- 1Laboratory of Functional and Structural Biology, Federal University of Pará, Belém, Brazil
- 2Laboratory of Cell Culture and Cytogenetics, Environment Section, Evandro Chagas Institute, Ananindeua, Brazil
- 3Regional Blood Center at University Hospital of the Ribeirão Preto Medical School of University of São Paulo, Ribeirão Preto, Brazil
- 4School of Dentistry, Federal University of Pará, Belém, Brazil
In Brazilian northern Amazon, communities are potentially exposed and vulnerable to methylmercury (MeHg) toxicity through the vast ingestion of fish. In vivo and in vitro studies demonstrated that the salivary glands as a susceptible organ to this potent environmental pollutant, reporting alterations on physiological, biochemical, and proteomic parameters. However, the alterations caused by MeHg on the gene expression of the exposed human salivary gland cells are still unknown. Therefore, the goal was to perform the transcriptome profile of the human salivary gland cell line after exposure to MeHg, using the microarray technique and posterior bioinformatics analysis. The cell exposure was performed using 2.5 µM MeHg. A previously published study demonstrated that this concentration belongs to a range of concentrations that caused biochemical and metabolic alterations in this linage. As a result, the MeHg exposure did not cause lethality in the human salivary gland cells line but was able to alter the expression of 155 genes. Downregulated genes (15) are entirety relating to the cell metabolism impairment, and according to KEGG analysis, they belong to the glycosphingolipid (GSL) biosynthesis pathway. On the other hand, most of the 140 upregulated genes were related to cell-cycle progression, DNA repair, and replication pathway, or cellular defenses through the GSH basal metabolism. These genomic changes revealed the effort to the cell to maintain physiological and genomic stability to avoid cell death, being in accordance with the nonlethality in the toxicity test. Last, the results support in-depth studies on nonlethal MeHg concentrations for biomarkers identification that interpret transcriptomics data in toxicological tests serving as an early alert of physiological changes in vitro biological models.
Introduction
Methylmercury (MeHg) is an organic form of mercury considered a potent environmental pollutant (Clarkson, 2002). In Brazilian northern Amazon, the artisanal small-scale gold mining (ASGM) is the main source of anthropogenic mercury emissions and contamination (Hacon et al., 2020). Communities whose diet involves vast ingestion of fish are potentially exposed and vulnerable to MeHg toxicity, which endangers the food security and livelihoods of traditional communities (Crespo-López et al., 2009; Crespo-Lopez et al., 2016; Hacon et al., 2020). Contaminated seafood is widely absorbed in the gastrointestinal tract and ubiquitously distributed to the body with an extensive effect in several organ systems (Kershaw et al., 1980). The central nervous system (CNS) is considered the principal target of MeHg due to the high affinity of this metal to the brain (Antunes Dos Santos et al., 2016); however, in vivo and in vitro studies have demonstrated that the salivary glands and cells from the oral cavity are also susceptible to MeHg toxicity (Bittencourt et al., 2017; Farias-Junior et al., 2017; Nogueira et al., 2019; Nascimento et al., 2020; Nogueira et al., 2021).
The salivary glands are essential for oral cavity health, playing the direct impact on the salivary production and also an important role as a diagnostic window into human disease (Saitou et al., 2020). The sub-chronic (35 days) and chronic (60 days) exposure of rats to MeHg-generated oxidative imbalance in the parotid and submandibular glands, by the depression of total antioxidant capacity and increased lipid peroxidation (Bittencourt et al., 2017; Farias-Junior et al., 2017). Besides that, the MeHg was able to induce changes in the proteomic profile with impairments on structural components of the cytoskeleton, metabolic pathways, and oxidative parameters (Bittencourt et al., 2017).
Through in vitro experiments, the human salivary gland cell line exposed to MeHg had compromised their metabolism and oxidative balance (Nogueira et al., 2021). A low dose of MeHg (0.25 µM) induced an increase in metabolism. A low dose of MeHg (0.25 µM) induced an increase in metabolism. This concentration represents the LC50 previously calculated in the toxicity tests performed with the human salivary gland cell line, and also represents the Hg accumulated in the parotid and submandibular glands of rats exposed chronically in vivo (Bittencourt et al., 2017; Nogueira et al., 2021). However, the in vitro exposure to a concentration 10-fold higher (2.5 mM) caused depression of cell metabolic status, generating an oxidative stress status by the depression of the GSH:GSSG rate and a significant increase of lipid peroxidation and protein carbonyl (Nogueira et al., 2021). Interestingly, although it is known that one of the exposure to MeHg causes DNA strand breaks (Crespo-López et al., 2007; Crespo-López et al., 2009; Antunes Dos Santos et al., 2018), this effect has not been observed in the exposed human salivary gland cell line to 2.5 µM MeHg.
To deepen the understanding of the mechanisms related to exposure of human salivary gland cells line to MeHg, the present study aimed to investigate the transcriptome profile of these cells after exposure to a nonlethal concentration of MeHg. The methodology performed was the microarray, which allowed the description in a genome-wide level of alterations in 26.000 genes through a full range of RNA molecules expressed by the cells.
