- 1Department of Anesthesiology, Intensive Care Medicine and Pain Therapy, University Hospital Frankfurt, Frankfurt, Germany
- 2Clinic of Internal Medicine III, Division of Nephrology, University Hospital Frankfurt, Frankfurt, Germany
- 3Institute of Biochemistry I, Goethe University Frankfurt, Frankfurt, Germany
- 4Clinic of Urology and Pediatric Urology, Philipps University of Marburg, Marburg, Germany
- 5Department of Biomedicine, Aarhus University, Aarhus, Denmark
Early and adequate restoration of endothelial and tubular renal function is a substantial step during regeneration after ischemia reperfusion (IR) injury, occurring, e.g., in kidney transplantation, renal surgery, and sepsis. While tubular epithelial cell injury has long been of central importance, recent perception includes the renal vascular endothelium. In this regard, the fibrin cleavage product fibrinopeptide Bβ15-42 mitigate IR injury by stabilizing interendothelial junctions through its affinity to VE-cadherin. Therefore, this study focused on the effect of Bβ15-42 on post-acute physiological renal regeneration. For this, adult male C57BL/6 mice were exposed to a 30 min bilateral renal ischemia and reperfusion for 24 h or 48 h. Animals were randomized in a non-operative control group, two operative groups each treated with i.v. administration of either saline or Bβ15-42 (2.4 mg/kg) immediately prior to reperfusion. Endothelial activation and inflammatory response was attenuated in renal tissue homogenates by single application of Bβ15-42. Meanwhile, Bβ15-42 did not affect acute kidney injury markers. Regarding the angiogenetic players VEGF-A, Angiopoietin-1, Angiopoietin-2, however, we observed significant higher expressions at mRNA and trend to higher protein level in Bβ15-42 treated mice, compared to saline treated mice after 48 h of IR, thus pointing toward an increased angiogenetic activity. Similar dynamics were observed for the intermediate filament vimentin, the cytoprotective protein klotho, stathmin and the proliferation cellular nuclear antigen, which were significantly up-regulated at the same points in time. These results suggest a beneficial effect of anatomical contiguously located endothelial cells on tubular regeneration through stabilization of endothelial integrity. Therefore, it seems that Bβ15-42 represents a novel pharmacological approach in the targeted therapy of acute renal failure in everyday clinical practice.
Introduction
Ischemia reperfusion injury (IR) leads to the development of acute kidney injury (AKI) characterized by a massive tubular cell death, mainly affecting proximal tubules within the outer stripe of the outer medulla (Bonventre, 2010). At the same time, endothelial cells in the renal vasculature undergo an early swelling, leading to the narrowing of the lumen (Flores et al., 1972; Brodsky et al., 2002). Additionally, loosening of interendothelial connections and the subsequent formation of interendothelial gaps with leak formation leads to dysfunction of the blood–tissue barrier (Basile, 2007), possibly influencing recovery of the affected segments. These renal alterations rapidly evoke a process of regeneration, leading to restoration of both normal tubular and endothelial architecture. Hence, endothelial injury and capillary loss in the immediate vicinity of damaged renal epithelial tubules seem to affect tubular recovery and are therefore of particular interest.
In this context, vascular endothelial (VE)-cadherin is one of the most important endothelial anchor proteins which is connected to the actin-based cytoskeleton and one of the key molecules integrating and tightening endothelial cell junctions maintaining the vascular barrier integrity (Dejana et al., 2001; Broman et al., 2007; Vestweber, 2008). In turn, endothelial cell VE-cadherin interacts with the β15-42 sequence of fibrin (Bach et al., 1998; Gorlatov and Medved, 2002; Petzelbauer et al., 2005). Bβ15-42, also known as FX06, is a naturally occurring 28 amino acid cleavage product of fibrin. Its biological properties were first described in Petzelbauer et al. (2005). In supra-physiologic doses, this peptide has proven its efficacy to prevent VE-cadherin disruption, and thus micro-vascular dysfunction, finally achieving an organo-protection in myocardial IR injury in rodents (Petzelbauer et al., 2005), pigs (Roesner et al., 2007) as well as in humans (Atar et al., 2009), and recently in a clinical case of vascular leak syndrome during Ebola virus disease (Wolf et al., 2015).
Within murine ischemic kidneys, fibrinogen α, β, and γ chain mRNA were identified to be significantly up-regulated (Krishnamoorthy et al., 2011). Furthermore, application of fibrinogen-derived Bβ15-42 peptide (3.6 mg/kg at reperfusion) demonstrated a therapeutic potential in terms of vascular congestion, kidney dysfunction, and proximal tubular damage (Krishnamoorthy et al., 2011). In a different study, Bβ15-42 proved to alleviate the development of IR damage in mouse models of ischemic AKI as well as renal transplantation (3 mg/kg shortly before and 5 min after reperfusion, respectively) (Sorensen et al., 2011). Own recent findings identified Bβ15-42 to exert beneficial effects during the early phase of murine IR injury through reduction of endothelial activation, invasion of neutrophil granulocytes as well as tubular damage (2.4 mg/kg) (Urbschat et al., 2014, 2015).
The aforementioned studies support the concept that supra-physiological presence of Bβ15-42 serves as a protective agent in renal IR injury. In the present study, we thus focus on the initiation of physiologic regenerative processes regarding endothelial as well as tubular epithelial cell repair and their linkage in sequential points of time in a murine model of renal IR.
Materials and Methods
Animals
50–60 days old male C57BL/6 mice (Janvier) were kept in the central research facility of the University Hospital Frankfurt. They were housed with water and food ad libitum in rooms with a 12 h light cycle. All procedures involving animals were approved by the Animal Care and Use Committee of the state of Hesse in Germany (V54-19c20/15-F35/04). Surgery and animal care were performed in accordance with the “Guide for the care and use of laboratory animals” (National Institutes of Health, volume 25, no. 28, revised 1996), EU Directive 86/609 EEC and German Protection of Animals Act.
Interventional Groups
Mice were randomized into five groups (n = 8 per group): one control group without intervention (CTRL), two interventional groups with 24 h (saline 24 h) or 48 h (saline 48 h) of IR and intravenous (i.v.) application of saline immediately prior to reperfusion as well as two treated interventional groups with 24 h (Bβ15-42 24 h) or 48 h (Bβ15-42 48 h) of IR and i.v. application of the fibrin fragment Bβ15-42 immediately prior to reperfusion. Before the procedure, mice were anesthetized with an intra peritoneal (i.p.) injection of ketamine (100 mg/kg body weight) and xylazine (5 mg/kg body weight). After bilateral dorsal flank incision, renal arteries were clamped for 30 min under microscopic control using non-traumatic microvascular clamps with a jaw pressure of 85 g (Micro-Serrefine 8 mm, Fine surgical instruments). Bβ15-42 or saline was administered i.v. immediately prior to the removal of the clamp. Hereafter, the recovery of blood flow was visually inspected. Subsequently, incisions were closed in layers and mice were allowed to recover. Animals were sacrificed after 24 or 48 h of IR, complete kidneys carefully removed, and divided longitudinally into halves. One half of which was placed in 4% paraformaldehyde overnight, the other was stored at -80°C together with blood samples, pending further processing.
Reagents and Dosage
Bβ15-42 (amino acid sequence GHRPLDKKREEAPSLRPAPPPISGGGYR) was kindly provided by Prof. Petzelbauer, Medical University of Vienna. Mice received a single bolus of 2.4 mg/kg body weight Bβ15-42 in a total volume of 100 μl via i.v. application immediately prior to reperfusion. The selected dose was based on a previous study in rodent myocardial IR evaluating various dosing regimens (Zacharowski et al., 2007) and according to previous own studies (Urbschat et al., 2014, 2015). 0.9% saline was used as control which has no effect on prevention of renal injury induced by IR nor on renal function recovery (Li et al., 2016).
Isolation and Culture of Murine Proximal Tubular Epithelial Cells
Murine primary proximal tubular cells (PTCs) were obtained from C57BL/6 mice, as described earlier (Baer et al., 1997). In brief, after kidney removal, the tissue was minced and digested with collagenase/dispase. The digested fragments were passed through a 106-μm mesh. The cell pellet was preincubated with mouse immunoglobulin G (mIgG, 5 mg/ml) to block unspecific binding. To enrich PTCs, we used a rat-anti-mouse antibody against aminopeptidase M (CD13, GTX62507, GeneTex). Finally, cells were incubated with a bead-conjugated anti-rat secondary antibody and isolated by immunomagnetic separation applying the Mini-MACS system (Miltenyi). Isolated cells were grown in DMEM/HAM’s F12 (1:1) with GlutaMAX (31331-028, Gibco), supplemented with 10% FCS and 1% Penicillin/Streptomycin at 37°C and 5% CO2 in a humidified atmosphere.
In Vitro Experimental Anoxia
For anoxia assays PTCs were plated in 96-well plates and chamberslides in DMEM containing 10% FCS. Subconfluent cells were exposed to anoxia for 1 h by either adding a layer of mineral oil (M5310, Sigma-Aldrich) on top of medium (designated as medium+oil) or completely immersed in sterile filtered mineral oil (designated as pure oil). Oil immersion mimicks in vivo ischemic conditions by restricting cellular exposure to oxygen and nutrients as well as limiting metabolite washout (Meldrum et al., 2001). After 1 h, mineral oil and medium was removed, cells were washed with PBS, and incubated either with or without addition of 6 μM Bβ15-42 to the medium with the start of reoxygenation for 24 or 48 h, which is in line with the animal trial.
RNA Isolation and Real-Time-PCR
Total RNA was isolated from homogenized kidney samples (n = 8 per group) using TRI Reagent (T9424, Sigma-Aldrich) according to the manufacturer’s protocol. cDNA was synthesized using an iScript cDNA Synthesis kit (1708891, Bio-Rad Laboratories). Gene expression profiles from all samples (n = 8 per group) were assessed in duplicates by quantitative real-time polymerase chain reaction using a StepOne Plus Realtime PCR device (Applied Biosystems). Primer sequences are listed in Table 1. Relative changes in mRNA expression were calculated by normalizing the values to their corresponding 18S mRNA expression using the 2-dCt method (Livak and Schmittgen, 2001).
