- 1Institute of Integrated Traditional Chinese and Western Medicine, Tongji Hospital, Tongji Medical College, Huazhong University of Science and Technology, Wuhan, China
- 2Department of Integrated Traditional Chinese and Western Medicine, Tongji Hospital, Tongji Medical College, Huazhong University of Science and Technology, Wuhan, China
- 3Department of Emergency, Tongji Hospital, Tongji Medical College, Huazhong University of Science and Technology, Wuhan, China
Objectives: Natural product, osthole, has been proven to have a protective effect on organ fibrosis, including renal fibrosis. All of these studies are mainly focused on the regulation of TGF-β/Smad signaling pathway. However, due to the pleiotropic roles of TGF-β/Smad signaling, direct TGF-β-targeted treatments are unlikely to be therapeutically feasible in clinic. Recently, the downstream IL-11/ERK1/2 signaling of TGF-β has become an attractive therapeutic target without upstream disadvantages. Based on that, this study was designed to identify the potential effects of osthole on IL-11/ERK1/2 signaling pathway in renal fibrosis.
Methods: The renal fibrosis model was established in vivo and in vitro, we investigated the effects of osthole on unilateral ureteral obstruction (UUO)-induced renal fibrosis and TGF-β-induced HK-2 cells. After preliminarily confirming the antifibrogenic effects of osthole and the link between its antifibrogenic effects and the inhibition of IL-11/ERK1/2 signaling, we applied a direct IL-11-induced HK-2 cells fibrosis model to further explore the inhibitory effects of osthole on IL-11/ERK1/2 signaling pathway.
Results: Our results confirmed that osthole can decrease the secretion of fibrosis proteins, such as α-smooth muscle actin (α-SMA), collagen I, and fibronectin, ameliorate experimental renal fibrosis in vivo and in vitro, and the effect was associated with suppressing TGF-β1/Smad signaling. More importantly, we found that IL-11/ERK1/2 signaling in UUO-induced renal fibrosis and TGF-β-induced HK-2 cell model was obviously upregulated, and osthole treatment also significantly inhibited the abnormal IL-11/ERK1/2 signaling activation. Given the direct link between TGF-β/Smad signaling and IL-11/ERK1/2 signaling pathway, we have verified that osthole has a direct inhibitory effect on IL-11/ERK1/2 signaling independent of TGF-β signaling by using an IL-11-induced HK-2 cells fibrosis model. Osthole treatment decreased the protein expression of α-SMA, collagen I and fibronectin without changing their mRNA levels in IL-11-induced HK-2 cells. Moreover, it was observed that the IL-11/ERK1/2 inhibitor, U0126, partly blocked the antifibrogenic effects of osthole.
Conclusion: In this study, we found that osthole has a previously unrecognized role in inhibiting IL-11/ERK1/2 signaling pathway. Our work demonstrated that the antifibrogenic effect of osthole is not only mediated by TGF-β/Smad2/3 signaling, but also directly mediated by IL-11/ERK1/2 signaling pathway independent of TGF-β1 signaling.
Introduction
Chronic kidney disease (CKD) has become a worldwide health challenge, which brings the great concerns on public health and leads to a massive increase in healthcare burden (Levey and Coresh, 2012). CKD can be caused by various factors, such as diabetes, hypertension, nephrotic syndrome and others, and ultimately progresses to renal failure. Currently, there is still a lack of satisfactory therapeutic strategy for that in clinic. Renal fibrosis is the final common pathological feature in almost all kinds of CKDs, especially in the obstructive nephropathy, which is characterized by ECM deposition, fibroblast activation, tubular atrophy, and inflammatory cell infiltration (Boor et al., 2010; Zeisberg and Neilson, 2010). These histopathological changes of renal fibrosis directly lead to the loss of renal function and the development of end-stage renal damage (Kaissling et al., 2013). Therefore, protecting renal fibrosis is the crucial step for exploring the effective treatments for CKDs (Tampe and Zeisberg, 2014).
It is well known that transforming growth factor-β1 (TGF-β1) is the principal profibrotic factor in organ fibrosis (Schafer et al., 2017). The activation of TGF-β1/Smad signaling directly promotes the transcription of fibrogenic genes, like α-SMA, collagen and fibronectin (Meng et al., 2015), and induced the characteristic pathological manifestations. Targeting TGF-β1 signaling has been proven to have certain effects on organ fibrosis. However, due to the pleiotropic roles of TGF-β1/Smad signaling, its inhibition maybe be related to many side effects (Shull et al., 1992; Bierie et al., 2009). Thus, the downstream molecules of TGF-β1 could be attractive therapeutic targets without upstream disadvantages.
Interleukin-11 (IL-11) is a member of IL-6 family with distinct biological functions. IL-11 was obviously up-regulated in response to TGF-β1 stimulation, and its expression level was strongly correlated with fibroblast activation (Schafer et al., 2017). Unlike IL-6, a classic inflammatory cytokine that signals predominantly via JAK/STAT pathway, recent studies demonstrated that IL-11 causes sustained ERK1/2 activation without activation of STAT pathway (Widjaja et al., 2019). In contrast to TGF-β1, which exerts a remarked effect on the transcription of fibrogenic genes, IL-11 enhances the translation of fibrogenic genes through ERK1/2 signaling (Schafer et al., 2017; Ng et al., 2019; Corden et al., 2020), with a negligible effect on the transcriptional levels. In clinic, IL-11 was initially used to treat chemotherapy-induced thrombocytopenia due to its ability to stimulate megakaryocyte colony formation (Kawashima and Takiguchi, 1992; Isaacs et al., 1997). However, the clinical use of IL-11 brought some common side effects, include atrial arrhythmia, pulmonary congestion and others (Liu et al., 2019; Smith, 2000). These evidences suggested that IL-11 may have a pathogenic role in fibrotic cardiac disease. Meanwhile, animal studies demonstrated that the inhibition of IL-11/ERK1/2 signaling by IL-11 receptor (IL-11RA) knock out or ERK1/2 inhibitors administration apparently relieved the progression of organ fibrosis (Schafer et al., 2017; Corden et al., 2020). Taken together, these findings suggested that the regulation of IL-11/ERK1/2 axis may be a promising strategy for combating renal fibrosis.
Cnidium monnieri (L.) Cusson (Chinese name: She chuang zi) is an important herbal medicine that has been used to treat chronic renal diseases, female genitals, male impotence, frigidity, and skin diseases for centuries in China (Sun et al., 2020). It was documented that the biological activities of Cnidium monnieri (L.) Cusson are mainly mediated by its coumarin components, among which osthole has been studied extensively and is considered to play a vital role in the clinical efficacy of this herb (Li et al., 2015). So, this is why we are interested in this component. Studies have indicated that osthole possesses various pharmacological effects, such as anti-inflammatory, antioxidative, anti-tumor effects, and others (Tsai et al., 2015; Wang et al., 2015; Zhang et al., 2015). The protective effect of osthole on organ fibrosis has been studied. It was found that osthole exerted the obvious protective effects on liver, pulmonary and myocardial fibrosis (Chen et al., 2011; Hao and Liu, 2016; Liu et al., 2015). Additionally, a recent study also indicated that osthole can ameliorate renal fibrosis by suppressing TGF-β1/Smad signaling pathway and epithelial-to-mesenchymal transition (EMT) (Zhang et al., 2018). However, all of these studies mainly focus on the effects of osthole on TGF-β1/Smad signaling pathway, and few studies were conducted to investigate the further downstream mechanisms of TGF-β1 signaling by osthole treatment. Besides, no studies report the pharmacological effects of osthole on the novel therapeutic targets, IL-11/ERK1/2 axis, in renal fibrosis. Thus, this study was designed to address the pharmacological effects of osthole on renal fibrosis and figure out the underlying downstream mechanisms of osthole on TGF-β1 signaling. Our study established osthole having a previously unrecognized role in inhibiting IL-11/ERK1/2 signaling pathway, which provided a new idea for its mechanism explanation and further clinical application.
Materials and Methods
Antibodies and Chemicals
Collagen I and α-SMA antibodies were from Abcam (Cambridge, MA); p-Smad2/3 and Smad2/3 antibodies were obtained from Cell Signaling Technology (Beverly, MA, United States); Fibronectin and ERK1/2 antibodies were purchased from Proteintech (Wuhan, China); IL-11 and p-ERK1/2 antibodies were obtained from ABclonal Technology (Wuhan, China); IL-11RA antibody was from Santa Cruz Biotechnology (Santa Cruz, CA); GAPDH antibody was obtained from Wuhan Gugeshengwu Technology Co., Ltd (Wuhan, China); all secondary antibodies were purchased from Cell Signaling Technology (Beverly, MA, United States).
Osthole (Ost, Figure 1A) was obtained from Sigma-Aldrich (St. Louis, MO); Fetal bovine serum (FBS) was purchased from Gibco (Thermo Fisher Scientific, Waltham, MA); Dulbecco’s modified Eagle medium/Ham’s F-12 medium (DMEM/F12) was purchased from Hyclone Laboratories Inc (Logan, UT, United States); Recombinant human TGF-β1 and IL-11 were obtained from Peprotech (Rocky Hill, NJ, United States); U0126 was provided by Selleck Chemicals (Shanghai, China); Trizol, Hifair™ II 1st Strand cDNA Synthesis Super Mix and Hieff®qPCR SYBR®Green Master Mix were purchased from Yeasen (Shanghai, China); The immunohistochemistry kit was from Wuhan Gugeshengwu Technology Co., Ltd. (Wuhan, China). All other regular reagents were obtained from Wuhan Gugeshengwu Technology Co., Ltd. unless otherwise specified.