Material and Methods
Cell Culture
The human salivary glands cells line, obtained from nonneoplastic cells isolated from the submandibular salivary gland and cultured in a Dulbecco’s modified Eagle’s medium (DMEM) and Ham’s F-12 nutrient medium (1:1), supplemented with 10% fetal bovine serum (FBS), 100 U/ml penicillin, and 100 μg/ml streptomycin, incubated at 37°C in a 5% CO2 environment. The medium was changed every 48 h. When cells became fully confluent, they were detached from the flask using 0.25% trypsin solution, and seeded into new flasks.
MeHg Exposure
Cells were seeded in a concentration of 1 × 104 cells/well in a 96-well/plate and cultured in a fresh culture medium for 24 h. After this, the medium was replaced by the exposure solution containing MeHg with no FBS supplementation, plus a control treatment (no metal addition). We used a concentration of 2.5 µM MeHg, which represents 10-fold higher the mercury accumulation found in the parotid and submandibular glands of rats chronically in vivo exposed to this compound (∼0.06 ppm; Bittencourt et al., 2017). At the end of the exposure time (24 h), the medium was removed, and the cells were washed with the EDTA solution (10 mM) to remove possible loosely bound mercury of the cell surface. Following that, cells were detached using trypsin and centrifuged (1000 g, 3 min) to proceed with the further analysis. Figure 1 represents a general research design and scheme of this study.
Cell Viability
Percentage of viable cells from the total number of exposed cells was determined using the trypan Blue (0.04%) exclusion assay. The exclusion test is based on dye permeability in compromised cell membranes due the cell death. If cells have their metabolic integrity maintained, the dye is not permeable to their membrane. In this study, after 2.5 µM MeHg exposure, the exposed cells were re-suspended in a fresh culture medium, and 5 µL of this homogenate was added into 5 µL of trypan blue solution. Following that, the cells were counted under a light microscope using the Neubauer chamber (×200 magnifications). The results were expressed in percentage (%).
Gene Expression
RNA Extraction
The human salivary gland cell lines were exposed as described above. At the end of 24 h, the cells were detached, and the pellet resulted from centrifugation had their mRNA extraction performed according to the SV total RNA isolation system kit assay from Promega®. Cells were homogenized in RNA lysis buffer contained beta-mercaptoethanol and transferred into a new tube containing RNA dilution buffer. After being centrifuged, samples were transferred into a clean tube, and 95% ethanol was added. Next step, samples were transferred into a spin column and three steps of centrifugation (1 min) occurred. First, using the RNA wash solution, next DNAse stop solution, and finally RNA wash solution. The RNA extracted was eluted into an elution tube using 15 µL of nuclease-free water. The RNA quantification was performed using TapeStation 4200 (Agilent Technologies), and the A260/280 ration was analyzed by a Nanodrop ND-1000 UV-VIS 3.2.1 version. The purified RNA was stored at −80°C to further gene expression assay.
Gene Expression Analysis–Microarray
The microarray analysis was performed according to the “One-color microarrays-based gene expression analysis” (Agilent technologies, EUA) protocol. This protocol was applied in nonexposed (control) and 2.5 µM MeHg exposed cells. Briefly, the obtained RNA was used as a template to synthesize the first cDNA, using a reverse transcription assisted by T7 RNA polymerase. A cRNA was transcripted from the second cDNA strand. Following that, the 3-cyanine was incorportated in the cRNA, and purified with the aid of the RNeasy mini spin kit assay. At the end, the cRNA was quantified by spectrophotometry, when the A260/280 ration and concentration were also analyzed (Nanodrop ND-1000 UV-VIS 3.2.1 version). For the hybridization, fragmentation mix was added to the RNA, and incubated at 60°C for 30 min 25 µL of 2x-RPM hybridization buffer were added to each sample, at 4°C. Finally, 40 µL of each sample was added to the hybridization slide, and left in the hybridization oven for 17 h, 10 rpm and 65°C. After that, the slides were washed and scanned immediately on the microarray Scanner (Agilent, G4900DA). Data were extracted from the raw microarray image file using the software Feature Extraction v10.10 and analyzed by GeneSpring GX (Version 11.0) software.
Bioinformatic Analyses
Differentially expressed genes were identified based on an absolute log2 fold change level >1 and the p-value adjusted by FDR <0.05. Quality control, quantile normalization, and batch effects removal were performed using the limma package. The over-representation analysis for differently expressed genes of gene ontology (GO) terms or KEGG pathways were also performed using the limma package. Over-represented p-values were adjusted by the Bonferroni method, and only adjusted p-values < 0.05 were considered.
Results
Cytotoxicity
The cell viability of the human salivary gland cell line exposed to 2.5 µM MeHg indicated a value of 87.5 ± 3.8%, while the nonexposed cells a value of 93.5 ± 0.4% (control). According to the statistical analysis, there are no differences between groups, showing a nonlethal MeHg concentration for the exposed salivary gland cell line at least for the 24 h tested.
