- 1Department of Drug and Health Sciences, University of Catania, Catania, Italy
- 2Oasi Research Institute—IRCCS, Troina, Italy
- 3Department of Biomedical and Biotechnological Sciences, University of Catania, Catania, Italy
- 4Center for Neuroscience and Neurotechnology, University of Modena and Reggio Emilia, Modena, Italy
- 5Department of Life Sciences, University of Modena and Reggio Emilia, Modena, Italy
Depression is a risk factor for the development of Alzheimer’s disease (AD). A neurobiological and clinical continuum exists between AD and depression, with neuroinflammation and oxidative stress being involved in both diseases. Second-generation antidepressants, in particular selective serotonin reuptake inhibitors (SSRIs), are currently investigated as neuroprotective drugs in AD. By employing a non-transgenic AD model, obtained by intracerebroventricular (i.c.v.) injection of amyloid-β (Aβ) oligomers in 2-month-old C57BL/6 mice, we recently demonstrated that the SSRI fluoxetine (FLX) and the multimodal antidepressant vortioxetine (VTX) reversed the depressive-like phenotype and memory deficits induced by Aβ oligomers rescuing the levels of transforming growth factor-β1 (TGF-β1). Aim of our study was to test FLX and VTX for their ability to prevent oxidative stress in the hippocampus of Aβ-injected mice, a brain area strongly affected in both depression and AD. The long-term intraperitoneal (i.p.) administration of FLX (10 mg/kg) or VTX (5 and 10 mg/kg) for 24 days, starting 7 days before Aβ injection, was able to prevent the over-expression of inducible nitric oxide synthase (iNOS) and NADPH oxidase 2 (Nox2) induced by Aβ oligomers. Antidepressant pre-treatment was also able to rescue the mRNA expression of glutathione peroxidase 1 (Gpx1) antioxidant enzyme. FLX and VTX also prevented Aβ-induced neurodegeneration in mixed neuronal cultures treated with Aβ oligomers. Our data represent the first evidence that the long-term treatment with the antidepressants FLX or VTX can prevent the oxidative stress phenomena related to the cognitive deficits and depressive-like phenotype observed in a non-transgenic animal model of AD.
Introduction
Alzheimer’s disease (AD) represents a type of dementia affecting memory, global cognitive function, and behavior, severe enough to interfere with activities of daily living (Kumar et al., 2021). This disease also presents neuropsychiatric symptoms, such as depression, along with neurodegeneration, neuroinflammation, and oxidative stress phenomena (Caruso et al., 2021). The latter occurs when the homeostatic equilibrium between pro-oxidants species and antioxidants is missing, with the pro-oxidants being in excess (Caruso et al., 2019a). With regard to depression, a neurobiological and clinical continuum has been demonstrated between this disease and AD (Caraci et al., 2018b). In fact, depression represents a risk factor for AD development, while the occurrence of depressive symptoms significantly increases the conversion from mild cognitive impairment (MCI) into AD (Petersen et al., 2014).
It is now well-known that amyloid-β (Aβ), the peptide involved in the pathogenesis of AD, can undergo aggregation, starting with soluble monomers and forming species characterized by higher molecular weight such as oligomers, protofibrils, and mature fibrils (Brorsson et al., 2010). Among the above species, oligomers represent the most toxic species of Aβ, leading to synaptic loss and neuronal death in AD brain (Klein, 2013). It has been shown that oxidative stress plays a crucial role in mediating the toxicity of Aβ oligomers; in fact, neurodegeneration and neuroinflammation as well as the impairment of synaptic plasticity are, at least in part, due to the oxidative stress Aβ oligomers-induced (Varadarajan et al., 2000; Gelain et al., 2012). Oxidative stress is able to promote Aβ oligomerization (Zhao and Zhao, 2013) and β- and γ-secretase activation, the two enzymes involved in the formation of the different Aβ species (Zhao and Zhao, 2013). Markers of oxidative stress have been found in AD animal models before plaques deposition as well as in brain, plasma, and erythrocytes from MCI and AD patients (Glenner and Wong, 1984; Minati et al., 2009), suggesting that redox imbalance and oxidative damage play a key role in an early stage of AD pathophysiology, as well as in the disease progression (Cheignon et al., 2018).
Different groups are currently studying antidepressants in AD (Lozupone et al., 2018). Second-generation antidepressants have been associated with a reduced risk of developing AD, but there are still no clear findings demonstrating the ability of these drugs to counteract the progression of this disease (Correia and Vale, 2021). Positive outcomes have been observed by using selective serotonin reuptake inhibitors (SSRIs) (Dafsari and Jessen, 2020). Long-term SSRI treatment (>4 years) was significantly associated with a delayed progression from MCI to AD (Bartels et al., 2018). The immune regulatory effect of antidepressants observed in depressed patients is attributable to their ability to decrease the levels of pro-inflammatory cytokines (e.g., tumor necrosis factor-α (TNF-α)) and increase those of anti-inflammatory cytokines, such as transforming growth factor-β1 (TGF-β1) (Szałach et al., 2019). Antidepressant drugs could also exert their therapeutic effect by suppressing the production of reactive oxygen and nitrogen species, ROS and RNS respectively, and/or rescuing the antioxidant defense (Behr et al., 2012; Wu et al., 2013). The SSRI fluoxetine (FLX) is able to revert the brain oxidative damage by reducing lipid peroxidation at hippocampal level, also increasing the activity of antioxidant enzymes, such as superoxide dismutase (SOD) and catalase (CAT), in different animal models of depression (Chung et al., 2010; Moretti et al., 2012). Evidence also exists that FLX prevents amyloid pathology, also reverting memory impairment in different animal models of AD (Wang et al., 2014; Jin et al., 2017). Furthermore, FLX exerts neuroprotection in an established in vitro model of Aβ-induced neurodegeneration via a paracrine signaling mediated by TGF-β1 (Caraci et al., 2016). Acute and long-term treatments with the new multimodal antidepressant vortioxetine (VTX) improve cognitive function in preclinical models of depression (Bennabi et al., 2019). This drug also exhibits an increased efficacy, compared to FLX, in aged mice in counteracting depressive-like behavior and memory deficits (Li et al., 2017; Bennabi et al., 2019). At clinical level, VTX has proven more effective than SSRIs in the treatment of specific clinical domains, such as cognitive deficits in elderly depressed patients (McIntyre et al., 2016; Thase et al., 2016), underlining its therapeutic potential for the treatment of cognitive impairment in depression (Bennabi et al., 2019). Interestingly, VTX exerts antioxidant activity and anti-inflammatory effects in human monocytes/macrophages stimulated with phorbol 12-myristate 13-acetate (PMA), also inducing the shift of macrophages from M1 (pro-inflammatory) to M2 (anti-inflammatory) phenotype (Talmon et al., 2018).
