- 1Department of Cardiology, University of Heidelberg, Heidelberg, Germany
- 2DZHK (German Centre for Cardiovascular Research), partner site Heidelberg/Mannheim, University of Heidelberg, Heidelberg, Germany
- 3HCR, Heidelberg Centre for Heart Rhythm Disorders, University of Heidelberg, Heidelberg, Germany
Background: Atrial fibrillation (AF) is the most common sustained cardiac arrhythmia and one of the major causes of cardiovascular morbidity and mortality. Despite good progress within the past years, safe and effective treatment of AF remains an unmet clinical need. The anti-anginal agent ranolazine has been shown to exhibit antiarrhythmic properties via mainly late INa and IKr blockade. This results in prolongation of the atrial action potential duration (APD) and effective refractory period (ERP) with lower effect on ventricular electrophysiology. Furthermore, ranolazine has been shown to be effective in the treatment of AF. TASK-1 is a two-pore domain potassium (K2P) channel that shows nearly atrial specific expression within the human heart and has been found to be upregulated in AF, resulting in shortening the atrial APD in patients suffering from AF. We hypothesized that inhibition TASK-1 contributes to the observed electrophysiological and clinical effects of ranolazine.
Methods: We used Xenopus laevis oocytes and CHO-cells as heterologous expression systems for the study of TASK-1 inhibition by ranolazine and molecular drug docking simulations to investigate the ranolazine binding site and binding characteristics.
Results: Ranolazine acts as an inhibitor of TASK-1 potassium channels that inhibits TASK-1 currents with an IC50 of 30.6 ± 3.7 µM in mammalian cells and 198.4 ± 1.1 µM in X. laevis oocytes. TASK-1 inhibition by ranolazine is not frequency dependent but shows voltage dependency with a higher inhibitory potency at more depolarized membrane potentials. Ranolazine binds within the central cavity of the TASK-1 inner pore, at the bottom of the selectivity filter.
Conclusions: In this study, we show that ranolazine inhibits TASK-1 channels. We suggest that inhibition of TASK-1 may contribute to the observed antiarrhythmic effects of Ranolazine. This puts forward ranolazine as a prototype drug for the treatment of atrial arrhythmia because of its combined efficacy on atrial electrophysiology and lower risk for ventricular side effects.
Introduction
Atrial fibrillation (AF) is a common cardiac rhythm disorder and one of the major causes of stroke, acute heart failure, sudden death, and cardiovascular morbidity (January et al., 2014). Despite good progress within the last years, safe and effective management of patients suffering from AF remains a major health issue, as current pharmacological, interventional or surgical therapeutic strategies are restricted by insufficient efficacy and often severe adverse effects (Kirchhof et al., 2016). Annual recurrence rates of AF after pharmacological cardioversion, for instance, range from 40 to 70% (Guerra et al., 2017). Furthermore, drug treatment with antiarrhythmic drugs (AADs) is often discontinued because of poor tolerability or adverse effects (Lafuente-Lafuente et al., 2015). For this reason, the development of safe and effective AADs for the treatment of AF is crucial (Dobrev and Nattel 2010).
Ranolazine is a drug originally introduced as an anti-anginal agent (Nash and Nash 2008) that has later been shown to exhibit antiarrhythmic properties via inhibition of different ion currents, especially the late phase of the inward sodium current (late INa) and the rapidly activating delayed rectifier potassium current (IKr) (Gupta et al., 2015). Because ranolazine predominantly prolongs atrial rather than ventricular action potential duration (APD) and effective refractory period (ERP) (Burashnikov et al., 2007; Antzelevitch and Burashnikov 2009), it appears to be particularly effective in AF (Guerra et al., 2017), thus far attributed to an atrial-selective sodium channel block (Sossalla et al., 2010; Antzelevitch et al., 2011).
TASK-1 (tandem of P domains in a weak inward rectifying K+ channel (TWIK)-related acid sensitive K+ channel 1; K2P3.1) is a member of the two-pore-domain potassium channel (K2P) family. This heterologous group comprises 15 members that share a unique structure of four transmembrane domains and two pore-forming loops per subunit which assemble as dimers (Goldstein et al., 2001). Regulated by a variety of physiological stimuli (extracellular pH, G-protein-mediated pathways, polyunsaturated fatty acids, temperature and mechanical stress) they provide a background “leak” potassium conductance modulating the cell’s resting membrane potential and cellular excitability (Feliciangeli et al., 2015). Their role in controlling cellular excitability predestines K2P channels as potential players in diverse biological functions.
TASK-1 channels are widely expressed in various tissues, including the cerebral cortex (Vu et al., 2015), the brainstem retrotrapezoid (Mulkey et al., 2007) and pre-Botzinger regions (Koizumi et al., 2010), the carotid bodies (Buckler et al., 2000), hypoglossal and spinal cord motor neurons (Lazarenko et al., 2010), pulmonary artery smooth muscle (Olschewski et al., 2006), and the adrenal cortex (Czirjak and Enyedi 2002). They contribute in the regulation of oxygen sensing (Koizumi et al., 2010), endocrine secretion (Davies et al., 2008), auto-immune inflammation (Bittner et al., 2009), apoptosis (Lauritzen et al., 2003), and pulmonary blood pressure (Olschewski et al., 2006).
In the heart, TASK-1 is reported to modulate cardiac conduction, repolarization, and heart rate (Decher et al., 2011; Donner et al., 2011). Knockout or pharmacological inhibition of TASK-1 results in prolonged atrial APD and atrial ERP (Wirth et al., 2003; Putzke et al., 2007; Wirth et al., 2007; Decher et al., 2011; Skarsfeldt et al., 2016). Please note that some of the mentioned studies used inhibitors that are referred to as KV1.5 blockers (AVE0118 and AVE1231 (A293), developed by Sanofi, Paris, France). These inhibitors, however, later turned out to be much more potent TASK-1 blockers (Kiper et al., 2015). Wirth et al. (2007) demonstrated that TASK-1 blockade induced a prolongation of only atrial but not ventricular refractoriness and an associated inhibition of atrial vulnerability to arrhythmia. The prolongation of atrial refractoriness was even more pronounced in tachypacing induced AF and there were no effects on ECG intervals and ventricular repolarization. Within the human heart, TASK-1 has recently been shown to be predominantly expressed in the atrium as well, and TASK-1 inhibition results in prolonged APD on isolated human atrial cardiomyocytes (Schmidt et al., 2015). Because of enhanced TASK-1 currents under the condition of AF, the effect is even more pronounced and thus similar to the results obtained from large animal models. APD prolongation via TASK-1 blockade is expected to suppress AF and the ‘atrial selectivity’ of TASK-1 blockade by limiting the mode of action to atrial tissue, thereby reducing the risk of pro-arrhythmogenic effects in the ventricles, highlights the potential clinical significance of TASK-1 blockade for the treatment of AF in patients (Schmidt et al., 2017).
We hypothesized that ranolazine inhibits TASK-1 currents and that TASK-1 inhibition contributes to the observed antiarrhythmic effects of ranolazine. We chose Xenopus laevis oocytes and Chinese Hamster Ovary (CHO) cells as heterologous expression systems for detailed study of the biophysical characteristics of TASK-1 blockade by ranolazine. We further used in silico docking simulations and mutagenesis screen to explore structural determinants of TASK-1 blockade.
Materials and Methods
Molecular Biology
Complementary DNAs encoding human TWIK-1 (KCNK1; GenBank accession number NM_002245), TREK-1 (KCNK2; EF165334), TASK-1 (KCNK3; NM_002246), and TASK-3 (KCNK9; NM_016601) were kindly provided by Steve Goldstein (Chicago, IL, USA). Human TRESK cDNA (KCNK18; NM_181840) was obtained from C. Spencer Yost (San Francisco, CA, USA). Amplification of human TRAAK (KCNK4; EU978935), TASK-2 (KCNK5; EU978936), TWIK-2 (KCNK6; EU978937), TREK-2 (KCNK10; EU978939), THIK-1 (KCNK13; EU978942), TALK-1 (KCNK16; EU978943), and TALK-2 (KCNK17; EU978944) was previously described (Gierten et al., 2008). For in vitro transcription, cDNAs were subcloned into pRAT, a dual-purpose expression vector containing a cytomegalovirus promotor for mammalian expression and a T7 promotor for copy (c)RNA synthesis. All TASK-1 mutants reported in this study were generated using the QuikChange II Site-Directed Mutagenesis Kit (Agilent, Santa Clara, CA, USA) and synthetic mutant oligonucleotide primers. Sequences of all plasmid constructs were verified by DNA sequencing (GATC Biotech, Konstanz, Germany). After vector linearization with XbaI (New England Biolabs, Ipswich, MA, USA), plasmids were transcribed using the T7 mMessage mMachine kit (Thermo Fisher Scientific Inc., Waltham, MA, USA). Integrity of cRNA transcripts was assessed by agarose gel electrophoresis and cRNA concentrations were determined using Nanodrop spectrophotometry (ND-1000, peqLab Biotechnology GmbH, Erlangen, Germany).
Cell Culture
Chinese hamster ovary (CHO) cells (CLS Cat# 603479/p746_CHO, RRID : CVCL_0213) were cultured in Dulbecco’s modified Eagle’s medium (DMEM, Thermo Fisher Scientific Inc., Waltham, MA, USA) supplemented with 10% fetal bovine serum (FBS, Thermo Fisher Scientific Inc., Waltham, MA, USA), 100 U/ml penicillin G sodium and 100 µg/ml streptomycin sulphate in an atmosphere of 95% humidified air and 5% CO2 at 37 °C. Cells were passaged regularly and seeded on glass cover slips prior to treatment. Transient transfections of CHO cells (passage 10–20) were performed using FuGENE HD transfection reagent (Promega, Madison, WI, USA) according to the manufacturer’s instructions. Cells were co-transfected with 2.2 µg pRAT-hTASK-1 plasmid DNA and 1.1 µg green fluorescent protein (GFP) plasmid DNA (pEGFP-N1; Clontech Laboratories, Mountain View, CA, USA) per 35 mm petri dish. Patch Clamp recordings were performed 24–36 h after transfection only on green fluorescent cells.
X. Laevis Oocyte Preparation
This study was carried out in accordance with the directive 2010/63/EU of the European Parliament, and the current version of the German Law on the Protection of Animals. Approval for experiments involving X. laevis was granted by Regierungspräsidium Karlsruhe (institutional approval numbers A-38/11 and G-221/12). For detailed information on oocyte preparation please refer to the supplementary methods.
Electrophysiology
Two-electrode voltage clamp (TEVC) recordings from X. laevis oocytes were performed one to three days after cRNA injection using an OC-725C Oocyte Clamp amplifier (Warner Instruments, Hamden, CT, USA), a Digidata 1322A Series (Axon Instruments, Foster City, CA, USA) and pClamp 10 software (Molecular Devices, San José, CA, USA). Current recordings from CHO cells were carried out using the whole-cell patch clamp technique with an Axopatch 200B amplifier (Axon Instruments, Foster City, CA, USA), an Axon Digidata 1550B series (Axon Instruments, Foster City, CA, USA), and pClamp 10 software (Molecular Devices, San José, CA, USA). For detailed information about solutions and test protocols please refer to the supplementary methods.
Molecular Modelling and In Silico Drug Docking
Because the crystal structure of the human TASK-1 channel was not revealed yet, we built homology models using the SWISS-MODEL platform (Benkert et al., 2011; Bertoni et al., 2017; Waterhouse et al., 2018). Four models were generated based on the structures of TWIK-1 (protein data bank (PDB) ID: 3UMK) (Miller and Long 2012), TREK-1 (PDB ID: 6CQ6) (Lolicato et al., 2017), TREK-2 (PDB ID: 4XDL) (Dong et al., 2015) and TRAAK (PDB ID: 4RUE) (Lolicato et al., 2014). Quality assessment of the newly generated TASK-1 homology models was performed using MolProbidity (Davis et al., 2007).
Molecular docking calculations were performed using AutoDock Vina (Trott and Olson 2010). Analysis of protein–ligand interactions was performed using PLIP (Salentin et al., 2015). Three-dimensional visualizations of in silico simulations and dockings were generated with PyMOL 1.8 (PyMOL Molecular Graphics System, Schrödinger, LLC, New York, NY, USA).
Data Analysis and Statistics
PCLAMP (Molecular Devices, San José, CA, USA), GraphPad Prism 6 (GraphPad Software Inc., La Jolla, CA, USA) and Microsoft Excel (Microsoft, Redmond, WA, USA) software was used for data acquisition and analysis. The concentration required for 50% block of current (half-maximal inhibitory concentration (IC50)) was calculated from Hill plots using Prism 6 (GraphPad). Data are expressed as the mean ± standard error of the mean (SEM) unless stated otherwise. Paired and unpaired t-tests (two-tailed tests) were applied to compare the statistical significance of the results. P < 0.05 was considered statistically significant. Multiple comparisons were performed using one-way analysis of variance (ANOVA). If the hypothesis of equal means could be rejected, post-hoc comparisons of groups were made and the probability values were adjusted for multiple comparisons using the Bonferroni correction.
Materials
Ranolazine-dihydrochloride was obtained from Selleck Chemicals (Munich, Germany) and dissolved in water to a 100 mM stock solution. Aliquots of the stock solution were stored at −20 °C and diluted to the desired concentration with the bath solution on the day of experiments. The dilution of Ranolazine did not affect the pH of the bath solution.
Results
Ranolazine Inhibits Human Task-1 Channels
To probe the inhibitory effects of ranolazine on human TASK-1 channels, TASK-1 was expressed in X. laevis oocytes. After a stabilization period with no significant current amplitude changes (15 min) ranolazine was administered for 30 min. Application of 100 µM ranolazine inhibited TASK-1 currents by 17.4 ± 2% (Figure 1A, n = 6, p = 0.018). Inhibitory effects were completely reversible. Current levels reached 100 ± 30% (n = 6) after 15 min washout period. TASK-1 channels were blocked with an IC50 of 30.6 ± 3.7 µM in CHO cells, and 198.4 ± 1.1 µM in oocytes, analyzed at +20 mV (Figures 1C, D). This 6.5-fold difference is consistent with previous findings that IC50 values determined in oocytes are mostly higher than those determined in mammalian cells (Streit et al., 2011; Schmidt et al., 2013). The maximum inhibition of 67.3 ± 4.5% in oocytes and 58.5 ± 6.9% in CHO cells was achieved with ranolazine concentrations of 1 mM and 100 µM, respectively. Administration of higher concentrations (3 mM in oocytes, 300 µM in CHO cells) led to cell instability and death. We used a concentration of 300 µM ranolazine for further experiments in X. laevis oocytes in order to achieve 50% current inhibition.
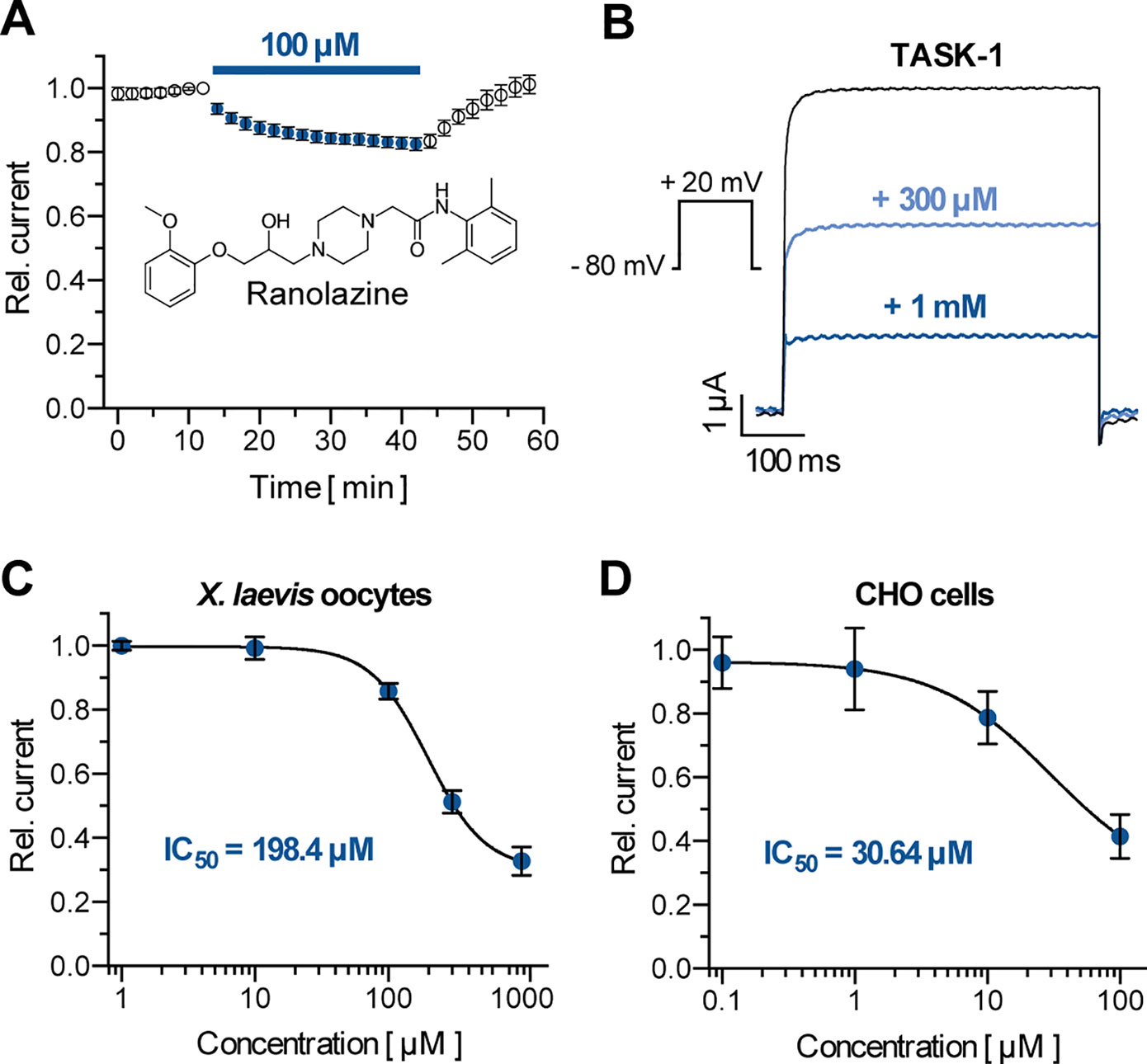
Figure 1 Effect of ranolazine on TASK-1 current. (A) Time course of TASK-1 current reduction during ranolazine application (n = 6); currents are normalized to their respective value before ranolazine application after a stabilization period with no significant amplitude changes. (B) Representative TASK-1 current recordings evoked by applying a test pulse from −80 mV to +20 mV under control conditions and after 30 min incubation with 300 µM and 1 mM ranolazine. (C) Dose–response curve of TASK-1 inhibition by ranolazine in X. laevis oocytes. Currents are normalized to their respective values under control conditions (n = 5 – 10). (D) Dose–response curve of TASK-1 inhibition by ranolazine in CHO cells. Currents are normalized to their respective values under control conditions (n = 5). Data are given as mean ± SEM.
Biophysical Characteristics of TASK-1 Channel Blockade by Ranolazine
TASK-1 channels show electrophysiologic characteristics that are typical for most K2P channels. They exhibit strong outward rectification with minimal inward current (0.69 ± 0.07 µA at −140 mV, n = 10) and a higher open probability at more depolarized membrane potentials (Figures 2A, C, D). Figure 2A illustrates representative current recordings of TASK-1 under control conditions and after application of 300 µM ranolazine for 30 min. Ranolazine (300 µM) inhibited TASK-1 currents by 48.79 ± 3.52% (analyzed at the end of the +20 mV test pulse, n = 10, p = 0.0004). Ranolazine also altered the resting membrane potential (RMP) from −66.57 ± 2.62 mV (control conditions, n = 10) to −63.55 ± 2.89 mV (300 µM ranolazine, n = 10, p = 0.0012, Figure 2B). Inhibitory effects of ranolazine showed voltage dependency, with less inhibition of inward currents (6.57 ± 2.55% to 11.07 ± 4.51% inhibition at voltages between −140 and −100 mV, n = 10) as compared to outward currents (44.56 ± 3.42% to 55.3 ± 3.87% inhibition at voltages between −40 and +60 mV, n = 10, Figure 2E). This resulted in an altered current-voltage (I-V) relationship under ranolazine treatment (Figure 2D).
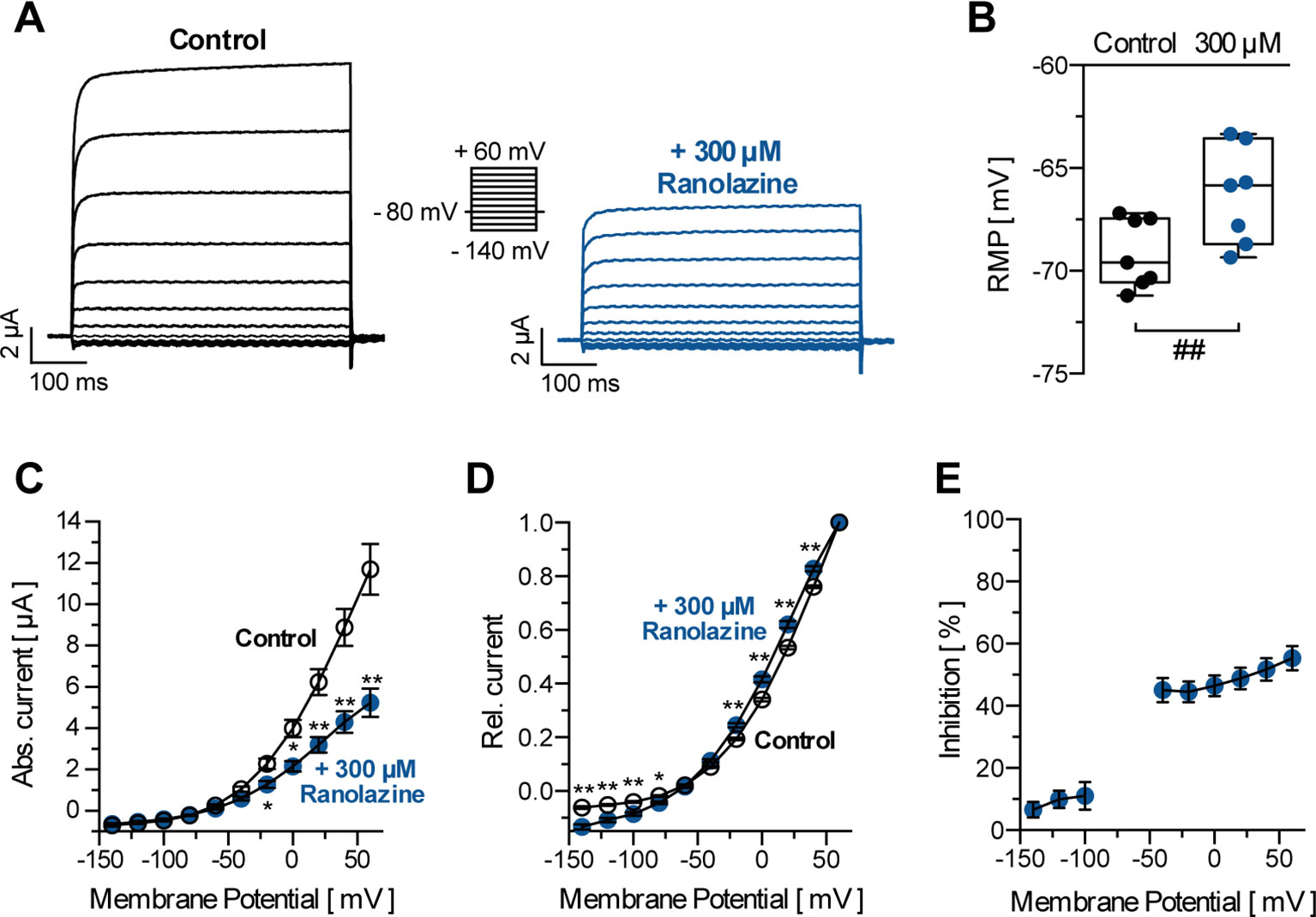
Figure 2 (A) Representative TASK-1 current recordings evoked by applying the displayed pulse protocol under control conditions and after 30 min incubation with ranolazine (300 µM). (B) Resting membrane potentials (RMP) in X. laevis oocytes before and after 30 min incubation with 300 µM ranolazine (n = 7). Boxes indicate the first and third quartile, whiskers indicate the minimum and maximum, and bands inside the boxes indicate the median; ##p < 0.01, paired t-test. (C) Activation curve of TASK-1 current; amplitudes are plotted against the respective test potential under control conditions and after 30 min incubation with ranolazine (n = 10); *p < 0.05, **p < 0.01, unpaired t-test vs. control conditions. (D) Amplitudes are normalized to the maximum current amplitude at +60 mV (n = 10); *p < 0.05, **p < 0.01, unpaired t-test vs. control conditions. (E) Dependency of TASK-1 current inhibition by ranolazine on membrane potential (n = 10). Data are given as mean ± SEM.
Macroscopic TASK-1 currents in heterologous expression systems activate in two phases. Currents activate quickly to approximately 85% of their respective maximum amplitude within the first 50 ms, followed by a markedly slower additional activation time course. Thus, TASK-1 currents may be divided into an instantaneous and a sustained current component. Figures 3A, B illustrate the inhibition of TASK-1 currents by ranolazine during a 7.5 s test pulse from −80 mV to +20 mV (n = 6). The maximum inhibition of 48.67 ± 4.45% was reached after 10 ms and remained unchanged over the 7.5 s test pulse. Additionally, TASK-1 inhibition by ranolazine did not show frequency dependency (Figure 3C). The slight difference between 0.1 Hz and 1 Hz may be attributed to higher cell instability at higher stimulation frequencies, as this difference was also observed under control conditions in absence of ranolazine.
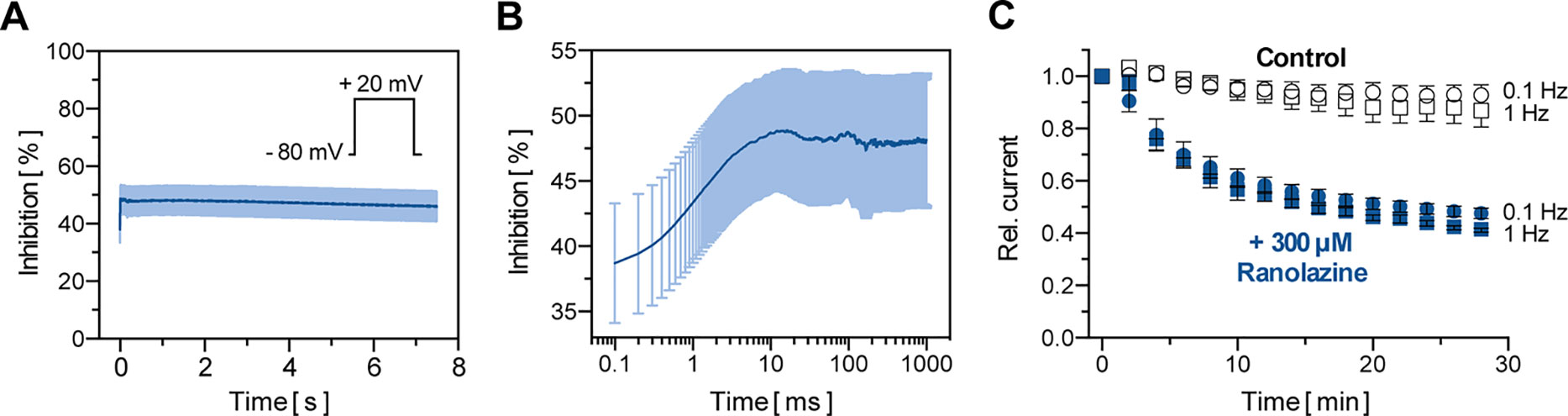
Figure 3 Biophysical properties of TASK-1 blockade by ranolazine. (A) The inhibition of TASK-1 current is displayed over a 7.5 s test pulse from −80 mV to +20 mV (n = 6). (B) Representation of the first 1000 ms of (A) on a logarithmic time scale (n = 6). (C) Frequency dependency of TASK-1 blockade by ranolazine; current amplitudes obtained during 30 min stimulation from −80 mV to +20 mV at stimulation rates of 0.1 Hz and 1 Hz are displayed under control conditions and during administration of 300 µM Ranolazine (n = 5–6). Currents are normalized to their respective values after a stabilization period. Data are given as mean ± SEM.
Effects of Ranolazine on Other Human Two-Pore Domain Potassium (K2P) Channels
We further tested inhibitory effects of ranolazine on other channels within the K2P channel family using a similar approach as reported in Figure 1A. Ranolazine (300 µM) was administered after a stabilization period with no significant current amplitude changes (15–20 min). Inhibition of ion currents was quantified after 30 min of ranolazine administration (Figure 4). Ranolazine showed small inhibitory effects on TREK-1 (7.35 ± 1.66%, n = 5, p = 0.026) and TRAAK (3.32 ± 1.29%, n = 5, p = 0.045), and more pronounced inhibition of TASK-2 (30.02 ± 6.42%, n = 5, p = 0.037), TALK-1 (23.04 ± 3.06%, n = 5, p = 0.0003), TALK-2 (34.88 ± 2.47%, n = 5, p = 0.024) and TASK-3 currents (28.28 ± 2.1%, n = 5, p = 0.028). Effects on TREK-2 and TRESK were not significant. Ranolazine treatment of THIK-1 lead to slightly enhanced currents (+4.98 ± 0.66%, n = 5, p = 0.002). Taken together, ranolazine favorably inhibits K2P channels that are acid sensitive (TASK-1, TASK-2, TASK-3) or alkaline sensitive (TALK-1, TALK-2).
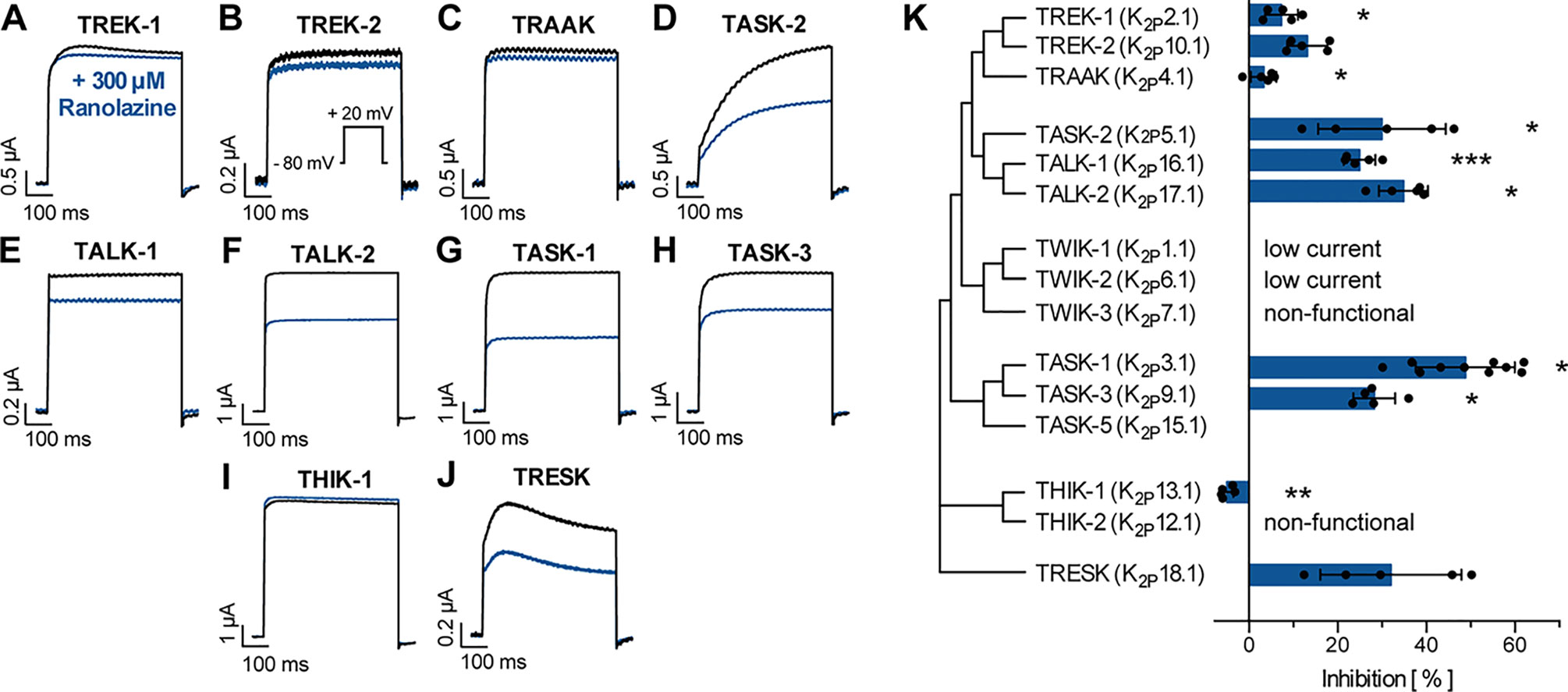
Figure 4 Effect of ranolazine on different members of the K2P channel family. (A–J) Representative current recordings evoked by a test pulse from −80 mV to +20 mV under control conditions and after 30 min incubation ranolazine. (K) Current inhibition by 300 µM ranolazine is displayed for the different K2P channel family members. Data are given as mean ± SEM. *p < 0.05, **p < 0.01, ***p < 0.001, paired t-test vs. control measurements.
Protein–Ligand Interaction Profile of Ranolazine in the TASK-1 Inner Pore
TASK-1 potassium channels contain four ion binding positions (S1–S4) within the selectivity filter. For in silico docking calculations potassium ions were either positioned at S1 and S3 or at S2 and S4 (Figures 5A, B). Ten ranked docking poses were calculated for each configuration. The amount and character of the protein–ligand interactions of all docking poses is summarized in Figure 5C and displayed separately for the different ion binding configurations. Ranolazine is suggested to form hydrogen bonds with the threonine residues on position T93 and T199 and additional hydrophobic interactions with other pore lining residues. Different ion occupations within the selectivity filter only resulted in a slightly altered interaction profile with fewer hydrogen bonds formed by ranolazine and the threonine residues T93 and T199, and more hydrophobic interactions at position L232.
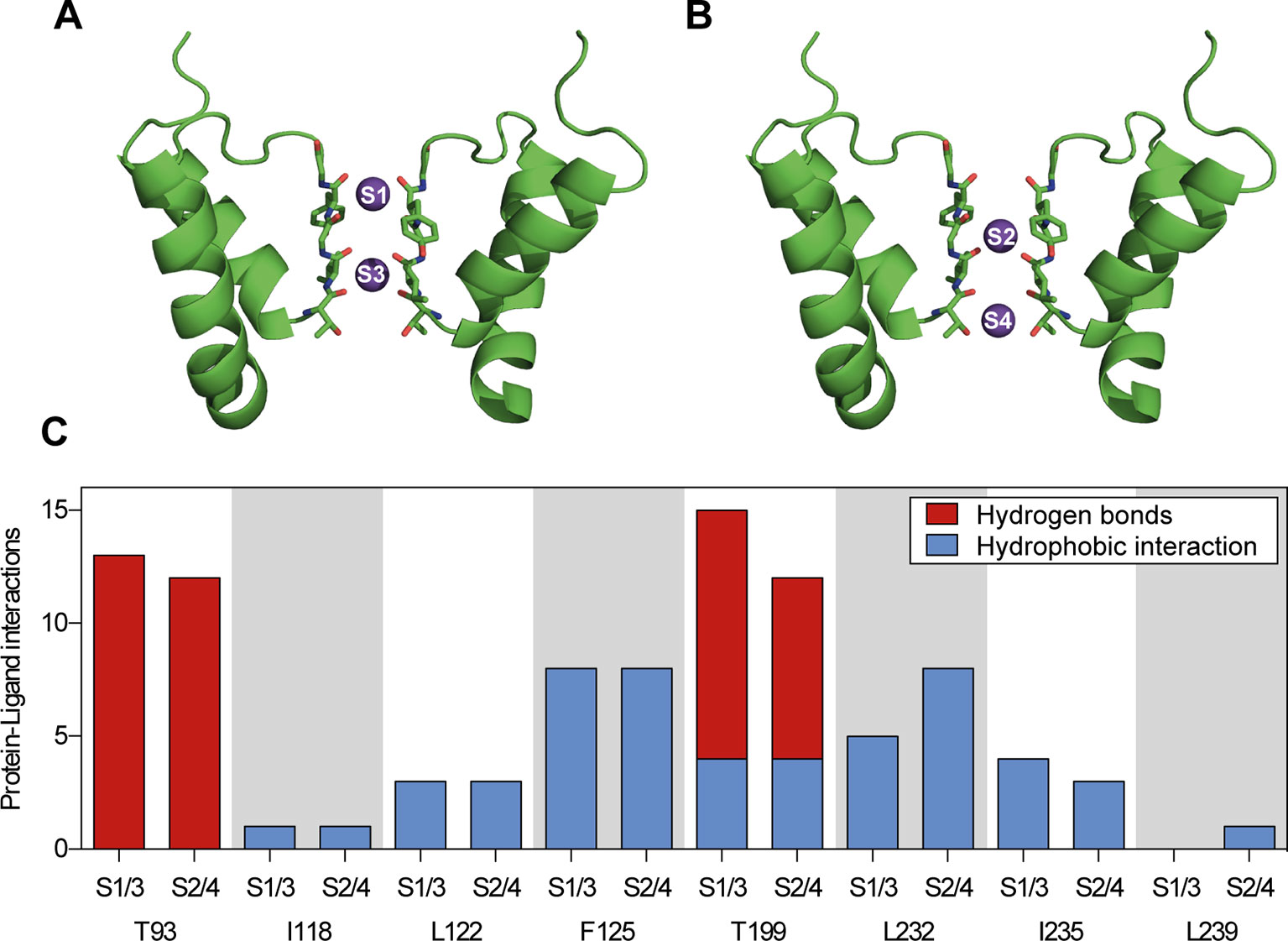
Figure 5 Summary of protein–ligand interactions of all calculated docking poses of ranolazine at the inner pore of the wild type (WT) TASK-1 model (T16cq6). (A, B) Docking calculations have been performed with potassium ions either at positions S1 and S3, or at positions S2 and S4. (C) Each calculation yielded 10 ranked docking poses, resulting in a total of 20 poses. Protein–ligand interactions have been analyzed using PLIP (Salentin et al., 2015). The amount and character of the interactions is displayed for the individual amino acid residues that interact with ranolazine.
Ranolazine Inhibition Depends on Amino Acid Residues Located at the Inner Pore of TASK-1
We further investigated the binding site of ranolazine within the TASK-1 inner pore, by individually mutating amino acids that were either predicted to contribute to ranolazine binding in molecular docking simulations, or have been identified as binding site for the high affinity blockers S20951 (A1899) and AVE1231 (A293) (Streit et al., 2011; Wiedmann et al., 2019). Variants T93A, I118A and T199A produced very low current amplitudes that did not allow reasonable pharmacology testing. Inhibitory effects of ranolazine on the functionally active TASK-1 mutants were quantified after 30 min ranolazine incubation at the end of a 500 ms test pulse from −80 mV to +20 mV. Figure 6 A-H indicates representative current recordings evoked by a test pulse from -80 mV to +20 mV under control conditions and after 30 min incubation with ranolazine. Figure 6I summarizes the current inhibition by 300 µM ranolazine on the different TASK-1 pore mutants. Inhibition of TASK-1 by 300 µM ranolazine was significantly reduced in channel variants L122A (from 48.79 ± 3.52% in wild type (WT) channels to 8.1 ± 3.94% in the mutant variant, n = 5, p < 0.0001), L239A (reduced to 22.69 ± 6.73%, n = 5, p = 0.0007), and N240A (reduced to 24.29 ± 1.92%, n = 5, p = 0.0016). The inhibitory potency of ranolazine remained unchanged in channel variants F125A, Q126A, L232A, and I235A. Note that the amino acids that were included in our mutagenesis screen all line the central cavity of the TASK-1 inner pore (Figure 6 J-N).
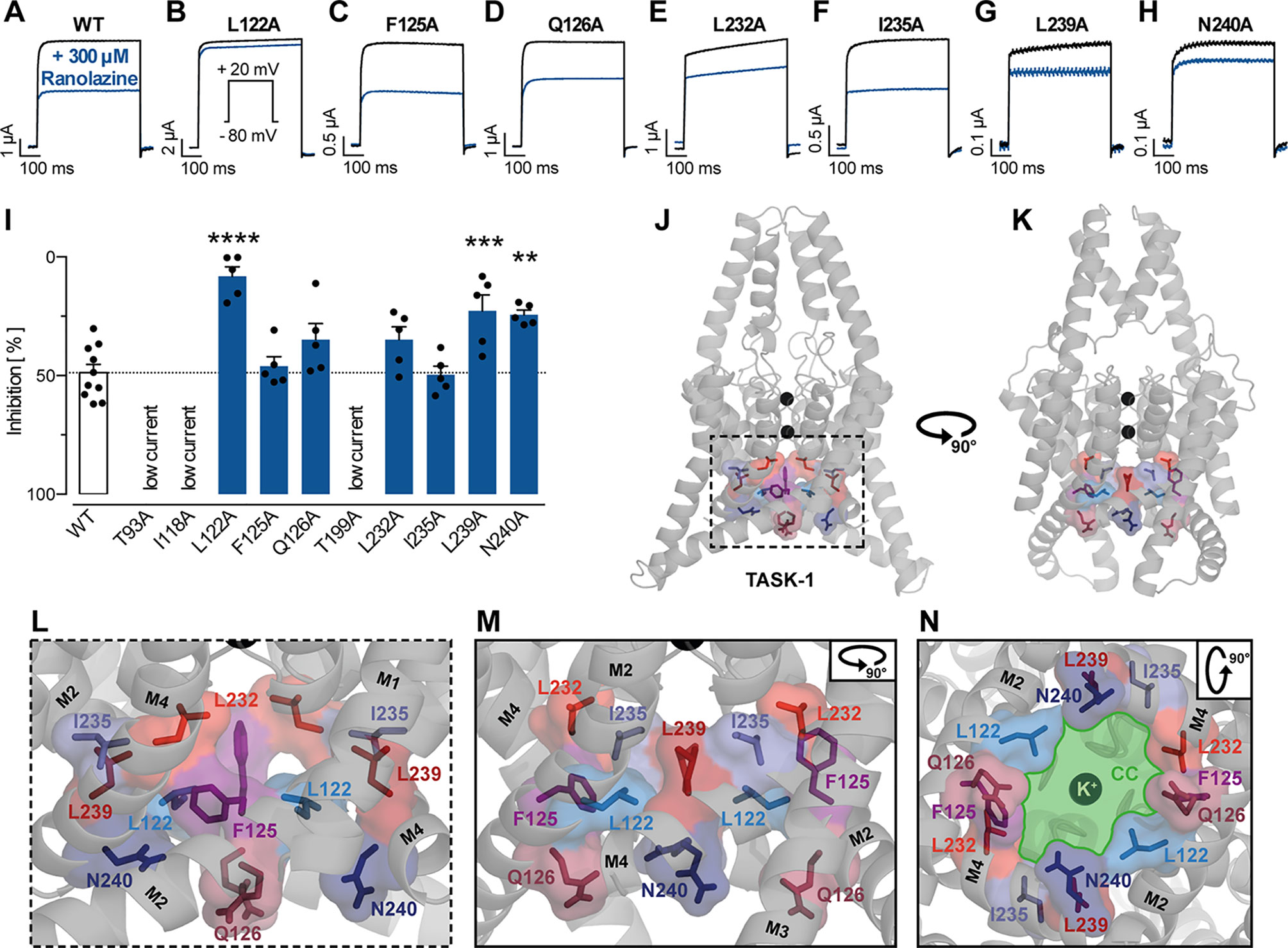
Figure 6 Effect of ranolazine on TASK-1 pore mutants. (A–H) Representative current recordings evoked by a test pulse from −80 mV to +20 mV under control conditions and after 30 min incubation with ranolazine. (I) Current inhibition by 300 µM ranolazine on the different TASK-1 pore mutants, **p < 0.01, ***p < 0.001, ****p < 0.0001, one-way ANOVA and post-hoc Bonferroni’s multiple comparisons test of WT vs. the respective mutant. (J, K) TASK-1 homology model based on TREK-1 illustrating the location of amino acid residues included in the mutagenesis screen. (L) Zoom into the inner pore region. (M) Lateral view as in panel (L) but from a different angle [as in panel (K)]. (N) View from inside the cell into the central cavity (CC) of the inner pore. Note how the displayed residues line the central cavity (illustrated in green) of the TASK-1 inner pore.
We further performed in silico docking simulations on TASK-1 alanine mutants at positions T93, L122, F125, T199, L232, and I235 that had been identified as the most relevant residues for ranolazine binding in the initial WT docking simulation. The results (summarized in Figure 7) suggest, that in channel variants F125A, L232A and I235A, ranolazine was still able to bind and form protein–ligand interactions at the altered binding site. In channel variant L122A the ability to form protein–ligand interactions was impaired; especially the number of relatively stable hydrogen bonds was significantly reduced compared to WT. More detailed information on the individual interaction profiles of ranolazine and the different TASK-1 mutant variants can be found in the supplementary material (Supplementary Figure S3).
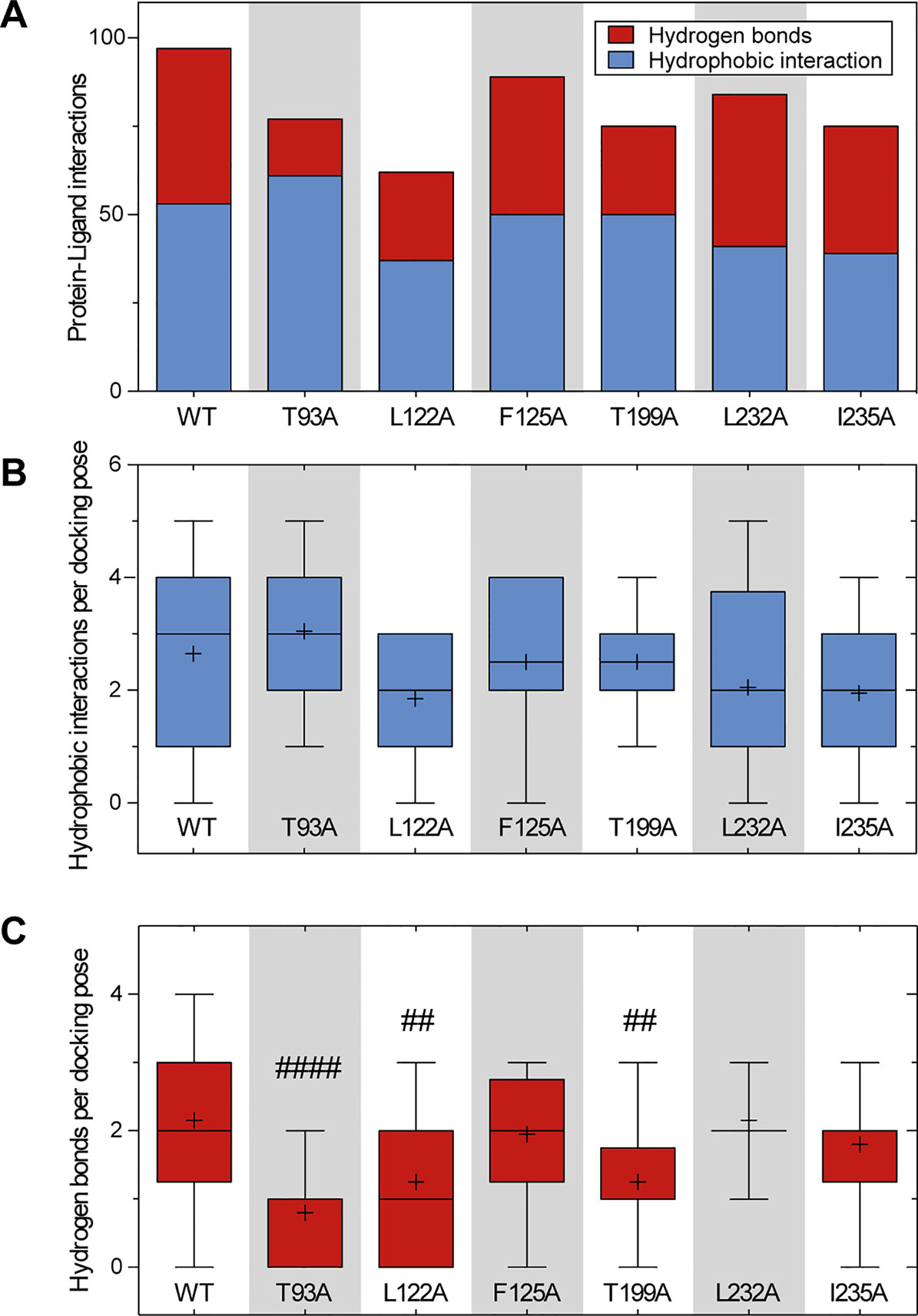
Figure 7 Comparison of the amount of protein–ligand interactions for the docking poses of WT and mutant channels. (A) Total amount and character of protein–ligand interactions of all 20 docking poses for the respective channel variant. (B, C) Boxplots indicating the amount of hydrophobic interactions (B) and hydrogen bonds (C) per docking pose. Boxes indicate the first and third quartile, whiskers indicate the minimum and maximum, bands inside the boxes indicate the median, and + indicates the mean. ##p < 0.01, ####p < 0.0001vs. WT. Note that variant L122A that has been identified as being most relevant for ranolazine binding in the experimental data, also forms the fewest interactions in the in silico simulations. More detailed information on the interaction profiles of ranolazine and the individual mutant variants can be found in Supplementary Figure S3.
Figure 8 illustrates the best ranked ranolazine docking pose for WT TASK-1 calculated by AutoDock Vina. Ranolazine is suggested to build a flat layer that binds within the central cavity of the TASK-1 inner pore at the bottom of the selectivity filter occluding the lumen. Ranolazine is located in close proximity (< 4 Å) to amino acid residues L122 and L239 that have also been shown to be relevant for drug binding in the mutagenesis screen. However, N240 is not predicted to contribute to direct drug binding, although the mutant N240A showed reduced ranolazine inhibition.
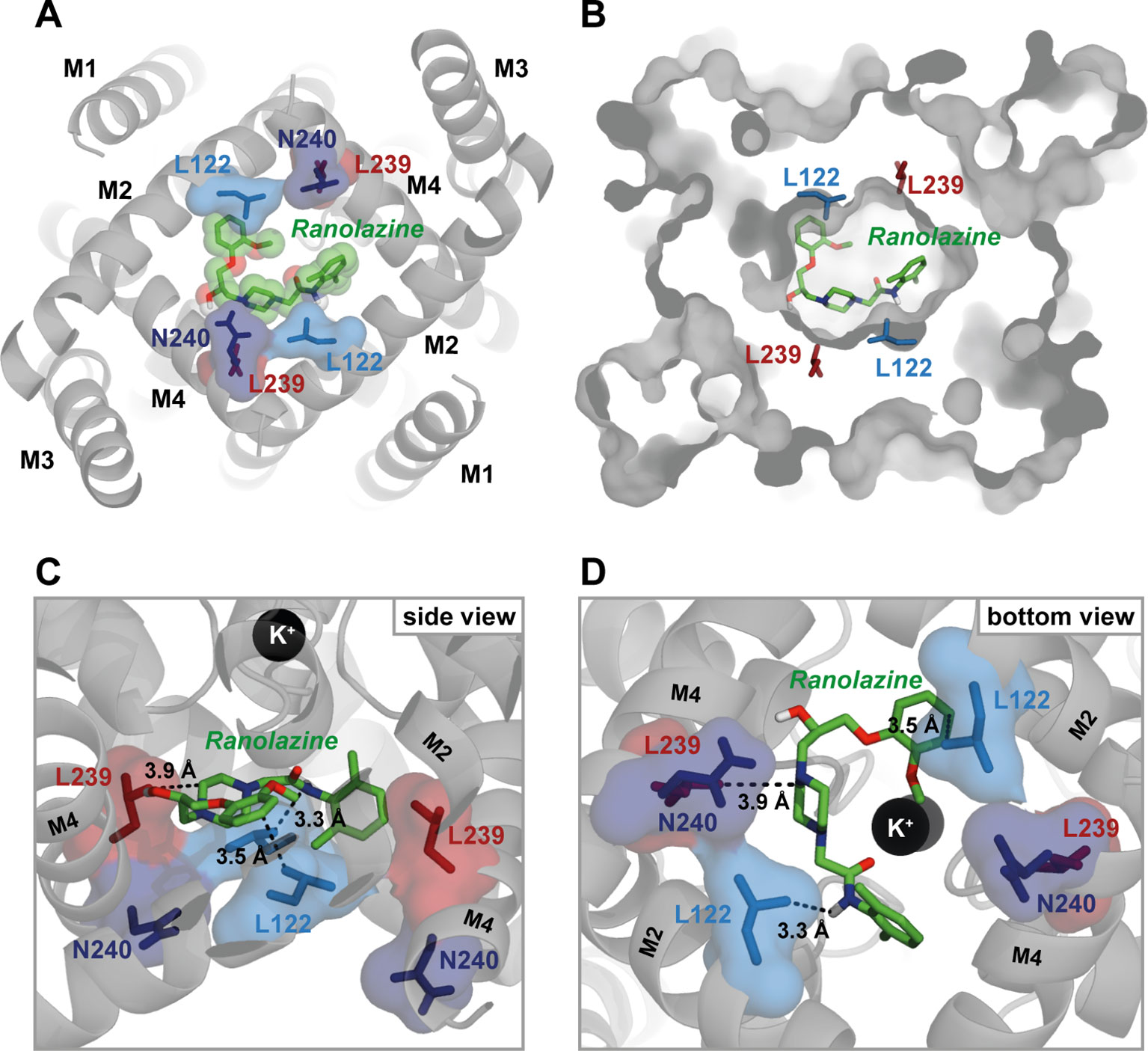
Figure 8 Ranolazine binds to the central cavity of the inner pore. (A) View from inside the cell into the central cavity. Ranolazine binds in close proximity to L122 and L239 occluding the lumen of the inner pore. (B) Same view as in (A) but with TASK-1 surface representation illustrating how ranolazine embeds within the inner pore. (C) Zoom into the central cavity illustrating the binding mode and the distance to the residues identified as binding site in the mutagenesis screen. Note that ranolazine does not directly interact with N240. (D) Similar zoom as in panel (C) but from inside the cell. Note that ranolazine interacts with L122 residues from both monomers.
In the illustrated docking pose ranolazine is also predicted to interact with residues F125 and L232, that showed no alteration of inhibition in the experimental data. Ranolazine is further predicted to form hydrogen bonds with residues T93 and T199. The relevance of T93 and T199 could not be validated experimentally due to too small current amplitudes of channel variants T93A and T199A.
Discussion
The development of safe and effective AADs for the treatment of AF is a major clinical challenge (Dobrev and Nattel 2010). Within the past years ranolazine has been identified to deploy potent antiarrhythmic properties and to be effective in the treatment of AF (Guerra et al., 2017). Here we show that ranolazine acts as an inhibitor of the atrial selective TASK-1 potassium channel.
For the prevention of post-operative atrial fibrillation (POAF), ranolazine has been shown to be even more effective than amiodarone (Miles et al., 2011). In this retrospective trial there was no difference in the incidence of adverse events. Ranolazine, however, is considered to be substantially safer in use than amiodarone or other AADs, because it has less proarrhythmic effects on the ventricles (Gupta et al., 2015). This seems to be due to a differential impact on atrial and ventricular cardiomyocytes (Antzelevitch et al., 2011). Burashnikov et al. (2007) noticed that ranolazine was able to prolong the atrial APD and ERP in canine cardiomyocytes with only little effect on the ventricular action potential (AP). They attributed this effect to the inhibition of peak INa only in atrial but not ventricular cardiomyocytes and therefore proposed atrial-selective sodium channel blockade by ranolazine as a strategy for the treatment of atrial fibrillation (Burashnikov et al., 2007). The mechanism of the atrial-selective block of Na+ channels has been explained primarily by the rapid dissociation kinetics of ranolazine, a more negative half-inactivation voltage (V0.5) in atrial cells than in ventricular cells and a more depolarized RMP in atrial cells. Unblocking of sodium channels is commonly associated with the resting state of the sodium channel (Carmeliet and Mubagwa 1998). Because of a more negative half-inactivation voltage (V0.5) in atrial cells than in ventricular cells, a greater fraction of atrial sodium channels would remain in the inactivated state. Therefore the proportion of time that channels are in the resting state would be reduced and hence the dissociation of ranolazine would be slower. Additionally, the availability of sodium channels and the number of channels in the resting state would be further reduced by a more depolarized RMP in atrial cells and the recovery of sodium channels from the inactivated to the resting state is reported to be slower in atrial cells (Antzelevitch and Burashnikov 2009; Nesterenko et al., 2011). Literature regarding a different V0.5 in atrial vs. ventricular cardiomyocytes, however, is inconsistent (Sakakibara et al., 1992; Sakakibara et al., 1993; Hiroe et al., 1997; Li et al., 2002). Caves et al. (2017) could recently reproduce atrial selectivity of INa block by ranolazine in rabbit, providing further evidence that this might be a key element in the atrial-ventricular differences in the action of ranolazine (Caves et al., 2017).
Another explanation for the differential impact on APD prolongation in atrial and ventricular cardiomyocytes lies in the differences of AP configuration between the two cell types that have direct influence on the net effect of simultaneous late INa and IKr blockade. Ventricular APs have a more prominent plateau phase than atrial APs. This suggest that there is a smaller late INa in atrial cells that may result in a shift towards a greater AP prolonging effect of IKr blockade in atrial cells, whereas in ventricular cells the inhibition of a larger late INa may offset the IKr blockade (Du et al., 2014).
We propose that inhibition of TASK-1 potassium channels may contribute to the observed electrophysiological effects of ranolazine. Inhibition of TASK-1 by specific inhibitors has been described to prolong atrial APD and ERP before (Wirth et al., 2003; Wirth et al., 2007; Decher et al., 2011; Donner et al., 2011; Skarsfeldt et al., 2016). Furthermore, the nearly atrial specific expression of TASK-1 could, at least in part, explain the differential behavior of ranolazine between atrial and ventricular tissue (Limberg et al., 2011; Schmidt et al., 2015; Schmidt et al., 2017). The IC50 of TASK-1 inhibition by ranolazine is determined at 30.64 µM in mammalian cells which is slightly beyond therapeutic free plasma levels of 2–13.35 µM (Jerling 2006; Undrovinas et al., 2006; EMA 2009; Antzelevitch et al., 2011). Considering that ranolazine has its highest antiarrhythmic potency when administered in high doses, inhibition of TASK-1 current would still be expected in vivo. At plasma levels of 10 µM an inhibition of 20% of TASK-1 current is expected. It has to be noticed, however, that TASK-1 blockade by ranolazine is always likely to be less extensive than late INa (IC50 5.9–15 µM) (Fredj et al., 2006; Antzelevitch et al., 2011) or IKr blockade (IC50 8–12 µM) (Rajamani et al., 2008; Du et al., 2014). Therefore, the clinical relevance of TASK-1 blockade by ranolazine remains speculative.
We identified the TASK-1 central cavity of the inner pore as the binding site of ranolazine. This binding site overlaps with that of other both high and low affinity blockers of TASK-1 (Streit et al., 2011; Kiper et al., 2015; Schmidt et al., 2018; Wiedmann et al., 2019). The binding configuration of ranolazine within the TASK-1 inner pore is also similar to results published by Du et al. (2014) for the human Ether à go go Related Gene (hERG) channel, the recombinant equivalent of IKr. In both TASK-1 and hERG, ranolazine lies high within the inner pore in a horizontal orientation below the selectivity filter where it may form hydrogen bonds with serine or threonine residues (Du et al., 2014). In both channels ranolazine can make additional hydrophobic interactions with pore lining residues (Figures 5 and 8). The structural basis for the higher inhibitory potency of ranolazine in hERG may result from a more optimal orientation of the pore lining side chains in hERG, especially in the inactivated state (Chen et al., 2002; Du et al., 2014). Evidence for this hypothesis comes from the observation that mutations of hERG outside the binding site that attenuate channel inactivation and influence configuration of the pore lining side chains to non-optimal arrangements, largely reduce the inhibitory potency of ranolazine without changing the binding site itself (Du et al., 2014).
The binding site is also similar to that in sodium channels, where ranolazine has been shown to interact over a larger surface area that spans from the inner pore to the side fenestration region (Fredj et al., 2006; Nguyen et al., 2019). In sodium channels, access to this binding site is most likely through the cytosolic mouth to the pore and therefore requires opening of the activation gate (Fredj et al., 2006; Wang et al., 2008; Caves et al., 2017). This mechanism provides the structural basis of the use-dependent block of sodium channels (Catterall 2012). In TASK-1, however, the inner pore region is constitutively open and therefore accessible for inhibitors. This may explain why we do not observe use-dependent block of TASK-1 by ranolazine. Another consequence of the TASK-1 drug binding site being constitutively accessible is that drug dissociation can occur at all time, whereas in sodium channels it requires the channel to be in the resting state (Antzelevitch et al., 2011). This may partly explain the higher inhibitory potency of ranolazine in sodium channels than in the constitutively open TASK-1 channel.
The side fenestrations mentioned earlier as being part of the drug binding site have recently been suggested to play an important role in K2P channel pharmacology by providing an “anchor” for stable binding of inhibitors (Ramirez et al., 2017). In our T16cq6 model (where the model template is TREK-2) the side fenestrations are closed. Therefore, ranolazine is binding solely within the inner pore of TASK-1. When docking ranolazine to the T13ukm model (model template TWIK-1), where the side fenestrations are open, we observe similar binding modes as in the T16cq6 model (Supplementary Figure S4). However, with the side fenestrations open, the binding site indeed spans from the inner pore to the entrance of the side fenestrations, because the side chains of L122 and L239 are partly facing towards the fenestrations. Nevertheless, the significance of the side fenestrations in TASK-1 remains speculative, because the structure of TASK-1 has not been revealed yet, and the fenestration state of TASK-1 homology models is ultimately depending on the template used for homology modelling (open fenestrations in TWIK-1, closed fenestrations in TREK-2).
Single mutants F125A, Q126A, L232A and I235A showed no difference in ranolazine blockade, although being predicted to be relevant for ranolazine binding in the in silico docking calculations or being identified as binding site for the high affinity blockers S20951 (A1899) and AVE1231 (A293). The reason could be the relatively small size of the ranolazine molecule compared to S20951 and AVE1231. Ranolazine appears to be able to fold into different conformations and hence bind at different sites within the inner pore. The molecular interactions between ranolazine and its binding site are mostly hydrophobic interactions. Only with residues T93 and T199 it forms more stable hydrogen bonds. The ability to form hydrogen bonds appears to be impaired in channel variant L122A, possibly explaining the fact that inhibition by ranolazine is almost abolished in this single mutant.
Study Limitations
Of note, channel variant N240A impedes ranolazine binding without being proposed to be part of the binding site in the in silico simulations. It remains unclear whether this is due to methodological limitations of homology modelling and docking simulations or results from interferences of the large asparagine side chain with structural domain morphology, therefore impeding the accessibility to the TASK-1 inner pore for inhibitors. The latter would be supported by the observation that movements or alterations of the M4 transmembrane domain (where N240 is located) affect channel gaiting in other K2P channels (Bagriantsev et al., 2011; Lolicato et al., 2014). However, results obtained by homology modelling are naturally largely influenced by the template that is chosen for modelling. We therefore tried to assess the models quality to our best possibilities. To assess possible influences of the fenestration state of our model, we performed additional docking simulations on the T1ukm model. Of course, deeper insights in this regard would require the solvation of the TASK-1 crystal structure.
Another limitation of this study is that a direct effect of the TASK-1 mutants on channel gating and therefore possible allosteric effects on TASK-1 pharmacology cannot be ruled out. Nevertheless, measurements of an inhibitor’s potency on different central pore mutants and comparisons with in silico docking simulations on homology models has been a widely accepted strategy in the field thus far.
Conclusions
This study adds to the action profile of ranolazine. We demonstrate that ranolazine is a TASK-1 inhibitor and suggest that TASK-1 inhibition may contribute to the antiarrhythmic effects of ranolazine. We propose that TASK-1 inhibition could, at least in part, explain the atrial selectivity of APD-prolongation by ranolazine. Even though the efficacy of ranolazine in AF might not yet be optimal, our findings put forward ranolazine as a prototype drug for the treatment of atrial arrhythmia because of its combined efficacy on atrial electrophysiology and lower risk for ventricular side effects.
Data Availability Statement
The raw data supporting the conclusions of this manuscript will be made available by the authors upon reasonable request.
Ethics Statement
The animal study was reviewed and approved by Regierungspräsidium Karlsruhe.
Author Contributions
AR, CS, and HK conceived and designed the experiments. AR, FW, and MK carried out the experiments. AR, FW, MK, and CS contributed to the interpretation of the results. AR and CS visualized the data and wrote the manuscript. HK and CS supervised the project. All authors provided critical feedback and helped shape the research, analysis and manuscript by providing important intellectual content. Further, all persons designated as authors qualify for authorship, and all those who qualify for authorship are listed. All authors agree to be accountable for all aspects of the work in ensuring that questions related to the accuracy or integrity of any part of the work are appropriately investigated and resolved. All authors approved the final version of the manuscript.
Funding
This study was supported in part by research grants from the University of Heidelberg Faculty of Medicine [Rahel Goitein-Straus Scholarship and Olympia-Morata Scholarship (to CS)], the German Center for Cardiovascular Research [Excellence Grant (to CS)], the German Heart Foundation/German Foundation of Heart Research [Grant F/41/15 (to CS), Grant F/35/18 (to FW and CS) and a Kaltenbach Scholarship (to AR and FW)], and the German Research Foundation [Grant SCHM 3358/1-1 (to CS)]. FW was supported by a German Cardiac Society Otto-Hess Scholarship and Research Scholarship. We acknowledge financial support by Deutsche Forschungsgemeinschaft within the funding programme Open Access Publishing, by the Baden-Württemberg Ministry of Science, Research and the Arts and by Ruprecht-Karls-Universität Heidelberg.
Conflict of Interest
The authors declare that the research was conducted in the absence of any commercial or financial relationships that could be construed as a potential conflict of interest.
Acknowledgments
We thank Katrin Kupser and Sabine Höllriegel for the excellent technical support.
Supplementary Material
The Supplementary Material for this article can be found online at: https://www.frontiersin.org/articles/10.3389/fphar.2019.01367/full#supplementary-material
References
Antzelevitch, C., Burashnikov, A. (2009). A trial-selective sodium channel block as a novel strategy for the management of atrial fibrillation. J. Electrocardiol. 42 (6), 543–548. doi: 10.1016/j.jelectrocard.2009.07.007
Antzelevitch, C., Burashnikov, A., Sicouri, S., Belardinelli, L. (2011). Electrophysiologic basis for the antiarrhythmic actions of ranolazine. Heart Rhythm 8 (8), 1281–1290. doi: 10.1016/j.hrthm.2011.03.045
Bagriantsev, S. N., Peyronnet, R., Clark, K. A., Honore, E., Minor, D. L., Jr. (2011). Multiple modalities converge on a common gate to control K2P channel function. Embo J. 30 (17), 3594–3606. doi: 10.1038/emboj.2011.230
Benkert, P., Biasini, M., Schwede, T. (2011). Toward the estimation of the absolute quality of individual protein structure models. Bioinformatics 27 (3), 343–350. doi: 10.1093/bioinformatics/btq662
Bertoni, M., Kiefer, F., Biasini, M., Bordoli, L., Schwede, T. (2017). Modeling protein quaternary structure of homo- and hetero-oligomers beyond binary interactions by homology. Sci. Rep. 7 (1), 10480. doi: 10.1038/s41598-017-09654-8
Bittner, S., Meuth, S. G., Gobel, K., Melzer, N., Herrmann, A. M., Simon, O. J. (2009). TASK1 modulates inflammation and neurodegeneration in autoimmune inflammation of the central nervous system. Brain 132 (Pt 9), 2501–2516. doi: 10.1093/brain/awp163
Buckler, K. J., Williams, B. A., Honore, E. (2000). An oxygen-, acid- and anaesthetic-sensitive TASK-like background potassium channel in rat arterial chemoreceptor cells. J. Physiol. 525 Pt 1, 135–142. doi: 10.1111/j.1469-7793.2000.00135.x
Burashnikov, A., Di Diego, J. M., Zygmunt, A. C., Belardinelli, L., Antzelevitch, C. (2007). Atrium-selective sodium channel block as a strategy for suppression of atrial fibrillation: differences in sodium channel inactivation between atria and ventricles and the role of ranolazine. Circulation 116 (13), 1449–1457. doi: 10.1161/CIRCULATIONAHA.107.704890
Carmeliet, E., Mubagwa, K. (1998). Antiarrhythmic drugs and cardiac ion channels: mechanisms of action. Prog. Biophys. Mol. Biol. 70 (1), 1–72. doi: 10.1016/S0079-6107(98)00002-9
Catterall, W. A. (2012). Voltage-gated sodium channels at 60: structure, function and pathophysiology. J. Physiol. 590 (11), 2577–2589. doi: 10.1113/jphysiol.2011.224204
Caves, R. E., Cheng, H., Choisy, S. C., Gadeberg, H. C., Bryant, S. M., Hancox, J. C. (2017). Atrial-ventricular differences in rabbit cardiac voltage-gated Na+ currents: basis for atrial-selective block by ranolazine. Heart Rhythm 14 (11), 1657–1664. doi: 10.1016/j.hrthm.2017.06.012
Chen, J., Seebohm, G., Sanguinetti, M. C. (2002). Position of aromatic residues in the S6 domain, not inactivation, dictates cisapride sensitivity of HERG and eag potassium channels. Proc. Natl. Acad. Sci. U. S. A. 99 (19), 12461–12466. doi: 10.1073/pnas.192367299
Czirjak, G., Enyedi, P. (2002). TASK-3 dominates the background potassium conductance in rat adrenal glomerulosa cells. Mol. Endocrinol. 16 (3), 621–629. doi: 10.1210/mend.16.3.0788
Davies, L. A., Hu, C., Guagliardo, N. A., Sen, N., Chen, X., Talley, E. M. (2008). TASK channel deletion in mice causes primary hyperaldosteronism. Proc. Natl. Acad. Sci. U. S. A. 105 (6), 2203–2208. doi: 10.1073/pnas.0712000105
Davis, I. W., Leaver-Fay, A., Chen, V. B., Block, J. N., Kapral, G. J., Wang, X. (2007). MolProbity: all-atom contacts and structure validation for proteins and nucleic acids. Nucleic Acids Res. 35 (Web Server issue), W375–W383. doi: 10.1093/nar/gkm216
Decher, N., Wemhoner, K., Rinne, S., Netter, M. F., Zuzarte, M., Aller, M. I. (2011). Knock-out of the potassium channel TASK-1 leads to a prolonged QT interval and a disturbed QRS complex. Cell Physiol. Biochem. 28 (1), 77–86. doi: 10.1159/000331715
Dobrev, D., Nattel, S. (2010). New antiarrhythmic drugs for treatment of atrial fibrillation. Lancet 375 (9721), 1212–1223. doi: 10.1016/S0140-6736(10)60096-7
Dong, Y. Y., Pike, A. C., Mackenzie, A., McClenaghan, C., Aryal, P., Dong, L. (2015). K2P channel gating mechanisms revealed by structures of TREK-2 and a complex with Prozac. Science 347 (6227), 1256–1259. doi: 10.1126/science.1261512
Donner, B. C., Schullenberg, M., Geduldig, N., Huning, A., Mersmann, J., Zacharowski, K. (2011). Functional role of TASK-1 in the heart: studies in TASK-1-deficient mice show prolonged cardiac repolarization and reduced heart rate variability. Basic Res. Cardiol. 106 (1), 75–87. doi: 10.1007/s00395-010-0128-x
Du, C., Zhang, Y., El Harchi, A., Dempsey, C. E., Hancox, J. C. (2014). Ranolazine inhibition of hERG potassium channels: drug-pore interactions and reduced potency against inactivation mutants. J. Mol. Cell Cardiol. 74, 220–230. doi: 10.1016/j.yjmcc.2014.05.013
EMA (2009, 20/05/2019). Ranexa: EPAR - Product Information. Eur. Med. Agency. https://www.ema.europa.eu/en/documents/product-information/ranexa-epar-product-information_en.pdf.
Feliciangeli, S., Chatelain, F. C., Bichet, D., Lesage, F. (2015). The family of K2P channels: salient structural and functional properties. J. Physiol. 593 (12), 2587–2603. doi: 10.1113/jphysiol.2014.287268
Fredj, S., Sampson, K. J., Liu, H., Kass, R. S. (2006). Molecular basis of ranolazine block of LQT-3 mutant sodium channels: evidence for site of action. Br. J. Pharmacol. 148 (1), 16–24. doi: 10.1038/sj.bjp.0706709
Gierten, J., Ficker, E., Bloehs, R., Schlomer, K., Kathofer, S., Scholz, E. (2008). Regulation of two-pore-domain (K2P) potassium leak channels by the tyrosine kinase inhibitor genistein. Br. J. Pharmacol. 154 (8), 1680–1690. doi: 10.1038/bjp.2008.213
Goldstein, S. A., Bockenhauer, D., O’Kelly, I., Zilberberg, N. (2001). Potassium leak channels and the KCNK family of two-P-domain subunits. Nat. Rev. Neurosci. 2 (3), 175–184. doi: 10.1038/35058574
Guerra, F., Romandini, A., Barbarossa, A., Belardinelli, L., Capucci, A. (2017). “Ranolazine for rhythm control in atrial fibrillation: a systematic review and meta-analysis.”. Int. J. Cardiol. 227, 284–291. doi: 10.1016/j.ijcard.2016.11.103
Gupta, T., Khera, S., Kolte, D., Aronow, W. S., Iwai, S. (2015). Antiarrhythmic properties of ranolazine: a review of the current evidence. Int. J. Cardiol. 187, 66–74. doi: 10.1016/j.ijcard.2015.03.324
Hiroe, K., Hisatome, I., Tanaka, Y., Ahmmed, G. U., Sasaki, N., Shimoyama, M. (1997). Tonic block of the Na+ current in single atrial and ventricular guinea-pig myocytes, by a new antiarrhythmic drug, Ro 22-9194 . Fundam. Clin. Pharmacol. 11 (5), 402–407. doi: 10.1111/j.1472-8206.1997.tb00202.x
January, C. T., Wann, L. S., Alpert, J. S., Calkins, H., Cigarroa, J. E., Cleveland, J. C. Jr. (2014). 2014 AHA/ACC/HRS guideline for the management of patients with atrial fibrillation: a report of the American College of Cardiology/American Heart Association Task Force on Practice Guidelines and the Heart Rhythm Society. J. Am. Coll. Cardiol. 64 (21), e1–76. doi: 10.1016/j.jacc.2014.03.022
Jerling, M. (2006). Clinical pharmacokinetics of ranolazine. Clin. Pharmacokinet. 45 (5), 469–491. doi: 10.2165/00003088-200645050-00003
Kiper, A. K., Rinne, S., Rolfes, C., Ramirez, D., Seebohm, G., Netter, M. F. (2015). Kv1.5 blockers preferentially inhibit TASK-1 channels: TASK-1 as a target against atrial fibrillation and obstructive sleep apnea? Pflugers Arch. 467 (5), 1081–1090. doi: 10.1007/s00424-014-1665-1
Kirchhof, P., Benussi, S., Kotecha, D., Ahlsson, A., Atar, D., Casadei, B. (2016). 2016 ESC Guidelines for the management of atrial fibrillation developed in collaboration with EACTS. Eur. Heart J. 37 (38), 2893–2962. doi: 10.1093/eurheartj/ehw210
Koizumi, H., Smerin, S. E., Yamanishi, T., Moorjani, B. R., Zhang, R., Smith, J. C. (2010). TASK channels contribute to the K+-dominated leak current regulating respiratory rhythm generation in vitro. J. Neurosci. 30 (12), 4273–4284. doi: 10.1523/JNEUROSCI.4017-09.2010
Lafuente-Lafuente, C., Valembois, L., Bergmann, J. F., Belmin, J. (2015). Antiarrhythmics for maintaining sinus rhythm after cardioversion of atrial fibrillation. Cochrane Database Syst. Rev. (3), Cd005049. doi: 10.1002/14651858.CD005049.pub4
Lauritzen, I., Zanzouri, M., Honore, E., Duprat, F., Ehrengruber, M. U., Lazdunski, M. (2003). K+-dependent cerebellar granule neuron apoptosis. Role of task leak K+ channels. J. Biol. Chem. 278 (34), 32068–32076. doi: 10.1074/jbc.M302631200
Lazarenko, R. M., Willcox, S. C., Shu, S., Berg, A. P., Jevtovic-Todorovic, V., Talley, E. M. (2010). Motoneuronal TASK channels contribute to immobilizing effects of inhalational general anesthetics. J. Neurosci. 30 (22), 7691–7704. doi: 10.1523/JNEUROSCI.1655-10.2010
Li, G. R., Lau, C. P., Shrier, A. (2002). Heterogeneity of sodium current in atrial vs epicardial ventricular myocytes of adult guinea pig hearts. J. Mol. Cell Cardiol. 34 (9), 1185–1194. doi: 10.1006/jmcc.2002.2053
Limberg, S. H., Netter, M. F., Rolfes, C., Rinne, S., Schlichthorl, G., Zuzarte, M. (2011). TASK-1 channels may modulate action potential duration of human atrial cardiomyocytes. Cell Physiol. Biochem. 28 (4), 613–624. doi: 10.1159/000335757
Lolicato, M., Riegelhaupt, P. M., Arrigoni, C., Clark, K. A., Minor, D. L., Jr. (2014). Transmembrane helix straightening and buckling underlies activation of mechanosensitive and thermosensitive K(2P) channels. Neuron 84 (6), 1198–1212. doi: 10.1016/j.neuron.2014.11.017
Lolicato, M., Arrigoni, C., Mori, T., Sekioka, Y., Bryant, C., Clark, K. A. (2017). K2P2.1 (TREK-1)-activator complexes reveal a cryptic selectivity filter binding site. Nature 547 (7663), 364–368. doi: 10.1038/nature22988
Miles, R. H., Passman, R., Murdock, D. K. (2011). Comparison of effectiveness and safety of ranolazine versus amiodarone for preventing atrial fibrillation after coronary artery bypass grafting. Am. J. Cardiol. 108 (5), 673–676. doi: 10.1016/j.amjcard.2011.04.017
Miller, A. N., Long, S. B. (2012). Crystal structure of the human two-pore domain potassium channel K2P1 . Science 335 (6067), 432–436. doi: 10.1126/science.1213274
Mulkey, D. K., Talley, E. M., Stornetta, R. L., Siegel, A. R., West, G. H., Chen, X. (2007). TASK channels determine pH sensitivity in select respiratory neurons but do not contribute to central respiratory chemosensitivity. J. Neurosci. 27 (51), 14049–14058. doi: 10.1523/JNEUROSCI.4254-07.2007
Nash, D. T., Nash, S. D. (2008). Ranolazine for chronic stable angina. Lancet 372 (9646), 1335–1341. doi: 10.1016/S0140-6736(08)61554-8
Nesterenko, V. V., Zygmunt, A. C., Rajamani, S., Belardinelli, L., Antzelevitch, C. (2011). Mechanisms of atrial-selective block of Na(+) channels by ranolazine: II. Insights from a mathematical model. Am. J. Physiol. Heart Circ. Physiol. 301 (4), H1615–H1624. doi: 10.1152/ajpheart.00243.2011
Nguyen, P. T., DeMarco, K. R., Vorobyov, I., Clancy, C. E., Yarov-Yarovoy, V. (2019). Structural basis for antiarrhythmic drug interactions with the human cardiac sodium channel. Proc. Natl. Acad. Sci. U.S.A. 116 (8), 2945–2954. doi: 10.1073/pnas.1817446116
Olschewski, A., Li, Y., Tang, B., Hanze, J., Eul, B., Bohle, R. M. (2006). Impact of TASK-1 in human pulmonary artery smooth muscle cells. Circ. Res. 98 (8), 1072–1080. doi: 10.1161/01.RES.0000219677.12988.e9
Putzke, C., Wemhoner, K., Sachse, F. B., Rinne, S., Schlichthorl, G., Li, X. T. (2007). The acid-sensitive potassium channel TASK-1 in rat cardiac muscle. Cardiovasc. Res. 75 (1), 59–68. doi: 10.1016/j.cardiores.2007.02.025
Rajamani, S., Shryock, J. C., Belardinelli, L. (2008). Rapid kinetic interactions of ranolazine with HERG K+ current. J. Cardiovasc. Pharmacol. 51 (6), 581–589. doi: 10.1097/FJC.0b013e3181799690
Ramirez, D., Arevalo, B., Martinez, G., Rinne, S., Sepulveda, F. V., Decher, N. (2017). Side Fenestrations Provide anAnchorfor a Stable Binding of A1899 to the Pore of TASK-1 Potassium Channels. Mol. Pharm. 14 (7), 2197–2208. doi: 10.1021/acs.molpharmaceut.7b00005
Sakakibara, Y., Wasserstrom, J. A., Furukawa, T., Jia, H., Arentzen, C. E., Hartz, R. S. (1992). Characterization of the sodium current in single human atrial myocytes. Circ. Res. 71 (3), 535–546. doi: 10.1161/01.RES.71.3.535
Sakakibara, Y., Furukawa, T., Singer, D. H., Jia, H., Backer, C. L., Arentzen, C. E. (1993). Sodium current in isolated human ventricular myocytes. Am. J. Physiol. 265 (4 Pt 2), H1301–H1309. doi: 10.1152/ajpheart.1993.265.4.H1301
Salentin, S., Schreiber, S., Haupt, V. J., Adasme, M. F., Schroeder, M. (2015). PLIP: fully automated protein-ligand interaction profiler. Nucleic Acids Res. 43 (W1), W443–W447. doi: 10.1093/nar/gkv315
Schmidt, C., Wiedmann, F., Schweizer, P. A., Becker, R., Katus, H. A., Thomas, D. (2013). Class I antiarrhythmic drugs inhibit human cardiac two-pore-domain K(+) (K2 (2)p) channels. Eur. J. Pharmacol. 721 (1-3), 237–248. doi: 10.1016/j.ejphar.2013.09.029
Schmidt, C., Wiedmann, F., Voigt, N., Zhou, X. B., Heijman, J., Lang, S. (2015). Upregulation of K(2P)3.1 K+ Current Causes Action Potential Shortening in Patients With Chronic Atrial Fibrillation. Circulation 132 (2), 82–92. doi: 10.1161/CIRCULATIONAHA.114.012657
Schmidt, C., Wiedmann, F., Zhou, X. B., Heijman, J., Voigt, N., Ratte, A. (2017). Inverse remodelling of K2P3.1 K+ channel expression and action potential duration in left ventricular dysfunction and atrial fibrillation: implications for patient-specific antiarrhythmic drug therapy. Eur. Heart J. 38 (22), 1764–1774. doi: 10.1093/eurheartj/ehw559
Schmidt, C., Wiedmann, F., Gaubatz, A. R., Ratte, A., Katus, H. A., Thomas, D. (2018). New Targets for Old Drugs: Cardiac Glycosides Inhibit Atrial-Specific K2P3.1 (TASK-1) Channels. J. Pharmacol. Exp. Ther. 365 (3), 614–623. doi: 10.1124/jpet.118.247692
Skarsfeldt, M. A., Jepps, T. A., Bomholtz, S. H., Abildgaard, L., Sorensen, U. S., Gregers, E. (2016). pH-dependent inhibition of K(2)P3.1 prolongs atrial refractoriness in whole hearts. Pflugers Arch. 468 (4), 643–654. doi: 10.1007/s00424-015-1779-0
Sossalla, S., Kallmeyer, B., Wagner, S., Mazur, M., Maurer, U., Toischer, K. (2010). Altered Na(+) currents in atrial fibrillation effects of ranolazine on arrhythmias and contractility in human atrial myocardium. J. Am. Coll. Cardiol. 55 (21), 2330–2342. doi: 10.1016/j.jacc.2009.12.055
Streit, A. K., Netter, M. F., Kempf, F., Walecki, M., Rinne, S., Bollepalli, M. K. (2011). A specific two-pore domain potassium channel blocker defines the structure of the TASK-1 open pore. J. Biol. Chem. 286 (16), 13977–13984. doi: 10.1074/jbc.M111.227884
Trott, O., Olson, A. J. (2010). AutoDock Vina: improving the speed and accuracy of docking with a new scoring function, efficient optimization, and multithreading. J. Comput. Chem. 31 (2), 455–461. doi: 10.1002/jcc.21334
Undrovinas, A. I., Belardinelli, L., Undrovinas, N. A., Sabbah, H. N. (2006). Ranolazine improves abnormal repolarization and contraction in left ventricular myocytes of dogs with heart failure by inhibiting late sodium current. J. Cardiovasc. Electrophysiol. 17 Suppl 1, S169–s177. doi: 10.1111/j.1540-8167.2006.00401.x
Vu, M. T., Du, G., Bayliss, D. A., Horner, R. L. (2015). TASK Channels on Basal Forebrain Cholinergic Neurons Modulate Electrocortical Signatures of Arousal by Histamine. J. Neurosci. 35 (40), 13555–13567. doi: 10.1523/JNEUROSCI.1445-15.2015
Wang, G. K., Calderon, J., Wang, S. Y. (2008). State- and use-dependent block of muscle Nav1.4 and neuronal Nav1.7 voltage-gated Na+ channel isoforms by ranolazine. Mol. Pharmacol. 73 (3), 940–948. doi: 10.1124/mol.107.041541
Waterhouse, A., Bertoni, M., Bienert, S., Studer, G., Tauriello, G., Gumienny, R. (2018). SWISS-MODEL: homology modelling of protein structures and complexes. Nucleic Acids Res. 46 (W1), W296–w303. doi: 10.1093/nar/gky427
Wiedmann, F., Kiper, A. K., Bedoya, M., Ratte, A., Rinne, S., Kraft, M. (2019). Identification of the A293 (AVE1231) binding site in the cardiac two-pore-domain potassium channel task-1: a common low affinity antiarrhythmic drug binding site. Cell Physiol. Biochem. 52 (5), 1223–1235. doi: 10.33594/000000083
Wirth, K. J., Paehler, T., Rosenstein, B., Knobloch, K., Maier, T., Frenzel, J. (2003). Atrial effects of the novel K(+)-channel-blocker AVE0118 in anesthetized pigs. Cardiovasc. Res. 60 (2), 298–306. doi: 10.1016/S0008-6363(03)00543-1
Keywords: atrial fibrillation, ranolazine, antiarrhythmic drugs, TASK-1, K2P3.1, KCNK3
Citation: Ratte A, Wiedmann F, Kraft M, Katus HA and Schmidt C (2019) Antiarrhythmic Properties of Ranolazine: Inhibition of Atrial Fibrillation Associated TASK-1 Potassium Channels. Front. Pharmacol. 10:1367. doi: 10.3389/fphar.2019.01367
Received: 15 August 2019; Accepted: 28 October 2019;
Published: 26 November 2019.
Edited by:
László Virág, University of Szeged, HungaryReviewed by:
Jules Hancox, University of Bristol, United KingdomCees Korstanje, Astellas Pharma (Europe), Netherlands
Copyright © 2019 Ratte, Wiedmann, Kraft, Katus and Schmidt. This is an open-access article distributed under the terms of the Creative Commons Attribution License (CC BY). The use, distribution or reproduction in other forums is permitted, provided the original author(s) and the copyright owner(s) are credited and that the original publication in this journal is cited, in accordance with accepted academic practice. No use, distribution or reproduction is permitted which does not comply with these terms.
*Correspondence: Constanze Schmidt, Q29uc3RhbnplLlNjaG1pZHRAbWVkLnVuaS1oZWlkZWxiZXJnLmRl