- 1Chinese Cochrane Center, West China Hospital, Sichuan University, Chengdu, China
- 2Key Laboratory of Chinese Internal Medicine of Ministry of Education and Beijing, Dongzhimen Hospital, Beijing University of Chinese Medicine, Beijing, China
- 3Institute of Integration of Traditional Chinese and Western Medicine, Guangzhou Medical University, Guangzhou, China
Cardiovascular diseases (CVDs) are the major public health problem and a leading cause of morbidity and mortality on a global basis. Wenxin Keli (WXKL), a formally classical Chinese patent medicine with obvious efficacy and favorable safety, plays a great role in the management of patients with CVDs. Accumulating evidence from various animal and cell studies has showed that WXKL could protect myocardium and anti-arrhythmia against CVDs. WXKL exhibited its cardioprotective roles by inhibiting inflammatory reaction, decreasing oxidative stress, regulating vasomotor disorders, lowering cell apoptosis, and protection against endothelial injure, myocardial ischemia, cardiac fibrosis, and cardiac hypertrophy. Besides, WXKL could effectively shorten the QRS and Q-T intervals, decrease the incidence of atrial/ventricular fibrillation and the number of ventricular tachycardia episodes, improve the severity of arrhythmias by regulating various ion channels with different potencies, mainly comprising peak sodium current (INa), late sodium current (INaL), transient outward potassium current (Ito), L-type calcium current (ICaL), and pacemaker current (If).
Introduction
Cardiovascular diseases (CVDs) are the major public health problem and a leading cause of premature death throughout the world. Approximately 17.7 million people died from CVDs in 2015, accounting for 31% of all global deaths (WHO, 2017). As a result of population growth, the aging of populations, and epidemiologic changes in disease, the prevalence of cardiovascular morbidity and mortality continues to rise (Roth et al., 2015). Cardiac arrhythmias, a type of CVDs, is a disease that is caused by abnormal electrical activity in the heart rate (HR) or rhythm, further affecting the pumping function of the heart (Nattel et al., 2014). Currently, the electrophysiological mechanisms responsible for cardiac arrhythmias mainly divided into two aspects: enhanced or abnormal impulse formation (i.e., focal activity) and conduction disturbances (i.e., re-entry) (Antzelevitch and Burashnikov, 2011), which results in premature beat, atrial fibrillation (AF), ventricular fibrillation (VF), atrioventricular block, and other arrhythmic diseases.
It is estimated that the number of patients with AF in 2030 in Europe will be 14–17 million and the number of new cases of AF per year at 120,000–215,000 (Zoni-Berisso et al., 2014). Around 40–50% of all cardiovascular deaths are sudden cardiac deaths, while approximately 80% of these are caused by ventricular tachyarrhythmias (Mehra, 2007). In addition, cardiac arrhythmia is also caused by cardiovascular organic lesions, such as myocardial ischemia, fibrosis, cardiac hypertrophy, impaired cardiac function and so forth, which further influence electrophysiological activity by changing the structure of the heart (Nguyen et al., 2017; Wu et al., 2017a). Traditional Chinese medicine (TCM) has more than 2,000 years of history and has gained widespread clinical applications in patients with CVDs for thousands of years and is still being commonly used in modern times in both China and elsewhere worldwide (Hao et al., 2017).
Wenxin Keli (WXKL), a formally classical Chinese patent medicine developed at the Guang’anmen Hospital of the Chinese Academy of Chinese Medical Sciences, is the first Chinese anti-arrhythmic medicine to be approved by the China Food and Drug Administration and has been increasingly used as an alternative approach for CVDs globally. The main ingredients of WXKL consist of Codonopsis Radix (Dang Shen), Polygonati Rhizoma (Huang Jing), Notoginseng Radix Et Rhizoma (San Qi), Ambrum (Hu Po), and Nardostachyos Radix Et Rhizoma (Gan Song), which can tonify qi, supply yin, promote blood circulation and remove blood stasis according to the TCM theory (Pharmacopoeia, 2015). Detailed extraction process and drug instruction were shown in Supplementary Material 1. Several studies exhibited that WXKL had better clinical efficacy in the treatment of CVDs (Wang et al., 2016). WXKL appeared to be more effective in improving P-wave dispersion as well as maintenance of sinus rhythm in patients with paroxysmal AF (Chen et al., 2013b). WXKL also reduced the frequency of ventricular premature complexes (VPCs), increased left ventricular ejection fraction (LVEF) and 6-min walking test in patients with VPCs and heart failure (HF) (Chen et al., 2014; He et al., 2016; Li et al., 2017a). Similarly, many studies demonstrated the key role of WXKL against CVDs in animal and cell experiments, but there is a lack of comprehensive and systematic evidence. This review summarizes extensively current experimental and mechanism studies on the use of WXKL in CVDs, and our understanding of WXKL’s cardioprotective and antiarrhythmic effects.
Methodology
The terms “Wenxin” or “Wen xin” were searched as “Title/Abstract” and MeSH terms in PubMed, China National Knowledge Infrastructure(CNKI) and SinoMed database. Articles related to therapeutic effects in CVDs were picked out manually. All articles with abstract were included and no language restrictions was applied.
Results
The Cardioprotective Effects of WXKL
The damage of endothelial cell structure and function is the main pathological basis of many CVDs (Hadi et al., 2005; Gutiérrez et al., 2013). When stimulated by various factors such as dyslipidemia immune, oxidative stress and virus, the endothelium will be injured. Masses of low density lipoprotein (LDL) enters intima and is converted into oxidized LDL (ox-LDL), which will lead to the release of cytokines (interleukin-1, IL-1; interleukin-6, IL-6; monocyte chemoattractant protein-1, MCP-1) and monocyte emigration into intima. When monocytes enter the intima through the endothelium, they will become macrophages and provide antigens for the lymphocytes, producing a local immune response. Macrophages consume a substantial number of ox-LDL and convert them into macrophage-derived foam cells, which release large amounts of cytokines and growth factors, resulting in the migration and proliferation of smooth muscle cells from media to intima. And smooth muscle cells further uptake lipid then become smooth muscle cell-derived foam cells. Those foam cells accumulate into masses, forming pale-yellow lipid plaques. With collagen fibrils proliferation, yellow-gray fibrous cap will cover intima surface, causing coronary atherosclerosis and angina. In addition, some immune cells, inflammatory factors and proteolytic enzymes easily weaken the thin fibrous cap and transform stable plaque to an unstable vulnerable plaque, contributing to plaque disruption, platelet aggregation and thrombosis, finally resulting in myocardial ischemia and necrosis, generating acute coronary syndrome (ACS) and sudden death (Santos-Gallego et al., 2014; Androulakis et al., 2017). Thus, the main pathological mechanism of atherosclerosis in CVDs is closely related to endothelial erosion, disorders of lipid metabolism, inflammatory reaction, myocardial apoptosis, and so forth (Figure 1).
Twenty-two articles exerted the cardioprotective properties of WXKL in CVDs, involving in myocardial ischemia, myocardial infarction (MI), ischemia/reperfusion (I/R), transverse aortic constriction (TAC), congestive heart failure, spontaneously hypertensive animal models and Ang II/Norepinephrine (NE)-induced H9C2 cells, the basic characteristic of included studies was shown in Table 1. And the detailed experimental mechanism was as follows.
Anti-inflammation
Inflammation dominates in atherosclerosis and CVDs. Immune cells gather in the early atherosclerotic lesions and induce migration and proliferation of smooth muscle cells. The aggravation of inflammation in the arterial wall also causes instability of atheromatous plaques and formation of occlusive thrombosis, contributing to atherosclerotic CVD events, including ACS and stroke (Ohira et al., 2017). WXKL exhibited its anti-inflammation property by lowering the level of IL-6, tumor necrosis factor-α (TNF-α) and high sensitivity C-reactive protein (hs-CRP) (Cao et al., 2016). In addition, WXKL could downregulate inflammatory related gene expression, such as chemokine receptor 1 (CX3CR1), mannose receptor ctype1 (MRC1), and formyl peptide receptor 1 (FPR1) (Zheng et al., 2016), as shown in Figure 2A.