Intracerebroventricular (i.c.v.) injection of Aβ oligomers in mice has been used to obtain a non-transgenic (non-Tg) AD model characterized by memory deficits and depressive-like phenotype (Ledo et al., 2016; Ledo et al., 2020). An equivalent outcome has been observed in rats that underwent i.c.v. injection of Aβ oligomers (Colaianna et al., 2010; Schiavone et al., 2017). By using this non-Tg AD model, we have recently demonstrated that FLX or VTX revert the behavioral and memory alterations induced by Aβ oligomers (Torrisi et al., 2019). In the same study we also detected a significant reduction of the synaptic proteins synaptophysin and PSD-95 paralleled by a significant deficit of TGF-β1 at hippocampal level that was completely rescued by the long-term treatment with FLX or VTX.
Starting from these grounds, we hypothesized that the i.c.v. injection of Aβ oligomers could also induce oxidative stress in the hippocampus of our non-Tg model of AD and that a long-term treatment with FLX or VTX could prevent this phenomenon by regulating the subtle equilibrium between pro- and antioxidant factors.
Materials and Methods
Materials
All chemicals and reagents used in this study were of analytical grade and obtained from Sigma-Aldrich Inc. (St. Louis, MO, United States) or Thermo Fisher Scientific Inc. (Pittsburgh, PA, United States) unless specified otherwise.
Establishment of the Non-Tg AD Mouse Model
The cohorts of animals whose tissues were used for gene and protein analysis are the same described in Torrisi et al. (2019).
Eight-week-old male C57BL/6 mice, obtained from Envigo RMS s.r.l. laboratories (San Pietro al Natisone, Italy), were maintained and used as previously described (Torrisi et al., 2019), following procedures in accordance with the U.K. Animals (Scientific Procedures) Act, 1986 and associated guidelines, EU Directive 2010/63/EU for animal experiments.
As previously described, in order to obtain the non-Tg AD mouse model, 2 µL of the 10 μM Aβ oligomers solution (Bachem Distribution Services GmbH, Weil am Rhein, Germany), prepared according to the original protocol of Klein’s group (Gong et al., 2003), were i.c.v. injected by using a microsyringe with a 28-gauge stainless-steel needle 3.0-mm-long (Hamilton). The injection of this Aβ solution corresponds to 20 pmol of Aβ monomer equivalent, giving a final concentration of approximately 0.18 μg/g tissue.
Drug Treatment
Vortioxetine hydrobromide [purity > 98.0% (HPLC)] was obtained from H. Lundbeck A/S (Denmark) according to the MTA N.417394 signed by University of Catania (Department of Drug and Health Sciences) and H. Lundbeck A/S and Lundbeck Italia S.p.A. FLX and VTX were prepared and administered i.p. (FLX at 10 mg/kg; VTX at 5 or 10 mg/kg) daily for a total of 21 to 26 days starting from 7 days before Aβ i.c.v injection as previously described in details (Torrisi et al., 2019). Control animals received the vehicle i.p. A total of five groups of animals were employed in this study and are indicated as follows: 1) control group (phosphate-buffered saline [PBS + vehicle (VEH)]; 2) Aβ group (Aβ + VEH); 3) FLX10 group (Aβ + FLX 10 mg/kg); 4) VTX5 group (Aβ + VTX 5 mg/kg); 5) VTX10 group (Aβ + VTX 10 mg/kg). PBS and VEH were injected i.c.v. and i.p., respectively, while to test the drug activity per se, so in absence of Aβ, drugs were administered i.p. for a total of 21 days.
Gene Expression Analysis by Quantitative Real-Time PCR (qRT-PCR)
Gene expression analysis by qRT-PCR was carried out on hippocampal samples. The protocol employed for these experiments is the same previously described (Caruso et al., 2019c; Fidilio et al., 2021) with slight modifications. Briefly, NanoDrop® ND-1000 (Thermo Fisher Scientific, Waltham, MA, United States) was used to determine the RNA concentrations, while Qubit® 3.0 Fluorometer (Thermo Fisher Scientific) was employed to assess the quality of RNA (Fresta et al., 2020a). The reverse transcription was obtained by using the SuperScript III First-Strand Synthesis SuperMix kit. The quantification of all the cDNA samples obtained and loaded in a 384-well plate was measured through a LightCycler® 480 System (Roche Molecular Systems, Inc., Pleasanton, CA, United States). The information relative to the Quanti Tect Primer Assays (Qiagen, Hilden, Germany) used is reported in Table 1. Sample amplification, fluorescence data collection, and sample quantification is the same previously described elsewhere (Caruso et al., 2019c; Fidilio et al., 2021). The relative RNA expression level for each sample was calculated using the 2−ΔΔCT method in which the threshold cycle (CT) value of the target gene is compared to the CT value of the selected internal control (GAPDH gene in our case). The number of samples analyzed obtained by each animal group is indicated in the pertinent Figure legend.
Protein Expression Analysis by Western Blot (WB)
WB analysis was performed on hippocampal samples following the previously described procedure (Caraci et al., 2015). Briefly, once the protein concentration in tissue homogenate was determined (Pierce™ BCA protein assay kit), 30 µg of total proteins were denatured, separated by gel electrophoresis, and transferred to nitrocellulose membranes. The membranes were incubated overnight (4°C) with the following primary antibodies: rabbit anti-iNOS (Abcam ab136918, 1:1,000), rabbit anti-Nox2/gp91phox (Abcam ab80508, 1:4,000), rabbit anti-Gpx1 (Cell Signaling Technology 3206, 1:500), mouse anti-β-actin (Sigma Aldrich A4700, 1:1,000). Secondary goat anti-rabbit labeled with IRDye 800 (Li-COR Biosciences; 1:15,000) and goat anti-mouse labeled with IRDye 680 (Li-COR Biosciences; 1:15,000) were used at room temperature in the dark for 1 h after three washes in tris-buffered saline (TBS)/Tween 20× 0.1%. Hybridization signals were detected by the Odyssey Infrared Imaging System (LI-COR Biosciences) and the densitometry analysis was performed by using ImageJ software. The number of samples analyzed obtained by each animal group is indicated in the pertinent Figure legend.
Mixed Neuronal Cultures
Mixed neuronal cultures consisting of 35–40% neurons and 60–65% glial cells (astrocytes and microglia) were obtained from rats at embryonic day 15 (Harlan Laboratories, Italy) as previously described (Caraci et al., 2016). Cells were grown into DMEM/F12 (1:1) (American Type Culture Collection (ATCC), Manassas, VA, United States) supplemented with 10% horse serum, 10% fetal calf serum, 2 mM glutamine, and 6 mg/ml glucose. After 7–10 days in vitro, to avoid the proliferation of non-neuronal elements, cytosine-D-arabinoside (10 µM) was added, for a total of 3 days. Cells were then moved into a maintenance medium in absence of serum. Mixed neuronal cultures were treated with Aβ oligomers (2 µM) for 48 h both in absence or presence of FLX (1 µM) or increasing concentrations of VTX (100 nM, 250 nM, or 1 µM) (pre-treatment of 1 h). The Aβ oligomers-induced toxicity was quantitatively assessed by trypan blue exclusion assay. Cell counts were performed in three to four random microscopic fields/well.