Protein Isolation and Western Blot Analyses
Cryosections of whole renal tissue (n = 8 per group) were lysed in ice-cold lysis buffer with phosphatase-inhibitor and protease-inhibitor. After centrifugation, supernatants were removed and total protein was determined applying the bicinchoninic acid method. 10% SDS-gels were loaded with 50 μg protein. Two consecutive samples of all of five intervention groups were analyzed on four membranes with Spectra Multicolor Broad Range Protein Ladder and MagicMark (26623 and LC5602, Thermo Fisher Scientific) as a standard. Nitrocellulose membranes were incubated with the following antibodies: Angiopoietin-1 (AF923, R&D Systems, dilution 1:1000), Angiopoietin-2 (GTX100928, GeneTex, dilution 1:1000), klotho (MAB1819, R&D, dilution 1:500), phosphorylated Tie-2 (AF3909, R&D, 1:200), Tie-2 (GTX107838, GeneTex, dilution 1:500), vascular endothelial growth factor (VEGF-A) (ab68334, Abcam, dilution 1:1000), VE-cadherin (CD144, 138101, BioLegend, dilution 1:1000), vimentin (MA5-11883, Thermo Fisher Scientific, dilution 1:1000), and β-actin (A5441, Sigma-Aldrich, dilution 1:10000). Above proteins were investigated in all samples (n = 8 per group). Proteins were immunodetected by enhanced chemiluminescence (Santa Cruz Biotechnology). Digitalization and evaluation of the blots were performed with a Kodak Imager (Carestream) and protein densitometry was determined using ImageJ. For densitometry of Angiopoietin-2, phosphorylated Tie-2, Tie-2, and VE-cadherin both bands were included. Original western blot images are shown in Supplementary Figures S2–S4.
Immunohistochemical and Immunofluorescence Staining
Immunohistochemistry for Lipocalin-2 (Lcn-2), pTie-2, Tie-2, and immunofluorescence staining for stathmin and proliferation cellular nuclear antigen (PCNA) was performed. For specific immunohistochemical staining, slides were incubated with the following antibodies: Lcn-2 (MAB1857, R&D Systems, dilution 1:500), pTie-2 (Y1102/Y1100, AF3909, R&D Systems, dilution 1:100), Tie-2 (C1C3, GTX107838, GeneTex, dilution 1:500), and a non-primary antibody control for each antibody. For development, slides were incubated with catalyzed signal amplification system (REF K1500, DAKO). Counterstaining was done with hematoxylin for 10 min. Analyses were performed in a blinded manner. 10 fields of randomly taken pictures from each slide (n = 8 per group) were analyzed. Quantification of Lcn-2 was done using a script, programmed in Matlab (The Mathworks). The software determined the values of each pixel in the RGB space, thus allowing to measure the relative area occupied by brown pixels in relationship to blue pixels. Calculation was performed as previously published (Urbschat et al., 2014, 2015). In order to detect proliferation, slides were incubated with antibodies against stathmin (ab52630, Abcam, dilution 1:500) and Alexa Fluor 488-labeled secondary antibodies (A11070, Invitrogen, dilution 1:2000) as well as antibodies against PCNA (Santa-Cruz, sc-56, dilution 1:1000) and Alexa Fluor 546-labeled secondary antibodies (A11018, Invitrogen, dilution 1:2000). Immunofluorescence staining was performed in n = 4 per group. For analysis of F-actin fibers in primary mouse PTCs, cells were seeded in 8-well chamber slides and fixed with ice cold acetone and methanol. In order to visualize cell nuclei and actin fibers, the cells were stained with Hoechst (33342, Invitrogen by Thermo Fisher, dilution 1:5000) and rhodamine-phalloidin (P1951, Sigma, dilution 1:12000), diluted in 3% BSA in PBS for 1 h. Cells were washed in PBS and mounted with Glycergel (C0563, Dako) containing antifade. Images were acquired using the same settings for all samples. Brightness and contrast were adjusted individually for each image. Immunofluorescence staining of PTCs was performed once. Image analysis was performed with Image J software.
Measurement of Serum Creatinine
Colorimetric estimation of serum creatinine was performed in duplicate (n = 8 per group) using the alkaline picrate method (Jaffé’s Method, LT-SYS Creatinin Jaffé, LT-CR 0121, Eberhard Lehmann GmbH). Absorbance was measured at wavelength 492 nm.
XTT
Viability of murine PTCs was determined by a photometric assay using 2,3-Bis-(2-Methoxy-4-Nitro-5-Sulfophenyl)-2H-Tetrazolium-5-Carboxanilide (XTT). In brief, subconfluent cells in 96-well plates were exposed to anoxia for 1 h with subsequent reoxygenation for 24 h or 48 h, respectively. Hereafter, XTT reagent was added to each well as described by the manufacturer (A8088, Applichem) and incubation was continued at 37°C. Absorbance was measured in a microplate reader (ELx808, Bio-TEC Instruments, Inc.) at 450 nm vs. 630 nm. Experiments were conducted in triplicate in three independent experimental settings and are represented as mean ± SEM. The value of viability is expressed as percentage of viability of untreated control cells set as 100%.
Statistical Analyses
Statistical analyses were performed applying GraphPad Prism® 5.02 software (GraphPad Software). The distribution of variables was tested for normality using the Kolmogorov–Smirnov test. Accordingly, statistical significance was calculated using one-way ANOVA followed by Tukey’s multiple comparison test or Kruskal–Wallis test followed by Dunn’s post hoc test, where applicable. Significance of correlations was determined by Spearman’s test including all investigated groups. p-Values ≤ 0.05 were assumed as statistically significant. In the figures, horizontal lines within the boxes represent the medians, boxes represent the interquartile range (25–75%). Whiskers above and below the box indicate the 90th and 10th percentiles. The individual points that are plotted beyond the whiskers represent outliers, which were included in the statistical analyses.
Results
Reduced Endothelial Activation Upon Treatment With Bβ15-42
First, we examined gene expressions of intracellular adhesion molecule-1 (ICAM-1) and P-selectin, as typical markers of endothelial activation (Figure 1A). ICAM-1 as well as P-selectin mRNA increased significantly at 24 h after IR in saline treated mice (p < 0.001) and to a significantly lower extend in Bβ15-42 treated mice (p < 0.001), although this differences abolished 48 h after IR (Figure 1B). Accordingly, we could observe a positive correlation between ICAM-1 and P-selectin (Pearson r = 0.958; p < 0.001) (Figure 1A). VE-cadherin represents a key molecule integrating and tightening endothelial cell junctions. Its mRNA decreased in both saline and Bβ15-42 treated mice after 24 and 48 h, compared to the control group. However, no marked difference was found between saline and Bβ15-42 treated mice at both points in time (Figure 1B). The corresponding VE-cadherin protein expression significantly increased after 24 h of IR but reached again levels of the control group after 48 h of IR, still no marked difference was found between saline and Bβ15-42 treated mice at both points in time (Figure 1B). In summary, we observed a significant endothelial activation in Bβ15-42 treated mice 24 h after IR.
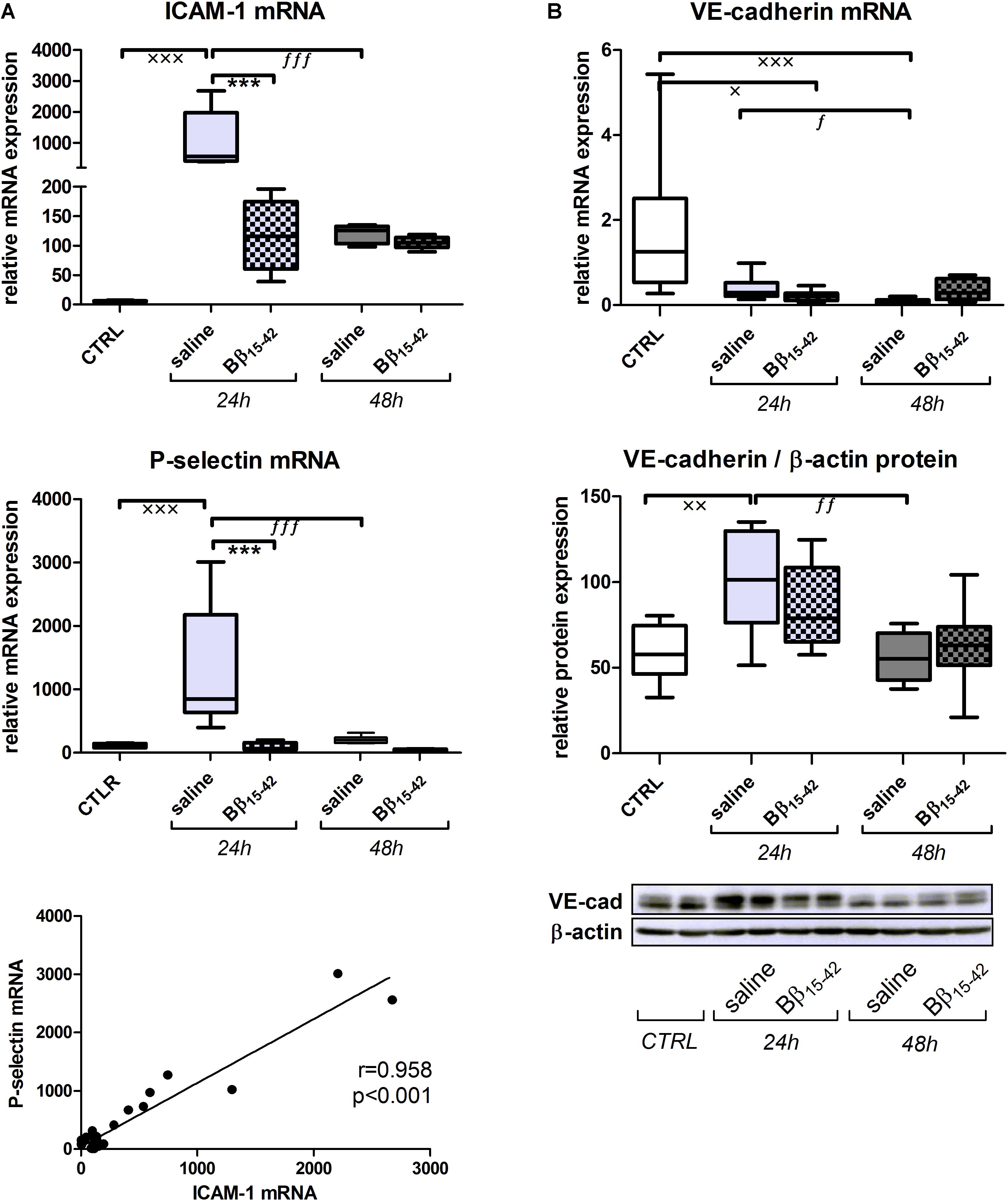
FIGURE 1. Bβ15-42 reduces inflammatory response and endothelial activation. To analyze the endothelial activation (A) and endothelial integrity (B) after IR injury, we performed RT-PCR analyses relative to 18S and western blot analyses relative to β-actin of relevant genes and proteins in kidney tissue homogenates (n = 8 per group for RT-PCR and western blot analyses). ∗ Significant difference between Bβ15-42 and saline treated mice at one point in time; x significant difference to CTRL; f significant difference in the time course within Bβ15-42 treated or saline treated mice. x/f p < 0.05; xx/ff p < 0.01; ∗∗∗/xxx/fff p < 0.001.
No Influence of Bβ15-42 on Kidney Injury Marker Within the Observed Points in Time
Lipocalin 2 (Lcn-2), also called neutrophil gelatinase associated lipocalin (NGAL) and kidney injury molecule 1 (KIM-1) represent early biomarkers of ischemic AKI. Their mRNA expression was markedly elevated in both Bβ15-42 and saline treated mice at 24 and 48 h of IR, when compared to controls (p < 0.001) (Figure 2A). Likewise, levels of serum creatinine, which accumulates over time, rose significantly after 24 h in comparison to control mice but declined after 48 h of IR. However, none of the injury markers displayed differences between Bβ15-42 and saline treated mice at any point in time (Figure 2A). In order to quantify Lcn-2 tissue distribution, histological staining was performed and 10 randomly taken pictures from each slide were analyzed focusing on the cortex and outer medulla (n = 8 per group) (Figure 2B). Animals which received saline or Bβ15-42 exhibited increased Lcn-2 expression over time mainly in the distal tubule. Its expression reached a significant increase at 48 h of IR relative to the controls (saline: p < 0.01; Bβ15-42: p < 0.05) but no difference between Bβ15-42 and saline treated mice could be detected at any point in time (Figure 2B).
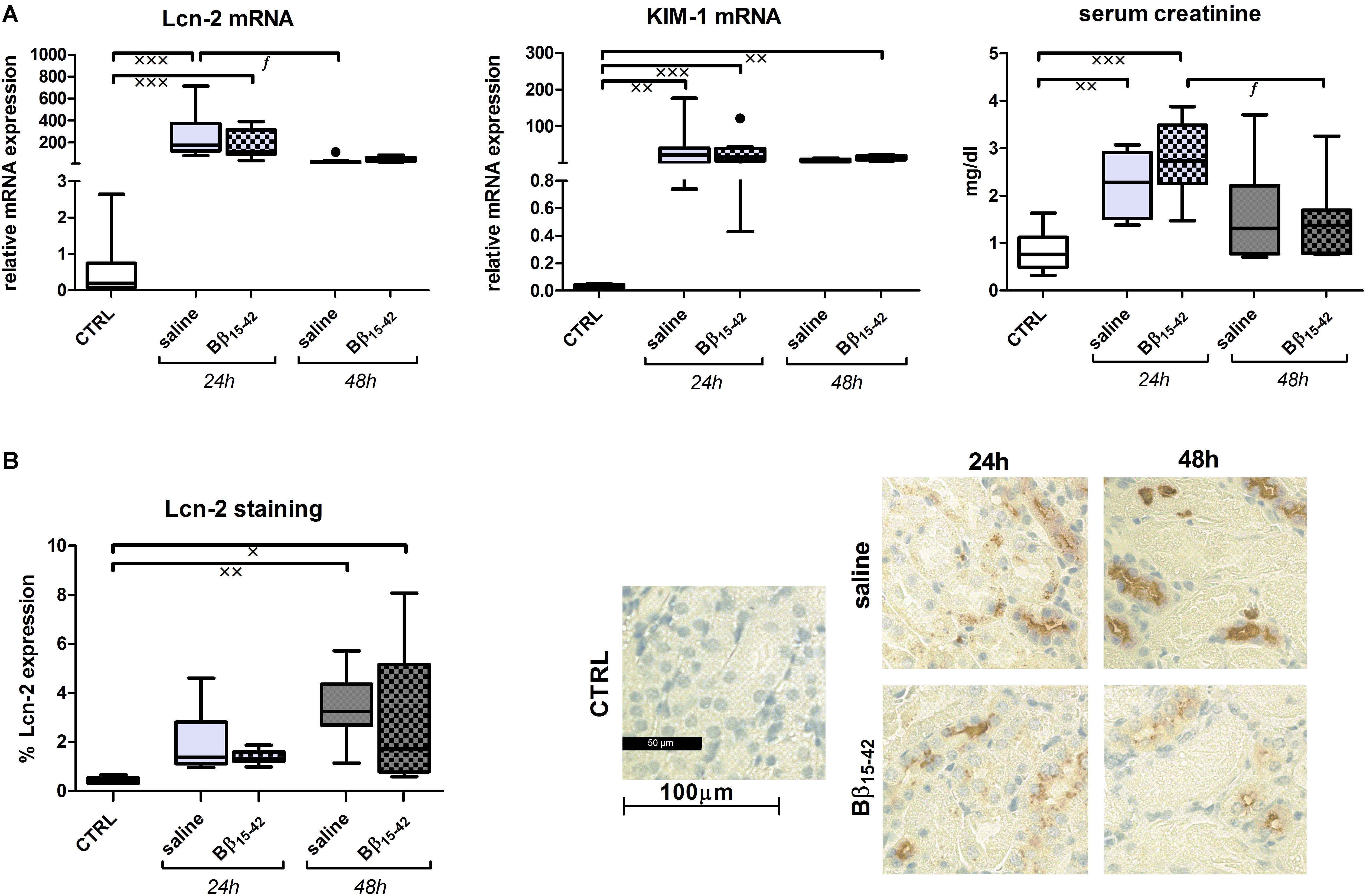
FIGURE 2. Evaluation of acute kidney injury (AKI). Tissue gene expression of the AKI markers Lcn-2, KIM-1, and serum creatinine (A). Values are expressed in relation to the housekeeping gene 18S for mRNA analyses and in mg/dl for creatinine measurements. All experiments were performed in duplicate. Representative micrographs of Lcn-2 stained kidney sections of untreated control, saline and Bβ15-42 treated mice at 24 and 48 h IR (B). Ten fields within the cortex and outer medulla in each group (n = 8) have been evaluated at 400-fold magnification. Quantification was performed using a script programmed in Matlab measuring proportional colored pixels in a defined relative area. Quantification was done by setting brown pixels into relationship to blue colored pixels. x significant difference to CTRL; f significant difference in the time course within Bβ15-42 treated or saline treated mice. x/f p < 0.05; xxp < 0.01; xxxp < 0.001.
Favored Angiogenesis Upon Treatment With Bβ15-42
Recovery from renal IR is associated with active angiogenesis, we thus investigated angiogenetic signaling by means of the following classical mediators of angiogenesis. Vascular endothelial cell growth factor (VEGF-A) mRNA and protein declined gradually over time upon IR. However, in Bβ15-42 treated mice, VEGF-A mRNA and protein was increased 48 h after IR compared to saline treated mice (Figure 3A). Similarly, Angiopoietin-1 mRNA displayed a higher expression in controls and decreased upon IR. Yet, Angiopoietin-1 mRNA and protein showed elevated expression at 48 h after IR in Bβ15-42 treated mice in comparison to saline treated mice (Figure 3B). In contrast, Angiopoietin-2 mRNA expression was lower in controls, and increased over time after IR. Again, after 48 h of IR, Angiopoietin-2 increased more firmly in Bβ15-42 mice compared to saline treated mice 48 h after IR. This is in contrast to the Angiopoietin-2 protein levels which decreased upon IR. Notably, Bβ15-42 counteracted the decrease in Angiopoietin-2 protein levels at 24 and 48 h of IR (Figure 3C). Tie-2 mRNA displayed similar levels in controls as well as saline and Bβ15-42 treated animals at 24 h after IR. However, a significant increase in Bβ15-42 treated mice after 48 h of IR versus control mice was seen. Western blot analyses of phosphorylated Tie-2 (pTie-2) put in ratio to total Tie-2 (tTie-2) did not display changes in the portion of phosphorylated protein (Figure 3D). When either phosphorylated Tie-2 or total Tie-2 protein expression was put in ratio to β-actin, we noticed a significant increase in pTie-2 protein expression in Bβ15-42 treated mice 48 h after IR (Figure 4A). Notably, immunohistochemical staining of pTie-2, and tTie-2 focusing on the renal cortex and outer medulla in control mice displayed a low expression of pTie-2 and tTie-2 mainly in the luminal surface of proximal tubules. Interestingly, pTie-2 and tTie-2 expression increased especially at 48 h of IR with accentuation rather in the collecting duct (Figure 4B).