Modulation of Transcriptomic Profile by MeHg Exposure
The whole transcriptome profile of the exposed human salivary gland cell line showed modulation of 155 genes, in which 140 were found upregulated and 15 downregulated, as observed in Figures 2 and 3 (log2fold change >1 or < −1; adj. p-value < 0.01). In Table 1, we highlighted the top 10 ranked genes with the highest (upregulated), and lowest (downregulated) fold changes. Figure 2 represents the distribution of differentially expressed genes in a volcano plot.
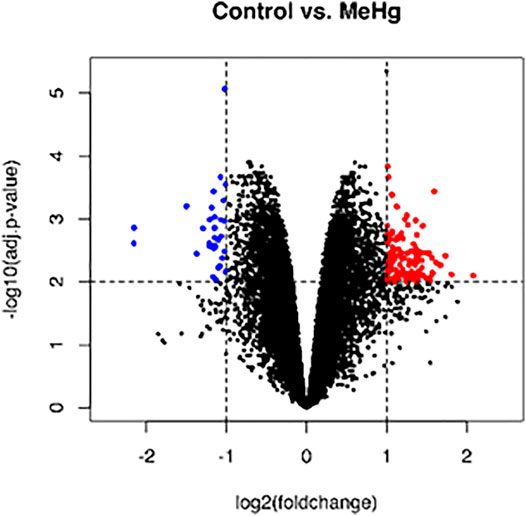
FIGURE 2. Global profile of gene expression of the exposed human salivary gland cells line to 2.5 µM of MeHg vs. nonexposed cells. The blue dots in the volcano plot represent downregulated genes, and red dots represent upregulated genes. Data were expressed as log2FC > 1 or < −1 and analyzed by t-Student test, adopting adj. p < 0.01 (n = 5/each group).
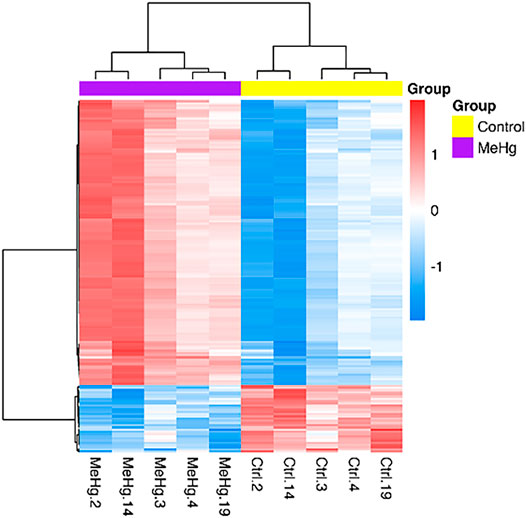
FIGURE 3. Differentially expressed genes in exposed human salivary gland cells line to 2.5 µM of MeHg vs. nonexposed cells (MeHg vs. Control) is demonstrated through the heat map. The dendrograms represent hierarchical cluster relationship between genes (left side) and among samples (upper), based on Pearson’s correlation; log2FC > 1 or < −1. log2fold change >1.5 or < −1.5; adj.; cutoff adj. p-value: < 0.01.
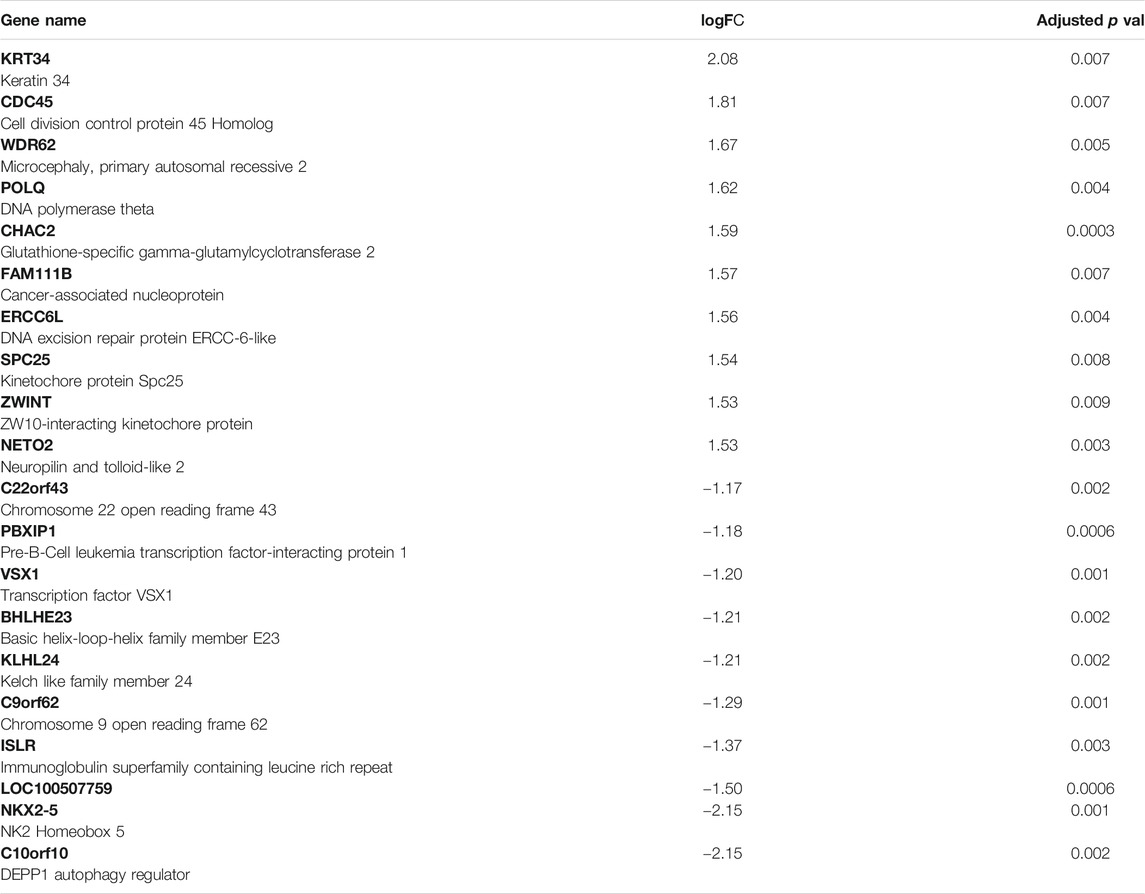
TABLE 1. Top 10 genes upregulated and top 10 downregulated in the exposed human salivary gland cells line to 2.5 µM of MeHg vs. nonexposed cells (MeHg vs. Control).
The genes modulated are involved in 14 different pathways, as observed in Figure 4. The genes are involved in pathways as Cell cycle, Oocyte meiosis, Progesterone-mediated oocyte maturation, Cellular senescence, Human T-cell leukemia virus one infection, and other nine pathways.
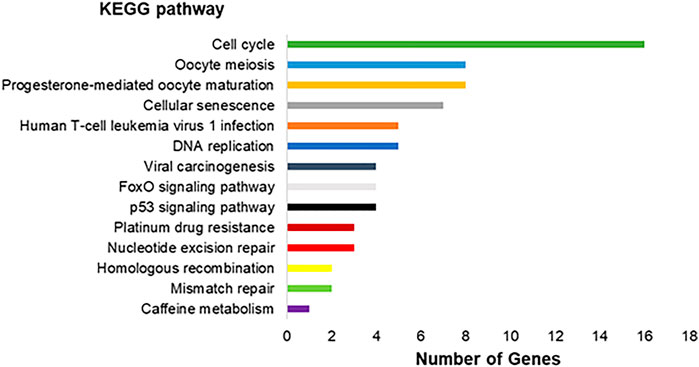
FIGURE 4. Molecular interaction, networks, and respective number of differentially expressed genes found in the transcriptomic analysis in the exposed human salivary gland cells line to 2.5 µM of MeHg vs. nonexposed cells (MeHg vs. Control) represented by KEGG pathways.
Analysis of Gene Ontology of the HSG Cells Transcriptome After the MeHg Exposure
The genes with expression significantly altered are involved in 672 different biological processes (p < 0.05). Among them, we highlighted 10 processes, as Biological Process, Biological Regulation, Regulation of Biological Processes, Nitrogen Compound Metabolic Process, Cellular Metabolic Process, and others five processes as shown in (Figure 5).
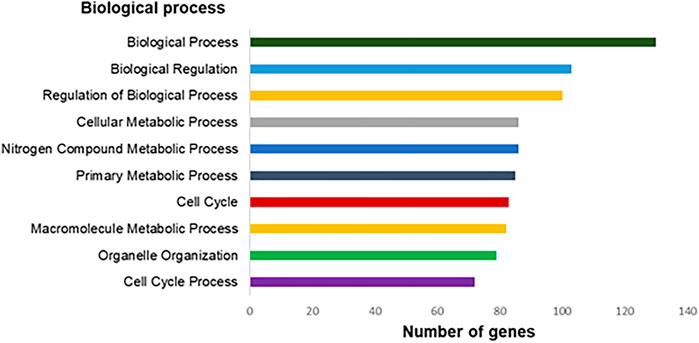
FIGURE 5. Top-ranking of biological processes based on Gene Ontology annotation (p < 0.05) related to differentially expressed genes in the exposed human salivary gland cells line to 2.5 µM of MeHg vs. nonexposed cells (MeHg vs. control).
Discussion
The human salivary gland cell line exposed to the nonlethal concentration of 2.5 µM MeHg revealed upregulated genes associated with pathways related to genomic stability such as DNA repair and replication, cell-cycle progression and cellular defenses (Table 2), while all downregulated genes were linked to the metabolic process. All these gene profile changes fully explain the metabolic and oxidative impairment in these cells exposed to MeHg (Nogueira et al., 2021), and suggest mechanisms related to the integrity of DNA through activation of DNA repair, integrity, and replication pathways.
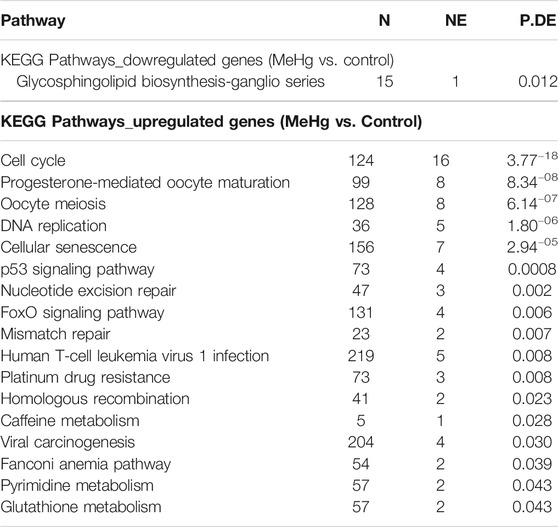
TABLE 2. Enrichment of pathways and ontologies in upregulated or downregulated in the exposed group of human salivary gland cells line to MeHg.
According to the enrichment of gene pathways and ontologies, all the 15 downregulated genes in human salivary gland cells exposed to MeHg are related to glycosphingolipid (GSLs) biosynthesis—ganglio series pathway. The GSLs are amphipathic molecules consisting of a ceramide lipid moiety linked to a glycan chain, in which gangliosides participate in many metabolic processes including growth, differentiation, migration, and apoptosis through modulating both cell signaling processes and cell-to-cell and cell-to-matrix interactions (Lopez and Schnaar, 2009), besides the immune system, cancer progression, and nervous system (Daniotti and Iglesias-Bartolomé, 2011). Alterations in energy availability trigger cell-cycle checkpoints, suggesting a bidirectional connection between cell division and general metabolism. In the exposed cells, cellular senescence pathway had a high number of altered genes. This pathway is important to limit the replication the replication in damaged cells (Van Nguyen et al., 2007; Herranz and Gil, 2018).
The second most downregulated gene was DEPP1, an autophagy regulator. The autophagy process reduces oxidative damage through self-degenerative mechanisms to remove misfolded proteins and damaged organelle, such as mitochondria (Salcher et al., 2017). Thus, the downregulation of DEPP1 may have collaborated to the oxidative imbalance detected in human salivary gland cells line after 2.5 µM MeHg exposure, occurring through the depletion of GSH:GSSG, and increasing of lipid peroxidation and protein carbonyl levels but no damage on DNA (Nogueira et al., 2021).
Although the human salivary gland cell lines had a significant decrease of GSH:GSSG ration after 2.5 µM MeHg exposure, there is an evidence of maintenance of basal glutathione. This is observed through the upregulated genes in the enrichment of gene pathways glutathione (GSH), the major thiol antioxidant, represents the key molecular targets involved in MeHg toxicity, responsible for detoxification, and/or metabolism of a significant number of potentially harmful molecules (Dringen et al., 2015). A recognized gene for maintaining GSH basal levels was upregulated in the exposed cells: ChaC glutathione specific gamma-glutamylcyclotransferase 2 (ChaC2). ChaC family proteins consist of two different branches represented by ChaC1 and ChaC2. While ChaC1 evolves high catalytic efficiency for carrying out an acute glutathione turnover under stress conditions (Kaur et al., 2017), the ChaC2 proteins are responsible for the continuous, but the basal, slow turnover of cytosolic glutathione, representing an additional pathway in the glutathione degradation (Kaur et al., 2017).
The absence of DNA alterations in exposed cells was elucidated by the transcriptome analysis performed here, revealing upregulated genes associated with pathways directly responsible for maintaining the DNA repair, integrity, and replication. The pathways were nucleotide excision repair, mismatch repair, homologous recombination, and DNA replication. Nucleotide excision repair removes bulky DNA lesions coordinated with other aspects of cell metabolism (Schärer, 2013), while the mismatch repair is a highly conserved biological pathway that plays a crucial role in maintaining genomic stability (Li, 2008). The homologous recombination repairs DNA double-stranded breaks (DSBs) and interstrand crosslinks (ICLs) damages, providing massive support for DNA replication in the recovery of stalled or broken replication forks, contributing to the tolerance of DNA damage (Li and Heyer, 2008). One of the important genes identified as upregulated in the exposed cells was the polymerase theta (POLQ). This gene is a widely conserved DNA polymerase and mediates a double-strand break (DSB) repair pathway (Feng et al., 2019).
Based on the KEGG pathway, the higher number of altered genes belongs to the cell cycle pathway. The cell cycle comprises a reproducible sequence of events, DNA replication (S phase), and mitosis (M phase) separated temporally by gaps in the G1 and G2 phases. The most overexpressed gene related to cell cycle arrest in the exposed cells was the cell division cycle 45 (CDC45). The CDC45 gene plays a critical role in DNA replication, assembling the replicative helicase Cdc45-MCM2-7-GINS (CMG), which forms in early S-phase (Speck, 2016). The arresting cell cycle progression responds to the cellular perception of extrinsic factors, such as oxidative stress (Van Nguyen et al., 2007; Herranz and Gil, 2018; Zhao et al., 2020), and is only released followed by complete DNA repair. Otherwise, cells with nonrepairable DNA damage undergo apoptosis (Martin et al., 2010). However, in the exposed human salivary gland cell line, it is possible to observe the upregulation of a gene associated to the apoptosis suppressor, the DNA damage-induced apoptosis suppressor (C11orf82). This gene also named DNA damage-induced apoptosis suppressor (DDIAS), negatively regulates any process of intrinsic apoptotic signaling pathways that shows in response to DNA damage.
Studies suggest that MeHg-induced cell cycle arrest occurs via both p53-dependent and p53-independent pathway manners, but cell death is highly dependent on p53 (Gribble et al., 2005). In exposed stem cells, lethal concentrations of MeHg (20–40 µM) plus an elevated nonlethal concentration (15 µM) actively regulated the p53 pathways (Waldmann et al., 2017). In this present study, although the p53 pathway was active in the exposed human salivary gland cell line, no change in the p53 gene expression profile was observed. However, although Waldman and coauthors (2017) associated p53 to apoptotic response, the p53 pathway is related to DNA repair, maintaining the dynamic equilibrium between cell growth and arrest in response to factors including DNA damage (Mandinova and Lee, 2011), mechanisms that directly agree with the results from exposed salivary gland cell line to MeHg.
The FoxO signaling pathway was also demonstrated after the enrichment of gene pathways and ontologies analysis. This pathway belongs to a family of transcription factors that are activated by oxidative stress signals and regulate cell proliferation and resistance to oxidative stress (Webb and Brunet, 2014). Among the overexpressed genes in the exposed salivary gland cell line, we can point out the forkhead box M. This gene belongs to FoxO signaling pathway and is responsible for protecting cells from the adverse effects of oxidative stress by upregulating the expression of scavenger enzymes. Besides that, this gene participates in the homologous recombination repair of DNA double-strand breaks, preventing polyploidy and aneuploidy (Wierstra, 2013).
Last, one of the main goals after analyzing the overall genes in a biological model is to identify possible candidates of cytotoxicity biomarkers that will better interpret transcriptomics data. A study published by Waldmann and coauthors (2017) identified genes in the stem cells related to lethal concentrations after two different compounds exposure, the MeHg and valproic acid. Comparing to our data, only one similar gene was found altered in the human salivary cells line after exposure to the MeHg, the gene Serpin family F Member 1 (SERPIN1). However, differently from Waldmann’s study, the SERPIN1 gene was downregulated in the present study. This absence of cytotoxic biomarker similarities was already expected since the MeHg concentration used here was nonlethal for cells, validating the Waldmann and coauthors cytotoxicity biomarkers list (2017).
Taking together, the results from the transcriptome profile and our previous study revealed physiological and genomic alterations caused by 2.5 µM MeHg in the human salivary gland cell line. Although nonlethal, this concentration was able to generate oxidative stress and induce different strategies to repair and maintain viable cells. The stress generated by the extrinsic factor (MeHg) triggers changes in gene pathways, directly and indirectly, related to DNA integrity and biochemical responses, such as GSH metabolism. Last, the absence of a common signature of gene expression changes for lethal concentrations of MeHg supports profound studies on gene identifications in nonlethal concentrations xenobiotics.
Data Availability Statement
The authors acknowledge that the data presented in this study must be deposited and made publicly available in an acceptable repository, prior to publication. The data presented in the study are deposited in the Gene Expression Omninus repository, accession number GSE182249.
Author Contributions
LN and RL: study concept and design. LN, CV, JP, LB, and MK: analysis and interpretation of data. LN, LB, and JP: preparation of manuscript. EO and RL: critical revision of manuscript.
Funding
This work was supported by Brazilian National Council for Scientific and Technological Development (CNPq); Brazilian Government/Coordenação de Aperfeiçoamento de Pessoal de Nível Superior (CAPES)-Finance Code 001 and Programa Nacional de Pós Doutorado/Capes (PNPD/CAPES). The APC was funded by Pró-Reitoria de Pesquisa e Pós-graduação da Universidade Federal do Pará (PROPESP-UFPA).
Conflict of Interest
The authors declare that the research was conducted in the absence of any commercial or financial relationships that could be construed as a potential conflict of interest.
Publisher’s Note
All claims expressed in this article are solely those of the authors and do not necessarily represent those of their affiliated organizations, or those of the publisher, the editors and the reviewers. Any product that may be evaluated in this article, or claim that may be made by its manufacturer, is not guaranteed or endorsed by the publisher.
Acknowledgments
We thanks to Pró-Reitoria de Pesquisa e Pós-Graduação of Federal University of Pará (PROPESP, UFPA, Brazil) and Brazilian National Council for Scientific and Technological Development (CNPq), Coordenação de Aperfeiçoamento de Pessoal de Nível Superior (CAPES), Institute Evandro Cahgas and Pró-Reitoria de Pesquisa e Pós-graduação da Universidade Federal do Pará (PROPESP-UFPA).