Statistics
Data are reported as mean ± standard error of the mean (S.E.M.) except in the case of cell experiments in which standard deviation (S.D.) was showed. One-way analysis of variance (ANOVA) followed by Tukey’s post hoc test were used for multiple comparisons. The version 8.0 of GraphPad Prism software® (GraphPad, La Jolla, CA, United States) was used to perform all the analyses. Only two-tailed p values < 0.05 were considered statistically significant.
Study Approval
The study was authorized by the Institutional Animal Care and Use Committee (IACUC) of the University of Catania and by the Italian Ministry of Health (DDL 26/2014 and previous legislation; OPBA Project #266/2016). Animal care followed Italian (D.M. 116192) and EEC (O.J. of E.C.L 358/1 12/18/1986) regulations on protection of animals used for experimental and scientific purposes.
Results
Fluoxetine and Vortioxetine Decreased the Expression of iNOS and Nox2 mRNAs
Oxidative stress and neuroinflammation play a significant role in the pathogenesis of depression (Bhattacharya and Drevets, 2017; Caruso et al., 2019a) and AD (Huang et al., 2016; Knezevic and Mizrahi, 2018). During the inflammation process, both inducible nitric oxide synthase (iNOS), responsible for nitric oxide production (Aktan, 2004; Metto et al., 2013), and NADPH oxidase 2 (Nox2), responsible for superoxide production (de Campos et al., 2015), are over-activated in immune cells including microglia (Siegel et al., 2019). When the above-mentioned enzymes are simultaneously activated, they synergistically promote neuronal cell death by generating peroxynitrite (Beckman and Crow, 1993). We therefore examined the effects of Aβ oligomers on the mRNAs levels of the pro-oxidant enzymes iNOS and Nox2 in the hippocampus (Figure 1), a brain area strongly affected in depression and AD (Villa et al., 2016; Setti et al., 2017). The i.c.v. injection of Aβ oligomers induced a statistically significant increase in the expression level of iNOS mRNA in the hippocampus compared with vehicle-treated controls (p < 0.05 vs. PBS + VEH; Figure 1A). Long-term i.p. treatment with FLX or VTX, administered at the same dose of 10 mg/kg, was able to abolish the over-expression of iNOS Aβ-induced (p < 0.05 vs. Aβ oligomers), whereas the lower dose of VTX (5 mg/kg) did not reach a statistically significant difference, even though a trend in iNOS mRNA enzyme expression decrease was observed. More robust effects were observed when measuring the variation of Nox2 mRNA expression levels under our experimental conditions. In fact, as shown in Figure 1B, the expression level of Nox2 mRNA was significantly increased in the hippocampus of Aβ-injected mice compared with vehicle-treated controls (p < 0.001 vs. PBS + VEH). Long-term i.p. treatment with FLX (10 mg/kg) or VTX, at both doses (5 or 10 mg/kg), was able to completely counteract the over-expression of this enzyme (p < 0.001 vs. Aβ oligomers for all of them). It is worth mentioning that treatment with FLX or VTX per se did not significantly modify the mRNA expression levels of iNOS and Nox2 enzymes (Supplementary Figure S1).
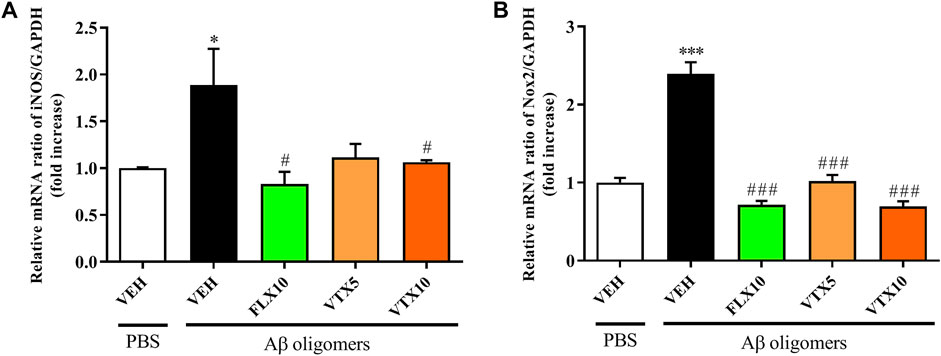
FIGURE 1. Fluoxetine and vortioxetine decrease the expression of iNOS and Nox2 mRNAs. Effects induced by i.c.v. administration of Aβ oligomers (Aβ + VEH) in absence or presence of FLX10, VTX5, or VTX10 on (A) iNOS and (B) Nox2 mRNAs expression measured by qRT-PCR. The abundance of each mRNA of interest was expressed relative to the abundance of GAPDH-mRNA, as an internal control. As a negative control, a reaction in absence of cDNA (no template control, NTC) was performed. qRT-PCR amplifications were performed at least in triplicate (mean of three to six determinations). Data are shown as mean ± S.E.M. *p < 0.05 vs. PBS + VEH, ***p < 0.001 vs. PBS + VEH, #p < 0.05 vs. Aβ oligomers + VEH, ###p < 0.001 vs. Aβ oligomers + VEH.
Fluoxetine and Vortioxetine Decreased the Expression of iNOS and Nox2 Proteins
We then carried out WB experiments in order to corroborate with protein data the results obtained by using qRT-PCR in which the expression levels of iNOS and Nox2 mRNA were measured. WB analysis confirmed that the i.c.v. injection of Aβ oligomers is able to induce a significant increase of iNOS protein expression at hippocampal level (p < 0.05 vs. PBS + VEH) and, most importantly, that the treatment with FLX and VTX is able to completely abolish Aβ-induced iNOS expression (p < 0.001 vs. Aβ oligomers for both of them; Figure 2A). A very similar profile was observed when measuring the variation of Nox2 protein levels. Figure 2B shows that the expression level of Nox2 protein was significantly increased in the hippocampus of Aβ-injected mice compared with vehicle-treated controls (p < 0.05 vs. PBS + VEH). Treatment with FLX at the dose of 10 mg/kg or with the lower dose of VTX (5 mg/kg) abolished Aβ oligomers induction (p < 0.05 vs. Aβ oligomers), while a stronger decrease was observed in the case of the higher dose of VTX (10 mg/kg) (p < 0.01 vs. Aβ oligomers). As expected based on the qRT-PCR results, the treatment with FLX or VTX per se did not significantly modify the protein expression levels of iNOS and Nox2 enzymes (Supplementary Figure S2).