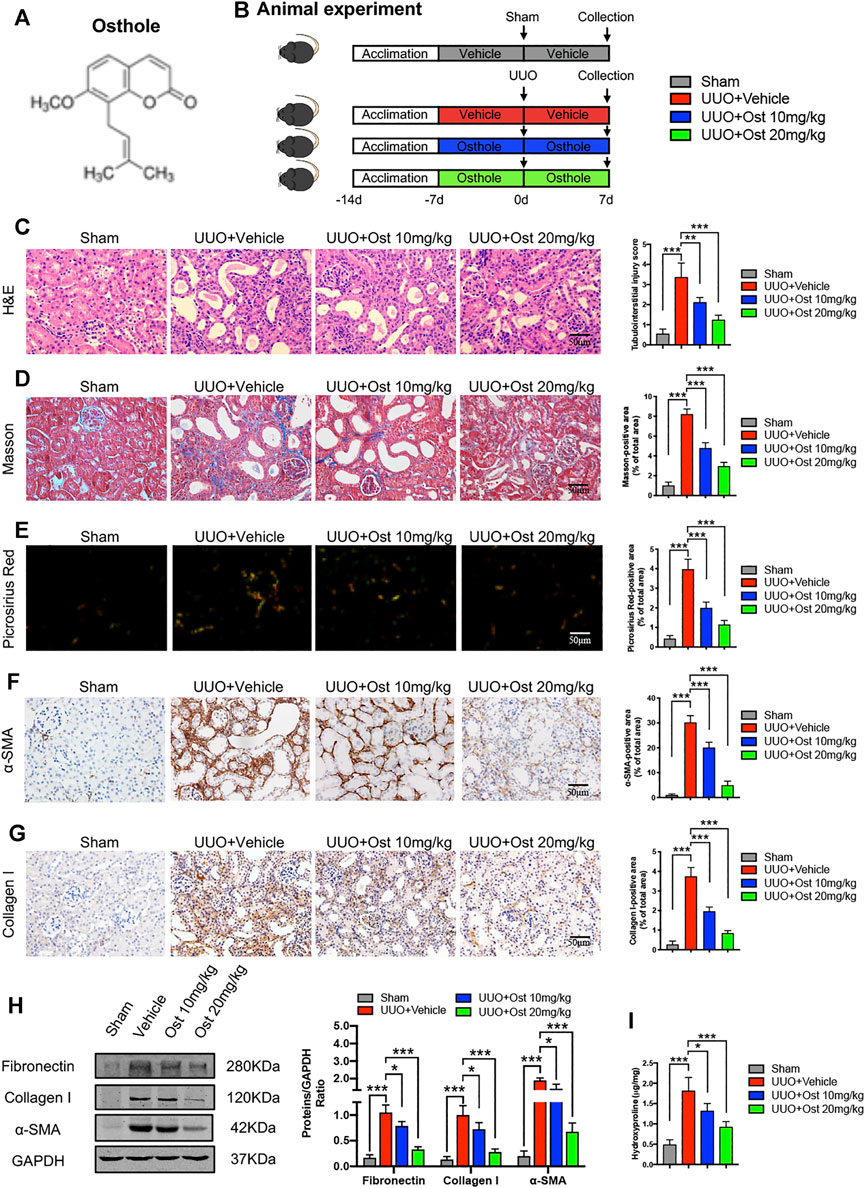
FIGURE 1. Osthole ameliorates UUO-induced renal pathological damage and renal fibrosis in vivo.(A) Chemical structure of osthole. (B) Animal experimental protocol of this study. (C) Representative kidney H&E staining of different groups; the quantification of tubulointerstitial injury score. Scale bar, 50 μm. (n = 6 mice/group) (D) Representative kidney Masson’s trichrome staining of different groups; the quantification of Masson’s trichrome staining. Scale bar, 50 μm. (n = 6 mice/group) (E) Representative kidney Picrosirius Red staining of different groups; the quantification of Picrosirius Red staining. Scale bar, 50 μm. (n = 6 mice/group) (F) Representative immunohistochemistry staining for α-SMA; the quantification of α-SMA immunohistochemistry staining. Scale bar, 50 μm. (n = 6 mice/group) (G) Representative immunohistochemistry staining for collagen I; the quantification of collagen I immunohistochemistry staining. Scale bar, 50 μm. (n = 6 mice/group). (H) Representative western blots for α-SMA, collagen I and fibronectin protein expressions in kidney; the quantification of α-SMA, collagen I and fibronectin western blots. (n = 6 mice/group) (I) Kidney hydroxyproline level of different groups. (n = 6 mice/group). All data are presented as means ± SD. *p < 0.05, **p < 0.01, ***p < 0.001.
Animals and Treatment
The animal studies were overseen and approved by the Animal Ethics Committee of Tongji Medical College, Huazhong University of Science and Technology before and during the experiment. Thirty-two 8 week-old male C57BL/6 mice were purchased from Hubei Province Experimental Animal Research Center (SPF-grade) and housed in an environmentally controlled breeding room (temperature: 20 ± 2°C, humidity: 60 ± 5%, 12°h dark/light cycle). Water and food were given ad libitum throughout the study period. After one-week of adaptive feeding, the mice were randomly divided into four groups (six mice in each group): sham group, model group, 10 mg/kg osthole group and 20 mg/kg osthole group. All mice were received oral gavage of vehicle or osthole daily, and drug treatment lasted for the next 2°weeks until the end of this study. Unilateral ureteral obstruction (UUO) or sham surgery was performed as described previously at the middle of drug treatment (Cheng et al., 2010). Briefly, the mice were anesthetized with 1% pentobarbital (65 μl/10 g), the left ureter was exposed by a midline incision, and then isolated and ligated with 4–0 silk sutures. Sham-operated mice followed the same procedure but their ureters were not ligated. Finally, animals were sacrificed under anesthesia and the kidneys were harvested. The experimental protocol is illustrated in Figure 1B.
Cell Culture and Treatment
Human proximal tubular cell line HK-2 was kindly provided by the Department of nephrology, Tongji hospital, Tongji Medical College, Huazhong University of Science and Technology. HK-2 cells were cultured in DMEM/F12 supplemented with 10% FBS, and maintained at 37°C in a humidified atmosphere of 5% CO2 and 95% air. The cells were synchronized by culturing in medium without FBS for 12 h before it’s used in experiments. After synchronizing, recombinant human TGF-β1 or IL-11, at a final concentration of 10 ng/ml, was added to the culture medium to induce fibrosis in vitro. Osthole treatment begins with the TGF-β1 induction, and lasted for an additional 24 h before harvesting. Osthole used in HK-2 cells was dissolved in 0.1% DMSO, and the equivalent amount of DMSO were applied as control.
Cell Viability Assay
Cell viability was examined by the MTT assay according to the manufacturer’s protocol. Briefly, HK-2 cells were seeded at 1 × 104 cells/well in 96-well plates. After cells adhered, the medium was replaced with DMEM/F12 containing different concentrations of osthole and maintained for another 24 h. 20°μl MTT working solution (5 mg/ml) was added to the medium. Then, the cells were incubated at 37°C for 4–6 h. The absorbance at 570 nm was measured on a Synergr2 multifunctional microplate reader (Bio-Tek, United States).
Kidney Histopathology
For histological analysis, kidney tissues were fixed in 10% formalin, embedded in paraffin, and cut into sections (4°μm thick), which were stained with hematoxylin and eosin (H&E), Masson’s trichrome and Picrosirius Red according to the manufacturer protocol. The tubulointerstitial injury level was evaluated by grading the tubular dilatation, brush border loss, and epithelial simplification. According to the extent of cortical involvement, the degree of damage between none (normal), <25, 25–50, 50–75, and >75% were assigned as 0, 1, 2, 3, and 4 points, respectively. At least 10 randomly non-overlapping cortical fields of each section were selected and evaluated in a blinded manner. Finally, the mean score of each tissue was calculated as the final tubulointerstitial injury score. The collagen deposition was assessed by Masson’s trichrome and Picrosirius Red staining. Images from each section were acquired using an Olympus BX51 system (Olympus, Japan) and analyzed, quantified using ImageJ software (National Institutes of Health, United States).
Immunohistochemistry Staining
For immunohistochemistry (IHC) staining, kidney sections were dewaxed by dimethylbenzene, polarized with descending concentrations of alcohol (75, 95, 100%, 5 min each), and rinsed with deionized water. After that, antigen retrieval was performed by heat-induced epitope retrieval using citrate buffer (10 mM, pH 6.0). The sections were heated to 98°C in microwave, and then maintained at a sub-boiling temperature for 10 min. Then, 3% H2O2 solution was used to block the endogenous peroxidase activity (room temperature, 30 min). The sections were blocked with 10% normal goat serum for 1 h and incubated at 4°C overnight with primary antibodies. At next day, horseradish peroxidase (HRP)-conjugated secondary antibodies were applied for 60 min at room temperature, and these sections were visualized by DAB and counterstained with hematoxylin. Images from each section were captured using an Olympus BX51 system (Olympus, Japan) and analyzed by ImageJ software.
