent clinical implementation in centres around the world, although outcome data from long-term follow-up are yet to be published.
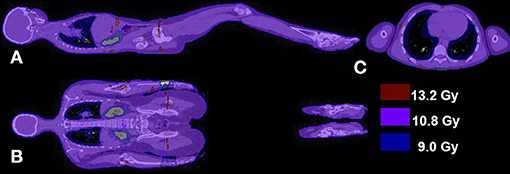
Figure 3. CT-planned VMAT total body irradiation technique dose distribution. Computed tomography (CT)-planned volumetric-modulated arc therapy (VMAT) total body irradiation technique dose distribution for a 12 Gy prescription dose in the sagittal (A), coronal (B), and transversal view (C). The isofill-depicted dose levels are 75% (9 Gy; blue), 90% (10.8 Gy; purple), and 110% (13.2 Gy; red) of the prescription dose.
The challenge of field junctioning in these techniques includes the combination of head-first and feet-first treatment, as the couch travel ability of linear accelerators is limited to 120–150 cm (295). Most centres plan five to nine isocentres along the patient's longitudinal axis (30, 125, 126, 294). Aspects that have to be considered include dose homogeneity in the junction areas, junction from head-first to feet-first treatment and robustness of the dose in junction areas.
Modern TPSs allow the combined optimization of multiple isocentres and, thus, homogeneity constraints will automatically include junction areas. This issue has been extensively addressed in the context of CSI, which has even more challenging homogeneity requirements (296–298). Special complexity in TBI results from isocentre extension over two separate datasets with different patient orientations. This is handled either by a mutual “bias dose” addition in each plan orientation (30, 127) or by the use of help contours to create decreasing or increasing doses in the transition areas (294, 295). If inhomogeneities resulting from missing divergence compensation are accepted, legs can also be treated with a simple AP-PA technique (125).
At junction areas, robustness against setup errors is primarily determined by two factors: 1) the length of the field overlap, and 2) the dose profile in the transitional region (299, 300). Whereas the former can be easily addressed by the choice of position and number of isocentres, the latter is largely influenced by the optimization and segmentation algorithm of the TPS and can be supported by techniques such as “gradient optimization” (299). In order to retain the planned inter-isocentre distance, setup corrections must never be made for single isocentres only but always for the entire beam set. This is substantially complicated by the length of the planning target volume (PTV): small rotational errors can produce significant lateral shifts in parts of the body. Thus, planning has to ensure PTV coverage with regard to setup as well as geometric and intrafraction motion uncertainties. Whereas open-field techniques imply an inherent robustness against those errors, robust VMAT planning is more challenging and—once again—is dependent on the TPS. In principle, the complexity of segments should be limited and field borders should be extended from the surface, which can be supported by the use of a virtual bolus (29, 292, 301). The prescription of the skin dose has to be handled carefully as some TPSs tend to compensate dose build-up with small highly weighted tangential fields (302). Usually, the PTV is contracted to 5 mm below the skin (30, 127) but, in practise, the combination of multiple arcs, oblique beam incidence and beam exit from all angles significantly reduces the intrinsic photon beam skin-sparing effect (31).
Other Physics Aspects
Energy
With both isocentric and extended SSD techniques, the choice of energy is pertinent. A beam energy of 6 or 10 MV does not produce an additional neutron dose to the patient or staff. For bilateral TBI setups, photon intensities of at least 10 MV provide more homogeneity than do lower intensities; homogeneity can increase with 18 to 24 MV beams but this is relevant mostly for patients with greater body diameters (162).
Treatment Imaging
If shielding or non-open fields are used for TBI delivery, treatment imaging may be used to monitor the position of the patient relative to the fields or the position of the shielding relative to the patient (123, 275).
The accuracy requirements of image guidance depend on the plan complexity. They are generally higher for highly conformal techniques and precision OAR dose reduction. Isocentric techniques require multiple images to cover at least part of the whole-body PTV but optical surface-guided devices might also be used (127). The beam size poses an additional challenge in extended SSD techniques: positioning the imager in the treatment beam requires considerable shielding to protect the electronics from radiation damage. Image acquisition using the megavoltage beam with a detector positioned downstream from the patient may facilitate online verification of organ shielding but the relatively poorer image resolution has to be taken into account.
In vivo Dosimetry
In vivo dosimetry allows the delivered dose to be monitored to ensure that it is sufficiently close to the prescribed dose, making it possible to adjust the fractional dose if needed. Possible measurement devices include diodes, thermo-luminescent dosimeters, optically stimulated luminescence dosimeters, ionisation chambers, and film (303). These devices have varying sensitivities to temperature, orientation with respect to the direction of the radiation, beam energy, and radiation exposure. Some devices offer instantaneous read-out while some do not. Their readings may differ somewhat (304). Dosimeters may be used to measure dose at the patient surface (at the beam entry and/or exit). The dose at that level within the patient must then be extrapolated from these measurements.
While the uncertainty in the measured dose in TBI may be considerable, in vivo dosimetry facilitates a check on the delivered dose. This is particularly pertinent when introducing a new technique or when not using a TPS.
Organ Sparing Total Body Irradiation, Total Marrow Irradiation, and Total Lymph Node Irradiation
Image guided highly conformal delivery of TBI allows the radiation oncology and the transplant teams to define what critical organs to spare, what anatomic structures to target, and the dose that each organ and target structure should receive. This offers the advantage to reduce acute and long-term toxicities (305), the potential to reduce risk of secondary malignancies (306), and the ability to dose escalate to target structures with acceptable toxicities and improved outcomes (307). This is particularly relevant to the paediatric population where, in patients with ALL receiving fractionated TBI, mean lung dose ≥8 Gy was associated with a statistically significant decrease in overall survival (111).
TMI (Figure 4A) and TMLI (Figure 4B) are defined as highly conformal organ sparing forms of TBI delivered to the bone marrow, lymph nodes, and spleen (308–310), while sparing lungs, kidneys, heart, oral cavity, GI tract, and other critical organs. In some studies the liver, brain and testes are included as target regions (Figure 4C) (311). Today the terms TMI/TMLI can be broadly applied to a spectrum of highly conformal IMRT TBI dose distributions, including TBI with only lung sparing, which has been shown to result superior dose reduction to the lungs compared to conventional TBI delivery using lung blocks (312).
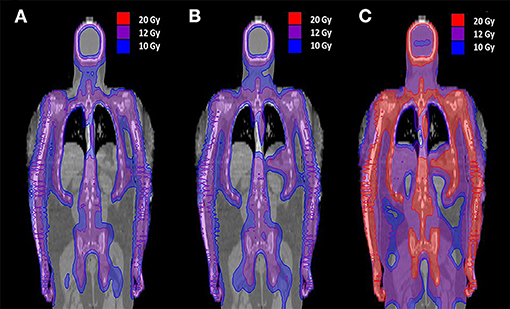
Figure 4. Radiation dose distribution in the coronal plane of TMI and TMLI with different TMI/TMLI approaches. (A) Total marrow irradiation (TMI) of 12 Gy to the bone marrow. (B) Total marrow and lymphoid irradiation (TMLI) of 12 Gy to bone marrow and the lymph nodes. (C) TMLI of 20 Gy to the bone, spleen, and lymph node chains, with a liver and brain prescription dose to 12 Gy. The isofill-depicted dose levels are 10 Gy (blue), 12 Gy (purple), and 20 Gy (red).
The advantages of IMRT based delivery of TBI and TMI/TMLI are clinically important for both adult and paediatric patients, particularly in patients with co-morbidities who cannot tolerate standard myeloablative TBI regimens, in paediatric patients to limit short and long term toxicities, and in patients with relapsed or refractory (R/R) disease who have no standard transplant options. Figure 5 and Table 2 provide an example of a TMLI plan of a 5 year old patient with ALL, with superior organ dose reduction compared to conventional SSD TBI.
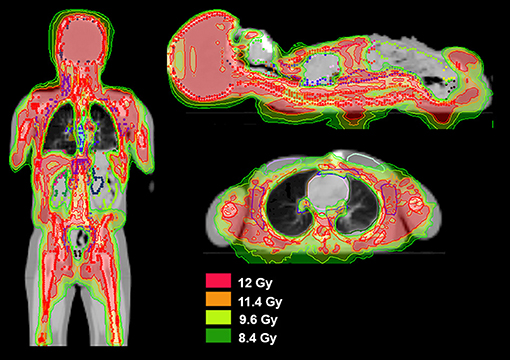
Figure 5. Radiation dose distribution of TMLI in a young patient. Isofill-depicted dose levels of a 12 Gy total marrow and lymphoid irradiation (TMLI) plan in a 5 year old patient with ALL. Target structures were bone, lymph nodes, spleen and brain. The isofill-depicted dose levels are 8.4 Gy (dark green), 9.6 Gy (light green), 11.4 Gy (orange), and 12 Gy (red).
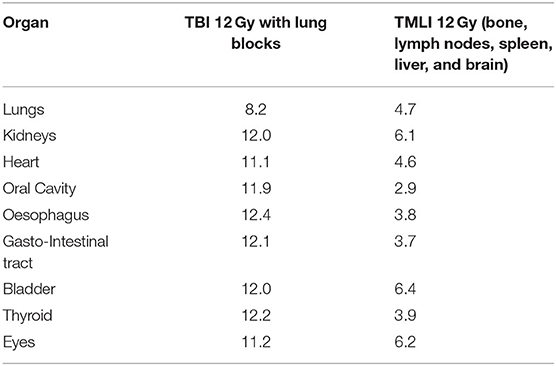
Table 2. Median doses (Gy) to organs at risk for conventional TBI with lung blocks vs. TMLI in a 5 year old patient with ALL.
TMI and TMLI are feasible because of advances in IMRT that have made targeted irradiation of large body regions possible (308, 310, 313–316). The first attempts to cover the whole bone marrow with a very conformal dose distribution were performed with helical TomoTherapy (HT) (308). The first planning studies of HT-based TMI showed that the technique was feasible and that good target coverage could be achieved while reducing doses to key normal tissues by 35–70% compared with conventional TBI (308, 310).
This was followed by the use of a standard linear accelerator to deliver TMI with a number of static (so-called “step and shoot”) IMRT fields (313, 314), with a dose reduction of 29–65% to various OARs in comparison with conventional TBI (314). VMAT-based TMI was shown to obtain comparable target coverage to that obtained with HT and IMRT, with similar dose reduction to normal tissues (314–316).
Clinical Trials in Acute Leukaemia Including TMI and TMLI
The majority of trials have focused on patients with refractory or relapsed (R/R) AML and ALL and are summarised below and in Table 3. Most clinical trials have included adult and adolescent patients, but the strategies being evaluated are applicable to younger paediatric patients (128). A prospective observational study including 37 children and adults treated with myeloablative TMI of 12 Gy in six fractions over 3 days, found favourable outcomes regarding GvHD- and relapse-free survival, as well as toxicity outcomes when compared with retrospective data of 33 patients receiving TBI (326).
Dose-Escalated TMI and TMLI
Dose escalation by conventional delivery of TBI has reduced relapse rates but has failed to increase OS because it increases toxicities and non-relapse mortality (NRM) (94, 327, 328), underscoring the need to develop targeted and organ-sparing forms of radiotherapy such as TMI. In a Phase I trial of 51 patients <60 years old with R/R AML and ALL, patients were conditioned with TMLI (12–20 Gy in 10 fractions on days −10 to −6), cyclophosphamide (100 mg/kg on day −3) and etoposide (60 mg/kg on day −5) prior to allogeneic HSCT (Figure 4C) (311). Dose escalation with acceptable toxicity to 20 Gy was achievable (327). NRM rates were 3.9% at day 100 and 8.1% at 1 year. A subsequent Phase II trial in 57 patients reported 1-year estimates of NRM, OS and PFS of 6, 67, and 48%, respectively, which are superior outcomes to those reported for standard-of-care regimens (318).
A Phase I trial of TMI (3–12 Gy delivered as two fractions of 1.5 Gy per day during 1–4 days) with fludarabine (40 mg/m2/day × 4) and busulfan (4,800 μM*min) reported a maximum tolerated dose (MTD) of 9 Gy. NRM was 29%, relapse-free survival (RFS) was 43% and OS was 50% (319). A Phase I trial combining dose-escalated TMI from 12 to 18 Gy (3 Gy/day) with fludarabine (25 mg/m2 on days −9 to −7) and cyclophosphamide (60 mg/m2 on days −8 and −7), established 15 Gy as the MTD (320). Other groups are evaluating larger fraction sizes of up to 5 Gy in ongoing trials (32, 329, 330).
TMI or TMLI Added to Reduced-Intensity Conditioning Regimens
Reduced-intensity conditioning (RIC) regimens were developed for patients who cannot tolerate standard myeloablative regimens (331) and for paediatric patients where there are concerns regarding feasibility of myeloablative conditioning. These regimens are better tolerated and less cytotoxic but can be associated with a significant increase in relapse rates and a decrease in OS (332). Adding TMI/TMLI may achieve myeloablative medullary radiotherapy doses while not increasing risks for OAR. Rosenthal et al. successfully added 12 Gy TMLI (in eight fractions on days −7 to −4) (Figure 4B) to an RIC regimen of fludarabine (25 mg/m2/day on days −7 to −4) and melphalan (140 mg/m2 on day −2) in 61 patients (309, 321). Two-year OS was 54%, EFS was 49% and NRM was 30%. A successor Phase I trial of dose-escalated TMLI is ongoing, with a modified schedule of TMLI 12–20 Gy (days −9 to −5), fludarabine (30 mg/m2/day on days −4 to −2) and melphalan (100 mg/m2 on day −2). Welliver et al. are conducting an ongoing trial evaluating TMI and cyclophosphamide in patients who were unable to undergo myeloablative TBI (322).
TMI or TMLI Combined With GvHD Reduction Strategies
Strategies to reduce GvHD include the use of post-transplant cyclophosphamide (PTCy) (333, 334) and regulatory T cell/conventional T cell (Treg/Tcon) adoptive immunotherapy (325, 335). These regimens can also reduce graft vs. leukaemia effects. TMLI has been added to counterbalance this. In a Phase I trial, 29 patients with high-risk AML, ALL or myelodysplastic syndrome (MDS) received TMLI (12–20 Gy on days −7 to −3) combined with a regimen of fludarabine (25 mg/m2/day on days −7 to −4), cyclophosphamide (14.5 mg/kg/day on days −7 and −6), and PTCy (50 mg/kg on days +3 and +4), and reported a MTD for TMLI of 20 Gy (323). At 1 year, the cumulative incidence rate of relapse/progression was 24% and OS was 83%. Cumulative incidence of chronic GvHD was 25%. Day 100 and 1-year NRM rates were 4 and 9%, respectively (324). A Phase II trial is ongoing.
A recent Phase II trial of 50 patients with high-risk AML used Treg/Tcon adoptive immunotherapy combined with myeloablative TMLI in patients >50 years (13.5 Gy to the bone marrow and 11.7 Gy to the lymph nodes in eight fractions) or TBI in patients ≤50 years (13.5 Gy in nine fractions or an 8 Gy single fraction) plus thiotepa, fludarabine, and cyclophosphamide. Moderate/severe chronic GvHD occurred in only one patient, NRM occurred in 10 patients, and only two patients relapsed. With a median follow-up of 29 months, the probability of moderate-to-severe chronic GvHD-free, relapse-free survival was 75% (325).