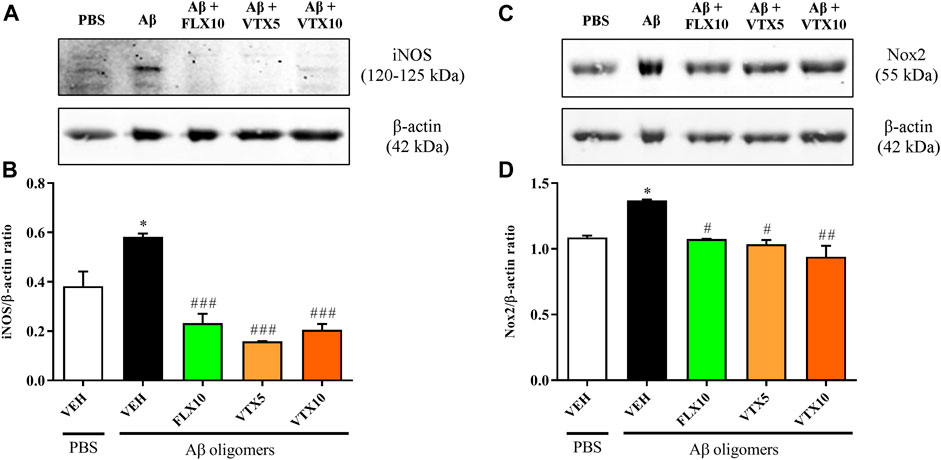
FIGURE 2. Fluoxetine and vortioxetine decrease the expression of iNOS and Nox2 proteins. Effects induced by i.c.v. administration of Aβ oligomers (Aβ + VEH) in absence or presence of FLX10, VTX5, or VTX10 on (B) iNOS and (D) Nox2 protein levels measured by WB. (A, C) show the representative immunoblots of iNOS (120–125 kDa) and Nox2 (55 kDa), respectively, in total protein extracts from hippocampal tissue. Histograms refer to the means ± S.E.M. of the densitometric values of iNOS or Nox2 bands normalized against β-actin (42 kDa). Each experiment was repeated three times. *p < 0.05 vs. PBS + VEH, #p < 0.05 vs. Aβ oligomers + VEH. ##p < 0.01 vs. Aβ oligomers + VEH, ###p < 0.001 vs. Aβ oligomers + VEH.
Fluoxetine or Vortioxetine Rescued the Expression of Gpx1 at Gene but Not at Protein Level
As previously mentioned, oxidative stress, a key factor in the progression of AD (Zhao and Zhao, 2013; Bajpai et al., 2014), reflects the imbalance between the production and quenching of reactive species in the biological system. This imbalance could also depend on the reduced activity of antioxidant enzymes such as glutathione peroxidase 1 (Gpx1) (Marcus et al., 1998; Katrenčíková et al., 2021). The lack of Gpx1 has been related to the exacerbation of Aβ-mediated neurotoxicity in cortical neurons (Crack et al., 2006). With this in mind, we investigated the effects of Aβ oligomers, in absence or presence of FLX10, VTX5, or VTX10, on the mRNA levels of Gpx1 in the hippocampus. The results depicted in Figure 3A show that the expression level of Gpx1 mRNA was significantly decreased in the hippocampus of Aβ-injected mice compared with vehicle-treated controls (p < 0.05 vs. PBS + VEH), while the treatment with FLX was able to completely restore Gpx1 mRNA levels (p < 0.05 vs. Aβ oligomers). A more significant effect was observed in the case of both doses of VTX (p < 0.001 vs. Aβ oligomers). Since it has been shown that oxidative stress can induce multimerization of Gpx1 enzyme by forming complexes via oxidative linkage between subunits (Park et al., 2004; Sultan et al., 2018), we performed WB analysis by measuring both monomeric and dimeric forms. However, the results obtained by WB showed that either the monomeric nor the dimeric form of Gpx1 protein in the hippocampus were significantly affected by Aβ treatment as compared to vehicle-treated controls (Figures 3B,C). The presence of antidepressants during Aβ treatment did not modulate the expression levels of either enzyme isoforms. The treatment with FLX or VTX per se, in the absence of Aβ treatment, did not significantly modify the mRNA (Supplementary Figure S1) and protein (Supplementary Figure S2) levels of Gpx1 enzyme.
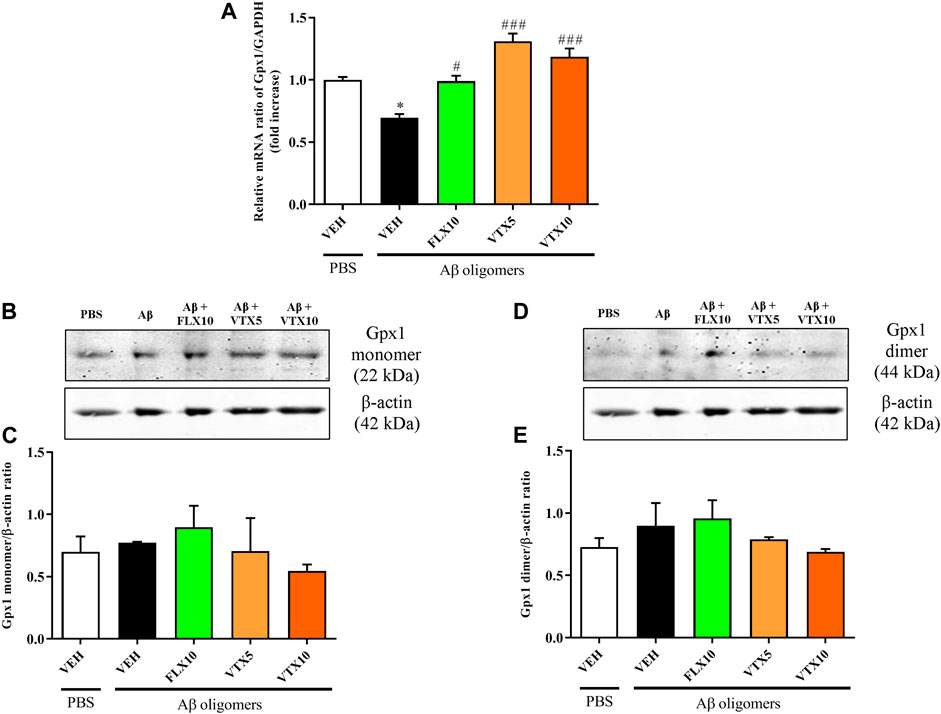
FIGURE 3. The treatment with fluoxetine or vortioxetine is needed to rescue the expression of Gpx1 at gene but not at protein level. Effects induced by i.c.v. administration of Aβ oligomers (Aβ + VEH) in absence or presence of FLX10, VTX5, or VTX10 on (A) Gpx1 mRNA expression measured by qRT-PCR; (C) monomer and (E) dimer of Gpx1 protein levels measured by WB. (B, D) show the representative immunoblots of Gpx1 monomer (22 kDa) and Gpx1 dimer (44 kDa) in total protein extracts from hippocampal tissue. In the case of gene expression measurements, the abundance of Gpx1 mRNA was expressed relative to the abundance of GAPDH-mRNA, as an internal control. As a negative control, a reaction in absence of cDNA (no template control, NTC) was performed. qRT-PCR amplifications were performed at least in triplicate (mean of three to five determinations). In the case of protein expression measurements, histograms refer to the means ± S.E.M. of the densitometric values of Gpx1 monomer or Gpx1 dimer bands normalized against β-actin (42 kDa). Each experiment was repeated three times. *p < 0.05 vs. PBS + VEH, #p < 0.05 vs. Aβ oligomers + VEH, ###p < 0.001 vs. Aβ oligomers + VEH.