Immunofluorescence Staining
Cultured cells were fixed in 10% formalin for 30 min and blocked with 10% normal goat serum for 1 h. After that, the cells were incubated with primary antibodies at 4°C overnight, followed by staining with Alexa Flour 488 or CY3-conjugated secondary antibody. The nuclei were stained using DAPI or PI solution and all operations were carried out under the light-protected conditions after incubating the secondary antibody. The images were taken by Olympus BX51 fluorescent microscope and analyzed, quantified using ImageJ software.
Western Blot Analysis
Total proteins were extracted from kidney tissues and HK-2 cells using standard protocols, and then protein concentrations were quantified by the bicinchoninic acid (BCA) protein assay kit. An equal amounts of protein extracts were loaded on SDS-PAGE (80 V, 0.5 h and then 120 V, 1 h) and electrotransferred to a 0.45 μm nitrocellulose membrane (280 mA, 1 kDa/min). The membranes were blocked with 5% nonfat milk for 1 h at room temperature and incubated with primary antibodies overnight at 4°C. On the next day, fluorescence-conjugated secondary antibodies were applied to the membranes for 1 h at room temperature. The membranes were visualized with Odyssey Infrared Imaging (LI-COR Biosciences, United States). Target proteins were normalized to GAPDH and quantified by ImageJ software.
Real-Time Quantitative Polymerase Chain Reaction
Total RNA was extracted from kidney tissues and HK-2 cells using Trizol reagent according to the standard protocol. After reverse-transcription, RT-qPCR was performed on LightCycler®96 system (Roche Diagnostics, Mannheim, Germany). The mRNA level of target genes was normalized and analyzed by the 2−ΔΔCT method. Sequences of the primers used in this study are listed in Table 1.
Statistical Analysis
All data are presented as means ± SD. Statistical analyses were performed followed this rule: Firstly, the normality of data is tested by Shapiro-Wilk test; Secondly, data fits the normal distribution are tested for homogeneity of variance via one-way ANOVA; Finally, Tukey’s multiple comparisons test can be performed to compare multiple groups only if there is no significant variance inhomogeneity between groups. Mann-Whitney U-test should be used as an alternative post hoc test when the data show non-normality or variance inhomogeneity. Statistics were analyzed using the GraphPad Prism 8 software, and p < 0.05 was considered as statistically significant.
Results
Osthole Ameliorates Unilateral Ureteral Obstruction-Induced Renal Pathological Damage and Renal Fibrosis in vivo
To verify the protective effects of osthole on renal fibrosis, UUO-induced in vivo renal fibrosis model was established in our study. As shown in Figure 1C, compared to sham group, there was an obvious tubulointerstitial injury including tubular dilatation, epithelial atrophy and interstitial cell infiltration. Osthole administration significantly ameliorated UUO-induced renal pathological damage (Figure 1C). Masson’s trichrome and Picrosirius Red staining were used to intuitively reflect the fibrosis levels between different groups. Both of these results indicated that model group has remarkable collagen deposition in interstitial area, while in the sham group, almost no significant fibrosis is observed. After osthole treatment, UUO-induced renal fibrosis was significantly ameliorated (Figures 1D,E). In addition, we further detected the expression of fibrosis markers (like α-SMA, collagen I and fibronectin) by IHC and western blot. As expected, osthole administration can significantly inhibit the secretion of α-SMA, collagen I and fibronectin, which directly leads to the improvement of renal fibrosis (Figures 1F–H). Hydroxyproline, a peculiar amino acid in collagen tissue, is considered as a sensitive biomarker for fibrosis level. Consistently, compared to control group, osthole treatment apparently reversed the elevated hydroxyproline level (Figure 1I). All together, these data indicated that osthole ameliorates UUO-induced renal pathological damage and renal fibrosis in vivo.
Osthole Ameliorates Transforming Growth Factor-β-Induced HK-2 Cells Fibrosis in vitro
After confirming the protective effects of osthole on renal fibrosis in vivo, we further explored the ameliorative effects of osthole on renal fibrosis in vitro as described previously (Figure 2A). The maximum nontoxic concentration of osthole used in vitro was 100 μM (Figure 2B). Firstly, the mRNA levels of α-SMA, collagen I and fibronectin were determined by RT-qPCR. Compared to control group, TGF-β induction led to the obvious increases in these fibrosis proteins transcription, while osthole treatment showed an inhibitory effect on mRNA expression levels of fibrosis proteins (Figure 2C). Then, we performed Immunofluorescence staining on α-SMA and fibronectin, the results were similar to that of RT-qPCR. Treatment of osthole significantly reduced the excessive expression of α-SMA and fibronectin (Figure 2D). In addition, the protein expression levels of α-SMA, collagen I and fibronectin were also verified by western blot. Figure 2E showed that TGF-β induced an obvious fibrosis phenotype in HK-2 cells compared with control group, while osthole administration led to a significant reduction in fibrosis proteins expression (Figure 2E). Our results demonstrated that osthole can also exert the ameliorative effects on TGF-β-induced HK-2 cells fibrosis in vitro.
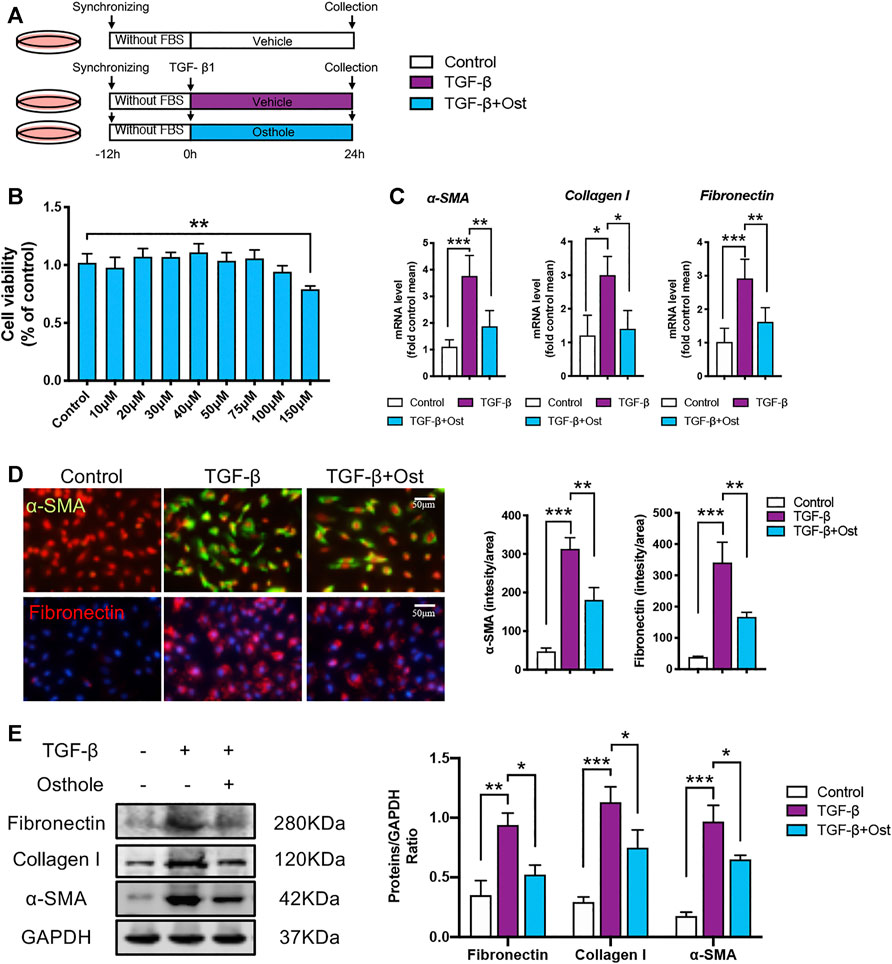
FIGURE 2. Osthole ameliorates TGF-β-induced HK-2 cells fibrosis in vitro.(A)in vitro experimental protocol of this part. (B) MTT assay was applied to determine the concentrations of osthole. (n = 4 repeats) (C) The mRNA levels of α-SMA, collagen I and fibronectin in HK-2 cells of different groups. (n = 4 repeats) (D) Representative immunofluorescence staining for α-SMA and fibronectin; the quantification of α-SMA and fibronectin immunofluorescence staining. Scale bar, 50 μm. (n = 4 repeats) (E) Representative western blots for α-SMA, collagen I and fibronectin protein expressions in HK-2 cells; the quantification of α-SMA, collagen I and fibronectin western blots. (n = 4 repeats). All data are presented as means ± SD. *p <0.05, **p < 0.01, ***p < 0.001.
Osthole Inhibits IL-11/ERK1/2 Signaling Pathway in Experimental Renal Fibrosis Model in vivo and in vitro.
Since the ameliorative effects of osthole on experimental renal fibrosis in vivo and in vitro have been confirmed, we planned to evaluate the underlying mechanism on the antifibrogenic effects of osthole. Recent study has confirmed that osthole inhibits classic TGF-β/Smad signaling in renal fibrosis, which was also verified in our study. Both in vivo and in vitro models, osthole treatment significantly inhibited the activation of Smad2/3 (Supplementary Figure S1). As mentioned above, few studies were conducted to investigate the further downstream mechanisms of TGF-β1 signaling by osthole treatment. Considering the critical role of IL-11, an important downstream effector of TGF-β1 signaling, in the progression of renal fibrosis, thus the key molecules of IL-11/ERK1/2 axis were examined.