TMI or TMLI in Patients in First Remission as a Possible Alternative to TBI
TMI and TMLI are under investigation for patients in remission who normally would be eligible for standard TBI regimens (307). A pilot trial of TMLI of 20 Gy and PTCy reported a 2 year OS 86.7%, RFS of 83.3%, chronic GVHD incidence of 35% (moderate to severe 7%) and NRM of 0%, which compares favourably to the historical TBI experience (307). Other centres are evaluating IMRT-based organ sparing TBI in this population (127, 292, 336, 337).
Long-Term Toxicities With TMI and TMLI
Long-term toxicities were recently reported in 142 patients receiving TMI (129, 305). The median dose was 14 Gy (range 10–19 Gy). One patient developed radiation pneumonitis (0.7%). Mean lung dose ≤8 vs. >8 Gy was predictive of significantly lower rates of both respiratory infection and IP at 2 years (21 vs. 32%, respectively, p = 0.01). The incidence of radiation-induced renal toxicity was 0%, hypothyroidism was 6% and cataract formation was 7%. The low incidence of toxicities compared with conventional TBI and the successful engraftment rates also suggest that higher dose rates with TMI do not significantly contribute to the incidence of marrow or organ toxicities.
Extramedullary Relapses After TMI and TMLI
In a study assessing the incidence of extramedullary recurrences in 101 patients undergoing allogeneic HSCT following conditioning with TMLI, 13 patients developed extramedullary relapses at 19 sites. The site of relapse was not dose dependent, and the risk of extramedullary relapse observed was comparable to that previously reported with standard TBI, suggesting that TMLI did not increase the risk of relapse in non-target regions (338). This possibly indicates that the main added value of radiotherapy to conditioning before HSCT lies in its immunosuppressive ability and the eradication of leukaemic deposits in bone marrow, lymphatic volumes and sanctuary sites, and not so much in depleting extramedullary or circulating leukaemic cell volumes. The lower integral dose given over the entire body during TMI/TMLI may still function in eradicating small numbers of extramedullary or circulating leukaemic cells (339). Therefore, TMI/TMLI delivery techniques should not be withheld based on concerns of dose heterogeneity to extramedullary/extralymphoid sites.
Conclusions and Future Directions
Myeloablative fractionated TBI delivered together with chemotherapy remains the standard for conditioning prior to HSCT in paediatric patients with high-risk or relapsed/refractory ALL. Since its introduction, TBI has undergone developments to decrease the risks of late sequelae. Still, survivors typically develop serious late effects and efforts to improve the balance between outcomes and toxicity need to continue. While TBI performance between different radiotherapy centres is heterogeneous, with many centres not changing practises for a long time, new techniques may have the potential to mitigate adverse effects while preserving efficacy. To properly evaluate real-world data, we need comparable TBI schedules, uniform specifications, and comprehensive standardised reporting of all relevant parameters. Cooperation between treatment centres and research groups can support new insights, implementation of new techniques and research regarding the potential to reduce the need for TBI, lower TBI doses, or decrease radiotherapy treatment volumes within the body. Future studies must identify whether highly conformal TBI or TMI/TMLI techniques offer equal disease outcomes while reducing toxicity.
Author Contributions
All authors fulfil the criteria for authorship, wrote significant sections of this review, and reviewed and approved the entire manuscript.
Conflict of Interest
The authors declare that the research was conducted in the absence of any commercial or financial relationships that could be construed as a potential conflict of interest.
Publisher's Note
All claims expressed in this article are solely those of the authors and do not necessarily represent those of their affiliated organizations, or those of the publisher, the editors and the reviewers. Any product that may be evaluated in this article, or claim that may be made by its manufacturer, is not guaranteed or endorsed by the publisher.
Acknowledgments
We thank Hannah Bridges, HB Health Comms Limited, UK, for language editing and proofreading.
References
1. Storb R. History of pediatric stem cell transplantation. Pediatr Transplant. (2004) 8 (Suppl. 5):5–11. doi: 10.1111/j.1398-2265.2004.00203.x
2. Armenian SH, Sun CL, Kawashima T, Arora M, Leisenring W, Sklar CA, et al. Long-term health-related outcomes in survivors of childhood cancer treated with HSCT versus conventional therapy: a report from the bone marrow transplant survivor study (BMTSS) and childhood cancer survivor study (CCSS). Blood. (2011) 118:1413–20. doi: 10.1182/blood-2011-01-331835
3. Bhatia S, Francisco L, Carter A, Sun CL, Baker KS, Gurney JG, et al. Late mortality after allogeneic hematopoietic cell transplantation and functional status of long-term survivors: report from the bone marrow transplant survivor study. Blood. (2007) 110:3784–92. doi: 10.1182/blood-2007-03-082933
4. Bresters D, Lawitschka A, Cugno C, Potschger U, Dalissier A, Michel G, et al. Incidence and severity of crucial late effects after allogeneic HSCT for malignancy under the age of 3 years: TBI is what really matters. Bone Marrow Transplant. (2016) 51:1482–9. doi: 10.1038/bmt.2016.139
5. Lawitschka A, Peters C. Long-term effects of myeloablative allogeneic hematopoietic stem cell transplantation in pediatric patients with acute lymphoblastic leukemia. Curr Oncol Rep. (2018) 20:74. doi: 10.1007/s11912-018-0719-5
6. Sun CL, Francisco L, Kawashima T, Leisenring W, Robison LL, Baker KS, et al. Prevalence and predictors of chronic health conditions after hematopoietic cell transplantation: a report from the Bone marrow transplant survivor study. Blood. (2010) 116:3129–39; quiz 377. doi: 10.1182/blood-2009-06-229369
7. Copelan EA. Conditioning regimens for allogeneic bone marrow transplantation. Blood Rev. (1992) 6:234–42. doi: 10.1016/0268-960X(92)90019-M
8. Hong S, Barker C, Klein JP, Shaw P, Bredeson C, Angelina A, et al. Trends in utilization of total body irradiation (TBI) prior to hematopoietic cell transplantation (HCT) worldwide. Biol Blood Marrow Transplant. (2012) 18:S336–S7. doi: 10.1016/j.bbmt.2011.12.348
9. Sureda A, Bader P, Cesaro S, Dreger P, Duarte RF, Dufour C, et al. Indications for allo- and auto-SCT for haematological diseases, solid tumours and immune disorders: current practice in Europe, 2015. Bone Marrow Transplant. (2015) 50:1037–56. doi: 10.1038/bmt.2015.6
10. Vettenranta K, Party EPW. Current European practice in pediatric myeloablative conditioning. Bone Marrow Transplant. (2008) 41 (Suppl. 2):S14–7. doi: 10.1038/bmt.2008.47
11. Copelan EA, Hamilton BK, Avalos B, Ahn KW, Bolwell BJ, Zhu X, et al. Better leukemia-free and overall survival in AML in first remission following cyclophosphamide in combination with busulfan compared with TBI. Blood. (2013) 122:3863–70. doi: 10.1182/blood-2013-07-514448
12. Dandoy CE, Davies SM, Woo Ahn K, He Y, Kolb AE, Levine J, et al. Comparison of total body irradiation versus non-total body irradiation containing regimens for de novo acute myeloid leukemia in children. Haematologica. (2021) 106:1839–45. doi: 10.3324/haematol.2020.249458
13. Ishida H, Kato M, Kudo K, Taga T, Tomizawa D, Miyamura T, et al. Comparison of outcomes for pediatric patients with acute myeloid leukemia in remission and undergoing allogeneic hematopoietic cell transplantation with myeloablative conditioning regimens based on either intravenous busulfan or total body irradiation: a report from the Japanese society for hematopoietic cell transplantation. Biol Blood Marrow Transplant. (2015) 21:2141–7. doi: 10.1016/j.bbmt.2015.08.011
14. Lucchini G, Labopin M, Beohou E, Dalissier A, Dalle JH, Cornish J, et al. Impact of conditioning regimen on outcomes for children with acute myeloid leukemia undergoing transplantation in first complete remission. An analysis on behalf of the pediatric disease working party of the European group for blood and marrow transplantation. Biol Blood Marrow Transplant. (2017) 23:467–74. doi: 10.1016/j.bbmt.2016.11.022
15. Bunin N, Aplenc R, Kamani N, Shaw K, Cnaan A, Simms S. Randomized trial of busulfan vs total body irradiation containing conditioning regimens for children with acute lymphoblastic leukemia: a pediatric blood and marrow transplant consortium study. Bone Marrow Transplant. (2003) 32:543–8. doi: 10.1038/sj.bmt.1704198
16. Davies SM, Ramsay NK, Klein JP, Weisdorf DJ, Bolwell B, Cahn JY, et al. Comparison of preparative regimens in transplants for children with acute lymphoblastic leukemia. J Clin Oncol. (2000) 18:340–7. doi: 10.1200/JCO.2000.18.2.340
17. Gupta T, Kannan S, Dantkale V, Laskar S. Cyclophosphamide plus total body irradiation compared with busulfan plus cyclophosphamide as a conditioning regimen prior to hematopoietic stem cell transplantation in patients with leukemia: a systematic review and meta-analysis. Hematol Oncol Stem Cell Ther. (2011) 4:17–29. doi: 10.5144/1658-3876.2011.17
18. Willasch AM, Peters C, Sedlacek P, Dalle JH, Kitra-Roussou V, Yesilipek A, et al. Myeloablative conditioning for allo-HSCT in pediatric ALL: FTBI or chemotherapy?-A multicenter EBMT-PDWP study. Bone Marrow Transplant. (2020) 55:1540–51. doi: 10.1038/s41409-020-0854-0
19. Peters C, Dalle JH, Locatelli F, Poetschger U, Sedlacek P, Buechner J, et al. Total body irradiation or chemotherapy conditioning in childhood ALL: a multinational, randomized, noninferiority phase III study. J Clin Oncol. (2021) 39:295–307.
20. Barrett A, Depledge MH, Powles RL. Interstitial pneumonitis following bone marrow transplantation after low dose rate total body irradiation. Int J Radiat Oncol Biol Phys. (1983) 9:1029–33. doi: 10.1016/0360-3016(83)90393-0
21. Lawrence G, Rosenbloom ME, Hickling P. A technique for total body irradiation in the treatment of patients with acute leukaemia. Br J Radiol. (1980) 53:894–7. doi: 10.1259/0007-1285-53-633-894
22. Cosset JM, Girinsky T, Malaise E, Chaillet MP, Dutreix J. Clinical basis for TBI fractionation. Radiother Oncol. (1990) 18 (Suppl. 1):60–7. doi: 10.1016/0167-8140(90)90179-Z
23. Deeg HJ, Flournoy N, Sullivan KM, Sheehan K, Buckner CD, Sanders JE, et al. Cataracts after total body irradiation and marrow transplantation: a sparing effect of dose fractionation. Int J Radiat Oncol Biol Phys. (1984) 10:957–64. doi: 10.1016/0360-3016(84)90163-9
24. Thomas ED, Clift RA, Hersman J, Sanders JE, Stewart P, Buckner CD, et al. Marrow transplantation for acute nonlymphoblastic leukemic in first remission using fractionated or single-dose irradiation. Int J Radiat Oncol Biol Phys. (1982) 8:817–21. doi: 10.1016/0360-3016(82)90083-9
25. Fog LS, Wirth A, MacManus M, Downes S, Grace M, Moggre A, et al. Total body irradiation in Australia and New Zealand: results of a practice survey. Phys Eng Sci Med. (2020) 43:825–35. doi: 10.1007/s13246-020-00878-z
26. Giebel S, Miszczyk L, Slosarek K, Moukhtari L, Ciceri F, Esteve J, et al. Extreme heterogeneity of myeloablative total body irradiation techniques in clinical practice: a survey of the acute leukemia working party of the European group for blood and marrow transplantation. Cancer. (2014) 120:2760–5. doi: 10.1002/cncr.28768
27. Hoeben BAW, Pazos M, Albert MH, Seravalli E, Bosman ME, Losert C, et al. Towards homogenization of total body irradiation practices in pediatric patients across SIOPE affiliated centers. A survey by the SIOPE radiation oncology working group. Radiother Oncol. (2021) 155:113–9. doi: 10.1016/j.radonc.2020.10.032
28. Rassiah P, Esiashvili N, Olch AJ, Hua CH, Ulin K, Molineu A, et al. Practice patterns of pediatric total body irradiation techniques: a children's oncology group survey. Int J Radiat Oncol Biol Phys. (2021). doi: 10.1016/j.ijrobp.2021.07.1715
29. Gruen A, Ebell W, Wlodarczyk W, Neumann O, Kuehl JS, Stromberger C, et al. Total body irradiation (TBI) using helical tomotherapy in children and young adults undergoing stem cell transplantation. Radiat Oncol. (2013) 8:92. doi: 10.1186/1748-717X-8-92
30. Losert C, Shpani R, Kiessling R, Freislederer P, Li M, Walter F, et al. Novel rotatable tabletop for total-body irradiation using a linac-based VMAT technique. Radiat Oncol. (2019) 14:244. doi: 10.1186/s13014-019-1445-3
31. Symons K, Morrison C, Parry J, Woodings S, Zissiadis Y. Volumetric modulated arc therapy for total body irradiation: a feasibility study using pinnacle(3) treatment planning system and Elekta Agility™ linac. J Appl Clin Med Phys. (2018) 19:103–10. doi: 10.1002/acm2.12257
32. Wong JYC, Filippi AR, Scorsetti M, Hui S, Muren LP, Mancosu P. Total marrow and total lymphoid irradiation in bone marrow transplantation for acute leukaemia. Lancet Oncol. (2020) 21:e477–87. doi: 10.1016/S1470-2045(20)30342-9
33. Hartman AR, Williams SF, Dillon JJ. Survival, disease-free survival and adverse effects of conditioning for allogeneic bone marrow transplantation with busulfan/cyclophosphamide vs total body irradiation: a meta-analysis. Bone Marrow Transplant. (1998) 22:439–43. doi: 10.1038/sj.bmt.1701334
34. Page KM, Labopin M, Ruggeri A, Michel G, Diaz de Heredia C, O'Brien T, et al. Factors associated with long-term risk of relapse after unrelated cord blood transplantation in children with acute lymphoblastic leukemia in remission. Biol Blood Marrow Transplant. (2017) 23:1350–8. doi: 10.1016/j.bbmt.2017.04.015
35. Tracey J, Zhang MJ, Thiel E, Sobocinski KA, Eapen M. Transplantation conditioning regimens and outcomes after allogeneic hematopoietic cell transplantation in children and adolescents with acute lymphoblastic leukemia. Biol Blood Marrow Transplant. (2013) 19:255–9. doi: 10.1016/j.bbmt.2012.09.019
36. Kotrova M, van der Velden VHJ, van Dongen JJM, Formankova R, Sedlacek P, Brüggemann M, et al. Next-generation sequencing indicates false-positive MRD results and better predicts prognosis after SCT in patients with childhood ALL. Bone Marrow Transplant. (2017) 52:962–8. doi: 10.1038/bmt.2017.16
37. Eckert C, Parker C, Moorman AV, Irving JA, Kirschner-Schwabe R, Groeneveld-Krentz S, et al. Risk factors and outcomes in children with high-risk B-cell precursor and T-cell relapsed acute lymphoblastic leukaemia: combined analysis of ALLR3 and ALL-REZ BFM 2002 clinical trials. Euro J Cancer. (2021) 151:175–89. doi: 10.1016/j.ejca.2021.03.034
38. Bader P, Salzmann-Manrique E, Balduzzi A, Dalle JH, Woolfrey AE, Bar M, et al. More precisely defining risk peri-HCT in pediatric ALL: pre- vs post-MRD measures, serial positivity, and risk modeling. Blood Adv. (2019) 3:3393–405. doi: 10.1182/bloodadvances.2019000449
39. Balduzzi A, Di Maio L, Silvestri D, Songia S, Bonanomi S, Rovelli A, et al. Minimal residual disease before and after transplantation for childhood acute lymphoblastic leukaemia: is there any room for intervention? Br J Haematol. (2014) 164:396–408. doi: 10.1111/bjh.12639
40. Introna M, Lussana F, Algarotti A, Gotti E, Valgardsdottir R, Micò C, et al. Phase II study of sequential infusion of donor lymphocyte infusion and cytokine-induced killer cells for patients relapsed after allogeneic hematopoietic stem cell transplantation. Biol Blood Marrow Transplant. (2017) 23:2070–8. doi: 10.1016/j.bbmt.2017.07.005
41. Sutton R, Shaw PJ, Venn NC, Law T, Dissanayake A, Kilo T, et al. Persistent MRD before and after allogeneic BMT predicts relapse in children with acute lymphoblastic leukaemia. Br J Haematol. (2015) 168:395–404. doi: 10.1111/bjh.13142
42. Rettinger E, Merker M, Salzmann-Manrique E, Kreyenberg H, Krenn T, Dürken M, et al. Pre-emptive immunotherapy for clearance of molecular disease in childhood acute lymphoblastic leukemia after transplantation. Biol Blood Marrow Transplant. (2017) 23:87–95. doi: 10.1016/j.bbmt.2016.10.006
43. Balduzzi A. The value of minimal residual disease (and diamonds). Biol Blood Marrow Transplant. (2017) 23:3–5. doi: 10.1016/j.bbmt.2016.11.012
44. Pulsipher MA, Langholz B, Wall DA, Schultz KR, Bunin N, Carroll W, et al. Risk factors and timing of relapse after allogeneic transplantation in pediatric ALL: for whom and when should interventions be tested? Bone Marrow Transplant. (2015) 50:1173–9. doi: 10.1038/bmt.2015.103
45. Friend BD, Bailey-Olson M, Melton A, Shimano KA, Kharbanda S, Higham C, et al. The impact of total body irradiation-based regimens on outcomes in children and young adults with acute lymphoblastic leukemia undergoing allogeneic hematopoietic stem cell transplantation. Pediatr Blood Cancer. (2020) 67:e28079. doi: 10.1002/pbc.28079
46. The The EndRAD Trial: Eliminating Total Body Irradiation (TBI) for NGS-MRD Negative Children Adolescents and Young Adults With B-ALL. Available online at: https://ClinicalTrials.gov/show/NCT03509961
47. Merli P, Ifversen M, Truong TH, Marquart HV, Buechner J, Wölfl M, et al. Minimal residual disease prior to and after haematopoietic stem cell transplantation in children and adolescents with acute lymphoblastic leukaemia: what level of negativity is relevant? Front Pediatr. (2021). doi: 10.3389/fped.2021.777108
48. Uckun FM, Song CW. Radiobiological features of human pluripotent bone marrow progenitor cells (CFU-GEMM). Int J Radiat Oncol Biol Phys. (1989) 17:1021–5. doi: 10.1016/0360-3016(89)90150-8
49. Kimler BF, Park CH, Yakar D, Mies RM. Radiation response of human normal and leukemic hemopoietic cells assayed by in vitro colony formation. Int J Radiat Oncol Biol Phys. (1985) 11:809–16. doi: 10.1016/0360-3016(85)90315-3
50. Li X, Cui W, Hull L, Smith JT, Kiang JG, Xiao M. Effects of low-to-moderate doses of gamma radiation on mouse hematopoietic system. Radiat Res. (2018) 190:612–22. doi: 10.1667/RR15087.1
51. van Bekkum DW. Radiation sensitivity of the hemopoietic stem cell. Radiat Res. (1991) 128 (1 Suppl):S4–8. doi: 10.2307/3577994
52. Shank B, Andreeff M, Li D. Cell survival kinetics in peripheral blood and bone marrow during total body irradiation for marrow transplantation. Int J Radiat Oncol Biol Phys. (1983) 9:1613–23. doi: 10.1016/0360-3016(83)90413-3
53. Wilke C, Holtan SG, Sharkey L, DeFor T, Arora M, Premakanthan P, et al. Marrow damage and hematopoietic recovery following allogeneic bone marrow transplantation for acute leukemias: effect of radiation dose and conditioning regimen. Radiother Oncol. (2016) 118:65–71. doi: 10.1016/j.radonc.2015.11.012
54. O'Donoghue JA, Wheldon TE, Gregor A. The implications of in-vitro radiation-survival curves for the optimal scheduling of total-body irradiation with bone marrow rescue in the treatment of leukaemia. Br J Radiol. (1987) 60:279–83. doi: 10.1259/0007-1285-60-711-279
55. Thomson AE, O'Connor TW, Peel WE, Slater NG. Heterogeneous X-ray survival characteristics of lymphocytes in prolymphocytic leukaemia: mathematical analysis distinguishing delayed cell death and true radioresistance. Eur J Haematol. (1994) 53:223–31. doi: 10.1111/j.1600-0609.1994.tb00194.x
56. Cosset JM, Socie G, Girinsky T, Dubray B, Fourquet A, Gluckman E. Radiobiological and clinical bases for total body irradiation in the leukemias and lymphomas. Semin Radiat Oncol. (1995) 5:301–15. doi: 10.1016/S1053-4296(05)80028-1
57. Cowen D, Richaud P, Landriau S, Lagarde P, Mahon FX, Baudet JJ, et al. Radiobiological features of acute myeloblastic leukemia: comparison of self-renewal versus terminally differentiated populations. Int J Radiat Oncol Biol Phys. (1994) 30:1133–40. doi: 10.1016/0360-3016(94)90320-4
58. Lehnert S, Rybka WB, Suissa S, Giambattisto D. Radiation response of haematopoietic cell lines of human origin. Int J Radiat Biol Relat Stud Phys Chem Med. (1986) 49:423–31. doi: 10.1080/09553008514552651
59. Weichselbaum RR, Greenberger JS, Schmidt A, Karpas A, Moloney WC, Little JB. In vitro radiosensitivity of human leukemia cell lines. Radiology. (1981) 139:485–7. doi: 10.1148/radiology.139.2.6939019
60. Uckun FM, Aeppli D, Song CW. Radiation resistance of primary clonogenic blasts from children with acute lymphoblastic leukemia. Int J Radiat Oncol Biol Phys. (1993) 27:899–906. doi: 10.1016/0360-3016(93)90466-9
61. Monzen S, Chiba M, Hosokawa Y. Genetic network profiles associated with established resistance to ionizing radiation in acute promyelocytic leukemia cells and their extracellular vesicles. Oncol Rep. (2016) 35:749–56. doi: 10.3892/or.2015.4471
62. Bentzen SM, Dorr W, Gahbauer R, Howell RW, Joiner MC, Jones B, et al. Bioeffect modeling and equieffective dose concepts in radiation oncology–terminology, quantities and units. Radiother Oncol. (2012) 105:266–8. doi: 10.1016/j.radonc.2012.10.006
63. Song CW, Kim TH, Khan FM, Kersey JH, Levitt SH. Radiobiological basis of total body irradiation with different dose rate and fractionation: repair capacity of hemopoietic cells. Int J Radiat Oncol Biol Phys. (1981) 7:1695–701. doi: 10.1016/0360-3016(81)90195-4
64. Uckun FM, Chandan-Langlie M, Jaszcz W, Obuz V, Waddick K, Song CW. Radiation damage repair capacity of primary clonogenic blasts in acute lymphoblastic leukemia. Cancer Res. (1993) 53:1431–6.
65. Shank B. Hyperfractionation versus single dose irradiation in human acute lymphocytic leukemia cells: application to TBI for marrow transplantation. Radiother Oncol. (1993) 27:30–5. doi: 10.1016/0167-8140(93)90041-6
66. FitzGerald TJ, McKenna M, Kase K, Daugherty C, Rothstein L, Greenberger JS. Effect of X-irradiation dose rate on the clonagenic survival of human and experimental animal hematopoietic tumor cell lines: evidence for heterogeneity. Int J Radiat Oncol Biol Phys. (1986) 12:69–73. doi: 10.1016/0360-3016(86)90417-7
67. Wheldon TE, Barrett A. Radiobiological modelling of the treatment of leukaemia by total body irradiation. Radiother Oncol. (2001) 58:227–33. doi: 10.1016/S0167-8140(00)00255-3
68. Kerns SL, West CM, Andreassen CN, Barnett GC, Bentzen SM, Burnet NG, et al. Radiogenomics: the search for genetic predictors of radiotherapy response. Future Oncol. (2014) 10:2391–406. doi: 10.2217/fon.14.173
69. Barnett GC, Coles CE, Elliott RM, Baynes C, Luccarini C, Conroy D, et al. Independent validation of genes and polymorphisms reported to be associated with radiation toxicity: a prospective analysis study. Lancet Oncol. (2012) 13:65–77. doi: 10.1016/S1470-2045(11)70302-3
70. Bergom C, West CM, Higginson DS, Abazeed ME, Arun B, Bentzen SM, et al. The implications of genetic testing on radiation therapy decisions: a guide for radiation oncologists. Int J Radiat Oncol Biol Phys. (2019) 105:698–712. doi: 10.1016/j.ijrobp.2019.07.026
71. Congdon CC. Experimental treatment of total-body irradiation injury: a brief review. Blood. (1957) 12:746–54. doi: 10.1182/blood.V12.8.746.746
72. Thomas E, Storb R, Clift RA, Fefer A, Johnson FL, Neiman PE, et al. Bone-marrow transplantation (first of two parts). N Engl J Med. (1975) 292:832–43. doi: 10.1056/NEJM197504172921605
73. Thomas ED, Storb R, Clift RA, Fefer A, Johnson L, Neiman PE, et al. Bone-marrow transplantation (second of two parts). N Engl J Med. (1975) 292:895–902. doi: 10.1056/NEJM197504242921706
74. Burchenal JH, Oettgen HF, Holmberg EA, Hemphill SC, Reppert JA. Effect of total-body irradiation on the transplantability of mouse leukemias. Cancer Res. (1960) 20:425–30.
75. Bortin MM. A compendium of reported human bone marrow transplants. Transplantation. (1970) 9:571–87. doi: 10.1097/00007890-197006000-00006
76. Thomas ED, Buckner CD, Banaji M, Clift RA, Fefer A, Flournoy N, et al. One hundred patients with acute leukemia treated by chemotherapy, total body irradiation, and allogeneic marrow transplantation. Blood. (1977) 49:511–33. doi: 10.1182/blood.V49.4.511.bloodjournal494511
77. Peters L. Total body irradiation conference: discussion: the radiobiological bases of TBI. Int J Radiat Oncol Biol Phys. (1980) 6:785–7. doi: 10.1016/0360-3016(80)90241-2
78. Deeg HJ, Sullivan KM, Buckner CD, Storb R, Appelbaum FR, Clift RA, et al. Marrow transplantation for acute nonlymphoblastic leukemia in first remission: toxicity and long-term follow-up of patients conditioned with single dose or fractionated total body irradiation. Bone Marrow Transplant. (1986) 1:151–7.
79. Quast U. Total body irradiation–review of treatment techniques in Europe. Radiother Oncol. (1987) 9:91–106. doi: 10.1016/S0167-8140(87)80197-4
80. Frassoni F, Scarpati D, Bacigalupo A, Vitale V, Corvo R, Miceli S, et al. The effect of total body irradiation dose and chronic graft-versus-host disease on leukaemic relapse after allogeneic bone marrow transplantation. Br J Haematol. (1989) 73:211–6. doi: 10.1111/j.1365-2141.1989.tb00254.x
81. Scarpati D, Frassoni F, Vitale V, Corvo R, Franzone P, Barra S, et al. Total body irradiation in acute myeloid leukemia and chronic myelogenous leukemia: influence of dose and dose-rate on leukemia relapse. Int J Radiat Oncol Biol Phys. (1989) 17:547–52. doi: 10.1016/0360-3016(89)90105-3
82. Petersen FB, Deeg HJ, Buckner CD, Appelbaum FR, Storb R, Clift RA, et al. Marrow transplantation following escalating doses of fractionated total body irradiation and cyclophosphamide–a phase I trial. Int J Radiat Oncol Biol Phys. (1992) 23:1027–32. doi: 10.1016/0360-3016(92)90909-2
83. Aristei C, Aversa F, Chionne F, Martelli MF, Latini P. Interstitial pneumonitis in acute leukemia patients submitted to T-depleted matched and mismatched bone marrow transplantation. Int J Radiat Oncol Biol Phys. (1998) 41:651–7. doi: 10.1016/S0360-3016(98)00068-6
84. Ferry C, Gemayel G, Rocha V, Labopin M, Esperou H, Robin M, et al. Long-term outcomes after allogeneic stem cell transplantation for children with hematological malignancies. Bone Marrow Transplant. (2007) 40:219–24. doi: 10.1038/sj.bmt.1705710
85. Pino y Torres JL, Bross DS, Lam WC, Wharam MD, Santos GW, Order SE. Risk factors in interstitial pneumonitis following allogenic bone marrow transplantation. Int J Radiat Oncol Biol Phys. (1982) 8:1301–7. doi: 10.1016/0360-3016(82)90579-X
86. Vitale V, Bacigalupo A, Van Lint MT, Frassoni F, Ricci G, Siracusa G, et al. Fractionated total body irradiation in marrow transplantation for leukaemia. Br J Haematol. (1983) 55:547–54. doi: 10.1111/j.1365-2141.1983.tb02169.x
87. Cohen A, Rovelli A, Bakker B, Uderzo C, van Lint MT, Esperou H, et al. Final height of patients who underwent bone marrow transplantation for hematological disorders during childhood: a study by the working party for late effects-EBMT. Blood. (1999) 93:4109–15.
88. Muller-Runkel R, Vijayakumar S. Fractionated total body irradiation: some radiobiological considerations. Clin Oncol. (1993) 5:39–42. doi: 10.1016/S0936-6555(05)80695-8
89. O'Donoghue JA. Fractionated versus low dose-rate total body irradiation. Radiobiological considerations in the selection of regimes. Radiother Oncol. (1986) 7:241–7. doi: 10.1016/S0167-8140(86)80035-4
90. Peters LJ, Withers HR, Cundiff JH, Dicke KA. Radiobiological considerations in the use of total-body irradiation for bone-marrow transplantation. Radiology. (1979) 131:243–7. doi: 10.1148/131.1.243
91. Belkacemi Y, Labopin M, Giebel S, Loganadane G, Miszczyk L, Michallet M, et al. Single-dose daily fractionation is not inferior to twice-a-day fractionated total-body irradiation before allogeneic stem cell transplantation for acute leukemia: a useful practice simplification resulting from the SARASIN study. Int J Radiat Oncol Biol Phys. (2018) 102:515–26. doi: 10.1016/j.ijrobp.2018.06.015
92. Sengelov H, Petersen PM, Fog L, Schmidt M, Specht L. Less mucositis toxicity after 6 versus 3 fractions of high-dose total body irradiation before allogeneic stem cell transplantation. Bone Marrow Transplant. (2019) 54:1369–71. doi: 10.1038/s41409-019-0470-z
93. Clift RA, Buckner CD, Appelbaum FR, Bearman SI, Petersen FB, Fisher LD, et al. Allogeneic marrow transplantation in patients with acute myeloid leukemia in first remission: a randomized trial of two irradiation regimens. Blood. (1990) 76:1867–71. doi: 10.1182/blood.V76.9.1867.bloodjournal7691867
94. Clift RA, Buckner CD, Appelbaum FR, Sullivan KM, Storb R, Thomas ED. Long-term follow-up of a randomized trial of two irradiation regimens for patients receiving allogeneic marrow transplants during first remission of acute myeloid leukemia. Blood. (1998) 92:1455–6. doi: 10.1182/blood.V92.4.1455.splL2_1455_1456
95. Corvo R, Paoli G, Barra S, Bacigalupo A, Van Lint MT, Franzone P, et al. Total body irradiation correlates with chronic graft versus host disease and affects prognosis of patients with acute lymphoblastic leukemia receiving an HLA identical allogeneic bone marrow transplant. Int J Radiat Oncol Biol Phys. (1999) 43:497–503. doi: 10.1016/S0360-3016(98)00441-6
96. Bieri S, Helg C, Chapuis B, Miralbell R. Total body irradiation before allogeneic bone marrow transplantation: is more dose better? Int J Radiat Oncol Biol Phys. (2001) 49:1071–7. doi: 10.1016/S0360-3016(00)01491-7
97. Kal HB, Loes van Kempen-Harteveld M, Heijenbrok-Kal MH, Struikmans H. Biologically effective dose in total-body irradiation and hematopoietic stem cell transplantation. Strahlenther Onkol. (2006) 182:672–9. doi: 10.1007/s00066-006-1528-6
98. Kornguth DG, Mahajan A, Woo S, Chan KW, Antolak J, Ha CS. Fludarabine allows dose reduction for total body irradiation in pediatric hematopoietic stem cell transplantation. Int J Radiat Oncol Biol Phys. (2007) 68:1140–4. doi: 10.1016/j.ijrobp.2007.01.003
99. Rossoff J, Jacobsohn D, Kwon S, Kletzel M, Duerst RE, Tse WT, et al. Reduced-toxicity conditioning regimen with busulfan, fludarabine, rATG, and 400 cGy TBI in pediatric patients undergoing hematopoietic stem cell transplant for high-risk hematologic malignancies. Pediatr Blood Cancer. (2021) 68:e29087. doi: 10.1002/pbc.29087
100. Vriesendorp HM, Chu H, Ochran TG, Besa PC, Champlin RE. Radiobiology of total body radiation. Bone Marrow Transplant. (1994) 14 (Suppl. 4):S4–8.
101. Travis EL, Peters LJ, McNeill J, Thames HD Jr., Karolis C. Effect of dose-rate on total body irradiation: lethality and pathologic findings. Radiother Oncol. (1985) 4:341–51. doi: 10.1016/S0167-8140(85)80122-5
102. Down JD, Tarbell NJ, Thames HD, Mauch PM. Syngeneic and allogeneic bone marrow engraftment after total body irradiation: dependence on dose, dose rate, and fractionation. Blood. (1991) 77:661–9. doi: 10.1182/blood.V77.3.661.661
103. Glass TJ, Hui SK, Blazar BR, Lund TC. Effect of radiation dose-rate on hematopoietic cell engraftment in adult zebrafish. PLoS ONE. (2013) 8:e73745. doi: 10.1371/journal.pone.0073745
104. van Os R, Konings AW, Down JD. Compromising effect of low dose-rate total body irradiation on allogeneic bone marrow engraftment. Int J Radiat Biol. (1993) 64:761–70. doi: 10.1080/09553009314552011
105. Tarbell NJ, Amato DA, Down JD, Mauch P, Hellman S. Fractionation and dose rate effects in mice: a model for bone marrow transplantation in man. Int J Radiat Oncol Biol Phys. (1987) 13:1065–9. doi: 10.1016/0360-3016(87)90046-0
106. Turesson I. Radiobiological aspects of continuous low dose-rate irradiation and fractionated high dose-rate irradiation. Radiother Oncol. (1990) 19:1–15. doi: 10.1016/0167-8140(90)90161-O
107. Lehnert S. Response of the lung to intermittent irradiation: the importance of average versus instantaneous dose rate. Int J Radiat Oncol Biol Phys. (1985) 11:2183–4. doi: 10.1016/0360-3016(85)90102-6
108. Storb R, Raff R, Deeg HJ, Graham T, Appelbaum FR, Schuening FG, et al. Dose rate-dependent sparing of the gastrointestinal tract by fractionated total body irradiation in dogs given marrow autografts. Int J Radiat Oncol Biol Phys. (1998) 40:961–6. doi: 10.1016/S0360-3016(97)00913-9
109. Deeg HJ, Storb R, Longton G, Graham TC, Shulman HM, Appelbaum F, et al. Single dose or fractionated total body irradiation and autologous marrow transplantation in dogs: effects of exposure rate, fraction size, and fractionation interval on acute and delayed toxicity. Int J Radiat Oncol Biol Phys. (1988) 15:647–53. doi: 10.1016/0360-3016(88)90307-0
110. Gao RW, Weisdorf DJ, DeFor TE, Ehler E, Dusenbery KE. Influence of total body irradiation dose rate on idiopathic pneumonia syndrome in acute leukemia patients undergoing allogeneic hematopoietic cell transplantation. Int J Radiat Oncol Biol Phys. (2019) 103:180–9. doi: 10.1016/j.ijrobp.2018.09.002
111. Esiashvili N, Lu X, Ulin K, Laurie F, Kessel S, Kalapurakal JA, et al. Higher reported lung dose received during total body irradiation for allogeneic hematopoietic stem cell transplantation in children with acute lymphoblastic leukemia is associated with inferior survival: a report from the children's oncology group. Int J Radiat Oncol Biol Phys. (2019) 104:513–21. doi: 10.1016/j.ijrobp.2019.02.034
112. Girinsky T, Benhamou E, Bourhis JH, Dhermain F, Guillot-Valls D, Ganansia V, et al. Prospective randomized comparison of single-dose versus hyperfractionated total-body irradiation in patients with hematologic malignancies. J Clin Oncol. (2000) 18:981–6. doi: 10.1200/JCO.2000.18.5.981
113. Ozsahin M, Pene F, Touboul E, Gindrey-Vie B, Dominique C, Lefkopoulos D, et al. Total-body irradiation before bone marrow transplantation. Results of two randomized instantaneous dose rates in 157 patients. Cancer. (1992) 69:2853–65. doi: 10.1002/1097-0142(19920601)69:11<2853::AID-CNCR2820691135>3.0.CO;2-2
114. Soejima T, Hirota S, Tsujino K, Yoden E, Fujii O, Ichimiya Y, et al. Total body irradiation followed by bone marrow transplantation: comparison of once-daily and twice-daily fractionation regimens. Radiat Med. (2007) 25:402–6. doi: 10.1007/s11604-007-0157-z
115. Abugideiri M, Nanda RH, Butker C, Zhang C, Kim S, Chiang KY, et al. Factors influencing pulmonary toxicity in children undergoing allogeneic hematopoietic stem cell transplantation in the setting of total body irradiation-based myeloablative conditioning. Int J Radiat Oncol Biol Phys. (2016) 94:349–59. doi: 10.1016/j.ijrobp.2015.10.054
116. Carruthers SA, Wallington MM. Total body irradiation and pneumonitis risk: a review of outcomes. Br J Cancer. (2004) 90:2080–4. doi: 10.1038/sj.bjc.6601751
117. Sampath S, Schultheiss TE, Wong J. Dose response and factors related to interstitial pneumonitis after bone marrow transplant. Int J Radiat Oncol Biol Phys. (2005) 63:876–84. doi: 10.1016/j.ijrobp.2005.02.032
118. Cheng JC, Schultheiss TE, Wong JY. Impact of drug therapy, radiation dose, and dose rate on renal toxicity following bone marrow transplantation. Int J Radiat Oncol Biol Phys. (2008) 71:1436–43. doi: 10.1016/j.ijrobp.2007.12.009
119. Kal HB, van Kempen-Harteveld ML. Renal dysfunction after total body irradiation: dose-effect relationship. Int J Radiat Oncol Biol Phys. (2006) 65:1228–32. doi: 10.1016/j.ijrobp.2006.02.021
120. Safwat A, Nielsen OS, abd el-Bakky H, Overgaard J. Renal damage after total body irradiation in a mouse model for bone marrow transplantation: effect of radiation dose rate. Radiother Oncol. (1995) 34:203–9. doi: 10.1016/0167-8140(95)01518-L
121. Belkacemi Y, Labopin M, Vernant JP, Prentice HG, Tichelli A, Schattenberg A, et al. Cataracts after total body irradiation and bone marrow transplantation in patients with acute leukemia in complete remission: a study of the European group for blood and marrow transplantation. Int J Radiat Oncol Biol Phys. (1998) 41:659–68. doi: 10.1016/S0360-3016(98)00077-7
122. Ozsahin M, Belkacemi Y, Pene F, Dominique C, Schwartz LH, Uzal C, et al. Total-body irradiation and cataract incidence: a randomized comparison of two instantaneous dose rates. Int J Radiat Oncol Biol Phys. (1994) 28:343–7. doi: 10.1016/0360-3016(94)90056-6
123. Fog LS, Hansen VN, Kjaer-Kristoffersen F, Berlon TE, Petersen PM, Mandeville H, et al. A step and shoot intensity modulated technique for total body irradiation. Tech Innov Pat Supp Radiat Oncol. (2019) 10:1–7. doi: 10.1016/j.tipsro.2019.05.002
124. Durie E, Nicholson E, Anthias C, Dunne EM, Potter M, Ethell M, et al. Determining the incidence of interstitial pneumonitis and chronic kidney disease following full intensity haemopoetic stem cell transplant conditioned using a forward-planned intensity modulated total body irradiation technique. Radiother Oncol. (2021) 158:97–103. doi: 10.1016/j.radonc.2021.02.020
125. Ouyang L, Folkerts M, Zhang Y, Hrycushko B, Lamphier R, Lee P, et al. Volumetric modulated arc therapy based total body irradiation: workflow and clinical experience with an indexed rotational immobilization system. Phys Imaging Radiat Oncol. (2017) 4:22–5. doi: 10.1016/j.phro.2017.11.002
126. Son J, Choi N, Kim JI, Park JM, Wu HG, Kang HC, et al. Effect of changes in monitor unit rate and energy on dose rate of total marrow irradiation based on linac volumetric arc therapy. Radiat Oncol. (2019) 14:87. doi: 10.1186/s13014-019-1296-y
127. Tas B, Durmus IF, Okumus A, Uzel OE, Gokce M, Goksoy HS, et al. Total-body irradiation using linac-based volumetric modulated arc therapy: its clinical accuracy, feasibility and reliability. Radiother Oncol. (2018) 129:527–33. doi: 10.1016/j.radonc.2018.08.005
128. Kobyzeva D, Shelikhova L, Shekhovtsova Z, Khismatullina R, Ilushina M, Loginova A, et al. Total body irradiation among recipients of Tcrαβ /CD19- depleted grafts in a cohort of children with hematologic malignances: single center experience. Blood. (2020) 136 (Suppl. 1):2. doi: 10.1182/blood-2020-139282
129. Shinde A, Yang D, Frankel P, Liu A, Han C, Del Vecchio B, et al. Radiation-related toxicities using organ sparing total marrow irradiation transplant conditioning regimens. Int J Radiat Oncol Biol Phys. (2019) 105:1025–33. doi: 10.1016/j.ijrobp.2019.08.010
130. Parmar K, Mauch P, Vergilio JA, Sackstein R, Down JD. Distribution of hematopoietic stem cells in the bone marrow according to regional hypoxia. Proc Natl Acad Sci USA. (2007) 104:5431–6. doi: 10.1073/pnas.0701152104
131. Spencer JA, Ferraro F, Roussakis E, Klein A, Wu J, Runnels JM, et al. Direct measurement of local oxygen concentration in the bone marrow of live animals. Nature. (2014) 508:269–73. doi: 10.1038/nature13034
132. Benito J, Zeng Z, Konopleva M, Wilson WR. Targeting hypoxia in the leukemia microenvironment. Int J Hematol Oncol. (2013) 2:279–88. doi: 10.2217/ijh.13.32
133. Deynoux M, Sunter N, Herault O, Mazurier F. Hypoxia and hypoxia-inducible factors in leukemias. Front Oncol. (2016) 6:41. doi: 10.3389/fonc.2016.00041
134. Frolova O, Samudio I, Benito JM, Jacamo R, Kornblau SM, Markovic A, et al. Regulation of HIF-1alpha signaling and chemoresistance in acute lymphocytic leukemia under hypoxic conditions of the bone marrow microenvironment. Cancer Biol Ther. (2012) 13:858–70. doi: 10.4161/cbt.20838
135. Aldoss I, Al Malki MM, Stiller T, Cao T, Sanchez JF, Palmer J, et al. Implications and management of central nervous system involvement before allogeneic hematopoietic cell transplantation in acute lymphoblastic leukemia. Biol Blood Marrow Transplant. (2016) 22:575–8. doi: 10.1016/j.bbmt.2015.10.016
136. Alexander BM, Wechsler D, Braun TM, Levine J, Herman J, Yanik G, et al. Utility of cranial boost in addition to total body irradiation in the treatment of high risk acute lymphoblastic leukemia. Int J Radiat Oncol Biol Phys. (2005) 63:1191–6. doi: 10.1016/j.ijrobp.2005.04.020
137. Barredo JC, Hastings C, Lu X, Devidas M, Chen Y, Armstrong D, et al. Isolated late testicular relapse of B-cell acute lymphoblastic leukemia treated with intensive systemic chemotherapy and response-based testicular radiation: a children's oncology group study. Pediatr Blood Cancer. (2018) 65:e26928. doi: 10.1002/pbc.26928
138. Clarke M, Gaynon P, Hann I, Harrison G, Masera G, Peto R, et al. CNS-directed therapy for childhood acute lymphoblastic leukemia: childhood ALL collaborative group overview of 43 randomized trials. J Clin Oncol. (2003) 21:1798–809. doi: 10.1200/JCO.2003.08.047
139. Hijiya N, Liu W, Sandlund JT, Jeha S, Razzouk BI, Ribeiro RC, et al. Overt testicular disease at diagnosis of childhood acute lymphoblastic leukemia: lack of therapeutic role of local irradiation. Leukemia. (2005) 19:1399–403. doi: 10.1038/sj.leu.2403843
140. Pinnix CC, Yahalom J, Specht L, Dabaja BS. Radiation in central nervous system leukemia: guidelines from the international lymphoma radiation oncology group. Int J Radiat Oncol Biol Phys. (2018) 102:53–8. doi: 10.1016/j.ijrobp.2018.05.067
141. Quaranta BP, Halperin EC, Kurtzberg J, Clough R, Martin PL. The incidence of testicular recurrence in boys with acute leukemia treated with total body and testicular irradiation and stem cell transplantation. Cancer. (2004) 101:845–50. doi: 10.1002/cncr.20413
142. Gao RW, Dusenbery KE, Cao Q, Smith AR, Yuan J. Augmenting total body irradiation with a cranial boost before stem cell transplantation protects against post-transplant central nervous system relapse in acute lymphoblastic leukemia. Biol Blood Marrow Transplant. (2018) 24:501–6. doi: 10.1016/j.bbmt.2017.11.013
143. Cherlow JM, Sather H, Steinherz P, Gaynon P, Tubergen D, Trigg M, et al. Craniospinal irradiation for acute lymphoblastic leukemia with central nervous system disease at diagnosis: a report from the children's cancer group. Int J Radiat Oncol Biol Phys. (1996) 36:19–27. doi: 10.1016/S0360-3016(96)00272-6
144. Leiper AD. Non-endocrine late complications of bone marrow transplantation in childhood: part II. Br J Haematol. (2002) 118:23–43. doi: 10.1046/j.1365-2141.2002.03471.x
145. Socie G, Stone JV, Wingard JR, Weisdorf D, Henslee-Downey PJ, Bredeson C, et al. Long-term survival and late deaths after allogeneic bone marrow transplantation. Late effects working committee of the international bone marrow transplant registry. N Engl J Med. (1999) 341:14–21. doi: 10.1056/NEJM199907013410103
146. Bhatia S, Dai C, Landier W, Hageman L, Wu J, Schlichting E, et al. Trends in late mortality and life expectancy after allogeneic blood or marrow transplantation over 4 decades: a blood or marrow transplant survivor study report. JAMA Oncol. (2021). doi: 10.1001/jamaoncol.2021.3676
147. Mulcahy Levy JM, Tello T, Giller R, Wilkening G, Quinones R, Keating AK, et al. Late effects of total body irradiation and hematopoietic stem cell transplant in children under 3 years of age. Pediatr Blood Cancer. (2013) 60:700–4. doi: 10.1002/pbc.24252
148. Willard VW, Leung W, Huang Q, Zhang H, Phipps S. Cognitive outcome after pediatric stem-cell transplantation: impact of age and total-body irradiation. J Clin Oncol. (2014) 32:3982–8. doi: 10.1200/JCO.2014.56.2223
149. Stone HB, Coleman CN, Anscher MS, McBride WH. Effects of radiation on normal tissue: consequences and mechanisms. Lancet Oncol. (2003) 4:529–36. doi: 10.1016/S1470-2045(03)01191-4
150. Resbeut M, Cowen D, Blaise D, Gluckman E, Cosset JM, Rio B, et al. Fractionated or single-dose total body irradiation in 171 acute myeloblastic leukemias in first complete remission: is there a best choice? SFGM. Societe Francaise de Greffe de Moelle. Int J Radiat Oncol Biol Phys. (1995) 31:509–17. doi: 10.1016/0360-3016(94)00446-R
151. Bernard F, Auquier P, Herrmann I, Contet A, Poiree M, Demeocq F, et al. Health status of childhood leukemia survivors who received hematopoietic cell transplantation after BU or TBI: an LEA study. Bone Marrow Transplant. (2014) 49:709–16. doi: 10.1038/bmt.2014.3
152. Oya N, Sasai K, Tachiiri S, Sakamoto T, Nagata Y, Okada T, et al. Influence of radiation dose rate and lung dose on interstitial pneumonitis after fractionated total body irradiation: acute parotitis may predict interstitial pneumonitis. Int J Hematol. (2006) 83:86–91. doi: 10.1532/IJH97.05046
153. Buchali A, Feyer P, Groll J, Massenkeil G, Arnold R, Budach V. Immediate toxicity during fractionated total body irradiation as conditioning for bone marrow transplantation. Radiother Oncol. (2000) 54:157–62. doi: 10.1016/S0167-8140(99)00178-4
154. Vogel J, Hui S, Hua C-H, Dusenbery K, Rassiah P, Kalapurakal J, et al. Pulmonary toxicity after total body irradiation – critical review of the literature and recommendations for toxicity reporting. Front Oncol. (2021) 11:708906. doi: 10.3389/fonc.2021.708906
155. Schneider RA, Schultze J, Jensen JM, Hebbinghaus D, Galalae RM. Long-term outcome after static intensity-modulated total body radiotherapy using compensators stratified by pediatric and adult cohorts. Int J Radiat Oncol Biol Phys. (2008) 70:194–202. doi: 10.1016/j.ijrobp.2007.05.035
156. Keane TJ, Van Dyk J, Rider WD. Idiopathic interstitial pneumonia following bone marrow transplantation: the relationship with total body irradiation. Int J Radiat Oncol Biol Phys. (1981) 7:1365–70. doi: 10.1016/0360-3016(81)90032-8
157. Belkacémi Y, Pène F, Touboul E, Rio B, Leblond V, Gorin NC, et al. Total-body irradiation before bone marrow transplantation for acute leukemia in first or second complete remission. Results and prognostic factors in 326 consecutive patients. Strahlenther Onkol. (1998) 174:92–104. doi: 10.1007/BF03038482
158. Clift RA, Buckner CD, Thomas ED, Sanders JE, Stewart PS, Sullivan KM, et al. Allogeneic marrow transplantation using fractionated total body irradiation in patients with acute lymphoblastic leukemia in relapse. Leukemia Res. (1982) 6:401–7. doi: 10.1016/0145-2126(82)90104-7
159. Della Volpe A, Ferreri AJ, Annaloro C, Mangili P, Rosso A, Calandrino R, et al. Lethal pulmonary complications significantly correlate with individually assessed mean lung dose in patients with hematologic malignancies treated with total body irradiation. Int J Radiat Oncol Biol Phys. (2002) 52:483–8. doi: 10.1016/S0360-3016(01)02589-5
160. Gerrard GE, Vail A, Taylor RE, Pitchford WG, Gilson D, Povall JM, et al. Toxicity and dosimetry of fractionated total body irradiation prior to allogeneic bone marrow transplantation using a straightforward radiotherapy technique. Clin Oncol. (1998) 10:379–83. doi: 10.1016/S0936-6555(98)80034-4
161. Ozsahin M, Belkacémi Y, Pène F, Laporte J, Rio B, Leblond V, et al. Interstitial pneumonitis following autologous bone-marrow transplantation conditioned with cyclophosphamide and total-body irradiation. Int J Radiat Oncol Biol Phys. (1996) 34:71–7. doi: 10.1016/0360-3016(95)02063-2
162. Bradley J, Reft C, Goldman S, Rubin C, Nachman J, Larson R, et al. High-energy total body irradiation as preparation for bone marrow transplantation in leukemia patients: treatment technique and related complications. Int J Radiat Oncol Biol Phys. (1998) 40:391–6. doi: 10.1016/S0360-3016(97)00578-6
163. Demirer T, Petersen FB, Appelbaum FR, Barnett TA, Sanders J, Deeg HJ, et al. Allogeneic marrow transplantation following cyclophosphamide and escalating doses of hyperfractionated total body irradiation in patients with advanced lymphoid malignancies: a phase I/II trial. Int J Radiat Oncol Biol Phys. (1995) 32:1103–9. doi: 10.1016/0360-3016(95)00115-F
164. de Felice F, Grapulin L, Musio D, Pomponi J, C DIF, Iori AP, et al. Treatment complications and long-term outcomes of total body irradiation in patients with acute lymphoblastic leukemia: a single institute experience. Anticancer Res. (2016) 36:4859–64. doi: 10.21873/anticanres.11049
165. Hoffmeister PA, Madtes DK, Storer BE, Sanders JE. Pulmonary function in long-term survivors of pediatric hematopoietic cell transplantation. Pediatr Blood Cancer. (2006) 47:594–606. doi: 10.1002/pbc.20531
166. Kunkele A, Engelhard M, Hauffa BP, Mellies U, Muntjes C, Huer C, et al. Long-term follow-up of pediatric patients receiving total body irradiation before hematopoietic stem cell transplantation and post-transplant survival of >2 years. Pediatr Blood Cancer. (2013) 60:1792–7. doi: 10.1002/pbc.24702
167. Leung W, Hudson MM, Strickland DK, Phipps S, Srivastava DK, Ribeiro RC, et al. Late effects of treatment in survivors of childhood acute myeloid leukemia. J Clin Oncol. (2000) 18:3273–9. doi: 10.1200/JCO.2000.18.18.3273
168. Linsenmeier C, Thoennessen D, Negretti L, Bourquin JP, Streller T, Lütolf UM, et al. Total body irradiation (TBI) in pediatric patients. A single-center experience after 30 years of low-dose rate irradiation. Strahlenther Onkol. (2010) 186:614–20. doi: 10.1007/s00066-010-2089-2
169. Morgan TL, Falk PM, Kogut N, Shah KH, Tome M, Kagan AR. A comparison of single-dose and fractionated total-body irradiation on the development of pneumonitis following bone marrow transplantation. Int J Radiat Oncol Biol Phys. (1996) 36:61–6. doi: 10.1016/S0360-3016(96)00246-5
170. Lawton CA, Barber-Derus S, Murray KJ, Casper JT, Ash RC, Gillin MT, et al. Technical modifications in hyperfractionated total body irradiation for T-lymphocyte deplete bone marrow transplant. Int J Radiat Oncol Biol Phys. (1989) 17:319–22. doi: 10.1016/0360-3016(89)90446-X
171. Bruno B, Souillet G, Bertrand Y, Werck-Gallois MC, So Satta A, Bellon G. Effects of allogeneic bone marrow transplantation on pulmonary function in 80 children in a single paediatric centre. Bone Marrow Transplant. (2004) 34:143–7. doi: 10.1038/sj.bmt.1704549
172. Cerveri I, Zoia MC, Fulgoni P, Corsico A, Casali L, Tinelli C, et al. Late pulmonary sequelae after childhood bone marrow transplantation. Thorax. (1999) 54:131–5. doi: 10.1136/thx.54.2.131
173. Faraci M, Barra S, Cohen A, Lanino E, Grisolia F, Miano M, et al. Very late nonfatal consequences of fractionated TBI in children undergoing bone marrow transplant. Int J Radiat Oncol Biol Phys. (2005) 63:1568–75. doi: 10.1016/j.ijrobp.2005.04.031
174. Coppell JA, Richardson PG, Soiffer R, Martin PL, Kernan NA, Chen A, et al. Hepatic veno-occlusive disease following stem cell transplantation: incidence, clinical course, and outcome. Biol Blood Marrow Transplant. (2010) 16:157–68. doi: 10.1016/j.bbmt.2009.08.024
175. de Ledinghen V, Villate A, Robin M, Decraecker M, Valla D, Hillaire S, et al. Sinusoidal obstruction syndrome. Clin Res Hepatol Gastroenterol. (2020) 44:480–5. doi: 10.1016/j.clinre.2020.03.019
176. Helmy A. Review article: updates in the pathogenesis and therapy of hepatic sinusoidal obstruction syndrome. Aliment Pharmacol Ther. (2006) 23:11–25. doi: 10.1111/j.1365-2036.2006.02742.x
177. Radhakrishnan K, Bishop J, Jin Z, Kothari K, Bhatia M, George D, et al. Risk factors associated with liver injury and impact of liver injury on transplantation-related mortality in pediatric recipients of allogeneic hematopoietic stem cell transplantation. Biol Blood Marrow Transplant. (2013) 19:912–7. doi: 10.1016/j.bbmt.2013.02.019
178. Lee SH, Yoo KH, Sung KW, Koo HH, Kwon YJ, Kwon MM, et al. Hepatic veno-occlusive disease in children after hematopoietic stem cell transplantation: incidence, risk factors, and outcome. Bone Marrow Transplant. (2010) 45:1287–93. doi: 10.1038/bmt.2009.349
179. Pulsipher MA, Langholz B, Wall DA, Schultz KR, Bunin N, Carroll WL, et al. The addition of sirolimus to tacrolimus/methotrexate GVHD prophylaxis in children with ALL: a phase 3 children's oncology group/pediatric blood and marrow transplant consortium trial. Blood. (2014) 123:2017–25. doi: 10.1182/blood-2013-10-534297
180. Qiao J, Fu J, Fang T, Huang Y, Mi H, Yang N, et al. Evaluation of the effects of preconditioning regimens on hepatic veno-occlusive disease in mice after hematopoietic stem cell transplantation. Exper Mol Pathol. (2015) 98:73–8. doi: 10.1016/j.yexmp.2014.12.008
181. Hasegawa S, Horibe K, Kawabe T, Kato K, Kojima S, Matsuyama T, et al. Veno-occlusive disease of the liver after allogeneic bone marrow transplantation in children with hematologic malignancies: incidence, onset time and risk factors. Bone Marrow Transplant. (1998) 22:1191–7. doi: 10.1038/sj.bmt.1701506
182. Belkacemi Y, Ozsahin M, Rio B, Laporte JP, Leblond V, Pene F, et al. Is veno-occlusive disease incidence influenced by the total-body irradiation technique? Strahlenther Onkol. (1995) 171:694–7.
183. Ellis MJ, Parikh CR, Inrig JK, Kanbay M, Patel UD. Chronic kidney disease after hematopoietic cell transplantation: a systematic review. Am J Transplant. (2008) 8:2378–90. doi: 10.1111/j.1600-6143.2008.02408.x
184. Cohen EP, Pais P, Moulder JE. Chronic kidney disease after hematopoietic stem cell transplantation. Semin Nephrol. (2010) 30:627–34. doi: 10.1016/j.semnephrol.2010.09.010
185. Miralbell R, Bieri S, Mermillod B, Helg C, Sancho G, Pastoors B, et al. Renal toxicity after allogeneic bone marrow transplantation: the combined effects of total-body irradiation and graft-versus-host disease. J Clin Oncol. (1996) 14:579–85. doi: 10.1200/JCO.1996.14.2.579
186. Abboud I, Porcher R, Robin M, de Latour RP, Glotz D, Socie G, et al. Chronic kidney dysfunction in patients alive without relapse 2 years after allogeneic hematopoietic stem cell transplantation. Biol Blood Marrow Transplant. (2009) 15:1251–7. doi: 10.1016/j.bbmt.2009.05.016
187. Esiashvili N, Chiang KY, Hasselle MD, Bryant C, Riffenburgh RH, Paulino AC. Renal toxicity in children undergoing total body irradiation for bone marrow transplant. Radiother Oncol. (2009) 90:242–6. doi: 10.1016/j.radonc.2008.09.017
188. Gerstein J, Meyer A, Sykora KW, Frühauf J, Karstens JH, Bremer M. Long-term renal toxicity in children following fractionated total-body irradiation (TBI) before allogeneic stem cell transplantation (SCT). Strahlenther Onkol. (2009) 185:751–5. doi: 10.1007/s00066-009-2022-8
189. Gronroos MH, Bolme P, Winiarski J, Berg UB. Long-term renal function following bone marrow transplantation. Bone Marrow Transplant. (2007) 39:717–23. doi: 10.1038/sj.bmt.1705662
190. Klaus R, Niyazi M, Lange-Sperandio B. Radiation-induced kidney toxicity: molecular and cellular pathogenesis. Radiat Oncol. (2021) 16:43. doi: 10.1186/s13014-021-01764-y
191. Igaki H, Karasawa K, Sakamaki H, Saito H, Nakagawa K, Ohtomo K, et al. Renal dysfunction after total-body irradiation. Significance of selective renal shielding blocks. Strahlenther Onkol. (2005) 181:704–8. doi: 10.1007/s00066-005-1405-8
192. Lawton CA, Cohen EP, Murray KJ, Derus SW, Casper JT, Drobyski WR, et al. Long-term results of selective renal shielding in patients undergoing total body irradiation in preparation for bone marrow transplantation. Bone Marrow Transplant. (1997) 20:1069–74. doi: 10.1038/sj.bmt.1701022
193. Fahnehjelm KT, Törnquist AL, Olsson M, Winiarski J. Visual outcome and cataract development after allogeneic stem-cell transplantation in children. Acta Ophthalmol Scand. (2007) 85:724–33. doi: 10.1111/j.1600-0420.2007.00991.x
194. Hall MD, Schultheiss TE, Smith DD, Nguyen KH, Wong JY. Dose response for radiation cataractogenesis: a meta-regression of hematopoietic stem cell transplantation regimens. Int J Radiat Oncol Biol Phys. (2015) 91:22–9. doi: 10.1016/j.ijrobp.2014.07.049
195. Kal HB, Van Kempen-Harteveld ML. Induction of severe cataract and late renal dysfunction following total body irradiation: dose-effect relationships. Anticancer Res. (2009) 29:3305–9.
196. van Kempen-Harteveld ML, van Weel-Sipman MH, Emmens C, Noordijk EM, van der Tweel I, Revesz T, et al. Eye shielding during total body irradiation for bone marrow transplantation in children transplanted for a hematological disorder: risks and benefits. Bone Marrow Transplant. (2003) 31:1151–6. doi: 10.1038/sj.bmt.1704076
197. Tauchmanova L, Selleri C, Rosa GD, Pagano L, Orio F, Lombardi G, et al. High prevalence of endocrine dysfunction in long-term survivors after allogeneic bone marrow transplantation for hematologic diseases. Cancer. (2002) 95:1076–84. doi: 10.1002/cncr.10773
198. Jung MH, Cho KS, Lee JW, Chung NG, Cho B, Suh BK, et al. Endocrine complications after hematopoietic stem cell transplantation during childhood and adolescence. J Korean Med Sci. (2009) 24:1071–7. doi: 10.3346/jkms.2009.24.6.1071
199. Saglio F, Zecca M, Pagliara D, Giorgiani G, Balduzzi A, Calore E, et al. Occurrence of long-term effects after hematopoietic stem cell transplantation in children affected by acute leukemia receiving either busulfan or total body irradiation: results of an AIEOP (associazione italiana ematologia oncologia pediatrica) retrospective study. Bone Marrow Transplant. (2020) 55:1918–27. doi: 10.1038/s41409-020-0806-8
200. Sanders JE, Hoffmeister PA, Woolfrey AE, Carpenter PA, Storer BE, Storb RF, et al. Thyroid function following hematopoietic cell transplantation in children: 30 years' experience. Blood. (2009) 113:306–8. doi: 10.1182/blood-2008-08-173005
201. Oudin C, Auquier P, Bertrand Y, Chastagner P, Kanold J, Poirée M, et al. Late thyroid complications in survivors of childhood acute leukemia. An L.E.A. study. Haematologica. (2016) 101:747–56. doi: 10.3324/haematol.2015.140053
202. Ricardi U, Filippi AR, Biasin E, Ciammella P, Botticella A, Franco P, et al. Late toxicity in children undergoing hematopoietic stem cell transplantation with TBI-containing conditioning regimens for hematological malignancies. Strahlenther Onkol. (2009) 185 (Suppl. 2):17–20. doi: 10.1007/s00066-009-1008-x
203. Shalitin S, Pertman L, Yackobovitch-Gavan M, Yaniv I, Lebenthal Y, Phillip M, et al. Endocrine and metabolic disturbances in survivors of hematopoietic stem cell transplantation in childhood and adolescence. Hormone Res Paediatr. (2018) 89:108–21. doi: 10.1159/000486034
204. Vrooman LM, Millard HR, Brazauskas R, Majhail NS, Battiwalla M, Flowers ME, et al. Survival and late effects after allogeneic hematopoietic cell transplantation for hematologic malignancy at less than three years of age. Biol Blood Marrow Transplant. (2017) 23:1327–34. doi: 10.1016/j.bbmt.2017.04.017
205. Felicetti F, Manicone R, Corrias A, Manieri C, Biasin E, Bini I, et al. Endocrine late effects after total body irradiation in patients who received hematopoietic cell transplantation during childhood: a retrospective study from a single institution. J Cancer Res Clin Oncol. (2011) 137:1343–8. doi: 10.1007/s00432-011-1004-2
206. Bizzarri C, Pinto RM, Ciccone S, Brescia LP, Locatelli F, Cappa M. Early and progressive insulin resistance in young, non-obese cancer survivors treated with hematopoietic stem cell transplantation. Pediatr Blood Cancer. (2015) 62:1650–5. doi: 10.1002/pbc.25603
207. Baker KS, Ness KK, Steinberger J, Carter A, Francisco L, Burns LJ, et al. Diabetes, hypertension, and cardiovascular events in survivors of hematopoietic cell transplantation: a report from the bone marrow transplantation survivor study. Blood. (2007) 109:1765–72. doi: 10.1182/blood-2006-05-022335
208. Taskinen M, Saarinen-Pihkala UM, Hovi L, Lipsanen-Nyman M. Impaired glucose tolerance and dyslipidaemia as late effects after bone-marrow transplantation in childhood. Lancet. (2000) 356:993–7. doi: 10.1016/S0140-6736(00)02717-3
209. Chow EJ, Friedman DL, Yasui Y, Whitton JA, Stovall M, Robison LL, et al. Decreased adult height in survivors of childhood acute lymphoblastic leukemia: a report from the childhood cancer survivor study. J Pediatr. (2007) 150:370–5.e1. doi: 10.1016/j.jpeds.2006.11.036
210. Sanders JE. Growth and development after hematopoietic cell transplant in children. Bone Marrow Transplant. (2008) 41:223–7. doi: 10.1038/sj.bmt.1705875
211. Hoeben BA, Carrie C, Timmermann B, Mandeville HC, Gandola L, Dieckmann K, et al. Management of vertebral radiotherapy dose in paediatric patients with cancer: consensus recommendations from the SIOPE radiotherapy working group. Lancet Oncol. (2019) 20:e155–66. doi: 10.1016/S1470-2045(19)30034-8
212. Bakker B, Oostdijk W, Geskus RB, Stokvis-Brantsma WH, Vossen JM, Wit JM. Patterns of growth and body proportions after total-body irradiation and hematopoietic stem cell transplantation during childhood. Pediatr Res. (2006) 59:259–64. doi: 10.1203/01.pdr.0000199550.71887.ba
213. Couto-Silva AC, Trivin C, Esperou H, Michon J, Baruchel A, Lemaire P, et al. Final height and gonad function after total body irradiation during childhood. Bone Marrow Transplant. (2006) 38:427–32. doi: 10.1038/sj.bmt.1705455
214. Bernard F, Bordigoni P, Simeoni MC, Barlogis V, Contet A, Loundou A, et al. Height growth during adolescence and final height after haematopoietic SCT for childhood acute leukaemia: the impact of a conditioning regimen with BU or TBI. Bone Marrow Transplant. (2009) 43:637–42. doi: 10.1038/bmt.2008.370
215. Thomas BC, Stanhope R, Plowman PN, Leiper AD. Growth following single fraction and fractionated total body irradiation for bone marrow transplantation. Eur J Pediatr. (1993) 152:888–92. doi: 10.1007/BF01957523
216. Bakker B, Oostdijk W, Geskus RB, Stokvis-Brantsma WH, Vossen JM, Wit JM. Growth hormone (GH) secretion and response to GH therapy after total body irradiation and haematopoietic stem cell transplantation during childhood. Clin Endocrinol. (2007) 67:589–97. doi: 10.1111/j.1365-2265.2007.02930.x
217. Sanders JE, Guthrie KA, Hoffmeister PA, Woolfrey AE, Carpenter PA, Appelbaum FR. Final adult height of patients who received hematopoietic cell transplantation in childhood. Blood. (2005) 105:1348–54. doi: 10.1182/blood-2004-07-2528
218. Isfan F, Kanold J, Merlin E, Contet A, Sirvent N, Rochette E, et al. Growth hormone treatment impact on growth rate and final height of patients who received HSCT with TBI or/and cranial irradiation in childhood: a report from the French leukaemia long-term follow-up study (LEA). Bone Marrow Transplant. (2012) 47:684–93. doi: 10.1038/bmt.2011.139
219. Carreras E, Barcelona Endothelium T. Vascular endothelial syndromes after HCT: 2020 update. Bone Marrow Transplant. (2020) 55:1885–7. doi: 10.1038/s41409-020-0852-2
220. Tichelli A, Bhatia S, Socie G. Cardiac and cardiovascular consequences after haematopoietic stem cell transplantation. Br J Haematol. (2008) 142:11–26. doi: 10.1111/j.1365-2141.2008.07165.x
221. Baker KS, Chow E, Steinberger J. Metabolic syndrome and cardiovascular risk in survivors after hematopoietic cell transplantation. Bone Marrow Transplant. (2012) 47:619–25. doi: 10.1038/bmt.2011.118
222. Bielorai B, Weintraub Y, Hutt D, Hemi R, Kanety H, Modan-Moses D, et al. The metabolic syndrome and its components in pediatric survivors of allogeneic hematopoietic stem cell transplantation. Clin Transplant. (2017) 31. doi: 10.1111/ctr.12903
223. Chow EJ, Simmons JH, Roth CL, Baker KS, Hoffmeister PA, Sanders JE, et al. Increased cardiometabolic traits in pediatric survivors of acute lymphoblastic leukemia treated with total body irradiation. Biol Blood Marrow Transplant. (2010) 16:1674–81. doi: 10.1016/j.bbmt.2010.05.016
224. Dengel DR, Kelly AS, Zhang L, Wang Q, Hodges JS, Steinberger J, et al. Vascular structure and function in cancer survivors after hematopoietic stem cell transplantation. Biol Blood Marrow Transplant. (2019) 25:151–6. doi: 10.1016/j.bbmt.2018.08.005
225. Dengel DR, Ness KK, Glasser SP, Williamson EB, Baker KS, Gurney JG. Endothelial function in young adult survivors of childhood acute lymphoblastic leukemia. J Pediatr Hematol Oncol. (2008) 30:20–5. doi: 10.1097/MPH.0b013e318159a593
226. Takatsuka H, Wakae T, Mori A, Okada M, Okamoto T, Kakishita E. Effects of total body irradiation on the vascular endothelium. Clin Transplant. (2002) 16:374–7. doi: 10.1034/j.1399-0012.2002.02035.x
227. Sadurska E, Brodzisz A, Zaucha-Prazmo A, Kowalczyk J. The estimation of intima-media thickness and cardiovascular risk factors in young survivors of childhood cancer. J Pediatr Hematol Oncol. (2016) 38:549–54. doi: 10.1097/MPH.0000000000000513
228. Chow EJ, Mueller BA, Baker KS, Cushing-Haugen KL, Flowers ME, Martin PJ, et al. Cardiovascular hospitalizations and mortality among recipients of hematopoietic stem cell transplantation. Ann Intern Med. (2011) 155:21–32. doi: 10.7326/0003-4819-155-1-201107050-00004
229. Tichelli A, Bucher C, Rovo A, Stussi G, Stern M, Paulussen M, et al. Premature cardiovascular disease after allogeneic hematopoietic stem-cell transplantation. Blood. (2007) 110:3463–71. doi: 10.1182/blood-2006-10-054080
230. Armenian SH, Sun CL, Vase T, Ness KK, Blum E, Francisco L, et al. Cardiovascular risk factors in hematopoietic cell transplantation survivors: role in development of subsequent cardiovascular disease. Blood. (2012) 120:4505–12. doi: 10.1182/blood-2012-06-437178
231. Oudin C, Auquier P, Bertrand Y, Contet A, Kanold J, Sirvent N, et al. Metabolic syndrome in adults who received hematopoietic stem cell transplantation for acute childhood leukemia: an LEA study. Bone Marrow Transplant. (2015) 50:1438–44. doi: 10.1038/bmt.2015.167
232. Daly KP, Colan SD, Blume ED, Margossian R, Gauvreau K, Duncan C, et al. Changes in echocardiographic measures of systolic and diastolic function in children 1 year after hematopoietic SCT. Bone Marrow Transplant. (2011) 46:1532–9. doi: 10.1038/bmt.2010.345
233. Hoffmeister PA, Hingorani SR, Storer BE, Baker KS, Sanders JE. Hypertension in long-term survivors of pediatric hematopoietic cell transplantation. Biol Blood Marrow Transplant. (2010) 16:515–24. doi: 10.1016/j.bbmt.2009.11.018
234. Wei C, Hunt L, Cox R, Bradley K, Elson R, Shield J, et al. Identifying cardiovascular risk in survivors of childhood leukaemia treated with haematopoietic stem cell transplantation and total body irradiation. Hormone Res Paediatr. (2017) 87:116–22. doi: 10.1159/000455046
235. Vatanen A, Sarkola T, Ojala TH, Turanlahti M, Jahnukainen T, Saarinen-Pihkala UM, et al. Radiotherapy-related arterial intima thickening and plaque formation in childhood cancer survivors detected with very-high resolution ultrasound during young adulthood. Pediatr Blood Cancer. (2015) 62:2000–6. doi: 10.1002/pbc.25616
236. Bates JE, Howell RM, Liu Q, Yasui Y, Mulrooney DA, Dhakal S, et al. Therapy-related cardiac risk in childhood cancer survivors: an analysis of the childhood cancer survivor study. J Clin Oncol. (2019) 37:1090–101. doi: 10.1200/JCO.18.01764
237. Barrera M, Atenafu E, Andrews GS, Saunders F. Factors related to changes in cognitive, educational and visual motor integration in children who undergo hematopoietic stem cell transplant. J Pediatr Psychol. (2008) 33:536–46. doi: 10.1093/jpepsy/jsm080
238. Buchbinder D, Kelly DL, Duarte RF, Auletta JJ, Bhatt N, Byrne M, et al. Neurocognitive dysfunction in hematopoietic cell transplant recipients: expert review from the late effects and quality of life working committee of the CIBMTR and complications and quality of life working party of the EBMT. Bone Marrow Transplant. (2018) 53:535–55. doi: 10.1038/s41409-017-0055-7
239. Chou RH, Wong GB, Kramer JH, Wara DW, Matthay KK, Crittenden MR, et al. Toxicities of total-body irradiation for pediatric bone marrow transplantation. Int J Radiat Oncol Biol Phys. (1996) 34:843–51. doi: 10.1016/0360-3016(95)02178-7
240. Parth P, Dunlap WP, Kennedy RS, Ordy JM, Lane NE. Motor and cognitive testing of bone marrow transplant patients after chemoradiotherapy. Percept Mot Skills. (1989) 68 (3 Pt. 2):1227–41. doi: 10.2466/pms.1989.68.3c.1227
241. Perkins JL, Kunin-Batson AS, Youngren NM, Ness KK, Ulrich KJ, Hansen MJ, et al. Long-term follow-up of children who underwent hematopoeitic cell transplant (HCT) for AML or ALL at less than 3 years of age. Pediatr Blood Cancer. (2007) 49:958–63. doi: 10.1002/pbc.21207
242. Smedler AC, Ringden K, Bergman H, Bolme P. Sensory-motor and cognitive functioning in children who have undergone bone marrow transplantation. Acta Paediatr Scand. (1990) 79:613–21. doi: 10.1111/j.1651-2227.1990.tb11525.x
243. Smedler AC, Winiarski J. Neuropsychological outcome in very young hematopoietic SCT recipients in relation to pretransplant conditioning. Bone Marrow Transplant. (2008) 42:515–22. doi: 10.1038/bmt.2008.217
244. Kupst MJ, Penati B, Debban B, Camitta B, Pietryga D, Margolis D, et al. Cognitive and psychosocial functioning of pediatric hematopoietic stem cell transplant patients: a prospective longitudinal study. Bone Marrow Transplant. (2002) 30:609–17. doi: 10.1038/sj.bmt.1703683
245. Parris KR, Russell KM, Triplett BM, Phipps S. Neurocognitive functioning in long-term survivors of pediatric hematopoietic cell transplantation. Bone Marrow Transplant. (2021) 56:873–82. doi: 10.1038/s41409-020-01125-5
246. Phipps S, Rai SN, Leung WH, Lensing S, Dunavant M. Cognitive and academic consequences of stem-cell transplantation in children. J Clin Oncol. (2008) 26:2027–33. doi: 10.1200/JCO.2007.13.6135
247. Simms S, Kazak AE, Golomb V, Goldwein J, Bunin N. Cognitive, behavioral, and social outcome in survivors of childhood stem cell transplantation. J Pediatr Hematol Oncol. (2002) 24:115–9. doi: 10.1097/00043426-200202000-00011
248. Iyer NS, Balsamo LM, Bracken MB, Kadan-Lottick NS. Chemotherapy-only treatment effects on long-term neurocognitive functioning in childhood ALL survivors: a review and meta-analysis. Blood. (2015) 126:346–53. doi: 10.1182/blood-2015-02-627414
249. Reddick WE, Conklin HM. Impact of acute lymphoblastic leukemia therapy on attention and working memory in children. Expert Rev Hematol. (2010) 3:655–9. doi: 10.1586/ehm.10.65
250. Mahajan A, Stavinoha PL, Rongthong W, Brodin NP, McGovern SL, El Naqa I, et al. Neurocognitive effects and necrosis in childhood cancer survivors treated with radiation therapy: a PENTEC comprehensive review. Int J Radiat Oncol Biol Phys. (2021). doi: 10.1016/j.ijrobp.2020.11.073
251. Zajac-Spychala O, Pawlak MA, Karmelita-Katulska K, Pilarczyk J, Jonczyk-Potoczna K, Przepiora A, et al. Long-term brain status and cognitive impairment in children treated for high-risk acute lymphoblastic leukemia with and without allogeneic hematopoietic stem cell transplantation: a single-center study. Pediatr Blood Cancer. (2020) 67:e28224. doi: 10.1002/pbc.28224
252. Wu NL, Krull KR, Cushing-Haugen KL, Ullrich NJ, Kadan-Lottick NS, Lee SJ, et al. Long-term neurocognitive and quality of life outcomes in survivors of pediatric hematopoietic cell transplant. J Cancer Surviv. (2021). doi: 10.1007/s11764-021-01063-1. [Epub ahead of print].