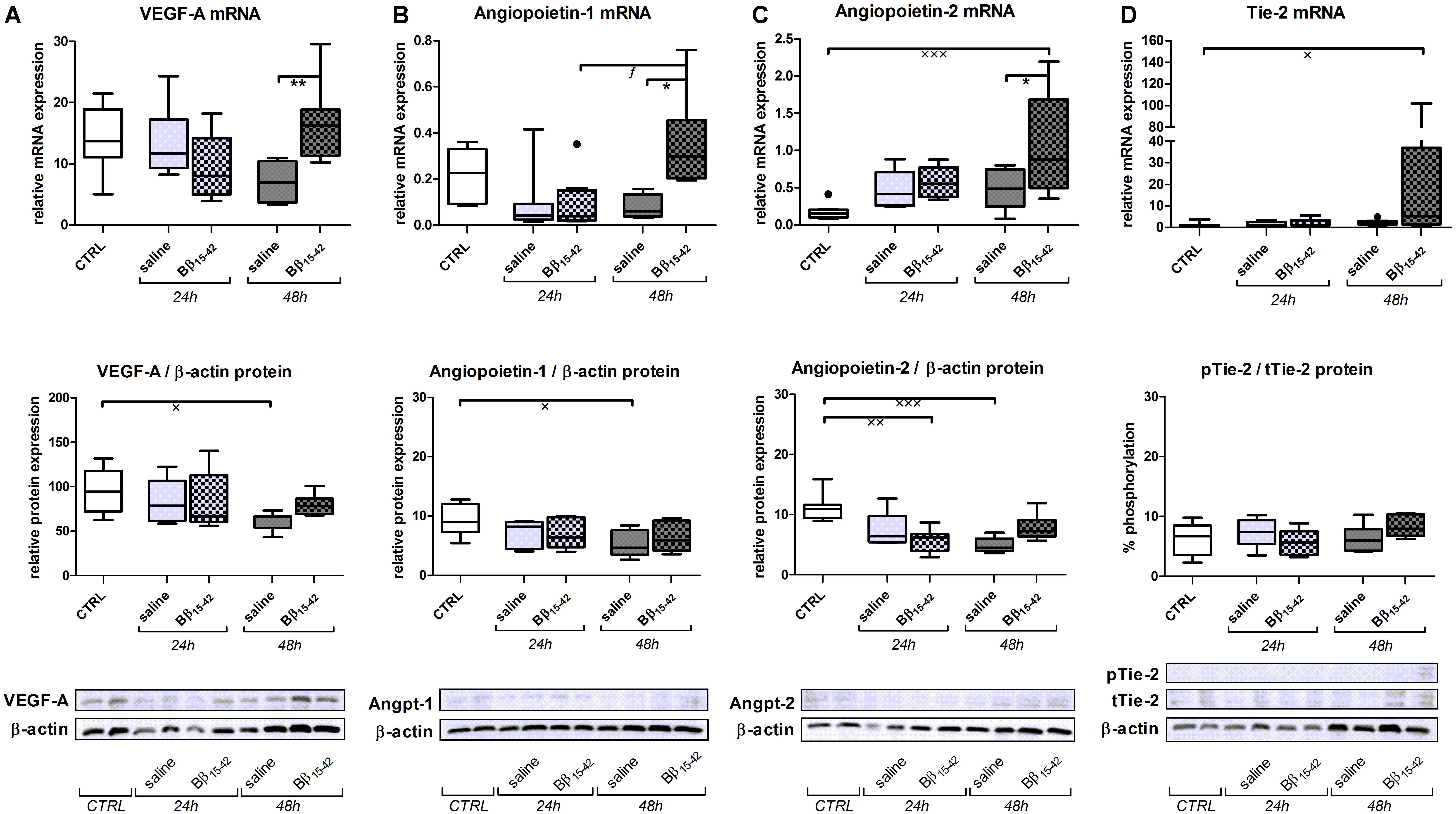
FIGURE 3. Bβ15-42 favors angiogenesis. In order to investigate angiogenetic signaling within IR injury, we performed RT-PCR analyses relative to 18S (upper graphs) and western blot analyses relative to β-actin (middle graphs and lower image) of relevant genes and proteins in kidney tissue homogenates (n = 8 per group) (A–D). ∗ Significant difference between Bβ15-42 and saline treated mice at one point in time; x significant difference to CTRL; f significant difference in the time course within Bβ15-42 treated or saline treated mice. ∗/x/f p < 0.05; ∗∗/xxp < 0.01; xxxp < 0.001.
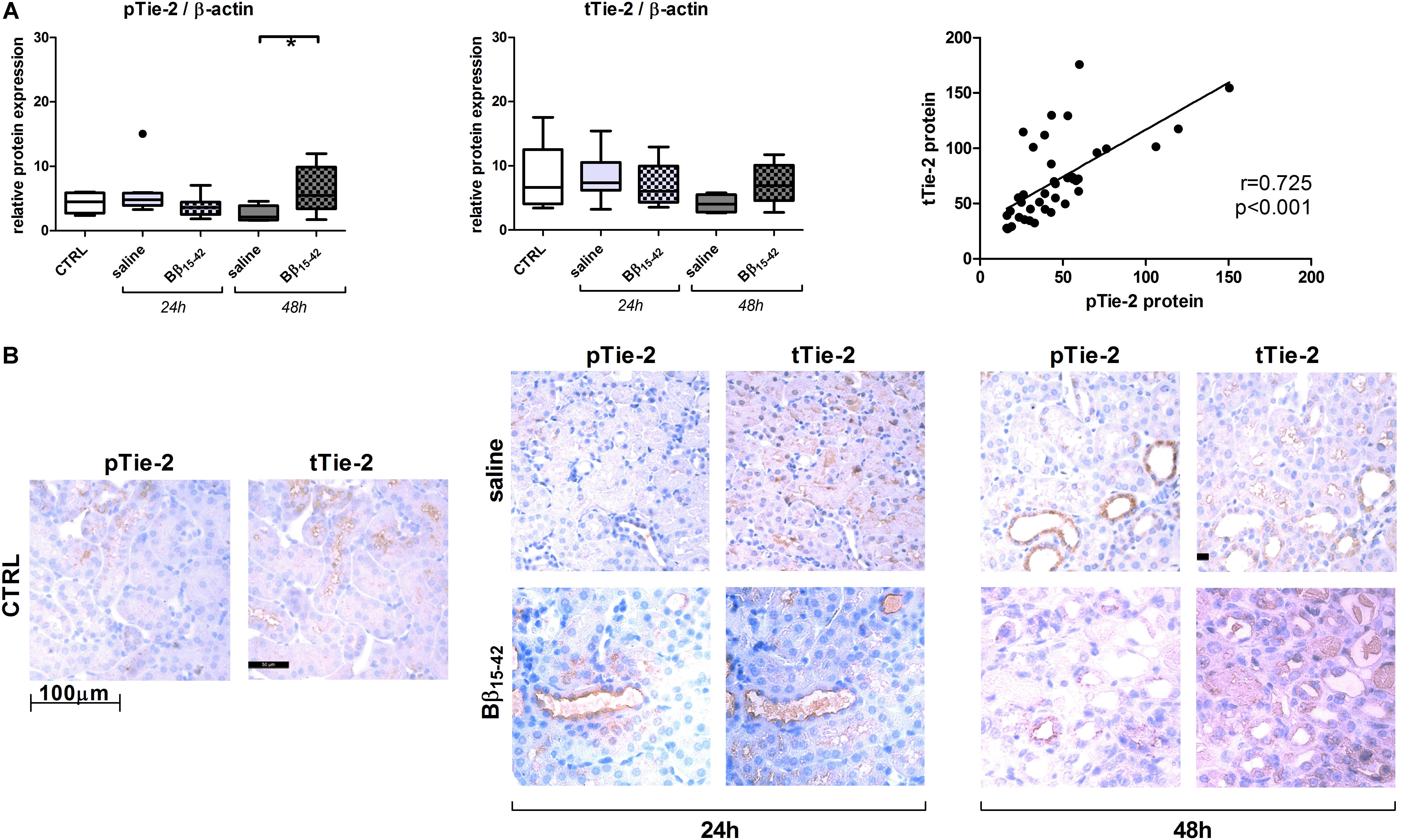
FIGURE 4. Protein expression and immunhistochemical staining of kidney sections on phosphorylated Tie-2 and total Tie-2. Protein expression of phosphorylated Tie-2 (pTie-2) and total Tie-2 (tTie-2) each relative to β-actin and their correlation (A). Representative juxtaposed micrographs of phosphorylated Tie-2 (pTie-2) and total Tie-2 (tTie-2) stained kidney sections within the cortex and outer medulla of control, Bβ15-42 and saline treated mice at 24 and 48 h IR (B). ∗p < 0.05.
Increased Tubular Regeneration Upon Bβ15-42 Treatment
Conclusively, we investigated biomarkers of tubular recovery after injury. Interestingly, vimentin exhibited markedly increased mRNA expression in Bβ15-42 treated mice after 48 h of IR compared to the saline control group (p < 0.05) (Figure 5A). Protein levels of vimentin were constantly very low and close to the detection limit throughout all samples with the exception of mice after 48 h of IR in Bβ15-42 treated mice (p < 0.05 versus saline). Since vimentin protein expression was rather week, we added a positive control which constituted of in vitro cultured mouse tubular epithelial cells known to co-express vimentin and cytokeratin as intermediate filament proteins (Baer et al., 1999) (Figure 5A). By contrast, the mRNA levels of the cytoprotective klotho decreased significantly in both Bβ15-42 and saline treated mice after 24 h of IR (p < 0.001). After 48 h of IR, klotho mRNA levels remained at a similar low level in saline treated mice but rose again significantly in Bβ15-42 treated mice (p < 0.05) (Figure 5B). Meanwhile, klotho protein expression remained relatively similar in CTRL and Bβ15-42 treated mice after 24 and 48 h of IR (Figure 5B). To further investigate regenerative and proliferative parameters, we performed gene expression analyses on stathmin and PCNA (Figure 6A). Both stathmin and PCNA mRNA exhibited markedly increased mRNA expression in Bβ15-42 treated mice after 48 h of IR compared to the saline control group (p < 0.001). Moreover, stathmin mRNA expression was significantly increased after 48 h of IR in Bβ15-42 compared to saline treated mice (p < 0.05), thereby indicating its pro-regenerative effect in kidney repair (Figure 6A). The effect of Bβ15-42 treatment on stathmin and PCNA was corroborated by immunofluorescence staining of stathmin (green) and PCNA (red) expression in renal tissue, focusing on the renal cortex and outer medulla (Figure 6B). Consistent with gene expression analyses, stathmin and PCNA expression showed that Bβ15-42 treated mice exhibited increased expression of stathmin and PCNA compared to the saline treated group after 48 h. Representative pictures out of four independent experiments are shown in Figure 6B.
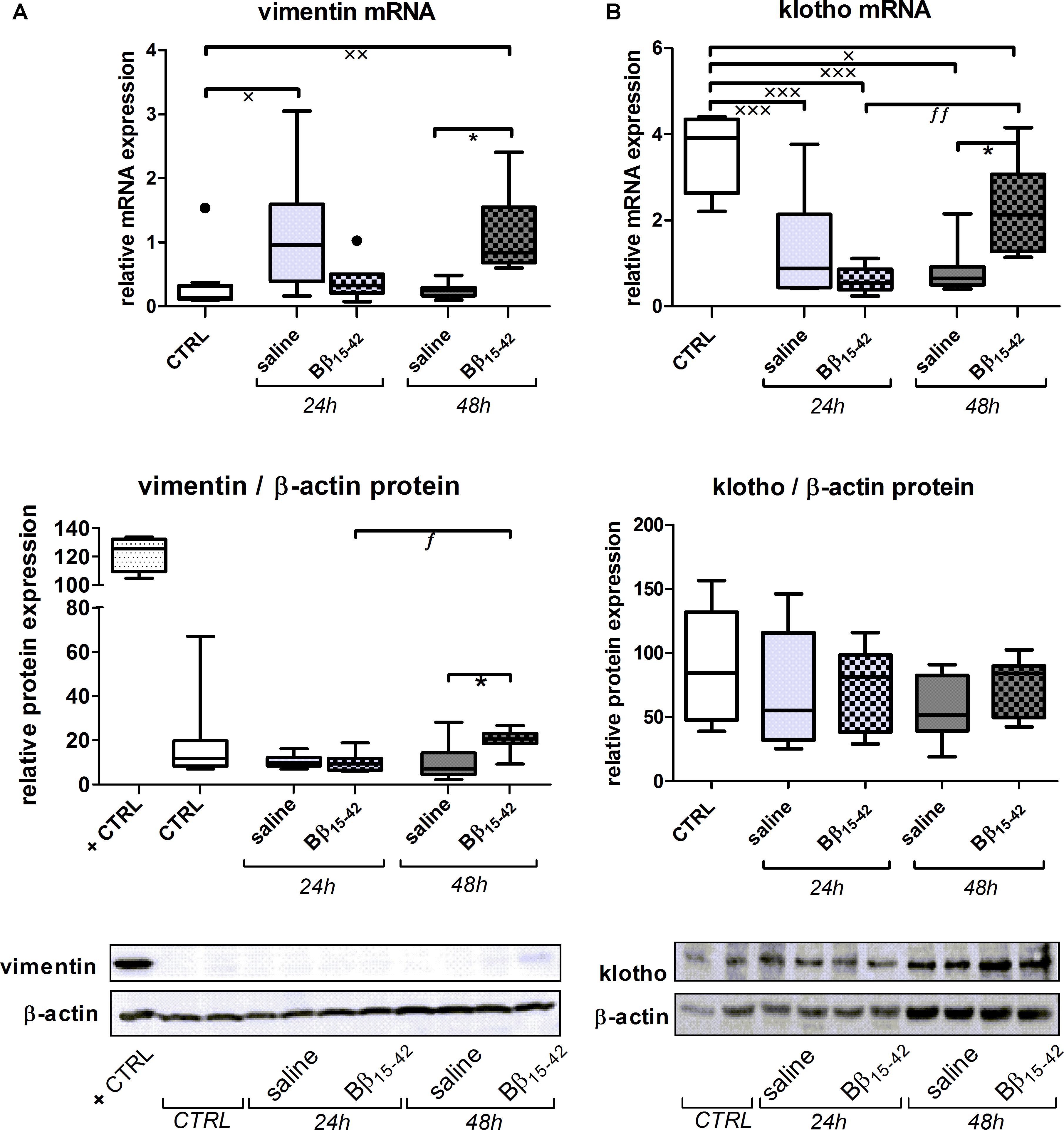
FIGURE 5. Increased tubular regeneration upon Bβ15-42 treatment. To provide an insight in tubular regeneration, proliferation and cell survival upon application of Bβ15-42 we performed RT-PCR analyses relative to 18S (upper graph) and Western blot analyses relative to β-actin (middle graph and lower image) of relevant genes and proteins in kidney tissue homogenates (n = 8 per group) (A,B). Corresponding correlation (Spearman’s test) (B). ∗Significant difference between Bβ15-42 and saline treated mice at one point in time; x significant difference to CTRL; f significant difference in the time course within Bβ15-42 treated or saline treated mice. ∗/x/f p < 0.05; xx/ff p < 0.01; xxxp < 0.001.
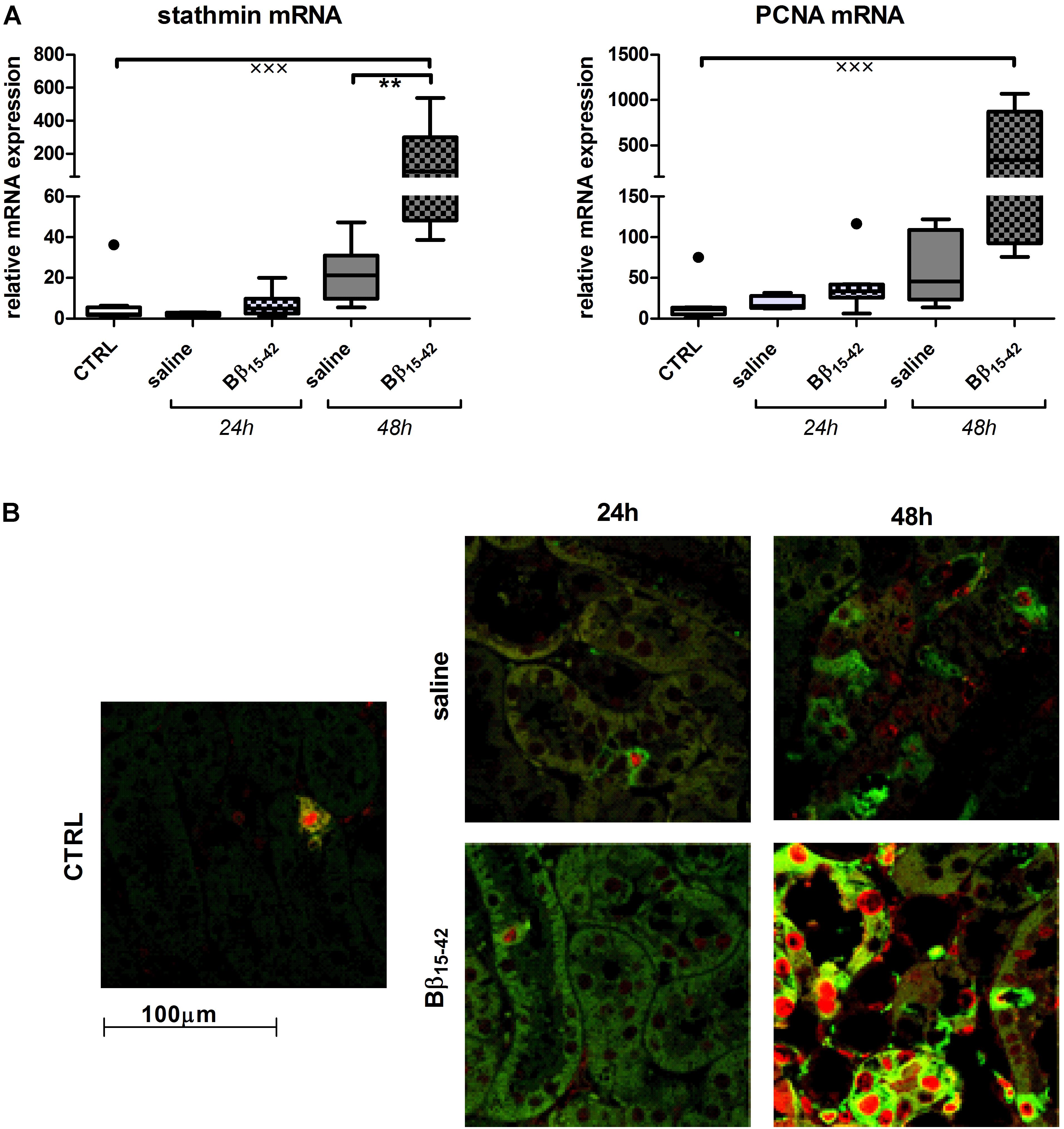
FIGURE 6. Increased tubular regeneration upon Bβ15-42 treatment. The effect of Bβ15-42 treatment on stathmin and proliferation cellular nuclear antigen (PCNA) was assessed by RT-PCR analyses relative to 18S in kidney tissue homogenates (n = 8 per group) (A) and immunofluorescence staining of stathmin (green) and PCNA (red) (400-fold magnification) in renal tissue (n = 4 per group) (B). ∗Significant difference between Bβ15-42 and saline treated mice at one point in time; x significant difference to CTRL. ∗∗p < 0.01; xxxp < 0.001.
No Immediate Beneficial Effect of Bβ15-42 Treatment on PTCs
In order to examine a potential immediate effect of Bβ15-42 on PTCs we mimicked in vivo conditions in in vitro anoxia experiments using primary PTCs from the same mouse strain (C57BL/6). In these experiments, anoxia significantly decreased cell viability (measured by XTT) in pure oil treated cells compared to the control groups (24 h reoxygenation p < 0.001, 48 h reoxygenation p < 0.05) and moderately decreased cell viability in cells immersed in medium and oil layer atop (Supplementary Figure S1). For morphological analyses we performed Hoechst and phalloidin staining. In line with XTT results, we observed rarefaction of PTCs, implying preceding cell death. Furthermore, stress fiber formation decreased in mineral oil treated PTCs and was hardly detectable in pure oil treated PTCs. In contrast, actin aggregation tended to change from focal to more ubiquitous distribution upon increasing damage. However, no immediate benefit of Bβ15-42 on PTCs was noted (Supplementary Figure S1).
Discussion
Vascular injury, in particular endothelial cell injury, participates in extent and maintenance of AKI. Over-expression of ICAM-1 and P-selectin by vascular endothelium of the ischemic kidney has been demonstrated to play a major patho-physiological role in the development of renal dysfunction (Kelly et al., 1994; Singbartl et al., 2001). IR injury also induces the generation of inflammatory mediators like cytokines and chemokines by both tubular and endothelial cells (Supavekin et al., 2003; Bonventre and Zuk, 2004). Accordingly, we observed a significant minor increase of ICAM-1 and P-selectin in Bβ15-42 treated mice, even though no effect on classical kidney injury markers could be sighted. Yet, these findings indicate that the applied treatment with Bβ15-42 effectively attenuated the extend of endothelial activation in our mouse model, which is in accordance with previous findings (Sorensen et al., 2011).
Given that fibrinogen β chain transcript levels were described to be one of the highest up regulated after kidney injury (Krishnamoorthy et al., 2011), modulation of endothelial integrity by means of Bβ15-42 application represents an attractive therapeutic approach. This holds true not only for early IR injury (Urbschat et al., 2014), but also for the post-acute period, which is characterized by the facilitation of endothelial and tubular repair mechanisms. In this context activation of angiogenesis plays a major role. Surprisingly, two independent studies demonstrated that neither mRNA nor protein expression of VEGF-A, the classic angiogenic factor, were increased in the post-ischemic rodent kidney (Kanellis et al., 2000; Basile et al., 2008), even though one would expect that hypoxia occurring during IR may stimulate the expression of pro-angiogenic molecules (Neufeld et al., 1999). This is in line with our observation, that both VEGF-A mRNA and protein expression declined over time in IR. However, its expression increased markedly merely in Bβ15-42 treated mice after 48 h of IR.
Aside from VEGF, Angiopoietins represent another family of endothelial specific growth factors (Satchell and Mathieson, 2003). Angiopoietin-1 is a secreted growth factor which binds to and activates the Tie-2 receptor tyrosine kinase. The latter regulates amongst others angiogenesis, endothelial cell survival, proliferation, and reorganization of the actin cytoskeleton. Angiopoietin-2, a further ligand of the Tie-2 receptor (Davis et al., 1996; Maisonpierre et al., 1997) was recently identified as partial agonist and antagonist for Tie-2 (Kim et al., 2000; Yuan et al., 2009). In our study not only VEGF-A but also Angiopoietin-1 and Angiopoietin-2 mRNA was significantly elevated in Bβ15-42 treated mice after 48 h with only a tendency for the corresponding protein expression, which might be due to temporally delayed protein expression. Noticeable discrepancy in Angiopoietin-2 mRNA and protein expression might be due to not immediate protein translation considering its function as partial agonist and antagonist for Tie-2 (Kim et al., 2000; Yuan et al., 2009). Yet, this indicates an earlier onset of vascular repair mechanisms which is particularly relevant owing to the fact that Angiopoietin-2 is only expressed at sites of vascular remodeling (Maisonpierre et al., 1997) and that application of synthetic Tie-2 agonistic peptide (Rubig et al., 2016) as well as VEGF (Kang et al., 2001) protects renal vascular function in experimental AKI.
Indeed, renal peritubular capillaries, which encounter endothelial swelling, capillary disintegration, and microvascular rarefaction during IR injury (Sutton et al., 2002; Molitoris and Sutton, 2004; Basile, 2007; Kwon et al., 2008) are morphologically and functionally closely connected with adjacent renal tubules. Yet, in in vitro co-cultures, a cross talk between primary human renal tubular and endothelial cells (Tasnim and Zink, 2012), and moreover, regulation of tubular recovery processes after injury by surrounding peritubular capillaries cells (Miya et al., 2011) have been discovered.
Above findings underline our observations as not only VEGF-A and angiopoietins are increased in Bβ15-42 treated mice after 48 h of IR, but also the intermediate filament protein vimentin and the cytoprotective protein klotho. Vimentin is typically detected in glomeruli, vessels, and interstitial cells, but not in tubular epithelial cells in the mature kidney (Holthofer et al., 1984; Witzgall et al., 1994). However, it becomes detectable in regenerating, mitotically active PTCs after IR (Nouwen et al., 1994) most prominently in the S3 segment 2 days post-ischemia (Witzgall et al., 1994). In this regard, it is also considered as a marker of epithelial dedifferentiation (Bonventre, 2003). Given that the fibrinopeptid Bβ15-42 binds to VE-cadherin (Zacharowski et al., 2006) we previously detected a significant alleviated loss of VE-cadherin in Bβ15-42 treated mice in very early IR injury (1 and 3 h after IR) (Urbschat et al., 2014). In this study however, no alteration in VE-cadherin expression in Bβ15-42 treated mice was noticed. Taking into account the short half-time of Bβ15-42 with 12–17 min in dogs and humans (Roesner et al., 2007), stabilization of VE-cadherin after single-shot application of Bβ15-42 might not be viewable in those later phases of IR. Nevertheless, VE-cadherin is linked not only by β- and α-catenin to cortical actin but also by γ-catenin and desmoplakin to the intermediate filament protein vimentin (Valiron et al., 1996; Kowalczyk et al., 1998). This linkage might irrespectively of our measured VE-cadherin expression influence the expression of vimentin to a certain extent, as vimentin expression rose in Bβ15-42 treated mice after 48 h of IR.
Along the same line, klotho is considered as cyto-protective mediator that is predominantly expressed in the kidney (Kuro-o et al., 1997). Immunostaining revealed constitutive klotho expression in the distal, cortical, and medullary collecting duct cells (Mitobe et al., 2005), whereas klotho mRNA and protein is also present in PTCs, although to a lesser extend (Hu et al., 2010a). Expression of both klotho mRNA and protein is known to significantly decrease during IR injury in the rat kidney, but rises gradually to nearly the control level during recovery (Sugiura et al., 2005; Hu et al., 2010b), suggesting a therapeutic potential in managing the ischemic challenge, too (Sugiura et al., 2005; Shi et al., 2016). As we detected a significant retrieval of klotho mRNA in Bβ15-42 treated mice after 48 h of IR, approaching constitutive expression. Klotho protein expression, still did only display a tendency to increased expression in Bβ15-42 treated mice 48 h after IR, which is presumably due to delayed protein translation.
Alike, immunofluorescence staining revealed a significant number of actively proliferating cells (stathmin and PCNA positive) within the renal cortex and outer medulla particularly 48 h after administration of Bβ15-42 compared to saline treated mice, which goes along with the respective mRNA expression in tissue homogenates. Increased detection of PCNA in nuclei of epithelial cells of the cortical proximal tubule has previously been described after renal IR (Witzgall et al., 1994). The maximum levels of expression were seen 48 h after IR mainly in the S3 zone, whereas in controls the number of labeled nuclei was considerably lower. In a further study immunofluorescent labeling of stathmin and PCNA demonstrated that the expression of stathmin increased markedly after 24 h and even more at 48 h of reperfusion (Zahedi et al., 2004; Jung et al., 2012), which is equally in accordance to our findings. As Bβ15-42 treated mice displayed higher expression of both, stathmin and PCNA 48 h after IR, this might be indicative for enhanced cell proliferation and regeneration within Bβ15-42 treated injured kidneys.
Still, because tubules and capillaries are simultaneously injured during IR injury, it is difficult to distinguish the primary initiating factors that drive endothelial-tubular crosstalk in these contexts. As we analyzed kidney tissue homogenates in gene and protein analyses both endothelial and epithelial cells were considered together, which certainly limits the deduction of conclusions.
Finally, as PTCs are known to be mainly affected by IR injury, in vitro experiments using primary murine PTCs from the same mouse strain (C57BL/6) were performed to mimic in vivo conditions. In these experiments, anoxic injury followed by reoxygenation significantly decreased cell viability and morphologically led to rarefaction of PTCs and reduced stress fiber formation. However, no direct effect of Bβ15-42 on PTCs could be observed. This observation reinforces the assumption, that Bβ15-42 promotes its protective effect through preservation of the endothelial integrity.
In the present study, angiogenetic factors as well as markers of tubular regeneration increased 48 h after IR injury upon treatment with Bβ15-42 suggesting a linkage between renal endothelial and tubular epithelial cells during cellular repair, as previously described in in vitro co-culture-assays. This cross-talk between injured tubular and endothelial cells is of particular interest and it is conceivable that these may either favor or prevent tubular recovery. Notably, the fibrinopeptid Bβ15-42 binds to VE-Cadherin, which in turn is relevant in preserving the endothelial barrier function.
As a physiologically occurring fibrinopeptide, Bβ15-42 represents a promising anti-inflammatory therapeutic agent channeling regeneration of the endothelium as well as tubules from ischemic injury. However, given the short half-time of Bβ15-42, during translation into efficient treatment in humans, a repeated application should be considered. In this regard, further investigations with focus on effected endothelial and tubular regeneration are warranted.