Firstly, in order to figure out whether IL-11 signaling was upregulated in renal fibrosis and its changing trend under osthole treatment. The protein expressions of IL-11 and IL-11RA were determined in vivo and in vitro. As shown in Figures 3A–C, in UUO-induced in vivo renal fibrosis, the IL-11 and IL-11RA expressions of model group were largely increased compared with sham group. In TGF-β-induced in vitro fibrosis model, the IL-11 and IL-11RA expressions were also upregulated compared with control group. Osthole treatment significantly reduced the protein expressions of IL-11 and IL-11RA in vivo and in vitro (Figures 3A–C). These results suggested the antifibrogenic effects of osthole may be associated with its inhibition on IL-11 signaling. These results were also confirmed by RT-qPCR (Figures 3D,E). Next, we further assessed the important downstream signal molecule of IL-11: ERK1/2. Western blot analysis of IL-11/ERK1/2 axis both in vivo and in vitro was conducted to explore the underlying mechanisms. It was observed that IL-11/ERK1/2 signaling in renal fibrosis model was significantly upregulated, which was consistent with reported previously (Schafer et al., 2017). Osthole treatment significantly decreased the expressions of IL-11, IL-11RA, and p-ERK1/2, and inhibited the abnormal IL-11/ERK1/2 signaling activation. These results preliminarily verified our hypothesis and suggested that the antifibrogenic effects of osthole may not only be associated with TGF-β/Smad2/3 signaling, but may also be mediated by IL-11/ERK1/2 signaling pathway.
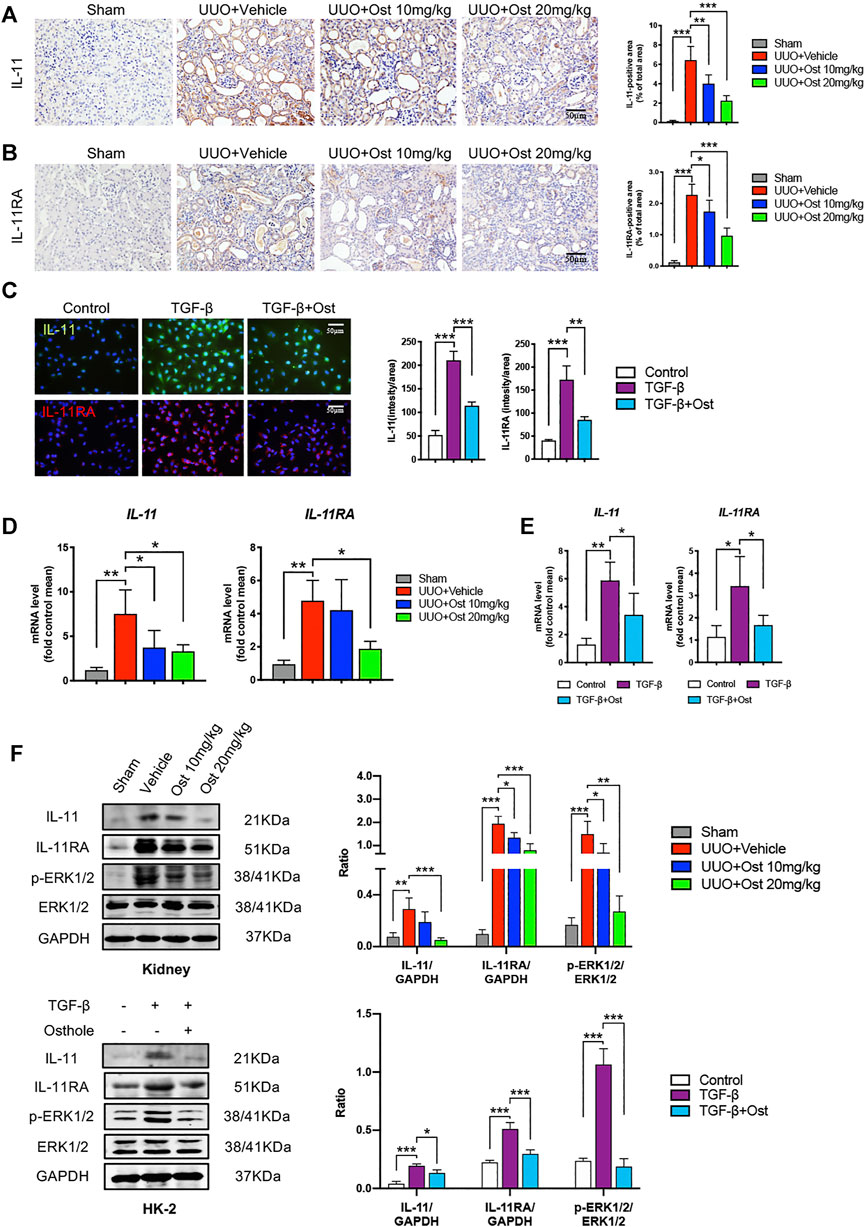
FIGURE 3. Osthole inhibits IL-11/ERK1/2 signaling pathway in experimental renal fibrosis model in vivo and in vitro.(A) Representative immunohistochemistry staining for IL-11; the quantification of IL-11 immunohistochemistry staining. Scale bar, 50 μm. (n = 6 mice/group) (B) Representative immunohistochemistry staining for IL-11RA; the quantification of IL-11RA immunohistochemistry staining. Scale bar, 50 μm. (n = 6 mice/group) (C) Representative immunofluorescence staining for IL-11 and IL-11RA; the quantification of IL-11 and IL-11RA immunofluorescence staining. Scale bar, 50 μm. (n = 4 repeats) (D) The mRNA levels of IL-11 and IL-11RA in kidney of different groups. (n = 6 mice/group) (E) The mRNA levels of IL-11 and IL-11RA in HK-2 cells of different groups. (n = 4 repeats) (F) Representative western blots for IL-11, IL-11RA, p-ERK1/2 and ERK1/2 protein expressions in kidney and HK-2 cells; the quantification of IL-11, IL-11RA, p-ERK1/2 and ERK1/2 western blots. (n = 6 mice/group or 4 repeats in vitro). All data are presented as means ± SD. *p <0.05, **p < 0.01, ***p < 0.001.
The Antifibrogenic Effects of Osthole can be Mediated by IL-11/ERK1/2 Signaling Pathway
However, mechanically, TGF-β1 signaling activation can lead to an obvious improvement in the transcription of fibrogenic genes, and our research object IL-11 is also one of these target genes. This phenomenon was also observed in our study, TGF-β stimulation led to the obvious increases of IL-11 and IL-11RA mRNA expression levels (Figure 3E). It can be expected that the inhibition of TGF-β1 signaling can reduce IL-11 expression, thereby inhibiting IL-11/ERK1/2 signaling pathway. Thus, there is a question whether the inhibition of osthole on IL-11/ERK1/2 signaling pathway is fully attributed to its inhibitory effect on upstream TGF-β1 signaling, or whether there is another mechanism that mediates the inhibition of osthole on IL-11/ERK1/2 signaling pathway.
Therefore, to eliminate the influence of upstream TGF-β1 signaling on mechanism studies, we applied IL-11-induced HK-2 cells model to further explore the mechanisms involved the inhibitory effects of osthole on IL-11/ERK1/2 signaling pathway (Figure 4A). Previous studies revealed that the activation of IL-11/ERK1/2 signaling mainly promotes the translation of fibrogenic genes without changes the transcriptional levels (Corden et al., 2020). Consistently, in our study, there was no statistically significance between the mRNA levels of α-SMA, collagen I and fibronectin in different groups under IL-11 stimulation (Figure 4B). But compared with control group, the protein expressions of α-SMA and fibronectin were still obviously induced by IL-11 stimulation, and osthole treatment still significantly inhibited the secretion of these fibrosis proteins (Figures 4C,D). These data showed that the activation of IL-11 signaling can induce fibrosis phenotype, and this effect is independent of TGF-β1 signaling, because the latter can obviously promote fibrosis proteins transcription. Meanwhile, we also detected the protein expressions of IL-11/ERK1/2 axis. As expected, it was found that IL-11/ERK1/2 signaling was obviously upregulated under IL-11 stimulation, while osthole administration significantly recovered this trend (Figure 4E). Taken together, our data confirmed the effects of IL-11/ERK1/2 axis on the translation of fibrogenic genes and demonstrated that the antifibrogenic effects of osthole can also be mediated by IL-11/ERK1/2 signaling pathway independent of TGF-β1 signaling.
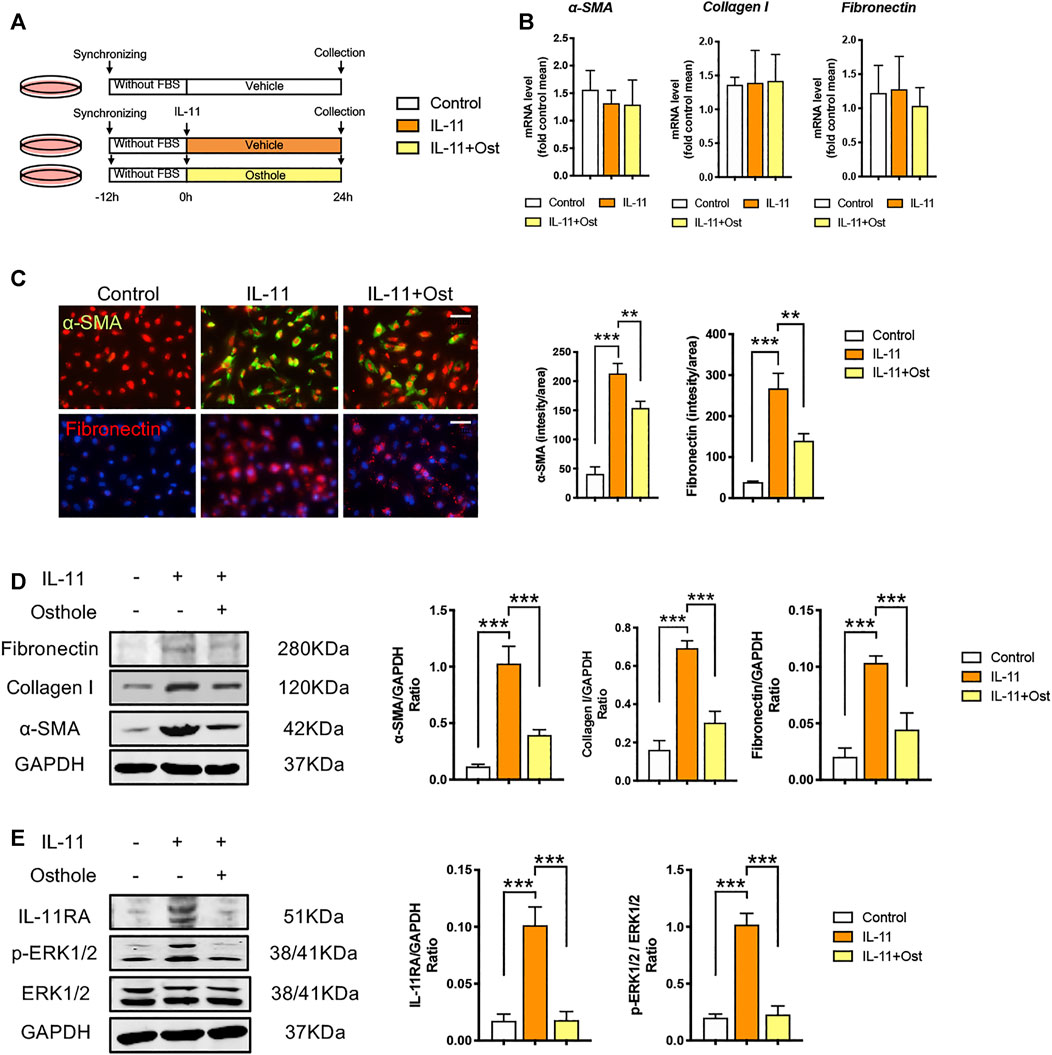
FIGURE 4. The antifibrogenic effects of osthole can be mediated by IL-11/ERK1/2 signaling pathway. (A)in vitro experimental protocol of this part. (B) The mRNA levels of α-SMA, collagen I and fibronectin in HK-2 cells of different groups. (n = 4 repeats) (C) Representative immunofluorescence staining for α-SMA and fibronectin; the quantification of α-SMA and fibronectin immunofluorescence staining. Scale bar, 50 μm. (n = 4 repeats) (D) Representative western blots for α-SMA, collagen I and fibronectin protein expressions in HK-2 cells; the quantification of α-SMA, collagen I and fibronectin western blots. (n = 4 repeats) (E) Representative western blots for IL-11RA, p-ERK1/2 and ERK1/2 protein expressions in HK-2 cells; the quantification of IL-11RA, p-ERK1/2, and ERK1/2 western blots. (n = 4 repeats). All data are presented as means ± SD. *p <0.05, **p < 0.01, ***p < 0.001.
Interleukin-11/ERK1/2 Inhibition Partly Blocks the Antifibrogenic Effects of Osthole
In order to further verify the role of IL-11/ERK1/2 signaling on the antifibrogenic effects of osthole, we conducted an IL-11/ERK1/2 signaling inhibition study. An ERK1/2 inhibitor, U0126, was used to inhibit IL-11/ERK1/2 signaling transduction in TGF-β-induced HK-2 cells fibrosis, while we can assess the role of IL-11/ERK1/2 signaling on the antifibrogenic effects of osthole (Figure 5A).
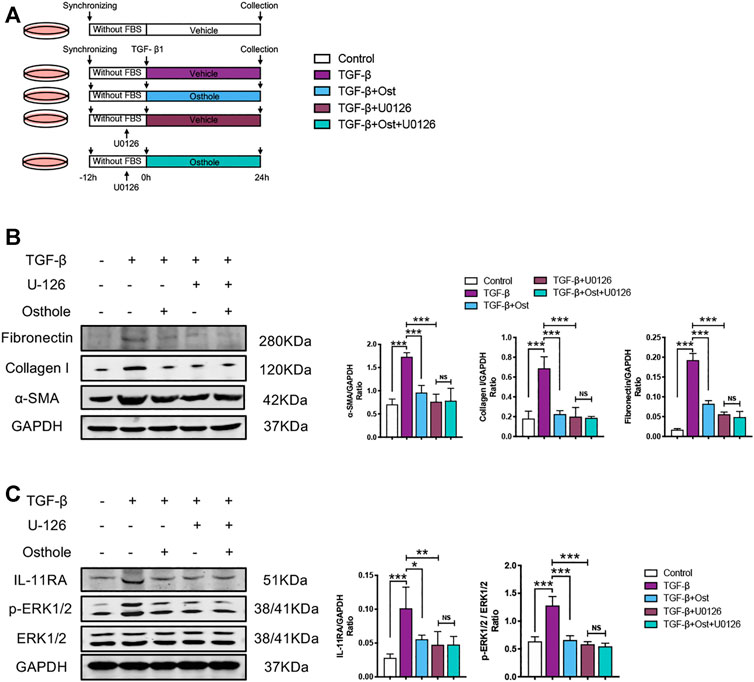
FIGURE 5. IL-11/ERK1/2 inhibition partly blocks the antifibrogenic effects of osthole. (A)in vitro experimental protocol of this part. (B) Representative western blots for α-SMA, collagen I and fibronectin protein expressions in HK-2 cells; the quantification of α-SMA, collagen I and fibronectin western blots. (n = 4 repeats) (C) Representative western blots for IL-11RA, p-ERK1/2 and ERK1/2 protein expressions in HK-2 cells; the quantification of IL-11RA, p-ERK1/2 and ERK1/2 western blots. (n = 4 repeats). All data are presented as means ± SD. *p <0.05, **p < 0.01, ***p < 0.001.
Compared with model group, both osthole and U0126 treatment group all significantly decreased the TGF-β-induced increases in α-SMA, collagen I and fibronectin protein expressions. Under U0126 application, osthole administration did not further reduce the expressions of these fibrosis proteins (Figure 5B). Similarly, the changes of IL-11/ERK1/2 signaling molecules were also consistent with above trend. IL-11/ERK1/2 signaling pathway was obviously inhibited both in osthole and U0126 treatment group, and osthole treatment did not bring another reduction in the expressions of IL-11RA and p-ERK1/2 when compared U0126 alone treatment group with U0126 plus osthole treatment group (Figure 5C). These results demonstrated that the inhibition of IL-11/ERK1/2 axis can partly block the antifibrogenic effects of osthole, which supported our above conclusion.
Discussion
Renal fibrosis represents the common final pathway of nearly all chronic and progressive nephropathies, which showed a rapid increase in prevalence due to the sustained growth in CKDs, regardless of causes (Jha et al., 2013). The initial deposition of fibrotic matrix may occur in the tissue repair process after injury, and it could be resorbed when physiologic repairing can compensate for damaged tissue. Otherwise, in the present of chronic injury, fibrotic matrix deposition continues, eventually leads to organ architecture disruption, blood supply reduction and organ function damage (Wynn, 2008). Collectively, renal fibrosis and CKDs affect half of adults above age 70 and 10% of the world’s population (Humphreys, 2018). Although the therapeutic strategies for end-stage renal diseases have made great progress in the past few decades, but the outcome of renal fibrosis remains poor (Garcia et al., 2012). Therefore, there is an urgent need to seek alternative treatments for that.
Many of the pathophysiologic features underlying renal fibrosis are similar with other fibrotic diseases, like cirrhosis, intestinal fibrosis, cardiomyopathies, and idiopathic pulmonary fibrosis (Wynn, 2011; Ke and Chen, 2012; Weber et al., 2013; Wu et al., 2020). Among that, TGF-β signaling is recognized as the central mediator in renal fibrosis (Meng et al., 2015). A number of studies have demonstrated that TGF-β levels are significantly elevated in patients with various renal diseases, which is strongly correlated with the degree of renal fibrosis (Yamamoto et al., 1996; Murakami et al., 1997). Meanwhile, it was well documented that inhibiting TGF-β with neutralizing antibody, antisense oligonucleotides, inhibitors, or genetic deletion of receptors can attenuate renal fibrosis in vivo and in vitro, whereas the overexpression of TGF-β1 leads to renal fibrosis (Kopp et al., 1996; Border and Noble, 1998; Moon et al., 2006; Petersen et al., 2008; Meng et al., 2012). Mechanically, TGF-β signals through both canonical and non-canonical pathways, in which TGF-β canonical signaling via Smads family plays a major role in the development of renal fibrosis (Meng et al., 2016). Studies revealed that TGF-β signaling mediates renal fibrosis may mainly through followed pathways: 1) TGF-β directly promotes the transcription of fibrogenic genes via Smad2/3-dependent or independent manners; 2) TGF-β inhibits the degradation of ECM by suppressing matrix metalloproteinases (MMPs) and inducing natural inhibitors of MMPs; 3) TGF-β enhances the transdifferentiation of other cell types to increase myofibroblasts pool, such as epithelial-to-mesenchymal transition (EMT) and endothelial-to-mesenchymal transition (EndoMT); 4) TGF-β induces mesangial cells proliferation and loss of tubular epithelial cells, podocytes and endothelial cells (Lopez-Hernandez and Lopez-Novoa, 2012; Meng et al., 2015; Ma and Meng, 2019).
The inhibitory effects of osthole on TGF-β/Smad signaling has been well identified (Chen et al., 2011; Hao and Liu, 2016; Liu et al., 2017; Liu et al., 2015; Zhang et al., 2018). Actually, this point was also confirmed in our study, osthole treatment can significantly reduce the mRNA and protein levels of α-SMA, collagen I and fibronectin, inhibit TGF-β-induced HK-2 cells fibrosis in vitro (Figure 2). Western blot analysis of p-Smad2/3 and Smad2/3 showed that osthole obviously inhibited the TGF-β/Smad2/3 signaling transduction. Based on these results, we can draw a conclusion that the antifibrogenic effects of osthole are related to its inhibitory effects on TGF-β/Smad2/3 signaling. However, we must recognize that TGF-β signaling not only plays a crucial role in fibrosis, but also exerts multiple important biological effects. Thus, in clinic, direct TGF-β-targeted treatments are unlikely to be therapeutically feasible due to the involvement of TGF-β signaling in other systems (Chen et al., 2018). Increasing evidences suggested that the downstream molecules of TGF-β signaling may be attractive therapeutic targets in the treatment of fibrotic diseases (Schafer et al., 2017; Ng et al., 2019). Therefore, exploring the potential effective drugs from the downstream of TGF-β signaling will bring more meaningful value. That’s also the reason why we conducted this study.
As mentioned above, activated TGF-β signaling largely induces the transcription of fibrogenic genes, and IL-11 is one of these target genes. It was reported that IL-11 level is associated with renal injury in diverse animal models and humans (Mitazaki et al., 2009; Grgic et al., 2014; Xu et al., 2014; Menendez-Castro et al., 2020). Except mild craniosynostosis, delayed tooth eruption and variable joint laxity, humans with null mutations in IL-11RA have no other major abnormalities (Nieminen et al., 2011; Keupp et al., 2013; Brischoux-Boucher et al., 2018). IL-11RA−/− mice having slight developmental abnormalities in skeletal system but being otherwise healthy with a normal life span (Nandurkar et al., 1997). Thus, IL-11 signaling may be a promising target downstream of TGF-β. As a member of IL-6 family, IL-11 is a less frequently studied cytokine which has only recently been shown to be an important downstream regulator of TGF-β signaling in organ fibrosis (Schafer et al., 2017). IL-11 signals via combining IL-6 to form a heterodimer complex, which leads to a belief that the biological activities of these cytokines are partly overlapping. However, studies demonstrated that IL-11 causes ERK1/2 activation instead of JAK/STAT pathway (the classic target of IL-6) in organ fibrosis. Unlike TGF-β signaling, IL-11/ERK1/2 axis promotes the expressions of fibrosis proteins mainly by enhances the mRNA translation, and exerts a negligible effect on the transcriptional levels of fibrogenic genes. This effect seems to be dependent on the downstream targets of p-ERK1/2, such as eurkaryotic translation initiation factor 4E (eIF4E) and 40S ribosomal protein S6 kinase (RSK), which are closely associated with the activation of protein translation (Schafer et al., 2017; Corden et al., 2020). Additionally, another recent study indicated that the IL-11-induced protein translation may be mediated by the activation of glutamyl-prolyl-tRNA synthetase (EPRS) (Wu et al., 2020). Regardless of how the activated IL-11/ERK1/2 axis promotes fibrosis protein translation, which was consistent with our expectations. IL-11 stimulation increased the protein levels of fibrosis proteins without changing their mRNA levels (Figure 4). In IL-11-induced HK-2 cells fibrosis model, osthole inhibited the protein translation of fibrosis proteins by suppressing IL-11/ERK1/2 pathway. Our data introduced osthole possessing a previously unrecognized role in inhibiting IL-11/ERK1/2 signaling pathway, which provided a new idea for its antifibrogenic effects and supported its further clinical application. In a word, our work demonstrated that the antifibrogenic effect of osthole is not only mediated by TGF-β/Smad2/3 signaling, but also directly mediated by IL-11/ERK1/2 signaling pathway.
Of course, there are still some limitations in our study. 1) Given the direct link between TGF-β/Smad2/3 signaling and IL-11/ERK1/2 signaling pathway, a genetically engineered mouse model may provide more evidences; 2) Theoretically, we confirmed the effects of IL-11/ERK1/2 signaling induction/inhibition on fibrosis model in vitro, but the in vivo experiments on inhibiting IL-11/ERK1/2 signaling are still lacking; 3) Although, the final effects of activated IL-11/ERK1/2 axis on fibrosis proteins translation and osthole’s inhibitory activities on that have been well identified in our work. How osthole inhibits the translation of fibrosis proteins via IL-11/ERK1/2 signaling is still unclear, and more research is needed in this field.
Conclusion
In this study, we found that osthole has a previously unrecognized role in inhibiting IL-11/ERK1/2 signaling pathway. Osthole can inhibit the translation of fibrosis proteins by suppressing activated IL-11/ERK1/2 signaling in renal fibrosis, eventually contributing to the amelioration of fibrosis. In summary, our work demonstrated that the antifibrogenic effect of osthole is not only mediated by TGF-β/Smad2/3 signaling, but also directly mediated by IL-11/ERK1/2 signaling pathway independent of TGF-β1 signaling (Figure 6).
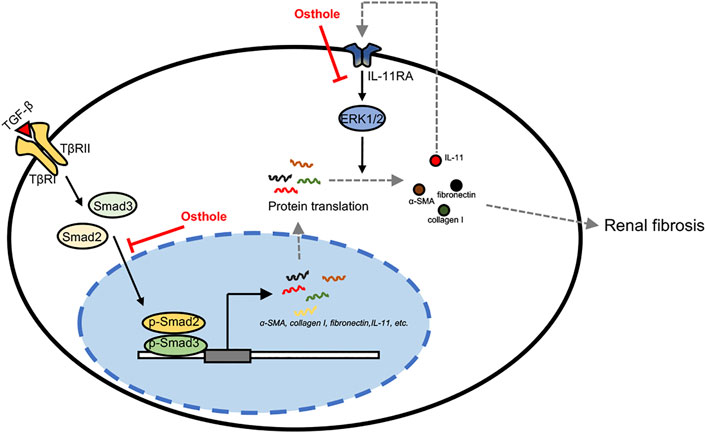
FIGURE 6. The putative mechanism of osthole’s ameliorative effects on renal fibrosis. In the progression of renal fibrosis, activated TGF-β signaling promotes the transcription of fibrogenic genes, like α-SMA, collagen I, fibronectin, IL-11, etc., by transcription factor Smad2/3 nuclear translocation. Meanwhile, the increased IL-11 may also enhance fibrosis proteins translation via activating IL-11/ERK1/2 signaling pathway. These processes eventually contribute to ECM deposition and fibrosis. In this study, we found that osthole has a previously unrecognized role in inhibiting IL-11/ERK1/2 signaling pathway. On the one hand, osthole can inhibit the activation of TGF-β/Smad2/3 signaling, then reducing the transcription of fibrogenic genes, and thereby ameliorating fibrosis. In this process, IL-11/ERK1/2 signaling pathway is also inhibited due to IL-11 is one of the target genes of TGF-β/Smad2/3 signaling. On the other hand, osthole can directly inhibit IL-11/ERK1/2 signaling pathway independent of TGF-β1 signaling, then decreasing the translation of fibrosis proteins, finally ameliorating fibrosis. In summary, our work demonstrated that the antifibrogenic effect of osthole is not only mediated by TGF-β/Smad2/3 signaling, but also directly mediated by IL-11/ERK1/2 signaling pathway independent of TGF-β1 signaling.
Data Availability Statement
The raw data supporting the conclusions of this article will be made available by the authors, without undue reservation.
Ethics Statement
The animal study was reviewed and approved by the Animal Ethics Committee of Tongji Medical College, Huazhong University of Science and Technology.
Author Contributions
GC and JL designed the study. FW and YZ performed the experiments. QS, KF, RD, and SJ contributed to the data analysis. FW wrote the article. GC, JL, and FL reviewed and edited the article. All the authors read and approved the article.
Funding
This work was supported by the National Natural Science Foundation of China (No. 81774259 and 81770413).
Conflict of Interest
The authors declare that the research was conducted in the absence of any commercial or financial relationships that could be construed as a potential conflict of interest.