253. Schmiegelow K, Levinsen MF, Attarbaschi A, Baruchel A, Devidas M, Escherich G, et al. Second malignant neoplasms after treatment of childhood acute lymphoblastic leukemia. J Clin Oncol. (2013) 31:2469–76. doi: 10.1200/JCO.2012.47.0500
254. Deeg HJ, Socie G. Malignancies after hematopoietic stem cell transplantation: many questions, some answers. Blood. (1998) 91:1833–44.
255. Keslova P, Formankova R, Riha P, Sramkova L, Snajderova M, Malinova B, et al. Total body irradiation is a crucial risk factor for developing secondary carcinomas after allogeneic hematopoietic stem cell transplantation in childhood. Neoplasma. (2020) 67:1164–9. doi: 10.4149/neo_2020_200214N131
256. Baker KS, DeFor TE, Burns LJ, Ramsay NK, Neglia JP, Robison LL. New malignancies after blood or marrow stem-cell transplantation in children and adults: incidence and risk factors. J Clin Oncol. (2003) 21:1352–8. doi: 10.1200/JCO.2003.05.108
257. Pole JD, Darmawikarta D, Gassas A, Ali M, Egler M, Greenberg ML, et al. Subsequent malignant neoplasms in pediatric cancer patients treated with and without hematopoietic SCT. Bone Marrow Transplant. (2015) 50:721–6. doi: 10.1038/bmt.2015.4
258. Kolb HJ, Socie G, Duell T, Van Lint MT, Tichelli A, Apperley JF, et al. Malignant neoplasms in long-term survivors of bone marrow transplantation. Late effects working party of the European cooperative group for blood and marrow transplantation and the European late effect project group. Ann Intern Med. (1999) 131:738–44. doi: 10.7326/0003-4819-131-10-199911160-00004
259. Gabriel M, Shaw BE, Brazauskas R, Chen M, Margolis DA, Sengelov H, et al. Risk factors for subsequent central nervous system tumors in pediatric allogeneic hematopoietic cell transplant: a study from the center for international blood and marrow transplant research (CIBMTR). Biol Blood Marrow Transplant. (2017) 23:1320–6. doi: 10.1016/j.bbmt.2017.04.004
260. Socie G, Curtis RE, Deeg HJ, Sobocinski KA, Filipovich AH, Travis LB, et al. New malignant diseases after allogeneic marrow transplantation for childhood acute leukemia. J Clin Oncol. (2000) 18:348–57. doi: 10.1200/JCO.2000.18.2.348
261. Inamoto Y, Shah NN, Savani BN, Shaw BE, Abraham AA, Ahmed IA, et al. Secondary solid cancer screening following hematopoietic cell transplantation. Bone Marrow Transplant. (2015) 50:1013–23. doi: 10.1038/bmt.2015.63
262. Lee CJ, Kim S, Tecca HR, Bo-Subait S, Phelan R, Brazauskas R, et al. Late effects after ablative allogeneic stem cell transplantation for adolescent and young adult acute myeloid leukemia. Blood Adv. (2020) 4:983–92. doi: 10.1182/bloodadvances.2019001126
263. Arunagiri N, Kelly SM, Dunlea C, Dixon O, Cantwell J, Bhudia P, et al. The spleen as an organ at risk in paediatric radiotherapy: a SIOP-Europe radiation oncology working group report. Euro J Cancer. (2021) 143:1–10. doi: 10.1016/j.ejca.2020.10.025
264. Inaba H, Yang J, Kaste SC, Hartford CM, Motosue MS, Chemaitilly W, et al. Longitudinal changes in body mass and composition in survivors of childhood hematologic malignancies after allogeneic hematopoietic stem-cell transplantation. J Clin Oncol. (2012) 30:3991–7. doi: 10.1200/JCO.2011.40.0457
265. Mejdahl Nielsen M, Mathiesen S, Suominen A, Sorensen K, Ifversen M, Molgaard C, et al. Altered body composition in male long-term survivors of paediatric allogeneic haematopoietic stem cell transplantation: impact of conditioning regimen, chronic graft-versus-host disease and hypogonadism. Bone Marrow Transplant. (2021) 56:457–60. doi: 10.1038/s41409-020-01038-3
266. Jackson TJ, Mostoufi-Moab S, Hill-Kayser C, Balamuth NJ, Arkader A. Musculoskeletal complications following total body irradiation in hematopoietic stem cell transplant patients. Pediatr Blood Cancer. (2018) 65:e26905. doi: 10.1002/pbc.26905
267. Faraci M, Calevo MG, Lanino E, Caruso S, Messina C, Favr C, et al. Osteonecrosis after allogeneic stem cell transplantation in childhood. A case-control study in Italy. Haematologica. (2006) 91:1096–9.
268. Rizzo JD, Wingard JR, Tichelli A, Lee SJ, Van Lint MT, Burns LJ, et al. Recommended screening and preventive practices for long-term survivors after hematopoietic cell transplantation: joint recommendations of the European group for blood and marrow transplantation, the center for international blood and marrow transplant research, and the American society of blood and marrow transplantation. Biol Blood Marrow Transplant. (2006) 12:138–51. doi: 10.1016/j.bbmt.2005.09.012
269. Ishibashi N, Soejima T, Kawaguchi H, Akiba T, Hasegawa M, Isobe K, et al. National survey of myeloablative total body irradiation prior to hematopoietic stem cell transplantation in Japan: survey of the Japanese radiation oncology study group (JROSG). J Radiat Res. (2018) 59:477–83. doi: 10.1093/jrr/rry017
270. Koken PW, Murrer LHP. Total body irradiation and total skin irradiation techniques in Belgium and the Netherlands: current clinical practice. Adv Radiat Oncol. (2021) 6:100664. doi: 10.1016/j.adro.2021.100664
271. Quast U. Whole body radiotherapy: a TBI-guideline. J Med Phys. (2006) 31:5–12. doi: 10.4103/0971-6203.25664
272. Jahnke A, Jahnke L, Molina-Duran F, Ehmann M, Kantz S, Steil V, et al. Arc therapy for total body irradiation–a robust novel treatment technique for standard treatment rooms. Radiother Oncol. (2014) 110:553–7. doi: 10.1016/j.radonc.2013.12.009
273. Ramm U, Licher J, Moog J, Scherf C, Kara E, Bottcher HD, et al. In vivo dosimetry with semiconducting diodes for dose verification in total-body irradiation. A 10-year experience. Strahlenther Onkol. (2008) 184:376–80. doi: 10.1007/s00066-008-1823-5
274. Van Dyk J, Galvin JM, Glasgow GP, Podgorsak EB. AAPM Report No. 17: The Physical Aspects of Total and Half Body Photon Irradiation. New York, NY: American Association of Physicists in Medicine (1986).
275. Berlon T, Specht L, Petersen PM, Fog L. EP-1829: dose delivery accuracy in total body irradiation delivered with step and shoot IMRT. Radiother Oncol. (2017) 123:S1001–2. doi: 10.1016/S0167-8140(17)32264-8
276. van Leeuwen RGH, Verwegen D, van Kollenburg PGM, Swinkels M, van der Maazen RWM. Early clinical experience with a total body irradiation technique using field-in-field beams and on-line image guidance. Phys Imaging Radiat Oncol. (2020) 16:12–7. doi: 10.1016/j.phro.2020.09.004
277. Pemberton M, Brady C, Taylor B, Tyrrell D, Sim L, Zawlodzka-Bednarz S, et al. Modification of a modulated arc total body irradiation technique: implementation and first clinical experience for paediatric patients. J Med Radiat Sci. (2018) 65:291–9. doi: 10.1002/jmrs.305
278. Pierce G, Balogh A, Frederick R, Gordon D, Yarschenko A, Hudson A. Extended SSD VMAT treatment for total body irradiation. J Appl Clin Med Phys. (2019) 20:200–11. doi: 10.1002/acm2.12519
279. Wong JYC, Filippi AR, Dabaja BS, Yahalom J, Specht L. Total body irradiation: guidelines from the international lymphoma radiation oncology group (ILROG). Int J Radiat Oncol Biol Phys. (2018) 101:521–9. doi: 10.1016/j.ijrobp.2018.04.071
280. Bailey DW, Wang IZ, Lakeman T, Hales LD, Singh AK, Podgorsak MB. TBI lung dose comparisons using bilateral and anteroposterior delivery techniques and tissue density corrections. J Appl Clin Med Phys. (2015) 16:5293. doi: 10.1120/jacmp.v16i2.5293
281. Hui SK, Das RK, Thomadsen B, Henderson D. CT-based analysis of dose homogeneity in total body irradiation using lateral beam. J Appl Clin Med Phys. (2004) 5:71–9. doi: 10.1120/jacmp.2022.25311
282. Hussein S, Kennelly GM. Lung compensation in total body irradiation: a radiographic method. Med Phys. (1996) 23:357–60. doi: 10.1118/1.597799
283. Ekstrand K, Greven K, Wu Q. The influence of x-ray energy on lung dose uniformity in total-body irradiation. Int J Radiat Oncol Biol Phys. (1997) 38:1131–6. doi: 10.1016/S0360-3016(97)00286-1
284. Ho A, Kishel S, Proulx G. Partial lung shield for TBI. Med Dosim. (1998) 23:299–301. doi: 10.1016/S0958-3947(98)00029-6
285. Shank B, O'Reilly RJ, Cunningham I, Kernan N, Yaholom J, Brochstein J, et al. Total body irradiation for bone marrow transplantation: the memorial sloan-kettering cancer center experience. Radiother Oncol. (1990) 18 (Suppl. 1):68–81. doi: 10.1016/0167-8140(90)90180-5
286. Soule BP, Simone NL, Savani BN, Ning H, Albert PS, Barrett AJ, et al. Pulmonary function following total body irradiation (with or without lung shielding) and allogeneic peripheral blood stem cell transplant. Bone Marrow Transplant. (2007) 40:573–8. doi: 10.1038/sj.bmt.1705771
287. Van Dyk J. Dosimetry for total body irradiation. Radiother Oncol. (1987) 9:107–18. doi: 10.1016/S0167-8140(87)80198-6
288. Bragg CM, Wingate K, Conway J. Clinical implications of the anisotropic analytical algorithm for IMRT treatment planning and verification. Radiother Oncol. (2008) 86:276–84. doi: 10.1016/j.radonc.2008.01.011
289. Nakagawa K, Kanda Y, Yamashita H, Hosoi Y, Oshima K, Ohtomo K, et al. Preservation of ovarian function by ovarian shielding when undergoing total body irradiation for hematopoietic stem cell transplantation: a report of two successful cases. Bone Marrow Transplant. (2006) 37:583–7. doi: 10.1038/sj.bmt.1705279
290. Konishi T, Ogawa H, Najima Y, Hashimoto S, Wada A, Adachi H, et al. Safety of total body irradiation using intensity-modulated radiation therapy by helical tomotherapy in allogeneic hematopoietic stem cell transplantation: a prospective pilot study. J Radiat Res. (2020) 61:969–76. doi: 10.1093/jrr/rraa078
291. Salz H, Bohrisch B, Howitz S, Banz N, Weibert K, Wiezorek T, et al. Intensity-modulated total body irradiation (TBI) with TomoDirect. Radiat Oncol. (2015) 10:58. doi: 10.1186/s13014-015-0362-3
292. Sarradin V, Simon L, Huynh A, Gilhodes J, Filleron T, Izar F. Total body irradiation using helical tomotherapy(R): treatment technique, dosimetric results and initial clinical experience. Cancer Radiother. (2018) 22:17–24. doi: 10.1016/j.canrad.2017.06.014
293. Wilhelm-Buchstab T, Leitzen C, Schmeel LC, Simon B, Koch D, Schmeel FC, et al. Total body irradiation: significant dose sparing of lung tissue achievable by helical tomotherapy. Z Med Phys. (2020) 30:17–23. doi: 10.1016/j.zemedi.2019.05.002
294. Springer A, Hammer J, Winkler E, Track C, Huppert R, Bohm A, et al. Total body irradiation with volumetric modulated arc therapy: dosimetric data and first clinical experience. Radiat Oncol. (2016) 11:46. doi: 10.1186/s13014-016-0625-7
295. Mancosu P, Navarria P, Castagna L, Reggiori G, Stravato A, Gaudino A, et al. Plan robustness in field junction region from arcs with different patient orientation in total marrow irradiation with VMAT. Phys Med. (2015) 31:677–82. doi: 10.1016/j.ejmp.2015.05.012
296. Fogliata A, Bergstrom S, Cafaro I, Clivio A, Cozzi L, Dipasquale G, et al. Cranio-spinal irradiation with volumetric modulated arc therapy: a multi-institutional treatment experience. Radiother Oncol. (2011) 99:79–85. doi: 10.1016/j.radonc.2011.01.023
297. Cao F, Ramaseshan R, Corns R, Harrop S, Nuraney N, Steiner P, et al. A three-isocenter jagged-junction IMRT approach for craniospinal irradiation without beam edge matching for field junctions. Int J Radiat Oncol Biol Phys. (2012) 84:648–54. doi: 10.1016/j.ijrobp.2012.01.010
298. Zhou Y, Ai Y, Han C, Zheng X, Yi J, Xie C, et al. Impact of setup errors on multi-isocenter volumetric modulated arc therapy for craniospinal irradiation. J Appl Clin Med Phys. (2020) 21:115–23. doi: 10.1002/acm2.13044
299. Myers P, Stathakis S, Mavroidis P, Esquivel C, Papanikolaou N. Evaluation of localization errors for craniospinal axis irradiation delivery using volume modulated arc therapy and proposal of a technique to minimize such errors. Radiother Oncol. (2013) 108:107–13. doi: 10.1016/j.radonc.2013.05.026
300. Strojnik A, Mendez I, Peterlin P. Reducing the dosimetric impact of positional errors in field junctions for craniospinal irradiation using VMAT. Rep Pract Oncol Radiother. (2016) 21:232–9. doi: 10.1016/j.rpor.2016.03.002