Fluoxetine and Vortioxetine Exert Neuroprotection Against the Toxicity Induced by Aβ Oligomers
The neuroprotective activity of FLX in mixed cultures of cortical cells treated with Aβ oligomers, representing an established experimental model of Aβ-induced neurodegeneration (Caruso et al., 2019c), has been already reported (Caraci et al., 2016). However, it is presently unknown whether a treatment with VTX can prevent the neuronal cell death due to Aβ treatment. We then investigated the neuroprotective activity of increasing concentrations of VTX in mixed cultures of cortical cells treated with Aβ oligomers (2 µM) for 48 h. In this set of experiments, FLX at the concentration of 1 µM was used as a gold standard. Since Aβ is known to promote glutamate release and toxicity (Caraci et al., 2011), the experiments were performed in the presence of a cocktail of ionotropic glutamate receptor antagonists [MK-801 (10 µM) and DNQX (30 µM)] to exclude the contribution of endogenous excitotoxicity to the overall process of neuronal death. The treatment of mixed cultures of cortical cells with Aβ oligomers for 48 h led to a significant increase (about 300%) in the number of trypan blue positive cells (dead neurons) compared to untreated (CTRL) cells (p < 0.001) (Figure 4). VTX, starting at a concentration of 250 nM, significantly prevented Aβ toxicity in mixed neuronal cultures (p < 0.05 vs. Aβ), though not completely. The maximal neuroprotective effect was observed in the case of VTX 1 µM (p < 0.001 vs. Aβ), with a number of dead cells comparable to that observed for untreated cells or for FLX-treated cells (positive control).
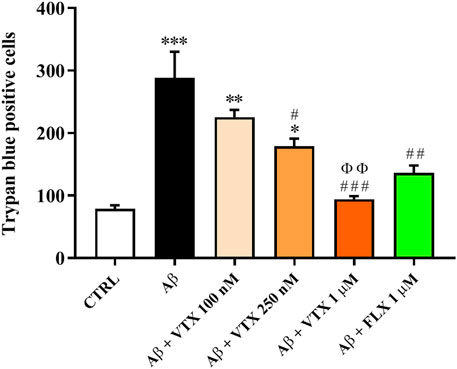
FIGURE 4. Fluoxetine and vortioxetine exert neuroprotection against the toxicity induced by Aβ oligomers. Primary mixed neuronal cultures were treated with Aβ oligomers (2 µM) for 48 h both in absence or presence of FLX (1 µM) or increasing concentrations of VTX (100 nM, 250 nM, and 1 µM) (pre-treatment of 1 h). The toxicity of Aβ oligomers in mixed neuronal cultures was assessed by cell counting after trypan blue staining. Cell counts was performed in three random microscopic fields/well. Data are the mean of three to four determinations. S.D. are represented by vertical bars. *p < 0.05 vs. CTRL, **p < 0.01 vs. CTRL, ***p < 0.001 vs. CTRL, #p < 0.05 vs. Aβ, ##p < 0.01 vs. Aβ, ###p < 0.001 vs. Aβ, ՓՓp < 0.01 vs. VTX 100 nM.
Discussion
Reactive species such as reactive oxygen species (ROS) and reactive nitrogen species (RNS) play a crucial role in numerous human pathophysiological processes. These species, when produced at physiological concentration, are able to regulate growth, apoptosis, and complex functions such as blood pressure, immune and cognitive functions. When overproduced, they can contribute to a well-known and deleterious phenomenon called oxidative stress (Estévez and Jordán, 2002). During acute oxidative stress, the components of the antioxidant system are able to counteract the increased levels of pro-oxidants, such as ROS and RNS, resetting them to the physiological levels. Whenever ROS and RNS levels overcome the antioxidant defense, chronic oxidative stress takes place, as it has been observed in neuropsychiatric disorders such as depression and AD (Caruso et al., 2019d). Both ROS and RNS are involved in the pathogenesis of depression by influencing neuronal processes such as neurogenesis and neuroplasticity, also inducing neuroinflammation and neurodegeneration (Cheignon et al., 2018; Solleiro-Villavicencio and Rivas-Arancibia, 2018). When considering AD, the over-production of ROS and RNS, and then oxidative stress, has been related to increased Aβ production and/or aggregation, which in turn exacerbates neuronal oxidative damage, contributing to neuronal death in AD brain (Cheignon et al., 2018).
In the present work we employed a non-Tg model of AD, obtained by i.c.v. injection of Aβ oligomers, allowing to investigate the molecular mechanisms through which the oligomeric form of Aβ causes cognitive dysfunction, and finally to test novel pharmacological approaches (Balducci and Forloni, 2014). As previously demonstrated, this non-Tg AD model is characterized by cerebral concentrations of soluble Aβ oligomers comparable to those observed in the AD brain, sufficient to induce a memory deficit that persists for 2–3 weeks (Leggio et al., 2016). By adopting this model, we have recently demonstrated that both FLX and VTX possess the ability to reverse the depressive-like phenotype and memory deficits induced by the i.c.v. injection of Aβ oligomers in mice, also rescuing the levels of the synaptic proteins synaptophysin and PSD-95 as well as of TGF-β1, the deficit of which has been shown to contribute to inflammation and cognitive decline both in depression and AD (Caraci et al., 2018b; Torrisi et al., 2019). In order to correlate the above-mentioned preclinical efficacy of these two second-generation antidepressants with an antioxidant activity of these drugs, we examined the mRNA levels of iNOS and Nox2, two pro-oxidant enzymes synergistically able to promote neuronal death through the production of ROS and RNS (Beckman and Crow, 1993), in the hippocampus of Aβ-injected mice, a brain area strongly affected in both depression and AD (Villa et al., 2016; Setti et al., 2017). Our results show that the i.c.v. injection of Aβ oligomers in mice induced a significant increase in iNOS and Nox2 enzymes in the hippocampus, both at mRNA (Figure 1) and protein (Figure 2) level, compared with vehicle-treated controls. These findings are in agreement with an in vivo study carried out by Medeiros et al. (2007) in which the i.c.v. injection of Aβ1–40 induced iNOS protein expression in hippocampus and prefrontal cortex of mice that was paralleled by marked deficits of learning and memory, emphasizing the deleterious effects of aberrant expression of NOS isoforms in AD brain (Lüth et al., 2002). Our findings are also in line with other studies showing that AD is characterized by inflammatory processes in which the production of nitric oxide from iNOS and/or superoxide from Nox2 is strongly increased (Murphy, 2000; Brown and Bal-Price, 2003; Zekry et al., 2003). In the present study we showed for the first time that a long-term i.p. treatment with FLX or VTX was able to abolish the over-expression of iNOS and Nox2 induced by Aβ oligomers (Figures 1, 2). These results are also consistent with previous findings, showing the ability of antidepressant drugs to exert immune-regulatory effects by reducing the levels of pro-inflammatory cytokines, also decreasing the production of reactive species and/or enhancing key elements of the antioxidant machinery. Along this line, FLX has been shown to revert brain oxidative damage by reducing lipid peroxidation and increasing the activity of the antioxidant enzymes (i.e., CAT and SOD) in the hippocampus of an animal model of depression (Moretti et al., 2012). In a study carried out by Talmon et al. (2018) the multimodal antidepressant VTX was able to reduced the oxidative burst induced by PMA in monocytes and in macrophages (M1 and M2), also leading to a shift of macrophage polarization from the pro-inflammatory (M1) to the anti-inflammatory (M2) phenotype. The above reduction of oxidative stress was also paralleled by a decrease of nuclear factor kappa-light-chain-enhancer of activated B cells (NF-kB) translocation and TNF-α release. The present data on the antioxidant activity of FLX and VTX in vivo reinforce the above-described previous findings obtained in translational models of inflammation. Our findings on the antioxidant activity of FLX and VTX might be therapeutically relevant in the context of depression and AD, when considering that depression acts as a risk factor for AD and, most importantly, that oxidative stress processes play a key role in the pathophysiology of cognitive deficits in depression (Scapagnini et al., 2012).