Supplementary Material
The Supplementary Material for this article can be found online at: https://www.frontiersin.org/articles/10.3389/fphar.2021.646331/full#supplementary-material
Abbreviations
BCA, bicinchoninic acid; CKD, chronic kidney disease; DMEM/F12, dulbecco’s modified Eagle medium/Ham’s F-12 medium; ECM, extracellular matrix; eIF4E, eurkaryotic translation initiation factor 4E; EMT, epithelial-to-mesenchymal transition; EndoMT, endothelial-to-mesenchymal transition; EPRS, glutamyl-prolyl-tRNA synthetase. FBS, fetal bovine serum; HRP, horseradish peroxidase; IHC, immunohistochemistry; MMP, matrix metalloproteinases; OST, osthole; IL-11, interleukin-11; RSK, 40S ribosomal protein S6 kinase; RT-qPCR, real-time quantitative polymerase chain reaction; TGF-β, transforming growth factor-β; UUO, unilateral ureteral obstruction; α-SMA, α-smooth muscle actin
References
Bierie, B., Chung, C. H., Parker, J. S., Stover, D. G., Cheng, N., Chytil, A., et al. (2009). Abrogation of TGF-β Signaling Enhances Chemokine Production and Correlates with Prognosis in Human Breast Cancer. J. Clin. Invest. 119 (6), 1571–1582. doi:10.1172/JCI37480
Boor, P., Ostendorf, T., and Floege, J. (2010). Renal Fibrosis: Novel Insights into Mechanisms and Therapeutic Targets. Nat. Rev. Nephrol. 6 (11), 643–656. doi:10.1038/nrneph.2010.120
Border, W. A., and Noble, N. A. (1998). Evidence that TGF-β Should Be a Therapeutic Target in Diabetic Nephropathy. Kidney Int. 54 (4), 1390–1391. doi:10.1046/j.1523-1755.1998.00127.x
Brischoux-Boucher, E., Trimouille, A., Baujat, G., Goldenberg, A., Schaefer, E., Guichard, B., et al. (2018). IL11RA-related Crouzon-like Autosomal Recessive Craniosynostosis in 10 New Patients: Resemblances and Differences. Clin. Genet. 94 (3-4), 373–380. doi:10.1111/cge.13409
Chen, L., Yang, T., Lu, D.-W., Zhao, H., Feng, Y.-L., Chen, H., et al. (2018). Central Role of Dysregulation of TGF-β/Smad in CKD Progression and Potential Targets of its Treatment. Biomed. Pharmacother. 101, 670–681. doi:10.1016/j.biopha.2018.02.090
Chen, R., Xue, J., and Xie, M.-L. (2011). Reduction of Isoprenaline-Induced Myocardial TGF-Β1 Expression and Fibrosis in Osthole-Treated Mice. Toxicol. Appl. Pharmacol. 256 (2), 168–173. doi:10.1016/j.taap.2011.08.005
Cheng, J., Truong, L. D., Wu, X., Kuhl, D., Lang, F., and Du, J. (2010). Serum- and Glucocorticoid-Regulated Kinase 1 Is Upregulated Following Unilateral Ureteral Obstruction Causing Epithelial-Mesenchymal Transition. Kidney Int. 78 (7), 668–678. doi:10.1038/ki.2010.214
Corden, B., Adami, E., Sweeney, M., Schafer, S., and Cook, S. A. (2020). IL‐11 in Cardiac and Renal Fibrosis: Late to the Party but a Central Player. Br. J. Pharmacol. 177 (8), 1695–1708. doi:10.1111/bph.15013
Garcia, G. G., Harden, P., and Chapman, J. (2012). The Global Role of Kidney Transplantation. Curr. Opin. Nephrol. Hypertens. 21 (3), 229–234. doi:10.1097/MNH.0b013e328352586d
Grgic, I., Krautzberger, A. M., Hofmeister, A., Lalli, M., DiRocco, D. P., Fleig, S. V., et al. (2014). Translational Profiles of Medullary Myofibroblasts during Kidney Fibrosis. Jasn 25 (9), 1979–1990. doi:10.1681/ASN.2013101143
Hao, Y., and Liu, Y. (2016). Osthole Alleviates Bleomycin-Induced Pulmonary Fibrosis via Modulating Angiotensin-Converting Enzyme 2/Angiotensin-(1-7) Axis and Decreasing Inflammation Responses in Rats. Biol. Pharm. Bull. 39 (4), 457–465. doi:10.1248/bpb.b15-00358
Humphreys, B. D. (2018). Mechanisms of Renal Fibrosis. Annu. Rev. Physiol. 80, 309–326. doi:10.1146/annurev-physiol-022516-034227
Isaacs, C., Robert, N. J., Bailey, F. A., Schuster, M. W., Overmoyer, B., Graham, M., et al. (1997). Randomized Placebo-Controlled Study of Recombinant Human Interleukin-11 to Prevent Chemotherapy-Induced Thrombocytopenia in Patients with Breast Cancer Receiving Dose-Intensive Cyclophosphamide and Doxorubicin. Jco 15 (11), 3368–3377. doi:10.1200/JCO.1997.15.11.3368
Jha, V., Garcia-Garcia, G., Iseki, K., Li, Z., Naicker, S., Plattner, B., et al. (2013). Chronic Kidney Disease: Global Dimension and Perspectives. The Lancet 382 (9888), 260–272. doi:10.1016/S0140-6736(13)60687-X
Kaissling, B., Lehir, M., and Kriz, W. (2013). Renal Epithelial Injury and Fibrosis. Biochim. Biophys. Acta (Bba) - Mol. Basis Dis. 1832 (7), 931–939. doi:10.1016/j.bbadis.2013.02.010
Kawashima, I., and Takiguchi, Y. (1992). Interleukin-11: a Novel Stroma-Derived Cytokine. Prog. Growth Factor. Res. 4 (3), 191–206. doi:10.1016/0955-2235(92)90019-e
Ke, P.-Y., and Chen, S. S.-L. (2012). Hepatitis C Virus and Cellular Stress Response: Implications to Molecular Pathogenesis of Liver Diseases. Viruses 4 (10), 2251–2290. doi:10.3390/v4102251
Keupp, K., Li, Y., Vargel, I., Hoischen, A., Richardson, R., Neveling, K., et al. (2013). Mutations in the Interleukin Receptor IL 11 RA Cause Autosomal Recessive Crouzon‐like Craniosynostosis. Mol. Genet. Genomic Med. 1 (4), 223–237. doi:10.1002/mgg3.28
Kopp, J. B., Factor, V. M., Mozes, M., Nagy, P., Sanderson, N., Böttinger, E. P., et al. (1996). Transgenic Mice with Increased Plasma Levels of TGF-Beta 1 Develop Progressive Renal Disease. Lab. Invest. 74 (6), 991–1003.
Levey, A. S., and Coresh, J. (2012). Chronic Kidney Disease. The Lancet 379 (9811), 165–180. doi:10.1016/S0140-6736(11)60178-5
Li, Y.-M., Jia, M., Li, H.-Q., Zhang, N.-D., Wen, X., Rahman, K., et al. (2015). Cnidium Monnieri: A Review of Traditional Uses, Phytochemical and Ethnopharmacological Properties. Am. J. Chin. Med. 43 (5), 835–877. doi:10.1142/S0192415X15500500
Liu, J.-C., Wang, F., Xie, M.-L., Cheng, Z.-Q., Qin, Q., Chen, L., et al. (2017). Osthole Inhibits the Expressions of Collagen I and III through Smad Signaling Pathway after Treatment with TGF-Β1 in Mouse Cardiac Fibroblasts. Int. J. Cardiol. 228, 388–393. doi:10.1016/j.ijcard.2016.11.202
Liu, N.-w., Huang, X., Liu, S., Liu, W.-j., Wang, H., Wang, W.-d., et al. (2019). Elevated BNP Caused by Recombinant Human Interleukin-11 Treatment in Patients with Chemotherapy-Induced Thrombocytopenia. Support Care Cancer 27 (11), 4293–4298. doi:10.1007/s00520-019-04734-z
Liu, Y.-W., Chiu, Y.-T., Fu, S.-L., and Huang, Y.-T. (2015). Osthole Ameliorates Hepatic Fibrosis and Inhibits Hepatic Stellate Cell Activation. J. Biomed. Sci. 22, 63. doi:10.1186/s12929-015-0168-5
López-Hernández, F. J., and López-Novoa, J. M. (2012). Role of TGF-β in Chronic Kidney Disease: an Integration of Tubular, Glomerular and Vascular Effects. Cell Tissue Res 347 (1), 141–154. doi:10.1007/s00441-011-1275-6
Ma, T.-T., and Meng, X.-M. (2019). TGF-β/Smad and Renal Fibrosis. Adv. Exp. Med. Biol. 1165, 347–364. doi:10.1007/978-981-13-8871-2_16
Menendez-Castro, C., Cordasic, N., Dambietz, T., Veelken, R., Amann, K., Hartner, A., et al. (2020). Correlations between Interleukin-11 Expression and Hypertensive Kidney Injury in a Rat Model of Renovascular Hypertension. Am. J. Hypertens. 33 (4), 331–340. doi:10.1093/ajh/hpz194
Meng, X.-M., Huang, X. R., Xiao, J., Chen, H.-y., Zhong, X., Chung, A. C., et al. (2012). Diverse Roles of TGF-β Receptor II in Renal Fibrosis and Inflammation In Vivo and In Vitro. J. Pathol. 227 (2), 175–188. doi:10.1002/path.3976