References
Antunes Dos Santos, A., Appel Hort, M., Culbreth, M., López-Granero, C., Farina, M., Rocha, J. B. T., et al. (2016). Methylmercury and Brain Development: A Review of Recent Literature. J. Trace Elem. Med. Biol. 38, 99–107. doi:10.1016/j.jtemb.2016.03.001
Antunes Dos Santos, A., Ferrer, B., Marques Gonçalves, F., Tsatsakis, A., Renieri, E., Skalny, A., et al. (2018). Oxidative Stress in Methylmercury-Induced Cell Toxicity. Toxics 6, 47. doi:10.3390/toxics6030047
Bittencourt, L. O., Puty, B., Charone, S., Aragão, W. A. B., Farias-Junior, P. M., Silva, M. C. F., et al. (2017). Oxidative Biochemistry Disbalance and Changes on Proteomic Profile in Salivary Glands of Rats Induced by Chronic Exposure to Methylmercury. Oxid. Med. Cell Longev. 2017, 5653291. doi:10.1155/2017/5653291
Clarkson, T. W. (2002). The Three Modern Faces of Mercury. Environ. Health Perspect. 110 (Suppl. 1), 11–23. doi:10.1289/ehp.02110s111
Crespo-López, M. E., Lima de Sá, A., Herculano, A. M., Rodríguez Burbano, R., and Martins do Nascimento, J. L. (2007). Methylmercury Genotoxicity: a Novel Effect in Human Cell Lines of the central Nervous System. Environ. Int. 33, 141–146. doi:10.1016/j.envint.2006.08.005
Crespo-López, M. E., Macêdo, G. L., Pereira, S. I. D., Arrifano, G. P. F., Picanço-Diniz, D. L. W., Nascimento, J. L. M. d., et al. (2009). Mercury and Human Genotoxicity: Critical Considerations and Possible Molecular Mechanisms. Pharmacol. Res. 60, 212–220. doi:10.1016/j.phrs.2009.02.011
Crespo-Lopez, M. E., Costa-Malaquias, A., Oliveira, E. H. C., Miranda, M. S., Arrifano, G. P. F., Souza-Monteiro, J. R., et al. (2016). Is Low Non-lethal Concentration of Methylmercury Really Safe? A Report on Genotoxicity with Delayed Cell Proliferation. PLoS One 11, e0162822. doi:10.1371/journal.pone.0162822
Daniotti, J. L., and Iglesias-Bartolomé, R. (2011). Metabolic Pathways and Intracellular Trafficking of Gangliosides. IUBMB Life 63, 513–520. doi:10.1002/iub.477
Dringen, R., Brandmann, M., Hohnholt, M. C., and Blumrich, E. M. (2015). Glutathione-Dependent Detoxification Processes in Astrocytes. Neurochem Res. 40, 2570–2582. doi:10.1007/s11064-014-1481-1
Farias-Junior, P. M. A., Teixeira, F. B., Fagundes, N. C. F., Miranda, G. H. N., Oliveira Bittencourt, L., de Oliveira Paraense, R. S., et al. (2017). Chronic Intoxication by Methylmercury Leads to Oxidative Damage and Cell Death in Salivary Glands of Rats. Metallomics 9, 1778–1785. doi:10.1039/c7mt00168a
Feng, W., Simpson, D. A., Carvajal-Garcia, J., Price, B. A., Kumar, R. J., Mose, L. E., et al. (2019). Genetic Determinants of Cellular Addiction to DNA Polymerase Theta. Nat. Commun. 10, 4286. doi:10.1038/s41467-019-12234-1
Gribble, E. J., Hong, S.-W., and Faustman, E. M. (2005). The Magnitude of Methylmercury-Induced Cytotoxicity and Cell Cycle Arrest Is P53-dependent. Birth Defect Res. A. 73, 29–38. doi:10.1002/bdra.20104
Hacon, S. d. S., Oliveira-da-Costa, M., Gama, C. d. S., Ferreira, R., Basta, P. C., Schramm, A., et al. (2020). Mercury Exposure through Fish Consumption in Traditional Communities in the Brazilian Northern Amazon. Ijerph 17, 5269. doi:10.3390/ijerph17155269
Herranz, N., and Gil, J. (2018). Mechanisms and Functions of Cellular Senescence. J. Clin. Invest. 128, 1238–1246. doi:10.1172/JCI95148
Kaur, A., Gautam, R., Srivastava, R., Chandel, A., Kumar, A., Karthikeyan, S., et al. (2017). ChaC2, an Enzyme for Slow Turnover of Cytosolic Glutathione. J. Biol. Chem. 292, 638–651. doi:10.1074/jbc.M116.727479
Kershaw, T. G., Dhahir, P. H., and Clarkson, T. W. (1980). The Relationship between Blood Levels and Dose of Methylmercury in Man. Arch. Environ. Health Int. J. 35, 28–36. doi:10.1080/00039896.1980.10667458
Li, X., and Heyer, W.-D. (2008). Homologous Recombination in DNA Repair and DNA Damage Tolerance. Cel Res 18, 99–113. doi:10.1038/cr.2008.1
Li, G.-M. (2008). Mechanisms and Functions of DNA Mismatch Repair. Cell Res 18, 85–98. doi:10.1038/cr.2007.115
Lopez, P. H., and Schnaar, R. L. (2009). Gangliosides in Cell Recognition and Membrane Protein Regulation. Curr. Opin. Struct. Biol. 19, 549–557. doi:10.1016/j.sbi.2009.06.001
Mandinova, A., and Lee, S. W. (2011). The P53 Pathway as A Target In Cancer Therapeutics: Obstacles And Promise. Sci. Transl. Med. 3, 64rv1. doi:10.1126/scitranslmed.3001366
Martin, L. M., Marples, B., Coffey, M., Lawler, M., Lynch, T. H., Hollywood, D., et al. (2010). DNA Mismatch Repair and the DNA Damage Response to Ionizing Radiation: Making Sense of Apparently Conflicting Data. Cancer Treat. Rev. 36, 518–527. doi:10.1016/j.ctrv.2010.03.008
Nascimento, P. C., Ferreira, M. K. M., Balbinot, K. M., Alves-Júnior, S. M., Viana Pinheiro, J. d. J., Silveira, F. M., et al. (2020). Methylmercury-Induced Toxicopathologic Findings in Salivary Glands of Offspring Rats after Gestational and Lactational Exposure. Biol. Trace Elem. Res. 199, 2983–2991. doi:10.1007/s12011-020-02409-z
Nogueira, L. S., Vasconcelos, C. P., Mitre, G. P., da Silva Kataoka, M. S., Lima, M. O., de Oliveira, E. H. C., et al. (2019). Oxidative Damage in Human Periodontal Ligament Fibroblast (hPLF) after Methylmercury Exposure. Oxid. Med. Cell Longev. 2019, 1–7. doi:10.1155/2019/8470857
Nogueira, L. S., Vasconcelos, C. P., Mitre, G. P., Kataoka, M. S. d. S., Bittencourt, L. O., Lima, M. O., et al. (2021). Metabolic and Oxidative Impairments in Human Salivary Gland Cells Line Exposed to MeHg. J. Trace Elem. Med. Biol. 66, 126747. doi:10.1016/j.jtemb.2021.126747
Saitou, M., Gaylord, E., Xu, E., May, A., Neznanova, L., Nathan, S., et al. (2020). Functional Specialization of Human Salivary Glands and Origins of Proteins Intrinsic to Human Saliva. Cell Rep. 33, 108402. doi:10.1016/j.celrep.2020.108402
Salcher, S., Hermann, M., Kiechl-Kohlendorfer, U., Ausserlechner, M. J., and Obexer, P. (2017). C10ORF10/DEPP-mediated ROS Accumulation Is a Critical Modulator of FOXO3-Induced Autophagy. Mol. Cancer 16, 95. doi:10.1186/s12943-017-0661-4
Schärer, O. D. (2013). Nucleotide Excision Repair in Eukaryotes. Cold Spring Harbor Perspect. Biol. 5, a012609. doi:10.1101/cshperspect.a012609
Speck, C. (2016). Exceeding the Limits - Cdc45 Overexpression Turns Bad. Cell Cycle 15, 1809–1810. doi:10.1080/15384101.2016.1184512
Van Nguyen, T., Puebla-Osorio, N., Pang, H., Dujka, M. E., and Zhu, C. (2007). DNA Damage-Induced Cellular Senescence Is Sufficient to Suppress Tumorigenesis: a Mouse Model. J. Exp. Med. 204, 1453–1461. doi:10.1084/jem.20062453
Waldmann, T., Grinberg, M., König, A., Rempel, E., Schildknecht, S., Henry, M., et al. (2017). Stem Cell Transcriptome Responses and Corresponding Biomarkers That Indicate the Transition from Adaptive Responses to Cytotoxicity. Chem. Res. Toxicol. 30, 905–922. doi:10.1021/acs.chemrestox.6b00259
Webb, A. E., and Brunet, A. (2014). FOXO Transcription Factors: Key Regulators of Cellular Quality Control. Trends Biochem. Sci. 39, 159–169. doi:10.1016/j.tibs.2014.02.003
Wierstra, I. (2013). FOXM1 (Forkhead Box M1) in Tumorigenesis. Adv. Cancer Res. 119, 191–419. doi:10.1016/B978-0-12-407190-2.00016-2
Keywords: toxicology, salivary gland, transcriptome, methylmercury, biomarker
Citation: Nogueira LS, Vasconcelos CP, Plaça JR, Mitre GP, Bittencourt LO, Kataoka MSdS, de Oliveira EHC and Lima RR (2021) Non-Lethal Concentration of MeHg Causes Marked Responses in the DNA Repair, Integrity, and Replication Pathways in the Exposed Human Salivary Gland Cell Line. Front. Pharmacol. 12:698671. doi: 10.3389/fphar.2021.698671
Received: 21 April 2021; Accepted: 07 July 2021;
Published: 25 August 2021.
Edited by:
Fatma Mohamady El-Demerdash, Alexandria University, EgyptReviewed by:
Fayed Megahed, City of Scientific Research and Technological Applications, EgyptAlex Boye, University of Cape Coast, Ghana
Copyright © 2021 Nogueira, Vasconcelos, Plaça, Mitre, Bittencourt, Kataoka, de Oliveira and Lima. This is an open-access article distributed under the terms of the Creative Commons Attribution License (CC BY). The use, distribution or reproduction in other forums is permitted, provided the original author(s) and the copyright owner(s) are credited and that the original publication in this journal is cited, in accordance with accepted academic practice. No use, distribution or reproduction is permitted which does not comply with these terms.
*Correspondence: Rafael Rodrigues Lima, rafalima@ufpa.br