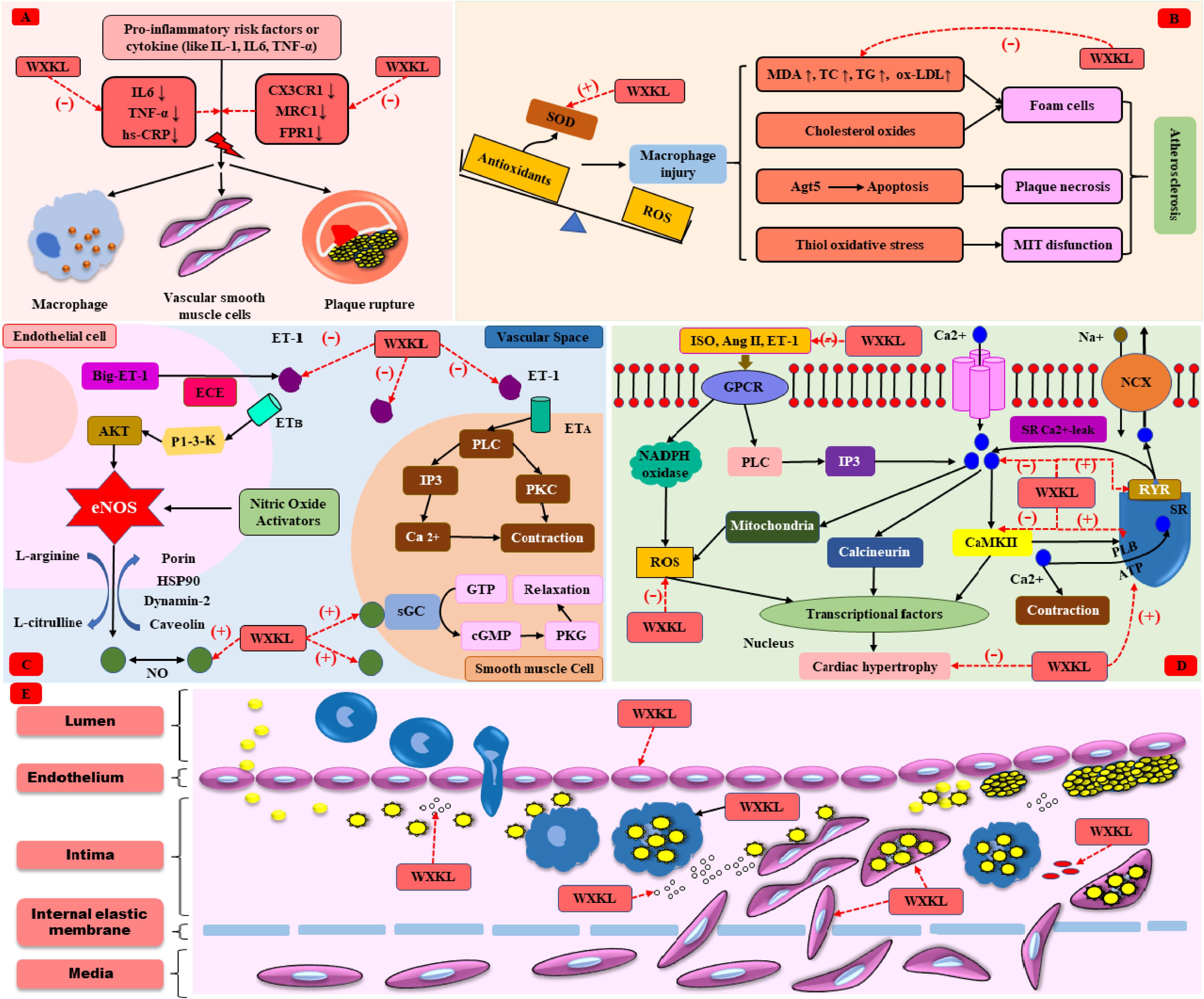
FIGURE 2. The cardioprotective mechanism of WXKL. (A) Anti-inflammation. (B) Anti-oxidative stress. (C) Regulation of vasomotor. (D) Anti-cardiac hypertrophy. (E) Anti-atherosclerosis.
Anti-oxidative Stress
Oxidative stress is defined as an imbalance between the generation of reactive oxygen species (ROS) and the ability to scavenge these ROS by endogenous antioxidative systems, where ROS overwhelms the antioxidative capacity. Oxidative stress enables to result in irreversible damage to cell membranes, DNA and cellular metabolism, and plays a crucial role in the pathogenesis of CVDs (Farías et al., 2017). As far as we know, superoxide dismutase (SOD) is a well-known, first-line defiance antioxidative enzyme that protects cells from the toxic effects of superoxide radicals, while malondialdehyde (MDA) is the final product of lipid peroxidation induced by ROS, which can result in cross-linking in lipids, proteins, and nucleic acids, and is frequently used to define oxidative stress (Bulut et al., 2007; Valko et al., 2016). Oxidative stress mediated atherosclerosis and causes CVDs (Yang et al., 2017b). Recent articles indicated that MDA was slightly increased and SOD was slightly decreased in the I/R rats/rabbits when compared with the controls, and WXKL could recovery the level of SOD and MDA, play a crucial role in anti-oxidative stress (Wang et al., 2014; Zhou and Sun, 2015). Moreover, WXKL could improve the secretions of taurine and ketone bodies to overcome the oxidative stress and the shortage of energy sources induced by I/R (Jiang et al., 2017). The mechanism of atherosclerosis caused by oxidative stress (Yang et al., 2017b) and the anti-oxidative role of WXKL were shown in Figure 2B.
Endothelial Protective Effect
The endothelium is a monolayer of cells covering the internal lumen of all blood vessels, thereby separating the blood from the vascular wall and organ tissues. Dysfunction of endothelial cells is directly associated with impaired vasorelaxation, increased inflammation, and increased migration and proliferation of smooth muscle cells, is the initiating factor in CVDs. Thus, protecting VECs from injury is very significant. In ischemic myocardial tissue, the nucleus of vascular endothelial cells was irregular, the chromatin agglomerated, the basement membrane was dissolved, the endothelia cells appeared protuberance, the cytoplasm was dense, and the pericytes were edema and cavitation. The mitochondria were slightly swollen in the endothelial cells. Current animal evidence indicated that WXKL enabled to effectively recovery ultrastructure of damaged endothelial cells in a dose-depended way (Liu et al., 2007a; Guan et al., 2011; Wang et al., 2014). Besides, anti-inflammatory and anti-oxidative effects were also beneficial to protect endothelium.
Regulation of Vasomotor
Nitric oxide (NO) is a multifunctional versatile molecule, especially regulating vascular tone. In the vasculature, NO directly activates the heme moiety of soluble guanylate cyclase leading to the production of cyclic GMP (cGMP). An increase in intracellular cGMP further result in the activation of protein kinase G (PKG), finally contributing to vasodilatation. Endothelin-1 (ET-1) is also a kind of endothelium-derived mediator, mediating vasoconstriction by activating PLC-induced endothelin signaling pathway. The delicate equilibrium between NO and ET-1 is essential for maintaining vascular homeostasis (Khimji and Rockey, 2010). A lot of results indicate that plasma ET-1 levels are upregulated in patients with coronary heart disease (Hoffmann et al., 1998; Zhu et al., 2011). In addition, many studies in our review showed that NO decreased and ET-1 increased in MI or I/R rats. And WXKL could restore this imbalance between NO and ET-1 (Liu et al., 2007a; Guan et al., 2011; Wang et al., 2014). The role of NO and ET-1 in regulating vasomotor (Khimji and Rockey, 2010) and effects of WXKL were shown in Figure 2C.
Anti-myocardial Ischemia
Myocardial ischemia refers to the decrease in the blood perfusion of the heart, leading to the reduction of oxygen supply to the heart and the abnormal metabolism of the myocardial energy. Minutes after the onset of ischemia, reversible ultrastructural cardiomyocyte changes appear, including cellular and mitochondrial swelling and glycogen depletion, causing irreversible myocardial necrosis. MI defined as the death of cardiac myocytes due to prolonged ischemia, affecting millions of individuals each year and represents a considerable economic burden to healthcare systems worldwide (Tao et al., 2017). When AMI occurs, reperfusion of the ischemic myocardium is a valuable approach for limiting infarct size. However, reperfusion itself paradoxically result in further complications involving acceleration of cell death, diminished contractile function (stunning), and arrhythmias, which is called myocardial I/R injury. No effective therapy is currently available to protect the heart from this I/R injury (Eltzschig and Eckle, 2011).
In isoproterenol (ISO) or adriamycin hydrochloride (ADR) induced myocardial ischemia animal model, WXKL enabled to decrease the maximal value of ST segment elevation and the level of creatine kinase (CK), improve the systolic and diastolic function of left ventricle and prevented left ventricular dilatation (Liu et al., 2007b; Zhou et al., 2007; Xiang et al., 2015). Underwent the surgery of ligation of the left anterior descending coronary artery, MI rats or rabbits inhibited that myocardial fibers arrangement was discorded, numerous neutrophil granulocytes were seen to be infiltrating, and wide range of necrosis observed, while some cytoplasts showed intense staining according to myocardial histopathology. In addition, there was a large number of myocardial apoptotic nuclei and the apoptosis rates were significantly increased after the coronary artery occlusion surgery. WXKL effectively restored the LVEF and left ventricular fractional shortening (LVFS), improved left ventricular end-diastolic dimension (LViDd) and left ventricular end-systolic dimension (LViDs), reduced MI size and apoptosis rate (Zeng et al., 2006; Liu et al., 2012; Guo et al., 2013; Wu et al., 2013, 2017a,b; Xing et al., 2013; Wang and Liu, 2014; Zheng et al., 2016; Jiang et al., 2017). And WXKL also could downregulate genes associated with apoptosis, such as cathepsin C (CTSC), tetratricopeptide repeat domain 5 (TTC5), and upregulated angiogenesis promoting genes such as R-spondin 3 (RSPO3) (Zheng et al., 2016).
Anti-cardiac Fibrosis and Anti-cardiac Hypertrophy
Cardiac fibrosis is a common reaction of the heart to many kinds of injuries and is the key pathological process in various CVDs (Mohammed et al., 2015). In cardiac fibrosis, excessive collagen deposition and accumulation of extracellular matrix lead to a decline in myocardial compliance and electrical conduction is also affected, further result in cardiac dysfunction, myocardial hypertrophy, HF, and arrhythmias (Creemers and Pinto, 2011; Donekal et al., 2014). Cardiac hypertrophy is originally an adaptive response of the heart to pathophysiologic stimuli, in an attempt to balance the stress in the ventricular wall and preserve cardiac function. However, persistent hypertrophy caused by heart damage such as hypertension or MI can eventually lead to arrhythmias, dilated cardiomyopathy, and HF, which are the leading cause of sudden death (Burchfield et al., 2013; Xie et al., 2013). Nearly 50% of patients with HF have preserved LVEF, with elevated interstitial myocardial collagen content, interstitial fibrosis and cardiomyocyte hypertrophy as prominent features of tissue remodeling (Borlaug and Paulus, 2011; Shah, 2013). Altogether, cardiac fibrosis and hypertrophy are associated with an increased risk of CVDs.
Masson’s trichrome staining showed that the TAC rats had fibrosis, and collagen deposition was apparent. The mitochondria were swollen, and the ridges were broken in the TAC group. Several studies (Xing et al., 2013; Yang et al., 2017a) indicated that WXKL decreased the accumulation of type III collagen fibers via regulating the calcium/calmodulin dependent kinase II (CaMKII) signaling pathway. In addition, many of the cardiac muscle cells had been dissolved, and some gaps appeared, after WXKL drug treatment, the myocardial cells showed slight recovery, but there was still a significant change in the morphology of mitochondria. Moreover, the myocardial cells from TAC/MI rats usually presented abnormal electrophysiological activity, for instance, the action potential duration at 90% repolarization (APD90) was significantly prolonged or abnormalities of calcium channel. WXKL-treated group thus showed a shortened APD90, decreased the incidences of early afterdepolarizations (EAD) and delayed afterdepolarizations (DADs) (Xing et al., 2013; Yang et al., 2017a). In TAC-induced HF rabbits model, WXKL could promote the mRNA and protein expression of sarcoplasmic reticulum Ca2+ ATPase (SERCA2a) (Lian et al., 2014), the pathophysiology of cardiac hypertrophy (Karmazyn et al., 2008; Stansfield et al., 2014; Yano and Okuda, 2014) and the function of WXKL were shown in Figure 2D.
Gap junction channels represent the best-known intercellular communication in the cardiovascular system for maintenance of the normal cardiac rhythm, regulation of vascular tone and endothelial function as well as metabolic interchange between the cells (Pieperhoff and Franke, 2007). In the normal adult heart, there exists three main isoforms: Connexin 40 (Cx40), Connexin 43 (Cx43), and Connexin 45 (Cx45), Cx43 is the most abundant and is expressed in atrial and ventricular myocytes (Severs et al., 2008). Previous studies exhibited that Cx43 expression is down-regulated when cardiac hypertrophy occurs (Yang et al., 2012; Wu et al., 2017a,c; Long et al., 2017), further leading to arrhythmia. WXKL protected the ultrastructure of the gap junctions and raised the phosphorylated Cx43 (Cx43-p)/non-phosphorylated Cx43 (Cx43-np) ratio (Wu et al., 2017a,c) and Cx43 mRNA (Yang et al., 2012; Long et al., 2017). Besides, WXKL enabled to significantly enhance the VF threshold (VFT) and reduced Q-T dispersion (Long et al., 2017; Wu et al., 2017a) to suppress arrhythmia. At the level of single cardiomyocytes, hypertrophy is simply defined as an increase in the cardiomyocyte size. WXKL also shortened the Ang II/NE-induced H9C2 cells length and width (Yang et al., 2012; Ren et al., 2016). In addition, WXKL could reduce the expression of the β-catenin protein and c-myc, involving in the Wnt/β-catenin signaling pathway (Wang et al., 2011a).
As stated above, WXKL exerted its cardioprotective effects through inhibiting inflammatory reaction, reducing oxidative stress, regulating vasomotor disorders, decreasing cell apoptosis, and protection against endothelial injure, atherosclerosis, myocardial ischemia, cardiac fibrosis, and cardiac hypertrophy, as shown in Figures 2A–E, 3. In addition, WXKL could raise the serum globulin and serum lysozyme, reduce the serum myocardial enzymes, such as CK isoenzyme (CK-MB), lactate dehydrogenase (LDH), aspartate aminotransferase (AST) (Jin et al., 2006; Zhang et al., 2012), and WXKL also increased the levels of miR-1 and miR-133 (Wu et al., 2017a,b).
The Antiarrhythmic Effects of WXKL
Several articles exhibited that WXKL played a great role in arrhythmia. In a model of isolated guinea pig hearts, rapid perfusion of quinidine altered the HR and prolonged the Q-T interval, pretreatment with WXKL significantly shortened the QRS and Q-T intervals (Xue et al., 2013; Wang et al., 2017). Besides, WXKL reduced the incidence of VF and the number of ventricular tachycardia (VT) episodes, improved the severity of arrhythmias (Jin et al., 2006; Liang et al., 2012; Liu et al., 2012; Guo et al., 2013; Minoura et al., 2013; Wang et al., 2013). HF was produced by combined aortic insufficiency and abdominal aortic constriction, WXKL could prevent arrhythmias via shortening sinoatrial conduction time (SACT) in HF rabbits (Liu et al., 2011). WXKL also enabled to decreased the maximal diastolic potential (MDP), amplitude of action potential (APA), rate of pacemaker firing (PRF), velocity of diastolic depolarization (VDD), maximal rate of depolarization (Vmax), and so forth (Xu et al., 2010). In addition, persistent AF was inducible in 100% atria pre-treated with Acetylcholine, WXKL prevented the induction of persistent AF in 100% of preparations tested (Wang et al., 2011b) (Table 2).
The generation of myocardial cell action potentials is the basis of cardiac electrical activity, which includes five phases (from 0 to 4) (Tse, 2016). Depolarization from the sinoatrial node brings the membrane potential to the threshold, opening the voltage-gated sodium channels and giving rise to the peak current of sodium (INa) and the rapid upstroke (phase 0) of the cardiac action potential. Inactivation of the sodium channel and activation of transient outward potassium current (Ito) are the predominant contributors to the partial membrane repolarization in the first phase. Phase 2, the videlicet plateau, is a long phase due to the delicate balance between the inward currents (L-type calcium current, ICaL and late sodium current, INaL) and the outward currents (slow delayed rectifier K+ channels, Iks). As the inward currents (ICaL) become inactivated, the outward currents (Iks; rapid delayed rectifier K+ channels, Ikr and inwardly rectifier K+ channels Ik1) predominate, causing further repolarization and bringing the membrane potential toward the potassium equilibrium potential (phase 3). Then, the membrane potential returns to its resting potential after full repolarization during phase 4, which depends on numerous components (Na+/K+ – ATPase pump, the Na+/Ca2+ exchanger) to recover the normal concentration gradient of the myocardial cell membrane (Figure 4). WXKL inhibits various cardiac ion channels with different potencies acting mainly on peak and late INa, with some effect on Ito and ICaL channels.
Role of WXKL in Sodium Channels
Sodium channels are widely distributed in myocardial cells in mammals and mediate the excitability and conduction of the heart. There are main two sub-types of sodium currents, one is the INa, the other is the INaL. INa is primarily responsible for depolarization (phase 0) in myocardial action potentials while INaL maintains balance in the plateau as a kind of inward current. WXKL produces atrial-selective depression of INa-dependent parameters in canine isolated coronary perfused preparations and was effective in reducing INa and shifting steady-state inactivation to more negative potentials in HEK293 cells stably expressing SCN5A (Burashnikov et al., 2012). Moreover, WXKL could inhibit INa more preferentially in the atria and lowered resting membrane potential, resulting in post-repolarization refractoriness in atrial myocytes, and therefore suppressed AF via an anti-re-entrant mechanism (Hu et al., 2016).
More recent evidence suggests the INaL, which lasts hundreds of milliseconds after a depolarizing pulse, may delay repolarization, prolong APD, alter intracellular sodium and calcium homeostasis, and lead to EAD/DAD and a series of triggered activities, potentially predisposing to arrhythmias and sudden cardiac death (Shryock et al., 2013; Zaza and Rocchetti, 2013; Horvath and Bers, 2014; Remme and Wilde, 2014). WXKL exhibited antiarrhythmic properties via selective inhibition of INaL (Xue et al., 2013; Hou et al., 2016). In addition, WXKL attenuated intracellular Ca2+ overload induced by hypoxia-reoxygenation in ventricular myocytes via inhibiting INaL and finally prevented the occurrence of arrhythmia (Luo et al., 2017). It is all known that cardiac Purkinje cells (PCs) played a crucial role in the propagation of impulse from atrioventricular node to ventricular muscle and may initiate a variety of ventricular arrhythmias (Huang et al., 2014). WXKL effectively abbreviated the APD of PCs in a dose- and rate-dependent manner by recording ion currents through whole-cell patch clamp technique, and inhibited ISO-induced EADs, DADs, and TAs by selective inhibition of INaL (Hou et al., 2016). The mechanism of sodium channel regulating arrhythmia (Remme and Wilde, 2014) and the effects of WXKL were shown in Figure 5A.
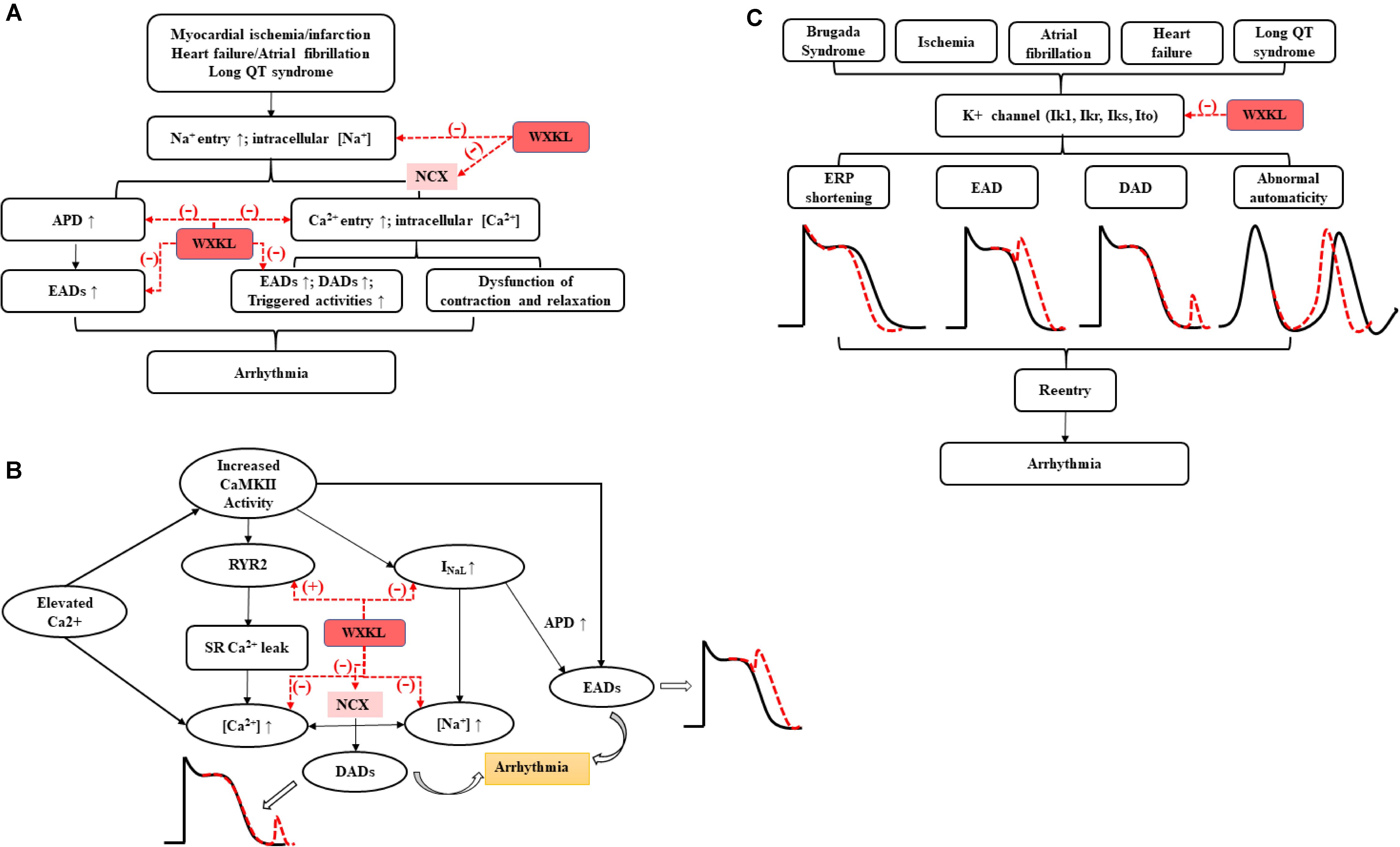
FIGURE 5. The antiarrhythmic effects of WXKL on (A) sodium channels, (B) calcium channels, and (C) potassium channels.
Role of WXKL in Calcium Channels
There are three types of calcium channels in myocardial cells, i.e., B-type, L-type, and T-type (Rosenberg et al., 1988). L-type and T-type calcium channels plays major roles in myocardial electrophysiological activity. The activated L-type calcium channel is capable of generating a slow ICaL, which is the ionic basis of ventricular cardiac action potentials during phase 2 (Yamaoka and Kameyama, 2003). T-type calcium channels mainly exist in cardiac autonomic cells, such as sinoatrial node cells, and are activated to affect the pacemaker activity of the heart through a slow inward calcium current (ICaT) (Ono and Iijima, 2010).
Wenxin Keli could attenuate intracellular Ca2+ overload in ventricular myocytes by suppressing the ICaL (Luo et al., 2017). Additionally, WXKL inhibits ICaL decelerating the activation process and delaying recovery from inactivation without changing the inactivation process (Wang et al., 2013). In the normal and hypertrophied myocytes, WXKL decreased the ICaL by accelerating the inactivation of the channels and delaying the recovery time from inactivation, which ultimately resulted in the treatment of arrhythmias (Chen et al., 2013a). WXKL also inhibited the current density of ICaL in a concentration-dependent manner, decreased rates of the peak ICaL and shifted up the current–voltage curve (Wang et al., 2011b). Besides, Nardostachys chinensis Batal (Gan Song), one of the WK components, dose-dependently blocked its predicted target CaV 1.2 channel in an electrophysiological assay (Wang et al., 2017).
The past decade has seen the emergence of CaMKII as a critical regulator in many cardiac pathologies, especially arrhythmia. Altered L-type Ca2+ channel gating due to CaMKII hyper-activity could elicit EADs by increasing ICaL. NaV 1.5 phosphorylation increases INaL and may result in non-equilibrium reactivation of INa, both of which can prolong APD and trigger EADs. Besides, Increased CaMKII activity in disease has been linked to elicit gain-of-function effects at ICaL, thereby increasing Ca2+ influx and leading to Ca2+ overload. CaMKII also could contribute to Na+-induced Ca2+-overload through NCX. Moreover, CaMKII directly phosphorylates RyR2, which has been shown to promote spontaneous Ca2+ release. Both of above processes can further trigger DADs, resulting in the cardiac arrhythmia (Asakura et al., 2014; Vincent et al., 2014). Many studies demonstrated the functions of WXKL on arrhythmia via regulating calcium channels, reducing the ICaL, preventing the occurring of EAD and DAD via acting on CaMKII signaling pathway (Chen et al., 2013a; Xing et al., 2013; Xue et al., 2013; Hou et al., 2016; Luo et al., 2017; Yang et al., 2017a) (Figure 5B).
Role of WXKL in Potassium Channels
There are four types of potassium channels in myocardial cells, i.e., Ito, Ik1, Ikr, and Iks. The role of Ik1, Ikr, and Iks are to influence the resting potential of the myocardial cell membrane and repolarization (phases 2 and 3) in myocardial cells (Grandi et al., 2017). Ito, the main current responsible for the early rapid repolarization (phase 1) in fast response cells, has been proven to exist extensively in myocardial cells, especially in atrial and ventricular myocytes in mammals. It has a significant effect on the shape and the duration of the cardiac action potential (Barry et al., 1998). In addition, the Ito is characterized by a transmural gradient in current density across the ventricular myocardium that leads to significant differences in cardiac action potentials between the endocardium and the epicardium (Perrin et al., 2014). This distribution gives rise to repolarization heterogeneity and is probably responsible for the main pathogenesis of VT and VF. WXKL mainly acted on potassium ion channels and regulated the abnormal production of Ito (Wang et al., 2010, 2013; Minoura et al., 2013).
Brugada syndrome (BrS) is an inherited cardiac disorder characterized by a coved-type ST-segment elevation in the right precordial leads and increased risk of associated with a high incidence of sudden death due to the development of life-threatening polymorphic VT and VF (Sieira and Brugada, 2017). The present study showed the effect of WXKL to suppress the electrocardiographic and arrhythmic manifestations of BrS in a coronary-perfused canine RV wedge model of the syndrome. Its function to inhibit phase 2 re-entry and VT/VF involved the prevention of Ito as well as an indirect adrenergic stimulation via a tyramine-like effect (Minoura et al., 2013). In addition, the present study demonstrated that WXKL enabled to reduce ischemia-induced ventricular arrhythmias in rats by inhibiting Ito in a concentration-dependent manner (Wang et al., 2013). WXKL also suppressed the current density of Ito, decreased rates of the peak Ito and shifted down the current–voltage curve in single ventricular myocytes (Wang et al., 2011b). The functional roles of potassium currents in the arrhythmias (Chiamvimonvat et al., 2017) and intervention of WXKL were shown in Figure 5C.
Role of WXKL in HCN Channels
Hyperpolarization-activated, cyclic nucleotide-gated (HCN) channels belong to the superfamily of voltage-gated pore-loop cation channels, including HCN1–HCN4 isoforms (Santoro and Tibbs, 1999). Human genetic and animal studies have highlighted the involvement of HCN family of channels in the heart where they are responsible for the pacemaker current (If) current in the sinoatrial node (Herrmann et al., 2012). In the ventricular tachypacing-induced CHF dogs, HCN2 and HCN4 expression was higher in sinoatrial node than the right atrium. CHF significantly reduced sinus node HCN expression at both protein and mRNA levels (Zicha et al., 2005). WXKL enhanced the amplitude of instantaneous current of HCN2 in a concentration-dependent manner, slowed channel activation and deactivation processes (Li et al., 2011).
Discussion
In the past two decades, a breakthrough has been achieved in the pharmacology of WXKL. The knowledge of WXKL offers a new chance for the prevention and treatment of CAD, mainly reflecting in two aspects: cardioprotective effects and anti-arrhythmias. WXKL exerted its cardioprotective properties by inhibiting inflammatory reaction, decreasing oxidative stress, regulating vasomotor disorders, lowering cell apoptosis, and protection against endothelial injure, myocardial ischemia, cardiac fibrosis, and cardiac hypertrophy. Besides, WXKL could effectively shorten the QRS and Q-T intervals, decreased the incidence of VF and the number of VT episodes, improved the severity of arrhythmias by regulating various ion channels with different potencies, mainly comprising peak and late INa, Ito, ICaL, and If. WXKL also enabled to decreased the MDP, APA, PRF, VDD, Vmax, and so forth.
The pharmacological effects of WXKL were closely related to its components. As we all know, WXKL is a Chinese herb extract that includes five main components: Codonopsis Radix (Dang Shen), Polygonati Rhizoma (Huang Jing), Notoginseng Radix Et Rhizoma (San Qi), Ambrum (Hu Po), and Nardostachyos Radix Et Rhizoma (Gan Song). Developed in various forms over the past 2,000 years, the main components of WXKL were used by millions today for the treatment of a variety of CVDs. Codonopsis Radix (Dang Shen), a TCM herb, has been used in clinical applications for hundreds of years. Several studies have focused on its immune-enhancing properties through acting on the regulation of the lymphocytes, cytokines, antibody level, and the neuroendocrine-immune network (Zhao et al., 2013). Notoginseng Radix Et Rhizoma (San Qi), a valuable herb in TCM with obvious efficacy and favorable safety, played a crucial role in CADs and was increasingly recognized clinically. Many experimental and clinical studies have demonstrated that Notoginseng Radix Et Rhizoma (San Qi) could regulate lipid metabolism, reduce the inflammatory reaction and myocardial damage, improve the energy metabolism of myocardial cells, inhibit ischemia-induced cardiomyocyte apoptosis in acute MI rats and so forth (Duan et al., 2017). Nardostachyos Radix Et Rhizoma (Gan Song), the rhizomes and roots of Nardostachys jatamansi DC, could rectify qi, relieve pain, resolve constraint and fortify the spleen. In addition, Nardostachyos Radix Et Rhizoma (Gan Song) exhibited the cardioprotective effects via inhibiting myocardial apoptosis, inflammation, oxidative stress and showed a great promise as a novel option for arrhythmia by regulating several ion channels (Ik, Ik1, INa, ICaL, and Ito) (Li et al., 2017b).
Genetic, drug and environment factors, oxidative stress, inflammatory cytokines, disorder of lipid metabolism, endothelial injury, all of which cannot only lead to atherosclerosis, myocardial injury and fibrosis, but also result in cardiac autonomic changes and electrophysiological abnormalities of cardiomyocytes, eventually causing cardiac remodeling. Such cardiac remodeling involves complex structural remodeling and electrical remodeling, which is more commonly seen in the myocardial injury, especially in coronary artery disease. Cardiac remodeling is often used as an adaptive response to functional or structural stress, which initially compensates and maintains cardiac function, but it may then turn into a non-adaptive change, causing progressive pump failure and/or malignant arrhythmia (Lazzerini et al., 2017) (Figure 6). WXKL exhibited multiple protective effects on myocardium and regulation functions on ion channels, these two properties were complementary and beneficial to each other. In brief, WXKL comprehensively treats and regulates CVDs.
A large number of studies have shown that lipid metabolism disorders play an important role in the pathogenesis of atherosclerosis and cardiac death in CVDs, specifically increased total cholesterol (TC), triglycerides (TG), LDL-C, and ox-LDL (Charland and Stanek, 2014). In clinical practice, WXKL could regulate lipid metabolism by reducing TC, TG, LDL, and raising HDL and the level of apolipoprotein A (ApoA)/apolipoprotein B (ApoB) in patients with incidental atrial premature (Li, 2018). Panax notoginseng (San Qi), one of main components of WXKL, could decrease cholesterol ester in foam cells by up-regulation of ATP-binding cassette transporter A1 (ABCA1) and ATP-binding cassette transporter G1 (ABCG1) (Jia et al., 2010; Fan et al., 2012). However, there is no report of WXKL to regulate dyslipidemia in the current experimental study. The effect of WXKL on regulating lipid metabolism remains to be further studied.
Nowadays, anti-arrhythmic drugs were divided into four categories according to the Vaughan-Williams classification scheme (Vaughan-Williams, 1977). Among them, the third anti-arrhythmic drugs (mainly inhibiting Ik+) have the functions of prolonging ADP. Several studied presented that WXKL may own the similar effect (Cui, 2007; Chen et al., 2010; Xu et al., 2010). However, compared with Ik+, some experiment studies exhibited that INa-L and ICa have opposite effects on APD, causing the prolongation of APD and the triggering of EADs/DADs. WXKL has played an important role in shortening APD90 and decreasing the incidences of EADs/DADs (Chen et al., 2013a; Xue et al., 2013; Hou et al., 2016; Luo et al., 2017). WXKL could inhibit above currents simultaneously, thus, WXKL has a double-sided effect on APD, the anti-arrhythmic mechanism will depend on the cell type, as well as the degree of contribution of these currents in repolarization at any given time.
Limitations and Perspectives
There were some limitations in this review. Firstly, taking nuclear factor-κB, PLC, protein kinase B for instance, many mechanisms of protecting myocardium with WXKL are still not clear. Secondly, the studies mainly involved cardiac working cells (atrial and ventricular myocytes), the other autonomous cells, such as sinoatrial node cells and Purkinje cells, were lack of more research, resulting in the insufficient evidence on the mechanism of WXKL. Then, no studies have reported the effects of WXKL on ICaT, Iks, or Ikr. And current studies showed that WXKL enabled to inhibit the tachyarrhythmia, however, as for the bradyarrhythmia, whether WXKL is an appropriate medicine remains unclear. In view of above deficiencies, more studies need to be further explored.
Conclusion
Wenxin Keli has been observed to have multiple positive functions in CVDs, including myocardial protection and anti-arrhythmia. WXKL played its cardioprotective roles by inhibiting inflammatory reaction, decreasing oxidative stress, regulating vasomotor disorders, lowering cell apoptosis, and protection against endothelial injure, myocardial ischemia, cardiac fibrosis, and cardiac hypertrophy. Besides, WXKL could effectively shorten the QRS and Q-T intervals, decreased the incidence of VF and the number of VT episodes, improved the severity of arrhythmias by regulating various ion channels with different potencies, mainly comprising peak and late INa, Ito, ICaL, and If.
Author Contributions
HS, ML, and YL provided guidelines for this review. ML, GT, and YS wrote the main manuscript and prepared the Figures 1–6. SL, CL, SC, RQ, and XZ reviewed the literature available on this topic and prepared the Tables 1, 2.
Funding
This study was supported by the National Natural Science Foundation of China (Nos. 81430098 and 81674050) and National high-level talents special support plan (W02020052).
Conflict of Interest Statement
The authors declare that the research was conducted in the absence of any commercial or financial relationships that could be construed as a potential conflict of interest. The reviewer RZ and handling Editor declared their shared affiliation.
Acknowledgments
We would like to thank Prof. Baoshan Liu for his guidance on the design of the article.
Supplementary Material
The Supplementary Material for this article can be found online at: https://www.frontiersin.org/articles/10.3389/fphar.2018.01005/full#supplementary-material
References
Androulakis, E., Zacharia, E., Papageorgiou, N., Lioudaki, E., Bertsias, D., Charakida, M., et al. (2017). High-density lipoprotein and low-density lipoprotein therapeutic approaches in acute coronary syndromes. Curr. Cardiol. Rev. 13, 168–182. doi: 10.2174/1573403X13666170209145622
Antzelevitch, C., and Burashnikov, A. (2011). Overview of basic mechanisms of cardiac arrhythmia. Card Electrophysiol. Clin. 3, 23–45. doi: 10.1016/j.ccep.2010.10.012
Asakura, K., Cha, C. Y., Yamaoka, H., Horikawa, Y., Memida, H., Powell, T., et al. (2014). EAD and DAD mechanisms analyzed by developing a new human ventricular cell model. Prog. Biophys. Mol. Biol. 116, 11–24. doi: 10.1016/j.pbiomolbio.2014.08.008
Barry, D. M., Xu, H., Schuessler, R. B., and Nerbonne, J. M. (1998). Functional knockout of the transient outward current, long-QT syndrome, and cardiac remodeling in mice expressing a dominant-negative Kv4 alpha subunit. Circ. Res. 83, 560–567. doi: 10.1161/01.RES.83.5.560
Borlaug, B. A., and Paulus, W. J. (2011). Heart failure with preserved ejection fraction: pathophysiology, diagnosis, and treatment. Eur. Heart J. 32, 670–679. doi: 10.1093/eurheartj/ehq426
Bulut, M., Selek, S., Gergerlioglu, H. S., Savas, H. A., Yilmaz, H. R., Yuce, M., et al. (2007). Malondialdehyde levels in adult attention-deficit hyperactivity disorder. J. Psychiatry Neurosci. 32, 435–438.
Burashnikov, A., Petroski, A., Hu, D., Barajas-Martinez, H., and Antzelevitch, C. (2012). Atrial-selective inhibition of sodium-channel current by Wenxin Keli is effective in suppressing atrial fibrillation. Heart Rhythm. 9, 125–131. doi: 10.1016/j.hrthm.2011.08.027
Burchfield, J. S., Xie, M., and Hill, J. A. (2013). Pathological ventricular remodeling: mechanisms: part 1 of 2. Circulation 128, 388–400. doi: 10.1161/CIRCULATIONAHA.113.001878
Cao, W. Z., Zhang, T., Zhong, D. C., Shi, Z. Y., and Yu, J. H. (2016). The influence of heart-stabilizing granules on inflammatory factor of patients with atrial fibrillation. Henan Tradit. Chin. Med. 36, 2101–2103. doi: 10.16367/j.issn.1003-5028.2016.12.0839
Charland, S. L., and Stanek, E. J. (2014). Sigmoidal maximal effect modeling of low-density lipoprotein cholesterol concentration and annual incidence of coronary heart disease events in secondary prevention trials. Pharmacotherapy 34, 452–463. doi: 10.1002/phar.1368
Chen, W. T., Huang, C. X., Guo, K., and Wang, X. (2010). Effect of wenxin particle on transmembrane action potential in rat left ventricle. Chin. J. Cardiac Pacing Electrophysiol. 24, 341–343. doi: 10.3969/j/issn.1007-2659.2010.04.016
Chen, Y., Li, Y., Guo, L., Chen, W., Zhao, M., Gao, Y., et al. (2013a). Effects of wenxin keli on the action potential and L-type calcium current in rats with transverse aortic constriction-induced heart failure. Evid. Based Complement. Alternat. Med. 2013:572078. doi: 10.1155/2013/572078
Chen, Y., Nie, S., Gao, H., Sun, T., Liu, X., Teng, F., et al. (2013b). The effects of wenxin keli on p-wave dispersion and maintenance of sinus rhythm in patients with paroxysmal atrial fibrillation: a meta-analysis of randomized controlled trials. Evid. Based Complement. Alternat. Med. 2013:245958. doi: 10.1155/2013/245958
Chen, Y., Xiong, X., Wang, C., Wang, C., Zhang, Y., Zhang, X., et al. (2014). The effects of wenxin keli on left ventricular ejection fraction and brain natriuretic Peptide in patients with heart failure: a meta-analysis of randomized controlled trials. Evid. Based Complement. Alternat. Med. 2014:242589. doi: 10.1155/2014/242589
Chiamvimonvat, N., Chen-Izu, Y., Clancy, C. E., Deschenes, I., Dobrev, D., Heijman, J., et al. (2017). Potassium currents in the heart: functional roles in repolarization, arrhythmia and therapeutics. J. Physiol. 595, 2229–2252. doi: 10.1113/JP272883
Creemers, E. E., and Pinto, Y. M. (2011). Molecular mechanisms that control interstitial fibrosis in the pressure-overloaded heart. Cardiovasc. Res. 89, 265–272. doi: 10.1093/cvr/cvq308
Cui, C. Z. (2007). Effect of wenxin granule on electrophysiological characteristics of adventitia and endomembrane in rabbit. World Chin. Med. 2, 304–305. doi: 10.3969/j.issn.1673-7202.2007.05.024
Donekal, S., Venkatesh, B. A., Liu, Y. C., Liu, C. Y., Yoneyama, K., et al. (2014). Interstitial fibrosis, left ventricular remodeling, and myocardial mechanical behavior in a population-based multiethnic cohort: the Multi-Ethnic Study of Atherosclerosis (MESA) study. Circ. Cardiovasc. Imaging 7, 292–302. doi: 10.1161/CIRCIMAGING.113.001073
Duan, L., Xiong, X., Hu, J., Liu, Y., Li, J., and Wang, J. (2017). Panax notoginseng saponins for treating coronary artery disease: a functional and mechanistic overview. Front. Pharmacol. 8:702. doi: 10.3389/fphar.2017.00702
Eltzschig, H. K., and Eckle, T. (2011). Ischemia and reperfusion–from mechanism to translation. Nat. Med. 17, 1391–1401. doi: 10.1038/nm.2507
Fan, J. S., Liu, D. N., Huang, G., Xu, Z. Z., Jia, Y., Zhang, H. G., et al. (2012). Panax notoginseng saponins attenuate atherosclerosis via reciprocal regulation of lipid metabolism and inflammation by inducing liver X receptor alpha expression. J. Ethnopharmacol. 142, 732–738. doi: 10.1016/j.jep.2012.05.053
Farías, J. G., Molina, V. M., Carrasco, R. A., Zepeda, A. B., Figueroa, E., Letelier, P., et al. (2017). Antioxidant therapeutic strategies for cardiovascular conditions associated with oxidative stress. Nutrients 9:E966. doi: 10.3390/nu9090966
Grandi, E., Sanguinetti, M. C., Bartos, D. C., Bers, D. M., Chen-Izu, Y., Chiamvimonvat, N., et al. (2017). Potassium channels in the heart: structure, function and regulation. J. Physiol. 595, 2209–2228. doi: 10.1113/JP272864
Guan, X. M., Meng, R. S., Chen, S. W., Jin, W., Xi, C., Pei, Z. H., et al. (2011). Effects of wenxinkeli on the ultrastructure of vascular endothelia cells in rats with myocardial infarction. Clin. Med. Eng. 18, 191–192. doi: 10.3969/j.issn.1674-4659.2011.02.0191
Guo, L., Li, Y., Zhao, F. J., and Tian, F. (2013). Anti-arrhythmia effect of Wenxin Keli in rats. J. Beijing Univ. Trad. Chin. 36, 472–475. doi: 10.3969/j.issn.1006-2157.2013.07.010
Gutiérrez, E., Flammer, A. J., Lerman, L. O., Elízaga, J., Lerman, A., and Fernández-Avilés, F. (2013). Endothelial dysfunction over the course of coronary artery disease. Eur. Heart J. 34, 3175–3181. doi: 10.1093/eurheartj/eht351
Hadi, H. A., Carr, C. S., and Al Suwaidi, J. (2005). Endothelial dysfunction: cardiovascular risk factors, therapy, and outcome. Vasc Health Risk Manage. 1, 183–198.
Hao, P., Jiang, F., Cheng, J., Ma, L., Zhang, Y., and Zhao, Y. (2017). Traditional chinese medicine for cardiovascular disease: evidence and potential mechanisms. J. Am. Coll. Cardiol. 69, 2952–2966. doi: 10.1016/j.jacc.2017.04.041
He, M., Lv, Z., Yang, Z. W., Huang, J. L., and Liu, F. (2016). Efficacy and safety of Chinese herbal medicine Wenxin Keli for ventricular premature beats: a systematic review. Complement. Ther. Med. 29, 181–189. doi: 10.1016/j.ctim.2016.10.007
Herrmann, S., Hofmann, F., Stieber, J., and Ludwig, A. (2012). HCN channels in the heart: lessons from mouse mutants. Br. J. Pharmacol. 166, 501–509. doi: 10.1111/j.1476-5381.2011.01798.x
Hoffmann, E., Assennato, P., Donatelli, M., Colletti, I., and Valenti, T. M. (1998). Plasma endothelin-1 levels in patients with angina pectoris and normal coronary angiograms. Am. Heart. J. 135, 684–688. doi: 10.1016/S0002-8703(98)70286-8
Horvath, B., and Bers, D. M. (2014). The late sodium current in heart failure: pathophysiology and clinical relevance. ESC Heart Fail. 1, 26–40. doi: 10.1002/ehf2.12003
Hou, J. W., Li, W., Guo, K., Chen, X. M., Chen, Y. H., Li, C. Y., et al. (2016). Antiarrhythmic effects and potential mechanism of WenXin KeLi in cardiac Purkinje cells. Heart Rhythm. 13, 973–982. doi: 10.1016/j.hrthm.2015.12.023
Hu, D., Barajas-Martínez, H., Burashnikov, A., Panama, B. K., Cordeiro, J. M., and Antzelevitch, C. (2016). Mechanisms underlying atrial-selective block of sodium channels by Wenxin Keli: experimental and theoretical analysis. Int. J. Cardiol. 207, 326–334. doi: 10.1016/j.ijcard.2016.01.016
Huang, J., Dosdall, D. J., Cheng, K. A., Li, L., Rogers, J. M., and Ideker, R. E. (2014). The importance of Purkinje activation in long duration ventricular fibrillation. J. Am. Heart. Assoc. 3:e000495. doi: 10.1161/JAHA.113.000495
Jia, Y., Li, Z. Y., Zhang, H. G., Li, H. B., Liu, Y., and Li, X. H. (2010). Panax notoginseng saponins decrease cholesterol ester via up-regulating ATP-binding cassette transporter A1 in foam cells. J. Ethnopharmacol. 132, 297–302. doi: 10.1016/j.jep.2010.08.033
Jiang, M., Wang, Q., Chen, J., Wang, Y., Fan, G., and Zhu, Y. (2017). Comparative metabonomics of Wenxin Keli and Verapamil reveals differential roles of gluconeogenesis and fatty acid β-oxidation in myocardial injury protection. Sci. Rep. 7:8739. doi: 10.1038/s41598-017-09547-w
Jin, L. L., Zhou, R. L., Chen, Y. X., Zeng, X. H., and Wang, Q. H. (2006). The protective effect of Wenxin keli on myocardial injury. Chin. J. Integr. Med. Cardio/Cerebrovasc. Dis. 4, 698–699. doi: 10.3969/j.issn.1672-1349.2006.08.022
Karmazyn, M., Kiliæ, A., and Javadov, S. (2008). The role of NHE-1 in myocardial hypertrophy and remodelling. J. Mol. Cell Cardiol. 44, 647–653. doi: 10.1016/j.yjmcc.2008.01.005
Khimji, A. K., and Rockey, D. C. (2010). Endothelin–biology and disease. Cell Signal. 22, 1615–1625. doi: 10.1016/j.cellsig.2010.05.002
Lazzerini, P. E., Capecchi, P. L., and Laghi-Pasini, F. (2017). Systemic inflammation and arrhythmic risk: lessons from rheumatoid arthritis. Eur. Heart J. 38, 1717–1727. doi: 10.1093/eurheartj/ehw208
Li, F. (2018). Effect of Wenxin granules on incidental atrial premature and lipid metabolism. Chin. J. Mod. Drug Appl. 12, 90–91.
Li, M., Qiu, R., Tian, G., Zhang, X., Li, C., Chen, S., et al. (2017a). Wenxin keli for ventricular premature complexes with heart failure: a systematic review and meta-analysis of randomized clinical trials. Complement. Ther. Med. 33, 85–93. doi: 10.1016/j.ctim.2017.06.006
Li, M., Xu, X., Yang, X., Kwong, J. S. W., and Shang, H. (2017b). The cardioprotective and antiarrhythmic effects of Nardostachys chinensis in animal and cell experiments. BMC Complement. Altern. Med. 17:398. doi: 10.1186/s12906-017-1910-1
Li, X. W., Huang, C. X., Fan, X. R., Chen, Y. J., Tang, Y. H., Wang, X., et al. (2011). Effects of wenxin particle on electrophysiological properties of human HCN2 channel. Chin. J. Cardiac Pacing Electrophysiol. 25, 249–252. doi: 10.13333/j.cnki.cjcpe.2011.03.037
Lian, Z. M., Quan, L., Liu, M. X., Wang, X., Wang, P., Hu, T., et al. (2014). The effects of wenxin keli on sarcoplasmic reticulum Ca 2 + ATPase in rabbits with heart failure. Chin. J. Cardiac Pacing Electrophysiol. 28, 527–531. doi: 10.13333/j.cnki.cjcpe.2014.06.012
Liang, J. J., Shi, S. B., Shen, J. J., Wang, F., Shen, Y. Y., Shi, X. R., et al. (2012). Influence of Wenxin particle on cardiac electrophysiology in depressed rats after myocardial infarction. Chin. J. Cardiovasc. Rehabil. Med. 21, 649–655. doi: 10.3969/j.issn.1008-0074.2012.06.29
Liu, A. D., Dai, N., and Wang, X. H. (2007a). Effect of Wenxinkeli on endothelin and myocardium cell structure of congestive heart failure rats. Chin. Heart J. 19, 424–427. doi: 10.3969/j.issn.1672-1349.2006.12.021
Liu, A. D., Wang, X. H., and Dai, N. (2007b). Effect of Wenxin Granule on hemodynamics and angiotensin II in rats with chronic heart failure. J. Tradit. Chin. Med. 48:31. doi: 10.3321/j.issn:1001-1668.2007.01.053
Liu, M. X., Quan, L., Lian, Z. M., Zhang, D., Wang, X., Wang, T., et al. (2012). Influence of Wenxin Keli on ventricular electrophysiology in rabbits with congestive heart failure. Chin. J. Cardiac Pacing Electrophysiol. 26, 543–546. doi: 10.13333/j.cnki.cjcpe.2012.06.028
Liu, M. X., Tang, Y. H., Lian, Z. M., Zhang, D., Wang, X., et al. (2011). Effect of Wenxin Keli on sinusatrial node function and atrial electrophysiology in rabbits with congestive heart failure. Chin. J. Cardiac Pac. Electrophysiol. 25, 540–542. doi: 10.13333/j.cnki.cjcpe.2011.06.006
Long, J. R., Deng, B., Liu, S. Q., Tang, F., Tan, W. T., Liu, M. J., et al. (2017). Effects of Wenxin granules on cardiac hypertrophy and the expression of connexin43 in rats. China Med. Herald 14, 8–11.
Luo, A., Liu, Z., Cao, Z., Hao, J., Wu, L., Fu, C., et al. (2017). Wenxin Keli diminishes Ca2+ overload induced by hypoxia/ reoxygenation in cardiomyocytes through inhibiting INaL and ICaL. Pacing Clin. Electrophysiol. 40, 1412–1425. doi: 10.1111/pace.13206
Mehra, R. (2007). Global public health problem of sudden cardiac death. J. Electrocardiol. 40, S118–S122. doi: 10.1016/j.jelectrocard.2007.06.023
Minoura, Y., Panama, B. K., Nesterenko, V. V., Betzenhauser, M., Barajas-Martínez, H., Hu, D., et al. (2013). Effect of Wenxin Keli and quinidine to suppress arrhythmogenesis in an experimental model of Brugada syndrome. Heart Rhythm. 10, 1054–1062. doi: 10.1016/j.hrthm.2013.03.011
Mohammed, S. F., Hussain, S., Mirzoyev, S. A., Edwards, W. D., Maleszewski, J. J., and Redfield, M. M. (2015). Coronary microvascular rarefaction and myocardial fibrosis in heart failure with preserved ejection fraction. Circulation 131, 550–559. doi: 10.1161/CIRCULATIONAHA.114.009625
Nattel, S., Andrade, J., Macle, L., Rivard, L., Dyrda, K., Mondesert, B., et al. (2014). New directions in cardiac arrhythmia management: present challenges and future solutions. Can. J. Cardiol. 30, S420–S430. doi: 10.1016/j.cjca.2014.09.027
Nguyen, M. N., Kiriazis, H., Gao, X. M., and Du, X. J. (2017). Cardiac fibrosis and arrhythmogenesis. Compr. Physiol. 7, 1009–1049. doi: 10.1002/cphy.c160046
Ohira, H., Tsutsui, W., and Fujioka, Y. (2017). Are short chain fatty acids in gut microbiota defensive players for inflammation and atherosclerosis? J. Atheroscler. Thromb. 24, 660–672. doi: 10.5551/jat.RV17006
Ono, K., and Iijima, T. (2010). Cardiac T-type Ca (2+) channels in the heart. J. Mol. Cell Cardiol. 48, 65–70. doi: 10.1016/j.yjmcc.2009.08.021
Perrin, M. J., Adler, A., Green, S., Al-Zoughool, F., Doroshenko, P., Orr, N., et al. (2014). Evaluation of genes encoding for the transient outward current (Ito) identifies the KCND2 gene as a cause of J-wave syndrome associated with sudden cardiac death. Circ. Cardiovasc. Genet. 7, 782–789. doi: 10.1161/CIRCGENETICS.114.000623
Pharmacopoeia. (2015). The Pharmacopoeia of the People’s Republic of China, Vol. 1. Beijing: China Medical Science Press, 1681–1683.
Pieperhoff, S., and Franke, W. W. (2007). The area composita of adhering junctions connecting heart muscle cells of vertebrates – IV: coalescence and amalgamation of desmosomal and adhaerens junction components-late processes in mammalian heart development. Eur. J. Cell Biol. 86, 377–391. doi: 10.1016/j.ejcb.2007.04.001
Remme, C. A., and Wilde, A. A. (2014). Targeting sodium channels in cardiac arrhythmia. Curr. Opin. Pharmacol. 15, 53–60. doi: 10.1016/j.coph.2013.11.014
Ren, X. M., Chen, Y., Li, J., Wu, L. J., Yang, X. Y., Wu, A. M., et al. (2016). The influence of Wenxin granule on the cytoskeleton Protein in Ang II induced H9C2 cell hypertrophy model. Chin. J. Integr. Med. Cardio/Cerebrovasc. Dis. 14, 2609–2612. doi: 10.3969/j.issn.1672-1349.2016.22.006
Rosenberg, R. L., Hess, P., and Tsien, R. W. (1988). Cardiac calcium channels in planar lipid bilayers. L-type channels and calcium-permeable channels open at negative membrane potentials. J. Gen. Physiol. 92, 27–54. doi: 10.1085/jgp.92.1.27
Roth, G. A., Forouzanfar, M. H., Moran, A. E., Barber, R., Nguyen, G., Feigin, V. L., et al. (2015). Demographic and epidemiologic drivers of global cardiovascular mortality. N. Engl. J. Med. 372, 1333–1341. doi: 10.1056/NEJMoa1406656
Santoro, B., and Tibbs, G. R. (1999). The HCN gene family: molecular basis of the hyperpolarization- activated pacemaker channels. Ann. N. Y. Acad. Sci. 868, 741–764. doi: 10.1111/j.1749-6632.1999.tb11353.x
Santos-Gallego, C. G., Picatoste, B., and Badimón, J. J. (2014). Pathophysiology of acute coronary syndrome. Curr. Atheroscler. Rep. 16:401. doi: 10.1007/s11883-014-0401-9
Severs, N. J., Bruce, A. F., Dupont, E., and Rothery, S. (2008). Remodelling of gap junctions and connexin expression in diseased myocardium. Cardiovasc. Res. 80, 9–19. doi: 10.1093/cvr/cvn133
Shah, A. M. (2013). Ventricular remodeling in heart failure with preserved ejection fraction. Curr. Heart Fail. Rep. 10, 341–349. doi: 10.1007/s11897-013-0166-4
Shryock, J. C., Song, Y., Rajamani, S., Antzelevitch, C., and Belardinelli, L. (2013). The arrhythmogenic consequences of increasing late INa in the cardiomyocyte. Cardiovasc. Res. 99, 600–611. doi: 10.1093/cvr/cvt145
Sieira, J., and Brugada, P. (2017). The definition of the Brugada syndrome. Eur. Heart J. 38, 3029–3034. doi: 10.1093/eurheartj/ehx490
Stansfield, W. E., Ranek, M., Pendse, A., Schisler, J. C., Wang, S., Pulinilkunnil, T., et al. (2014). “The pathophysiology of cardiac hypertrophy and heart failure,” in Cellular and Molecular Pathobiology of Cardiovascular Disease, eds M. S. Willis, J. W. Homeister, and J. R. Stone (Amsterdam: Elsevier Inc.), 51–78. doi: 10.1016/B978-0-12-405206-2.00004-1
Tao, Y. K., Zeng, H., Zhang, G. Q., Chen, S. T., Xie, X. J., He, X., et al. (2017). Notch3 deficiency impairs coronary microvascular maturation and reduces cardiac recovery after myocardial ischemia. Int. J. Cardiol. 236, 413–422. doi: 10.1016/j.ijcard.2017.01.096
Tse, G. (2016). Mechanisms of cardiac arrhythmias. J. Arrhythm. 32, 75–81. doi: 10.1016/j.joa.2015.11.003
Valko, M., Jomova, K., Rhodes, C. J., Kuča, K., and Musílek, K. (2016). Redox- and non-redox-metal-induced formation of free radicals and their role in human disease. Arch. Toxicol. 90, 1–37. doi: 10.1007/s00204-015-1579-5
Vaughan-Williams, E. M. (1977). Research on a rational basis for the origin and treatment of arrhythmia. Acta Cardiol. Suppl. 22, 13–32.
Vincent, K. P., McCulloch, A. D., and Edwards, A. G. (2014). Toward a hierarchy of mechanisms in CaMKII-mediated arrhythmia. Front. Pharmacol. 5:110. doi: 10.3389/fphar.2014.00110
Wang, D., and Liu, Q. (2014). Effect of wenxin granule on cardiomyocytes in rats with myocardial ischemia/reperfusion injury. Chin. J. Gerontol. 34, 2820–2821. doi: 10.3969/j.issn.1005-9202.2014.10.097
Wang, D., Liu, Y. P., and Liu, Q. (2014). Effect of wenxin granule on coronary endothelial cells in rats with myocardial ischemia/reperfusion injury. Chin. J. Gerontol. 34, 3700–3701. doi: 10.3969/j.issn.1005-9202.2014.13.089
Wang, G. Q., Jin, W., Xi, M. Y., Peng, Z. H., Chen, Q., Dai, G., et al. (2011a). Effect and mechanism of wenxin granule on cardiac hypertrophy in rats. Chin. J. Integr. Med. Cardio/Cerebrovasc. Dis. 9, 462–463. doi: 10.3969/j.issn.1672-1349.2011.04.044
Wang, T., Lu, M., Du, Q., Yao, X., Zhang, P., Chen, X., et al. (2017). An integrated anti-arrhythmic target network of a Chinese medicine compound, Wenxin Keli, revealed by combined machine learning and molecular pathway analysis. Mol. Biosyst. 13, 1018–1030. doi: 10.1039/c7mb00003k
Wang, X., Wang, X., Gu, Y., Wang, T., and Huang, C. (2013). Wenxin Keli attenuates ischemia-induced ventricular arrhythmias in rats: involvement of L-type calcium and transient outward potassium currents. Mol. Med. Rep. 7, 519–524. doi: 10.3892/mmr.2012.1195
Wang, X., Wang, X., Tang, Y. H., Wang, T., Xu, C., and Huang, C. X. (2010). Effects of wenxin granule on transient outward potassium current in acute isolated ventricular myocytes of adult rats. Herald Med. 29, 1539–1542. doi: 10.3870/yydb.2010.12.002
Wang, X., Wang, X., Tang, Y. H., Wang, T., Xu, C., and Huang, C. X. (2011b). Effects of wenxin granule on L-type calcium current in ventricular myocytes of rat. South China J. Cardiovasc. Dis. 17, 60–63. doi: 10.3969/j.issn.1007-9688.2011.01.019
Wang, X., Wang, Y., Feng, X., Lu, Y., Zhang, Y., Wang, W., et al. (2016). Systematic review and meta-analysis of randomized controlled trials on Wenxin keli. Drug Des. Dev. Ther. 10, 3725–3736. doi: 10.2147/DDDT.S112333
WHO (2017). Cardiovascular Diseases (CVDs). Geneva: World Health Organization. Available at: http://www.who.int/news-room/fact-sheets/detail/cardiovascular-diseases-(cvds)
Wu, A., Lou, L., Zhai, J., Zhang, D., Chai, L., Nie, B., et al. (2017a). miRNA expression profile and effect of wenxin granule in rats with ligation-induced myocardial infarction. Int. J. Genomics 2017:2175871. doi: 10.1155/2017/2175871
Wu, A., Zhao, M., Lou, L., Zhai, J., Zhang, D., Zhu, H., et al. (2017b). Effect of wenxin granules on gap junction and MiR-1 in rats with myocardial infarction. Biomed. Res. Int. 2017:3495021. doi: 10.1155/2017/3495021
Wu, Y. T., Chen, Y., Liu, H. T., Wu, Y. Y., Wu, W., and Liu, X. C. (2017c). Effects of shensong yangxin capsule and wenxin granule on myocardial fibrosis in rat and its antiarrhythmic mechanism. Chin. J. Integr. Med. Cardio/Cerebrovasc. Dis. 15, 924–927.
Wu, A., Zhai, J., Zhang, D., Lou, L., Zhu, H., Gao, Y., et al. (2013). Effect of wenxin granule on ventricular remodeling and myocardial apoptosis in rats with myocardial infarction. Evid. Based Complement. Alternat. Med. 2013:967986. doi: 10.1155/2013/967986
Xiang, J. P., Wang, X. E., and Zhang, F. G. (2015). Effect of wenxin granule on myocardial ischemic disease in rats. Chin. J. Integr. Med. Cardio/Cerebrovasc. Dis. 13, 1728–1729. doi: 10.3969/j.issn.1672-1349.2015.15.011
Xie, M., Burchfield, J. S., and Hill, J. A. (2013). Pathological ventricular remodeling: therapies: part 2 of 2. Circulation 128, 1021–1030. doi: 10.1161/CIRCULATIONAHA.113.001879
Xing, Y., Gao, Y., Chen, J., Zhu, H., Wu, A., Yang, Q., et al. (2013). Wenxin-keli regulates the calcium/Calmodulin-dependent protein kinase II signal transduction pathway and inhibits cardiac arrhythmia in rats with myocardial infarction. Evid. Based Complement. Alternat. Med. 2013:464508. doi: 10.1155/2013/464508
Xu, T., Chen, L. F., Li, F. J., and Fan, L. (2010). The electrophysiological effects of wenxin granule off arrhythmia induced by isoprenaline in guinea pig. Chin. J. Integr. Med. Cardio/Cerebrovasc. Dis. 8, 308–309. doi: 10.3969/j.issn.1672-1349.2010.03.033
Xue, X., Guo, D., Sun, H., Wang, D., Li, J., Liu, T., et al. (2013). Wenxin Keli suppresses ventricular triggered arrhythmias via selective inhibition of late sodium current. Pacing Clin. Electrophysiol. 36, 732–740. doi: 10.1111/pace.12109
Yamaoka, K., and Kameyama, M. (2003). Regulation of L-type Ca2+ channels in the heart: overview of recent advances. Mol. Cell Biochem. 253, 3–13. doi: 10.1023/A:1026036931170
Yang, J., Deng, B., Ding, S. L., Wang, G. H., Zhang, Y., Ding, S. Y., et al. (2012). Effects of wenxin granule on noradrenaline- induced H9C2 proliferation and Cx43 mRNA expression in cardiomyocytes. Shandong Med. J. 52, 31–33.
Yang, X., Chen, Y., Li, Y., Ren, X., Xing, Y., and Shang, H. (2017a). Effects of wenxin keli on cardiac hypertrophy and arrhythmia via regulation of the calcium/calmodulin dependent kinase II signaling pathway. Biomed. Res. Int. 2017:1569235. doi: 10.1155/2017/1569235
Yang, X., Li, Y., Li, Y., Ren, X., Zhang, X., Hu, D., et al. (2017b). Oxidative stress-mediated atherosclerosis: mechanisms and therapies. Front. Physiol. 8:600. doi: 10.3389/fphys.2017.00600
Yano, M., and Okuda, S. (2014). Does a ripple of Ca (2+) leak develop into a rogue wave that can trigger pathological hypertrophy? J. Am. Coll. Cardiol. 63, 1580–1582. doi: 10.1016/j.jacc.2013.10.064
Zaza, A., and Rocchetti, M. (2013). The late Na+ current–origin and pathophysiological relevance. Cardiovasc. Drugs. Ther. 27, 61–68. doi: 10.1007/s10557-012-6430-0
Zeng, X. H., Zhou, R. L., Jin, L. L., Chen, Y. X., and Wang, Q. H. (2006). The effect of buchangwenxin granule on arrhythemias in a mouse model of ischemia-reperfusion injury. J. Clin. Cardiol. 22, 742–743. doi: 10.3969/j.issn.1001-1439.2006.12.013
Zhang, C., Shi, D., and Chen, Y. (2012). Protective effect of wenxin granule on myocardial injury and immune function damage caused by epirubicin in dogs. China Pract. Med. 7, 257–259. doi: 10.3969/j.issn.1673-7555.2012.12.202
Zhao, X., Hu, Y., Wang, D., Liu, J., and Guo, L. (2013). The comparison of immune-enhancing activity of sulfated polysaccharidses from Tremella and Condonpsis pilosula. Carbohydr. Polym. 98, 438–443. doi: 10.1016/j.carbpol.2013.06.043
Zheng, M., Liu, Z., Liu, N., Hou, C., Pu, J., and Zhang, S. (2016). The effect of wenxin keli on the mRNA expression profile of rabbits with myocardial infarction. Evid. Based Complement. Alternat. Med. 2016:2352614. doi: 10.1155/2016/7893710
Zhou, C. S., and Sun, X. X. (2015). Cardioprotective effects of wenxin granules on myocardial ischemia/reperfusion injury in rabbits. Pract. Pharm. Clin. Remed. 18, 901–903. doi: 10.14053/j.cnki.ppcr.201508006
Zhou, F., Hu, S. J., and Mou, Y. (2007). Effect of wenxin granule on isoproterenol induced cardiac dysfunction in rats. China J. Chin. Mater. Med. 32, 1676–1679. doi: 10.3321/j.issn:1001-5302.2007.16.019
Zhu, H. J., Lu, S., Su, W., Gong, S. Y., Zhang, Z. B., Li, P., et al. (2011). Effects of liandou qingmai recipe on endothelin-1, nitric oxide, interleukin-6 and interleukin-10 levels in patients with coronary heart disease. J. Tradit. Chin. Med. 31, 173–177. doi: 10.1016/S0254-6272(11)60035-6
Zicha, S., Fernández-Velasco, M., Lonardo, G., L’Heureux, N., and Nattel, S. (2005). Sinus node dysfunction and hyperpolarization-activated (HCN) channel subunit remodeling in a canine heart failure model. Cardiovasc. Res. 66, 472–481. doi: 10.1016/j.cardiores.2005.02.011
Keywords: Wenxin Keli, WXKL, cardioprotective effects, antiarrhythmic effects, mechanism
Citation: Tian G, Sun Y, Liu S, Li C, Chen S, Qiu R, Zhang X, Li Y, Li M and Shang H (2018) Therapeutic Effects of Wenxin Keli in Cardiovascular Diseases: An Experimental and Mechanism Overview. Front. Pharmacol. 9:1005. doi: 10.3389/fphar.2018.01005
Received: 06 June 2018; Accepted: 16 August 2018;
Published: 05 September 2018.
Edited by:
Min Ye, Peking University, ChinaCopyright © 2018 Tian, Sun, Liu, Li, Chen, Qiu, Zhang, Li, Li and Shang. This is an open-access article distributed under the terms of the Creative Commons Attribution License (CC BY). The use, distribution or reproduction in other forums is permitted, provided the original author(s) and the copyright owner(s) are credited and that the original publication in this journal is cited, in accordance with accepted academic practice. No use, distribution or reproduction is permitted which does not comply with these terms.
*Correspondence: Youping Li, eXpteWxhYkBob3RtYWlsLmNvbQ== Min Li, bGltaW45MDMwQDE2My5jb20= Hongcai Shang, c2hhbmdob25nY2FpQDEyNi5jb20=
†These authors have contributed equally to this work