301. Moliner G, Izar F, Ferrand R, Bardies M, Ken S, Simon L. Virtual bolus for total body irradiation treated with helical tomotherapy. J Appl Clin Med Phys. (2015) 16:164–76. doi: 10.1120/jacmp.v16i6.5580
302. Thomas SJ, Hoole AC. The effect of optimization on surface dose in intensity modulated radiotherapy (IMRT). Phys Med Biol. (2004) 49:4919–28. doi: 10.1088/0031-9155/49/21/005
303. Ganapathy K, Kurup PG, Murali V, Muthukumaran M, Bhuvaneshwari N, Velmurugan J. Patient dose analysis in total body irradiation through in vivo dosimetry. J Med Phys. (2012) 37:214–8. doi: 10.4103/0971-6203.103607
304. Malicki J. The accuracy of dose determination during total body irradiation. Strahlenther Onkol. (1999) 175:208–12. doi: 10.1007/BF02742397
305. Shinde A, Wong J. Acute and late toxicities with total marrow irradiation. In: Wong J, Hui SK, editors. Total Marrow Irradiation. 1st ed. New York, NY: Springer Nature (2020). p. 187–96. doi: 10.1007/978-3-030-38692-4_14
306. Han C, Schultheiss TE, Wong JYC. Estimation of radiation-induced secondary cancer risks for early-stage non-small cell lung cancer patients after stereotactic body radiation therapy. Pract Radiat Oncol. (2017) 7:e185–94. doi: 10.1016/j.prro.2016.10.009
307. Stein AS, Al Malki M, Yang D, Palmer JM, Tsai N-C, Aldoss I, et al. Total marrow and lymphoid irradiation (TMLI) at a dose of 2000 cGy in combination with post-transplant cyclophosphamide (PTCy)-based graft versus host disease (GHVD) prophylaxis is safe and associated with favorable GVHD-free/relapse-free survival at 1 year in patients with acute myeloid leukemia (AML). In: 62nd Amercian Society of Hematology Annual Meeting and Exposition (San Diego, CA). (2020). doi: 10.1182/blood-2020-141469
308. Wong JYC, Liu A, Schultheiss T, Popplewell L, Stein A, Rosenthal J, et al. Targeted total marrow irradiation using three-dimensional image-guided tomographic intensity-modulated radiation therapy: an alternative to standard total body irradiation. Biol Blood Marrow Transplant. (2006) 12:306–15. doi: 10.1016/j.bbmt.2005.10.026
309. Rosenthal J, Wong J, Stein A, Qian D, Hitt D, Naeem H, et al. Phase 1/2 trial of total marrow and lymph node irradiation to augment reduced-intensity transplantation for advanced hematologic malignancies. Blood. (2011) 117:309–15. doi: 10.1182/blood-2010-06-288357
310. Hui SK, Kapatoes J, Fowler J, Henderson D, Olivera G, Manon RR, et al. Feasibility study of helical tomotherapy for total body or total marrow irradiation. Med Phys. (2005) 32:3214–24. doi: 10.1118/1.2044428
311. Stein A, Palmer J, Tsai NC, Al Malki MM, Aldoss I, Ali H, et al. Phase I trial of total marrow and lymphoid irradiation transplantation conditioning in patients with relapsed/refractory acute leukemia. Biol Blood Marrow Transplant. (2017) 23:618–24. doi: 10.1016/j.bbmt.2017.01.067
312. Zhuang AH, Liu A, Schultheiss TE, Wong JY. Dosimetric study and verification of total body irradiation using helical tomotherapy and its comparison to extended SSD technique. Med Dosim. (2010) 35:243–9. doi: 10.1016/j.meddos.2009.07.001
313. Aydogan B, Mundt AJ, Roeske JC. Linac-based intensity modulated total marrow irradiation (IM-TMI). Technol Cancer Res Treat. (2006) 5:513–9. doi: 10.1177/153303460600500508
314. Wilkie JR, Tiryaki H, Smith BD, Roeske JC, Radu CG, Aydogan B. Feasibility study for linac-based intensity modulated total marrow irradiation. Med Phys. (2008) 35:5609–18. doi: 10.1118/1.2990779
315. Aydogan B, Yeginer M, Kavak GO, Fan J, Radosevich JA, Gwe-Ya K. Total marrow irradiation with rapidArc volumetric arc therapy. Int J Radiat Oncol Biol Phys. (2011) 81:592–9. doi: 10.1016/j.ijrobp.2010.11.035
316. Fogliata A, Cozzi L, Clivio A, Ibatici A, Mancosu P, Navarria P, et al. Preclinical assessment of volumetric modulated arc therapy for total marrow irradiation. Int J Radiat Oncol Biol Phys. (2011) 80:626–36. doi: 10.1016/j.ijrobp.2010.11.028
317. Wong JY, Forman S, Somlo G, Rosenthal J, Liu A, Schultheiss T, et al. Dose escalation of total marrow irradiation with concurrent chemotherapy in patients with advanced acute leukemia undergoing allogeneic hematopoietic cell transplantation. Int J Radiat Oncol Biol Phys. (2013) 85:148–56. doi: 10.1016/j.ijrobp.2012.03.033
318. Stein A, Tsai N-C, Palmer J, Al Malki M, Aldoss I, Ali H, et al. Total marrow and lymphoid irradiation (TMLI) in combination with cyclophosphamide and etoposide in patients with relapsed/refractory acute leukemia undergoing allogeneic hematopoietic cell transplantation. In: European Society for Blood and Marrow Transplantation Meeting. Frankfurt (2019).
319. Patel P, Aydogan B, Koshy M, Mahmud D, Oh A, Saraf SL, et al. Combination of linear accelerator-based intensity-modulated total marrow irradiation and myeloablative fludarabine/busulfan: a phase I study. Biol Blood Marrow Transplant. (2014) 20:2034–41. doi: 10.1016/j.bbmt.2014.09.005
320. Hui S, Brunstein C, Takahashi Y, DeFor T, Holtan SG, Bachanova V, et al. Dose escalation of total marrow irradiation in high-risk patients undergoing allogeneic hematopoietic stem cell transplantation. Biol Blood Marrow Transplant. (2017) 23:1110–6. doi: 10.1016/j.bbmt.2017.04.002
321. Jensen LJ, Stiller T, Wong JYC, Palmer J, Stein A, Rosenthal J. Total marrow lymphoid irradiation/fludarabine/melphalan conditioning for allogeneic hematopoietic cell transplantation. Biol Blood Marrow Transplant. (2018) 24:301–7. doi: 10.1016/j.bbmt.2017.09.019
322. Welliver MX, Vasu S, Weldon M, Zoller W, Addington M, Eiler D, et al. Utilizing organ-sparing marrow-targeted irradiation (OSMI) to condition patients with high-risk hematologic malignancies prior to allogeneic hematopoietic stem cell transplantation: results from a prospective pilot study. Int J Radiat Oncol Biol Phys. (2018) 102:E370. doi: 10.1016/j.ijrobp.2018.07.1108
323. Al Malki MM, Palmer J, Wong J, Hui S, Mokhtari S, Gendzekhadze K, et al. Phase I study of escalating doses of total marrow and lymphoid irradiation (TMLI) during conditioning for HLA-haploidentical hematopoietic cell transplantation (HaploHCT) with post-transplant cyclophosphamide (PTCy) in patients with myelodysplasia or acute leukemia. In: European Society for Blood and Marrow Transplantation Meeting. Frankfurt (2019).
324. Arslan S, Al-Malki M. Total marrow and lymphoid irradiation in patient undergoing haplo-identical transplants. In: Wong J, Hui SK, editors. Total Marrow Irradiation. 1st ed. New York, NY: Springer Nature (2020). p. 123–34. doi: 10.1007/978-3-030-38692-4_8
325. Pierini A, Ruggeri L, Carotti A, Falzetti F, Saldi S, Terenzi A, et al. Haploidentical age-adapted myeloablative transplant and regulatory and effector T cells for acute myeloid leukemia. Blood Adv. (2021) 5:1199–208. doi: 10.1182/bloodadvances.2020003739
326. Haraldsson A, Wichert S, Engstrom PE, Lenhoff S, Turkiewicz D, Warsi S, et al. Organ sparing total marrow irradiation compared to total body irradiation prior to allogeneic stem cell transplantation. Eur J Haematol. (2021) 107:393–407. doi: 10.1111/ejh.13675
327. Bearman SI, Appelbaum FR, Buckner CD, Petersen FB, Fisher LD, Clift RA, et al. Regimen-related toxicity in patients undergoing bone marrow transplantation. J Clin Oncol. (1988) 6:1562–8. doi: 10.1200/JCO.1988.6.10.1562
328. Clift RA, Buckner CD, Appelbaum FR, Bryant E, Bearman SI, Peterson FB, et al. Allogeneic marrow transplantation in patients with chronic myeloid leukemia in the chronic phase: a randomized trial of two irradiation regimens. Blood. (1991) 77:1660–5. doi: 10.1182/blood.V77.8.1660.bloodjournal7781660
329. Wong J. Total marrow irradiation: redefining the role of radiotherapy in bone marrow transplantation. In: Wong J, Hui SK, editors. Total Marrow Irradiation. 1st ed. New York, NY: Springer Nature (2020). p. 1–28. doi: 10.1007/978-3-030-38692-4_1
330. Bao Z, Zhao H, Wang D, Gong J, Zhong Y, Xiong Y, et al. Feasibility of a novel dose fractionation strategy in TMI/TMLI. Radiat Oncol. (2018) 13:248. doi: 10.1186/s13014-018-1201-0
331. Deeg HJ, Sandmaier BM. Who is fit for allogeneic transplantation? Blood. (2010) 116:4762–70. doi: 10.1182/blood-2010-07-259358
332. Scott BL, Pasquini MC, Logan B, Wu J, Devine S, Porter DL, et al. Results of a phase III randomized, multicenter study of allogeneic stem cell transplantation after high versus reduced intensity conditioning in patients with myelodysplastic syndrome (MDS) or acute myeloid leukemia (AML): blood and marrow transplant clinical trials network (BMT CTN) 0901. Blood. (2015) 126:LBA−8. doi: 10.1182/blood.V126.23.LBA-8.LBA-8
333. Luznik L, Jalla S, Engstrom LW, Iannone R, Fuchs EJ. Durable engraftment of major histocompatibility complex-incompatible cells after nonmyeloablative conditioning with fludarabine, low-dose total body irradiation, and posttransplantation cyclophosphamide. Blood. (2001) 98:3456–64. doi: 10.1182/blood.V98.12.3456
334. O'Donnell PV, Luznik L, Jones RJ, Vogelsang GB, Leffell MS, Phelps M, et al. Nonmyeloablative bone marrow transplantation from partially HLA-mismatched related donors using postransplantation cyclophosphamide. Biol Blood Marrow Transplant. (2002) 8:377–86. doi: 10.1053/bbmt.2002.v8.pm12171484
335. Aristei C, Saldi S, Pierini A, Ruggeri L, Piccine S, Ingrosso G, et al. Total marrow/lymphoid irradiation in the conditioning regimen for haploidentical T-cell depleted hematopoietic stem cell transplantation for acute myeloid leukemia. In: Wong J, Hui SK, editors. Total Marrow Irradiation. 1st ed. New York, NY: Springer Nature (2020). p. 111–22. doi: 10.1007/978-3-030-38692-4_7
336. Dandapani SV, Wong JYC. Modern total body irradiation (TBI): intensity-modulated radiation treatment (IMRT). In: Wong JYC, Hui SK, editors. Total Marrow Irradiation: A Comprehensive Review. Cham: Springer International Publishing (2020). p. 177–85. doi: 10.1007/978-3-030-38692-4_13
337. Sun R, Cuenca X, Itti R, Nguyen Quoc S, Vernant JP, Mazeron JJ, et al. First French experiences of total body irradiations using helical TomoTherapy((R)). Cancer Radiother. (2017) 21:365–72. doi: 10.1016/j.canrad.2017.01.014
338. Kim JH, Stein A, Tsai N, Schultheiss TE, Palmer J, Liu A, et al. Extramedullary relapse following total marrow and lymphoid irradiation in patinets undergoing allogenejic hematopoietic cell transplantation. Int J Radiat Oncol Biol Phys. (2014) 89:75–81. doi: 10.1016/j.ijrobp.2014.01.036
Keywords: haematopoietic stem cell transplantation (HSCT), total body irradiation (TBI), total marrow irradiation (TMI), total lymph node irradiation (TLI), acute lymphoblastic leukaemia (ALL), total marrow and lymphatic irradiation, paediatric
Citation: Hoeben BAW, Wong JYC, Fog LS, Losert C, Filippi AR, Bentzen SM, Balduzzi A and Specht L (2021) Total Body Irradiation in Haematopoietic Stem Cell Transplantation for Paediatric Acute Lymphoblastic Leukaemia: Review of the Literature and Future Directions. Front. Pediatr. 9:774348. doi: 10.3389/fped.2021.774348
Received: 11 September 2021; Accepted: 03 November 2021;
Published: 03 December 2021.
Edited by:
Daniele Zama, Sant'Orsola-Malpighi Polyclinic, ItalyReviewed by:
Charalampos Dokos, Aristotle University of Thessaloniki, GreeceSajad Khazal, University of Texas MD Anderson Cancer Center, United States
Alisa B. Lee Sherick, University of Colorado Denver, United States
Copyright © 2021 Hoeben, Wong, Fog, Losert, Filippi, Bentzen, Balduzzi and Specht. This is an open-access article distributed under the terms of the Creative Commons Attribution License (CC BY). The use, distribution or reproduction in other forums is permitted, provided the original author(s) and the copyright owner(s) are credited and that the original publication in this journal is cited, in accordance with accepted academic practice. No use, distribution or reproduction is permitted which does not comply with these terms.
*Correspondence: Bianca A. W. Hoeben, Yi5hLncuaG9lYmVuQHVtY3V0cmVjaHQubmw=