About 30% of depressed patients show a poor response to conventional antidepressants associated with a significant cognitive impairment and a poor quality of life, a clinical phenotype classified as treatment-resistant depression (TRD) (Caraci et al., 2018a). It has been recently shown how TRD is characterized by increased oxidative stress coupled to inflammation (Sowa-Kućma et al., 2018). Furthermore, plasma levels of Coenzyme Q10 (CoQ10), a strong antioxidant with anti-inflammatory activity, are lower in TRD patients compared to responders depressed patients (Maes et al., 2009) and CoQ10 (200 mg/die) has been proposed as an adjuvating agent for the treatment of depression (Mehrpooya et al., 2018). According to this scenario second-generation antidepressant drugs, endowed with antioxidant activity, may display an increased clinical efficacy in TRD patients; studies in animal models are useful to test this hypothesis. As previously mentioned, antioxidants play a significant role in maintaining redox homeostasis. It is also worth mentioning that an increase in Nox2 protein expression and the related oxidative stress have also been observed in sleep deprivation known to induce memory impairments, serotoninergic system dysfunction, and depression in mice (Wang et al., 2020). In our experimental model of amyloid-related depression the presence of depressive-like behavior and memory deficit was paralleled by an increase in Nox2 protein levels in Aβ-injected mice that was rescued by the long-term treatment with FLX at 10 mg/kg and more significantly by VTX, being effective at the lowest dose of 5 mg/kg, suggesting that the antidepressant activity of these drugs is not simply related to the inhibition of serotonin transporter (SERT), but it is also includes antioxidants effects.
In our study we also measured the mRNA and protein levels of Gpx1, an antioxidant enzyme known to play a protective role against Aβ-toxicity and the related ROS accumulation at intracellular level (Barkats et al., 2000), and the deficit of which has been related to increased Aβ-mediated neurotoxicity (Crack et al., 2006). Our data show that the treatment with FLX or VTX is needed to rescue the expression of Gpx1 at mRNA, but not at protein level. We cannot exclude a post-trascriptional regulation of Gpx1 gene expression. In our case, the discrepancy observed when comparing mRNA and protein levels within the same time frame might be due to a compensatory response of the antioxidant system with an additional role played by FLX or VTX, which remains to be elucidated.
We know from our previous work in the non-Tg model of AD that FLX and VTX not only counteract oxidative stress, but also rescue the hippocampal TGF-β1 levels. This neurobiological link could be of utmost importance since it has already been demonstrated that other multimodal drugs that counteract oxidative stress can also rescue TGF-β1 levels (Caruso et al., 2019b; Fresta et al., 2020b). In two very recent research studies it has also demonstrated the ability of TGF-β1 to protect retinal ganglion cells against oxidative stress through the modulation of the HO-1/Nrf2 pathway (Chen et al., 2020) and chondrocytes via FOXO1-autophagy axis (Kurakazu et al., 2021), strongly suggesting a key role of TGF-β1 in counteracting oxidative stress induced by Aβ.
In the AD brain, oxidative stress promotes the generation of ROS that contribute to neurodegeneration (Shimohama et al., 2000; Abramov and Duchen, 2005). During the last decade, based on their ability to exert neuroprotection, the use of antidepressants to reduce the risk to develop AD has been proposed (Kessing et al., 2009; Kessing, 2012; Yuste et al., 2015; Bartels et al., 2018). By using a well-established in vitro model of Aβ-induced neurodegeneration consisting of mixed cultures of cortical cells challenged with Aβ oligomers, we were able to compare, for the first time, the well-known neuroprotective activity of FLX (Caraci et al., 2016) with the protective effects of VTX. Interestingly we found that VTX pre-treatment started to exert significant neuroprotective effects at nanomolar concentrations (250 nM) with a maximal effect at 1 μM, similar to that observed for FLX pre-treatment and untreated cells (Figure 4). The neuroprotective effects exerted in vitro, as well as the antidepressant effects exerted in vivo by FLX and VTX could be related to the anti-amyloidogenic and anti-aggregant activity of these drugs. In particular, SSRIs including FLX have shown the potential to prevent Aβ aggregation by direct binding and could be beneficial to AD patients (Tin et al., 2019). FLX also possesses a recognized ability to revert soluble Aβ-induced depressive phenotype with a specific “Aβ-lowering” effect (Schiavone et al., 2017). Furthermore, it cannot be excluded that both FLX and VTX could exert their therapeutic potential by enhancing the release of TGF-β1 from microglial cells, as observed in our in vivo experiments, then rescuing the antioxidant system through the activation of TGF-β1 signaling. The results observed in our experimental models with therapeutic concentrations of VTX also stimulate further studies both in rodents and MCI patients with amyloid-related depression.
All together the data presented in this study, obtained by using a non-Tg model of AD, demonstrated that oxidative stress, taking place as a consequence of pro-oxidant enzymes (i.e., iNOS and Nox2) activation, along with the previously showed deficit of TGF-β1, represents one of the neurobiological links between depression and AD. We also showed for the first time how a long-term administration of the antidepressants fluoxetine and vortioxetine, able to rescue the TGF-β1 pathway, can also contribute to prevent amyloid-induced depression and cognitive decline by counteracting Aβ-induced oxidative stress.
