- 1Department of Biomedical and Biotechnological Sciences, School of Medicine, University of Catania, Catania, Italy
- 2Department of Experimental Medicine, Division of Pharmacology, University of Campania “Luigi Vanvitelli”, Naples, Italy
- 3Eye Clinic, Multidisciplinary Department of Medical, Surgical and Dental Sciences, University of Campania “Luigi Vanvitelli”, Naples, Italy
- 4Center for Research in Ocular Pharmacology-CERFO, University of Catania, Catania, Italy
Retinal hypoxia is one of the causative factors of diabetic retinopathy and is also one of the triggers of VEGF release. We hypothesized that specific dysregulated miRNAs in diabetic retinopathy could be linked to hypoxia-induced damage in human retinal endothelial cells (HRECs). We investigated in HRECs the effects of chemical (CoCl2) hypoxia on the expression of HIF-1α, VEGF, PlGF, and of a focused set of miRNAs. We found that miR-20a-5p, miR-20b-5p, miR-27a-3p, miR-27b-3p, miR-206-3p, miR-381-3p correlated also with expression of TGFβ signaling pathway genes in HRECs, challenged with chemical hypoxic stimuli. In conclusion, our data suggest that retinal angiogenesis would be promoted, at least under HIF-1α activation, by upregulation of PlGF and other factors such as miRNAs, VEGFA, and TGFβ1.
Introduction
Diabetic retinopathy (DR), a complication of diabetes, is a microvascular disease with a strong inflammatory imprinting. Vascular endothelial growth factor (VEGF) is a key player in retinal neovascularization, and intraocular injections of anti-VEGF agents are currently the established therapies for diabetic macular edema, along with steroids (Bandello et al., 2012). Although not fully elucidated, alterations in retinal hemodynamics and reduced blood flow may be detrimental for DR, along with uncontrolled hyperglycemia (Schmetterer and Wolzt, 1999; Schmidl et al., 2015). Furthermore, during DR progression, local or global changes in retinal oxygenation may cause the development of hypoxic areas (Arden and Sivaprasad, 2012) and oxidative stress (Bucolo et al., 2006). Similar to the etiopathogenesis of retinopathy of prematurity (ROP), induction of hypoxia-inducible factor-1 α (HIF-1α) may be responsible for the production of vascular endothelial growth factor (VEGFA), which is the main cause of retinal neovascularization (Aiello et al., 1994; Arjamaa and Nikinmaa, 2006; Abu El-Asrar et al., 2012). Furthermore, HIF-1α and VEGFA crosstalk in ocular neovascularization has been widely investigated (Ozaki et al., 1999; Rodrigues et al., 2016). In particular, the HIF-1α inhibition strategy has also been explored for treatment of retinal neovascularization (Iwase et al., 2013; D’Amico et al., 2015; D’Amico et al., 2017; Zeng et al., 2017).
Besides VEGFA, HIF-1α can also induce the placental growth factor (PlGF) (Zimna and Kurpisz, 2015; Charnock-Jones, 2016; Mitsui et al., 2018), an emerging target in retinal neovascular diseases (Kwon and Jee, 2018; Lee et al., 2018; Saddala et al., 2018; Lazzara et al., 2019; Van Bergen et al., 2019). Furthermore, HIF-1α is involved in expression of several microRNAs (miRNAs), that are named HypoxamiRs if they bear in their promoter region the hypoxia responsive elements (HREs) (Nallamshetty et al., 2013; Bertero et al., 2017). Indeed, HypoxamiRs, regulated by HIF-1α dependent or independent mechanisms, are tightly involved in molecular and cellular changes triggered by hypoxia (Cottrill et al., 2014; Gee et al., 2014; Greco et al., 2014; Bertero et al., 2017). Moreover, several genes, that are target of HypoxamiRs, belong to the VEGFR2 signaling pathway (Gupta et al., 2018). This pathway regulates angiogenic response of endothelial cells (Abhinand et al., 2016), and represents the target of current approved treatments for neovascular retinal degenerations (Bandello et al., 2012). We recently evidenced the dysregulation of expression pattern of 8 miRNAs (miR-20a-5p, miR-20a3p, miR-20b-5p, miR-106a-5p, miR-27a-5p, miR-27b-3p, miR-206-3p, and miR-381-3p) in retina and serum of diabetic mice, representing intriguing and potent mediators in the DR pathological mechanisms (Platania et al., 2019). HREs were found in promoter region of miR-20a, miR-20b, miR-106, miR-27a, that indeed, have been enlisted as Hypoxamirs (Nallamshetty et al., 2013). Although HREs are not present in miR-206-3p, miR-381 and miR-27b promoter regions, these miRNAs were found to be modulated in several hypoxic experimental setting (Yue et al., 2013; Choudhry and Mole, 2016; Gupta et al., 2018; Lu et al., 2018).
Therefore, we hereby hypothesized that these eight miRNAs could also be involved in activation of HIF-1/angiogenic axis in retinal endothelial cells. With this aim, we stabilized, by cobalt chloride treatment, HIF-1α protein in human retinal endothelial cells (HRECs), in order to analyze the activation of HIF-1/VEGFA-PlGF axis, along with expression of a focused set of miRNAs, previously found to be dysregulated in an in vivo model of DR (Platania et al., 2019). A bioinformatic approach guided the identification and in vitro validation of alternative target genes of miRNAs, dysregulated after inhibition of HIF-1α degradation. We analyzed the expression of genes of the TGFβ (Transforming growth factor beta) signaling pathway, which is an emerging target in DR (Li et al., 2018; Stafiej et al., 2018) and was found to be one of top pathways modulated by HypoxamiRs target genes (Gupta et al., 2018).
Material and Methods
Reagents
Mouse monoclonal anti-HIF-1α (catalog n. sc-13515), mouse anti-GAPDH (catalog n. 2118) antibodies were purchased from Santa Cruz Biotechnology, Inc. (CA, USA), and Cell-Signaling Technology (Leiden, Netherlands), respectively. Secondary goat anti-mouse IRDye 680LT, (catalog n. 926-68020) were purchased from LI-COR (Lincoln, NE, USA). Cobalt chloride (0.1 M solution, catalog n. 15862) from Sigma-Aldrich (Saint Louis, MO, USA).
Cell Culture
Human retinal endothelial cells were purchased from Innoprot® (Derio – Bizkaia, Spain). Cells were cultured at 37°C, in humidified atmosphere (5% CO2), in Endothelial cell medium (ECM) supplemented with 5% fetal bovine serum (FBS), 1% ECGS (Endothelial Cell Growth Supplement) and 100 U/ml penicillin 100 μg/ml streptomycin. HRECs (cell passage number 4) for each experiment were seeded setting 4×105 as final cell density.
Induction of Chemical Hypoxia In Vitro
Cobalt chloride (CoCl2) is commonly used to stabilize HIF-1α, because it inhibits the HIF-1α degradation, as shown in several in vitro settings, including primary human retinal endothelial cells cultures as previously described (Gao et al., 2008; Hu et al., 2012; Li et al., 2017; He et al., 2019). Preliminary studies were carried out and HRECs cultures were treated with various concentrations of CoCl2 (100–200 μM), in order to assess cell tolerability for 24 h with MTT test (Supplementary Data). The concentration used for all experiments was 200 μM, accordingly to previous CoCl2 concentrations tested on retinal ganglion cells (Balaiya et al., 2012; Li et al., 2017). Cells were seeded in Petri dishes (passage number 4, cell density 4×105); after reaching confluence (approximately 80%), cells were treated with CoCl2 for 30 min, 2 and 8 h to induce HIF-1α accumulation/nuclear translocation.
Western Blot
HRECs were cultured in 60 mm Petri dishes (cell density 4×105). Proteins from cell lysates were extracted with RIPA Buffer, including protease and phosphatase inhibitors cocktail (Sigma-Aldrich, St. Louis, MO, USA). Total protein content, in each cell lysate sample, was determined by the BCA Assay Kit (Pierce™ BCA Protein Assay Kit, Invitrogen, Life Technologies, Carlsbad, CA, USA). Extracted proteins (40 µg) were loaded on 4%–12% tris-glycine gel. After electrophoresis proteins were transferred into a nitrocellulose membrane (Invitrogen, Life Technologies, Carlsbad, CA, USA). Immunoblot was preceded by addition of Odyssey Blocking Buffer (LI-COR Lincoln, NE, USA) to membranes. Therefore, membranes were incubated overnight (4°C) with appropriate primary HIF-1α (1:200 dilution) and anti-GAPDH (1:500 dilution) antibodies. GAPDH was selected as control for protein expression, accordingly to previous reports (Botlagunta et al., 2011; Ao et al., 2015; Evrard et al., 2016; Gao et al., 2019). After overnight incubation, the membranes were then incubated with secondary fluorescent antibodies (1: 10,000 dilution) for 1 h at room temperature. Immunoblot was detected through Odyssey imaging system (LI-COR, Lincoln, NE, USA). Densitometry analyses of blots were performed at nonsaturating exposures and analyzed using the ImageJ software (NIH, Bethesda, MD, USA; available at http://rsb.info.nih.gov/ij/index.html). Values were normalized to GAPDH, which was also used as loading control (see supplemental information for whole gel membranes immunoblots).
Extraction of Total RNA and cDNA Synthesis
Extraction of the total RNA was performed with TRIzol Reagent (Invitrogen, Life Technologies, Carlsbad, CA, USA). The A260/A280 ratio of the optical density of RNA samples (measured with Multimode Reader Flash di Varioskan™) was 1.95–2.01. This RNA purity was confirmed with the electrophoresis in nondenaturing 1% agarose gel (in TAE), that showed an adequate RNA purity, concentration, and integrity. cDNA was synthesized from 2 µg RNA with a reverse transcription kit (SuperScript™ II Reverse Transcriptase, Invitrogen, ThermoFisher Scientific, Carlsbad, CA, USA).