Author Contributions
KZ and AU conceived and financed the study. DF, CS, CM, BS, and AU performed the analyses. DF, CS, PB, MJ, CM, RH, TM, KZ, and AU analyzed and interpreted the data and wrote the manuscript.
Funding
The work emanated in part from a junior researchers grant by the Goethe University Frankfurt (Fokus Programm, Förderlinie A) to AU and from a grant provided by the DFG (SFB 834-B4) to KZ.
Conflict of Interest Statement
The authors declare that the research was conducted in the absence of any commercial or financial relationships that could be construed as a potential conflict of interest.
Acknowledgments
We especially thank Lene Niemann Nejsum and Christina Lundgaard Ernstsen for their technical support in immunofluorescence analyses of PTCs.
Supplementary Material
The Supplementary Material for this article can be found online at: https://www.frontiersin.org/articles/10.3389/fphar.2018.00369/full#supplementary-material
FIGURE S1 | No immediate effect of Bβ15-42 on primary murine proximal tubular cells. Exemplary image of mouse PTCs in passage 2 (A). Viability was measured via XTT after 1 h of anoxia and 24 and 48 h of reoxygenation with or without addition of 6 μM Bβ15-42 to the medium at the time of reoxygenation (n = 3) (B). Analysis of actin fibers in primary mouse PTCs at the same conditions (n = 1) (C). x/f p < 0.05; xxp < 0.01; xxx/f p < 0.001.
FIGURE S2 | Original western blot images Figure 1.
FIGURE S3 | Original western blot images Figure 3.
FIGURE S4 | Original western blot images Figure 5.
References
Atar, D., Petzelbauer, P., Schwitter, J., Huber, K., Rensing, B., Kasprzak, J. D., et al. (2009). Effect of intravenous FX06 as an adjunct to primary percutaneous coronary intervention for acute ST-segment elevation myocardial infarction results of the F.I.R.E. (Efficacy of FX06 in the Prevention of Myocardial Reperfusion Injury) trial. J. Am. Coll. Cardiol. 53, 720–729. doi: 10.1016/j.jacc.2008.12.017
Bach, T. L., Barsigian, C., Yaen, C. H., and Martinez, J. (1998). Endothelial cell VE-cadherin functions as a receptor for the beta15-42 sequence of fibrin. J. Biol. Chem. 273, 30719–30728. doi: 10.1074/jbc.273.46.30719
Baer, P. C., Nockher, W. A., Haase, W., and Scherberich, J. E. (1997). Isolation of proximal and distal tubule cells from human kidney by immunomagnetic separation. Technical note. Kidney Int. 52, 1321–1331. doi: 10.1038/ki.1997.457
Baer, P. C., Tunn, U. W., Nunez, G., Scherberich, J. E., and Geiger, H. (1999). Transdifferentiation of distal but not proximal tubular epithelial cells from human kidney in culture. Exp. Nephrol. 7, 306–313. doi: 10.1159/000020618
Basile, D. P. (2007). The endothelial cell in ischemic acute kidney injury: implications for acute and chronic function. Kidney Int. 72, 151–156. doi: 10.1038/sj.ki.5002312
Basile, D. P., Fredrich, K., Chelladurai, B., Leonard, E. C., and Parrish, A. R. (2008). Renal ischemia reperfusion inhibits VEGF expression and induces ADAMTS-1, a novel VEGF inhibitor. Am. J. Physiol. Renal Physiol. 294, F928–F936. doi: 10.1152/ajprenal.00596.2007
Bonventre, J. V. (2003). Dedifferentiation and proliferation of surviving epithelial cells in acute renal failure. J. Am. Soc. Nephrol. 14(Suppl. 1), S55–S61. doi: 10.1097/01.ASN.0000067652.51441.21
Bonventre, J. V. (2010). Pathophysiology of AKI: injury and normal and abnormal repair. Contrib. Nephrol. 165, 9–17. doi: 10.1159/000313738
Bonventre, J. V., and Zuk, A. (2004). Ischemic acute renal failure: an inflammatory disease? Kidney Int. 66, 480–485. doi: 10.1111/j.1523-1755.2004.761_2.x
Brodsky, S. V., Yamamoto, T., Tada, T., Kim, B., Chen, J., Kajiya, F., et al. (2002). Endothelial dysfunction in ischemic acute renal failure: rescue by transplanted endothelial cells. Am. J. Physiol. Renal Physiol. 282, F1140–F1149. doi: 10.1152/ajprenal.00329.2001
Broman, M. T., Mehta, D., and Malik, A. B. (2007). Cdc42 regulates the restoration of endothelial adherens junctions and permeability. Trends Cardiovasc. Med. 17, 151–156. doi: 10.1016/j.tcm.2007.03.004
Davis, S., Aldrich, T. H., Jones, P. F., Acheson, A., Compton, D. L., Jain, V., et al. (1996). Isolation of angiopoietin-1, a ligand for the TIE2 receptor, by secretion-trap expression cloning. Cell 87, 1161–1169. doi: 10.1016/S0092-8674(00)81812-7
Dejana, E., Spagnuolo, R., and Bazzoni, G. (2001). Interendothelial junctions and their role in the control of angiogenesis, vascular permeability and leukocyte transmigration. Thromb. Haemost. 86, 308–315. doi: 10.1055/s-0037-1616228
Flores, J., Dibona, D. R., Beck, C. H., and Leaf, A. (1972). The role of cell swelling in ischemic renal damage and the protective effect of hypertonic solute. J. Clin. Invest. 51, 118–126. doi: 10.1172/JCI106781
Gorlatov, S., and Medved, L. (2002). Interaction of fibrin(ogen) with the endothelial cell receptor VE-cadherin: mapping of the receptor-binding site in the NH2-terminal portions of the fibrin beta chains. Biochemistry 41, 4107–4116. doi: 10.1021/bi0160314
Holthofer, H., Miettinen, A., Lehto, V. P., Lehtonen, E., and Virtanen, I. (1984). Expression of vimentin and cytokeratin types of intermediate filament proteins in developing and adult human kidneys. Lab. Invest. 50, 552–559.
Hu, M. C., Shi, M., Zhang, J., Pastor, J., Nakatani, T., Lanske, B., et al. (2010a). Klotho: a novel phosphaturic substance acting as an autocrine enzyme in the renal proximal tubule. FASEB J. 24, 3438–3450. doi: 10.1096/fj.10-154765
Hu, M. C., Shi, M., Zhang, J., Quinones, H., Kuro-O, M., and Moe, O. W. (2010b). Klotho deficiency is an early biomarker of renal ischemia-reperfusion injury and its replacement is protective. Kidney Int. 78, 1240–1251. doi: 10.1038/ki.2010.328
Jung, M., Sola, A., Hughes, J., Kluth, D. C., Vinuesa, E., Vinas, J. L., et al. (2012). Infusion of IL-10-expressing cells protects against renal ischemia through induction of lipocalin-2. Kidney Int. 81, 969–982. doi: 10.1038/ki.2011.446
Kanellis, J., Mudge, S. J., Fraser, S., Katerelos, M., and Power, D. A. (2000). Redistribution of cytoplasmic VEGF to the basolateral aspect of renal tubular cells in ischemia-reperfusion injury. Kidney Int. 57, 2445–2456. doi: 10.1046/j.1523-1755.2000.00103.x
Kang, D. H., Hughes, J., Mazzali, M., Schreiner, G. F., and Johnson, R. J. (2001). Impaired angiogenesis in the remnant kidney model: II. Vascular endothelial growth factor administration reduces renal fibrosis and stabilizes renal function. J. Am. Soc. Nephrol. 12, 1448–1457.
Kelly, K. J., Williams, W. W. Jr., Colvin, R. B., and Bonventre, J. V. (1994). Antibody to intercellular adhesion molecule 1 protects the kidney against ischemic injury. Proc. Natl. Acad. Sci. U.S.A. 91, 812–816. doi: 10.1073/pnas.91.2.812
Kim, I., Kim, J. H., Moon, S. O., Kwak, H. J., Kim, N. G., and Koh, G. Y. (2000). Angiopoietin-2 at high concentration can enhance endothelial cell survival through the phosphatidylinositol 3’-kinase/Akt signal transduction pathway. Oncogene 19, 4549–4552. doi: 10.1038/sj.onc.1203800
Kowalczyk, A. P., Navarro, P., Dejana, E., Bornslaeger, E. A., Green, K. J., Kopp, D. S., et al. (1998). VE-cadherin and desmoplakin are assembled into dermal microvascular endothelial intercellular junctions: a pivotal role for plakoglobin in the recruitment of desmoplakin to intercellular junctions. J. Cell Sci. 111(Pt 20), 3045–3057.
Krishnamoorthy, A., Ajay, A. K., Hoffmann, D., Kim, T. M., Ramirez, V., Campanholle, G., et al. (2011). Fibrinogen beta-derived Bbeta(15-42) peptide protects against kidney ischemia/reperfusion injury. Blood 118, 1934–1942. doi: 10.1182/blood-2011-02-338061
Kuro-o, M., Matsumura, Y., Aizawa, H., Kawaguchi, H., Suga, T., Utsugi, T., et al. (1997). Mutation of the mouse klotho gene leads to a syndrome resembling ageing. Nature 390, 45–51. doi: 10.1038/36285
Kwon, O., Hong, S. M., Sutton, T. A., and Temm, C. J. (2008). Preservation of peritubular capillary endothelial integrity and increasing pericytes may be critical to recovery from postischemic acute kidney injury. Am. J. Physiol. Renal Physiol. 295, F351–F359. doi: 10.1152/ajprenal.90276.2008
Li, J., Hong, Z., Liu, H., Zhou, J., Cui, L., Yuan, S., et al. (2016). Hydrogen-rich saline promotes the recovery of renal function after ischemia/reperfusion injury in rats via anti-apoptosis and anti-inflammation. Front. Pharmacol. 7:106. doi: 10.3389/fphar.2016.00106
Livak, K. J., and Schmittgen, T. D. (2001). Analysis of relative gene expression data using real-time quantitative PCR and the 2(-Delta Delta C(T)) method. Methods 25, 402–408. doi: 10.1006/meth.2001.1262
Maisonpierre, P. C., Suri, C., Jones, P. F., Bartunkova, S., Wiegand, S. J., Radziejewski, C., et al. (1997). Angiopoietin-2, a natural antagonist for Tie2 that disrupts in vivo angiogenesis. Science 277, 55–60. doi: 10.1126/science.277.5322.55
Meldrum, K. K., Meldrum, D. R., Hile, K. L., Burnett, A. L., and Harken, A. H. (2001). A novel model of ischemia in renal tubular cells which closely parallels in vivo injury. J. Surg. Res. 99, 288–293. doi: 10.1006/jsre.2001.6201
Mitobe, M., Yoshida, T., Sugiura, H., Shirota, S., Tsuchiya, K., and Nihei, H. (2005). Oxidative stress decreases klotho expression in a mouse kidney cell line. Nephron Exp. Nephrol. 101, e67–e74. doi: 10.1159/000086500
Miya, M., Maeshima, A., Mishima, K., Sakurai, N., Ikeuchi, H., Kuroiwa, T., et al. (2011). Enhancement of in vitro human tubulogenesis by endothelial cell-derived factors: implications for in vivo tubular regeneration after injury. Am. J. Physiol. Renal Physiol. 301, F387–F395. doi: 10.1152/ajprenal.00619.2010
Molitoris, B. A., and Sutton, T. A. (2004). Endothelial injury and dysfunction: role in the extension phase of acute renal failure. Kidney Int. 66, 496–499. doi: 10.1111/j.1523-1755.2004.761_5.x
Neufeld, G., Cohen, T., Gengrinovitch, S., and Poltorak, Z. (1999). Vascular endothelial growth factor (VEGF) and its receptors. FASEB J. 13, 9–22. doi: 10.1096/fasebj.13.1.9
Nouwen, E. J., Verstrepen, W. A., Buyssens, N., Zhu, M. Q., and De Broe, M. E. (1994). Hyperplasia, hypertrophy, and phenotypic alterations in the distal nephron after acute proximal tubular injury in the rat. Lab. Invest. 70, 479–493.
Petzelbauer, P., Zacharowski, P. A., Miyazaki, Y., Friedl, P., Wickenhauser, G., Castellino, F. J., et al. (2005). The fibrin-derived peptide Bbeta15-42 protects the myocardium against ischemia-reperfusion injury. Nat. Med. 11, 298–304. doi: 10.1038/nm1198
Roesner, J. P., Petzelbauer, P., Koch, A., Mersmann, J., Zacharowski, P. A., Boehm, O., et al. (2007). The fibrin-derived peptide Bbeta15-42 is cardioprotective in a pig model of myocardial ischemia-reperfusion injury. Crit. Care Med. 35, 1730–1735. doi: 10.1097/01.CCM.0000269035.30231.76
Rubig, E., Stypmann, J., Van Slyke, P., Dumont, D. J., Spieker, T., Buscher, K., et al. 2016. The synthetic Tie2 agonist peptide vasculotide protects renal vascular barrier function in experimental acute kidney injury. Sci. Rep. 6:22111. doi: 10.1038/srep22111
Satchell, S. C., and Mathieson, P. W. (2003). Angiopoietins: microvascular modulators with potential roles in glomerular pathophysiology. J. Nephrol. 16, 168–178.
Shi, M., Flores, B., Gillings, N., Bian, A., Cho, H. J., Yan, S., et al. (2016). αKlotho mitigates progression of AKI to CKD through activation of autophagy. J. Am. Soc. Nephrol. 27, 2331–2345. doi: 10.1681/ASN.2015060613
Singbartl, K., Forlow, S. B., and Ley, K. (2001). Platelet, but not endothelial, P-selectin is critical for neutrophil-mediated acute postischemic renal failure. FASEB J. 15, 2337–2344. doi: 10.1096/fj.01-0199com
Sorensen, I., Rong, S., Susnik, N., Gueler, F., Shushakova, N., Albrecht, M., et al. (2011). Bbeta(15-42) attenuates the effect of ischemia-reperfusion injury in renal transplantation. J. Am. Soc. Nephrol. 22, 1887–1896. doi: 10.1681/ASN.2011010031
Sugiura, H., Yoshida, T., Tsuchiya, K., Mitobe, M., Nishimura, S., Shirota, S., et al. (2005). Klotho reduces apoptosis in experimental ischaemic acute renal failure. Nephrol. Dial. Transplant. 20, 2636–2645. doi: 10.1093/ndt/gfi165
Supavekin, S., Zhang, W., Kucherlapati, R., Kaskel, F. J., Moore, L. C., and Devarajan, P. (2003). Differential gene expression following early renal ischemia/reperfusion. Kidney Int. 63, 1714–1724. doi: 10.1046/j.1523-1755.2003.00928.x
Sutton, T. A., Fisher, C. J., and Molitoris, B. A. (2002). Microvascular endothelial injury and dysfunction during ischemic acute renal failure. Kidney Int. 62, 1539–1549. doi: 10.1046/j.1523-1755.2002.00631.x
Tasnim, F., and Zink, D. (2012). Cross talk between primary human renal tubular cells and endothelial cells in cocultures. Am. J. Physiol. Renal Physiol. 302, F1055–F1062. doi: 10.1152/ajprenal.00621.2011
Urbschat, A., Rupprecht, K., Zacharowski, K., Obermuller, N., Scheller, B., Holfeld, J., et al. (2015). Combined peri-ischemic administration of Bbeta15-42 in treating ischemia reperfusion injury of the mouse kidney. Microvasc. Res. 101, 48–54. doi: 10.1016/j.mvr.2015.06.005
Urbschat, A., Zacharowski, K., Obermuller, N., Rupprecht, K., Penzkofer, D., Jennewein, C., et al. (2014). The small fibrinopeptide Bbeta15-42 as renoprotective agent preserving the endothelial and vascular integrity in early ischemia reperfusion injury in the mouse kidney. PLoS One 9:e84432. doi: 10.1371/journal.pone.0084432
Valiron, O., Chevrier, V., Usson, Y., Breviario, F., Job, D., and Dejana, E. (1996). Desmoplakin expression and organization at human umbilical vein endothelial cell-to-cell junctions. J. Cell Sci. 109(Pt 8), 2141–2149.
Vestweber, D. (2008). VE-cadherin: the major endothelial adhesion molecule controlling cellular junctions and blood vessel formation. Arterioscler. Thromb. Vasc. Biol. 28, 223–232. doi: 10.1161/ATVBAHA.107.158014
Witzgall, R., Brown, D., Schwarz, C., and Bonventre, J. V. (1994). Localization of proliferating cell nuclear antigen, vimentin, c-Fos, and clusterin in the postischemic kidney. Evidence for a heterogenous genetic response among nephron segments, and a large pool of mitotically active and dedifferentiated cells. J. Clin. Invest. 93, 2175–2188. doi: 10.1172/JCI117214
Wolf, T., Kann, G., Becker, S., Stephan, C., Brodt, H. R., De Leuw, P., et al. (2015). Severe Ebola virus disease with vascular leakage and multiorgan failure: treatment of a patient in intensive care. Lancet 385, 1428–1435. doi: 10.1016/S0140-6736(14)62384-9
Yuan, H. T., Khankin, E. V., Karumanchi, S. A., and Parikh, S. M. (2009). Angiopoietin 2 is a partial agonist/antagonist of Tie2 signaling in the endothelium. Mol. Cell Biol. 29, 2011–2022. doi: 10.1128/MCB.01472-08
Zacharowski, K., Zacharowski, P., Reingruber, S., and Petzelbauer, P. (2006). Fibrin(ogen) and its fragments in the pathophysiology and treatment of myocardial infarction. J. Mol. Med. 84, 469–477. doi: 10.1007/s00109-006-0051-7
Zacharowski, K., Zacharowski, P. A., Friedl, P., Mastan, P., Koch, A., Boehm, O., et al. (2007). The effects of the fibrin-derived peptide Bbeta(15-42) in acute and chronic rodent models of myocardial ischemia-reperfusion. Shock 27, 631–637. doi: 10.1097/SHK.0b013e31802fa038
Keywords: FX06, renal ischemia reperfusion injury, endothelial activation, tubular regeneration, angiogenesis, primary mouse proximal tubular cells
Citation: Fischer D, Seifen C, Baer P, Jung M, Mertens C, Scheller B, Zacharowski K, Hofmann R, Maier TJ and Urbschat A (2018) The Fibrin Cleavage Product Bβ15–42 Channels Endothelial and Tubular Regeneration in the Post-acute Course During Murine Renal Ischemia Reperfusion Injury. Front. Pharmacol. 9:369. doi: 10.3389/fphar.2018.00369
Received: 12 January 2018; Accepted: 29 March 2018;
Published: 27 April 2018.
Edited by:
Orina Belton, University College Dublin, IrelandReviewed by:
Vishal Diwan, The University of Queensland, AustraliaSergey V. Ryzhov, Maine Medical Center, United States
Copyright © 2018 Fischer, Seifen, Baer, Jung, Mertens, Scheller, Zacharowski, Hofmann, Maier and Urbschat. This is an open-access article distributed under the terms of the Creative Commons Attribution License (CC BY). The use, distribution or reproduction in other forums is permitted, provided the original author(s) and the copyright owner are credited and that the original publication in this journal is cited, in accordance with accepted academic practice. No use, distribution or reproduction is permitted which does not comply with these terms.
*Correspondence: Thorsten J. Maier, VGhvcnN0ZW5KdWVyZ2VuLk1haWVyQGtndS5kZQ==