Data Availability Statement
All datasets generated for this study are included in the article and the Supplementary Material. Further inquiries can be directed to the corresponding author.
Ethics Statement
The animal study was reviewed and approved by the Institutional Animal Care and Use Committee (IACUC) of the University of Catania and by the Italian Ministry of Health (DDL 26/2014 and previous legislation; OPBA Project #266/2016).
Author Contributions
GC and FC gave substantial contributions to the conception and design of the work. GC, MG, AF, ST, NM, FG, MT, and AP performed the experiments. GC, MG, AF, and FC analyzed the data. FT, DP, SS, GL, FD, and FC participated in the design and coordination of the study. GC, MG, AF, and GL drafted the work. All authors revised the work and approved the version to be published.
Funding
This research was funded by University of Catania, PIAno InCEntivi per la RIcerca di Ateneo UniCT 2020–2022-Linea 3 Project Asclepio and also conducted with a partial unrestricted support of Lundbeck. This research was also supported by PRIN 2017 (Program of Relevant National interest- 2017AY8BP4_004) from the Italian Ministry of University and Research.
Conflict of Interest
The authors declare that the research was conducted in the absence of any commercial or financial relationships that could be construed as a potential conflict of interest.
Publisher’s Note
All claims expressed in this article are solely those of the authors and do not necessarily represent those of their affiliated organizations, or those of the publisher, the editors and the reviewers. Any product that may be evaluated in this article, or claim that may be made by its manufacturer, is not guaranteed or endorsed by the publisher.
Acknowledgments
The authors would like to thank the BRIT laboratory at the University of Catania (Italy) for the valuable technical assistance and use of their laboratories.
Supplementary Material
The Supplementary Material for this article can be found online at: https://www.frontiersin.org/articles/10.3389/fphar.2021.809541/full#supplementary-material
References
Abramov, A. Y., and Duchen, M. R. (2005). The Role of an Astrocytic NADPH Oxidase in the Neurotoxicity of Amyloid Beta Peptides. Philos. Trans. R. Soc. Lond. B Biol. Sci. 360 (1464), 2309–2314. doi:10.1098/rstb.2005.1766
Aktan, F. (2004). iNOS-mediated Nitric Oxide Production and its Regulation. Life Sci. 75 (6), 639–653. doi:10.1016/j.lfs.2003.10.042
Bajpai, A., Verma, A. K., Srivastava, M., and Srivastava, R. (2014). Oxidative Stress and Major Depression. J. Clin. Diagn. Res. 8 (12), Cc04–7. doi:10.7860/jcdr/2014/10258.5292
Balducci, C., and Forloni, G. (2014). In Vivo application of Beta Amyloid Oligomers: a Simple Tool to Evaluate Mechanisms of Action and New Therapeutic Approaches. Curr. Pharm. Des. 20 (15), 2491–2505. doi:10.2174/13816128113199990497
Barkats, M., Millecamps, S., Abrioux, P., Geoffroy, M. C., and Mallet, J. (2000). Overexpression of Glutathione Peroxidase Increases the Resistance of Neuronal Cells to Abeta-Mediated Neurotoxicity. J. Neurochem. 75 (4), 1438–1446. doi:10.1046/j.1471-4159.2000.0751438.x
Bartels, C., Wagner, M., Wolfsgruber, S., Ehrenreich, H., and Schneider, A. (2018). Impact of SSRI Therapy on Risk of Conversion from Mild Cognitive Impairment to Alzheimer's Dementia in Individuals with Previous Depression. Am. J. Psychiatry 175 (3), 232–241. doi:10.1176/appi.ajp.2017.17040404
Beckman, J. S., and Crow, J. P. (1993). Pathological Implications of Nitric Oxide, Superoxide and Peroxynitrite Formation. Biochem. Soc. Trans. 21 (2), 330–334. doi:10.1042/bst0210330
Behr, G. A., Moreira, J. C., and Frey, B. N. (2012). Preclinical and Clinical Evidence of Antioxidant Effects of Antidepressant Agents: Implications for the Pathophysiology of Major Depressive Disorder. Oxid Med. Cel Longev 2012, 609421. doi:10.1155/2012/609421
Bennabi, D., Haffen, E., and Van Waes, V. (2019). Vortioxetine for Cognitive Enhancement in Major Depression: From Animal Models to Clinical Research. Front. Psychiatry 10, 771. doi:10.3389/fpsyt.2019.00771
Bhattacharya, A., and Drevets, W. C. (2017). Role of Neuro-Immunological Factors in the Pathophysiology of Mood Disorders: Implications for Novel Therapeutics for Treatment Resistant Depression. Curr. Top. Behav. Neurosci. 31, 339–356. doi:10.1007/7854_2016_43
Brorsson, A. C., Kumita, J. R., MacLeod, I., Bolognesi, B., Speretta, E., Luheshi, L. M., et al. (2010). Methods and Models in Neurodegenerative and Systemic Protein Aggregation Diseases. Front. Biosci. (Landmark Ed. 15, 373–396. doi:10.2741/3626
Brown, G. C., and Bal-Price, A. (2003). Inflammatory Neurodegeneration Mediated by Nitric Oxide, Glutamate, and Mitochondria. Mol. Neurobiol. 27 (3), 325–355. doi:10.1385/mn:27:3:325
Caraci, F., Calabrese, F., Molteni, R., Bartova, L., Dold, M., Leggio, G. M., et al. (2018a). International Union of Basic and Clinical Pharmacology CIV: The Neurobiology of Treatment-Resistant Depression: From Antidepressant Classifications to Novel Pharmacological Targets. Pharmacol. Rev. 70 (3), 475–504. doi:10.1124/pr.117.014977
Caraci, F., Gulisano, W., Guida, C. A., Impellizzeri, A. A., Drago, F., Puzzo, D., et al. (2015). A Key Role for TGF-Β1 in Hippocampal Synaptic Plasticity and Memory. Sci. Rep. 5, 11252. doi:10.1038/srep11252
