- 1Department of Physiology, Faculty of Medicine, University of Debrecen, Debrecen, Hungary
- 2Division of Cardiovascular Sciences, School of Medical Sciences, University of Manchester, Manchester, United Kingdom
- 3Department of Dental Physiology, Faculty of Dentistry, University of Debrecen, Debrecen, Hungary
Calcium ions (Ca2+) play a major role in the cardiac excitation-contraction coupling. Intracellular Ca2+ concentration increases during systole and falls in diastole thereby determining cardiac contraction and relaxation. Normal cardiac function also requires perfect organization of the ion currents at the cellular level to drive action potentials and to maintain action potential propagation and electrical homogeneity at the tissue level. Any imbalance in Ca2+ homeostasis of a cardiac myocyte can lead to electrical disturbances. This review aims to discuss cardiac physiology and pathophysiology from the elementary membrane processes that can cause the electrical instability of the ventricular myocytes through intracellular Ca2+ handling maladies to inherited and acquired arrhythmias. Finally, the paper will discuss the current therapeutic approaches targeting cardiac arrhythmias.
Introduction
Excitation-contraction coupling (E-C coupling) of the cardiac myocytes is a well studied phenomenon. We know that the calcium ion (Ca2+) plays a major role in controlling contraction and force, a feature that was originally described by Sidney Ringer more than a century ago (Ringer, 1883). Since this discovery, it has become clear that changes in intracellular Ca2+ concentration ([Ca2+]i) have a significant role in virtually all parts of the human body. Of particular importance is the fact, that within cardiac myocytes, [Ca2+]i changes must be tightly regulated, so that the heart can beat rhythmically. This means that during the cardiac systole, [Ca2+]i has to increase to certain levels to make contraction occur and must fall in diastole, allowing the muscle to relax and prepare for the next cardiac cycle. E-C coupling has been reviewed in detail (Bers, 2002; Eisner et al., 2017), here we consider the elementary steps and the events that can lead to electrical disturbances (Figure 1).
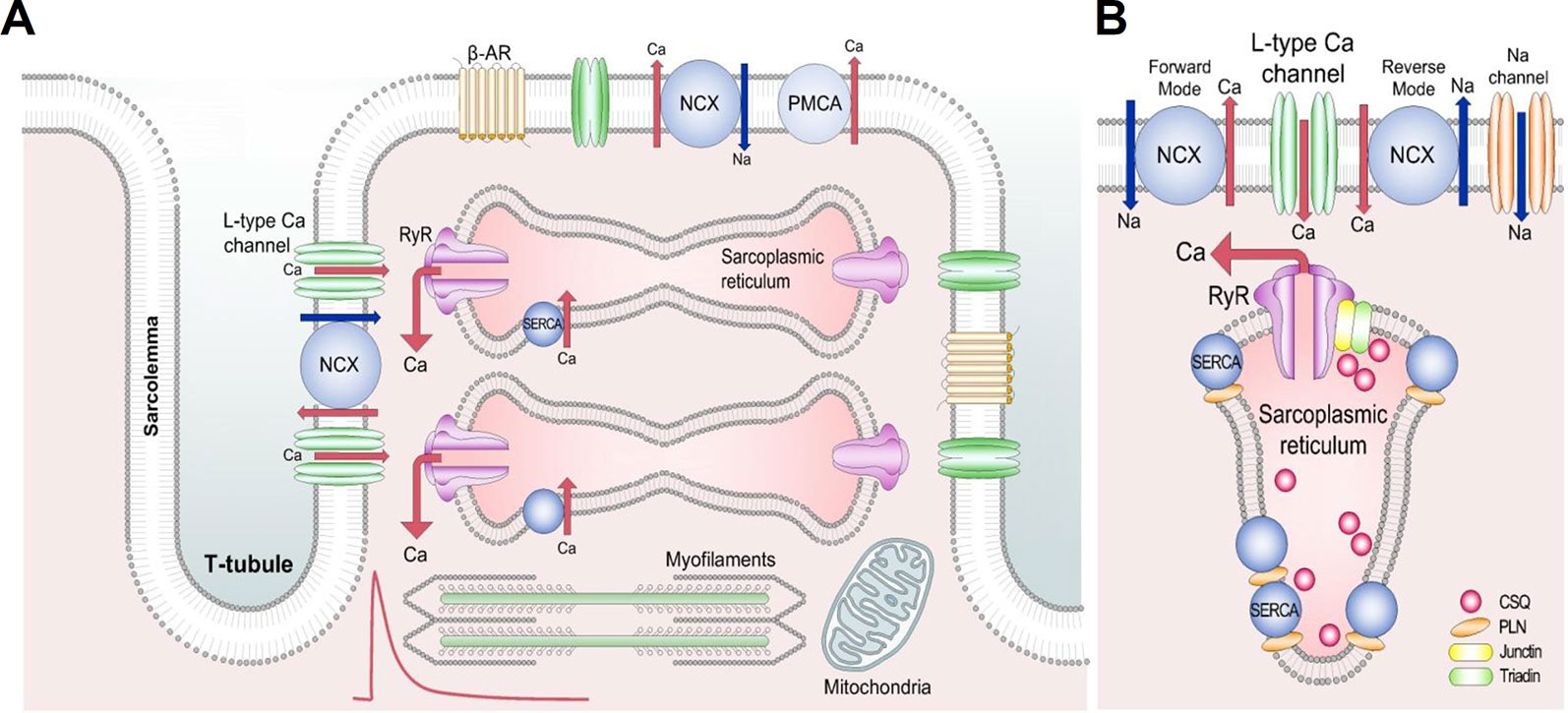
Figure 1 Schematic diagram of the cardiac excitation-contraction coupling. (A) Structures involved in Ca2+ transport in cardiac mycocytes. Red trace shows a typical systolic Ca2+ transient. Briefly, during the Ca2+-induced Ca2+ release process, Ca2+ entering the cell via L-type Ca2+ channels releases a larger amount of Ca2+ from the sarcoplasmic reticulum to activate the contractile machinery. Ca2+ extrusion requires NCX, PMCA, and SERCA. (B) Detailed section of the dyad showing the major proteins involved in Ca2+ cycling. Reproduced from Eisner et al. used with permission (Eisner et al., 2017). β-AR, β adrenoceptor; NCX, Na+-Ca2+ exchange; PMCA, plasma membrane Ca2+-ATPase; RyR, ryanodine receptor; SERCA, sarco/endoplasmic reticulum Ca2+-ATPase; CSQ, calsequestrin; PLN, phospholamban.
The normal cardiac action potential (AP) originates in the sinoatrial node and propagates through the heart. In the ventricle the initial depolarization opens voltage-gated sodium channels leading to further depolarization which, in turn, opens the L-type Ca2+ channels, causing a large Ca2+-influx (Figure 1A). Some Ca2+ can also enter via T-type Ca2+ channels and reverse mode Na+/Ca2+ exchange (NCX) (Kohomoto et al., 1994; Sipido et al., 1997). This Ca2+ entry triggers a process known as calcium-induced calcium release (CICR), in which Ca2+ is released from the sarcoplasmic reticulum (SR) into the cytoplasm via ryanodine receptors (RyR), allowing Ca2+ to bind to the myofilament protein troponin C, activating the contractile machinery. Normal cardiac function also requires relaxation to occur; this results from a decrease of free cytoplasmic Ca2+ levels. Several Ca2+ transport pathways are involved in this process, as Ca2+ reuptake into the SR by the SR Ca2+-ATPase (SERCA), Ca2+ extrusion by the sarcolemmal NCX and plasma membrane Ca2+-ATPase (PMCA) (Figure 1B) (Bers, 2000). This normal cardiac function requires perfect coordination of the ion currents and intracellular processes, as any imbalance in Ca2+ homeostasis of a cardiac myocyte can lead to electrical disturbances (from cellular AP prolongation to complex arrhythmic storms) (Eisner et al., 2017; Eisner, 2018).
Here we review the role of Ca2+ in generating and maintaining cardiac arrhythmias from basic arrhythmia mechanisms to recent progresses in pharmacological challenges and possible future therapies.
Calcium in Pathophysiology, Arrhythmia Mechanisms
Arrhythmia mechanisms have multiscale dynamics in the heart. The lower end is the molecular scale, originating from the stochastic behavior of ion channels, resulting from thermodynamic fluctuations (Qu and Weiss, 2015). Next is the cellular scale, with differences in the shape of the APs originating from distant parts of the myocardium (Figure 2A). Under some diseased conditions, several mechanisms can lead to electrical disturbances at the cellular level, including early or delayed afterdepolarizations (EAD or DAD, respectively) (Figures 3A–D). Whole-cell Ca2+ oscillations, developing into propagating Ca2+ waves arise when the molecular and cellular dynamics merge at the tissue and organ level. The lower and higher scales tend to have a bidirectional information flow. A good example is when EADs arising during an AP due to abnormal ion currents and Ca2+ dynamics, can bring an extra amount of Ca2+ into the cell due to L-type Ca2+ channel reopening and potentiate Ca2+ waves. These multiscale dynamics can lead to life threatening complex arrhythmias.
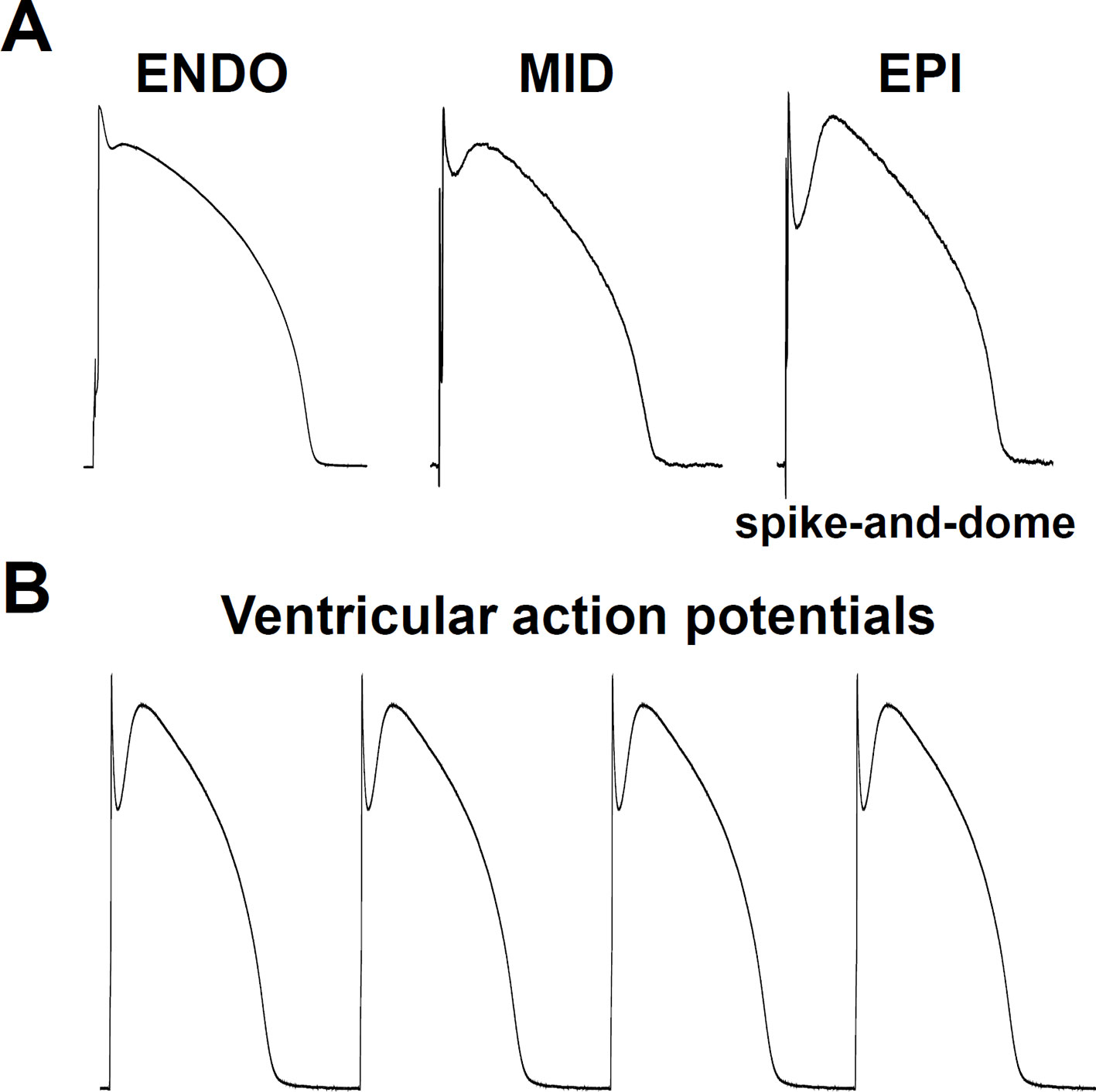
Figure 2 Cellular physiological electrical activities. (A) Transmural heterogeneity in the cardiac ventricular action potential, showing (from left to right) recordings from: subendocardium, midmyocardium, and subepicardium. Note the spike-and-dome action potential configuration in the subepicardium. ENDO, subendocardial mycocyte; MID, midmyocardial “M” myocyte; EPI, subepicardial myocyte. (B) Series of typical subepicardial ventricular action potentials at normal pacing activity.
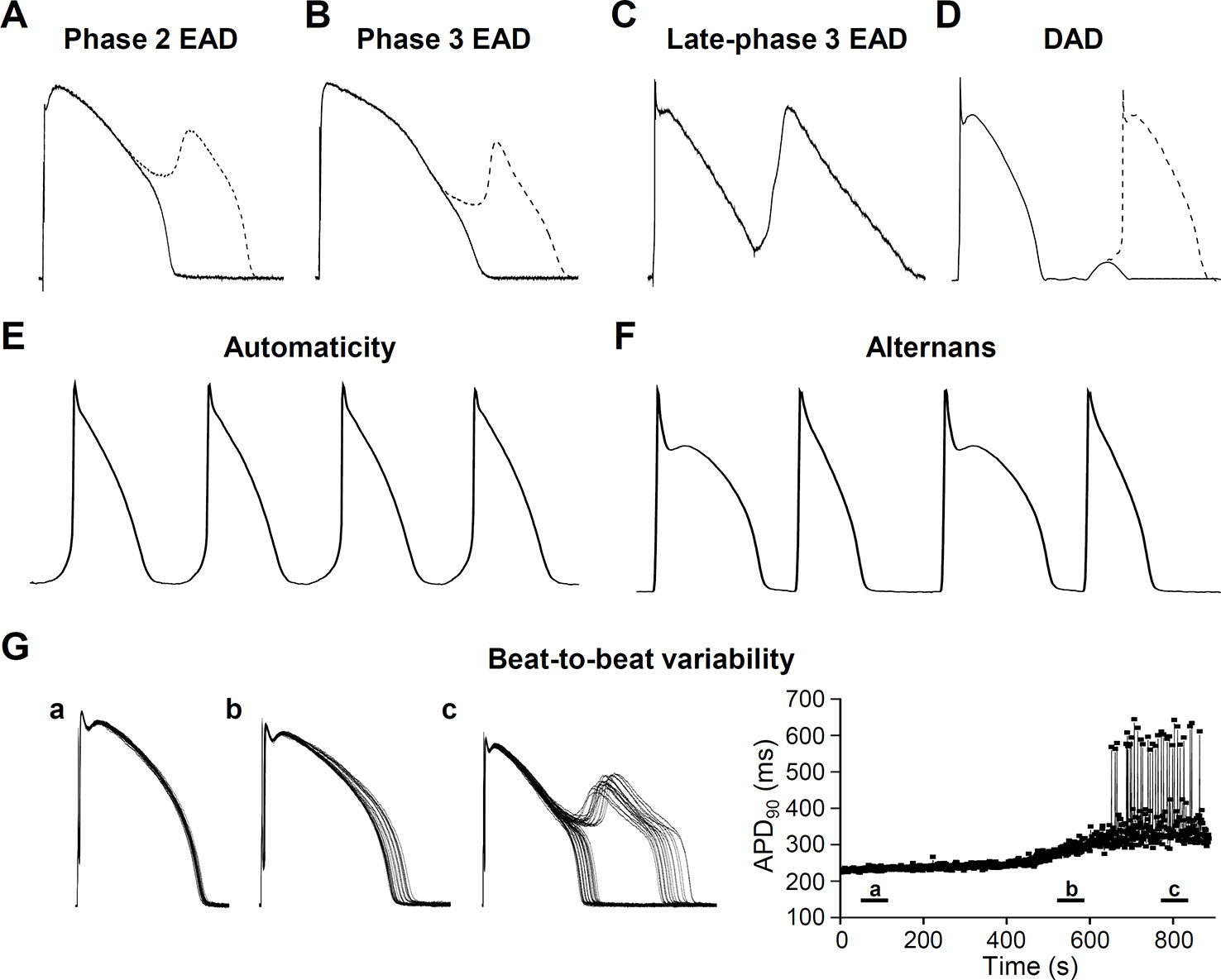
Figure 3 Cellular pathophysiological electrical activities. (A) Phase 2 early afterdepolarization (EAD), (B) Phase 3 EAD, (C) Late-phase 3 EAD, (D) Delayed afterdepolarization (DAD) manifesting triggered activity. Ca2+ has an important role in generating afterdepolarizations. Underlying mechanisms are described in the relevant sections. (E) Automaticity (spontaneous membrane potential oscillations) occurs if the membrane potential of the cells shift to more positive values causing abnormal activity. (F) Cardiac voltage alternans, manifesting a long-short-long-short pattern. (G) Short term beat-to-beat variability of the action potential duration. (a), (b), and (c) show different time points after interventions that increase action potential duration and beat-to-beat variability leading to EAD generation. Right panel of (G) shows action potential duration at 90% of the repolarization (APD90) as a function of time.
Normal cardiac automaticity originates in the sinoatrial (SA) node. If SA node impulse generation is impaired, atrioventricular node (AV node) and Purkinje fibers can show automatic activity. These secondary pacemakers are also called latent or subsidiary pacemakers (Antzelevitch and Burashnikov, 2011). SA node pacemaker activity depends on interactions of membrane potential and [Ca2+]i. This “coupled-clock” pacemaker system is produced by membrane proteins, driving the AP and the intracellular Ca2+ cycling molecules (Figure 4) (Maltsev et al., 2006; Lakatta, 2010; Joung et al., 2011).
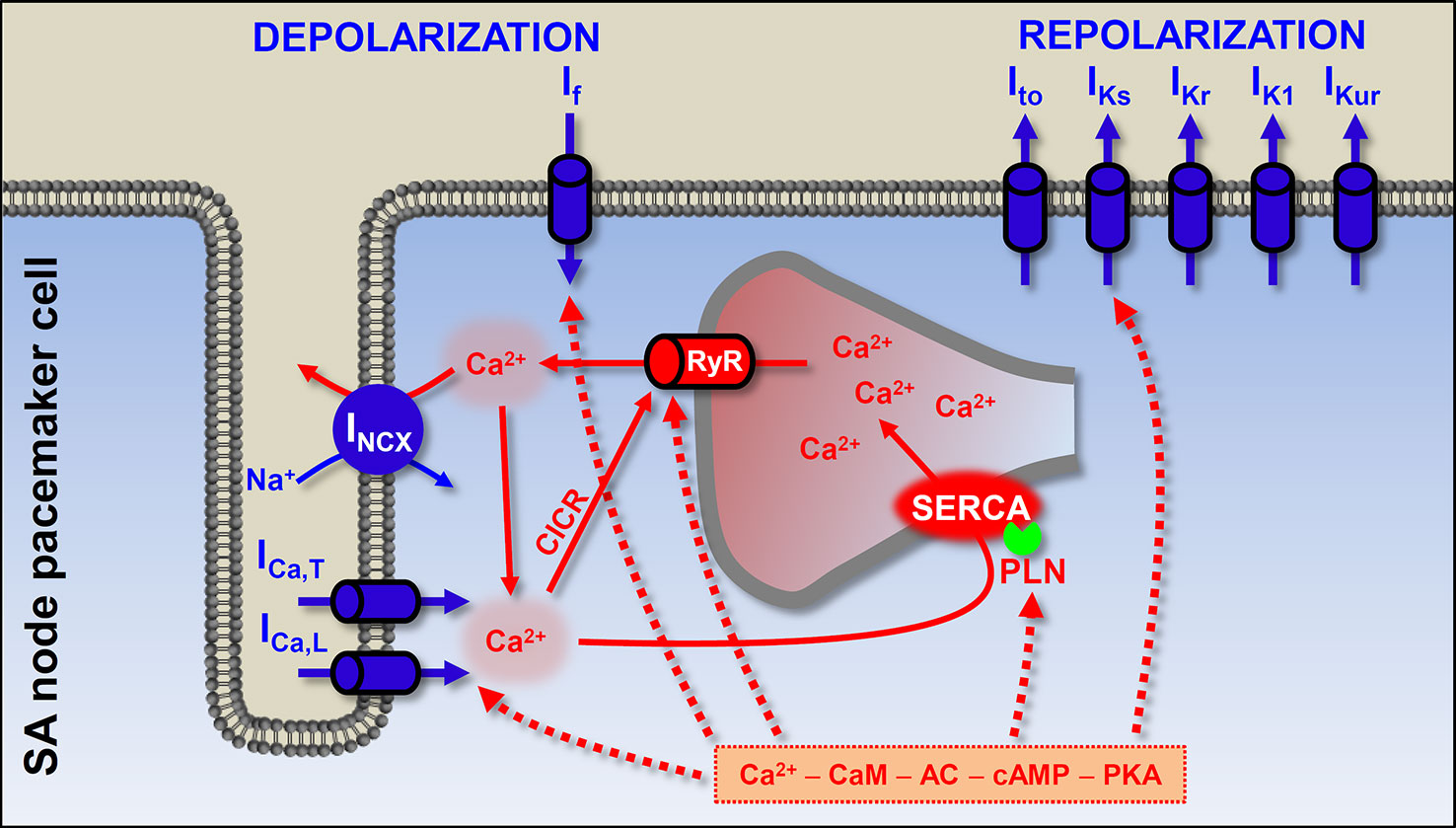
Figure 4 The origin of the heartbeat: coupled-clock pacemaker system in the sinoatrial cells. The pacemaker activity of the SA node originates from the membrane and calcium clock mechanisms. The former is composed of the sarcolemmal channel proteins, and the latter results from sarcoplasmic reticulum and sarcoplasmic Ca2+ turnover. At end of the SA action potential the hyperpolarization-activated If depolarizes the membrane to a level where Ca2+ channels open. In addition, during late diastole, spontaneous SR Ca2+ releases further depolarize the membrane by activating INCX. Ca2+ can bind to calmodulin and activate adenylyl cyclase (AC). High constitutive activation of AC leading to high basal level of cAMP (which is needed for protein kinase A-dependent phosphorylation) in SA node cells has been suggested to contribute to the Ca2+ overload state. PKA-dependent phosphorylation of phospholamban, ICa,L, and RyR promotes spontaneous Ca2+ release. Blue shows the membrane clock and red shows the calcium clock mechanism. Solid arrows show the Ca2+-induced Ca2+ release process and spontaneous Ca2+ release events via RyR; dashed arrows show the phosphorylation targets of the cAMP–PKA pathway. ICa,L, L-type Ca2+ current; ICa,T, T-type Ca2+ current; INCX, Na+-Ca2+ exchange; If, funny current; Ito, transient outward K+ current; IKs, slow component of delayed rectifier K+ current; IKr, rapid component of delayed rectifier K+ current; IK1, inward rectifier K+ current; IKur, ultra rapid component of delayed rectifier K+ current; RyR, ryanodine receptor; SERCA, sarcoplasmic reticulum Ca2+-ATPase; PLN, phospholamban; CaM, calmodulin; AC, adenylyl cyclase; PKA, protein kinase A; CICR, Ca2+-induced Ca2+ release; SA, sinoatrial.
The “membrane clock” implies sarcolemmal proteins, continuously driving the membrane potential to more positive or more negative values. The most important and well-known participant is the hyperpolarization-activated funny current (If), working mainly during early diastolic depolarization. The consequent depolarization opens Ca2+ channels (ICa,T and ICa,L) and the pacemaker (slow type) action potential occurs. As in the case of the working myocardium, K+ currents repolarize the membrane. In the last two decades it has become clear that spontaneous Ca2+ release in a cardiac cell is not always pathological. In the “calcium clock” mechanism, spontaneous SR Ca2+ release events, the Ca2+ sparks activate INCX and cause late diastolic membrane depolarization. Coupled clock pacemaker system comprises functional interactions between the membrane and calcium clock (Figure 4) (Vinogradova et al., 2006; Lakatta and DiFrancesco, 2009; Lakatta et al., 2010).