Meng, X.-m., Nikolic-Paterson, D. J., and Lan, H. Y. (2016). TGF-β: the Master Regulator of Fibrosis. Nat. Rev. Nephrol. 12 (6), 325–338. doi:10.1038/nrneph.2016.48
Meng, X.-M., Tang, P. M.-K., Li, J., and Lan, H. Y. (2015). TGF-β/Smad Signaling in Renal Fibrosis. Front. Physiol. 6, 82. doi:10.3389/fphys.2015.00082
Mitazaki, S., Kato, N., Suto, M., Hiraiwa, K., and Abe, S. (2009). Interleukin-6 Deficiency Accelerates Cisplatin-Induced Acute Renal Failure but Not Systemic Injury. Toxicology 265 (3), 115–121. doi:10.1016/j.tox.2009.10.005
Moon, J.-A., Kim, H.-T., Cho, I.-S., Sheen, Y. Y., and Kim, D.-K. (2006). IN-1130, a Novel Transforming Growth Factor-β Type I Receptor Kinase (ALK5) Inhibitor, Suppresses Renal Fibrosis in Obstructive Nephropathy. Kidney Int. 70 (7), 1234–1243. doi:10.1038/sj.ki.5001775
Murakami, K., Takemura, T., Hino, S., and Yoshioka, K. (1997). Urinary Transforming Growth Factor-β in Patients with Glomerular Diseases. Pediatr. Nephrol. 11 (3), 334–336. doi:10.1007/s004670050289
Nandurkar, H. H., Robb, L., Tarlinton, D., Barnett, L., Köntgen, F., and Begley, C. G. (1997). Adult Mice with Targeted Mutation of the Interleukin-11 Receptor (IL11Ra) Display Normal Hematopoiesis. Blood 90 (6), 2148–2159. doi:10.1182/blood.v90.6.2148.2148_2148_2159
Ng, B., Dong, J., D’Agostino, G., Viswanathan, S., Widjaja, A. A., Lim, W.-W., et al. (2019). Interleukin-11 Is a Therapeutic Target in Idiopathic Pulmonary Fibrosis. Sci. Transl. Med. 11 (511), eaaw1237. doi:10.1126/scitranslmed.aaw1237
Nieminen, P., Morgan, N. V., Fenwick, A. L., Parmanen, S., Veistinen, L., Mikkola, M. L., et al. (2011). Inactivation of IL11 Signaling Causes Craniosynostosis, Delayed Tooth Eruption, and Supernumerary Teeth. Am. J. Hum. Genet. 89 (1), 67–81. doi:10.1016/j.ajhg.2011.05.024
Petersen, M., Thorikay, M., Deckers, M., van Dinther, M., Grygielko, E. T., Gellibert, F., et al. (2008). Oral Administration of GW788388, an Inhibitor of TGF-β Type I and II Receptor Kinases, Decreases Renal Fibrosis. Kidney Int. 73 (6), 705–715. doi:10.1038/sj.ki.5002717
Schafer, S., Viswanathan, S., Widjaja, A. A., Lim, W.-W., Moreno-Moral, A., DeLaughter, D. M., et al. (2017). IL-11 Is a Crucial Determinant of Cardiovascular Fibrosis. Nature 552 (7683), 110–115. doi:10.1038/nature24676
Shull, M. M., Ormsby, I., Kier, A. B., Pawlowski, S., Diebold, R. J., Yin, M., et al. (1992). Targeted Disruption of the Mouse Transforming Growth Factor-Β1 Gene Results in Multifocal Inflammatory Disease. Nature 359 (6397), 693–699. doi:10.1038/359693a0
Smith, J. W. (2000). Tolerability and Side-Effect Profile of rhIL-11. Oncology (Williston Park) 14 (9 Suppl. 8), 41–47.
Sun, Y., Yang, A. W. H., and Lenon, G. B. (2020). Phytochemistry, Ethnopharmacology, Pharmacokinetics and Toxicology of Cnidium Monnieri (L.) Cusson. Ijms 21 (3), 1006. doi:10.3390/ijms21031006
Tampe, D., and Zeisberg, M. (2014). Potential Approaches to Reverse or Repair Renal Fibrosis. Nat. Rev. Nephrol. 10 (4), 226–237. doi:10.1038/nrneph.2014.14
Tsai, Y.-F., Yu, H.-P., Chung, P.-J., Leu, Y.-L., Kuo, L.-M., Chen, C.-Y., et al. (2015). Osthol Attenuates Neutrophilic Oxidative Stress and Hemorrhagic Shock-Induced Lung Injury via Inhibition of Phosphodiesterase 4. Free Radic. Biol. Med. 89, 387–400. doi:10.1016/j.freeradbiomed.2015.08.008
Wang, X.-l., Shang, X., Cui, Y., Zhao, X., Zhang, Y., and Xie, M.-l. (2015). Osthole Inhibits Inflammatory Cytokine Release through PPARα/γ-Mediated Mechanisms in LPS-Stimulated 3T3-L1 Adipocytes. Immunopharmacology and Immunotoxicology 37 (2), 185–192. doi:10.3109/08923973.2015.1009997
Weber, K. T., Sun, Y., Bhattacharya, S. K., Ahokas, R. A., and Gerling, I. C. (2013). Myofibroblast-mediated Mechanisms of Pathological Remodelling of the Heart. Nat. Rev. Cardiol. 10 (1), 15–26. doi:10.1038/nrcardio.2012.158
Widjaja, A. A., Singh, B. K., Adami, E., Viswanathan, S., Dong, J., D’Agostino, G. A., et al. (2019). Inhibiting Interleukin 11 Signaling Reduces Hepatocyte Death and Liver Fibrosis, Inflammation, and Steatosis in Mouse Models of Nonalcoholic Steatohepatitis. Gastroenterology 157 (3), 777–792. doi:10.1053/j.gastro.2019.05.002e714
Wu, F., Shao, Q., Hu, M., Zhao, Y., Dong, R., Fang, K., et al. (2020). Wu-Mei-Wan Ameliorates Chronic Colitis-Associated Intestinal Fibrosis through Inhibiting Fibroblast Activation. J. Ethnopharmacology 252, 112580. doi:10.1016/j.jep.2020.112580
Wu, J., Subbaiah, K. C. V., Xie, L. H., Jiang, F., Khor, E.-S., Mickelsen, D., et al. (2020). Glutamyl-Prolyl-tRNA Synthetase Regulates Proline-Rich Pro-fibrotic Protein Synthesis during Cardiac Fibrosis. Circ. Res. 127 (6), 827–846. doi:10.1161/CIRCRESAHA.119.315999
Wynn, T. A. (2011). Integrating Mechanisms of Pulmonary Fibrosis. J. Exp. Med. 208 (7), 1339–1350. doi:10.1084/jem.20110551
Wynn, T. (2008). Cellular and Molecular Mechanisms of Fibrosis. J. Pathol. 214 (2), 199–210. doi:10.1002/path.2277
Xu, L., Podok, P., Xie, J., and Lu, L. (2014). Comparative Analysis of Differential Gene Expression in Kidney Tissues of Moribund and Surviving Crucian Carp (Carassius auratus Gibelio) in Response to Cyprinid Herpesvirus 2 Infection. Arch. Virol. 159 (8), 1961–1974. doi:10.1007/s00705-014-2011-9
Yamamoto, T., Noble, N. A., Cohen, A. H., Nast, C. C., Hishida, A., Gold, L. I., et al. (1996). Expression of Transforming Growth Factor-β Isoforms in Human Glomerular Diseases. Kidney Int. 49 (2), 461–469. doi:10.1038/ki.1996.65
Zeisberg, M., and Neilson, E. G. (2010). Mechanisms of Tubulointerstitial Fibrosis. Jasn 21 (11), 1819–1834. doi:10.1681/ASN.2010080793
Zhang, L., Jiang, G., Yao, F., Liang, G., Wang, F., Xu, H., et al. (2015). Osthole Promotes Anti-tumor Immune Responses in Tumor-Bearing Mice with Hepatocellular Carcinoma. Immunopharmacology and Immunotoxicology 37 (3), 301–307. doi:10.3109/08923973.2015.1035391
Keywords: osthole, IL-11/ERK1/2 signaling, renal fibrosis, HK-2, unilateral ureteral obstruction
Citation: Wu F, Zhao Y, Shao Q, Fang K, Dong R, Jiang S, Lu F, Luo J and Chen G (2021) Ameliorative Effects of Osthole on Experimental Renal Fibrosis in vivo and in vitro by Inhibiting IL-11/ERK1/2 Signaling. Front. Pharmacol. 12:646331. doi: 10.3389/fphar.2021.646331
Received: 26 December 2020; Accepted: 28 April 2021;
Published: 13 May 2021.
Edited by:
Dan-Qian Chen, Northwest University, ChinaReviewed by:
Kyung Pyo Kang, Jeonbuk National University, South KoreaGeorge Bayliss, Brown University, United States
Copyright © 2021 Wu, Zhao, Shao, Fang, Dong, Jiang, Lu, Luo and Chen. This is an open-access article distributed under the terms of the Creative Commons Attribution License (CC BY). The use, distribution or reproduction in other forums is permitted, provided the original author(s) and the copyright owner(s) are credited and that the original publication in this journal is cited, in accordance with accepted academic practice. No use, distribution or reproduction is permitted which does not comply with these terms.
*Correspondence: Jinlong Luo, dG9uZ2ppbHVvamlubG9uZ0BhbGl5dW4uY29t; Guang Chen, Z3VhbmdjaGVuQHRqaC50am11LmVkdS5jbg==
†These authors have contributed equally to this work