Caraci, F., Molinaro, G., Battaglia, G., Giuffrida, M. L., Riozzi, B., Traficante, A., et al. (2011). Targeting Group II Metabotropic Glutamate (mGlu) Receptors for the Treatment of Psychosis Associated with Alzheimer's Disease: Selective Activation of mGlu2 Receptors Amplifies Beta-Amyloid Toxicity in Cultured Neurons, whereas Dual Activation of mGlu2 and mGlu3 Receptors Is Neuroprotective. Mol. Pharmacol. 79 (3), 618–626. doi:10.1124/mol.110.067488
Caraci, F., Spampinato, S. F., Morgese, M. G., Tascedda, F., Salluzzo, M. G., Giambirtone, M. C., et al. (2018b). Neurobiological Links between Depression and AD: The Role of TGF-Β1 Signaling as a New Pharmacological Target. Pharmacol. Res. 130, 374–384. doi:10.1016/j.phrs.2018.02.007
Caraci, F., Tascedda, F., Merlo, S., Benatti, C., Spampinato, S. F., Munafò, A., et al. (2016). Fluoxetine Prevents Aβ1-42-Induced Toxicity via a Paracrine Signaling Mediated by Transforming-Growth-Factor-Β1. Front. Pharmacol. 7, 389. doi:10.3389/fphar.2016.00389
Caruso, G., Benatti, C., Blom, J. M. C., Caraci, F., and Tascedda, F. (2019a). The Many Faces of Mitochondrial Dysfunction in Depression: From Pathology to Treatment. Front. Pharmacol. 10, 995. doi:10.3389/fphar.2019.00995
Caruso, G., Benatti, C., Musso, N., Fresta, C. G., Fidilio, A., Spampinato, G., et al. (2021). Carnosine Protects Macrophages against the Toxicity of Aβ1-42 Oligomers by Decreasing Oxidative Stress. Biomedicines 9 (5), 477. doi:10.3390/biomedicines9050477
Caruso, G., Fresta, C. G., Fidilio, A., O'Donnell, F., Musso, N., Lazzarino, G., et al. (2019b). Carnosine Decreases PMA-Induced Oxidative Stress and Inflammation in Murine Macrophages. Antioxidants (Basel) 8 (8), 281. doi:10.3390/antiox8080281
Caruso, G., Fresta, C. G., Musso, N., Giambirtone, M., Grasso, M., Spampinato, S. F., et al. (2019c). Carnosine Prevents Aβ-Induced Oxidative Stress and Inflammation in Microglial Cells: A Key Role of TGF-Β1. Cells 8 (1), 64. doi:10.3390/cells8010064
Caruso, G., Spampinato, S. F., Cardaci, V., Caraci, F., Sortino, M. A., and Merlo, S. (2019d). β-Amyloid and Oxidative Stress: Perspectives in Drug Development. Curr. Pharm. Des. 25 (45), 4771–4781. doi:10.2174/1381612825666191209115431
Cheignon, C., Tomas, M., Bonnefont-Rousselot, D., Faller, P., Hureau, C., and Collin, F. (2018). Oxidative Stress and the Amyloid Beta Peptide in Alzheimer's Disease. Redox Biol. 14, 450–464. doi:10.1016/j.redox.2017.10.014
Chen, H. Y., Ho, Y. J., Chou, H. C., Liao, E. C., Tsai, Y. T., Wei, Y. S., et al. (2020). TGF-β1 Signaling Protects Retinal Ganglion Cells from Oxidative Stress via Modulation of the HO-1/Nrf2 Pathway. Chem. Biol. Interact 331, 109249. doi:10.1016/j.cbi.2020.109249
Chung, E. S., Chung, Y. C., Bok, E., Baik, H. H., Park, E. S., Park, J. Y., et al. (2010). Fluoxetine Prevents LPS-Induced Degeneration of Nigral Dopaminergic Neurons by Inhibiting Microglia-Mediated Oxidative Stress. Brain Res. 1363, 143–150. doi:10.1016/j.brainres.2010.09.049
Colaianna, M., Tucci, P., Zotti, M., Morgese, M. G., Schiavone, S., Govoni, S., et al. (2010). Soluble Beta Amyloid(1-42): a Critical Player in Producing Behavioural and Biochemical Changes Evoking Depressive-Related State. Br. J. Pharmacol. 159 (8), 1704–1715. doi:10.1111/j.1476-5381.2010.00669.x
Correia, A. S., and Vale, N. (2021). Antidepressants in Alzheimer's Disease: A Focus on the Role of Mirtazapine. Pharmaceuticals (Basel) 14 (9), 930. doi:10.3390/ph14090930
Crack, P. J., Cimdins, K., Ali, U., Hertzog, P. J., and Iannello, R. C. (2006). Lack of Glutathione Peroxidase-1 Exacerbates Abeta-Mediated Neurotoxicity in Cortical Neurons. J. Neural Transm. (Vienna) 113 (5), 645–657. doi:10.1007/s00702-005-0352-y
Dafsari, F. S., and Jessen, F. (2020). Depression-an Underrecognized Target for Prevention of Dementia in Alzheimer's Disease. Transl Psychiatry 10 (1), 160. doi:10.1038/s41398-020-0839-1
de Campos, R. P., Siegel, J. M., Fresta, C. G., Caruso, G., da Silva, J. A., and Lunte, S. M. (2015). Indirect Detection of Superoxide in RAW 264.7 Macrophage Cells Using Microchip Electrophoresis Coupled to Laser-Induced Fluorescence. Anal. Bioanal. Chem. 407 (23), 7003–7012. doi:10.1007/s00216-015-8865-1
Estévez, A. G., and Jordán, J. (2002). Nitric Oxide and Superoxide, a Deadly Cocktail. Ann. N. Y Acad. Sci. 962, 207–211. doi:10.1111/j.1749-6632.2002.tb04069.x
Fidilio, A., Grasso, M., Turnaturi, R., Caruso, G., Spitale, F. M., Vicario, N., et al. (2021). The Multimodal MOPr/DOPr Agonist LP2 Reduces Allodynia in Chronic Constriction Injured Rats by Rescue of TGF-Β1 Signalling. Front. Pharmacol. 12, 749365. doi:10.3389/fphar.2021.749365
Fresta, C. G., Caruso, G., Fidilio, A., Platania, C. B. M., Musso, N., Caraci, F., et al. (2020a). Dihydrotanshinone, a Natural Diterpenoid, Preserves Blood-Retinal Barrier Integrity via P2X7 Receptor. Int. J. Mol. Sci. 21 (23), 9305. doi:10.3390/ijms21239305
Fresta, C. G., Fidilio, A., Lazzarino, G., Musso, N., Grasso, M., Merlo, S., et al. (2020b). Modulation of Pro-oxidant and Pro-inflammatory Activities of M1 Macrophages by the Natural Dipeptide Carnosine. Int. J. Mol. Sci. 21 (3), 776. doi:10.3390/ijms21030776
Gelain, D. P., Antonio Behr, G., Birnfeld de Oliveira, R., and Trujillo, M. (2012). Antioxidant Therapies for Neurodegenerative Diseases: Mechanisms, Current Trends, and Perspectives. Oxid Med. Cel Longev 2012, 895153. doi:10.1155/2012/895153
Glenner, G. G., and Wong, C. W. (1984). Alzheimer's Disease: Initial Report of the Purification and Characterization of a Novel Cerebrovascular Amyloid Protein. Biochem. Biophys. Res. Commun. 120 (3), 885–890. doi:10.1016/s0006-291x(84)80190-4
Gong, Y., Chang, L., Viola, K. L., Lacor, P. N., