- 1Shenzhen Traditional Chinese Medicine Hospital, the Fourth Clinical Medical College of Guangzhou University of Chinese Medicine, Shenzhen, China
- 2School of Pharmaceutical Sciences, Guangzhou University of Chinese Medicine, Guangzhou, China
- 3School of Basic Medical Science, Tianjin Medical University, Tianjin, China
- 4School of Medicine, Shenzhen University, Shenzhen, China
- 5Science and Technology Innovation Center, Guangzhou University of Chinese Medicine, Guangzhou, China
Helicobacter pylori was classified by the World Health Organization as a class 1 carcinogen. The development of drug-resistant strains of this pathogen poses a serious threat to human health worldwide. The cell invasion of H. pylori activates xenophagy in gastric epithelial cells by mediating miR-30b/c, and the emergence of autophagosomes provides a niche that enables the survival of intracellular H. pylori and promotes its drug resistance. This study revealed that some clinical drug-resistant H. pylori strains present much stronger invasive ability than standard strains. Patchouli alcohol (PA), a tricyclic sesquiterpene from Pogostemon cablin (Blanco) Benth (Labiatae), showed reliable activity against intracellular H. pylori. The mechanisms appeared to involve the downregulation of miR-30c-3p/5p and miR-30b-5p, thereby upregulating xenophagy-related gene expression (ULK1, ATG5, ATG12, and ATG14) and enhancing xenophagy. PA also inhibited the nuclear transfection of miR-30b-5p induced by H. pylori, thereby enhancing transcription factor EB function and increasing lysosome activity. The finding of strongly invasive intracellular H. pylori has great implications for clinical treatment, and PA can act against invasive H. pylori based on the improvement of miR-30b/c mediated xenophagy. Taken together, the results demonstrate that PA have potential use as a candidate medication for intracellular drug-resistant H. pylori.
Introduction
Helicobacter pylori was classified by the World Health Organization as a class 1 carcinogen, infecting half of the world’s population (Ferrero et al., 2012). H. pylori plays a major role in gastric ulcer, atrophic gastritis, and gastric carcinoma. Triple therapy (a proton pump inhibitor combined with two antibiotics, like clarithromycin [CLR], metronidazole [MTZ]) are adopted to treat the infection, however, the increasing drug-resistant rate of H. pylori definitely undermine the efficacy of eradication treatment. The infection process of H. pylori is fairly complex. First, the bacteria penetrate the gastric mucosa via its flagella and chemotaxis protein (Lertsethtakarn et al., 2011). It then adheres to the surface of gastric epithelial cells; some bacteria can invade and survive inside epithelial cells. The ability of H. pylori to survive intracellularly is believed to be one of the strategies through which it escapes the action of drugs (Necchi et al., 2007). As the bacterium invades host gastric epithelial cells, the tolerance against drugs H. pylori is significantly enhanced (Chu et al., 2010).
When H. pylori invades epithelial cells, the immune response of the cells is activated. Xenophagy is a key protective response against bacterial invasion (Zhang et al., 2014). Autophagosomes wrap around intracellular bacteria and then merge with lysosomes to form autolysosomes; the bacteria are digested and eliminated thereafter (Sharma et al., 2018). However, over the course of its evolution, H. pylori has developed the ability to escape from autophagosomes. Vacuolating cytotoxin A (VacA), a toxic factor secreted by H. pylori, disrupts the initiation and digestion processes of xenophagy, which leads to the survival of H. pylori inside autophagosomes (Greenfield and Jones, 2013). Compromised xenophagy by miR-30b is considered to be one of the major mechanisms for the self-protective abilities of H. pylori (Tang et al., 2012). Thus, promoting xenophagy can enhance the elimination of intracellular bacteria (Liao et al., 2016; Sui et al., 2017).
Patchouli alcohol (Figure 1, PubChem CID: 10955174) is one of the major ingredients of Pogostemon cablin (Blanco) Benth (Labiatae), which is widely used to treat gastrointestinal diseases in East Asian countries. The effects of PA against H. pylori infection and induced gastritis in vitro and in vivo were demonstrated in our previous research (Xu et al., 2017; Lian et al., 2018). The inhibitory effect of PA on H. pylori urease highlights its reliable promotive effects on macrophage-mediated H. pylori digestion (Lian et al., 2019). In this study, we compared the cell invasive ability of some clinical drug-resistant and standard H. pylori strains, and investigated the effects of PA against intracellular H. pylori and the underlying mechanisms.
Material and Methods
Chemicals and Drugs
DMEM–high-glucose medium and fetal bovine serum (FBS) were purchased from Gibco (United States). Brain heart infusion (BHI) and Columbia agar base were obtained from OXOID (United States). Sheep blood was procured from Nanjing Maojie Biotechnology (China). Saponins were purchased from Sigma–Aldrich (United States). Gentamicin sulfate was purchased from Toku-E (Japan). CytoTrace™ Green CMFDA was obtained from AAT Bioquest (United States). mRFP-LC3-GFP adenovirus was purchased from HANBIO (China).
PA (purity >99%) was provided by the Mathematical Engineering Academy of Chinese Medicine, and its structure was confirmed as previously described (Qing et al., 2014). The sesquiterpene was dissolved in DMSO and adjusted to the necessary concentration for in vitro assays. PA solid dispersions were prepared using Poloxamer 407 as described in a previous publication for in vivo assays (Liao et al., 2015).
H. pylori and Cell Culture
H. pylori strains NCTC11637 and NCTC26695 were purchased from the American Type Culture Collection. Sydney strain 1 (SS1) was gifted by Richard Ferrero from Monash University, Australia. The clinical strains Hp 1868, Hp 1869 (MTZ resistant strain), Hp 1870 (CLR and MTZ resistant strain), and Hp 1872 (MTZ resistant strain) were obtained from Renji Hospital, Shanghai Jiaotong University (The virulence genotypes of those H. pylori strains are shown in Supplementary Table S3). The identification and drug resistance of those strains was shown in our previous publication (Xu et al., 2017). H. pylori was cultured in Columbia agar base with 5% sheep blood at 37°C in a tri-gas incubator (Nuaire, United States) containing 10% CO2, 5% O2, and 85% N2, and the bacteria were passaged every 48 h. BHI supplemented with 30% glycerol was adopted as a cryoprotectant. The passage number of all strains were less than 20.
GES-1 and MKN45 cells were purchased from BNCC Biotechnology Company (China). GES-1 cells were cultured in DMEM–high-glucose medium (10% FBS), while MKN45 cells were cultured in RPMI 1640 (20% FBS). Both cell strains were cultured in an incubator at 37°C with 5% CO2. The cells were passaged using 0.25% trypsin when they covered over 80% of the dish. The passage number of those two cell lines were less than 20.
Cells and Treatment
GES-1 or MKN45 cells were cultured in six-well plates at a density of 1.5×106 cells/well. The cells were allowed to adhere to the walls of the wells for 12 h and then treated with 12.5, 25, or 50 μM PA or DMSO for 12 h. The doses of PA in vitro assay were selected based on our previous study (Lian et al., 2019). The cells were washed thrice with PBS. H. pylori was suspended in PBS, adjusted to appropriate quantity by a turbidity meter, and used to infect the cells at a multiplicity of infection (MOI) of 100:1. Rapamycin (100 nM, Meilune, China) and 3-MA (5 mM, Meilune, China) were selected as positive controls.
Gentamycin Protection Assay
After drug pretreatment and H. pylori infection as described in “Cells and treatment” section, the cell culture media were collected, diluted, and cultured onto Columbia blood agar. The number of colonies formed 5 days later was counted as the extracellular bacterial number. The rest of the cells were washed twice with PBS and then incubated with 100 μg/ml gentamycin for 6 h to clear the extracellular bacteria. Subsequently, 0.1% saponin was adopted to increase membrane permeability, and the suspension was diluted and flooded on culture plates to form colonies. The number of colonies formed 5 days later was counted as the intracellular bacterial number.
Lactate Dehydrogenase Release Measurement
GES-1 or MKN45 cells were cultured in six-well plates at a density of 1.5×106 cells/well. The cells were divided into five groups, including the control (DMSO), H. pylori (DMSO + H. pylori for 12 h), and 12.5, 25, and 50 μM PA (pretreatment with the indicated dose of PA for 12 h and then infected with H. pylori at MOI = 100:1) treatment groups. After 4 h of co-culture (Supplementary Material S1), the supernatant was removed for lactate dehydrogenase (LDH) measurement (Nanjing Jiancheng Company, China).
Immunofluorescence Assay and Confocal Microscopy
GES-1 cells were treated and infected with H. pylori for 12 h as described in “Cells and treatment” section. After treatment with 100 μg/ml gentamycin for 6 h, the adherent cells were washed thrice with PBS, fixed with 4% paraformaldehyde for 10 min, permeabilizated with 0.1% Triton-100 in PBST for 30 min, and blocked with 5% donkey serum in PBST for 1 h. The cells were then incubated with the primary antibodies, including anti-H. pylori (1:200, Abcam, ab20459, United States) and anti-transcription factor EB (TFEB, 1:500, Bethyl Laboratories, A303–673A, United States), dissolved in PBST containing 5% donkey serum at 4°C overnight. The next day, the samples were washed thrice with PBST and incubated with the secondary antibodies (1:200, goat anti-rabbit 488, Southern Biotech, 4,050–30, United States) for 2 h at room temperature. Hoechst 33,342 was used to stain the nucleus of the cells. The samples were mounted using Fluoromount-G™ (Invitrogen, United States) and observed by confocal microscopy (LSM 800, Zeiss, Germany).
Transmission Electron Microscopy
GES-1 cells were treated and infected with H. pylori for 12 h as described in “Cells and treatment” section. After treatment with 100 μg/ml gentamycin for 6 h, the adherent cells were washed thrice with PBS and fixed with transmission electron microscopy (TEM) fixing solution (2.5% glutaraldehyde, LEAGENE, China). Cell scrapers was used to separate the cells, which were then collected by centrifugation at 1,000 rpm for 5 min. Finally, the samples were treated with 1 ml of fresh TEM fixing solution, followed by 1% osmium tetroxide, dehydrated with an acetone gradient, and embedded using Epon-812. After ultrathin sectioning, the samples were stained with uranyl acetate and lead citrate and observed by TEM.
mRFP-LC3-GFP Adenovirus Transfection Assay
GES-1 cells were cultured in glass-bottomed dishes for 24 h and then added with 6.3 × 107 PFU/ml mRFP-LC3-GFP adenovirus for 24 h of transfection (Supplementary Material S2, S3). The samples were divided into the following groups: ① without clear extracellular bacteria: DMSO (control), 200 nM rapamycin, 6.25 μg/ml H. pylori protein, H. pylori (MOI = 100:1), and 25 μM PA groups; ② with clear extracellular bacteria: DMSO (control), 100 μg/ml gentamycin (to exclude the possible influence of gentamycin on xenophagy), H. pylori (MOI = 100:1), and PA + H. pylori (pretreatment with 12.5, 25, or 50 μM PA for 12 h, infection with H. pylori at MOI = 100:1 for 12 h, and then treatment with 100 μg/ml gentamycin to clear extracellular bacteria) groups. H. pylori total protein extraction was followed by freeze–thawing cycling and sonification as described in our previous publication (Ren et al., 2019). Confocal microscopy was used to observe autophagosomes (yellow dots) and autolysosomes (red dots), and the numbers of autophagosomes and autolysosomes were analyzed using ImageJ.
Lysosome Activity Assessment
LysoTracker Red DND-99 (Meilune, China) was used to identify the lysosome-rich areas of GES-1 cells. Briefly, GES-1 cells were cultured in glass-bottomed dishes, treated as described in “Cells and treatment” section, and then incubated in prewarmed 50 nM LysoTracker Red DND-99 for 1 h in a CO2 incubator. Finally, the staining liquid was replaced with fresh cell culture medium, and the samples were observed by confocal microscopy at 555 nm.
Cathepsin D activity was measured using a kit from Abcam (ab65302, United States). Briefly, GES-1 cells were treated as described in “Cells and treatment” section, washed twice with cold PBS, digested with 0.25% trypsin, and then resuspended in PBS. The cells were subsequently collected by centrifugation at 1,000 rpm and 4°C and resuspended in cell lysis buffer on ice for 10 min. The supernatants were obtained by centrifugation at 16,000× g for 5 min at 4°C. The cell lysis samples were mixed with reaction buffer and incubated with the substrate at 37°C for 2 h. A fluorescence microplate reader was used to measure the absorbance of the solution at excitation and emission wavelengths of 328 and 460 nm, respectively.
Nuclear and Cytoplasmic Protein Extraction and Western Blot Assay
For total protein extraction, the GES-1 cells were treated as described in “Cells and treatment” section, washed twice with cold PBS, and lyzed with precooled RIPA (1% protease inhibitor) on ice for 15 min. The cells were scraped, collected into vials, and placed on ice for another 15 min for cell lysis. Next, the samples were sonicated at 300 W for 10 s at 5 s intervals for 2 min. The samples were centrifuged at 10,000× g for 10 min at 4°C, and the supernatant was collected for BCA assay. For nuclear and cytoplasmic protein extraction, the cell protein samples were extracted following the instructions of the NE-PER™ Nuclear and Cytoplasmic Extraction Reagents kit (Thermo Fisher, United States). Exactly 30 μg (4 μg for nuclear and cytoplasmic protein) of the protein samples was loaded onto 4–20% SurePAGE™ gels (Genscript, China) for electrophoresis and then transferred onto PVDF membranes using eBlot L1 Trans-Blot (Genscript, China). The membranes were blocked with 5% nonfat-dried milk and then incubated with anti-LC3 (1:1,000, CST, 12741S, United States), anti-P62 (1:1,000, CST, 39749S, United States), anti-TFEB (1:2000, Bethyl Laboratories, A303–673A, United States), and anti-β-actin (1:5,000, SAB, 21,338, United States) antibodies overnight at 4°C. The following day, the membranes were incubated with the secondary antibodies (CST, 7074S for anti-rabbit, 7076S for anti-mouse, United States) for 2 h at room temperature. Finally, the blots were treated with ECL reagent (Millipore, United States), observed under a chemiluminescence apparatus (Bio-rad, United States), and analyzed using ImageJ software.
miRNA Chip Assay
GES-1 cells were cultured in a six-well plate at a density of 1.5×106 cells/well. The cells were allowed to adhere to the walls of the wells for 12 h and then treated with DMSO (control) or 50 μM PA for 12 h. The cells were subsequently collected with Trizol (Life Technology, United States), and miRNA chip assay was performed by RIBOBIO Co.
Nuclear and Cytoplasmic RNA Extraction and Quantitative Real-Time PCR Assay
GES-1 cells were infected with H. pylori (MOI = 100:1) for 6, 12, or 24 h or treated as described in “Cells and treatment” section. Total RNA samples were extracted using the RNApure Tissue and Cell Kit (CWBIO, China), while miRNA samples were extracted using the miRNA Purification Kit (CWBIO). Nuclear and cytoplasmic RNA samples were isolated using the Cytoplasmic and Nuclear RNA Purification Kit (Norgen, Canada). The purity of the nuclear RNA was determined using U2 snRNA (as a nuclear marker) and S14 (as a cytoplasmic marker).
The quality of the RNA samples was determined using a Nanodrop 2000 instrument (Thermo Scientific, United States), and samples with A260/A280 between 1.9 and 2.1 were considered to be of good quality. cDNA was generated using 1 μg of RNA with the FastKing-RT SuperMix Kit (TIANGEN, China), and real-time fluorescence quantitative qPCR assay was subsequently performed using Talent qPCR PreMix (TIANGEN, China). Here, GADPH was selected as the internal reference. The miRcute Plus miRNA First-Strand cDNA Kit (TIANGEN, China) was used to produce cDNA from the miRNA samples via the tailing reaction method, and the miRcute Plus miRNA qPCR Kit (TIANGEN, China) was subsequently used to explore the expression of the miRNA. U6 was selected as the internal reference for the miRNA assay (Supplementary Material S4).
Statistical Analysis
Results are expressed as mean ± SD. Enumeration data were analyzed by one-way ANOVA followed by Dunnett’s test or the LSD test to compare differences among groups. The chi-squared test was used to analyze enumerated data. p < 0.05 was considered to indicate statistical significance.
Results
H. pylori May Achieve Long-Term Infection Through Intracellular Invasion
The time-dependent invasion of different H. pylori strains was studied on the basis of the co-culture and gentamycin protection assays (Figure 2; Supplementary Material S5). The invasion of all strains continually increased over 12 h of culture. The intracellular/extracellular bacterial ratios of the three clinical strains, (i.e. Hp 1868, Hp 1869, and Hp 1870) were significantly higher than those of the three standard strains, (i.e. NCTC11637, NCTC26695, and SS1; p < 0.01). The intracellular/extracellular bacterial ratios obtained indicate that some clinical strains show stronger cell invasive ability than standard strains. Thus, intracellular invasion may be a strategy for the long-term infection of H. pylori.
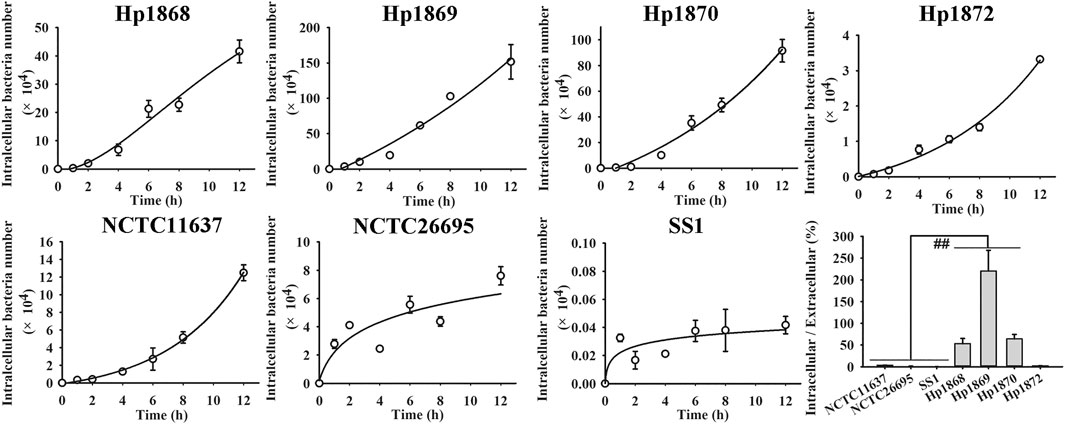
FIGURE 2. Time-dependent invasion of different H. pylori strains. The invasion rates of standard and clinical H. pylori strains increased over 12 h. The invasion rates of Hp 1868 (52.93 ± 24.23%), Hp 1869 (220.31 ± 95.68%), and Hp 1870 (63.88 ± 20.77%) were significantly higher than those of the standard strains NCTC11637 (3.46 ± 0.35%), NCTC26695 (1.24 ± 0.49%), and SS1 (0.01 ± 0.002%). **p < 0.01, n = 6.
PA Ameliorates the Effects of H. pylori Infection and the Intracellular H. pylori Burden on GES-1 and MKN45 Cells
In the intracellular H. pylori survival assay (Figure 3B), PA treatment (12.5, 25, or 50 μM) significantly reduced the numbers of H. pylori NCTC11637, Hp 1868, and Hp1872 in GES-1 cells and the number of H. pylori NCTC11637 in MKN45 cells. Treatment with 25 or 50 μM PA also decreased the number of H. pylori Hp1869 in GES-1 cells (p < 0.01, p < 0.05). However, PA showed no obvious influence on the invasion of Hp 1870. Visual and similar tendency (NCTC11637 in GES-1 cells) was observed by confocal microscopy, as shown in Figure 3A. Treatment with the autophagy agonist rapamycin reduced the number of intracellular H. pylori, whereas treatment with the autophagy inhibitor 3-MA increased the number of intracellular bacteria found (p < 0.01). High levels of extracellular LDH reflect cell damage, and results indicated that treatment with 25 or 50 μM PA could protect GES-1 cells from H. pylori infection (p < 0.01). In addition, treatment with 50 μM PA reduced the level of extracellular LDH in H. pylori-infected MKN45 cells (p < 0.01, p < 0.05).
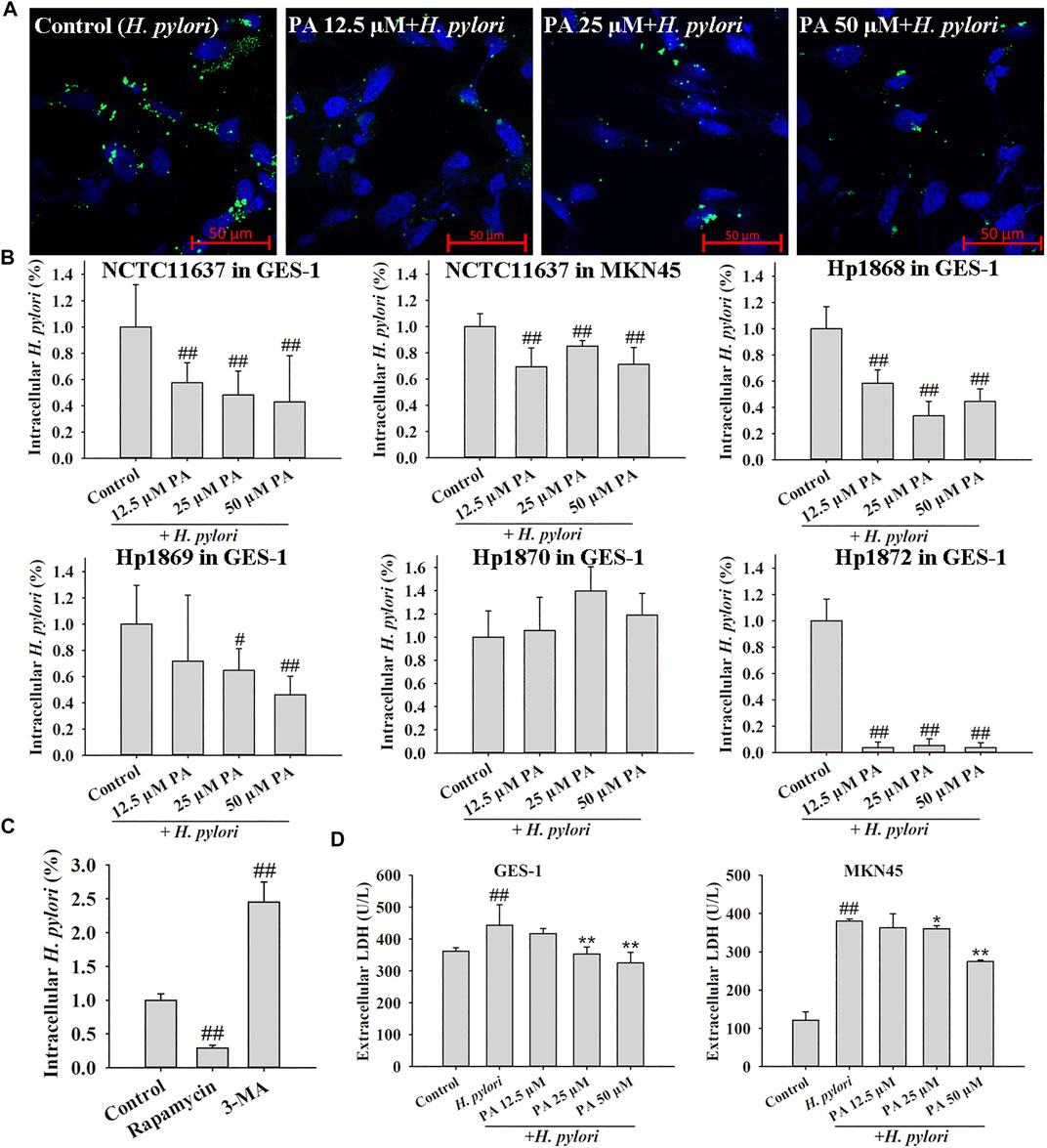
FIGURE 3. Effects of PA, an autophagy agonist (rapamycin), and an autophagy inhibitor (3-MA) on intracellular H. pylori survival. (A) Representative confocal images of intracellular H. pylori treated with PA. (B). Effects of PA on the intracellular H. pylori strains NCTC11637, Hp 1868, Hp 1869, Hp 1870, and Hp 1872. The control group includes cells infected with H. pylori without treatment (n = 6). (C) Rapamycin reduced the number of intracellular H. pylori NCTC11637 whereas 3-MA increased the intracellular number of H. pylori NCTC11637 in GES-1 cells. The control group includes cells infected with H. pylori without treatment (n = 3). (D) LDH release from GES-1 and MKN45 cells increased following H. pylori infection. PA (25 and 50 μM) protected these cells from H. pylori infection (n = 6). #p < 0.05, ##p < 0.01, compared with the control group. *p < 0.05, **p < 0.01, compared with the H. pylori group.
PA Increases Xenophagy After H. pylori Infection
As shown in Figure 4, obvious cell membrane damage could be observed following treatment with H. pylori (black arrows). Double-membraned autophagosomes (red arrows) and single-membraned autolysosomes (blue arrows) were also noted. Treatment with PA, especially at a dose of 50 μM, significantly increased autophagic flux levels, as observation of a mass of autophagosomes and autolysosomes.
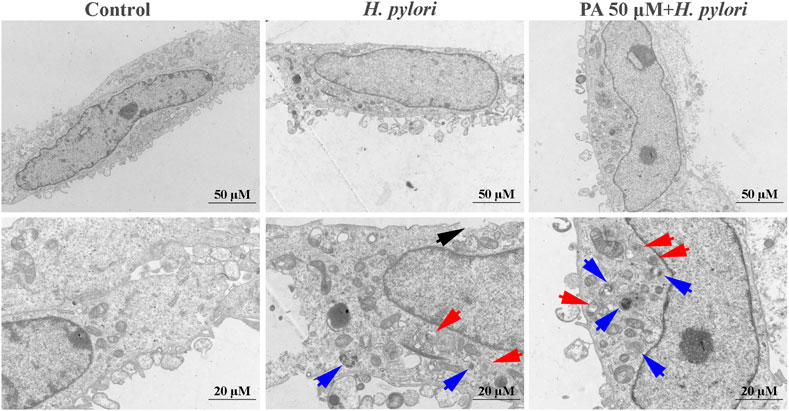
FIGURE 4. Representative TEM micrographs of the ultrastructures of H. pylori-infected GES-1 cells. Double-membraned autophagosomes, red arrows; single-membraned autolysosomes, blue arrows; damaged cell membranes, black arrows.
PA Promotes Xenophagy Against Intracellular H. pylori
As shown in Figure 5, the numbers of autophagosomes and autolysosomes significantly increased following treatment with rapamycin, H. pylori (MOI = 100:1), and H. pylori protein (6.25 μg/ml). Treatment with PA slightly increased the number of autophagosomes (p < 0.01, p < 0.05). The numbers of autophagosomes in the H. pylori and H. pylori protein groups were similar, but the number of autolysosomes in the H. pylori protein group was nearly twice that in the H. pylori group. This result indicates that intact H. pylori, but not the protein only, may cause the severe destruction of the autophagic flux. As shown in Figure 6A, gentamycin treatment had no effect on autophagy, and extracellular bacterial clearance clearly downregulated the autophagy level induced by H. pylori. Treatment with 25 or 50 μM PA remarkably increased the numbers of autophagosomes and autolysosomes induced by H. pylori infection (Figure 6B,C; p < 0.01). Similar results were observed in the Western blot assays. As shown in Figure 6D, although H. pylori infection induced the overexpression of LC3-II and P62 (p < 0.01), LC3-II levels further increased following 50 μM PA treatment (p < 0.05). P62 levels decreased in the 25 and 50 μM PA groups (p < 0.01, p < 0.05).
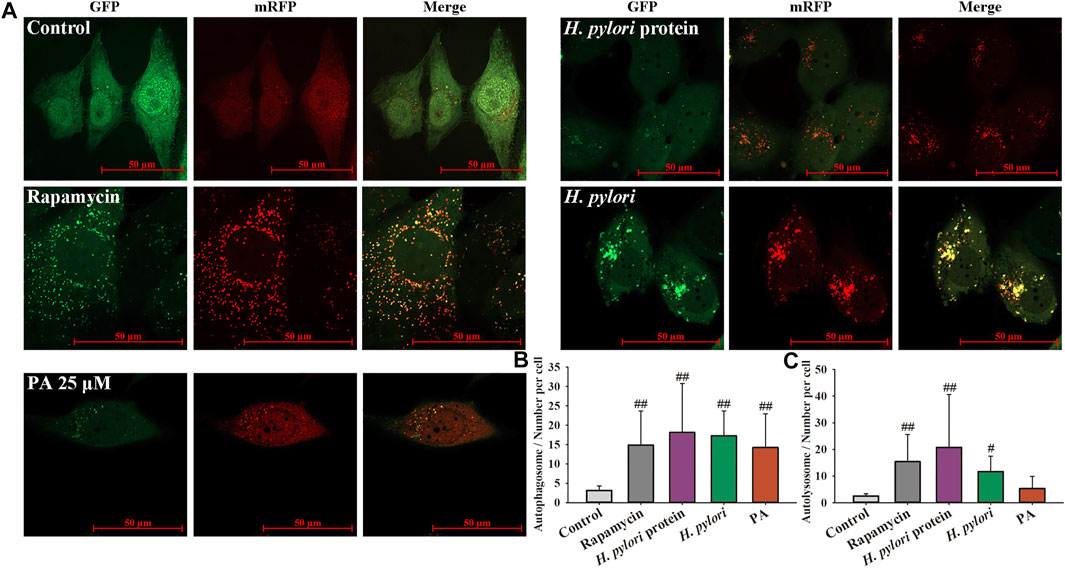
FIGURE 5. H. pylori infection induced xenophagy in GES-1 cells. mRFP-LC3-GFP adenovirus was adopted to label autophagosomes and autolysosomes. GFP fluorescence is quenched when autophagosomes fuse with lysosomes. Merged yellow dots indicate autophagosomes, and autolysosome numbers were calculated as number of red dots minus number of yellow dots. (A) Representative images of the xenophagy state after different treatments for 12 h. (B) Quantitative results of autophagosomes. (C) Quantitative results of autolysosomes. #p < 0.05, ##p < 0.01, compared with the control group, n = 4.
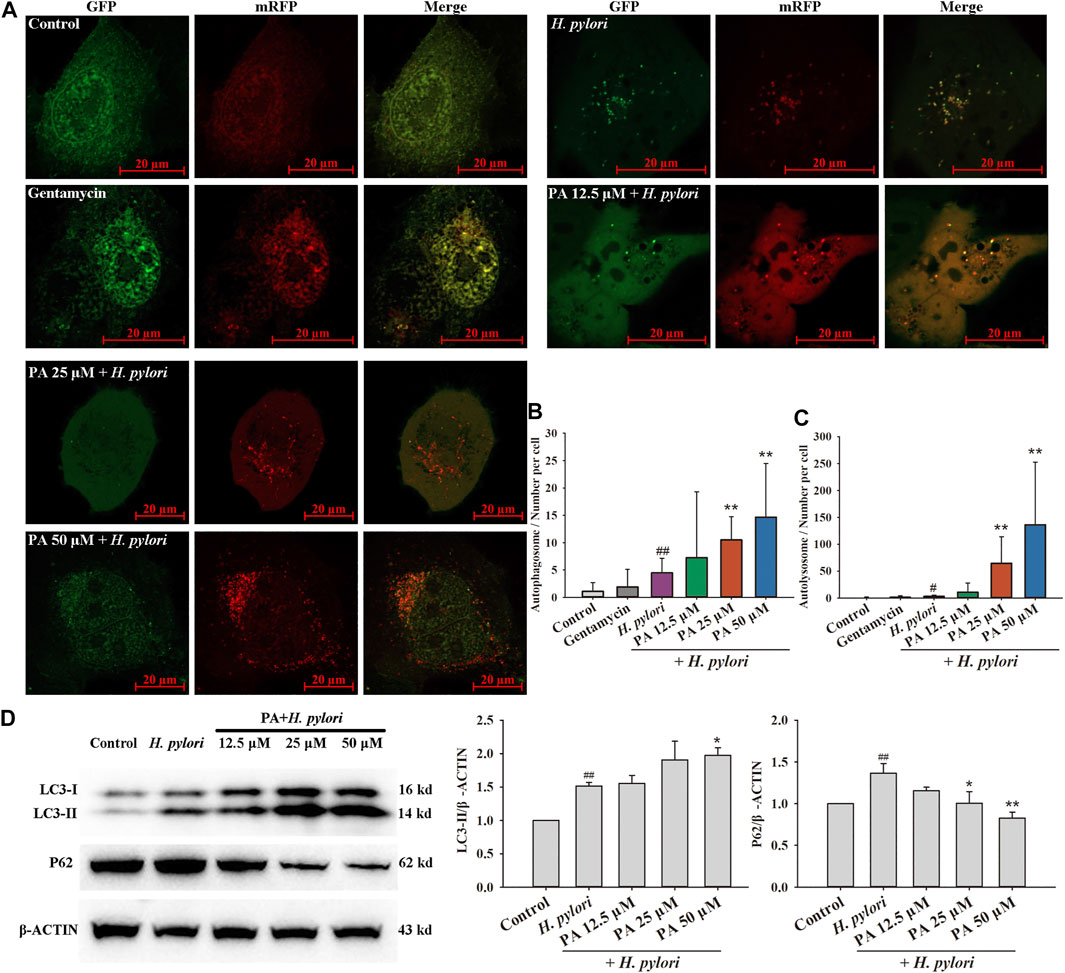
FIGURE 6. Effects of PA on H. pylori-induced xenophagy. (A) Representative images of xenophagy after treatment with gentamycin and H. pylori with or without PA. (B) Quantitative results of autophagosomes (n = 4). (C) Quantitative results of autolysosomes (n = 4). (D) Representative Western blot results of LC3 and P62. Upregulation of LC3-II confirmed the occurrence of xenophagy, while downregulation of P62 revealed the fusion of lysosomes with autophagosomes and digestion of the content (n = 5). The results indicate that PA improves the xenophagic flux and promotes digestion. ##p < 0.01, compared with the control group. *p < 0.05, **p < 0.01, compared with the H. pylori group.
PA May Defend Against Intracellular H. pylori Infection by Downregulating miR-30 b and miR-30c
The effect of PA on the miRNA expression of GES-1 cells was investigated by miRNA chip assay, and the results of KEGG analysis based on the differentially expressed miRNAs obtained revealed that PA may regulate several cellular processes, including ubiquitin-mediated proteolysis, protein processing in the endoplasmic reticulum, and autophagy (Figure 7A; Supplementary Material S6). The effects of PA on miR-30c-3p, miR-30c-5p, and miR-30b-5p were then investigated. The expression of miR-30c-3p, miR-30c-5p, and miR-30b-5p was remarkably upregulated by H. pylori infection (p < 0.01), but treatment with PA (12.5, 25, or 50 μM) significantly downregulated the expression of miR-30c-5p and miR-30b-5p (p < 0.01, p < 0.05). Moreover, treatment with 25 or 50 μM PA downregulated the expression of miR-30c-3p (Figure 7B; p < 0.01). The expression of the target genes of miR-30c-3p (ULK1 and ATG14), miR-30c-5p (ATG12), and miR-30b-5p (ATG5 and ATG12) were further analyzed (Figure 7C). The results revealed that H. pylori infection decreases the gene expression of ULK1, ATG5, and ATG12 (p < 0.01, p < 0.05), but treatment with 12.5, 25, or 50 μM PA increases the gene expression of ULK1, ATG12, and ATG14 (p < 0.01, p < 0.05). Moreover, treatment with 50 μM PA upregulated the gene expression of ATG5 (p < 0.01).
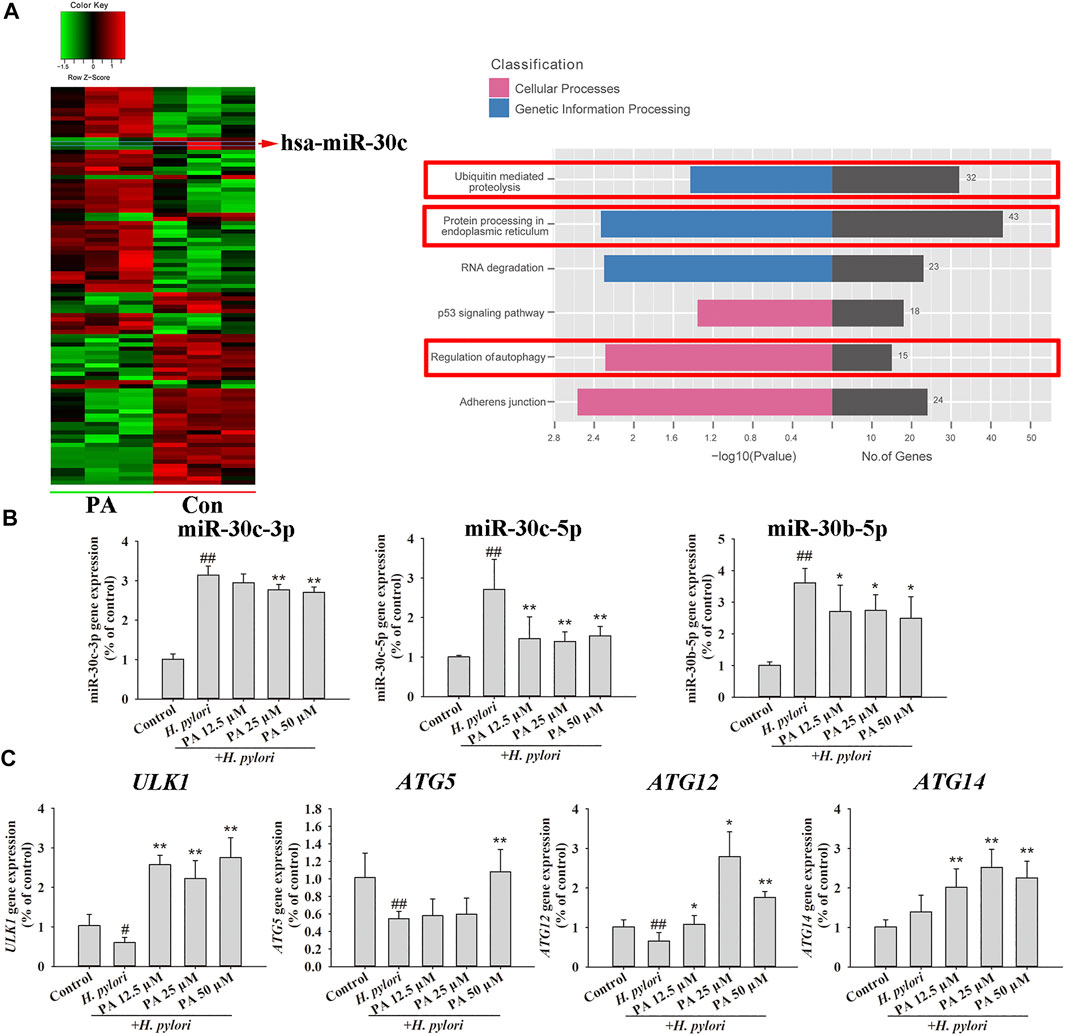
FIGURE 7. MiRNA regulation by PA promoted xenophagy. (A) MiRNA chip assay was performed on PA-treated or untreated GES-1 cells, and KEGG analysis was conducted to assess differentially expressed miRNAs (n = 3). (B) Quantitative gene expression results of miR-30c-3p, miR-30c-5p, and miR-30b-5p. (C) Quantitative gene expression results of ULK1, ATG5, ATG12, and ATG14. ##p < 0.01, compared with the control group. *p < 0.05, **p < 0.01, compared with the H. pylori group, n = 6.
PA Increases Lysosome Activity and Promotes Intracellular H. pylori Digestion by Downregulating miR-30b-5p in the Nucleus
Assays of lysosome activity (Figure 8A) indicated that H. pylori infection reduces the activity of cathepsin D (p < 0.01). Downregulation of galactosidase alpha (GLA) gene expression (p < 0.01) and upregulation of tripeptidyl peptidase one gene expression (p < 0.01) were observed after H. pylori infection; these genes are representative genes of lysosomes. Treatment with 50 μM PA significantly increased the expression of GLA and lysosome-associated membrane protein-1 (p < 0.01, p < 0.05). Acidic lysosomes were identified using LysoTracker Red DND-99, and the results indicated that H. pylori infection nearly completely eliminates the acidic lysosomes of GES-1 cells. Pretreatment with rapamycin and 50 μM PA partly recovered these lysosomes, and differences between these and the H. pylori infection groups were statistically significant (p < 0.01). In the co-localization assay (Figure 8B), the nuclear translocation of TFEB obviously increased in H. pylori-infected GES-1 cells, as reflected by alterations in the Mander and Pearson correlation coefficients. However, no changes in the location of TFEB were noted after treatment with rapamycin and pA. The time-dependent transfection of TFEB to the cell nucleus was assessed by Western blot assay (p < 0.01, p < 0.05), which revealed no distinct trend of nuclear TFEB translocation. The isolated nuclear RNA was purity, because the difference in CT value exceeded 5, as shown in Figure 8C. The transfection of miR-30b-5p to the cell nucleus remarkably increased 6, 12, and 24 h after H. pylori infection (p < 0.01, p < 0.05). Rapamycin and PA significantly reduced the amount of endonuclear miR-30b-5p (p < 0.01).
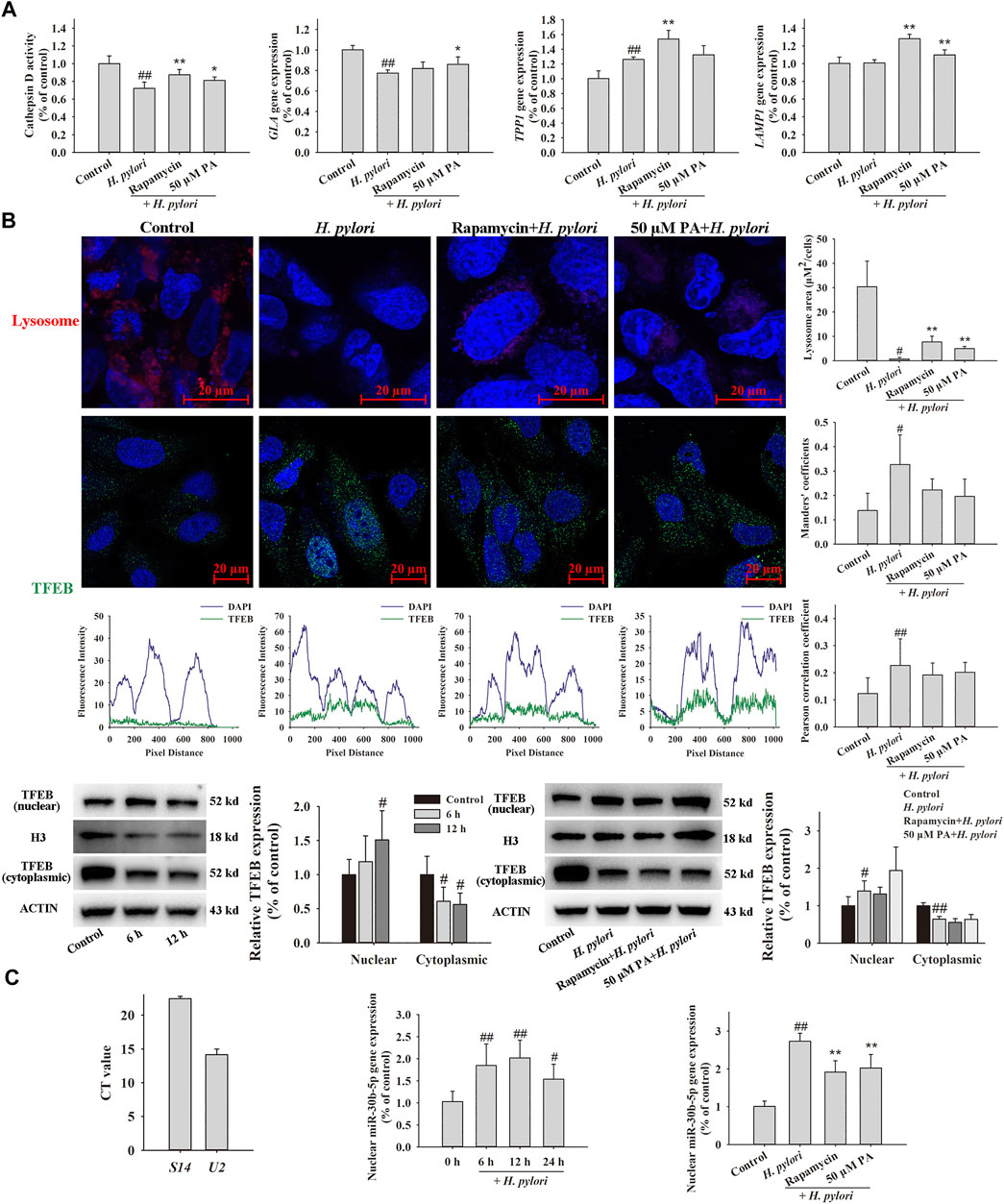
FIGURE 8. Effects of PA on lysosome function. GES-1 cells were pretreated with rapamycin or PA for 12 h, infected with H. pylori for 12 h (except for the time-dependent assay), and then analyzed by qRT-PCR, Western blot, and immunofluorescence assays. (A) Effects of PA on H. pylori-induced lysosome activity, including cathepsin D activity (n = 8), representative lysosome gene expression, and representative images of LysoTracker Red DND-99-labeled lysosome (n = 5). (B) Effects of PA on the lysosome mediator TFEB, including representative images of TFEB colocalized with nuclear DAPI and the corresponding quantitative data (n = 8), representative Western blots (n = 5), and quantitative data of nuclear and cytoplasmic TFEB. (C) Effects of PA on the nuclear transfection of miR-30b-5p, including confirmation of the successful isolation of nuclear RNA, time-dependent changes in nuclear miR-30b-5p expression, and downregulation of nuclear miR-30b-5p expression following PA treatment (n = 6).
Discussion
The increased resistance of H. pylori to antibiotics affects the clearance rate of triple and quadruple therapies worldwide, especially in developing countries such as China. Indeed, the resistance rate of the pathogen to metronidazole, clarithromycin, and levofloxacin has been reported to be as high as 78.2, 22.1, and 19.2%, respectively (Liu et al., 2018). The invasion of H. pylori into gastric epithelial cells exponentially increases the tolerance of the bacteria against drugs, and low-dose or inappropriate antibiotic treatment could produce an unfavorable external environment that may further promote H. pylori invasion. Therefore, sufficient first-time treatment is crucial. The rates of secondary resistance of H. pylori to multiple antibiotics may attributed to the high invasion phenomenon (Lyu et al., 2020). Our results of the comparative study indicated that some clinical strains have stronger invasive ability than standard strains, and the intracellular invasion may be a survival strategy of H. pylori because intracellular environments allow bacteria to avoid immune cells or exposure to antimicrobials (Thakur et al., 2019). Another research revealed that the invasive ratio of NCTC26695 is 2.5%, which is consistent with our results (Kwok et al., 2002). To date, whether the intracellular survival of H. pylori plays a role in pathopoiesia is unknown. And our lab has begun a clinical experiment to explore the influence of increasing invasion of H. pylori on patient illness and clinical therapy. Recent studies have revealed that impairment of the lysosomal calcium channel MCOLN1/TRPML1 by VacA can improve the intracellular survival ability of H. pylori (Capurro et al., 2020). In short, these findings strongly suggest that eradication of intracellular H. pylori should receive attention during clinical treatment.
Intracellular survival contributes to the drug tolerance of H. pylori (Lai et al., 2006), and the activation of xenophagy by H. pylori produce autophagosome, which provide a niche for the intracellular survival of H. pylori, thus increasing the frequency of drug-resistant bacteria (Greenfield and Jones, 2013). The drug-resistant H. pylori accompanied with strong invasive ability would bring a lot of trouble to clinical therapy undoubtedly. Besides, unfavorable environments with low concentrations of antibiotics may promote bacterial invasion. Pretreatment with PA decreased the intracellular number of H. pylori, reduced the release of LDH, and protected GES-1 cells. These findings demonstrate the promising potential clinical applications of PA in intracellular bacteria therapy. Immunofluorescence and gentamycin protection assays were adopted to analyze the intracellular number of H. pylori. Immunofluorescence images enable the detailed observation of intracellular bacteria. However, the time-consuming gentamycin protection assay provides more accurate data because dead bacteria can also be stained.
TEM revealed that H. pylori treatment could damage the cell membrane and increase the autophagic flux, whereas PA treatment could further improve it. Similar results were obtained in the adenovirus transfection assay, which showed that PA increases the abundance of autolysosomes and promotes the digestion of H. pylori. Previous studies revealed that the VacA secreted by H. pylori could react with low-density lipoprotein receptor-related protein 1 (LRP1) receptors in epithelial cells, which activates xenophagy (Chauhan et al., 2019). The bacteria then hijack the xenophagy process, inhibit autophagosome formation, and reduce cathepsin D in lysosomes, all of which contribute to the intracellular survival of H. pylori (Sit et al., 2020). H. pylori protein is sufficient for xenophagy activation (Zhu et al., 2017), but the invasion of intact H. pylori bacteria seems to lead to more lethal effects than exposure to the protein only.
MiRNA-30 families play a key role in H. pylori-related xenophagy. For example, the miRNAs miR-30b and miR-30 days have been directly associated with H. pylori-induced xenophagy (Tang et al., 2012; Zhang et al., 2019a). KEGG analysis of cellular processes and genetic information processing was performed on the basis of the results of miRNA chip assay, and findings indicated that PA affects ubiquitin, the endoplasmic reticulum, and autophagy. Further verification assay confirmed the effects of PA on miR-30c-3p, miR-30c-5p, and miR-30b-5p, and the related target genes. The time difference of gene and protein expression contribute to the confliction of downregulation of xenophagy gene (ULK1, ATG5 and ATG12) and increment of xenophagy protein expression (LC3) (Schwanhäusser et al., 2011). MiR-30b-5p was proven to be a nuclear miRNA that can transfer into the nucleus and inhibit the function of TFEB (Guo et al., 2020). TFEB is the master regulator of autophagy and lysosomal biogenesis and transfers to the nucleus to modulate the autophagic flux (Yang et al., 2020). Our data suggest that the nuclear translocation of TFEB occurs during H. pylori infection, which contributes to the activation of xenophagy. However, the role of TFEB in lysosome modulation could be partly blocked by the upregulation of nuclear miR-30b-5p induced by H. pylori, which is one of the strategies for its intracellular survival. Rapamycin activates lysosome function by TRP channel, but not TFEB, which is consistent with our result (Zhang et al., 2019b). Collectively, the elimination of lysosome activity by H. pylori could be attributed to two factors. On the one hand, H. pylori urease converts urea into ammonium ions, which raises the pH of the intracellular environment and impairs lysosome function (Schwartz and Allen, 2006). On the other hand, the upregulation of nuclear miR-30b-5p blocks the activity of TFEB and inhibits lysosome function. Although no effect of PA on TFEB translocation was shown, the downregulation of miR-30b-5p may contribute to the improvement of lysosome function in the studied system.
PA, from P. cablin (Blanco) Benth (Labiatae), is a naturally occurring tricyclic sesquiterpene characterized with high absorptivity and membrane permeability. The compound also shows high cellular uptake and transport efficiency and has been demonstrated to exert therapeutic effects against H. pylori-induced gastritis (Lian et al., 2018). The high cell-membrane permeability of PA renders it as an effective agent against intracellular H. pylori. Our data revealed the bactericidal effects of PA on intracellular H. pylori via the ability of improving miR-30b/c-mediated xenophagy and TFEB-related lysosome function. This study provides an extensive assessment of the multiple therapeutic effects of PA, which may present synergistic effects in combination with other antibiotics against H. pylori, especially highly invasive intracellular strains. The results reflect the potential use of PA as a complementary therapy against this highly pathogenic bacterium.
Conclusion
The strong cell invasive ability is observed in some clinical H. pylori strain, and the eradication of strongly invasive H. pylori should receive attention during clinical treatment. The relationship between clinical outcome and the cell invasive of H. pylori is still indistinct, which should be further illustrated based on clinical research. Moreover, PA can act against invasive H. pylori based on the improvement of miR-30b/c mediated xenophagy (Figure 9).
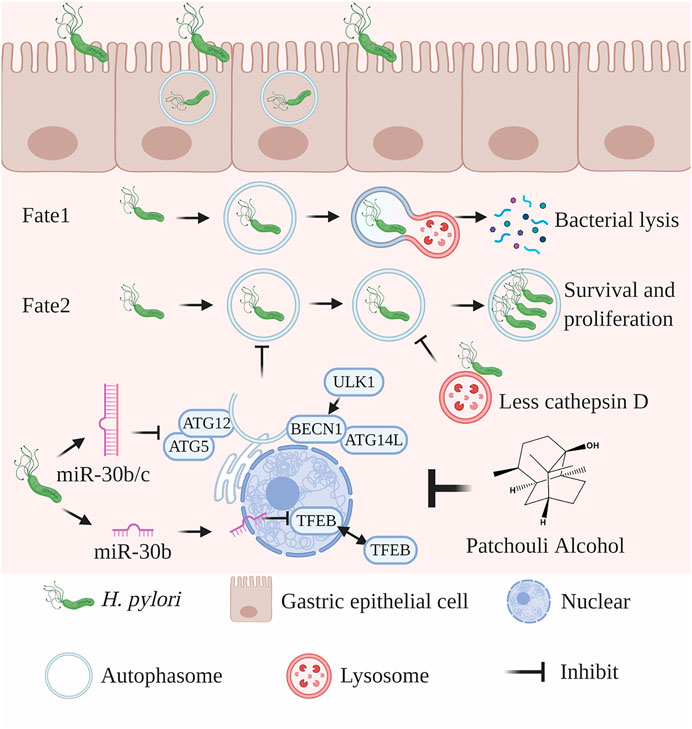
FIGURE 9. Proposed mechanism of PA against intracellular H. pylori. Some H. pylori bacteria could invade gastric epithelial cells. In the early stages of infection, H. pylori is enveloped by autophagosomes, which then fuse with lysosomes to form autolysosomes and digest the bacteria. As infection progresses, intracellular H. pylori secretes several toxic factors, such as VacA, to inhibit the xenophagic flux. The expression of miR-30c-3p, miR-30c-5p, and miR-30b-5p is typically upregulated by H. pylori infection and results in the downregulation of ULK1, ATG5, ATG12, and ATG14 and inhibition of the formation of autophagosomes. MiR-30c-5p could be transferred to the nucleus to inhibit the functions of TFEB and lysosomes. PA upregulates the expression of miR-30c-3p, miR-30c-5p, and miR-30b-5p to promote the xenophagic flux and lysosome function. The figure was created using BioRender.com supported by the Postdoc plan.
Data Availability Statement
The raw data supporting the conclusions of this article will be made available by the authors, without undue reservation, to any qualified researcher.
Author Contributions
YX, QD, and YZ performed the experiments and wrote manuscript. QD and SG summarized and analyzed the data. HY, LJ and JL revised the manuscript. HL carried out the anti-intracellular H. pylori assay. HP, HC, PH, and BH designed the study and finally revised the manuscript.
Funding
This research was supported by National Natural Science Foundation of China (81873074); Guangdong Basic and Applied Basic Research Foundation (2019A1515110742, 2020A1515110947); The Guangdong Provincial Key Research and development plan (2020B1111100011); Shenzhen Science and Technology Plan Project (JCYJ20170818094033689).
Conflict of Interest
The authors declare that the research was conducted in the absence of any commercial or financial relationships that could be construed as a potential conflict of interest.
Supplementary Material
The Supplementary Material for this article can be found online at: https://www.frontiersin.org/articles/10.3389/fphar.2021.666903/full#supplementary-material
References
Capurro, M. I., Prashar, A., and Jones, N. L. (2020). MCOLN1/TRPML1 Inhibition - a Novel Strategy Used by Helicobacter pylori to Escape Autophagic Killing and Antibiotic Eradication Therapy In Vivo. Autophagy 16, 169–170. doi:10.1080/15548627.2019.1677322
Chauhan, N., Tay, A. C. Y., Marshall, B. J., and Jain, U. (2019). Helicobacter pylori VacA, a Distinct Toxin Exerts Diverse Functionalities in Numerous Cells: An Overview. Helicobacter 24, e12544. doi:10.1111/hel.12544
Chu, Y.-T., Wang, Y.-H., Wu, J.-J., and Lei, H.-Y. (2010). Invasion and Multiplication of Helicobacter pylori in Gastric Epithelial Cells and Implications for Antibiotic Resistance. Infect. Immun. 78, 4157–4165. doi:10.1128/iai.00524-10
Ferrero, R. L., Wilson, J. E., and Sutton, P. (2012). Mouse Models of Helicobacter-Induced Gastric Cancer: Use of Cocarcinogens. Methods Mol. Biol. 921, 157–173. doi:10.1007/978-1-62703-005-2_20
Greenfield, L. K., and Jones, N. L. (2013). Modulation of Autophagy by Helicobacter pylori and its Role in Gastric Carcinogenesis. Trends Microbiol. 21, 602–612. doi:10.1016/j.tim.2013.09.004
Guo, H., Pu, M., Tai, Y., Chen, Y., Lu, H., Qiao, J., et al. (2020). Nuclear miR-30b-5p Suppresses TFEB-Mediated Lysosomal Biogenesis and Autophagy. Cell Death Differ 28 (1), 320–336. doi:10.1038/s41418-020-0602-4
Kwok, T., Backert, S., Schwarz, H., Berger, J., and Meyer, T. F. (2002). Specific Entry of Helicobacter pylori into Cultured Gastric Epithelial Cells via a Zipper-like Mechanism. Infect. Immun. 70, 2108–2120. doi:10.1128/iai.70.4.2108-2120.2002
Lai, C.-H., Kuo, C.-H., Chen, P.-Y., Poon, S.-K., Chang, C.-S., and Wang, W.-C. (2006). Association of Antibiotic Resistance and Higher Internalization Activity in Resistant Helicobacter pylori Isolates. J. Antimicrob. Chemother. 57, 466–471. doi:10.1093/jac/dki479
Lertsethtakarn, P., Ottemann, K. M., and Hendrixson, D. R. (2011). Motility and Chemotaxis inCampylobacterandHelicobacter. Annu. Rev. Microbiol. 65, 389–410. doi:10.1146/annurev-micro-090110-102908
Lian, D. W., Xu, Y. F., Ren, W. K., Fu, L. J., Chen, F. J., Tang, L. Y., et al. (2018). Unraveling the Novel Protective Effect of Patchouli Alcohol against Helicobacter Pylori-Induced Gastritis: Insights into the Molecular Mechanism In Vitro and In Vivo. Front. Pharmacol. 9, 1347. doi:10.3389/fphar.2018.01347
Lian, D., Xu, Y., Deng, Q., Lin, X., Huang, B., Xian, S., et al. (2019). Effect of Patchouli Alcohol on Macrophage Mediated Helicobacter pylori Digestion Based on Intracellular Urease Inhibition. Phytomedicine 65, 153097. doi:10.1016/j.phymed.2019.153097
Liao, J., Liang, Y., Chen, Y., Xie, J., Liu, W., Chen, J., et al. (2015). Novel Patchouli Alcohol Ternary Solid Dispersion Pellets Prepared by Poloxamers. Iran J. Pharm. Res. 14, 15–26.
Liao, W. C., Huang, M. Z., Wang, M. L., Lin, C. J., Lu, T. L., Lo, H. R., et al. (2016). Statin Decreases Helicobacter pylori Burden in Macrophages by Promoting Autophagy. Front Cel Infect Microbiol 6, 203. doi:10.3389/fcimb.2016.00203
Liu, D. S., Wang, Y. H., Zeng, Z. R., Zhang, Z. Y., Lu, H., Xu, J. M., et al. (2018). Primary Antibiotic Resistance of Helicobacter pylori in Chinese Patients: a Multiregion Prospective 7-year Study. Clin. Microbiol. Infect. 24, 780.e5–780.e8. doi:10.1016/j.cmi.2017.11.010
Lyu, T., Cheung, K. S., Ni, L., Guo, J., Mu, P., Li, Y., et al. (2020). High Prevalence and Risk Factors of Multiple Antibiotic Resistance in Patients Who Fail First-Line Helicobacter pylori Therapy in Southern China: a Municipality-wide, Multicentre, Prospective Cohort Study. J. Antimicrob. Chemother. 75, 3391–3394. doi:10.1093/jac/dkaa315
Necchi, V., Candusso, M. E., Tava, F., Luinetti, O., Ventura, U., Fiocca, R., et al. (2007). Intracellular, Intercellular, and Stromal Invasion of Gastric Mucosa, Preneoplastic Lesions, and Cancer by Helicobacter pylori. Gastroenterology 132, 1009–1023. doi:10.1053/j.gastro.2007.01.049
Qing, S. Z., Li, W. X., Jia, B. M., Wen, L. C., Zhi, K. S., Ren, S. Z., et al. (2014). Isolation of (-)-patchouli Alcohol from Patchouli Oil by Fractional Distillation and Crystallization. Trop. J. Pharm. Res. 13, 359–363. doi:10.4314/tjpr.v13i3.7
Ren, W.-K., Xu, Y.-F., Wei, W.-H., Huang, P., Lian, D.-W., Fu, L.-J., et al. (2019). Effect of Patchouli Alcohol on Helicobacter Pylori-Induced Neutrophil Recruitment and Activation. Int. Immunopharmacol. 68, 7–16. doi:10.1016/j.intimp.2018.12.044
Schwanhäusser, B., Busse, D., Li, N., Dittmar, G., Schuchhardt, J., Wolf, J., et al. (2011). Global Quantification of Mammalian Gene Expression Control. Nature 473, 337–342. doi:10.1038/nature10098
Schwartz, J. T., and Allen, L. A. (2006). Role of Urease in Megasome Formation and Helicobacter pylori Survival in Macrophages. J. Leukoc. Biol. 79, 1214–1225. doi:10.1189/jlb.0106030
Sharma, V., Verma, S., Seranova, E., Sarkar, S., and Kumar, D. (2018). Selective Autophagy and Xenophagy in Infection and Disease. Front Cel Dev Biol 6, 147. doi:10.3389/fcell.2018.00147
Sit, W. Y., Chen, Y.-A., Chen, Y.-L., Lai, C.-H., and Wang, W.-C. (2020). Cellular Evasion Strategies of Helicobacter pylori in Regulating its Intracellular Fate. Semin. Cel Dev. Biol. 101, 59–67. doi:10.1016/j.semcdb.2020.01.007
Sui, X., Liang, X., Chen, L., Guo, C., Han, W., Pan, H., et al. (2017). Bacterial Xenophagy and its Possible Role in Cancer: A Potential Antimicrobial Strategy for Cancer Prevention and Treatment. Autophagy 13, 237–247. doi:10.1080/15548627.2016.1252890
Tang, B., Li, N., Gu, J., Zhuang, Y., Li, Q., Wang, H.-G., et al. (2012). Compromised Autophagy byMIR30Bbenefits the Intracellular Survival ofHelicobacter Pylori. Autophagy 8, 1045–1057. doi:10.4161/auto.20159
Thakur, A., Mikkelsen, H., and Jungersen, G. (2019). Intracellular Pathogens: Host Immunity and Microbial Persistence Strategies. J. Immunol. Res. 2019, 1356540. doi:10.1155/2019/1356540
Xu, Y. F., Lian, D. W., Chen, Y. Q., Cai, Y. F., Zheng, Y. F., Fan, P. L., et al. (2017). In Vitro and In Vivo Antibacterial Activities of Patchouli Alcohol, a Naturally Occurring Tricyclic Sesquiterpene, against Helicobacter pylori Infection. Antimicrob. Agents Chemother. 61 (6), e00122–17. doi:10.1128/aac.00122-17
Yang, C., Zhu, Z., Tong, B. C.-K., Iyaswamy, A., Xie, W.-J., Zhu, Y., et al. (2020). A Stress Response P38 MAP Kinase Inhibitor SB202190 Promoted TFEB/TFE3-dependent Autophagy and Lysosomal Biogenesis Independent of P38. Redox Biol. 32, 101445. doi:10.1016/j.redox.2020.101445
Zhang, L., Sung, J. J., Yu, J., Ng, S. C., Wong, S. H., Cho, C. H., et al. (2014). Xenophagy in Helicobacter pylori - and Epstein-Barr Virus-Induced Gastric Cancer. J. Pathol. 233, 103–112. doi:10.1002/path.4351
Zhang, F., Chen, C., Hu, J., Su, R., Zhang, J., Han, Z., et al. (2019a). Molecular Mechanism of Helicobacter Pylori-Induced Autophagy in Gastric Cancer. Oncol. Lett. 18, 6221–6227. doi:10.3892/ol.2019.10976
Zhang, X., Chen, W., Gao, Q., Yang, J., Yan, X., Zhao, H., et al. (2019b). Rapamycin Directly Activates Lysosomal Mucolipin TRP Channels Independent of mTOR. Plos Biol. 17, e3000252. doi:10.1371/journal.pbio.3000252
Keywords: helicobacer pylori, patchouli alcohol, xenophagy, Intracellular, drug resistance, miR-30
Citation: Xu Y, Deng Q, Zhong Y, Jing L, Li H, Li J, Yu H, Pan H, Guo S, Cao H, Huang P and Huang B (2021) Clinical Strains of Helicobacter pylori With Strong Cell Invasiveness and the Protective Effect of Patchouli Alcohol by Improving miR-30b/C Mediated Xenophagy. Front. Pharmacol. 12:666903. doi: 10.3389/fphar.2021.666903
Received: 12 February 2021; Accepted: 19 April 2021;
Published: 30 April 2021.
Edited by:
Felipe Passero, São Paulo State University, BrazilReviewed by:
Jacek Baj, Medical University of Lublin, PolandAbbas Yadegar, Shahid Beheshti University of Medical Sciences, Iran
Paweł Krzyżek, Wroclaw Medical University, Poland
Copyright © 2021 Xu, Deng, Zhong, Jing, Li, Li, Yu, Pan, Guo, Cao, Huang and Huang. This is an open-access article distributed under the terms of the Creative Commons Attribution License (CC BY). The use, distribution or reproduction in other forums is permitted, provided the original author(s) and the copyright owner(s) are credited and that the original publication in this journal is cited, in accordance with accepted academic practice. No use, distribution or reproduction is permitted which does not comply with these terms.
*Correspondence: Yifei Xu, MzMwODUxNjM5QHFxLmNvbQ==; Ping Huang, aHBpbmdAZ3p1Y20uZWR1LmNu; Bin Huang, U1pUQ01odWFuZ2JpbkAxNjMuY29t; Hongying Cao, aHljYW9AZ3p1Y20uZWR1LmNu
†These authors have contributed equally to this work