For physiological contraction and relaxation, not only pacemaker automaticity, but also the impulse conduction system needs to work properly. Spontaneous depolarization from the SA node propagates and depolarizes the distant parts of the cardiac muscle (Figure 2B), via the SA node, AV node, Bundle of His, Bundle branches, and Purkinje fibers pathway.
Cardiac arrhythmia mechanisms can be divided into two main categories: abnormal impulse formation and abnormal impulse conduction. In general, these arrhythmic events occur when the electrical activity of the heart is slower or faster than normal and/or becomes irregular.
Abnormal Impulse Generation
Focal activity (enhanced or abnormal impulse generation) is an important arrhythmogenic mechanism and consists of abnormal automaticity and triggered activity.
Automaticity
In the normal human heart, the SA node generates the propagating APs and determine the heart rate. In the case of parasystole, when the primary pacemaker is bordered by ischemic, infarcted regions the impulse cannot leave the SA node. Under these conditions, parasystolic pacemakers can take over pacemaker activity and fire APs at a lower rate compared to that of the SA node (Gussak et al., 2003). The AV node produces a junctional rhythm of 40 to 60 bpm and Purkinje fibers of about 20 to 40 bpm (Tse, 2016). In diseased hearts (e.g. heart failure, HF) membrane potential of pacemaker cells can shift to more positive values and this depolarization causes abnormal automaticity. Enhanced activity (i.e. tachycardia) increases rate of AP discharge by three mechanisms: threshold potential shifts to more negative, maximum diastolic potential shifts to more positive, and the rate of phase 4 depolarization increases (Figure 3E) (Jalife et al., 2009).
Early Afterdepolarization
Aside from the abnormal automaticity, the most common causes of focal activity are the early and delayed afterdepolarizations (EAD and DAD, respectively). EADs occur before the terminal repolarization (phase 2 and phase 3 repolarization) of the AP, while DADs occur after the repolarization when membrane potential reaches the resting levels (Figure 5).
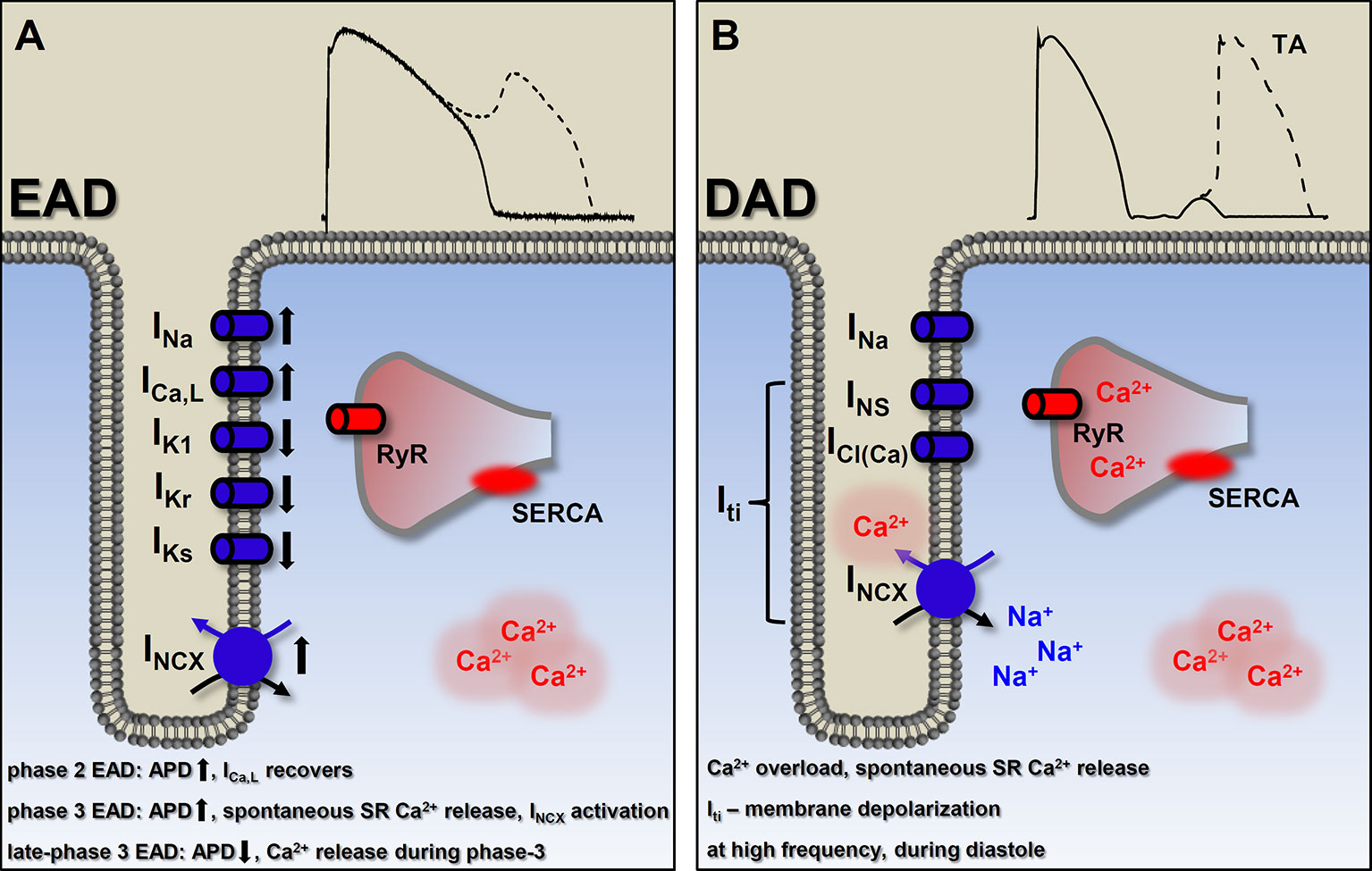
Figure 5 Basic mechanisms of ectopic activity. (A) Factors involved in the generation of early afterdepolarizations (EAD). In general, EADs occur when outward currents are reduced (reduced repolarization reserve) and/or the inward currents are enhanced. The currently known types of EADs are consequencies of different etiologies, indicated on (A). Detailed description in the text. Membrane potential recording shows a typical phase 2 EAD. (B) Delayed afterdepolarizations (DAD) originate from Ca2+ overload and consequently, spontaneous SR Ca2+ release which, in turn, generates a depolarizing transient inward (Iti) current. Suprathreshold depolarization can elicit triggered activity. Membrane potential recording shows a typical DAD. EAD, early afterdepolarization; DAD, delayed afterdepolarization; ICa,L, L-type Ca2+ current; INa, Na+ current; IKs, slow component of delayed rectifier K+ current; IKr, rapid component of delayed rectifier K+ current; IK1, inward rectifier K+ current; INCX, Na+-Ca2+ exchange; INS, nonselective Ca2+-sensitive cationic currents; ICl(Ca), Ca2+-activated chloride current; Iti, transient outward current; RyR, ryanodine receptor; SR, sarcoplasmic reticulum; SERCA, sarcoplasmic reticulum Ca2+-ATPase; TA, triggered activity.
EADs usually occur when repolarization reserve is compromised, i.e. reduced outward currents (IK1, IKr, IKs) and/or increased inward currents (INa, window ICa,L, INCX) (Damiano and Rosen, 1984; Sipido et al., 2007; Benitah et al., 2010; Horvath et al., 2015; Karagueuzian et al., 2017), that is, there is a change in the net membrane current during the plateau (Figure 5A). In most of the cases these conditions cause prolongation of the AP, allowing ICa,L to recover from inactivation (Chiamvimonvat et al., 2017) and as a positive feedback loop, triggering an AP (January and Riddle, 1989) (Figure 3A). Alternatively, at membrane potentials negative to the activation threshold for ICa,L, spontaneous Ca2+ release from the SR can activate INCX, driving a depolarizing current by reactivating INa (Figure 3B) (Szabo et al., 1994). In addition, although EADs usually occur when the AP duration (APD) is prolonged, some data suggests a novel mechanism, where even shortening of APD can be responsible for generation of EADs (late-phase 3 EAD) (Burashnikov and Antzelevitch, 2003). Late-phase 3 EADs occur particularly under elevated intracellular Ca2+ loading (i.e. large Ca2+ transient) and are considered as a hybrid between EAD and DAD (Figure 3C). At normal APD and at membrane potentials negative to the equilibrium of the INCX (and ICl(Ca)), these Ca2+-mediated currents are weakly inward. However, if APD is abbreviated, they become strongly inward, allowing an INCX-driven depolarizing current, when the shorter repolarization allows a stronger (and not spontaneous) Ca2+ release from the SR (Burashnikov and Antzelevitch, 2006). The EAD generated under these circumstances interrupts the final phase of the AP. A key difference compared to the previously described EADs (and DADs) is a non-spontaneous Ca2+ release in generating late-phase 3 EADs (Figure 5). Late-phase 3 EAD also has clinical relevance, as its appearance is immediately following termination of other tachyarrhythmias, such as atrial flutter and fibrillation or ventricular tachycardia and fibrillation (Burashnikov and Antzelevitch, 2006).
The contribution of spontaneous SR Ca2+ release and an inward INCX to the generation of EADs has been described (Priori and Corr, 1990; Volders et al., 1997), furthermore, Volders et al. elegantly demonstrated in isoproterenol induced canine ventricular myocytes that early Ca2+ aftertransients and their aftercontractions precede the upstroke of the subsequent EAD so that they are a primary event inducing EADs (Volders et al., 1997). The time course of the EAD generation is characterized by a conditional phase (in other words, an initial delay in repolarization, defined by net membrane current) and the EAD upstroke. In this regard, a significant role of INCX has been suggested in the initial delay in repolarization, thus in the conditional phase (Volders et al., 2000).
In previous studies, distinct spatial features of afterdepolarization-associated Ca2+ transients had been shown; i.e. a heterogenous pattern indicating focal, spontaneous SR Ca2+ release in DADs and a homogenous pattern suggesting ICa,L-induced Ca2+ release in EADs (Miura et al., 1993; Miura et al., 1995; De Ferrari et al., 1995). However, it must be noted, under certain circumstances (adrenergic stimulation mediated sudden [Ca2+]i changes), Ca2+ release during an EAD is not governed by sarcolemmal Ca2+ influx, so that it is spontaneous, which resembles as a heterogenous pattern, just like in the case of DADs (Volders et al., 1997).
In our previous work, EADs were evoked by IKr blockade (dofetilide), activation of Na+ current (INa,L) (veratridine), and activation of ICa,L (BAY K8644) at slow pacing rates. Additional application of the Ca2+ chelator BAPTA-AM decreased [Ca2+]i as expected, but either reduced EAD frequency in the presence of dofetilide and veratridine or further increased EAD frequency in the presence of BAY K8644 (direct augmentation of the ICa,L brings extra Ca2+ inflow and is a substrate for increased EAD likelihood). Since BAPTA-AM decreased EAD frequency in the presence of veratridine, but failed to shorten APD, these results contradicts the exclusive role of APD in EAD generation and indicate that an increase in [Ca2+]i is a significant factor not only for generating DADs, but for evoking EADs as well (Horvath et al., 2015). Moreover, in another set of experiments of Kistamas et al. H2O2 significantly increased APD and relative short term beat-to-beat variability (SV) (Kistamas et al., 2015a) and increased the occurrence of EADs on canine ventricular myocytes. Elevation of [Ca2+]i in H2O2 was shown by others which can account for the increased SV and EAD incidence (Goldhaber, 1996; Xie et al., 2009; Szentandrassy et al., 2015; Kistamas et al., 2015). Furthermore, we also showed in guinea pig cardiomyocytes, that spontaneous Ca2+ release from the SR mediates (INa,L) induced EADs (Horvath et al., 2013). The two possible mechanisms proposed by Zaza et al. by which INa,L promotes EAD genesis are (1) the reactivation of ICa,L during the plateau phase of AP and (2) SR Ca2+ overload (Zaza et al., 2008). In our experiments the first EAD occurred at a membrane potential more positive than the window Ca2+ current voltage range, meaning that not the reactivation of ICa,L was responsible for the generation of EADs. In fact, several mechanisms were addressed, showing the SR load was key in formation of the EADs: (a) anemone toxin II (ATX-II) facilitates INa,L that caused elevated systolic Ca2+ transient and SR load, (b) the spontaneous Ca2+ wave precedes the first EAD, and (c) Ca2+ buffering with BAPTA in the patch pipette abolished EADs (Horvath et al., 2013).
Therefore, our recent knowledge about the factors involved in the development of EADs includes changes in [Ca2+]i and the amplitude of Ca2+ transient, along with the APD and beat-to-beat variability of APD, AP morphology and plateau potential, net membrane current, and the actual availability of L-type Ca2+ channels. Regardless of the type of EAD mechanisms, if the depolarizing effect of the EAD on the membrane potential is sufficient to activate INa, the result will be an abnormal impulse generation, triggered activity (Hoffman and Rosen, 1981).
EADs are more likely to develop in midmyocardial cells and Purkinje fibers than in subepi- or subendocardial cells. There is a difference in ion current composition (less IKs, more INa,L in midmyocardial cells), consequently these regions are more prone to AP prolongation (Liu and Antzelevitch, 1995; Zygmunt et al., 2001; Szabo et al., 2005). EADs are generally observed under conditions of ventricular hypertrophy and HF, injured cardiac tissue, or when the myocardium is exposed to catecholamines, hypoxia, acidosis, and pharmacologic agents (Roden, 2004; Roden, 2006). The clinical significance of EADs is clear, as they can either serve as the trigger or as the substrate for initiation and perpetuation of torsade de pointes arrhythmia (Volders et al., 2000). Being as a trigger, as EADs can cause new APs which will be reflected on the ECG as ectopic beats. EADs provide a substrate by causing electrical inhomogeneity in the surrounding tissues.
Delayed Afterdepolarization
DADs are the other common causes of focal activity and were originally described as oscillatory afterpotentials (Ferrier et al., 1973). They occur in diastole, after complete repolarization of the cell (Figure 5B). DADs can originate from intracellular Ca2+ overload that induces spontaneous SR Ca2+ release, resulting in a depolarizing current via forward mode INCX (Mechmann and Pott, 1986). Other nonselective Ca2+-sensitive cationic currents (INS) and chloride current (ICl(Ca)) may also be involved in DAD generation (Asakura et al., 2014). These three depolarizing currents result in a transient inward current (Iti), which is responsible for the membrane depolarization (Figure 3D). Ca2+ overload of the cardiac myocytes can occur in several diseases and also in several experimental conditions, e.g. toxic levels of digitalis (Ferrier et al., 1973; Saunders et al., 1973; Rosen et al., 1973), catecholamines (Wit and Cranefield, 1977; Rozanski and Lipsius, 1985; Priori and Corr, 1990), hypokalemia and hypercalcemia (Tse, 2016), hypertrophy, HF (Aronson, 1981; Vermeulen et al., 1994), and rapid heart rates. The amplitude of the generated DAD depends on the size of the Ca2+ transient and on the properties of INCX and the inward rectifier K+ current (IK1) (Pogwizd et al., 2001; Sung et al., 2006; Maruyama et al., 2010). Subthreshold DADs [appearing as the U wave on the electrocardiogram (ECG)] are small voltage deflections, which although unable to trigger a propagating action potential, may still cause dispersion of excitability, thereby promoting regional conduction block (Rosen et al., 1975; Surawicz, 1998; di Bernardo and Murray, 2002). However, if DADs reach the threshold potential for the opening of Na+ channels, a spontaneous AP emerges and can result in premature ventricular contraction (PVC). The clinical significance of DAD generation lies in triggered activity that contributes to arrhythmogenesis with catecholaminergic polymorphic ventricular tachycardia (CPVT), atrial fibrillation (AF), and HF. In CPVT and HF, intracellular Ca2+ load combines with RyR dysfunction (“leaky” RyR). Under circumstances when the SR becomes loaded (high Ca2+ load, fast heart rate, and/or increased adrenergic tone) and/or RyR becomes leaky, spontaneous Ca2+ release is favored.
Considering the mechanism of the spontaneous Ca2+ release, there are two main patterns. First, focal Ca2+ release, when Ca2+ signal acts locally (Lipp and Niggli, 1994) and secondly, when the released Ca2+ leaves its focus and propagates as a global Ca2+ wave through the myocyte (Takamatsu and Wier, 1990; Wier et al., 1987; Cheng et al., 1993).
Unlike the EADs, DADs are always generated at relatively rapid rates (Antzelevitch and Burashnikov, 2011). As mentioned earlier, late-phase 3 EADs are considered as a hybrid between EAD and DAD. A key difference is the time of the SR Ca2+ release during the AP (Figure 5). Ca2+ release occurs during diastole in the case of DAD, while late-phase 3 EAD is generated at the late repolarization of the AP (Fink and Noble, 2010).
Beat-To-Beat Variability of Action Potential Duration
Variations (physiological or pathological) in AP configuration can cause disturbances in Ca2+ signaling and the electrical properties of cardiac muscle. In our previous experiments, we determined the beat-to-beat variability of AP duration in isolated canine left ventricular myocytes in several experimental settings (Kistamas et al., 2015a; Kistamas et al., 2015b; Szentandrassy et al., 2015; Magyar et al., 2016), as recent studies suggest the short term beat-to-beat variability (SV) of APD as a novel method for predicting imminent cardiac arrhythmias (Thomsen et al., 2004; Abi-Gerges et al., 2010). Higher variability is considered to be arrhythmic by increasing dispersion of refractoriness (Figure 3G). We established the concept of relative short term beat-to-beat variability of APD (RSV) by normalizing the changes of short term variability of APD to the concomitant changes in APD [see (Nanasi et al., 2017] for review). We summarized that RSV was decreased by ion currents involved in the negative feedback regulation of APD (ICa,L, IKs and IKr), while it was increased by INa and Ito, and in general, increased if repolarization reserved was compromised. RSV was also increased at faster rates and at increased [Ca2+]i. Transient changes of [Ca2+]i due to Ca2+ released from the SR were the dominant contributor to this process (Kistamas et al., 2015b). High RSV at faster rates can also be explained by the elevated [Ca2+]i, as faster pacing increases ICa,L, ultimately overloading the cell with Ca2+ which, in turn, increases RSV.
Cardiac Alternans
A severe form of this beat-to-beat variation is cardiac alternans, where short and long AP duration alternate (Figure 3F). Pulse and T-wave alternans can be clinically observed and are considered to be a precursor for cardiac arrhythmias (Rosenbaum et al., 1994; Verrier et al., 2011). Cardiac alternans originates from instabilities of membrane voltage or of Ca2+ cycling. At the cellular level, alternans is manifested as beat-to-beat alternations in contraction amplitude (mechanical alternans), APD (electrical or APD alternans), and Ca2+ transient amplitude (Ca2+ alternans) at constant heart rate. However, because of the bidirectional information flow between membrane voltage and Ca2+ cycling, electrical alternans is always influenced by Ca2+ alternans, and vice versa (Weiss et al., 2006).
Two mechanisms have been described for Ca2+-driven alternans. One depends on the relationship between SR Ca2+ content and the amount of Ca2+ released from the SR (Eisner et al., 2000). If this relationship is steep then a small increase of SR Ca2+ content will produce a large increase of the amplitude of the Ca2+ transient resulting in increased Ca2+ efflux via INCX and a decreased influx via ICa,L (Ca2+-dependent inactivation). The net result is a decrease of SR Ca2+ content. The next beat therefore arises from a depleted SR resulting in a smaller Ca2+ transient and decreased INCX, so that the cell will gain Ca2+ resulting in a larger SR content and Ca2+ transient for the third beat (Eisner et al., 2006). Later, it was shown that reduced SERCA pump activity is also needed for an alternating pattern to develop (Shiferaw et al., 2003; Qu et al., 2007; Xie et al., 2008; Li et al., 2009). Another mechanism for Ca2+-driven alternans has been proposed, when on every beat, the SR load is unchanged, however the released amount of Ca2+ is alternating beat-to-beat. This kind of alternans results from the refractoriness of the RyRs, without the need for SR Ca2+ content alternans (Picht et al., 2006; Shkryl et al., 2012).
Voltage-driven or electrical alternans is determined by APD restitution. Here, the shorter the preceding diastolic interval, the less the APD (Nolasco and Dahlen, 1968). The steeper this relationship, the more likely is alternans to occur. There may be several causes for this APD restitution. The rapid, pacing-induced electrical alternans occurs at fast heart rates (short diastolic intervals, where recovery of ICa,L is crucial, becoming a key factor in regulating the steepness of APD restitution (Mahajan et al., 2008). Another APD alternating mechanism is driven by Ito at slow or normal heart rates and possibly accounts for T-wave alternans in patients with Brugada syndrome (Hopenfeld, 2006). The third type of electrical alternans is mediated by non-inactivating ICa,L with IKs at normal or slow rates and possibly cause T-wave alternans in LQTS patients (Wegener et al., 2008). Electrical, restitution-based alternans has been associated with the breakdown of reentry into ventricular fibrillation (VF). At the tissue level, if cellular alternanses in different regions of the ventricle occur in phase with each other (spatially concordant), T-wave alternanses is observed on the ECG. A more malignant form, the spatially discordant APD alternans, manifesting as QRS alternans on the ECG, causes large dispersion of refractoriness, a substrate for reentry. Spatially discordant alternans is a significant cause of wave break, a phenomenon that is essential to VF (Garfinkel, 2007). It has been shown, that interventions that lower the slope of the APD restitution curve can turn multiwave VF to single-wave monomorphic ventricular tachycardia (VT) (Garfinkel et al., 2000; Wu et al., 2002).
Abnormal Impulse Conduction
Abnormal impulse conduction, i.e. reentry, occurs when the AP fails to terminate and has the ability to re-excite tissue regions which have already recovered. This mechanism can be divided into two main types, one with an obstacle (circus type with anatomical or functional barrier) and the other without an obstacle (phase-2 reentry and reflection). The key difference is in refractoriness. Circus movement reentry travels around an anatomic or functional obstacle and all cells are recovered from inactivation, while cells involved in reflection or phase-2 reentry show large differences in recovery from refractoriness with no obstacle in the way of the reentrant wave. In addition, classic nomenclature distinguishes between microreentry and macroreentry, where the reentrant circuit does not or does appear on the surface ECG, respectively.
The myocardium works as a functional syncytium (Figure 6A). The elemental components of this system are the gap junctions. Gap junctions form channels (comprised of two neighboring connexons) between adjacent cardiomyocytes and allow the cardiac AP to propagate from cell to cell and thereby initiate contraction. However, gap junction channels are unevenly distributed within the cells, expressing a larger portion of channel proteins at the longitudinal ends of the cells than at the transversal, lateral sides (De Maziere and Scheuermann, 1990; Oosthoek et al., 1993). This anisotropy allows a much larger longitudinal conduction velocity and effective electrical coupling between the adjacent cells (Figure 6B). Several conditions are reported to reduce or abolish gap junctional conductance, including increased [Ca2+]i, reduced pH, or lower ATP levels (Dhein, 1998). Uncoupling of the cells may lead to the formation of unidirectional conduction block and reentry type arrhythmias (Figure 6B). The hypothesis that Ca2+ overload conditions have arrhythmogenic behavior is also supported by experiments in neonatal rat myocytes, where gap junctional conductance was decreased by Ca2+ concentrations higher than physiological (Firek and Weingart, 1995), while it was proposed that elevation of [Ca2+]i by Ca2+ entry was more effective in decreasing gap junctional conductance than Ca2+ released from internal stores (Lazrak et al., 1994; Chanson et al., 1999). Furthermore, adequate coupling between the cells in the tissue (i.e. low longitudinal resistance) can suppress differences in APD, eliminate EADs, and reduce beat-to-beat variability (Magyar et al., 2015).
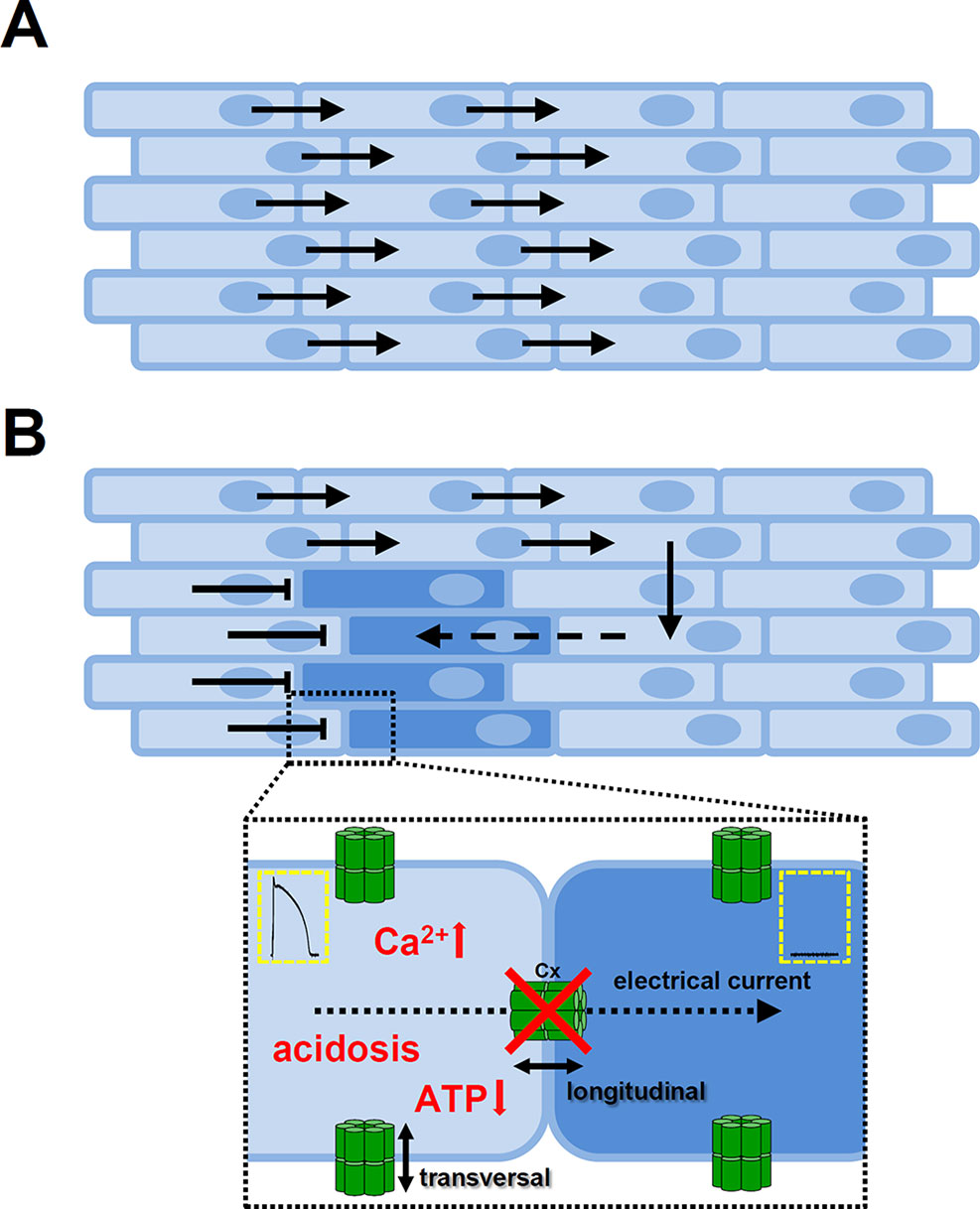
Figure 6 Role of gap junctions in propagating of the cardiac action potential. (A) The cardiac tissue is eletrically homogenous if the adjacent cells are coupled by gap junction channels. The anisotropic nature of gap junction channel distribution favors longitudinal over transversal conduction. (B) Conditions that decrease or abolish coupling between the cells may cause a unidirectional conduction block and as the electrical impulse propagates around the block it can re-excite those tissue regions due to differences in refractoriness. Insert shows cell-cell connections via gap junction channels. The main causes of uncoupling of the cells (showed in red) are elevated intracellular Ca2+ concentration, reduction in H+ concentration, or lower levels of ATP. Cx, connexon; ATP, adenosine triphosphate.
In the subsequent sections reentry types are discussed in detail.
Reentry With Anatomical Obstacle (Ring Model)
Reentry was first described in 1906 by Mayer in rings of tissue cut from jellyfish (ring model) (Mayer, 1906). Later work by Mines showed that circus-type reentry can be initiated by electrical stimulation in cardiac muscle and was the first to define the concept of circus movement reentry around an anatomical obstacle (Figure 7A) (Mines, 1913; Mines, 1914). The anatomical barrier can be a valve, vessel or scar. The possibility that circus-type reentry can form without an anatomical obstacle was proposed by Garrey (1914).
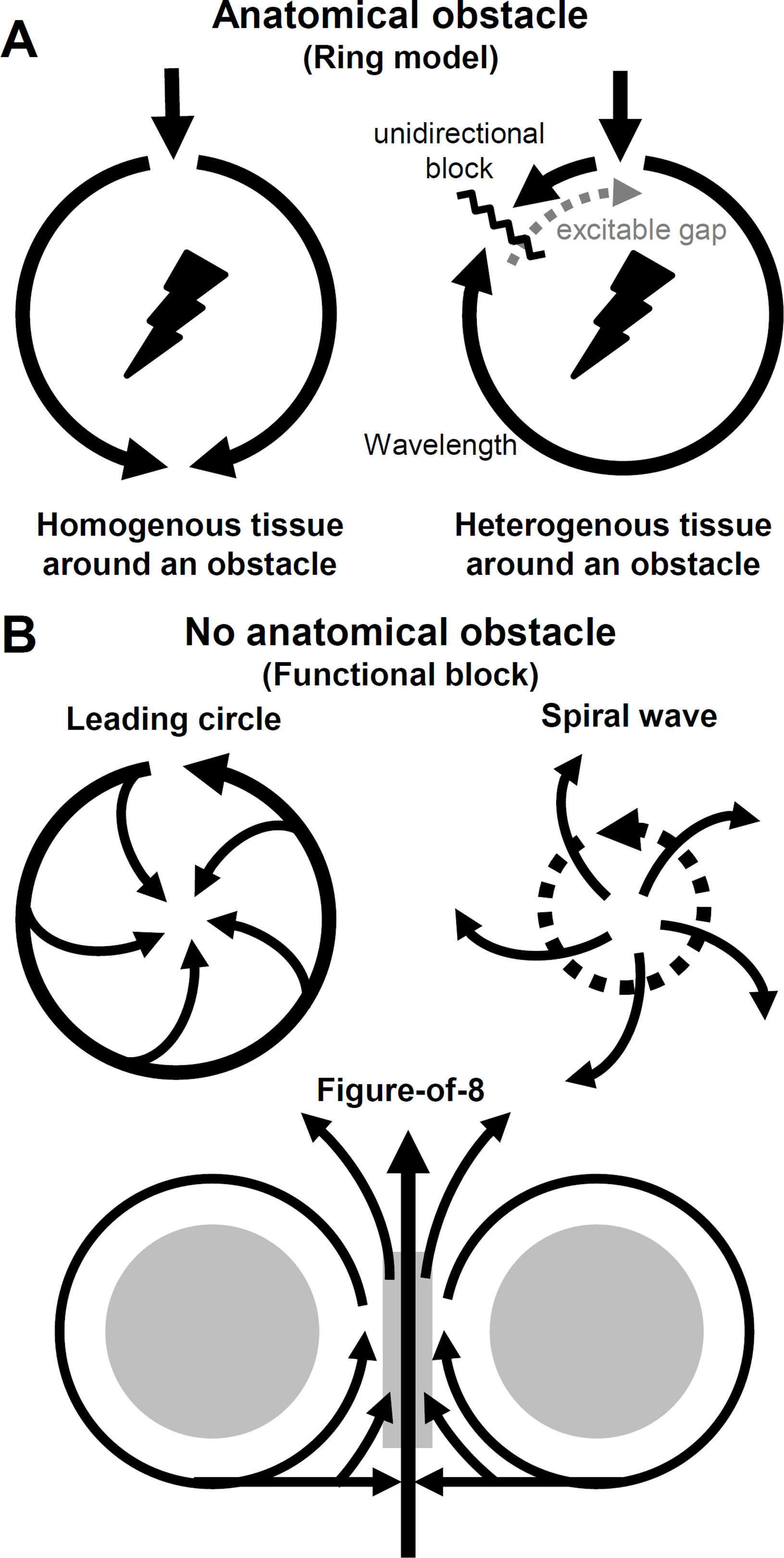
Figure 7 Abnormal impulse conduction. Circus movement reentry types. (A) Reentrant wave travels around an anatomical obstacle. If the cardiac tissue around the obstacle is homogenous the impulse conduction is favored in both directions. However, if the tissue is heterogenous (i.e. dispersion of refractoriness), unidirectional block can form initating a reentry circuit. Excitable gap consists of tissue regions that fully and/or partially recovered from refractory period, therefore excitable. (B) Circuit movement reentry can form in the absence of an anatomical obstacle (functional block). In the leading circle model the length of the circuit is not determined by the pathway around an obstacle, but rather by conduction velocity, refractory period, and stimulating efficacy where (in the absence of an obstacle) a shortcut of the circuit is possible. Spiral waves reentry (or scroll wave if three-dimensional) drifts through the tissue without an obstacle and the main wave can break up and radiate waves to the neighboring regions. In the model of figure-of-8 the circulating waves appear in pairs and the wavefront can circulate around the functional blocks clockwise and counterclockwise. If the intermediate area (central gray) can be activated by the colliding separated waves, reentry can form.
Initiation of reentry requires a trigger and a substrate. The trigger can be a premature contraction, while tissue substrate is the dispersion of refractoriness. On top of that, fundamental settings are needed for reentry excitation with anatomical obstacle: (1) the impulse initiating the circus movement must propagate in one direction (unidirectional block) and (2) the proportion of absolute and relative refractoriness in the tissue, that is, the reentrant circuit must be long enough to let all areas—within the circuit, distal from the stimulus—recover from refractory (excitable gap), so the circuit can return to its origin and continue as a new cycle (Figure 7A). Consequently, (3) the circulating movement would terminate in case of interruption of the reentrant circuit (Mines, 1913). These criteria proposed by Mines are still in use today. The above mentioned excitation is, in fact, a propagating wave. The length of this wave (wavelength) is determined by the distance between the wavefront (phase 0, AP depolarization) and waveback (phase 3, repolarization), that is, creating an arrhythmogenic excitation needs the special properties of refractoriness and conduction velocity (Weiss et al., 2000). If the above three criteria are not met, i.e. in sinus rhythm if the tissue around the anatomical obstacle is homogenous (and the impulse pathway is wide enough), the wavefront can simultaneously propagate in both pathways around the barrier. However, if the tissue is electrically heterogenous, due to dispersion of refractoriness, unidirectional conduction block can form caused by a PVC, i.e. initiating reentry (Figure 7A).
Reentry Without Anatomical Obstacle (Functional Block)
In the cases, when there is no anatomical barrier present, functional reentry can still form, maintained only by the electrical properties (dispersion of refractoriness) of the tissue. The best known examples are the leading circle, spiral wave, and figure-of-8 reentry (Figure 7B).
The leading circle model was described by Allessie et al., as “the head of the circulating wavefront is continuously biting in its own tail of refractoriness” (Allessie et al., 1977). The main differences compared to the ring model are (1) the length of the circuit is determined by conduction velocity, stimulating efficacy, and refractory period not by an anatomic obstacle, (2) while the length of the circuit is not fixed, it can be altered by changes in electrophysiological properties of the tissue. (3) There is no excitable gap in the leading circle model and (4) a shortcut of the circuit is possible and finally (5) revolution time is proportional to refractory period, while in the ring model, revolution time is inversely related to conduction velocity (Figure 7B) (Allessie et al., 1977).
Spiral waves and rotors can be induced in small two-dimensional pieces of cardiac muscle, without an anatomical barrier, and can drift through the tissue (Pertsov et al., 1993). Scroll waves are the three-dimensional forms of spiral waves. Spiral waves can develop both in homogenous and heterogenous tissues and either in stable or in an unstable form (Ikeda et al., 1996; Davidenko et al., 1992). The former might result in monomorphic VT, while the latter can cause polymorphic VT or torsade de pointes (Figure 7B) (Gray et al., 1995).
Figure-of-8 type reentry was first demonstrated by el-Sherif et al. In this case the reentrant wavefront reaches a functional conduction block surrounded by regions of reduced excitability. As conduction is not favored through such tissue, the wavefront drives clockwise and counterclockwise around the two arcs of functional block and beyond the barriers of low excitability the two separated waves can collide. If the conduction is slow enough and the intermediate area can be activated, reentry can form (Figure 7B) (el-Sherif et al., 1985; Lazzara, 1988).
Phase-2 Reentry
In the previous reentrant mechanisms, the trigger and the substrate originated from different etiologies, while in the case of phase-2 reentry, trigger and substrate are from the same source. Phase-2 reentry occurs in ischemia (Lukas and Antzelevitch, 1996), Brugada syndrome (Brugada and Brugada, 1992) or under conditions of higher pacing rates and higher extracellular Ca2+ concentration (Di Diego and Antzelevitch, 1994). It is caused by severe spatial dispersion of repolarization, that is, spike-and-dome configuration of AP morphology is lost at one site (predominantly at the epicardial region), while preserved at another site and is responsible for the transition to VT and VF. APs without the dome (short APD, early repolarization) can therefore be reexcited and reentry can be initiated (Antzelevitch, 2007). Loss of dome can be explained by a stronger transient outward current (Ito) current, and overall by the competitive behavior between INa and Ito (Greenstein et al., 2000; Szabo et al., 2005; Dong et al., 2010). If the actual membrane potential value is more negative than the activation threshold for the ICa,L then the AP dome vanishes. Cantalapiedra et al. showed in a simplified ionic and in a realistic cardiac model, that the origin of reexcitation is based on the presence of slow Ca2+ pulse, produced by the slow inward Ca2+ current (Isi), so that the slow pulse propagates to the regions of short APs until it triggers a fast pulse (Cantalapiedra et al., 2010). Interestingly, the same research group argued that conditions (e.g. drugs) increasing the ICa,L, to recover the dome or to prevent the loss of dome, decreases dispersion of repolarization, however, also increasing the probability of reexcitation, through the stabilizing effect of the Ca2+ conductance (ICa,L) on the slow Ca2+ pulse (Cantalapiedra et al., 2009).
Reflection
Reflection is another example of non-circus movement reentry, with a one-dimensional behavior and can be the cause of PVCs or even lethal arrhythmias (Wit et al., 1972; Rosenthal, 1988; Van Hemel et al., 1988). Reflection describes reentry in a linear bundle of a conductive tissue. A stimulus from the proximal region travels through an inexcitable gap and elicits an AP at the distal end. Slow electrotonic currents (inexcitable region can only transmit electrotonic currents) generated by this AP can then propagate in the retrograde direction and reenter and reexcite the proximal elements (Antzelevitch et al., 1980). There must be an adequate conduction delay to let reflection happen (proximal end can recover from refractoriness), depending on the pacing interval and stimulus strength. It was also shown that neither EADs nor automaticity was required for reflection (Cabo and Barr, 1992; Kandel and Roth, 2015).
Biexcitability
A novel wave dynamic, termed biexcitability has been described in recent studies (Chang et al., 2012). In pacemaker regions ICa,L causes the activation, while in working muscle cells, the upstroke of the AP is driven by INa and ICa,L. During biexcitability both form of activation can coexist at the same tissue. Under certain conditions, like long QT syndrome, repolarization reserve is compromised, APD prolongs, and EADs can occur. Consequently, there can be a situation where the cells develop two stable membrane potential values (−80 mV and −50 mV) and switches between them (Gadsby and Cranefield, 1977), resulting in a Na+- and Ca2+-mediated (fast) or a Ca2+-mediated (slow) propagating wavefront. This bi-stable behavior might serve as an explanation for the two different possible outcomes of torsade de pointes. According to Chang et al., in cases where the Ca2+-mediated slow spiral wave is terminated, leads to termination of the torsade de pointes, while if the tissue is sufficiently heterogenous, Na+ and Ca2+-mediated fast spiral waves degenerate torsade de pointes to VF (Chang et al., 2012; Chang et al., 2013).
DADs can induce focal VT by DAD-mediated triggered activity or initiate reentry. Moreover, unstable Ca2+ signaling can dynamically serve as a substrate for reentry, by promoting dispersion of excitability or promoting dispersion of refractoriness (Weiss et al., 2015). In those tissue regions, where subthreshold DADs do not trigger a propagating AP, the resultant small membrane depolarization can still be sufficient to depress excitability by inactivating the fast voltage gated Na+ channels. This condition can lead to reentry, as the inactivated Na+ channels form a regional conduction block for impulses generated by suprathreshold DADs (Rosen et al., 1975; Liu et al., 2015). In the latter case, DAD-mediated triggered activity at fast rates can promote Ca2+ transient alternans, which in turn causes APD alternans, thereby increasing the dispersion of refractoriness (Sato et al., 2006; Weiss et al., 2006). As previously mentioned, subthreshold EADs can also enhance the dispersion of refractoriness, also creating a reentry substrate.
For more detailed reviews on conduction disorders, see Qu and Weiss (2015) and Antzelevitch and Burashnikov (2011).
The following sections will provide further insights into intracellular Ca2+ handling maladies in the most prevalent inherited and acquired arrhythmia syndromes, caused by channelopathies and defects in Ca2+ handling genes. Ca2+ handling defects also have an arrhythmogenic role in diseases, such as heart failure and cardiomyopathies, however they are beyond the scope of the present review [see recent reviews (Coppini et al., 2018; Johnson and Antoons, 2018; Denham et al., 2018)].
Inherited Syndromes
Catecholaminergic Polymorphic Ventricular Tachycardia
Catecholaminergic Polymorphic Ventricular Tachycardia (CPVT) is a severe arrhythmogenic disorder, manifesting as a bidirectional or polymorphic VT, mainly in young patients with structurally healthy hearts after exercise or acute emotional stress (Reid et al., 1975). As heart rate increases as a result of exercise or emotional stress, the ectopic ventricular trigger increases in complexity, such that VT turns into VF and may lead to syncope or sudden cardiac death (Coumel, 1978; Leenhardt et al., 1995).
The main criteria for CPVT diagnosis are as follows: structurally normal heart (and normal coronary arteries in individuals above 40 years of age), normal QT interval, and adrenergic induced bidirectional or polymorphic VT (Venetucci et al., 2012). CPVT is also diagnosed in patients who carry a pathogenic mutation and in family members of a CPVT index case, fulfilling the above mentioned criteria (Priori et al., 2013). There are also nonspecific features, therefore not diagnostic criteria, including a prominent U wave on the ECG accompanied by sinus bradycardia (Postma et al., 2005).
In CPVT, arrhythmias are induced by Ca2+ release from the SR leading to a DAD. The fundamental feature of this process is the Ca2+ release unit (Ca2+ sparks), where the spontaneous Ca2+ release occurs. If sufficient number of release units are activated, a Ca2+ wave is born, which depends on the SR Ca2+ content and the SR Ca2+ threshold (Lukyanenko et al., 1999; Venetucci et al., 2007). Interventions that alter RyR opening will affect SR Ca2+ threshold. For example, caffeine increases the open probability of RyR, therefore it is easier to elicit spontaneous Ca2+ release (Trafford et al., 2000), on the other hand tetracaine has an opposite effect, by reducing RyR opening, SR Ca2+ release threshold is higher (Overend et al., 1997; Venetucci et al., 2006).
In the previous sections we detailed the normal Ca2+ cycling and consequences of elevated [Ca2+]i. Briefly, the main arrhythmogenic mechanism in CPVT is due to SR Ca2+ release increasing cytoplasmic Ca2+ levels, NCX exchanges Ca2+ with Na+, thereby generating Iti. Iti produces DADs and if DADs reach the activation threshold of Na+ channels, an elicited AP causes triggered activity, which in turn can lead to an extrasystolic heartbeat. Mutations in CPVT have been shown to alter RyR function and increase the occurrence of spontaneous Ca2+ release events after sympathetic stimulation (Liu et al., 2006). β-adrenergic activation increases SR Ca2+ content, while the same process enhances RyR phosphorylation by Ca2+/calmodulin-dependent protein kinase II (CaMKII) and protein kinase A (PKA) (Kashimura et al., 2010; Liu et al., 2011a; Venetucci et al., 2012). In addition to the phosphorylation by PKA, CaMKII-mediated phosphorylation increases the ICa,L and SERCA (by removing the inhibitory effect of phospholamban on SERCA) and activates RyR. Simultaneous activation of ICa,L, SERCA (increases SR Ca2+ content), and RyR therefore increases the possibility of spontaneous Ca2+ release (Maier and Bers, 2007; Hegyi et al., 2019). Experimental data confirmed that higher RyR Ca2+ sensitivity alone is not sufficient to elicit spontaneous Ca2+ release and that inhibition of CaMKII in a CPVT mouse model prevents arrhythmias (Venetucci et al., 2007; Liu et al., 2011a).
Several CPVT subtypes have been described to date, albeit the two most common types are the CPVT-1 and CPVT-2 (Table 1).
CPVT-1 is caused by an autosomal dominant mutation in the RyR2 gene (Swan et al., 1999). This subtype is the most common, accountable for about 60% of all CPVT cases (Laitinen et al., 2001; Priori et al., 2001). RyR exists as a macromolecular complex with many other molecules, such as calsequestrin 2 (CSQ2), FK506 binding protein 1B (FKBP1B or FKBP12.6), FK506 binding protein 1B (FKBP1B or FKBP12.6), PKA, CaMKII, phosphatase 1 (PP1), phosphatase 1 (PP1), phosphatase 2A (PP2A), histidine-rich Ca2+ binding protein (HRC), junctin and triadin (Wang et al., 1998; George et al., 2007; Yano et al., 2009; Arvanitis et al., 2011; Szabo et al., 2013). Junctin and triadin mediates interaction between RyR and CSQ2 (Eisner et al., 2017). Most RyR mutations in CPVT are gain-of-function mutations and thereby leading to increased Ca2+ sensitivity and RyR channels may open during diastole causing Ca2+ leak, particularly during adrenergic stress (Jones et al., 2008). Several hypotheses have been advanced to explain this phenomenon, including the role of FKBP12.6, store overload-induced Ca2+ entry (SOCE) and a defective mutation in the RyR 3D conformation (Reiken et al., 2003; Lehnart et al., 2004; Jiang et al., 2005; Yamamoto et al., 2008; Liu et al., 2009; Uchinoumi et al., 2010; Suetomi et al., 2011; Venetucci et al., 2012a).
CPVT-2 is an autosomal recessive gene anomaly in CASQ2-encoded CSQ2 and responsible for about 3–5% of CPVT patients (Lahat et al., 2001). The structure of this intra-SR Ca2+ buffer changes Ca2+ concentration. At low SR Ca2+ concentrations (< 0.6 mmol/L) CSQ2 is a monomer, which is converted to a dimer (0.6–3 mmol/L) or polymer (> 3 mmol/L) at higher Ca2+ concentrations (Mitchell et al., 1988; Wang et al., 1998). It has been shown that, in the absence of functional CSQ2, RyR channels open spontaneously, without the need for L-type Ca2+ current mediated trigger (Knollmann et al., 2006) and that mutation of CSQ2 destabilizes Ca2+ storing capacity of the SR, which in turn alters the Ca2+ sensitivity of RyR (Viatchenko-Karpinski et al., 2004). In all CSQ2 mutations (missense, deleterious, nonsense), level of CSQ2 protein is reduced or absent, perhaps because it is more susceptible to degradation (Rizzi et al., 2008; Faggioni et al., 2012). Impaired polymerization (Bal et al., 2010), reduced RyR binding and modulation (Houle et al., 2004; Terentyev et al., 2006) are generally associated with lower SR Ca2+ content, higher [Ca2+]i and Ca2+ leak through RyR, these effects can be augmented by β-stimulation (Song et al., 2007). An interesting feature of CSQ2 protein reduction is a subsequent reduction in triadin and junctin levels. Denegri et al. showed in CSQ2 knock-out animal model that viral gene transfer for in vivo replacement of CSQ2 restored normal CSQ2 levels along with triadin and junctin, and ultimately prevented arrhythmias (Denegri et al., 2012).
Other, less frequent gene mutations have also been described, such as autosomal recessive forms of CPVT, the CPVT-3 and CPVT-5, while CPVT-4 is an autosomal dominant form of the inherited syndrome. CPVT-3 subtype is related to the gene encoding trans-2,3-enoyl-CoA reductase-like protein (TECRL) and is first seen at an early age with high likelihood of infant sudden cardiac death (Bhuiyan et al., 2007). When CPVT-3 is studied in induced pluripotent stem cell-derived cardiomyocytes (iPSC-CM) slower Ca2+ reuptake, slower Ca2+ transient upstroke velocity, and increased APD has been observed, along with norepinephrine-induced DADs, which could be eliminated by flecainide (see below) (Devalla et al., 2016). Mutations in CALM1-encoded calmodulin (CaM) cause the CPVT-4 subtype. In vitro experiments showed that this gene anomaly in the C domain compromises Ca2+ binding to CaM and impairs interaction between RyR and its CaM-binding domain, leading to an increased open state of RyR (Nyegaard et al., 2012; Sondergaard et al., 2015; Sondergaard et al., 2017). TRDN-encoded triadin mutation results in CPVT-5 subtype, which may cause diastolic Ca2+ leak and Ca2+ overload. Electron microscopy experiments uncovered fragmentation and reduced contact at the dyadic cleft, thus possibly lacking the negative feedback of SR Ca2+ release on the L-type Ca2+ channels, so SR Ca2+ overload may arise from the uncontrolled Ca2+ influx (Chopra et al., 2009).
A possible loss-of-function RyR mutation has also been proposed in a case classified as idiopathic VF, where a reduced SR Ca2+ sensitivity was shown (Jiang et al., 2007). Moreover, exercise induced bidirectional VT has been reported in types of long QT syndromes (LQTS-4 and LQTS-7) (Table 1) (Mohler et al., 2004; Vega et al., 2009).
Because of the hiding nature of the disease, it is difficult to diagnose CPVT, as patients have normal heart structure and show no symptoms before syncope or sudden cardiac death. However, if diagnosed, there are several therapeutic approaches to CPVT.
Generally speaking, life-long administration of β-blockers is the first choice as treatment. Studies showed that nadolol was clinically effective and a useful prophylactic (Priori et al., 2013). In countries, where nadolol is not available, propranolol was also shown to be effective (Hayashi et al., 2009). Carvedilol has been shown to inhibit store overload-induced Ca2+ release (SOICR) and is the only β-blocker to have RyR inhibitory action, albeit it is a less potent β-blocker after all (Zhou et al., 2011). Patients with CPVT are recommended to remove the triggers, in other words to limit or avoid any vigorous physical activities and stressful environments (Priori et al., 2013). In some patients (lacking long-term studies yet) β-blocker and non–dihydropyridine Ca2+-channel blocker (verapamil) combination therapy was shown to be beneficial (Swan et al., 2005; Rosso et al., 2007).
Flecainide administration has been suggested on top of β-blockers to prevent arrhythmias, in CPVT patients refractory to β-blockers alone (Biernacka and Hoffman, 2011; Pott et al., 2011; van der Werf et al., 2011). Flecainide is a Na+-channel blocker drug, specifically a Class Ic antiarrhythmic agent. Several studies, including three retrospective cohorts in human patients with CPVT (Liu et al., 2011; Radwanski et al., 2016; Kannankeril et al., 2017) have shown the effectiveness of flecainide but there is still debate around the mechanism by which it exerts its antiarrhythmic effect. Watanabe et al. concluded that the most important effect of flecainide was blocking the RyR along with the Na+-channel blockade (Watanabe et al., 2009). They hypothesized that blocking RyR reduces the spontaneous Ca2+ release events and therefore DADs, while Na+-channel blockade prevents the possibility of triggered activity from any residual DADs (Hilliard et al., 2010). Of the Class Ic antiarrhythmic drugs, only flecainide and propafenone was shown to inhibit RyR activity (Hwang et al., 2011). On the other hand, Liu et al. showed in an animal model of CPVT that although flecainide prevents VT and triggered activity, spontaneous Ca2+ release and DADs were still detectable in single myocytes. They concluded that the antiarrhythmic effect of flecainide results from its Na+-channel blocker effect rather than via RyR inhibition (Liu et al., 2011b; Bannister et al., 2015). These conflicting results raise the question whether the different effects seen in the previous studies are dependent of a specific genetic mutation. In a recent study, isolated myocytes from Casq2-/- and RyR2R4496C+/- mice were compared (Hwang et al., 2019). It was found that the former produces a stronger proarrhythmic response upon isoproterenol stimulation, but flecainide prevented arrhythmias in both cases. Also independent from the underlying mutation, effect of flecainide decreased at high Ca2+ load. An additional drug has also been tested both in vitro and in vivo. 1,4-benzothiazepine derivative K201 (JTV519) was shown to prevent arrhythmias in mouse models by reducing RyR opening, SERCA activity and ICa,L (Lehnart et al., 2004; Loughrey et al., 2007).
The latest guidelines recommend implantable cardiac defibrillator (ICD) implantation in patients with diagnosis of CPVT who experience VT, syncope, or cardiac arrest despite the optimal medical treatment (Priori et al., 2013). However, the use of ICDs without concomitant use of β-blockers is dangerous because of the possibility of shock-related electrical storms in these patients (Mohamed et al., 2006; Pflaumer and Davis, 2012). Selective left cardiac sympathetic denervation (LCSD) can be a useful therapeutic method and may be considered in patients with uncontrollable arrhythmias (patients with contraindication to β-blockers; when ICD cannot be implanted; or when recurrent VTs manifest in patients with ICD and β-blockers treatment) (Priori et al., 2013). Pulmonary vein isolation (catheter ablation) was reported to be efficient in some patients with CPVT and AF (Sumitomo et al., 2010), while the possibility of gene therapy was suggested after successful adenoviral vector infection (CASQ2 gene) in R33Q knock-in mutant mouse with dysfunctional CSQ2 (Denegri et al., 2014). Family screening of first degree relatives (clinical evaluation and genetic testing) has been strongly suggested with an optional β-blocker therapy even in the absence of a positive exercise test (Bauce et al., 2002; Hayashi et al., 2009).
Congenital Long QT Syndrome
Congenital long QT syndrome (LQTS) is an inherited cardiac ion channelopathy. LQTS is characterized by a prolonged QT interval on the surface ECG, reflecting the ventricular APD prolongation, which gives rise to risk for syncope, seizures, VT or torsade de pointes and finally VF and sudden cardiac death (Schwartz et al., 2012). Prolongation of APD can happen in an inhomogenous pattern, resulting in an enhanced dispersion of repolarization across the tissue. Delay in repolarization can occur e.g. by genetic defects of key ion currents, namely IKs, IKr, or INa. As mentioned in a previous section, EADs can form if the repolarization reserve is compromised, outward currents are reduced and/or inward currents are increased. In the case of LQTS, inhomogeneity of refractoriness combined with EADs establishes the arrhythmia substrate for VT, torsade de pointes.
The above mentioned conditions are illustrated in the cases of LQTS-1, LQTS-2, and LQTS-3. LQTS-1 is caused by the loss-of-function mutation of KCNQ1 gene (Kv7.1) that encodes IKs (Sanguinetti et al., 1996; Barhanin et al., 1996) while LQTS-2 is also a loss-of-function mutation, but of the KCNH2 channel gene (Kv11.1), encoding IKr (Sanguinetti et al., 1995). LQTS-3 is an inherited gain-of-function mutation of SCN5A Na+ channel (Nav1.5) encoding INa (Wang et al., 1995). All three mutations play key role in determining the length of AP and all of them points towards compromised repolarization reserve with decreased outward currents (LQTS1-2) and increased inward current (LQTS-3). LQTS-1–3 account for ~75–85% of the congenital LQTS cases (El-Sherif et al., 2017).
Mutations of several other genes have been described in LQTS patients. Mutations of structural and channel interacting proteins result in: LQTS-4, a loss-of-function mutation of ANK2-encoded ankyrin B and leads to Ca2+ overload, QT prolongation, sinus bradycardia, AF, and CPVT (Bhuiyan et al., 2013; Mohler et al., 2003); LQTS-5, a loss-of-function KCNE1-encoded minK mutation, consequential reduction in IKs (Splawski et al., 1997); LQTS-6, a loss-of-function mutation of KCNE2-encoded MiRP1, causing a faster inactivation time course for IKr, enhanced ICa,L, and reduced If (Lu et al., 2003; Nawathe et al., 2013; Liu et al., 2014); LQTS-9, CAV3-encoded Caveolin 3, causing an enhanced INa,L; and LQTS-11, a mutant A-kinase anchoring protein (AKAP9-Yotiao) results in an abnormal response upon β-stimulation, as mutation reduces interaction between AKAP9 and KvLQT1 cannel α subunit (KCNQ1, IKs) leading to dysfunctional response to cAMP and a prolonged APD (QT) (Chen et al., 2007).
LQTS-9 and LQTS-10 (gain-of-function mutation in SCN4B-encoded Na+ channel Navβ4 β-subunit) together resemble the LQTS-3 phenotype as QT prolongation is achieved by increased Na+ current (Medeiros-Domingo et al., 2007). Mutation of SNTA1-encoded α1-syntrophin is a gain-of-function gene anomaly, causing LQTS-12 by enhancing Na+ current (Nav1.5) (Wu et al., 2008). LQTS-7 and LQTS-13 are affecting repolarizing K+ currents and channels. LQTS-7 or Andersen-Tawil type 1 syndrome is caused by the loss-of-function mutation of the KCNJ2-encoded Kir2.1 inward rectifier K+ channel, responsible for IK1, and as IK1 is an important player in terminal repolarization, reduction of Kir2.1 function prolongs QT interval (Plaster et al., 2001). In LQTS-13, a loss-of-function mutation on KCNJ5-encoded Kir3.4 causes loss of acetylcholine activated, G-protein-gated K+ (IKAch) channel function. IKAch is formed by Kir3.1 and Kir3.4. Mutation in Kir3.4 function disrupts membrane targeting and stability, i.e. reduced membrane expression has been suggested as the cause of LQTS-13 (Yang et al., 2010).
Although most of the LQTS mutant genes are related to K+ and Na+ channels (i.e. LQTS-1–3 being ~75–85% of total congenital LQTS), there are several Ca2+-signaling proteins that are linked to the occurrence of long QT intervals, typically causing LQTS-8, LQTS-14, LQTS-15, LQTS-16, and LQTS-17 (Table 1).
LQTS-8 is a gain-of-function mutation of the CACNA1C-encoded α1C subunit of L-type Ca2+ channel (Cav1.2) and is generally associated with Timothy syndrome. Timothy syndrome is a rare (less than 30 patients reported worldwide), but severe multisystem disorder, involving QT prolongation, syndactyly, congenital heart defects, cardiomyopathies, bradycardia (caused by AV block rather than sinus bradycardia), and autism (Splawski et al., 2004). LQTS-8 mutation of the Cav1.2 leads to (1) a significant reduction in voltage-dependent inactivation of ICa,L, (2) enhanced ICa,L, (3) decreased current density with enhanced window current, and (4) a steeper APD restitution curve (Thiel et al., 2008; Boczek et al., 2015; Landstrom et al., 2016). A lesser inactivation of the steady-state current and/or increased peak current means a higher Ca2+ influx, which can in turn prolong APD, therefore QT interval. A steeper APD restitution curve is proarrhythmic, being a substrate for alternans, as detailed in previous chapters. The mutation can also cause T-wave alternans on the ECG by increasing the dispersion of repolarization (Zhu and Clancy, 2007). In iPSC cells of a Timothy syndrome patient, a cyclin-dependent kinase inhibitor, roscovitine was found to shorten APD by partially recovering inactivation of the mutant channel (Yarotskyy et al., 2010; Yazawa et al., 2011). If Timothy syndrome/LQTS-8 is diagnosed, because of the high mortality, ICD implantation is the first choice. ICD is often supplemented with β-blockers, relying on the fact that they are generally effective in LQTS patients. Also, verapamil (Jacobs et al., 2006), mexiletine (Krause et al., 2011), and ranolazine (Shah et al., 2012) have been shown to shorten APD by affecting ICa,L and reducing the risk of arrhythmias.
LQTS-14–16 are newly described subtypes of LQT syndrome, caused by mutations in the genes coding the ubiquitous Ca2+ sensor and binder, calmodulin (CaM). Mutations in CALM1-encoding CaM1, CALM2-encoding CaM2, and CALM3-encoding CaM3 are responsible for producing LQTS-14, LQTS-15, and LQTS-16, respectively. Patients diagnosed with these conditions are usually young and have a high rate of cardiac arrest with severe QT prolongation (Gray and Behr, 2016). CaM is important in the inactivation of Na+ channels, Ca2+-dependent inactivation of ICa,L and also important in the trafficking, assembly, and gating of the IKs channel, KCNQ1 (Shamgar et al., 2006). Gene anomalies, affecting CaM, and therefore, Ca2+ binding and/or enhancing ICa,L can lead to severe APD prolongation. To date, over 20 mutations have been reported in the disease group of calmodulinopathies (Jensen et al., 2018; Wren et al., 2019) associated with LQTS, CPVT, and idiopathic VF. LQTS mutations, e.g. CaM-D130G, CaM-D96V, CaM-N98S, and CaM-F142L are all having impaired Ca2+ binding properties at the EF hand domains (Crotti et al., 2013). In CaM-D130G, CaM-D96V, and CaM-N98S mutations impaired CaM-dependent inhibition of RyR was reported, thereby increasing SR Ca2+ release due to an increased open state of RyR (Sondergaard et al., 2017; Jensen et al., 2018). Unexpectedly, an LQTS-associated CaM mutation, CaM-F142L did not diminish, but, increased the CaM-dependent RyR gating inhibition and caused faster RyR closing at high [Ca2+]i (Sondergaard et al., 2017). The authors proposed that the mutation displayed both gain-of-function and loss-of-function properties. In the process of gain-of-function, F142L mutation increases the interactions between the C-domain of CaM and the CaM binding domain of RyR, therefore enhancing RyR inhibition. On the other hand, the loss-of-function effect impairs the ability of the C-domain of CaM to bind free Ca2+, i.e. decreases RyR inhibition. However, at high [Ca2+]i C-domain of CaM saturates allowing the increased RyR inhibitory effect to be the dominant one (Sondergaard et al., 2017). One might assume an overlap between LQTS and CPVT as diminished inhibitory effect on RyR gating is generally associated with CPVT. In mutant guinea pig cells, it was shown that decreased inhibition of RyR gating with impaired CaM effect on the CaM-dependent inactivation of ICa,L (i.e. increased ICa,L) may contribute to APD prolongation and that LQTS associated CaM mutations can lead to electrical alternans, a pathological feature of LQTS (Limpitikul et al., 2014).
Recently a novel mutation, LQTS-17 has been proposed, however, the nomenclature is still indistinct. Some reviews refer to LQTS-17 as a mutation in TRDN-encoded triadin, which has also been linked to CPVT-5 (Landstrom et al., 2017). However, Altmann et al., originally identified the autosomal recessive homozygous or compound heterozygous frameshift loss-of-function mutations in TRDN, proposed the term Triadin Knockout Syndrome (TKOS) or TRDN-mediated autosomal-recessive LQTS, rather than LQTS-17 (Altmann et al., 2015). As in the previous case, here is also the possibility of an overlap with CPVT, as QT prolongation and disease appearance at young age is accompanied by arrhythmias that occur during exercise. The possible cellular mechanism includes reduced negative feedback on ICa,L (i.e. increased ICa,L), increased spontaneous Ca2+ release via RyR, and promotion of SR Ca2+ loading by NCX. It is not clear yet, whether the arrhythmogenic feature is mediated by DAD or EAD, but in a TRDN-null mice model, nifedipine aborted SR Ca2+ overload and spontaneous Ca2+ release (Chopra et al., 2009).
Although most of the LQTSs are inherited in an autosomal dominant form, there is a relatively rare, autosomal recessive inherited form, causing the Jervell and Lange-Nielsen syndrome (KCNQ1 or KCNE1, leading to reduced IKs) (Splawski et al., 1997; Duggal et al., 1998). LQTS-related arrhythmias can be triggered by either slow or fast heart rate or by sinus pauses, therefore the relation between the LQTSs and the sinoatrial node is an interesting topic; for details, see the mini-review from Wilders and Verkerk (2018). For a detailed summary chart about LQTSs with the genetic loci, see a recent review of Landstrom et al. (Landstrom et al., 2017).
Pharmacological management of congenital LQTS starts with the administration of β-blockers, irrespective of the genotype (Moss et al., 2000). In one study, propranolol was shown to be the most effective β-blocker (Na+ channel blockade with limited effects on K+ channels) (Chockalingam et al., 2012). It should be noted that care is required with the use of β-blockers at low heart rate in LQTS-3 since bradycardia-dependent arrhythmias occur more often in these patients (El-Sherif et al., 2017). It was shown in LQTS-2 patients that besides β-blockers, application of mexiletine may also have positive effects (Kim et al., 2010; Ildarova et al., 2012). As an add-on therapy, in the case of LQTS-3 patients mexiletine (Schwartz et al., 1995), lidocaine, tocainide (Rosero et al., 1997), flecainide (Moss et al., 2005), phenytoin (Vukmir and Stein, 1991), or ranolazine (Moss et al., 2008) can be useful (Priori et al., 2013). In LQTS where mutations cause reduction in K+ currents, drugs that enhance K+ currents, nicorandil (Shimizu et al., 1998) or RPR26043 (Kang et al., 2005) were shown to be effective. ICD implantation is recommended for survivors of cardiac arrest or with recurrent syncope while on β-blocker (Priori et al., 2013). Left cardiac sympathetic denervation (LCSD) can also be performed on high-risk patients (arrhythmic events even in the presence of β-blocker/ICD). In addition to drugs or surgical procedures, lifestyle changes, such as avoidance of drugs that lengthen QT interval, identification and correlation of electrolyte abnormalities, avoidance of strenuous exercise (especially swimming in LQTS-1 patients) and abrupt loud noises (LTQS-2) are recommended for patients (Priori et al., 2013).
Brugada Syndrome
Brugada syndrome (BrS) is characterized by ST elevation in V1–V3 ECG leads and is associated with elevated risk of polymorphic VT, VF, and sudden cardiac death (Brugada and Brugada, 1992). Two hypotheses have been proposed to describe the mechanism behind BrS and how ST segment elevation is linked to VT/VF. (Ringer, 1883) In the repolarization hypothesis, the loss of spike-and-dome AP morphology (heterogenous shortening of AP due to predominance of Ito over INa and ICa,L) is suggested in the epicardium of the right ventricular outflow tract, causing an enhanced transmural dispersion of repolarization, i.e. ST elevation (Yan and Antzelevitch, 1999). The arrhythmogenic mechanism is delivered by phase-2 reentry, when the produced extrasystole can occur on the preceding T wave (R-on-T phenomenon), finally initiating VT/VF. (Bers, 2002) The depolarization theory proposes a slowed conduction and delayed activation mechanism in the right ventricular outflow tract as a substrate for reentry (Meregalli et al., 2005).
To date, 23 gene (gain-of-function and also loss-of-function) mutations have been described generating BrS-1–BrS-23 (Gray and Behr, 2016). The most common subtype is BrS-1, mutation affects the SCN5A-encoded α-subunit of the Na+ channel (Nav1.5) and is accountable for about one third of all BrS (Antzelevitch et al., 2005). Genes, governing Ca2+-signaling molecules are also affected in BrS and causing 10–15% of cases (Burashnikov et al., 2010) (Table 1). Loss-of-function mutation of the CACNA1C-encoded α1C-subunit (Cav1.2α1; BrS-3), the CACNB2-encoded β2-subunit (Cavβ2; BrS-4), and the CACNA2D1-encoded α2δ1-subunit (Cavα2δ1; BrS-11) of the L-type Ca2+ channel (governing ICa,L) have been described with a concomitant reduction of ICa,L (Antzelevitch et al., 2007). Patients harboring these Ca2+ related mutations showed BrS like ECG but with shorter than normal QT intervals. Recently, a new Ca2+-related mutation has been linked to BrS, accounting for about 6% of the cases. Mutation of the TRPM4-encoded Ca2+ activated non-selective cation channel transient receptor potential melastatin 4 (TRPM4; BrS-15) can either be gain-of-function or loss-of-function (Liu et al., 2013). TRPM4-mediated current increases APD in atrial muscle and isolated myocytes (Simard et al., 2013), possibly by promoting the plateau (as it is more likely to activate when Ca2+ is elevated). Therefore, TRPM4 mutation may change the AP dome and be arrhythmogenic. TRMP4 may also slow down conduction by altering the availability of Na+ channels (Liu et al., 2013).
There have been pharmacological attempts to manage BrS (isoproterenol, quinidine, procainamide, propafenone, pilsicainide, flecainide), some of them were effective in preventing recurrent episodes of VF or electrical storms, but did not reduce the overall risk of VF (Brugada et al., 2000; Shimizu et al., 2000; Morita et al., 2003; Belhassen et al., 2004; Ohgo et al., 2007). Guidelines are also recommending lifestyle changes (omit drugs that aggravate ST elevation, avoid alcohol and immediate treatment if fevered) and implantation of ICD (Priori et al., 2013).
Short QT Syndrome
Short QT syndrome (SQTS) is a rare inherited syndrome characterized by QT intervals essentially shorter than 360 ms and by an increased incidence of VT/VF mainly in youngsters (Bjerregaard et al., 2010). There are eight different gene mutations, of which three affect ICa,L (Table 1). Loss-of-function mutation of the CACNA1C-encoded α1C-subunit (Cav1.2α1; SQTS-4), the CACNB2-encoded β2-subunit (Cavβ2; SQTS-5), and the CACNA2D1-encoded α2δ1-subunit (Cavα2δ1; SQTS-6) of the L-type Ca2+ channel, similar to the BrS-3, BrS-4, and BrS-11 phenotype. These mutations decrease ICa,L (alter current density and activation/inactivation kinetics), cause heterogenous shortening of APD and QT interval, therefore increases dispersion of repolarization (Antzelevitch et al., 2007). Transmural dispersion of repolarization (shortening effect is more pronounced in the epicardium compared to endocardium and midmyocardium) finally serves as a substrate for reentry. These mutations combined with the mutation of SCN5A-encoded α-subunit of the Na+ channel (Nav1.5) causes an overlapping phenotype of SQTS and BrS.
Early Repolarization Syndrome and Idiopathic Ventricular Fibrillation
Early repolarization syndrome (ERS) is characterized by J-point and ST segment elevation in two or more contiguous leads on ECG (Boineau, 2007). The early repolarization pattern (in the inferior and/or lateral precordial leads) had been considered harmless, but it has recently been associated with idiopathic ventricular fibrillation (IVF) (Rosso et al., 2008). ERS now is diagnosed in IVF survival patients, without other causes of cardiac arrest (channelopathies; structural or non-structural heart diseases, e.g. BrS; metabolic; toxicological; respiratory; and infectious) (Haissaguerre et al., 2008). Seven gene mutations were shown, to date, including loss-of-function mutations of CACNA1C, CACNAB2, and CACNA2D1, as seen in BrS or SQTS (Table 1). L-type channel mutations account for 16% of cases (Burashnikov et al., 2010). CaM-F90L mutation was proposed to be linked to IVF phenotype, where the authors speculated that CaM mutations could be arrhythmogenic by altering Ca2+ binding and/or binding of target proteins, thus generating a rather insensitive CaM and that the gene anomaly is more pronounced in the Purkinje system (Marsman et al., 2014). Recently, a novel single point mutation in RyR2 (RyR2-H29D) has been linked to IVF phenotype (Cheung et al., 2015). RyR2-H29D mutation was shown to be associated with short-coupled premature ventricular contractions, initiating polymorphic VT. This mutation caused diastolic Ca2+ leak at rest by higher open probability and higher frequency of opening of RyR at low diastolic Ca2+ levels in a non-PKA phosphorylated state, unlike the typical CPVT-related RyR mutations. Therefore, RyR dysfunction caused by RyR2-H29D mutation may play a role in short-coupled polymorphic VT.
J-point elevation associated malignant arrhythmias have recently been proposed with a new classification, as J-wave syndrome (Antzelevitch and Yan, 2010).
Acquired Syndromes
Acquired Long QT Syndrome
In addition to the congenital form, LQTS can also be acquired. The prevalence of acquired LQTS is greater than that of congenital forms (El-Sherif et al., 2019). It is generally caused by adverse, unwanted drug effects and/or electrolyte abnormalities and may predispose to the prolongation of the APD/QT interval, increase in dispersion of refractoriness and to a higher risk for generating EADs, being the substrates for VTs, especially for torsade de pointes VT (El-Sherif and Turitto, 1999).
The above mentioned effects are often seen for the hERG-encoded (human ether-à-go-go-related gene or KCNH2) Kv11.1 channel, responsible for IKr while effects on enhanced INa,L has also been reported (Yang et al., 2014). The role of dispersion of repolarization in generating tachyarrhythmias (and the role as a preclinical proarrhythmia marker) is further supported by a series of experiments, where DL-sotalol and amiodarone were compared (Milberg et al., 2004). It was shown, that both hERG-blockers increased QT interval, however only DL-sotalol increased transmural dispersion of refractoriness, EADs and torsade de pointes (and caused triangulation of the AP), while amiodarone caused phase-2 prolongation of the AP without triangulation, which is otherwise considered proarrhythmic.
Several other causes of acquired LQTS have been described, including electrolyte disorders (El-Sherif and Turitto, 2011), such as hypokalemia, hypomagnesemia or hypocalcemia, hypothyroidism, hypothermia, but also antidepressant and antipsychotic treatments (Sicouri and Antzelevitch, 2018), female gender, and autoimmune and inflammatory diseases (Lazzerini et al., 2015; Boutjdir et al., 2016). Hypocalcemia causes QT prolongation via phase-2 prolongation of AP (Eryol et al., 2003), also longer and late Ca2+ influx (due to reduced Ca2+-dependent inactivation of ICa,L) can favor the formation of EADs.
Atrial Fibrillation
The most prevalent cardiac arrhythmia is atrial fibrillation (AF) and this can be classified as paroxysmal (spontaneously self-terminates into sinus rhythm in less than 7 days), persistent (lasts for more than 7 days), long-lasting persistent (AF lasts for more than a year) or permanent AF (without active rhythm control) (Kirchhof et al., 2016). AF is multifactorial. Basic arrhythmogenic mechanisms include Ca2+ handling defects such as triggered activity (DAD, late-phase 3 EAD), conduction block (reentry), and Ca2+-driven cardiac alternans and altered Ca2+ buffering (Nattel and Dobrev, 2016). DAD-mediated triggered arrhythmias are underlined by Ca2+ handling instability in AF, namely RyR dysfunction (increased phosphorylation and open probability), increased SERCA function, increased diastolic SR Ca2+ leak and spontaneous SR Ca2+ release, increase in Ca2+ sparks and waves, enhanced CaMKII function (with subsequent RyR hyperphosphorylation), or reduced ICa,L (Sood et al., 2008; Neef et al., 2010; Shan et al., 2012; Voigt et al., 2012). Involvement of late-phase 3 EAD has also been shown (Burashnikov and Antzelevitch, 2006). As in most of the AF models APD is abbreviated, this observation can be somewhat surprising, since EADs generally occur at a prolonged APD. However, as we previously described, late-phase 3 EADs occur at shorter APD and at elevated Ca2+ loading conditions (such as rapid atrial pacing). These ectopic activities can serve as a trigger for reentry which is considered to be the main arrhythmogenic mechanism in AF. Also, ICa,L reduction in AF causes APD shortening and promotes reentrant activity (Heijman et al., 2014). Reduction of ICa,L might be governed by reduction of protein and mRNA levels of the channel (alpha subunit) after rapid pacing. This transcriptional downregulation of Ca2+ channel has been proposed to be mediated by activation of calcineurin by Ca2+/CaM, which in turn, regulates nuclear translocation of NFAT (Qi et al., 2008).
A novel, interesting theory has been proposed, namely, Ca2+ signaling silencing, as an antiarrhythmic adaptive mechanism in AF (Greiser et al., 2014). The key observation was, that sustained high atrial pacing may not lead to Ca2+ instability, suggesting a role of accompanying cardiovascular diseases (e.g. HF) rather than “lone AF” itself in those cases when unstable Ca2+ signaling occurs in AF. Ca2+ signaling silencing process includes the failure of centripetal intracellular Ca2+ signal propagation (also unchanged level of Ca2+ sparks and decreased amplitude of the systolic Ca2+ transient), remodeling of the RyR complex (reduced protein expression and CaMKII-mediated phosphorylation), and lower Na+ concentration (consequential reduction in Ca2+ load) (Greiser, 2017). The decreased propagation was associated with an increase of cytoplasmic buffer power possibly due to increased Ca2+ sensitivity of myofilaments resulting from decreased phosphorylation of troponin I (Greiser et al., 2014). The authors concluded that the Ca2+ signaling phenotype in AF patients is a net result of factors that stabilize (i.e. Ca2+ signaling silencing) or destabilize it (arrhythmogenic Ca2+ instability). Therefore, future therapeutic approaches should identify the substrate (arrhythmia enhancing abnormalities or arrhythmia suppressing Ca2+ signaling silencing) and tailor therapies for individual AF patients (Kirchhof et al., 2016; Schotten et al., 2016; Greiser, 2017).
For an excess review about the role of Ca2+ in the pathophsiology of AF see the review of Denham et al. (2018).
Conclusions
In summary, we have reviewed the roles of Ca2+ in cardiac E-C-coupling focusing on those defects which lead to cardiac arrhythmias in inherited and acquired syndromes. In the last few decades there have been great advances in the understanding of these arrhythmias, however, there is still a need for more work investigating the physiology and pathophysiology of Ca2+ related events. Designing drugs to treat a specific disease type has never been simple; it is enough to think of the early disappointing attempts to block the Na+ or K+ channels (CAST and SWORD trials, respectively). Multiple characteristics of novel therapeutic approaches have to be determined and to be considered as a complex, systems problem.
Along with the generally used β-blockers, newly developed selective drugs without proarrhythmic side effects are necessary. While implantable cardiac defibrillators provide longer life expectancy, they cannot prevent the onset of cardiac events. An additional helpful tool would be reliable and effective risk stratification and clinical guidance for all of the syndromes discussed. It should not be overlooked that in the future other genetic mutations may be discovered requiring novel biological therapies. Because of the diversity of inherited and acquired mutations individually tailored therapeutic approaches (gene-specific or mutation-specific pharmacological and/or gene therapy) will be required.
To gain a better understanding of the role of Ca2+ in the cardiac arrhythmias data from basic science should meet the clinical practice; translational aspects must be key in all fields of science.
Author Contributions
KK conceived the review and drafted the manuscript. KK, RV, BH, TB, PN, and DE revised the manuscript critically for important intellectual content. DE contributed to the critical review of the literature, editing of the manuscript text and review of the figures. All authors approved the final version of the manuscript submitted.
Funding
This work was funded by the National Research Development and Innovation Office (NKFIH-K115397). Further support was obtained from GINOP-2.3.2.-15-2016-00040 and EFOP-3.6.2-16-2017-00006 projects, which are co-financed by the European Union and the European Regional Development Fund. The research was financed by the Thematic Excellence Programme of the Ministry for Innovation and Technology in Hungary (ED_18-1-2019-0028), within the framework of the Space Sciences thematic program of the University of Debrecen. This work was supported by the British Heart Foundation Chair Award (grant number: CH/200004/12801).
Conflict of Interest
The authors declare that the research was conducted in the absence of any commercial or financial relationships that could be construed as a potential conflict of interest.
Acknowledgments
KK is grateful to his wife Viktoria Csato, PhD who is expecting their twin daughters Zoe Amira and Liza Jazmin, without whom this review would have been completed much earlier. The authors wish to thank Jessica L. Caldwell for the design of Figure 1.
Abbreviations
AF, atrial fibrillation; AP, action potential; APD, action potential duration; AV, atrioventricular; BrS, Brugada syndrome; CaM, calmodulin; CaMKII, Ca2+/calmodulin-dependent protein kinase II; CICR, Ca2+-induced Ca2+ release; CPVT, catecholaminergic polymorphic ventricular tachycardia; CSQ2, calsequestrin 2; DAD, delayed afterdepolarization; EAD, early afterdepolarization; EC, excitation-contraction coupling; ERS, early repolarization syndrome; HF, heart failure; ICD, implantable cardiac defibrillator; IVF, idiopathic ventricular fibrillation; LQTS, long QT syndrome; NCX, sodium-calcium exchange; NFAT, nuclear factor of activated T-cells; PKA, protein kinase A; PLN, phospholamban; PMCA, plasma membrane Ca2+-ATPase; PVC, premature ventricular contraction; RSV, relative short term beat-to-beat variability of action potential duration; RyR, ryanodine receptor; SA, sinoatrial; SERCA, sarco/endoplasmic reticulum Ca2+-ATPase; SOCE, store overload-induced Ca2+ entry; SOICR, store overload-induced Ca2+ release; SQTS, short QT syndrome; SR, sarcoplasmic reticulum; SV, short term beat-to-beat variability of action potential duration; VF, ventricular fibrillation; VT, ventricular tachycardia.
References
Abi-Gerges, N., Valentin, J. P., Pollard, C. E. (2010). Dog left ventricular midmyocardial myocytes for assessment of drug-induced delayed repolarization: short-term variability and proarrhythmic potential. Br. J. Pharmacol. 159 (1), 77–92. doi: 10.1111/j.1476-5381.2009.00338.x
Allessie, M. A., Bonke, F. I., Schopman, F. J. (1977). Circus movement in rabbit atrial muscle as a mechanism of tachycardia. III. The “leading circle” concept: a new model of circus movement in cardiac tissue without the involvement of an anatomical obstacle. Circ. Res. 41 (1), 9–18. doi: 10.1161/01.res.41.1.9
Altmann, H. M., Tester, D. J., Will, M. L., Middha, S., Evans, J. M., Eckloff, B. W., et al. (2015). Homozygous/compound heterozygous triadin mutations associated with autosomal-recessive long-QT syndrome and pediatric sudden cardiac arrest: elucidation of the triadin knockout syndrome. Circulation 131 (23), 2051–2060. doi: 10.1161/CIRCULATIONAHA.115.015397
Antzelevitch, C., Burashnikov, A. (2011). Overview of basic mechanisms of cardiac arrhythmia. Cardiac Electrophysiol. Clinics 3 (1), 23–45. doi: 10.1016/j.ccep.2010.10.012
Antzelevitch, C., Yan, G. X. (2010). J wave syndromes. Heart Rhythm 7 (4), 549–558. doi: 10.1016/j.hrthm.2009.12.006
Antzelevitch, C., Jalife, J., Moe, G. K. (1980). Characteristics of reflection as a mechanism of reentrant arrhythmias and its relationship to parasystole. Circulation 61 (1), 182–191. doi: 10.1161/01.cir.61.1.182
Antzelevitch, C., Brugada, P., Borggrefe, M., Brugada, J., Brugada, R., Corrado, D., et al. (2005). Brugada syndrome: report of the second consensus conference: endorsed by the heart rhythm society and the european heart rhythm association. Circulation 111 (5), 659–670. doi: 10.1161/01.CIR.0000152479.54298.51
Antzelevitch, C., Pollevick, G. D., Cordeiro, J. M., Casis, O., Sanguinetti, M. C., Aizawa, Y., et al. (2007). Loss-of-function mutations in the cardiac calcium channel underlie a new clinical entity characterized by ST-segment elevation, short QT intervals, and sudden cardiac death. Circulation 115 (4), 442–449. doi: 10.1161/CIRCULATIONAHA.106.668392
Antzelevitch, C. (2007). Heterogeneity and cardiac arrhythmias: an overview. Heart Rhythm 4 (7), 964–972. doi: 10.1016/j.hrthm.2007.03.036
Aronson, R. S. (1981). Afterpotentials and triggered activity in hypertrophied myocardium from rats with renal hypertension. Circ. Res. 48 (5), 720–727. doi: 10.1161/01.res.48.5.720
Arvanitis, D. A., Vafiadaki, E., Sanoudou, D., Kranias, E. G. (2011). Histidine-rich calcium binding protein: the new regulator of sarcoplasmic reticulum calcium cycling. J. Mol. Cell. Cardiol. 50 (1), 43–49. doi: 10.1016/j.yjmcc.2010.08.021
Asakura, K., Cha, C. Y., Yamaoka, H., Horikawa, Y., Memida, H., Powell, T., et al. (2014). EAD and DAD mechanisms analyzed by developing a new human ventricular cell model. Prog. Biophys. Mol. Biol. 116 (1), 11–24. doi: 10.1016/j.pbiomolbio.2014.08.008
Bal, N. C., Sharon, A., Gupta, S. C., Jena, N., Shaikh, S., Gyorke, S., et al. (2010). The catecholaminergic polymorphic ventricular tachycardia mutation R33Q disrupts the N-terminal structural motif that regulates reversible calsequestrin polymerization. J. Biol. Chem. 285 (22), 17188–17196. doi: 10.1074/jbc.M109.096354
Bannister, M. L., Thomas, N. L., Sikkel, M. B., Mukherjee, S., Maxwell, C., MacLeod, K. T., et al. (2015). The mechanism of flecainide action in CPVT does not involve a direct effect on RyR2. Circ. Res. 116 (8), 1324–1335. doi: 10.1161/CIRCRESAHA.116.305347
Barhanin, J., Lesage, F., Guillemare, E., Fink, M., Lazdunski, M., Romey, G. (1996). K(V)LQT1 and lsK (minK) proteins associate to form the I(Ks) cardiac potassium current. Nature 384 (6604), 78–80. doi: 10.1038/384078a0
Bauce, B., Rampazzo, A., Basso, C., Bagattin, A., Daliento, L., Tiso, N., et al. (2002). Screening for ryanodine receptor type 2 mutations in families with effort-induced polymorphic ventricular arrhythmias and sudden death: early diagnosis of asymptomatic carriers. J. Am. Coll. Cardiol. 40 (2), 341–349. doi: 10.1016/s0735-1097(02)01946-0
Belhassen, B., Glick, A., Viskin, S. (2004). Efficacy of quinidine in high-risk patients with Brugada syndrome. Circulation 110 (13), 1731–1737. doi: 10.1161/01.CIR.0000143159.30585.90
Benitah, J. P., Alvarez, J. L., Gomez, A. M. (2010). L-type Ca(2+) current in ventricular cardiomyocytes. J. Mol. Cell. Cardiol. 48 (1), 26–36. doi: 10.1016/j.yjmcc.2009.07.026
Bers, D. M. (2000). Calcium fluxes involved in control of cardiac myocyte contraction. Circ. Res. 87 (4), 275–281. doi: 10.1161/01.res.87.4.275
Bers, D. M. (2002). Cardiac excitation-contraction coupling. Nature 415 (6868), 198–205. doi: 10.1038/415198a
Bhuiyan, Z. A., Hamdan, M. A., Shamsi, E. T., Postma, A. V., Mannens, M. M., Wilde, A. A., et al. (2007). A novel early onset lethal form of catecholaminergic polymorphic ventricular tachycardia maps to chromosome 7p14-p22. J. Cardiovasc. Electrophysiol. 18 (10), 1060–1066. doi: 10.1111/j.1540-8167.2007.00913.x
Bhuiyan, Z. A., Al-Shahrani, S., Al-Aama, J., Wilde, A. A., Momenah, T. S. (2013). Congenital long QT syndrome: an update and present perspective in Saudi Arabia. Front. Pediatr. 1, 39. doi: 10.3389/fped.2013.00039
Biernacka, E. K., Hoffman, P. (2011). Efficacy of flecainide in a patient with catecholaminergic polymorphic ventricular tachycardia. Europace 13 (1), 129–130. doi: 10.1093/europace/euq279
Bjerregaard, P., Nallapaneni, H., Gussak, I. (2010). Short QT interval in clinical practice. J. Electrocardiol. 43 (5), 390–395. doi: 10.1016/j.jelectrocard.2010.06.004
Boczek, N. J., Miller, E. M., Ye, D., Nesterenko, V. V., Tester, D. J., Antzelevitch, C., et al. (2015). Novel Timothy syndrome mutation leading to increase in CACNA1C window current. Heart Rhythm. 12 (1), 211–219. doi: 10.1016/j.hrthm.2014.09.051
Boineau, J. P. (2007). The early repolarization variant–normal or a marker of heart disease in certain subjects. J. Electrocardiol. 40 (1), 3.e11–3.e16. doi: 10.1016/j.jelectrocard.2006.04.002
Boutjdir, M., Lazzerini, P. E., Capecchi, P. L., Laghi-Pasini, F., El-Sherif, N. (2016). Potassium channel block and novel autoimmune-associated long QT syndrome. Cardiac Electrophysiol. Clinics 8 (2), 373–384. doi: 10.1016/j.ccep.2016.02.002
Brugada, P., Brugada, J. (1992). Right bundle branch block, persistent ST segment elevation and sudden cardiac death: a distinct clinical and electrocardiographic syndrome. a multicenter report. J. Am. Coll. Cardiol. 20 (6), 1391–1396. doi: 10.1016/0735-1097(92)90253-j
Brugada, R., Brugada, J., Antzelevitch, C., Kirsch, G. E., Potenza, D., Towbin, J. A., et al. (2000). Sodium channel blockers identify risk for sudden death in patients with ST-segment elevation and right bundle branch block but structurally normal hearts. Circulation 101 (5), 510–515. doi: 10.1161/01.cir.101.5.510
Burashnikov, A., Antzelevitch, C. (2003). Reinduction of atrial fibrillation immediately after termination of the arrhythmia is mediated by late phase 3 early afterdepolarization-induced triggered activity. Circulation 107 (18), 2355–2360. doi: 10.1161/01.CIR.0000065578.00869.7C
Burashnikov, A., Antzelevitch, C. (2006). Late-phase 3 EAD. a unique mechanism contributing to initiation of atrial fibrillation. Pacing Clin. Electrophysiol. 29 (3), 290–295. doi: 10.1111/j.1540-8159.2006.00336.x
Burashnikov, E., Pfeiffer, R., Barajas-Martinez, H., Delpon, E., Hu, D., Desai, M., et al. (2010). Mutations in the cardiac L-type calcium channel associated with inherited J-wave syndromes and sudden cardiac death. Heart Rhythm 7 (12), 1872–1882. doi: 10.1016/j.hrthm.2010.08.026
Cabo, C., Barr, R. C. (1992). Propagation model using the DiFrancesco-Noble equations. Comparison to reported experimental results. Med. Biol. Eng. Comput. 30 (3), 292–302. doi: 10.1007/bf02446967
Cantalapiedra, I. R., Penaranda, A., Mont, L., Brugada, J., Echebarria, B. (2009). Reexcitation mechanisms in epicardial tissue: role of I(to) density heterogeneities and I(Na) inactivation kinetics. J. Theor. Biol. 259 (4), 850–859. doi: 10.1016/j.jtbi.2009.04.021
Cantalapiedra, I. R., Penaranda, A., Echebarria, B., Bragard, J. (2010). Phase-2 reentry in cardiac tissue: role of the slow calcium pulse. Phys. Rev. E Stat. Nonlinear Soft Matter Phys. 82 (1 Pt 1), 011907. doi: 10.1103/PhysRevE.82.011907
Chang, M. G., Sato, D., de Lange, E., Lee, J. H., Karagueuzian, H. S., Garfinkel, A., et al. (2012). Bi-stable wave propagation and early afterdepolarization-mediated cardiac arrhythmias. Heart Rhythm 9 (1), 115–122. doi: 10.1016/j.hrthm.2011.08.014
Chang, M. G., de Lange, E., Calmettes, G., Garfinkel, A., Qu, Z., Weiss, J. N. (2013). Pro- and antiarrhythmic effects of ATP-sensitive potassium current activation on reentry during early afterdepolarization-mediated arrhythmias. Heart Rhythm 10 (4), 575–582. doi: 10.1016/j.hrthm.2012.12.017
Chanson, M., Mollard, P., Meda, P., Suter, S., Jongsma, H. J. (1999). Modulation of pancreatic acinar cell to cell coupling during ACh-evoked changes in cytosolic Ca2+. J. Biol. Chem. 274 (1), 282–287. doi: 10.1074/jbc.274.1.282
Chen, L., Marquardt, M. L., Tester, D. J., Sampson, K. J., Ackerman, M. J., Kass, R. S. (2007). Mutation of an a-kinase-anchoring protein causes long-QT syndrome. Proc. Natl. Acad. Sci. U. S. A. 104 (52), 20990–20995. doi: 10.1073/pnas.0710527105
Cheng, H., Lederer, W. J., Cannell, M. B. (1993). Calcium sparks: elementary events underlying excitation-contraction coupling in heart muscle. Science 262 (5134), 740–744. doi: 10.1126/science.8235594
Cheung, J. W., Meli, A. C., Xie, W., Mittal, S., Reiken, S., Wronska, A., et al. (2015). Short-coupled polymorphic ventricular tachycardia at rest linked to a novel ryanodine receptor (RyR2) mutation: leaky RyR2 channels under non-stress conditions. Int. J. Cardiol. 180, 228–236. doi: 10.1016/j.ijcard.2014.11.119
Chiamvimonvat, N., Chen-Izu, Y., Clancy, C. E., Deschenes, I., Dobrev, D., Heijman, J., et al. (2017). Potassium currents in the heart: functional roles in repolarization, arrhythmia and therapeutics. J. Physiol. 595 (7), 2229–2252. doi: 10.1113/JP272883
Chockalingam, P., Crotti, L., Girardengo, G., Johnson, J. N., Harris, K. M., van der Heijden, J. F., et al. (2012). Not all beta-blockers are equal in the management of long QT syndrome types 1 and 2: higher recurrence of events under metoprolol. J. Am. Coll. Cardiol. 60 (20), 2092–2099. doi: 10.1016/j.jacc.2012.07.046
Chopra, N., Yang, T., Asghari, P., Moore, E. D., Huke, S., Akin, B., et al. (2009). Ablation of triadin causes loss of cardiac Ca2+ release units, impaired excitation-contraction coupling, and cardiac arrhythmias. Proc. Natl. Acad. Sci. U. S. A. 106 (18), 7636–7641. doi: 10.1073/pnas.0902919106
Coppini, R., Ferrantini, C., Mugelli, A., Poggesi, C., Cerbai, E. (2018). Altered Ca(2+) and Na(+) homeostasis in human hypertrophic cardiomyopathy: implications for arrhythmogenesis. Front. Physiol. 9, 1391. doi: 10.3389/fphys.2018.01391
Coumel, P. (1978). Catecholaminergic-induced severe ventricular arrhythmias with adams-Stokes syndrome in children: report of four cases. Br. Heart J. 40, 28–37.
Crotti, L., Johnson, C. N., Graf, E., De Ferrari, G. M., Cuneo, B. F., Ovadia, M., et al. (2013). Calmodulin mutations associated with recurrent cardiac arrest in infants. Circulation 127 (9), 1009–1017. doi: 10.1161/CIRCULATIONAHA.112.001216
Damiano, B. P., Rosen, M. R. (1984). Effects of pacing on triggered activity induced by early afterdepolarizations. Circulation 69 (5), 1013–1025. doi: 10.1161/01.cir.69.5.1013
Davidenko, J. M., Pertsov, A. V., Salomonsz, R., Baxter, W., Jalife, J. (1992). Stationary and drifting spiral waves of excitation in isolated cardiac muscle. Nature 355 (6358), 349–351. doi: 10.1038/355349a0
De Ferrari, G. M., Viola, M. C., D'Amato, E., Antolini, R., Forti, S. (1995). Distinct patterns of calcium transients during early and delayed afterdepolarizations induced by isoproterenol in ventricular myocytes. Circulation 91 (10), 2510–2515. doi: 10.1161/01.cir.91.10.2510
De Maziere, A. M., Scheuermann, D. W. (1990). Structural changes in cardiac gap junctions after hypoxia and reoxygenation: a quantitative freeze-fracture analysis. Cell Tissue Res. 261 (1), 183–194. doi: 10.1007/bf00329451
Denegri, M., Avelino-Cruz, J. E., Boncompagni, S., De Simone, S. A., Auricchio, A., Villani, L., et al. (2012). Viral gene transfer rescues arrhythmogenic phenotype and ultrastructural abnormalities in adult calsequestrin-null mice with inherited arrhythmias. Circ. Res. 110 (5), 663–668. doi: 10.1161/CIRCRESAHA.111.263939
Denegri, M., Bongianino, R., Lodola, F., Boncompagni, S., De Giusti, V. C., Avelino-Cruz, J. E., et al. (2014). Single delivery of an adeno-associated viral construct to transfer the CASQ2 gene to knock-in mice affected by catecholaminergic polymorphic ventricular tachycardia is able to cure the disease from birth to advanced age. Circulation 129 (25), 2673–2681. doi: 10.1161/CIRCULATIONAHA.113.006901
Denham, N. C., Pearman, C. M., Caldwell, J. L., Madders, G. W. P., Eisner, D. A., Trafford, A. W., et al. (2018). Calcium in the pathophysiology of atrial fibrillation and heart failure. Front. Physiol. 9, 1380. doi: 10.3389/fphys.2018.01380
Devalla, H. D., Gelinas, R., Aburawi, E. H., Beqqali, A., Goyette, P., Freund, C., et al. (2016). TECRL, a new life-threatening inherited arrhythmia gene associated with overlapping clinical features of both LQTS and CPVT. EMBO Mol. Med. 8 (12), 1390–1408. doi: 10.15252/emmm.201505719
Dhein, S. (1998). Gap junction channels in the cardiovascular system: pharmacological and physiological modulation. Trends Pharmacol. Sci. 19 (6), 229–241. doi: 10.1016/s0165-6147(98)01192-4
di Bernardo, D., Murray, A. (2002). Origin on the electrocardiogram of U-waves and abnormal U-wave inversion. Cardiovasc. Res. 53 (1), 202–208. doi: 10.1016/s0008-6363(01)00439-4
Di Diego, J. M., Antzelevitch, C. (1994). High [Ca2+]o-induced electrical heterogeneity and extrasystolic activity in isolated canine ventricular epicardium. Phase 2 reentry. Circulation 89 (4), 1839–1850. doi: 10.1161/01.cir.89.4.1839
Dong, M., Yan, S., Chen, Y., Niklewski, P. J., Sun, X., Chenault, K., et al. (2010). Role of the transient outward current in regulating mechanical properties of canine ventricular myocytes. J. Cardiovasc. Electrophysiol. 21 (6), 697–703. doi: 10.1111/j.1540-8167.2009.01708.x
Duggal, P., Vesely, M. R., Wattanasirichaigoon, D., Villafane, J., Kaushik, V., Beggs, A. H. (1998). Mutation of the gene for IsK associated with both jervell and lange-nielsen and romano-ward forms of long-QT syndrome. Circulation 97 (2), 142–146. doi: 10.1161/01.cir.97.2.142
Eisner, D. A., Choi, H. S., Diaz, M. E., O'Neill, S. C., Trafford, A. W. (2000). Integrative analysis of calcium cycling in cardiac muscle. Circ. Res. 87 (12), 1087–1094. doi: 10.1161/01.res.87.12.1087
Eisner, D. A., Li, Y., O'Neill, S. C. (2006). Alternans of intracellular calcium: mechanism and significance. Heart Rhythm 3 (6), 743–745. doi: 10.1016/j.hrthm.2005.12.020
Eisner, D. A., Caldwell, J. L., Kistamas, K., Trafford, A. W. (2017). Calcium and excitation-contraction coupling in the heart. Circ. Res. 121 (2), 181–195. doi: 10.1161/circresaha.117.310230
Eisner, D. A. (2018). Ups and downs of calcium in the heart. J. Physiol. 596 (1), 19–30. doi: 10.1113/jp275130
El-Sherif, N., Turitto, G. (1999). The long QT syndrome and torsade de pointes. Pacing Clin. Electrophysiol. 22 (1 Pt 1), 91–110. doi: 10.1111/j.1540-8159.1999.tb00305.x
El-Sherif, N., Turitto, G. (2011). Electrolyte disorders and arrhythmogenesis. Cardiol. J. 18 (3), 233–245.
el-Sherif, N., WB, G., RH, Z., Hariman, R. (1985). Reentrant ventricular arrhythmias in the late myocardial infarction period. 12. spontaneous versus induced reentry and intramural versus epicardial circuits. J. Am. Coll. Cardiol. 6 (1), 124–132. doi: 10.1016/s0735-1097(85)80263-1
El-Sherif, N., Turitto, G., Boutjdir, M. (2017). Congenital Long QT syndrome and torsade de pointes. Ann. Noninvasive Electrocardiol.: Off. J. Int. Soc. Holter Noninvasive Electrocardiol. Inc. 22 (6), e12481. doi: 10.1111/anec.12481
El-Sherif, N., Turitto, G., Boutjdir, M. (2019). Acquired long QT syndrome and electrophysiology of torsade de pointes. Arrhythmia Electrophysiol. Rev. 8 (2), 122–130. doi: 10.15420/aer.2019.8.3
Eryol, N. K., Colak, R., Ozdogru, I., Tanriverdi, F., Unal, S., Topsakal, R., et al. (2003). Effects of calcium treatment on QT interval and QT dispersion in hypocalcemia. Am. J. Cardiol. 91 (6), 750–752. doi: 10.1016/s0002-9149(02)03423-9
Faggioni, M., Kryshtal, D. O., Knollmann, B. C. (2012). Calsequestrin mutations and catecholaminergic polymorphic ventricular tachycardia. Pediatr. Cardiol. 33 (6), 959–967. doi: 10.1007/s00246-012-0256-1
Ferrier, G. R., Saunders, J. H., Mendez, C. (1973). A cellular mechanism for the generation of ventricular arrhythmias by acetylstrophanthidin. Circ. Res. 32 (5), 600–609. doi: 10.1161/01.res.32.5.600
Fink, M., Noble, D. (2010). Pharmacodynamic effects in the cardiovascular system: the modeller's view. Basic Clin. Pharmacol. Toxicol. 106 (3), 243–249. doi: 10.1111/j.1742-7843.2009.00534.x
Firek, L., Weingart, R. (1995). Modification of gap junction conductance by divalent cations and protons in neonatal rat heart cells. J. Mol. Cell. Cardiol. 27 (8), 1633–1643. doi: 10.1016/s0022-2828(95)90623-1
Gadsby, D. C., Cranefield, P. F. (1977). Two levels of resting potential in cardiac Purkinje fibers. J. Gen. Physiol. 70 (6), 725–746. doi: 10.1085/jgp.70.6.725
Garfinkel, A., Kim, Y. H., Voroshilovsky, O., Qu, Z., Kil, J. R., Lee, M. H., et al. (2000). Preventing ventricular fibrillation by flattening cardiac restitution. Proc. Natl. Acad. Sci. U. S. A. 97 (11), 6061–6066. doi: 10.1073/pnas.090492697
Garfinkel, A. (2007). Eight (or more) kinds of alternans. J. Electrocardiol. 40 (6 Suppl), S70–S74. doi: 10.1016/j.jelectrocard.2007.06.011
Garrey, W. E. (1914). The nature of fibrillary contraction of the heart.—its relation to tissue mass and form. Am. J. Physiology-Legacy Content 33 (3), 397–414. doi: 10.1152/ajplegacy.1914.33.3.397
George, C. H., Jundi, H., Thomas, N. L., Fry, D. L., Lai, F. A. (2007). Ryanodine receptors and ventricular arrhythmias: emerging trends in mutations, mechanisms and therapies. J. Mol. Cell. Cardiol. 42 (1), 34–50. doi: 10.1016/j.yjmcc.2006.08.115
Goldhaber, J. I. (1996). Free radicals enhance Na+/Ca2+ exchange in ventricular myocytes. Am. J. Physiol. 271 (3 Pt 2), H823–H833. doi: 10.1152/ajpheart.1996.271.3.H823
Gray, B., Behr, E. R. (2016). New insights into the genetic basis of inherited arrhythmia syndromes. Circ. Cardiovasc. Genet. 9 (6), 569–577. doi: 10.1161/CIRCGENETICS.116.001571
Gray, R. A., Jalife, J., Panfilov, A. V., Baxter, W. T., Cabo, C., Davidenko, J. M., et al. (1995). Mechanisms of cardiac fibrillation. Science 270 (5239), 1222–1223; author reply 4–5. doi: 10.1126/science.270.5239.1222
Greenstein, J. L., Wu, R., Po, S., Tomaselli, G. F., Winslow, R. L. (2000). Role of the calcium-independent transient outward current I(to1) in shaping action potential morphology and duration. Circ. Res. 87 (11), 1026–1033. doi: 10.1161/01.res.87.11.1026
Greiser, M., Kerfant, B. G., Williams, G. S., Voigt, N., Harks, E., Dibb, K. M., et al. (2014). Tachycardia-induced silencing of subcellular Ca2+ signaling in atrial myocytes. J. Clin. Invest. 124 (11), 4759–4772. doi: 10.1172/JCI70102
Greiser, M. (2017). Calcium signalling silencing in atrial fibrillation. J. Physiol. 595 (12), 4009–4017. doi: 10.1113/JP273045
Gussak, I., Antzelevitch, C., Hammill, S. C., Shen, W. K., Bjerregaard, P. (2003). Cardiac Repolarization - Bridging Basic and Clinical Science. Contemporary Cardiology. (Totowa, New Jersey: Humana Press).
Haissaguerre, M., Derval, N., Sacher, F., Jesel, L., Deisenhofer, I., de Roy, L., et al. (2008). Sudden cardiac arrest associated with early repolarization. New Engl. J. Med. 358 (19), 2016–2023. doi: 10.1056/NEJMoa071968
Hayashi, M., Denjoy, I., Extramiana, F., Maltret, A., Buisson, N. R., Lupoglazoff, J. M., et al. (2009). Incidence and risk factors of arrhythmic events in catecholaminergic polymorphic ventricular tachycardia. Circulation 119 (18), 2426–2434. doi: 10.1161/CIRCULATIONAHA.108.829267
Hegyi, B., Bers, D. M., Bossuyt, J. (2019). CaMKII signaling in heart diseases: Emerging role in diabetic cardiomyopathy. J. Mol. Cell. Cardiol. 127, 246–259. doi: 10.1016/j.yjmcc.2019.01.001
Heijman, J., Voigt, N., Nattel, S., Dobrev, D. (2014). Cellular and molecular electrophysiology of atrial fibrillation initiation, maintenance, and progression. Circ. Res. 114 (9), 1483–1499. doi: 10.1161/CIRCRESAHA.114.302226
Hilliard, F. A., Steele, D. S., Laver, D., Yang, Z., Le Marchand, S. J., Chopra, N., et al. (2010). Flecainide inhibits arrhythmogenic Ca2+ waves by open state block of ryanodine receptor Ca2+ release channels and reduction of Ca2+ spark mass. J. Mol. Cell. Cardiol. 48 (2), 293–301. doi: 10.1016/j.yjmcc.2009.10.005
Hoffman, B. F., Rosen, M. R. (1981). Cellular mechanisms for cardiac arrhythmias. Circ. Res. 49 (1), 1–15. doi: 10.1161/01.res.49.1.1
Hopenfeld, B. (2006). Mechanism for action potential alternans: the interplay between L-type calcium current and transient outward current. Heart Rhythm 3 (3), 345–352. doi: 10.1016/j.hrthm.2005.11.016
Horvath, B., Banyasz, T., Jian, Z., Hegyi, B., Kistamas, K., Nanasi, P. P., et al. (2013). Dynamics of the late Na(+) current during cardiac action potential and its contribution to afterdepolarizations. J. Mol. Cell. Cardiol. 64, 59–68. doi: 10.1016/j.yjmcc.2013.08.010
Horvath, B., Hegyi, B., Kistamas, K., Vaczi, K., Banyasz, T., Magyar, J., et al. (2015). Cytosolic calcium changes affect the incidence of early afterdepolarizations in canine ventricular myocytes. Can. J. Physiol. Pharmacol. 93 (7), 527–534. doi: 10.1139/cjpp-2014-0511
Houle, T. D., Ram, M. L., Cala, S. E. (2004). Calsequestrin mutant D307H exhibits depressed binding to its protein targets and a depressed response to calcium. Cardiovasc. Res. 64 (2), 227–233. doi: 10.1016/j.cardiores.2004.09.009
Hwang, H. S., Hasdemir, C., Laver, D., Mehra, D., Turhan, K., Faggioni, M., et al. (2011). Inhibition of cardiac Ca2+ release channels (RyR2) determines efficacy of class I antiarrhythmic drugs in catecholaminergic polymorphic ventricular tachycardia. Circ. Arrhythm. Electrophysiol. 4 (2), 128–135. doi: 10.1161/CIRCEP.110.959916
Hwang, H. S., Baldo, M. P., Rodriguez, J. P., Faggioni, M., Knollmann, B. C. (2019). Efficacy of flecainide in catecholaminergic polymorphic ventricular tachycardia is mutation-independent but reduced by calcium overload. Front. Physiol. 10, 992. doi: 10.3389/fphys.2019.00992
Ikeda, T., Uchida, T., Hough, D., Lee, J. J., Fishbein, M. C., Mandel, W. J., et al. (1996). Mechanism of spontaneous termination of functional reentry in isolated canine right atrium. Evidence for the presence of an excitable but nonexcited core. Circulation 94 (8), 1962–1973. doi: 10.1161/01.cir.94.8.1962
Ildarova, R., Shkolnikova, M. A., Kharlap, M., Bereznitskaya, V., Kalinin, L. (2012). Sodium-channel blockers might contribute to the prevention of ventricular tachycardia in patients with long QT syndrome type 2: a description of 4 cases. J. Electrocardiol. 45 (3), 237–243. doi: 10.1016/j.jelectrocard.2012.01.008
Jacobs, A., Knight, B. P., McDonald, K. T., Burke, M. C. (2006). Verapamil decreases ventricular tachyarrhythmias in a patient with timothy syndrome (LQT8). Heart Rhythm 3 (8), 967–970. doi: 10.1016/j.hrthm.2006.04.024
Jalife, J., Delmar, M., Anumonwo, J., Berenfeld, O., Kalifa, J. (2009). Basic mechanisms of cardiac arrhythmias. Basic Cardiac Electrophysiol. Clinician 2.
January, C. T., Riddle, J. M. (1989). Early afterdepolarizations: mechanism of induction and block. A role for L-type Ca2+ current. Circ. Res. 64 (5), 977–990. doi: 10.1161/01.res.64.5.977
Jensen, H. H., Brohus, M., Nyegaard, M., Overgaard, M. T. (2018). Human calmodulin mutations. Front. Mol. Neurosci. 11, 396. doi: 10.3389/fnmol.2018.00396
Jiang, D., Wang, R., Xiao, B., Kong, H., Hunt, D. J., Choi, P., et al. (2005). Enhanced store overload-induced Ca2+ release and channel sensitivity to luminal Ca2+ activation are common defects of RyR2 mutations linked to ventricular tachycardia and sudden death. Circ. Res. 97 (11), 1173–1181. doi: 10.1161/01.RES.0000192146.85173.4b
Jiang, D., Chen, W., Wang, R., Zhang, L., Chen, S. R. (2007). Loss of luminal Ca2+ activation in the cardiac ryanodine receptor is associated with ventricular fibrillation and sudden death. Proc. Natl. Acad. Sci. U. S. A. 104 (46), 18309–18314. doi: 10.1073/pnas.0706573104
Johnson, D. M., Antoons, G. (2018). Arrhythmogenic mechanisms in heart failure: linking beta-adrenergic stimulation, stretch, and calcium. Front. Physiol. 9, 1453. doi: 10.3389/fphys.2018.01453
Jones, P. P., Jiang, D., Bolstad, J., Hunt, D. J., Zhang, L., Demaurex, N., et al. (2008). Endoplasmic reticulum Ca2+ measurements reveal that the cardiac ryanodine receptor mutations linked to cardiac arrhythmia and sudden death alter the threshold for store-overload-induced Ca2+ release. Biochem. J. 412 (1), 171–178. doi: 10.1042/BJ20071287
Joung, B., Chen, P. S., Lin, S. F. (2011). The role of the calcium and the voltage clocks in sinoatrial node dysfunction. Yonsei Med. J. 52 (2), 211–219. doi: 10.3349/ymj.2011.52.2.211
Kandel, S. M., Roth, B. J. (2015). The mechanism of reflection type reentry: a simulation study. J. Cardiovasc. Electrophysiol. 26 (12), 1370–1375. doi: 10.1111/jce.12815
Kang, J., Chen, X. L., Wang, H., Ji, J., Cheng, H., Incardona, J., et al. (2005). Discovery of a small molecule activator of the human ether-a-go-go-related gene (HERG) cardiac K+ channel. Mol. Pharmacol. 67 (3), 827–836. doi: 10.1124/mol.104.006577
Kannankeril, P. J., Moore, J. P., Cerrone, M., Priori, S. G., Kertesz, N. J., Ro, P. S., et al. (2017). Efficacy of flecainide in the treatment of catecholaminergic polymorphic ventricular tachycardia: a randomized clinical trial. JAMA Cardiol. 2 (7), 759–766. doi: 10.1001/jamacardio.2017.1320
Karagueuzian, H. S., Pezhouman, A., Angelini, M., Olcese, R. (2017). Enhanced late na and ca currents as effective antiarrhythmic drug targets. Front. Pharmacol. 8, 36. doi: 10.3389/fphar.2017.00036
Kashimura, T., Briston, S. J., Trafford, A. W., Napolitano, C., Priori, S. G., Eisner, D. A., et al. (2010). In the RyR2(R4496C) mouse model of CPVT, beta-adrenergic stimulation induces Ca waves by increasing SR Ca content and not by decreasing the threshold for Ca waves. Circ. Res. 107 (12), 1483–1489. doi: 10.1161/CIRCRESAHA.110.227744
Kim, J. A., Lopes, C. M., Moss, A. J., McNitt, S., Barsheshet, A., Robinson, J. L., et al. (2010). Trigger-specific risk factors and response to therapy in long QT syndrome type 2. Heart Rhythm 7 (12), 1797–1805. doi: 10.1016/j.hrthm.2010.09.011
Kirchhof, P., Benussi, S., Kotecha, D., Ahlsson, A., Atar, D., Casadei, B., et al. (2016). ESC Guidelines for the management of atrial fibrillation developed in collaboration with EACTS. Eur. J. Cardiothorac. Surg. 50 (5), e1–e88. doi: 10.1093/ejcts/ezw313
Kistamas, K., Hegyi, B., Vaczi, K., Horvath, B., Banyasz, T., Magyar, J., et al. (2015a). Oxidative shift in tissue redox potential increases beat-to-beat variability of action potential duration. Can. J. Physiol. Pharmacol. 93 (7), 563–568. doi: 10.1139/cjpp-2014-0531
Kistamas, K., Szentandrassy, N., Hegyi, B., Vaczi, K., Ruzsnavszky, F., Horvath, B., et al. (2015b). Changes in intracellular calcium concentration influence beat-to-beat variability of action potential duration in canine ventricular myocytes. J. Physiol. Pharmacol. 66 (1), 73–81.
Knollmann, B. C., Chopra, N., Hlaing, T., Akin, B., Yang, T., Ettensohn, K., et al. (2006). Casq2 deletion causes sarcoplasmic reticulum volume increase, premature Ca2+ release, and catecholaminergic polymorphic ventricular tachycardia. J. Clin. Invest. 116 (9), 2510–2520. doi: 10.1172/JCI29128
Kohomoto, O., Levi, A. J., Bridge, J. H. (1994). Relation between reverse sodium-calcium exchange and sarcoplasmic reticulum calcium release in guinea pig ventricular cells. Circ. Res. 74 (3), 550–554. doi: 10.1161/01.res.74.3.550
Krause, U., Gravenhorst, V., Kriebel, T., Ruschewski, W., Paul, T. (2011). A rare association of long QT syndrome and syndactyly: Timothy syndrome (LQT 8). Clin. Res. Cardiol. 100 (12), 1123–1127. doi: 10.1007/s00392-011-0358-4
Lahat, H., Pras, E., Olender, T., Avidan, N., Ben-Asher, E., Man, O., et al. (2001). A missense mutation in a highly conserved region of CASQ2 is associated with autosomal recessive catecholamine-induced polymorphic ventricular tachycardia in Bedouin families from Israel. Am. J. Hum. Genet. 69 (6), 1378–1384. doi: 10.1086/324565
Laitinen, P. J., Brown, K. M., Piippo, K., Swan, H., Devaney, J. M., Brahmbhatt, B., et al. (2001). Mutations of the cardiac ryanodine receptor (RyR2) gene in familial polymorphic ventricular tachycardia. Circulation 103 (4), 485–490. doi: 10.1161/01.cir.103.4.485
Lakatta, E. G., DiFrancesco, D. (2009). What keeps us ticking: a funny current, a calcium clock, or both? J. Mol. Cell. Cardiol. 47 (2), 157–170. doi: 10.1016/j.yjmcc.2009.03.022
Lakatta, E. G., Maltsev, V. A., Vinogradova, T. M. (2010). A coupled SYSTEM of intracellular Ca2+ clocks and surface membrane voltage clocks controls the timekeeping mechanism of the heart's pacemaker. Circ. Res. 106 (4), 659–673. doi: 10.1161/CIRCRESAHA.109.206078
Lakatta, E. G. (2010). A paradigm shift for the heart's pacemaker. Heart Rhythm 7 (4), 559–564. doi: 10.1016/j.hrthm.2009.12.013
Landstrom, A. P., Boczek, N. J., Ye, D., Miyake, C. Y., De la Uz, C. M., Allen, H. D., et al. (2016). Novel long QT syndrome-associated missense mutation, L762F, in CACNA1C-encoded L-type calcium channel imparts a slower inactivation tau and increased sustained and window current. Int. J. Cardiol. 220, 290–298. doi: 10.1016/j.ijcard.2016.06.081
Landstrom, A. P., Dobrev, D., Wehrens, X. H. T. (2017). Calcium signaling and cardiac arrhythmias. Circ. Res. 120 (12), 1969–1993. doi: 10.1161/CIRCRESAHA.117.310083
Lazrak, A., Peres, A., Giovannardi, S., Peracchia, C. (1994). Ca-mediated and independent effects of arachidonic acid on gap junctions and Ca-independent effects of oleic acid and halothane. Biophys. J. 67 (3), 1052–1059. doi: 10.1016/S0006-3495(94)80570-3
Lazzara, R. (1988). Electrophysiological mechanisms for ventricular arrhythmias. Clin. Cardiol. 11 (3 Suppl 2), II1–II4.
Lazzerini, P. E., Capecchi, P. L., Laghi-Pasini, F. (2015). Long QT syndrome: an emerging role for inflammation and immunity. Front. Cardiovasc. Med. 2, 26. doi: 10.3389/fcvm.2015.00026
Leenhardt, A., Lucet, V., Denjoy, I., Grau, F., Ngoc, D. D., Coumel, P. (1995). Catecholaminergic polymorphic ventricular tachycardia in children. A 7-year follow-up of 21 patients. Circulation 91 (5), 1512–1519. doi: 10.1161/01.cir.91.5.1512
Lehnart, S. E., Wehrens, X. H., Laitinen, P. J., Reiken, S. R., Deng, S. X., Cheng, Z., et al. (2004). Sudden death in familial polymorphic ventricular tachycardia associated with calcium release channel (ryanodine receptor) leak. Circulation 109 (25), 3208–3214. doi: 10.1161/01.CIR.0000132472.98675.EC
Li, Y., Diaz, M. E., Eisner, D. A., O'Neill, S. (2009). The effects of membrane potential, SR Ca2+ content and RyR responsiveness on systolic Ca2+ alternans in rat ventricular myocytes. J. Physiol. 587 (Pt 6), 1283–1292. doi: 10.1113/jphysiol.2008.164368
Limpitikul, W. B., Dick, I. E., Joshi-Mukherjee, R., Overgaard, M. T., George, A. L., Jr., Yue, D. T. (2014). Calmodulin mutations associated with long QT syndrome prevent inactivation of cardiac L-type Ca(2+) currents and promote proarrhythmic behavior in ventricular myocytes. J. Mol. Cell. Cardiol. 74, 115–124. doi: 10.1016/j.yjmcc.2014.04.022
Lipp, P., Niggli, E. (1994). Modulation of Ca2+ release in cultured neonatal rat cardiac myocytes. Insight from subcellular release patterns revealed by confocal microscopy. Circ. Res. 74 (5), 979–990. doi: 10.1161/01.res.74.5.979
Liu, D. W., Antzelevitch, C. (1995). Characteristics of the delayed rectifier current (IKr and IKs) in canine ventricular epicardial, midmyocardial, and endocardial myocytes. a weaker IKs contributes to the longer action potential of the M cell. Circ. Res. 76 (3), 351–365. doi: 10.1161/01.res.76.3.351
Liu, N., Colombi, B., Memmi, M., Zissimopoulos, S., Rizzi, N., Negri, S., et al. (2006). Arrhythmogenesis in catecholaminergic polymorphic ventricular tachycardia: insights from a RyR2 R4496C knock-in mouse model. Circ. Res. 99 (3), 292–298. doi: 10.1161/01.RES.0000235869.50747.e1
Liu, N., Rizzi, N., Boveri, L., Priori, S. G. (2009). Ryanodine receptor and calsequestrin in arrhythmogenesis: what we have learnt from genetic diseases and transgenic mice. J. Mol. Cell. Cardiol. 46 (2), 149–159. doi: 10.1016/j.yjmcc.2008.10.012
Liu, N., Ruan, Y., Denegri, M., Bachetti, T., Li, Y., Colombi, B., et al. (2011a). Calmodulin kinase II inhibition prevents arrhythmias in RyR2(R4496C+/-) mice with catecholaminergic polymorphic ventricular tachycardia. J. Mol. Cell. Cardiol. 50 (1), 214–222. doi: 10.1016/j.yjmcc.2010.10.001
Liu, N., Denegri, M., Ruan, Y., Avelino-Cruz, J. E., Perissi, A., Negri, S., et al. (2011b). Short communication: flecainide exerts an antiarrhythmic effect in a mouse model of catecholaminergic polymorphic ventricular tachycardia by increasing the threshold for triggered activity. Circ. Res. 109 (3), 291–295. doi: 10.1161/CIRCRESAHA.111.247338
Liu, H., Chatel, S., Simard, C., Syam, N., Salle, L., Probst, V., et al. (2013). Molecular genetics and functional anomalies in a series of 248 Brugada cases with 11 mutations in the TRPM4 channel. PLoS One 8 (1), e54131. doi: 10.1371/journal.pone.0054131
Liu, W., Deng, J., Wang, G., Zhang, C., Luo, X., Yan, D., et al. (2014). KCNE2 modulates cardiac L-type Ca(2+) channel. J. Mol. Cell. Cardiol. 72, 208–218. doi: 10.1016/j.yjmcc.2014.03.013
Liu, M. B., de Lange, E., Garfinkel, A., Weiss, J. N., Qu, Z. (2015). Delayed afterdepolarizations generate both triggers and a vulnerable substrate promoting reentry in cardiac tissue. Heart Rhythm 12 (10), 2115–2124. doi: 10.1016/j.hrthm.2015.06.019
Loughrey, C. M., Otani, N., Seidler, T., Craig, M. A., Matsuda, R., Kaneko, N., et al. (2007). K201 modulates excitation-contraction coupling and spontaneous Ca2+ release in normal adult rabbit ventricular cardiomyocytes. Cardiovasc. Res. 76 (2), 236–246. doi: 10.1016/j.cardiores.2007.06.014
Lu, Y., Mahaut-Smith, M. P., Huang, C. L., Vandenberg, J. I. (2003). Mutant MiRP1 subunits modulate HERG K+ channel gating: a mechanism for pro-arrhythmia in long QT syndrome type 6. J. Physiol. 551 (Pt 1), 253–262. doi: 10.1113/jphysiol.2003.046045
Lukas, A., Antzelevitch, C. (1996). Phase 2 reentry as a mechanism of initiation of circus movement reentry in canine epicardium exposed to simulated ischemia. Cardiovasc. Res. 32 (3), 593–603. doi: 10.1016/S0008-6363(96)00115-0
Lukyanenko, V., Subramanian, S., Gyorke, I., Wiesner, T. F., Gyorke, S. (1999). The role of luminal Ca2+ in the generation of Ca2+ waves in rat ventricular myocytes. J. Physiol. 518 (Pt 1), 173–186. doi: 10.1111/j.1469-7793.1999.0173r.x
Magyar, J., Banyasz, T., Szentandrassy, N., Kistamas, K., Nanasi, P. P., Satin, J. (2015). Role of gap junction channel in the development of beat-to-beat action potential repolarization variability and arrhythmias. Curr. Pharma. Des. 21 (8), 1042–1052. doi: 10.2174/1381612820666141029102443
Magyar, J., Kistamas, K., Vaczi, K., Hegyi, B., Horvath, B., Banyasz, T., et al. (2016). Concept of relative variability of cardiac action potential duration and its test under various experimental conditions. Gen. Physiol. Biophys. 35 (1), 55–62. doi: 10.4149/gpb_2015019
Mahajan, A., Shiferaw, Y., Sato, D., Baher, A., Olcese, R., Xie, L. H., et al. (2008). A rabbit ventricular action potential model replicating cardiac dynamics at rapid heart rates. Biophys. J. 94 (2), 392–410. doi: 10.1529/biophysj.106.98160
Maier, L. S., Bers, D. M. (2007). Role of Ca2+/calmodulin-dependent protein kinase (CaMK) in excitation-contraction coupling in the heart. Cardiovasc. Res. 73 (4), 631–640. doi: 10.1016/j.cardiores.2006.11.005
Maltsev, V. A., Vinogradova, T. M., Lakatta, E. G. (2006). The emergence of a general theory of the initiation and strength of the heartbeat. J. Pharmacol. Sci. 100 (5), 338–369. doi: 10.1254/jphs.cr0060018
Marsman, R. F., Barc, J., Beekman, L., Alders, M., Dooijes, D., van den Wijngaard, A., et al. (2014). A mutation in CALM1 encoding calmodulin in familial idiopathic ventricular fibrillation in childhood and adolescence. J. Am. Coll. Cardiol. 63 (3), 259–266. doi: 10.1016/j.jacc.2013.07.091
Maruyama, M., Joung, B., Tang, L., Shinohara, T., On, Y. K., Han, S., et al. (2010). Diastolic intracellular calcium-membrane voltage coupling gain and postshock arrhythmias: role of purkinje fibers and triggered activity. Circ. Res. 106 (2), 399–408. doi: 10.1161/CIRCRESAHA.109.211292
Mayer, A. G. (1906). Rhythmical Pulsation in Scyphomedusae (Washington DC: Carnegie Institution of Washington).
Mechmann, S., Pott, L. (1986). Identification of Na-Ca exchange current in single cardiac myocytes. Nature 319 (6054), 597–599. doi: 10.1038/319597a0
Medeiros-Domingo, A., Kaku, T., Tester, D. J., Iturralde-Torres, P., Itty, A., Ye, B., et al. (2007). SCN4B-encoded sodium channel beta4 subunit in congenital long-QT syndrome. Circulation 116 (2), 134–142. doi: 10.1161/CIRCULATIONAHA.106.659086
Meregalli, P. G., Wilde, A. A., Tan, H. L. (2005). Pathophysiological mechanisms of Brugada syndrome: depolarization disorder, repolarization disorder, or more? Cardiovasc. Res. 67 (3), 367–378. doi: 10.1016/j.cardiores.2005.03.005
Milberg, P., Ramtin, S., Monnig, G., Osada, N., Wasmer, K., Breithardt, G., et al. (2004). Comparison of the in vitro electrophysiologic and proarrhythmic effects of amiodarone and sotalol in a rabbit model of acute atrioventricular block. J. Cardiovasc. Pharmacol. 44 (3), 278–286. doi: 10.1097/01.fjc.0000129581.81508.78
Mines, G. R. (1913). On dynamic equilibrium in the heart. J. Physiol. 46 (4-5), 349–383. doi: 10.1113/jphysiol.1913.sp001596
Mines, G. R. (1914). On circulating excitations in heart muscle and their possible relation to tachycardia and fibrillation. Trans. R Soc. Can. 8, 43–52.
Mitchell, R. D., Simmerman, H. K., Jones, L. R. (1988). Ca2+ binding effects on protein conformation and protein interactions of canine cardiac calsequestrin. J. Biol. Chem. 263 (3), 1376–1381.
Miura, M., Ishide, N., Oda, H., Sakurai, M., Shinozaki, T., Takishima, T. (1993). Spatial features of calcium transients during early and delayed afterdepolarizations. Am. J. Physiol. 265 (2 Pt 2), H439–H444. doi: 10.1152/ajpheart.1993.265.2.H439
Miura, M., Ishide, N., Numaguchi, H., Takishima, T. (1995). Diversity of early afterdepolarizations in guinea pig myocytes: spatial characteristics of intracellular Ca2+ concentration. Heart Vessels 10 (5), 266–274. doi: 10.1007/BF01744906
Mohamed, U., Gollob, M. H., Gow, R. M., Krahn, A. D. (2006). Sudden cardiac death despite an implantable cardioverter-defibrillator in a young female with catecholaminergic ventricular tachycardia. Heart Rhythm 3 (12), 1486–1489. doi: 10.1016/j.hrthm.2006.08.018
Mohler, P. J., Splawski, I., Napolitano, C., Bottelli, G., Sharpe, L., Timothy, K., et al. (2004). A cardiac arrhythmia syndrome caused by loss of ankyrin-B function. Proc. Natl. Acad. Sci. U. S. A. 101 (24), 9137–9142. doi: 10.1073/pnas.0402546101
Mohler, P. J., Schott, J. J., Gramolini, A. O., Dilly, K. W., Guatimosim, S., duBell, W. H., et al. (2003). Ankyrin-B mutation causes type 4 long-QT cardiac arrhythmia and sudden cardiac death. Nature 421 (6923), 634–639. doi: 10.1038/nature01335
Morita, H., Morita, S. T., Nagase, S., Banba, K., Nishii, N., Tani, Y., et al. (2003). Ventricular arrhythmia induced by sodium channel blocker in patients with Brugada syndrome. J. Am. Coll. Cardiol. 42 (9), 1624–1631. doi: 10.1016/j.jacc.2003.06.004
Moss, A. J., Zareba, W., Hall, W. J., Schwartz, P. J., Crampton, R. S., Benhorin, J., et al. (2000). Effectiveness and limitations of beta-blocker therapy in congenital long-QT syndrome. Circulation 101 (6), 616–623. doi: 10.1161/01.cir.101.6.616
Moss, A. J., Windle, J. R., Hall, W. J., Zareba, W., Robinson, J. L., McNitt, S., et al. (2005). Safety and efficacy of flecainide in subjects with Long QT-3 syndrome (DeltaKPQ mutation): a randomized, double-blind, placebo-controlled clinical trial. Ann. Noninvasive Electrocardiol. 10 (4 Suppl), 59–66. doi: 10.1111/j.1542-474X.2005.00077.x
Moss, A. J., Zareba, W., Schwarz, K. Q., Rosero, S., McNitt, S., Robinson, J. L. (2008). Ranolazine shortens repolarization in patients with sustained inward sodium current due to type-3 long-QT syndrome. J. Cardiovasc. Electrophysiol. 19 (12), 1289–1293. doi: 10.1111/j.1540-8167.2008.01246.x
Nanasi, P. P., Magyar, J., Varro, A., Ordog, B. (2017). Beat-to-beat variability of cardiac action potential duration: underlying mechanism and clinical implications. Can. J. Physiol. Pharmacol. 95 (10), 1230–1235. doi: 10.1139/cjpp-2016-0597
Nattel, S., Dobrev, D. (2016). Electrophysiological and molecular mechanisms of paroxysmal atrial fibrillation. Nat. Rev. Cardiol. 13 (10), 575–590. doi: 10.1038/nrcardio.2016.118
Nawathe, P. A., Kryukova, Y., Oren, R. V., Milanesi, R., Clancy, C. E., Lu, J. T., et al. (2013). An LQTS6 MiRP1 mutation suppresses pacemaker current and is associated with sinus bradycardia. J. Cardiovasc. Electrophysiol. 24 (9), 1021–1027. doi: 10.1111/jce.12163
Neef, S., Dybkova, N., Sossalla, S., Ort, K. R., Fluschnik, N., Neumann, K., et al. (2010). CaMKII-dependent diastolic SR Ca2+ leak and elevated diastolic Ca2+ levels in right atrial myocardium of patients with atrial fibrillation. Circ. Res. 106 (6), 1134–1144. doi: 10.1161/CIRCRESAHA.109.203836
Nolasco, J. B., Dahlen, R. W. (1968). A graphic method for the study of alternation in cardiac action potentials. J. Appl. Physiol. 25 (2), 191–196. doi: 10.1152/jappl.1968.25.2.191
Nyegaard, M., Overgaard, M. T., Sondergaard, M. T., Vranas, M., Behr, E. R., Hildebrandt, L. L., et al. (2012). Mutations in calmodulin cause ventricular tachycardia and sudden cardiac death. Am. J. Hum. Genet. 91 (4), 703–712. doi: 10.1016/j.ajhg.2012.08.015
Ohgo, T., Okamura, H., Noda, T., Satomi, K., Suyama, K., Kurita, T., et al. (2007). Acute and chronic management in patients with Brugada syndrome associated with electrical storm of ventricular fibrillation. Heart Rhythm 4 (6), 695–700. doi: 10.1016/j.hrthm.2007.02.014
Oosthoek, P. W., Viragh, S., Lamers, W. H., Moorman, A. F. (1993). Immunohistochemical delineation of the conduction system. II: The atrioventricular node and Purkinje fibers. Circ. Res. 73 (3), 482–491. doi: 10.1161/01.res.73.3.482
Overend, C. L., Eisner, D. A., O'Neill, S. C. (1997). The effect of tetracaine on spontaneous Ca2+ release and sarcoplasmic reticulum calcium content in rat ventricular myocytes. J. Physiol. 502 (Pt 3), 471–479. doi: 10.1111/j.1469-7793.1997.471bj.x
Pertsov, A. M., Davidenko, J. M., Salomonsz, R., Baxter, W. T., Jalife, J. (1993). Spiral waves of excitation underlie reentrant activity in isolated cardiac muscle. Circ. Res. 72 (3), 631–650. doi: 10.1161/01.res.72.3.631
Pflaumer, A., Davis, A. M. (2012). Guidelines for the diagnosis and management of catecholaminergic polymorphic ventricular tachycardia. Heart Lung Circ. 21 (2), 96–100. doi: 10.1016/j.hlc.2011.10.008
Picht, E., DeSantiago, J., Blatter, L. A., Bers, D. M. (2006). Cardiac alternans do not rely on diastolic sarcoplasmic reticulum calcium content fluctuations. Circ. Res. 99 (7), 740–748. doi: 10.1161/01.RES.0000244002.88813.91
Plaster, N. M., Tawil, R., Tristani-Firouzi, M., Canun, S., Bendahhou, S., Tsunoda, A., et al. (2001). Mutations in Kir2.1 cause the developmental and episodic electrical phenotypes of Andersen's syndrome. Cell 105 (4), 511–519. doi: 10.1016/s0092-8674(01)00342-7
Pogwizd, S. M., Schlotthauer, K., Li, L., Yuan, W., Bers, D. M. (2001). Arrhythmogenesis and contractile dysfunction in heart failure: Roles of sodium-calcium exchange, inward rectifier potassium current, and residual beta-adrenergic responsiveness. Circ. Res. 88 (11), 1159–1167. doi: 10.1161/hh1101.091193
Postma, A. V., Denjoy, I., Kamblock, J., Alders, M., Lupoglazoff, J. M., Vaksmann, G., et al. (2005). Catecholaminergic polymorphic ventricular tachycardia: RYR2 mutations, bradycardia, and follow up of the patients. J. Med. Genet. 42 (11), 863–870. doi: 10.1136/jmg.2004.028993
Pott, C., Dechering, D. G., Reinke, F., Muszynski, A., Zellerhoff, S., Bittner, A., et al. (2011). Successful treatment of catecholaminergic polymorphic ventricular tachycardia with flecainide: a case report and review of the current literature. Europace 13 (6), 897–901. doi: 10.1093/europace/euq517
Priori, S. G., Corr, P. B. (1990). Mechanisms underlying early and delayed afterdepolarizations induced by catecholamines. Am. J. Physiol. 258 (6 Pt 2), H1796–H1805. doi: 10.1152/ajpheart.1990.258.6.H1796
Priori, S. G., Napolitano, C., Tiso, N., Memmi, M., Vignati, G., Bloise, R., et al. (2001). Mutations in the cardiac ryanodine receptor gene (hRyR2) underlie catecholaminergic polymorphic ventricular tachycardia. Circulation 103 (2), 196–200. doi: 10.1161/01.cir.103.2.196
Priori, S. G., Wilde, A. A., Horie, M., Cho, Y., Behr, E. R., Berul, C., et al. (2013). Executive summary: HRS/EHRA/APHRS expert consensus statement on the diagnosis and management of patients with inherited primary arrhythmia syndromes. Heart Rhythm 10 (12), e85–108. doi: 10.1016/j.hrthm.2013.07.021
Qi, X. Y., Yeh, Y. H., Xiao, L., Burstein, B., Maguy, A., Chartier, D., et al. (2008). Cellular signaling underlying atrial tachycardia remodeling of L-type calcium current. Circ. Res. 103 (8), 845–854. doi: 10.1161/CIRCRESAHA.108.175463
Qu, Z., Weiss, J. N. (2015). Mechanisms of ventricular arrhythmias: from molecular fluctuations to electrical turbulence. Annu. Rev. Physiol. 77, 29–55. doi: 10.1146/annurev-physiol-021014-071622
Qu, Z., Shiferaw, Y., Weiss, J. N. (2007). Nonlinear dynamics of cardiac excitation-contraction coupling: an iterated map study. Phys. Rev. E Stat. Nonlinear Soft Matter Phys. 75 (1 Pt 1), 011927. doi: 10.1103/PhysRevE.75.011927
Radwanski, P. B., Ho, H. T., Veeraraghavan, R., Brunello, L., Liu, B., Belevych, A. E., et al. (2016). Neuronal Na(+) channels are integral components of pro-arrhythmic Na(+)/Ca(2+) signaling nanodomain that promotes cardiac arrhythmias during beta-adrenergic stimulation. JACC Basic to Trans. Sci. 1 (4), 251–266. doi: 10.1016/j.jacbts.2016.04.004
Reid, D. S., Tynan, M., Braidwood, L., Fitzgerald, G. R. (1975). Bidirectional tachycardia in a child. a study using his bundle electrography. Br. Heart J. 37 (3), 339–344. doi: 10.1136/hrt.37.3.339
Reiken, S., Gaburjakova, M., Guatimosim, S., Gomez, A. M., D'Armiento, J., Burkhoff, D., et al. (2003). Protein kinase a phosphorylation of the cardiac calcium release channel (ryanodine receptor) in normal and failing hearts. Role of phosphatases and response to isoproterenol. J. Biol. Chem. 278 (1), 444–453. doi: 10.1074/jbc.M207028200
Ringer, S. (1883). A further contribution regarding the influence of the different constituents of the blood on the contraction of the heart. J. Physiol. 4 (1), 29–42. doi: 10.1113/jphysiol.1883.sp000120
Rizzi, N., Liu, N., Napolitano, C., Nori, A., Turcato, F., Colombi, B., et al. (2008). Unexpected structural and functional consequences of the R33Q homozygous mutation in cardiac calsequestrin: a complex arrhythmogenic cascade in a knock in mouse model. Circ. Res. 103 (3), 298–306. doi: 10.1161/CIRCRESAHA.108.171660
Roden, D. M. (2004). Drug-induced prolongation of the QT interval. New Engl. J. Med. 350 (10), 1013–1022. doi: 10.1056/NEJMra032426
Roden, D. M. (2006). Long QT syndrome: reduced repolarization reserve and the genetic link. J. Internal Med. 259 (1), 59–69. doi: 10.1111/j.1365-2796.2005.01589.x
Rosen, M. R., Gelband, H., Merker, C., Hoffman, B. F. (1973). Mechanisms of digitalis toxicity. effects of ouabain on phase four of canine Purkinje fiber transmembrane potentials. Circulation 47 (4), 681–689. doi: 10.1161/01.cir.47.4.681
Rosen, M. R., Wit, A. L., Hoffman, B. F. (1975). Electrophysiology and pharmacology of cardiac arrhythmias. IV. Cardiac antiarrhythmic and toxic effects of digitalis. Am. Heart J. 89 (3), 391–399. doi: 10.1016/0002-8703(75)90090-3
Rosenbaum, D. S., Jackson, L. E., Smith, J. M., Garan, H., Ruskin, J. N., Cohen, R. J. (1994). Electrical alternans and vulnerability to ventricular arrhythmias. New Engl. J. Med. 330 (4), 235–241. doi: 10.1056/NEJM199401273300402
Rosenthal, J. E. (1988). Reflected reentry in depolarized foci with variable conduction impairment in 1 day old infarcted canine cardiac tissue. J. Am. Coll. Cardiol. 12 (2), 404–411. doi: 10.1016/0735-1097(88)90413-5
Rosero, S. Z., Zareba, W., Robinson, J. L., Moss, A. J. (1997). Gene-Specific Therapy for Long QT Syndrome. Ann. Noninvasive Electrocardiol. 2 (3), 274–278. doi: 10.1111/j.1542-474X.1997.tb00336.x
Rosso, R., Kalman, J. M., Rogowski, O., Diamant, S., Birger, A., Biner, S., et al. (2007). Calcium channel blockers and beta-blockers versus beta-blockers alone for preventing exercise-induced arrhythmias in catecholaminergic polymorphic ventricular tachycardia. Heart Rhythm 4 (9), 1149–1154. doi: 10.1016/j.hrthm.2007.05.017
Rosso, R., Kogan, E., Belhassen, B., Rozovski, U., Scheinman, M. M., Zeltser, D., et al. (2008). J-point elevation in survivors of primary ventricular fibrillation and matched control subjects: incidence and clinical significance. J. Am. Coll. Cardiol. 52 (15), 1231–1238. doi: 10.1016/j.jacc.2008.07.010
Rozanski, G. J., Lipsius, S. L. (1985). Electrophysiology of functional subsidiary pacemakers in canine right atrium. Am. J. Physiol. 249 (3 Pt 2), H594–H603. doi: 10.1152/ajpheart.1985.249.3.H594
Sanguinetti, M. C., Jiang, C., Curran, M. E., Keating, M. T. (1995). A mechanistic link between an inherited and an acquired cardiac arrhythmia: HERG encodes the IKr potassium channel. Cell 81 (2), 299–307. doi: 10.1016/0092-8674(95)90340-2
Sanguinetti, M. C., Curran, M. E., Zou, A., Shen, J., Spector, P. S., Atkinson, D. L., et al. (1996). Coassembly of K(V)LQT1 and minK (IsK) proteins to form cardiac I(Ks) potassium channel. Nature 384 (6604), 80–83. doi: 10.1038/384080a0
Sato, D., Shiferaw, Y., Garfinkel, A., Weiss, J. N., Qu, Z., Karma, A. (2006). Spatially discordant alternans in cardiac tissue: role of calcium cycling. Circ. Res. 99 (5), 520–527. doi: 10.1161/01.RES.0000240542.03986.e7
Saunders, J. H., Ferrier, G. R., Moe, G. K. (1973). Conduction block associated with transient depolarizations induced by acetylstrophanthidin in isolated canine Purkinje fibers. Circ. Res. 32 (5), 610–617. doi: 10.1161/01.res.32.5.610
Schotten, U., Dobrev, D., Platonov, P. G., Kottkamp, H., Hindricks, G. (2016). Current controversies in determining the main mechanisms of atrial fibrillation. J. Internal Med. 279 (5), 428–438. doi: 10.1111/joim.12492
Schwartz, P. J., Priori, S. G., Locati, E. H., Napolitano, C., Cantu, F., Towbin, J. A., et al. (1995). Long QT syndrome patients with mutations of the SCN5A and HERG genes have differential responses to Na+ channel blockade and to increases in heart rate. Implications for gene-specific therapy. Circulation 92 (12), 3381–3386. doi: 10.1161/01.cir.92.12.3381
Schwartz, P. J., Crotti, L., Insolia, R. (2012). Long-QT syndrome: from genetics to management. Circ. Arrhythmia Electrophysiol. 5 (4), 868–877. doi: 10.1161/CIRCEP.111.962019
Shah, D. P., Baez-Escudero, J. L., Weisberg, I. L., Beshai, J. F., Burke, M. C. (2012). Ranolazine safely decreases ventricular and atrial fibrillation in timothy syndrome (LQT8). Pacing Clin. Electrophysiol. 35 (3), e62–e64. doi: 10.1111/j.1540-8159.2010.02913.x
Shamgar, L., Ma, L., Schmitt, N., Haitin, Y., Peretz, A., Wiener, R., et al. (2006). Calmodulin is essential for cardiac IKS channel gating and assembly: impaired function in long-QT mutations. Circ. Res. 98 (8), 1055–1063. doi: 10.1161/01.RES.0000218979.40770.69
Shan, J., Xie, W., Betzenhauser, M., Reiken, S., Chen, B. X., Wronska, A., et al. (2012). Calcium leak through ryanodine receptors leads to atrial fibrillation in 3 mouse models of catecholaminergic polymorphic ventricular tachycardia. Circ. Res. 111 (6), 708–717. doi: 10.1161/CIRCRESAHA.112.273342
Shiferaw, Y., Watanabe, M. A., Garfinkel, A., Weiss, J. N., Karma, A. (2003). Model of intracellular calcium cycling in ventricular myocytes. Biophys. J. 85 (6), 3666–3686. doi: 10.1016/S0006-3495(03)74784-5
Shimizu, W., Kurita, T., Matsuo, K., Suyama, K., Aihara, N., Kamakura, S., et al. (1998). Improvement of repolarization abnormalities by a K+ channel opener in the LQT1 form of congenital long-QT syndrome. Circulation 97 (16), 1581–1588. doi: 10.1161/01.cir.97.16.1581
Shimizu, W., Antzelevitch, C., Suyama, K., Kurita, T., Taguchi, A., Aihara, N., et al. (2000). Effect of sodium channel blockers on ST segment, QRS duration, and corrected QT interval in patients with Brugada syndrome. J. Cardiovasc. Electrophysiol. 11 (12), 1320–1329. doi: 10.1046/j.1540-8167.2000.01320.x
Shkryl, V., Maxwell, J., Domeier, T., Blatter, L. (2012). Refractoriness of sarcoplasmic reticulum Ca2+ release determines Ca2+ alternans in atrial myocytes. Am. J. Physiol. Heart Circ. Physiol. 302 (11), H2310–H2320. doi: 10.1152/ajpheart.00079.2012
Sicouri, S., Antzelevitch, C. (2018). Mechanisms underlying the actions of antidepressant and antipsychotic drugs that cause sudden cardiac arrest. Arrhythmia Electrophysiol. Rev. 7 (3), 199–209. doi: 10.15420/aer.2018.29.2
Simard, C., Hof, T., Keddache, Z., Launay, P., Guinamard, R. (2013). The TRPM4 non-selective cation channel contributes to the mammalian atrial action potential. J. Mol. Cell. Cardiol. 59, 11–19. doi: 10.1016/j.yjmcc.2013.01.019
Sipido, K. R., Maes, M., Van de Werf, F. (1997). Low efficiency of Ca2+ entry through the Na(+)-Ca2+ exchanger as trigger for Ca2+ release from the sarcoplasmic reticulum. a comparison between L-type Ca2+ current and reverse-mode Na(+)-Ca2+ exchange. Circ. Res. 81 (6), 1034–1044. doi: 10.1161/01.res.81.6.1034
Sipido, K. R., Bito, V., Antoons, G., Volders, P. G., Vos, M. A. (2007). Na/Ca exchange and cardiac ventricular arrhythmias. Ann. N. Y. Acad. Sci. 1099, 339–348. doi: 10.1196/annals.1387.066
Sondergaard, M. T., Tian, X., Liu, Y., Wang, R., Chazin, W. J., Chen, S. R., et al. (2015). Arrhythmogenic calmodulin mutations affect the activation and termination of cardiac ryanodine receptor-mediated Ca2+ release. J. Biol. Chem. 290 (43), 26151–26162. doi: 10.1074/jbc.M115.676627
Sondergaard, M. T., Liu, Y., Larsen, K. T., Nani, A., Tian, X., Holt, C., et al. (2017). The Arrhythmogenic Calmodulin p.Phe142Leu mutation impairs c-domain ca2+ binding but not calmodulin-dependent inhibition of the cardiac ryanodine receptor. J. Biol. Chem. 292 (4), 1385–1395. doi: 10.1074/jbc.M116.766253
Song, L., Alcalai, R., Arad, M., Wolf, C. M., Toka, O., Conner, D. A., et al. (2007). Calsequestrin 2 (CASQ2) mutations increase expression of calreticulin and ryanodine receptors, causing catecholaminergic polymorphic ventricular tachycardia. J. Clin. Invest. 117 (7), 1814–1823. doi: 10.1172/JCI31080
Sood, S., Chelu, M. G., van Oort, R. J., Skapura, D., Santonastasi, M., Dobrev, D., et al. (2008). Intracellular calcium leak due to FKBP12.6 deficiency in mice facilitates the inducibility of atrial fibrillation. Heart Rhythm 5 (7), 1047–1054. doi: 10.1016/j.hrthm.2008.03.030
Splawski, I., Tristani-Firouzi, M., Lehmann, M. H., Sanguinetti, M. C., Keating, M. T. (1997). Mutations in the hminK gene cause long QT syndrome and suppress IKs function. Nat. Genet. 17 (3), 338–340. doi: 10.1038/ng1197-338
Splawski, I., Timothy, K. W., Sharpe, L. M., Decher, N., Kumar, P., Bloise, R., et al. (2004). Ca(V)1.2 calcium channel dysfunction causes a multisystem disorder including arrhythmia and autism. Cell 119 (1), 19–31. doi: 10.1016/j.cell.2004.09.011
Suetomi, T., Yano, M., Uchinoumi, H., Fukuda, M., Hino, A., Ono, M., et al. (2011). Mutation-linked defective interdomain interactions within ryanodine receptor cause aberrant Ca(2)(+)release leading to catecholaminergic polymorphic ventricular tachycardia. Circulation 124 (6), 682–694. doi: 10.1161/CIRCULATIONAHA.111.023259
Sumitomo, N., Nakamura, T., Fukuhara, J., Nakai, T., Watanabe, I., Mugishima, H., et al. (2010). Clinical effectiveness of pulmonary vein isolation for arrhythmic events in a patient with catecholaminergic polymorphic ventricular tachycardia. Heart Vessels 25 (5), 448–452. doi: 10.1007/s00380-009-1214-6
Sung, R. J., Wu, S. N., Wu, J. S., Chang, H. D., Luo, C. H. (2006). Electrophysiological mechanisms of ventricular arrhythmias in relation to Andersen-Tawil syndrome under conditions of reduced IK1: a simulation study. Am. J. Physiol. Heart Circ. Physiol. 291 (6), H2597–H2605. doi: 10.1152/ajpheart.00393.2006
Surawicz, B. (1998). U wave: facts, hypotheses, misconceptions, and misnomers. J. Cardiovasc. Electrophysiol. 9 (10), 1117–1128. doi: 10.1111/j.1540-8167.1998.tb00890.x
Swan, H., Piippo, K., Viitasalo, M., Heikkila, P., Paavonen, T., Kainulainen, K., et al. (1999). Arrhythmic disorder mapped to chromosome 1q42-q43 causes malignant polymorphic ventricular tachycardia in structurally normal hearts. J. Am. Coll. Cardiol. 34 (7), 2035–2042. doi: 10.1016/s0735-1097(99)00461-1
Swan, H., Laitinen, P., Kontula, K., Toivonen, L. (2005). Calcium channel antagonism reduces exercise-induced ventricular arrhythmias in catecholaminergic polymorphic ventricular tachycardia patients with RyR2 mutations. J. Cardiovasc. Electrophysiol. 16 (2), 162–166. doi: 10.1046/j.1540-8167.2005.40516.x
Szabo, B., Sweidan, R., Rajagopalan, C. V., Lazzara, R. (1994). Role of Na+:Ca2+ exchange current in Cs(+)-induced early afterdepolarizations in Purkinje fibers. J. Cardiovasc. Electrophysiol. 5 (11), 933–944. doi: 10.1111/j.1540-8167.1994.tb01133.x
Szabo, G., Szentandrassy, N., Biro, T., Toth, B. I., Czifra, G., Magyar, J., et al. (2005). Asymmetrical distribution of ion channels in canine and human left-ventricular wall: epicardium versus midmyocardium. Pflugers Archiv. 450 (5), 307–316. doi: 10.1007/s00424-005-1445-z
Szabo, L., Szentandrassy, N., Kistamas, K., Hegyi, B., Ruzsnavszky, F., Vaczi, K., et al. (2013). Effects of tacrolimus on action potential configuration and transmembrane ion currents in canine ventricular cells. Naunyn-Schmiedeberg's Arch. Pharmacol. 386 (3), 239–246. doi: 10.1007/s00210-012-0823-2
Szentandrassy, N., Kistamas, K., Hegyi, B., Horvath, B., Ruzsnavszky, F., Vaczi, K., et al. (2015). Contribution of ion currents to beat-to-beat variability of action potential duration in canine ventricular myocytes. Pflugers Archiv. 467 (7), 1431–1443. doi: 10.1007/s00424-014-1581-4
Takamatsu, T., Wier, W. G. (1990). Calcium waves in mammalian heart: quantification of origin, magnitude, waveform, and velocity. FASEB J. 4 (5), 1519–1525. doi: 10.1096/fasebj.4.5.2307330
Terentyev, D., Nori, A., Santoro, M., Viatchenko-Karpinski, S., Kubalova, Z., Gyorke, I., et al. (2006). Abnormal interactions of calsequestrin with the ryanodine receptor calcium release channel complex linked to exercise-induced sudden cardiac death. Circ. Res. 98 (9), 1151–1158. doi: 10.1161/01.RES.0000220647.93982.08
Thiel, W. H., Chen, B., Hund, T. J., Koval, O. M., Purohit, A., Song, L. S., et al. (2008). Proarrhythmic defects in Timothy syndrome require calmodulin kinase II. Circulation 118 (22), 2225–2234. doi: 10.1161/CIRCULATIONAHA.108.788067
Thomsen, M. B., Verduyn, S. C., Stengl, M., Beekman, J. D., de Pater, G., van Opstal, J., et al. (2004). Increased short-term variability of repolarization predicts d-sotalol-induced torsades de pointes in dogs. Circulation 110 (16), 2453–2459. doi: 10.1161/01.CIR.0000145162.64183.C8
Trafford, A. W., Sibbring, G. C., Diaz, M. E., Eisner, D. A. (2000). The effects of low concentrations of caffeine on spontaneous Ca release in isolated rat ventricular myocytes. Cell Calcium 28 (4), 269–276. doi: 10.1054/ceca.2000.0156
Tse, G. (2016). Mechanisms of cardiac arrhythmias. J. Arrhythmia 32 (2), 75–81. doi: 10.1016/j.joa.2015.11.003
Uchinoumi, H., Yano, M., Suetomi, T., Ono, M., Xu, X., Tateishi, H., et al. (2010). Catecholaminergic polymorphic ventricular tachycardia is caused by mutation-linked defective conformational regulation of the ryanodine receptor. Circ. Res. 106 (8), 1413–1424. doi: 10.1161/CIRCRESAHA.109.209312
van der Werf, C., Kannankeril, P. J., Sacher, F., Krahn, A. D., Viskin, S., Leenhardt, A., et al. (2011). Flecainide therapy reduces exercise-induced ventricular arrhythmias in patients with catecholaminergic polymorphic ventricular tachycardia. J. Am. Coll. Cardiol. 57 (22), 2244–2254. doi: 10.1016/j.jacc.2011.01.026
Van Hemel, N. M., Swenne, C. A., De Bakker, J. M., Defauw, J. J., Guiraudon, G. M. (1988). Epicardial reflection as a cause of incessant ventricular bigeminy. Pacing Clin. Electrophysiol. 11 (7), 1036–1044. doi: 10.1111/j.1540-8159.1988.tb03949.x
Vega, A. L., Tester, D. J., Ackerman, M. J., Makielski, J. C. (2009). Protein kinase A-dependent biophysical phenotype for V227F-KCNJ2 mutation in catecholaminergic polymorphic ventricular tachycardia. Circ. Arrhythm. Electrophysiol. 2 (5), 540–547. doi: 10.1161/CIRCEP.109.872309
Venetucci, L. A., Trafford, A. W., Diaz, M. E., O'Neill, S. C., Eisner, D. A. (2006). Reducing ryanodine receptor open probability as a means to abolish spontaneous Ca2+ release and increase Ca2+ transient amplitude in adult ventricular myocytes. Circ. Res. 98 (10), 1299–1305. doi: 10.1161/01.RES.0000222000.35500.65
Venetucci, L. A., Trafford, A. W., Eisner, D. A. (2007). Increasing ryanodine receptor open probability alone does not produce arrhythmogenic calcium waves: threshold sarcoplasmic reticulum calcium content is required. Circ. Res. 100 (1), 105–111. doi: 10.1161/01.RES.0000252828.17939.00
Venetucci, L., Denegri, M., Napolitano, C., Priori, S. G. (2012). Inherited calcium channelopathies in the pathophysiology of arrhythmias. Nat. Rev. Cardiol. 9 (10), 561–575. doi: 10.1038/nrcardio.2012.93
Vermeulen, J. T., McGuire, M. A., Opthof, T., Coronel, R., de Bakker, J. M., Klopping, C., et al. (1994). Triggered activity and automaticity in ventricular trabeculae of failing human and rabbit hearts. Cardiovasc. Res. 28 (10), 1547–1554. doi: 10.1093/cvr/28.10.1547
Verrier, R. L., Klingenheben, T., Malik, M., El-Sherif, N., Exner, D. V., Hohnloser, S. H., et al. (2011). Microvolt T-wave alternans physiological basis, methods of measurement, and clinical utility–consensus guideline by international society for holter and noninvasive electrocardiology. J. Am. Coll. Cardiol. 58 (13), 1309–1324. doi: 10.1016/j.jacc.2011.06.029
Viatchenko-Karpinski, S., Terentyev, D., Gyorke, I., Terentyeva, R., Volpe, P., Priori, S. G., et al. (2004). Abnormal calcium signaling and sudden cardiac death associated with mutation of calsequestrin. Circ. Res. 94 (4), 471–477. doi: 10.1161/01.RES.0000115944.10681.EB
Vinogradova, T. M., Lyashkov, A. E., Zhu, W., Ruknudin, A. M., Sirenko, S., Yang, D., et al. (2006). High basal protein kinase a-dependent phosphorylation drives rhythmic internal Ca2+ store oscillations and spontaneous beating of cardiac pacemaker cells. Circ. Res. 98 (4), 505–514. doi: 10.1161/01.RES.0000204575.94040.d1
Voigt, N., Li, N., Wang, Q., Wang, W., Trafford, A. W., Abu-Taha, I., et al. (2012). Enhanced sarcoplasmic reticulum Ca2+ leak and increased Na+-Ca2+ exchanger function underlie delayed afterdepolarizations in patients with chronic atrial fibrillation. Circulation 125 (17), 2059–2070. doi: 10.1161/CIRCULATIONAHA.111.067306
Volders, P. G., Kulcsar, A., Vos, M. A., Sipido, K. R., Wellens, H. J., Lazzara, R., et al. (1997). Similarities between early and delayed afterdepolarizations induced by isoproterenol in canine ventricular myocytes. Cardiovasc. Res. 34 (2), 348–359. doi: 10.1016/s0008-6363(96)00270-2
Volders, P. G., Vos, M. A., Szabo, B., Sipido, K. R., de Groot, S. H., Gorgels, A. P., et al. (2000). Progress in the understanding of cardiac early afterdepolarizations and torsades de pointes: time to revise current concepts. Cardiovasc. Res. 46 (3), 376–392. doi: 10.1016/s0008-6363(00)00022-5
Vukmir, R. B., Stein, K. L. (1991). Torsades de pointes therapy with phenytoin. Ann. Emerg. Med. 20 (2), 198–200. doi: 10.1016/s0196-0644(05)81223-5
Wang, Q., Shen, J., Splawski, I., Atkinson, D., Li, Z., Robinson, J. L., et al. (1995). SCN5A mutations associated with an inherited cardiac arrhythmia, long QT syndrome. Cell 80 (5), 805–811. doi: 10.1016/0092-8674(95)90359-3
Wang, S., Trumble, W. R., Liao, H., Wesson, C. R., Dunker, A. K., Kang, C. H. (1998). Crystal structure of calsequestrin from rabbit skeletal muscle sarcoplasmic reticulum. Nat. Struct. Biol. 5 (6), 476–483. doi: 10.1038/nsb0698-476
Watanabe, H., Chopra, N., Laver, D., Hwang, H. S., Davies, S. S., Roach, D. E., et al. (2009). Flecainide prevents catecholaminergic polymorphic ventricular tachycardia in mice and humans. Nat. Med. 15 (4), 380–383. doi: 10.1038/nm.1942
Wegener, F. T., Ehrlich, J. R., Hohnloser, S. H. (2008). Amiodarone-associated macroscopic T-wave alternans and torsade de pointes unmasking the inherited long QT syndrome. Europace 10 (1), 112–113. doi: 10.1093/europace/eum252
Weiss, J. N., Chen, P. S., Qu, Z., Karagueuzian, H. S., Garfinkel, A. (2000). Ventricular fibrillation: how do we stop the waves from breaking? Circ. Res. 87 (12), 1103–1107. doi: 10.1161/01.res.87.12.1103
Weiss, J. N., Karma, A., Shiferaw, Y., Chen, P. S., Garfinkel, A., Qu, Z. (2006). From pulsus to pulseless: the saga of cardiac alternans. Circ. Res. 98 (10), 1244–1253. doi: 10.1161/01.RES.0000224540.97431.f0
Weiss, J. N., Garfinkel, A., Karagueuzian, H. S., Nguyen, T. P., Olcese, R., Chen, P. S., et al. (2015). Perspective: a dynamics-based classification of ventricular arrhythmias. J. Mol. Cell. Cardiol. 82, 136–152. doi: 10.1016/j.yjmcc.2015.02.017
Wier, W. G., Cannell, M. B., Berlin, J. R., Marban, E., Lederer, W. J. (1987). Cellular and subcellular heterogeneity of [Ca2+]i in single heart cells revealed by fura-2. Science 235 (4786), 325–328. doi: 10.1126/science.3798114
Wilders, R., Verkerk, A. O. (2018). Long QT syndrome and sinus bradycardia-a mini review. Front. Cardiovasc. Med. 5, 106. doi: 10.3389/fcvm.2018.00106
Wit, A. L., Cranefield, P. F. (1977). Triggered and automatic activity in the canine coronary sinus. Circ. Res. 41 (4), 434–445. doi: 10.1161/01.res.41.4.434
Wit, A. L., Hoffman, B. F., Cranefield, P. F. (1972). Slow conduction and reentry in the ventricular conducting system. I. return extrasystole in canine purkinje fibers. Circ. Res. 30 (1), 1–10. doi: 10.1161/01.res.30.1.1
Wren, L. M., Jimenez-Jaimez, J., Al-Ghamdi, S., Al-Aama, J. Y., Bdeir, A., Al-Hassnan, Z. N., et al. (2019). Genetic mosaicism in calmodulinopathy. Circ. Genom. Precis. Med. 12 (9), 375–385. doi: 10.1161/CIRCGEN.119.002581
Wu, T. J., Lin, S. F., Weiss, J. N., Ting, C. T., Chen, P. S. (2002). Two types of ventricular fibrillation in isolated rabbit hearts: importance of excitability and action potential duration restitution. Circulation 106 (14), 1859–1866. doi: 10.1161/01.cir.0000031334.49170.fb
Wu, G., Ai, T., Kim, J. J., Mohapatra, B., Xi, Y., Li, Z., et al. (2008). alpha-1-syntrophin mutation and the long-QT syndrome: a disease of sodium channel disruption. Circ. Arrhythmia Electrophysiol. 1 (3), 193–201. doi: 10.1161/CIRCEP.108.769224
Xie, L. H., Sato, D., Garfinkel, A., Qu, Z., Weiss, J. N. (2008). Intracellular Ca alternans: coordinated regulation by sarcoplasmic reticulum release, uptake, and leak. Biophys. J. 95 (6), 3100–3110. doi: 10.1529/biophysj.108.130955
Xie, L. H., Chen, F., Karagueuzian, H. S., Weiss, J. N. (2009). Oxidative-stress-induced afterdepolarizations and calmodulin kinase II signaling. Circ. Res. 104 (1), 79–86. doi: 10.1161/CIRCRESAHA.108.183475
Yamamoto, T., Yano, M., Xu, X., Uchinoumi, H., Tateishi, H., Mochizuki, M., et al. (2008). Identification of target domains of the cardiac ryanodine receptor to correct channel disorder in failing hearts. Circulation 117 (6), 762–772. doi: 10.1161/CIRCULATIONAHA.107.718957
Yan, G. X., Antzelevitch, C. (1999). Cellular basis for the Brugada syndrome and other mechanisms of arrhythmogenesis associated with ST-segment elevation. Circulation 100 (15), 1660–1666. doi: 10.1161/01.cir.100.15.1660
Yang, Y., Yang, Y., Liang, B., Liu, J., Li, J., Grunnet, M., et al. (2010). Identification of a Kir3.4 mutation in congenital long QT syndrome. Am. J. Hum. Genet. 86 (6), 872–880. doi: 10.1016/j.ajhg.2010.04.017
Yang, T., Chun, Y. W., Stroud, D. M., Mosley, J. D., Knollmann, B. C., Hong, C., et al. (2014). Screening for acute IKr block is insufficient to detect torsades de pointes liability: role of late sodium current. Circulation 130 (3), 224–234. doi: 10.1161/CIRCULATIONAHA.113.007765
Yano, M., Yamamoto, T., Kobayashi, S., Matsuzaki, M. (2009). Role of ryanodine receptor as a Ca(2)(+) regulatory center in normal and failing hearts. J. Cardiol. 53 (1), 1–7. doi: 10.1016/j.jjcc.2008.10.008
Yarotskyy, V., Gao, G., Du, L., Ganapathi, S. B., Peterson, B. Z., Elmslie, K. S. (2010). Roscovitine binds to novel L-channel (CaV1.2) sites that separately affect activation and inactivation. J. Biol. Chem. 285 (1), 43–53. doi: 10.1074/jbc.M109.076448
Yazawa, M., Hsueh, B., Jia, X., Pasca, A. M., Bernstein, J. A., Hallmayer, J., et al. (2011). using induced pluripotent stem cells to investigate cardiac phenotypes in Timothy syndrome. Nature 471 (7337), 230–234. doi: 10.1038/nature09855
Zaza, A., Belardinelli, L., Shryock, J. C. (2008). Pathophysiology and pharmacology of the cardiac “late sodium current.” Pharmacol. Ther. 119 (3), 326–339. doi: 10.1016/j.pharmthera.2008.06.001
Zhou, Q., Xiao, J., Jiang, D., Wang, R., Vembaiyan, K., Wang, A., et al. (2011). Carvedilol and its new analogs suppress arrhythmogenic store overload-induced Ca2+ release. Nat. Med. 17 (8), 1003–1009. doi: 10.1038/nm.2406
Zhu, Z. I., Clancy, C. E. (2007). L-type Ca2+ channel mutations and T-wave alternans: a model study. Am. J. Physiol. Heart Circulatory Physiol. 293 (6), H3480–H3489. doi: 10.1152/ajpheart.00476.2007
Keywords: calcium signalling, cardiac arrhythmias, catecholaminergic polymorphic ventricular tachycardia, long QT syndrome, atrial fibrillation, reentry, early afterdepolarization, delayed afterdepolarization
Citation: Kistamás K, Veress R, Horváth B, Bányász T, Nánási PP and Eisner DA (2020) Calcium Handling Defects and Cardiac Arrhythmia Syndromes. Front. Pharmacol. 11:72. doi: 10.3389/fphar.2020.00072
Received: 15 November 2019; Accepted: 24 January 2020;
Published: 25 February 2020.
Edited by:
Ebru Arioglu Inan, Ankara University, TurkeyReviewed by:
Sung Joon Kim, Seoul National University College of Medicine, South KoreaMurat Oz, Health Science Center, Kuwait
Sanda Despa, University of Kentucky, United States
Copyright © 2020 Kistamás, Veress, Horváth, Bányász, Nánási and Eisner. This is an open-access article distributed under the terms of the Creative Commons Attribution License (CC BY). The use, distribution or reproduction in other forums is permitted, provided the original author(s) and the copyright owner(s) are credited and that the original publication in this journal is cited, in accordance with accepted academic practice. No use, distribution or reproduction is permitted which does not comply with these terms.
*Correspondence: Kornél Kistamás, a2lzdGFtYXMua29ybmVsQG1lZC51bmlkZWIuaHU=