Real-Time Reverse Transcriptase-Polymerase Chain Reaction (qRT-PCR) for PlGF and VEGFA
Real-time RT-PCR was carried out with LightCycler ® 2.0 (Real-Time PCR System Roche Life Science). The amplification reaction mix included iTaq™ Universal SYBR® Green Supermix (Bio-Rad, Hercules, CA, USA) and 1 µl (100 ng) of cDNA. Forty-five amplification cycles were carried out for each sample. Results were analyzed with the 2-ΔΔCt method. VEGF and PlGF mRNAs expression were normalized to human 18S mRNA levels. Primers used in qPCR for 18S, VEGF-A, PlGF expression are: 18S (human) Forward (5’-AGTCCCTGCCCTTTGTACACA-3’), Reverse (5’-GATCCGAGGGCCTCACTAAAC-3’); PlGF (human) Forward (5′-ATGTTCAGCCCATCCTGTGT-3′) Reverse (5′-CTTCATCTTCTCCCGCAGAG-3′); VEGF-A (human) Forward (5’-GAGGTTTGATCCGCATAATCTG-3’) Reverse (5’-ATCTTCAAGCCATCCTGTGTGC- 3’).
Analysis of miRNAs
HRECs total RNA, including small RNAs, was obtained following the miRNeasy Mini Kit (21700400, Qiagen), according to the manufacturer’s protocol “Purification of Total RNA, Including Small RNAs, from Animal Cells”. Particularly, for miRNAs isolation, Syn-cel-miR-39-3p miScript miRNA Mimic 5 nM (MSY0000010, Qiagen) was added to each sample before RNA purification in order to monitor miRNAs isolation efficacy. RNA quality and concentration were determined by using NanoDrop 2000c spectrophotometer (Thermo Fisher Scientific, Carlsbad, CA, USA). Gene Amp PCR System 9700 (Applied Biosystems Thermo Fisher Scientific, Carlsbad, CA, USA) was used for reverse-transcription phase. Mature miRNAs were converted in cDNA according the MiScript II Reverse Transcription Kit (218161, Qiagen, Germantown, MD, USA), starting from 615 ng of total RNA.CFX96 Real-Time System C1000 Touch Thermal Cycler (Bio-Rad, Hercules, CA, USA) was used to evaluate the expression levels of hsa-miR-20a-5p (Accession number MIMAT0000075), hsa-miR-20b-5p (Accession number MIMAT0001413); hsa-miR-27a-3p (Accession number MIMAT0000084), hsa-miR-27b-3p (Accession number MIMAT0000419), hsa-miR-206-3p (Accession number MIMAT0000462) and hsa-miR-381-3p (Accession number MIMAT0000736). Real time PCR was carried out with miScript SYBR Green PCR kit (218073, Qiagen, Germantown, MD, USA) and specific miScript primer Assays (MS00003199, MS00003206, MS00003241, MS00031668, MS00003787 and MS00004116, Qiagen, Germantown, MD, USA). The expression of the 6 miRNAs analyzed was normalized by using Ce_miR-39-3p (MIMAT0000010) as control (MS00019789, Qiagen, Germantown, MD, USA).
TGFβ Pathway qRT-PCR
Total RNA (615 ng) was subjected to reverse-transcription reaction with the Gene Amp PCR System 9700 (Applied Biosystems Life Technologies, Carlsbad, CA, USA) and Quantitect Reverse Transcription kit (205311, Qiagen, Germantown, MD, USA), following the manufacturer’s protocol “Reverse Transcription with Elimination of Genomic DNA for Quantitative, Real-Time PCR”. The expression levels of human TGFB1 (Transforming Growth Factor Beta 1-Gene ID 7040), TGFBR1 (Transforming growth factor beta receptor 1-Gene ID 7046), TGFBR2 (Transforming growth factor beta receptor 2-Gene ID 7048) and SMAD2 (Small mother against decapentaplegic 2-Gene ID 4087) genes were evaluated by real time PCR measurement, by using a CFX96 Real-Time System C1000 Touch Thermal Cycler (BioRad Laboratories, Inc), Quantitect SYBR Green PCR Kit (204143, Qiagen, Germantown, MD, USA) and specific Quantitect Primer Assays (QT00000728, QT00083412, QT00014350 and QT00004207, Qiagen, Germantown, MD, USA) following the manufacturer’s protocol “Two-Step RT-PCR (Standard Protocol)”. Human GAPDH (Gene ID 2597) (QT00079247, Qiagen, Germantown, MD, USA) was used as control to normalize the expression of the 4 genes analyzed; accordingly to previous reports (Botlagunta et al., 2011; Ao et al., 2015; Lin et al., 2015; Rosen et al., 2015; Evrard et al., 2016; Shao and Yao, 2016; Gao et al., 2019; Jiang and Xu, 2019).
MicroRNA or TGFβ Signaling Pathway Genes Expression Determination Analysis
CFX Manager™ Software (Bio-Rad, Hercules, CA, USA) was used to calculate Cycle threshold (Ct) values. Data analysis was carried out with the 2-ΔΔCt method. Particularly, ΔCt value for each miRNA or gene profiled was calculated as ΔCt = Ct miRNA – Ct Ce_miR-39-5p or as ΔCt = Ct gene – Ct GAPDH. Then, ΔΔCt was calculated as ΔCttime x – ΔCttime 0, where time x is the analyzed time point and time 0 is the expression of the target miRNA normalized to Ce-miR-39-5p or of the target gene normalized to GAPDH (Livak and Schmittgen, 2001). Where data are reported as fold-regulation, this was the inverse negative of fold change (2^-ΔΔCt) for fold change values lower than one (downregulation). In case of upregulation, the fold-regulation was equal to fold change (2^-ΔΔCt) for fold change values greater than 1.
Bioinformatics
In order to explore alternative factors and pathways regulated by miRNAs, dysregulated with induction of chemical hypoxia in human retinal endothelial cells, we predicted the combinatorial effect of hsa-miR-20a-5p, hsa-miR-20b-5p, hsa-miR-27a-3p, has-miR-27b-3p, has-miR-260b-3p, and has-miR-381-3p on biological pathways by means of the DIANA miRPath webserver (Vlachos et al., 2015). The miRNA:target interactions were analyzed with application of Tarbase algorithm (Riffo-Campos et al., 2016), which is based on experimental validated miRNA:target interaction.
Statistical Analysis
All results were reported as mean ± SD from four independent in-vitro experiments, where each group was triplicated in plates as technical replicate. The results were analyzed using one-way ANOVA, followed by Tukey-Kramer post-hoc multiple comparisons test. Differences between groups were considered significant for p-value < 0.05. Graphs design and statistical analysis were carried out with GraphPad Prism 5 software (GraphPad Inc., San Diego, CA, USA).
Results
Chemical Hypoxia in HRECs and Angiogenic Factors
CoCl2 treatment, by inhibition of HIF-1α degradation, significantly increased stabilization of HIF-1α protein in HRECs (Figure 1A and Supplementary Data). HIF-1α is a well-known inducer of VEGFA and PlGF (Aiello et al., 1994; Ozaki et al., 1999; Arjamaa and Nikinmaa, 2006; Abu El-Asrar et al., 2012; Zimna and Kurpisz, 2015; Charnock-Jones, 2016; Rodrigues et al., 2016; Mitsui et al., 2018), but the HIF-1α protein levels did not correlate with expression pattern of VEGFA, within the analyzed time-points (Figure 1B). Two hours after CoCl2 treatment, VEGFA expression increased, compared to control HRECs. While, after 8 h, VEGFA levels significantly (p<0.05) decreased, compared to levels detected 2 h after, CoCl2 treatment. On the other hand, the expression pattern of PlGF correlated with HIF-1α protein levels, within the analyzed time-points (Figure 1C).
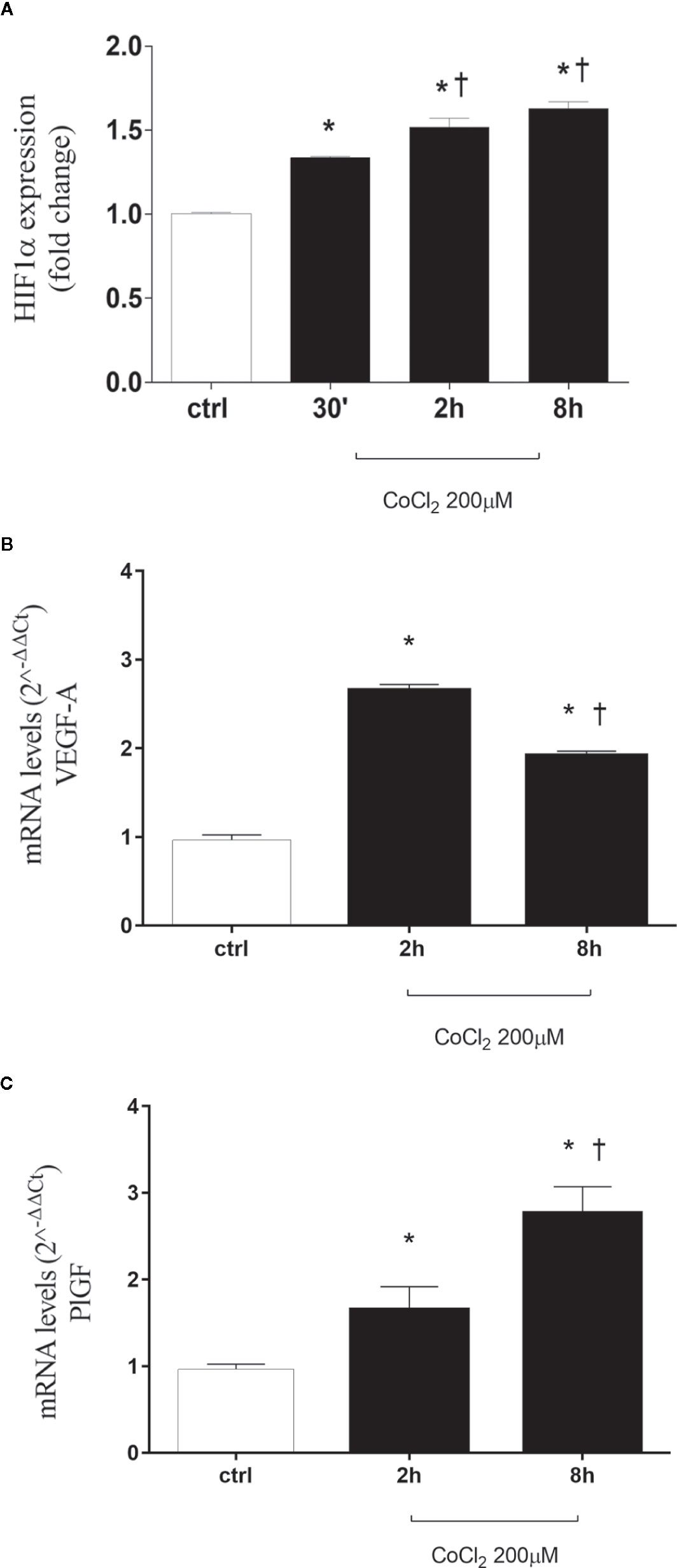
Figure 1 CoCl2 treatment induces HIF-1α stabilization, vascular endothelial growth factor (VEGFA), and placental growth factor (PlGF) expression in human retinal endothelial cells. (A) Densitometric analysis of western blot of HIF-1α and GAPDH in human retinal endothelial cells (HRECs) exposed to CoCl2 for 30 min, 2 h and 8 h; each bar represents the mean value ± SD (n=4). *p< 0.05 vs. control; †p < 0.05 vs. 30 min CoCl2 treatments. (B) CoCl2 treatment increased VEGF-A mRNA expression. Each bar represents the mean value ± SD. *p < 0.05 CoCl2 vs. control; †p < 0.05 8 h vs. 2 h CoCl2 treatment; (n=4). (C) CoCl2 treatment increased PlGF mRNA expression. Each bar represents the mean value ± SD. *p < 0.05 CoCl2 vs. control; †p < 0.05 8 h vs. 2 h CoCl2 treatment; (n=4). The mRNA levels were evaluated by qRT-PCR.
Expression Analysis of miRNAs Induced by CoCl2 Treatmet of HRECs
Six miRNAs (miR-20a-5p, miR-20b-5p, miR-27a-3p, miR-27b-3p, miR-260b-3p, miR-381-3p), out of eight analyzed, were found to be significantly (p<0.05) dysregulated in HRECs, treated with 200 µM CoCl2, compared to control cells (Figure 2). All dysregulated miRNAs were found to be significantly (p<0.05) upregulated, 2 h after CoCl2 treatment, compared to control cells (Figure 3A). On the contrary, four miRNAs were significantly (p<0.05) dysregulated (upregulated) 8 h after CoCl2 treatment, compared to control cells (Figure 3B). Furthermore, after 8 h of exposure to CoCl2, five miRNAs (miR-20a, miR-20b, miR-27a, miR-27b, miR-206-3p) were significantly downregulated (p<0.05), compared to levels detected in cells treated for 2 h with 200 µM CoCl2, with exception of miR-381-3p (Figure 3C).
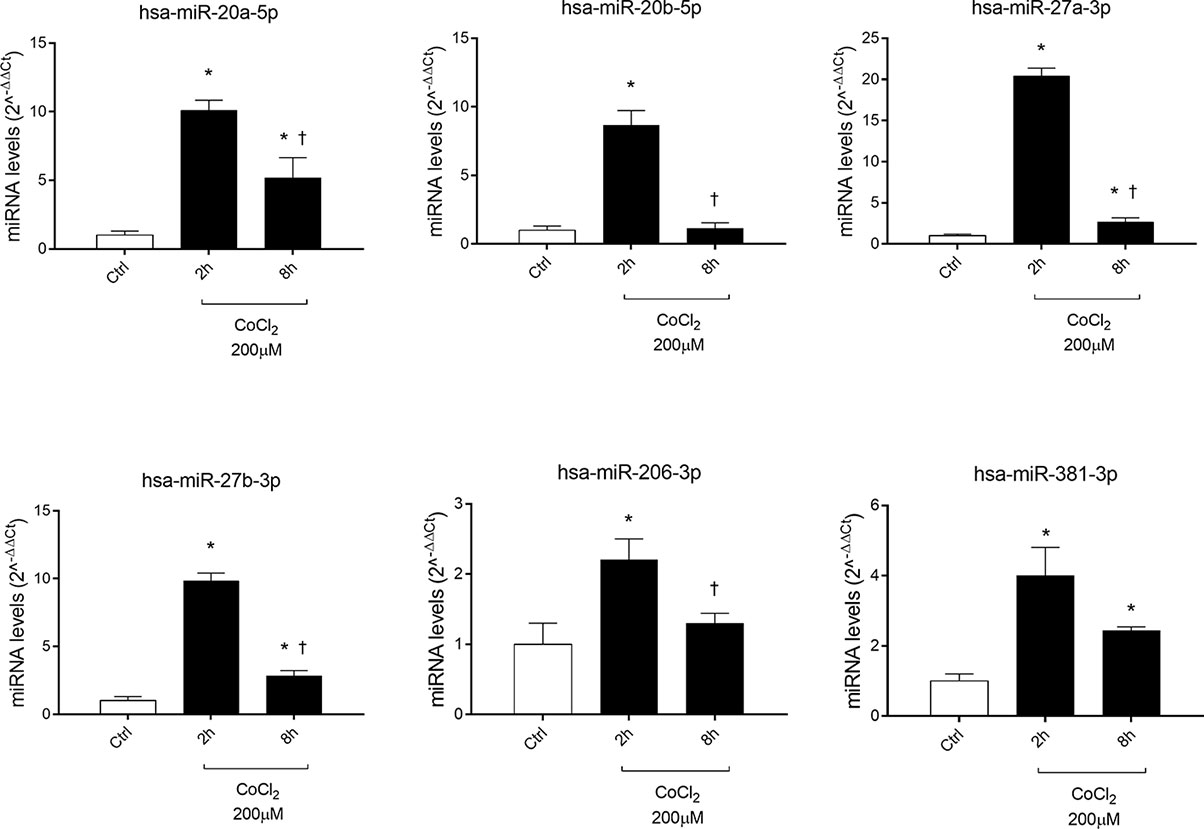
Figure 2 Pattern expression of microRNAs (miRNAs) in human retinal endothelial cells (HRECs) treated with CoCl2, for 2 and 8 h treatment. Expression of miRNAs was analyzed with qRT-PCR. Each bar represents the mean value ± SD. *p < 0.05 200 µM CoCl2 vs. control (ctrl); †p<0.05 8h vs. 2h CoCl2 treatment; (n=4).
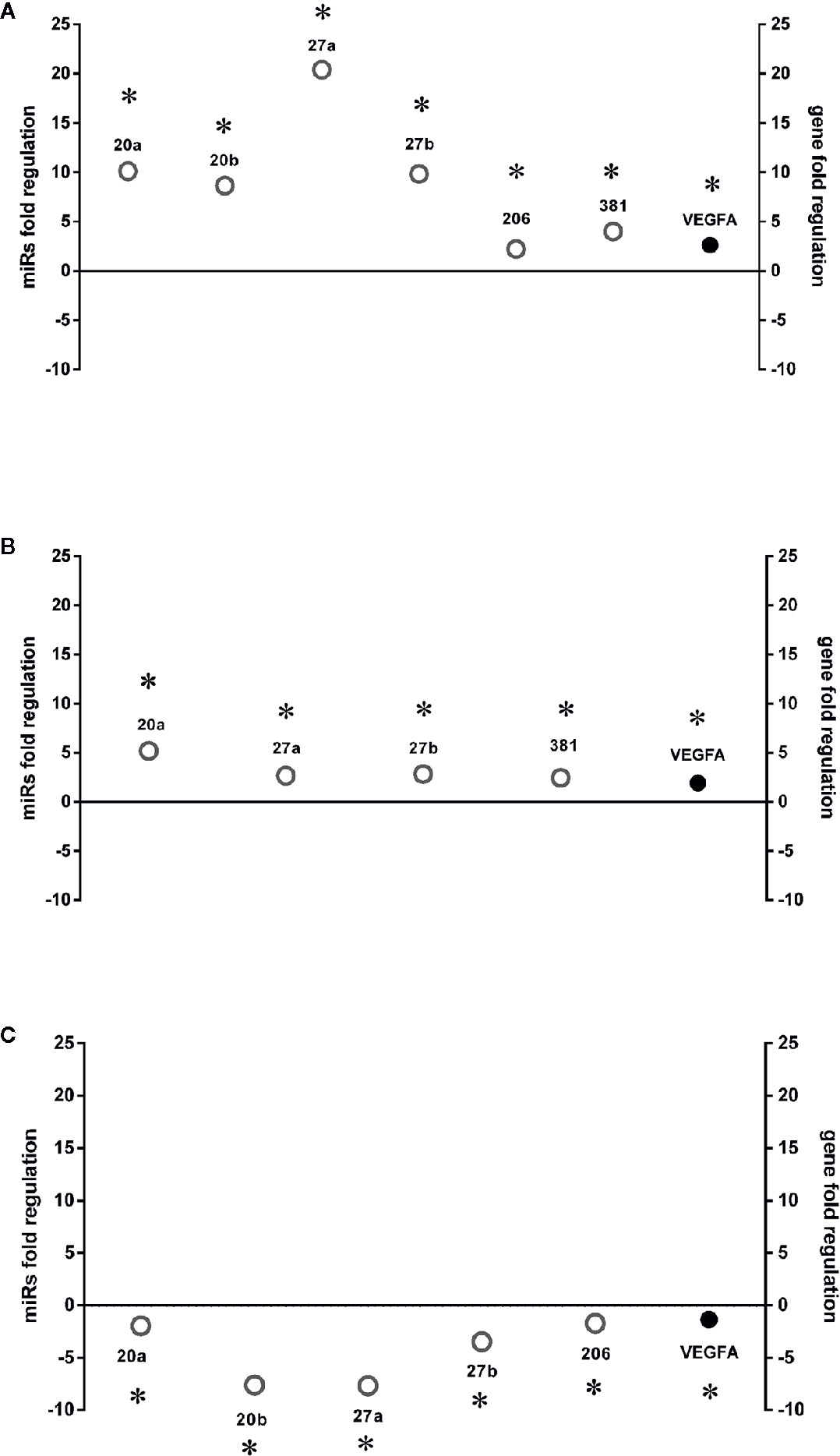
Figure 3 HIF-1α stabilization induced microRNAs (miRNAs) and correlation with vascular endothelial growth factor (VEGFA) expression. Correlation of microRNAs and VEGFA expression (Fold regulation). (A) *p < 0.05 2 h CoCl2 treatment vs. control; (B) *p < 0.05 8 h CoCl2 vs. control (ctrl); (C) *p < 0.05 8h vs. 2h CoCl2 treatment; (n=4).
TGFβ Signaling Pathway in HRECs Challenged With CoCl2
A bioinformatic approach was used to predict the combinatorial effect of miR-20a-5p, miR-20b-5p, miR-27a-3p, miR-27b-3p, miR-206-3p, and miR-381-3p on biological pathways. The pathways dysregulated by these miRNAs were predicted by means of DIANA miRPath, applying the Tarbase algorithm, which generates, as output, pathways related to experimental validated miRNA:mRNA interactions (Vlachos et al., 2015). Based on this bioinformatic approach, we found that the TGFβ signaling pathway was the top-scored among the pathways significantly (p<0.05) dysregulated by hypoxia-induced miRNA in HRECs (Figure 4). The HIF-1α pathway was predicted to be regulated by miR-20a-5p, miR-20b-5p, miR-27a-3p, miR-27b-3p, miR-260-3p, miR-381-3p, according to the in vitro model of retinal chemical hypoxia. Moreover, PI3K-AKT, MAP kinases and Jak-STAT signaling pathways were predicted to be modulated by the six miRNAs, that were dysregulated in HRECs treated with CoCl2.
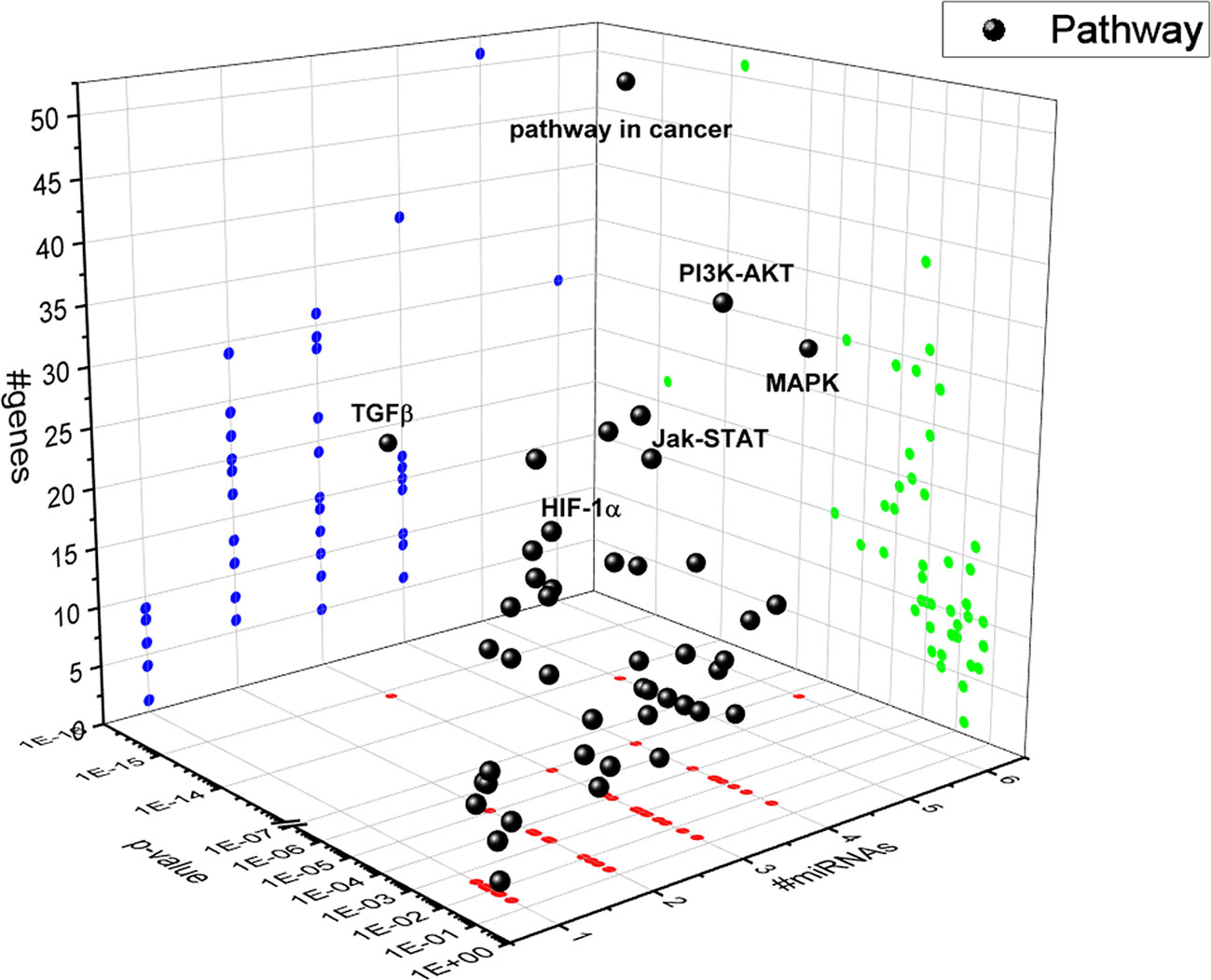
Figure 4 3D scatter plot of pathways regulated by HypoxamiRs in human retinal endothelial cells (HRECs), challenged with CoCl2. Blue dots represent #gene projection of #miRNAs dimension. Green dots represent p-value projection of #genes dimension. Red dots represent #miRNA projection of p-value dimension.
Therefore, we focused our study on analysis of transcription of TGFβ signaling pathway genes (TGFB1 encoding for TGFβ1, TGFBR1 encoding for the TGFβR1 receptor, TGFBR2 encoding for the TGFβR2 receptor and SMAD2 encoding for SMAD2), in HRECs treated with 200µM CoCl2 (Table 1). These genes were significantly (p<0.05) dysregulated in HRECs, 2 and 8 h after CoCl2 treatment (Figure 5). Furthermore, we correlated gene expression with dysregulated miRNAs in the analyzed time-points (Figure 6, Table 1). After 2 h of CoCl2 treatment, TGFBR2 and TGFB1 gene expression increased significantly, TGFBR1 decreased (p<0.05) (Figure 6A), and all analyzed miRNA were significantly upregulated, particularly miR-27a. The mRNA of TGFBR1 is an experimental validated target of miR-20a, miR-20b, miR-27a, miR-27b, and miR-381, therefore the upregulation of this miRNAs significantly decreased the TGFBR1 mRNA levels (Table 1). Moreover TGFB1, and TGFBR2 are experimental validated targets of miR-27a and miR-20a, respectively, which even if overexpressed did not reduce the expression of these two genes, 2 h after CoCl2 treatment (Figure 6A). Eight hours after CoCl2 treatment, miR-20a, miR-27a, miR-27b, and miR-381-3p were significantly (p<0.05) upregulated in HRECs, compared to control cells (Figure 6B). This pattern of miRNA expression positively correlated with TGFB1, TGFBR2, and SMAD2 expression (Figure 6B). Although not significantly, 8h after CoCl2 treatment, mRNA levels of TGFβ1, TGFβR2 and SMAD2 were higher, compared to HRECs treated for 2 h with CoCl2 (Table 1). On the other hand, TGFβR1 mRNA expression levels were significantly upregulated 8 h after CoCl2 treatment, compared to cells treated for 2 h with CoCl2 (Figure 6C). This expression pattern negatively correlated with downregulation of miR-20a, miR-20b, miR-27a, miR-27b, and miR-206, according to the opposite trend observed 2 h after chemical hypoxia.
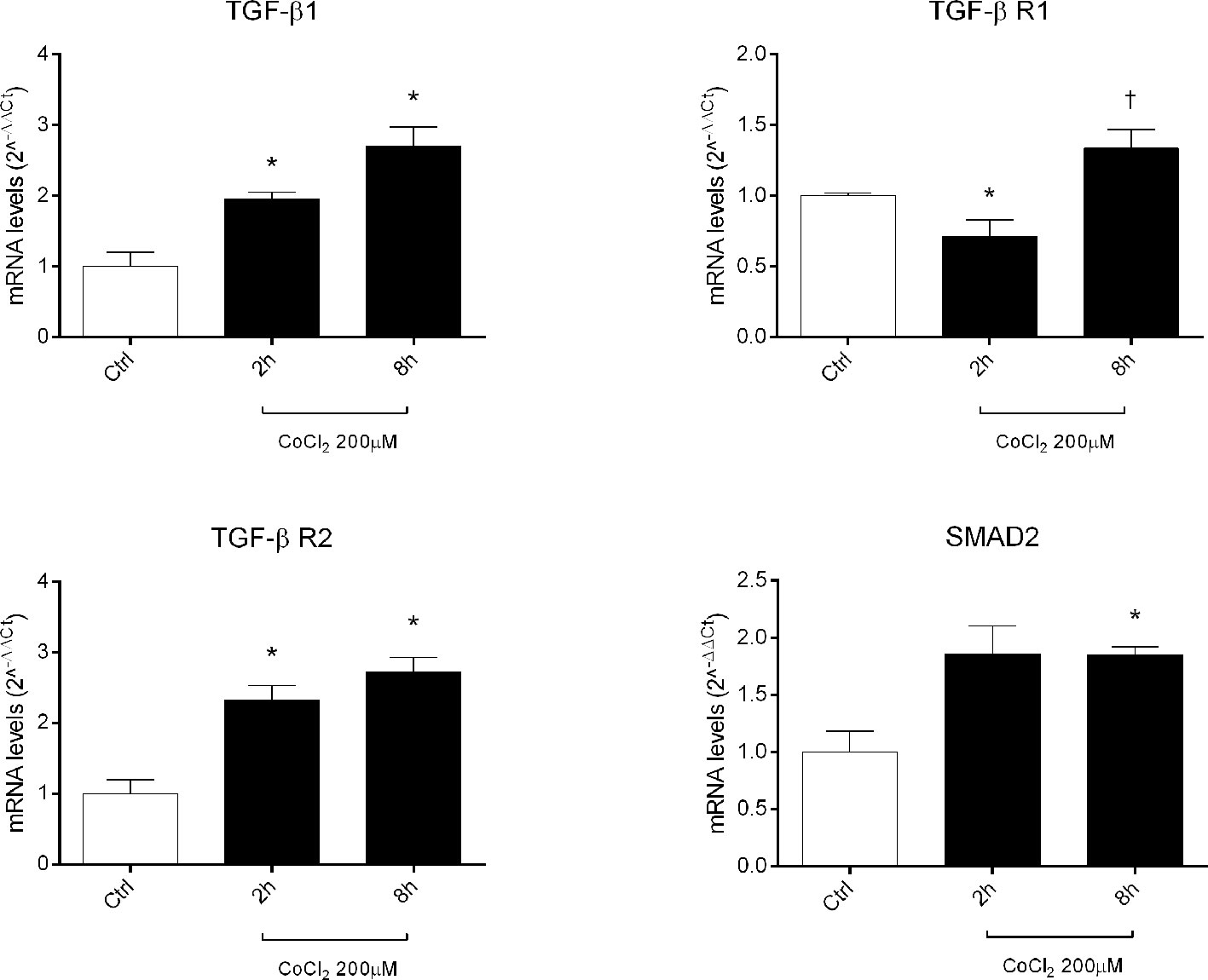
Figure 5 Expression of genes of TGFβ signaling pathway in human retinal endothelial cells (HRECs) treated with CoCl2, for 2 and 8 h. The mRNA levels were evaluated by qRT-PCR. Each bar represents the mean value ± SD. *p < 0.05 CoCl2 vs. control (ctrl); †p < 0.05 8h vs. 2h CoCl2 treatment. (n=4).
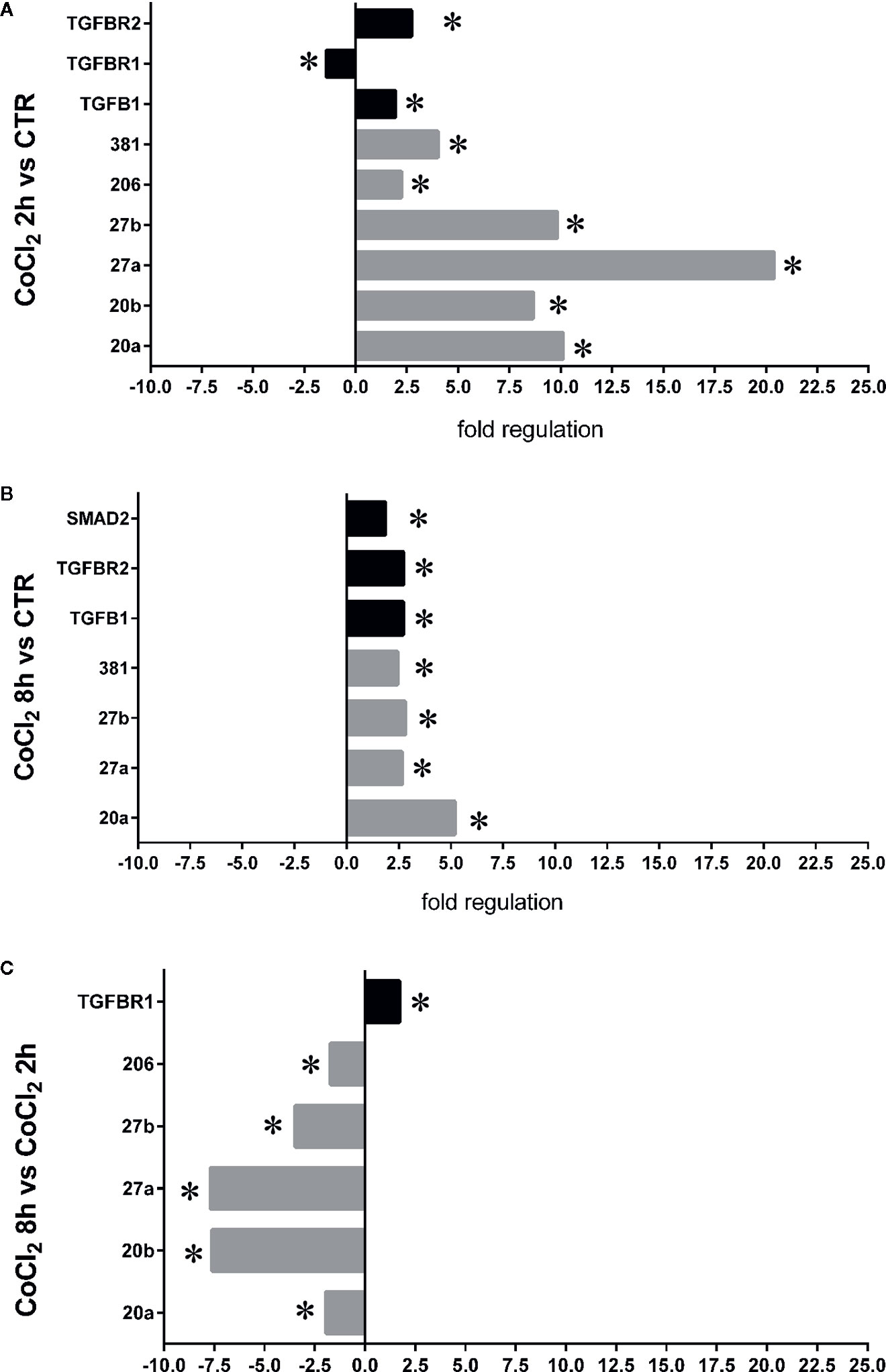
Figure 6 Correlation of microRNAs (miRNAs) and TGFβ signaling pathway genes expression in human retinal endothelial cells (HRECs), under chemical hypoxic stimuli. Fold regulation of miRNAs and TGFβ signaling genes: (A) *p < 0.05 2 h CoCl2 treatment vs. control (ctr); (B) *p < 0.05 8 h CoCl2 treatment vs. control (ctr); (C) *p < 0.05 8 h vs. 2 h CoCl2 treatment; (n=4).
Discussion
Previous data report that eight miRNAs (miR-20a-5p, miR-20a3p, miR-20b-5p, miR-106a-5p, miR-27a-5p, miR-27b-3p, miR-206-3p, and miR-381-3p) were significantly dysregulated both in serum and retina of 5–10 months diabetic mice (Platania et al., 2019). Because retinal hypoxia is detrimental in DR, exacerbating retinal damage and angiogenesis (Aiello et al., 1994; Arjamaa and Nikinmaa, 2006; Abu El-Asrar et al., 2012), we aimed at testing the hypothesis that these miRNAs would be modulated in human retinal endothelial cells, treated with CoCl2 in order to stabilize HIF-1α.
In DR, the role of angiogenesis linked to hypoxic events (i.e. increased VEGFA production stimulated by HIF-1α) has been largely proven (Arjamaa and Nikinmaa, 2006; B. Arden and Sivaprasad, 2012; Kurihara et al., 2014; Li et al., 2017). Furthermore, HIF-1α can induce expression of another proangiogenic factor, the PlGF (Tudisco et al., 2014; Lazzara et al., 2019). In this study we found a correlation, in terms of time-dependent expression, between HIF-1α and PlGF, after CoCl2 treatment (Figure 1). Instead, VEGF mRNA levels did not correlate with HIF-1α protein (Figure 1). For this reason, we hypothesized that other factors could regulate VEGFA expression in an in vitro model of chemical hypoxia, such as miRNAs. Involvement of miRNAs in retinal neovascular diseases has been widely studied (Romano et al., 2017; Natoli and Fernando, 2018; Martinez and Peplow, 2019; Platania et al., 2019). We found that six miRNAs (miR-20a-5p, miR-20b-5p, miR-27a-3p, miR-27b-3p, miR-206-3p, miR-381-3p), out of eight tested, were dysregulated in human retinal endothelial cells after CoCl2 treatment (Figure 2). These miRNAs have been previously found to be either HypoxamiRs (bearing HREs in their promoting region) or linked to hypoxic microenvironment (Nallamshetty et al., 2013; Yue et al., 2013; Choudhry and Mole, 2016; Gupta et al., 2018; Lu et al., 2018).
After 8 h, similarly to VEGFA expression, we found a shift in expression pattern of miRNAs, compared levels detected 2 h after CoCl2 treatment (Figure 3). Experimental validated miRNA : VEGFA mRNA interactions were found for miR-20a-5p and miR-20b-5p (Platania et al., 2019), and in hepatocellular carcinoma for miR-381-3p (Tsai et al., 2017; Wang et al., 2018). Therefore, VEGFA expression levels could be related to the expression pattern of miRNAs, 2 to 8 h after stabilization of HIF-1α, because VEGFA is a target of miR-20a, miR-20b, miR-381, and indirectly of miR-27b (Veliceasa et al., 2015). On the contrary, PlGF is not a validated or predicted target of any miRNAs dysregulated in HRECs treated with CoCl2. Particularly, the role of PlGF in regulation retinal angiogenesis, under hypoxic stimuli, is still unknown. On the other hand, several reports support the detrimental role of PlGF in the pathogenesis and progression of DR (Carmeliet et al., 2001; Huang et al., 2015), likely through HIF-1α, or indirectly by miRNAs and the PI3K/AKT signaling pathways (Figure 4) (Zhou et al., 2016; Jin et al., 2018).
Therefore, our hypothesis is based on retinal angiogenesis regulated by miRNAs under hypoxic stimuli, and miRNAs can be considered alternative and/or ancillary components to VEGFA and PlGF pathways. Indeed, we analyzed other putative miRNAs targets (gene and pathways) and identified, through a bioinformatic approach, the TGFβ signaling pathway as the top-scored pathway dysregulated by identified miRNAs (Figure 4). Then, we found that miRNAs, dysregulated after CoCl2 treatment, (miR-20a-5p, miR-20b-5p, miR-27a-3p, miR-27b-3p, miR-206-3p, miR-381-3p) influenced mRNA levels of TGFβ1, TGFβR1, TGFβR2 and SMAD2, according to experimental validated miRNA:mRNA interactions (Figures 5 and 6, Table 1). TGFβ1, TGFβR2 and SMAD2, were upregulated 2 and 8 h after HIF-1α stabilization. Interestingly, the expression of TGFβR1 receptor, which is target of most of analyzed miRNAs (Table 1), correlated with expression pattern shift of miRNAs at 2 h and 8 h after CoCl2 treatment. Several reports support a detrimental role of TGFβR1 in DR, particularly, TGFβR1 immunoreactivity was found to be increased in retinal capillaries of diabetic rats (Gerhardinger et al., 2009; van Geest et al., 2010).
The HIF-1/TGF-β1 axis, and related stimulation of angiogenesis, has been investigated in different experimental settings (Han et al., 2013; Mingyuan et al., 2018), including endothelial cells (Iruela-Arispe and Sage, 1993; Peshavariya et al., 2014). On the contrary, few reports demonstrated a putative link between HIF-1α/miRNAs/TGFβ signaling pathway and angiogenesis (Xing et al., 2014). Furthermore, only one study analyzed the role miRNAs in regulation of hypoxia-TGFβ-angiogenesis pathway in a model of corneal neovascularization (Zhang Y. et al., 2019). According to our findings, miR-27 was reported to be involved in regulation of HIF-1/TGFβ axis, at least in an in vitro model of cardiac ischemia (Zhang X. L. et al., 2019). However, there are still no evidences about a putative link in retinal disease between hypoxia, miRNAs, VEGFA, and TGFβ pathway.
High throughput miRNA expression analysis on retinal endothelial cells, challenged with chemical hypoxic stimuli, could reveal the involvement of other miRNAs, along with the focused set analyzed in this study. However, those high throughput analyses are expensive and need quantitative qPCR validation (de Ronde et al., 2018). Despite the small set of analyzed miRNAs, our study suggested that ocular neovascularization, during hypoxia, would be promoted by the upregulation of PlGF and other factors induced by HIF-1α/miRNAs, i.e. VEGFA, and genes of the TGFβ1 signaling pathway (Figure 7). Therefore, these data warranting further in vivo studies to explore the use of pharmacological/molecular approach such as antagomiRs and agomir.
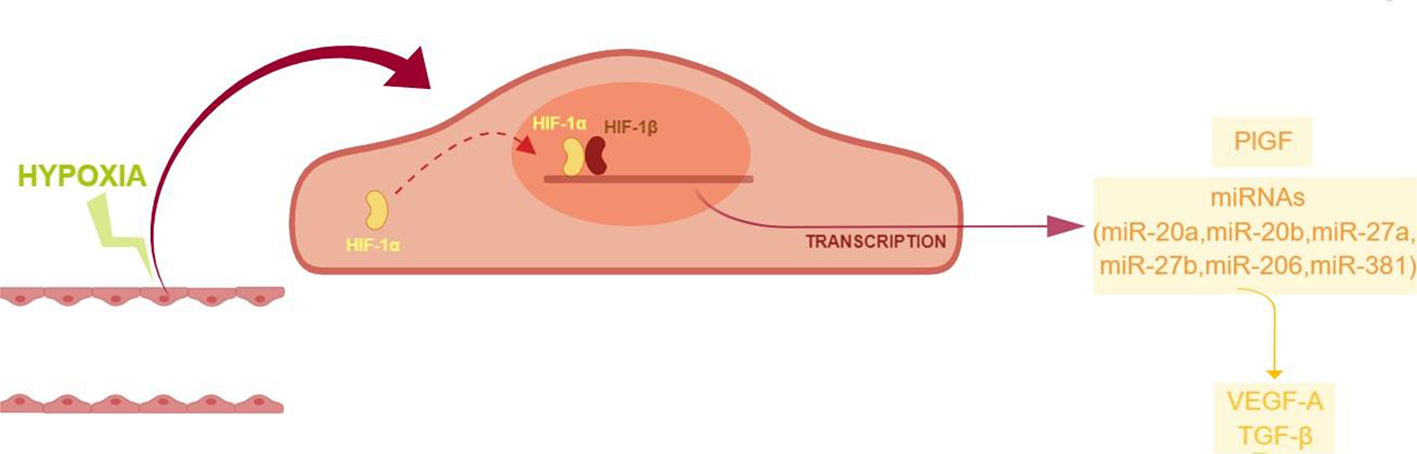
Figure 7 Proposed model of angiogenic shift in retinal endothelial cells exposed to chemical hypoxia. CoCl2-induced hypoxia leads to the stabilization of HIF-1α, with the subsequent translocation into the nucleus and transcription of hypoxia-related genes.
Indeed, the present findings highlighted that proangiogenic factors are worthy to be further explored as potential targets for pharmacological modulation of local retinal hypoxic events, which are generally transient but detrimental in retinal degenerations.
Data Availability Statement
The datasets generated for this study are available on request to the corresponding author.
Author Contributions
CB, MD’M, and SR made substantial contributions to conception, design, and interpretation of data. FL, MT, and CP carried out experiments. FL, MT, CP, FP, and CG carried out formal analysis of data. FL, MT, CP, and CB wrote initial draft of the manuscript. CB, MD’M, SR, FD, and MG reviewed the manuscript critically for important intellectual content and gave final approval of the version to be submitted.
Conflict of Interest
The authors declare that the research was conducted in the absence of any commercial or financial relationships that could be construed as a potential conflict of interest.
Funding
This work was supported by National Grant PRIN 2015JXE7E8 from the Italian Ministry of Education, University and Research (MIUR) and the Italian Ministry of Economic Development (MISE) PON-Innovative PhD Program. Grant from University of Catania, Piano Triennale Ricerca Linea Intervento 2. Fondi di Ateneo 2020-2022, Università di Catania, linea intervento 4 Open Access.
Supplementary Material
The Supplementary Material for this article can be found online at: https://www.frontiersin.org/articles/10.3389/fphar.2020.01063/full#supplementary-material
References
Abhinand, C. S., Raju, R., Soumya, S. J., Arya, P. S., Sudhakaran, P. R. (2016). VEGF-A/VEGFR2 signaling network in endothelial cells relevant to angiogenesis. J. Cell Commun. Signal. 10, 347–354. doi: 10.1007/s12079-016-0352-8
Abu El-Asrar, A. M., Nawaz, M., II, Kangave, D., Abouammoh, M., Mohammad, G. (2012). High-mobility group box-1 and endothelial cell angiogenic markers in the vitreous from patients with proliferative diabetic retinopathy. Mediators Inflamm. 2012, 697489. doi: 10.1155/2012/697489
Aiello, L. P., Arrigg, P. G., Shah, S. T., Keyt, B. A., Avery, R. L., Jampel, H. D., et al. (1994). Vascular Endothelial Growth Factor in Ocular Fluid of Patients with Diabetic Retinopathy and Other Retinal Disorders. New Engl. J. Med. 331, 1480–1487. doi: 10.1056/NEJM199412013312203
Ao, Q., Su, W., Guo, S., Cai, L., Huang, L. (2015). SENP1 desensitizes hypoxic ovarian cancer cells to cisplatin by up-regulating HIF-1α. Sci. Rep. 5, 16396. doi: 10.1038/srep16396
Arden, G. B., Sivaprasad, S. (2012). Hypoxia and Oxidative Stress in the Causation of Diabetic Retinopathy. Curr. Diabetes Rev. 7, 291–304. doi: 10.2174/157339911797415620
Arjamaa, O., Nikinmaa, M. (2006). Oxygen-dependent diseases in the retina: Role of hypoxia-inducible factors. Exp. Eye Res. 83, 473–483. doi: 10.1016/j.exer.2006.01.016
Balaiya, S., Ferguson, L. R., Chalam, K. V. (2012). Evaluation of sirtuin role in neuroprotection of retinal ganglion cells in hypoxia. Invest. Ophthalmol. Visual Sci. 53 (7), 4315–4322. doi: 10.1167/iovs.11-9259
Bandello, F., Berchicci, L., La Spina, C., Battaglia Parodi, M., Iacono, P. (2012). Evidence for anti-VEGF treatment of diabetic macular edema. Ophthal. Res. 48, 16–20. doi: 10.1159/000339843
Bertero, T., Rezzonico, R., Pottier, N., Mari, B. (2017). Impact of MicroRNAs in the Cellular Response to Hypoxia. Int. Rev. Cell Mol. Biol. 333, 91–158. doi: 10.1016/bs.ircmb.2017.03.006
Botlagunta, M., Krishnamachary, B., Vesuna, F., Winnard, P. T., Bol, G. M., Patel, A. H., et al. (2011). Expression of DDX3 is directly modulated by hypoxia inducible factor-1 alpha in breast epithelial cells. PLoS One 6 (3), e17563. doi: 10.1371/journal.pone.0017563
Bucolo, C., Drago, F., Lin, L. R., Reddy, V. N. (2006). Sigma receptor ligands protect human retinal cells against oxidative stress. NeuroReport 17 (3), 287–291. doi: 10.1097/01.wnr.0000199469.21734.e1
Carmeliet, P., Moons, L., Luttun, A., Vincenti, V., Compernolle, V., De Mol, M., et al. (2001). Synergism between vascular endothelial growth factor and placental growth factor contributes to angiogenesis and plasma extravasation in pathological conditions. Nat. Med. 7, 575–583. doi: 10.1038/87904
Charnock-Jones, D. S. (2016). Placental hypoxia, endoplasmic reticulum stress and maternal endothelial sensitisation by sFLT1 in pre-eclampsia. J. Reprod. Immunol. 114, 81–85. doi: 10.1016/j.jri.2015.07.004
Choudhry, H., Mole, D. R. (2016). Hypoxic regulation of the noncoding genome and NEAT1. Briefings Funct. Genomics 15, 174–185. doi: 10.1093/bfgp/elv050
Cottrill, K. A., Chan, S. Y., Loscalzo, J. (2014). Hypoxamirs and mitochondrial metabolism. Antioxid. Redox Signal. 21, 1189–1201. doi: 10.1089/ars.2013.5641
D’Amico, A. G., Maugeri, G., Reitano, R., Bucolo, C., Saccone, S., Drago, F., et al. (2015). PACAP Modulates Expression of Hypoxia-Inducible Factors in Streptozotocin-Induced Diabetic Rat Retina. J. Mol. Neurosci. 57 (4), 501–509. doi: 10.1007/s12031-015-0621-7
D’Amico, A. G., Maugeri, G., Bucolo, C., Saccone, S., Federico, C., Cavallaro, S., et al. (2017). Nap Interferes with Hypoxia-Inducible Factors and VEGF Expression in Retina of Diabetic Rats. J. Mol. Neurosci. 61 (2), 256–266. doi: 10.1007/s12031-016-0869-6
de Ronde, M. W. J., Ruijter, J. M., Moerland, P. D., Creemers, E. E., Pinto-Sietsma, S.-J. (2018). Study Design and qPCR Data Analysis Guidelines for Reliable Circulating miRNA Biomarker Experiments: A Review. Clin. Chem. 64 (9), 1308–1318. doi: 10.1373/clinchem.2017.285288
Evrard, S. M., Lecce, L., Michelis, K. C., Nomura-Kitabayashi, A., Pandey, G., Purushothaman, K. R., et al. (2016). Endothelial to mesenchymal transition is common in atherosclerotic lesions and is associated with plaque instability. Nat. Commun. 7, 11853. doi: 10.1038/ncomms11853
Gao, R., Zhu, B. H., Tang, S. B., Wang, J. F., Ren, J. (2008). Scutellarein inhibits hypoxia- and moderately-high glucose-induced proliferation and VEGF expression in human retinal endothelial cells. Acta Pharmacol. Sin. 29 (6), 707–712. doi: 10.1111/j.1745-7254.2008.00797.x
Gao, L., Dou, Z. C., Ren, W. H., Li, S. M., Liang, X., Zhi, K. Q. (2019). CircCDR1as upregulates autophagy under hypoxia to promote tumor cell survival via AKT/ERK½/mTOR signaling pathways in oral squamous cell carcinomas. Cell Death Dis. 10 (10), 745. doi: 10.1038/s41419-019-1971-9
Gee, H. E., Ivan, C., Calin, G. A., Ivan, M. (2014). HypoxamiRs and cancer: From biology to targeted therapy. Antioxid. Redox Signal. 21, 1220–1238. doi: 10.1089/ars.2013.5639
Gerhardinger, C., Dagher, Z., Sebastiani, P., Yong, S. P., Lorenzi, M. (2009). The transforming growth factor-β pathway is a common target of drugs that prevent experimental diabetic retinopathy. Diabetes. doi: 10.2337/db08-1008
Greco, S., Gaetano, C., Martelli, F. (2014). HypoxamiR regulation and function in ischemic cardiovascular diseases. Antioxid. Redox Signal. 21, 1202–1219. doi: 10.1089/ars.2013.5403
Gupta, A., Sugadev, R., Sharma, Y. K., Ahmad, Y., Khurana, P. (2018). Role of miRNAs in hypoxia-related disorders. J. Biosci. 43, 739–749. doi: 10.1007/s12038-018-9789-7
Han, W. Q., Zhu, Q., Hu, J., Li, P. L., Zhang, F., Li, N. (2013). Hypoxia-inducible factor prolyl-hydroxylase-2 mediates transforming growth factor beta 1-induced epithelial-mesenchymal transition in renal tubular cells. Biochim. Biophys. Acta - Mol. Cell Res. 1833, 1454–1462. doi: 10.1016/j.bbamcr.2013.02.029
He, C., Shan, N., Xu, P., Ge, H., Yuan, Y., Liu, Y., et al. (2019). Hypoxia-induced Downregulation of SRC-3 Suppresses Trophoblastic Invasion and Migration Through Inhibition of the AKT/mTOR Pathway: Implications for the Pathogenesis of Preeclampsia. Sci. Rep. 9, 10349. doi: 10.1038/s41598-019-46699-3
Hu, J., Song, X., He, Y. Q., Freeman, C., Parish, C. R., Yuan, L., et al. (2012). Heparanase and vascular endothelial growth factor expression is increased in hypoxia-induced retinal neovascularization. Invest. Ophthalmol. Visual Sci. 53, 6810–6817. doi: 10.1167/iovs.11-9144
Huang, H., He, J., Johnson, D., Wei, Y., Liu, Y., Wang, S., et al. (2015). Deletion of placental growth factor prevents diabetic retinopathy and is associated with akt activation and HIF1α-VEGF pathway inhibition. Diabetes 64, 200–212. doi: 10.2337/db14-0016
Iruela-Arispe, M. L., Sage, E. H. (1993). Endothelial cells exhibiting angiogenesis in vitro proliferate in response to TGF-β1. J. Cell. Biochem. 52, 414–430. doi: 10.1002/jcb.240520406
Iwase, T., Fu, J., Yoshida, T., Muramatsu, D., Miki, A., Hashida, N., et al. (2013). Sustained delivery of a HIF-1 antagonist for ocular neovascularization. J. Controlled Release 172 (3), 625–633. doi: 10.1016/j.jconrel.2013.10.008
Jiang, S., Xu, Y. (2019). Annexin A2 upregulation protects human retinal endothelial cells from oxygen−glucose deprivation injury by activating autophagy. Exp. Ther. Med. 18 (4), 2901–2908. doi: 10.3892/etm.2019.7909
Jin, Y.-P., Hu, Y.-P., Wu, X.-S., Wu, Y.-S., Ye, Y.-Y., Li, H.-F., et al. (2018). miR-143-3p targeting of ITGA6 suppresses tumour growth and angiogenesis by downregulating PLGF expression via the PI3K/AKT pathway in gallbladder carcinoma. Cell Death Dis. 9, 182. doi: 10.1038/s41419-017-0258-2
Kurihara, T., Westenskow, P. D., Friedlander, M. (2014). Hypoxia-inducible factor (HIF)/vascular endothelial growth factor (VEGF) signaling in the retina. Adv. Exp. Med. Biol. 801, 275–281. doi: 10.1007/978-1-4614-3209-8_35
Kwon, J. W., Jee, D. (2018). Aqueous humor cytokine levels in patients with diabetic macular edema refractory to anti-VEGF treatment. PLoS One 13 (9), e0203408. doi: 10.1371/journal.pone.0203408
Lazzara, F., Fidilio, A., Platania, C. B. M., Giurdanella, G., Salomone, S., Leggio, G. M., et al. (2019). Aflibercept regulates retinal inflammation elicited by high glucose via the PlGF/ERK pathway. Biochem. Pharmacol. 168, 341–351. doi: 10.1016/j.bcp.2019.07.021
Lee, Y. J., Ke, C. Y., Tien, N., Lin, P. K. (2018). Hyperhomocysteinemia Causes Chorioretinal Angiogenesis with Placental Growth Factor Upregulation. Sci. Rep. 8, 15755. doi: 10.1038/s41598-018-34187-z
Li, T., Hu, J., Gao, F., Du, X., Chen, Y., Wu, Q. (2017). Transcription factors regulate GPR91-mediated expression of VEGF in hypoxia-induced retinopathy. Sci. Rep. 7, 45807. doi: 10.1038/srep45807
Li, Q., Pang, L., Yang, W., Liu, X., Su, G., Dong, Y. (2018). Long non-coding RNA of myocardial infarction associated transcript (LncRNA-MIAT) promotes diabetic retinopathy by upregulating transforming growth factor-β1 (TGF-β1) signaling. Med. Sci. Monitor. 24, 9497–9503. doi: 10.12659/MSM.911787
Lin, K., Ye, P., Liu, J., He, F., Xu, W. (2015). Endostar inhibits hypoxia-induced cell proliferation and migration via the hypoxia-inducible factor-1alpha/vascular endothelial growth factor pathway in vitro. Mol. Med. Rep. 11, 3780–3785. doi: 10.3892/mmr.2014.3131
Livak, K. J., Schmittgen, T. D. (2001). Analysis of relative gene expression data using real-time quantitative PCR and the 2-ΔΔCT method. Methods 25 (4), 402–428. doi: 10.1006/meth.2001.1262
Lu, L., Zhang, H., Dong, W., Peng, W., Yang, J. (2018). MiR-381 negatively regulates cardiomyocyte survival by suppressing Notch signaling. In Vitro Cell. Dev. Biol. - Anim. 54 (8), 610–619. doi: 10.1007/s11626-018-0277-z
Martinez, B., Peplow, P. (2019). MicroRNAs as biomarkers of diabetic retinopathy and disease progression. Neural Regen. Res. 14 (11), 1858–1869. doi: 10.4103/1673-5374.259602
Mingyuan, X., Qianqian, P., Shengquan, X., Chenyi, Y., Rui, L., Yichen, S., et al. (2018). Hypoxia-inducible factor-1α activates transforming growth factor-β1/Smad signaling and increases collagen deposition in dermal fibroblasts. Oncotarget 9, 3188–3197. doi: 10.18632/oncotarget.23225
Mitsui, T., Tani, K., Maki, J., Eguchi, T., Tamada, S., Eto, E., et al. (2018). Upregulation of angiogenic factors via protein kinase c and hypoxia-induced factor-1a pathways under high-glucose conditions in the placenta. Acta Med. Okayama 72, 359–367. doi: 10.18926/AMO/56171
Nallamshetty, S., Chan, S. Y., Loscalzo, J. (2013). Hypoxia: A master regulator of microRNA biogenesis and activity. Free Radical Biol. Med. 64, 20–30. doi: 10.1016/j.freeradbiomed.2013.05.022
Natoli, R., Fernando, N. (2018). MicroRNA as therapeutics for age-related macular degeneration. Adv. Exp. Med. Biol. 1074, 37–43. doi: 10.1007/978-3-319-75402-4_5
Ozaki, H., Yu, A. Y., Della, N., Ozaki, K., Luna, J. D., Yamada, H., et al. (1999). Hypoxia inducible factor-1alpha is increased in ischemic retina: temporal and spatial correlation with VEGF expression. Invest. Ophthalmol. Visual Sci. 40, 182–189.
Peshavariya, H. M., Chan, E. C., Liu, G. S., Jiang, F., Dusting, G. J. (2014). Transforming growth factor-β1 requires NADPH oxidase 4 for angiogenesis in vitro and in vivo. J. Cell. Mol. Med. 18, 1172–1183. doi: 10.1111/jcmm.12263
Platania, C. B. M., Maisto, R., Trotta, M. C., D’Amico, M., Rossi, S., Gesualdo, C., et al. (2019). Retinal and circulating miRNA expression patterns in diabetic retinopathy: An in silico and in vivo approach. Br. J. Pharmacol. 176, 2179–2194. doi: 10.1111/bph.14665
Riffo-Campos, A. L., Riquelme, I., Brebi-Mieville, P., Riffo-Campos, Á. L., Riquelme, I., Brebi-Mieville, P. (2016). Tools for Sequence-Based miRNA Target Prediction: What to Choose? Int. J. Mol. Sci. 17 (12), 1987. doi: 10.3390/ijms17121987
Rodrigues, M., Kashiwabuchi, F., Deshpande, M., Jee, K., Goldberg, M. F., Lutty, G., et al. (2016). Expression pattern of HIF-1α and VEGF supports circumferential application of scatter laser for proliferative sickle retinopathy. Invest. Ophthalmol. Visual Sci. 57 (15), 6739–6746. doi: 10.1167/iovs.16-19513
Romano, G. L. G. L., Platania, C. B. M. C. B., Drago, F., Salomone, S., Ragusa, M., Barbagallo, C., et al. (2017). Retinal and Circulating miRNAs in Age-Related Macular Degeneration: An In vivo Animal and Human Study. Front. Pharmacol. 8, 168. doi: 10.3389/fphar.2017.00168
Rosen, R., Vagaggini, T., Chen, Y., Hu, D. N. (2015). Zeaxanthin 7inhibits hypoxia-induced VEGF secretion by RPE cells through decreased protein levels of hypoxia-inducible factors-1 α. BioMed. Res. Int. 2015, 687386. doi: 10.1155/2015/687386
Saddala, M. S., Lennikov, A., Grab, D. J., Liu, G. S., Tang, S., Huang, H. (2018). Proteomics reveals ablation of PlGF increases antioxidant and neuroprotective proteins in the diabetic mouse retina. Sci. Rep. 8, 16728. doi: 10.1038/s41598-018-34955-x
Schmetterer, L., Wolzt, M. (1999). Ocular blood flow and associated functional deviations in diabetic retinopathy. Diabetologia 42, 387–405. doi: 10.1007/s001250051171
Schmidl, D., Schmetterer, L., Garhöfer, G., Popa-Cherecheanu, A. (2015). Gender differences in ocular blood flow. Curr. Eye Res. 40, 201–212. doi: 10.3109/02713683.2014.906625
Shao, J., Yao, Y. (2016). Transthyretin represses neovascularization in diabetic retinopathy. Mol. Vision 22, 1188–1197.
Stafiej, J., Kazmierczak, K., Linkowska, K., Zuchowski, P., Grzybowski, T., Malukiewicz, G. (2018). Evaluation of TGF-Beta 2 and VEGFalpha Gene Expression Levels in Epiretinal Membranes and Internal Limiting Membranes in the Course of Retinal Detachments, Proliferative Diabetic Retinopathy, Macular Holes, and Idiopathic Epiretinal Membranes. J. Ophthalmol. 2018, 8293452. doi: 10.1155/2018/8293452
Tsai, H. C., Tzeng, H. E., Huang, C. Y., Huang, Y. L., Tsai, C. H., Wang, S. W., et al. (2017). WISP-1 positively regulates angiogenesis by controlling VEGF-A expression in human osteosarcoma. Cell Death Dis. 13, e2750. doi: 10.1038/cddis.2016.421
Tudisco, L., Ragione, F. D., Tarallo, V., Apicella, I., D’Esposito, M., Matarazzo, M. R., et al. (2014). Epigenetic control of hypoxia inducible factor-1α-dependent expression of placental growth factor in hypoxic conditions. Epigenetics 9, 600–610. doi: 10.4161/epi.27835
Van Bergen, T., Etienne, I., Cunningham, F., Moons, L., Schlingemann, R. O., Feyen, J. H. M., et al. (2019). The role of placental growth factor (PlGF) and its receptor system in retinal vascular diseases. Prog. Retin. Eye Res. 69, 116–136. doi: 10.1016/j.preteyeres.2018.10.006
van Geest, R. J., Klaassen, I., Vogels, I. M. C., van Noorden, C. J. F., Schlingemann, R. O. (2010). Differential TGF-β signaling in retinal vascular cells: A role in diabetic retinopathy? Invest. Ophthalmol. Visual Sci. 51 (4), 1857–1865. doi: 10.1167/iovs.09-4181
Veliceasa, D., Biyashev, D., Qin, G., Misener, S., Mackie, A. R., Kishore, R., et al. (2015). Therapeutic manipulation of angiogenesis with miR-27b. Vasc. Cell. 7, 6. doi: 10.1186/s13221-015-0031-1
Vlachos, I. S., Zagganas, K., Paraskevopoulou, M. D., Georgakilas, G., Karagkouni, D., Vergoulis, T., et al. (2015). DIANA-miRPath v3.0: deciphering microRNA function with experimental support. Nucleic Acids Res. 43, W460–W466. doi: 10.1093/nar/gkv403
Wang, J., Wu, S., Huang, T. (2018). Expression and role of VEGFA and miR-381 in portal vein tumor thrombi in patients with hepatocellular carcinoma. Exp. Ther. Med. 15, 5450–5456. doi: 10.3892/etm.2018.6129
Xing, Y., Hou, J., Guo, T., Zheng, S., Zhou, C., Huang, H., et al. (2014). MicroRNA-378 promotes mesenchymal stem cell survival and vascularization under hypoxic-Ischemic conditions in vitro. Stem Cell Res. Ther. 5 (6), 130. doi: 10.1186/scrt520
Yue, J., Guan, J., Wang, X., Zhang, L., Yang, Z., Ao, Q., et al. (2013). MicroRNA-206 is involved in hypoxia-induced pulmonary hypertension through targeting of the HIF-1α/Fhl-1 pathway. Lab. Investig. 93 (7), 748–759. doi: 10.1038/labinvest.2013.63
Zeng, M., Shen, J., Liu, Y., Lu, L. Y., Ding, K., Fortmann, S. D., et al. (2017). The HIF-1 antagonist acriflavine: visualization in retina and suppression of ocular neovascularization. J. Mol. Med. 95 (4), 417–429. doi: 10.1007/s00109-016-1498-9
Zhang, X. L., An, B. F., Zhang, G. C. (2019). MiR-27 alleviates myocardial cell damage induced by hypoxia/reoxygenation via targeting TGFBR1 and inhibiting NF-κB pathway. Kaohsiung J. Med. Sci. 35 (10), 607–614. doi: 10.1002/kjm2.12092
Zhang, Y., Yuan, F., Liu, L., Chen, Z., Ma, X., Lin, Z., et al. (2019). The Role of the miR-21/SPRY2 Axis in Modulating Proangiogenic Factors, Epithelial Phenotypes, and Wound Healing in Corneal Epithelial Cells. Invest. Ophthalmol. Visual Sci. 60, 3854–3862. doi: 10.1167/iovs.19-27013
Zhou, Y., Tu, C., Zhao, Y., Liu, H., Zhang, S. (2016). Placental growth factor enhances angiogenesis in human intestinal microvascular endothelial cells via PI3K/Akt pathway: Potential implications of inflammation bowel disease. Biochem. Biophys. Res. Commun. 470, 967–974. doi: 10.1016/j.bbrc.2016.01.073
Keywords: hypoxia-inducible-factor-1α, vascular endothelial growth factor, transforming growth factor beta, retina, diabetic retinopathy, inflammation
Citation: Lazzara F, Trotta MC, Platania CBM, D’Amico M, Petrillo F, Galdiero M, Gesualdo C, Rossi S, Drago F and Bucolo C (2020) Stabilization of HIF-1α in Human Retinal Endothelial Cells Modulates Expression of miRNAs and Proangiogenic Growth Factors. Front. Pharmacol. 11:1063. doi: 10.3389/fphar.2020.01063
Received: 16 March 2020; Accepted: 30 June 2020;
Published: 17 July 2020.
Edited by:
Annalisa Bruno, University of Studies G. d’Annunzio Chieti and Pescara, ItalyReviewed by:
Neeru M. Sharma, University of Nebraska Medical Center, United StatesMassimo Dal Monte, University of Pisa, Italy
Copyright © 2020 Lazzara, Trotta, Platania, D’Amico, Petrillo, Galdiero, Gesualdo, Rossi, Drago and Bucolo. This is an open-access article distributed under the terms of the Creative Commons Attribution License (CC BY). The use, distribution or reproduction in other forums is permitted, provided the original author(s) and the copyright owner(s) are credited and that the original publication in this journal is cited, in accordance with accepted academic practice. No use, distribution or reproduction is permitted which does not comply with these terms.
*Correspondence: Claudio Bucolo, Y2xhdWRpby5idWNvbG9AdW5pY3QuaXQ=
†These authors have contributed equally to this work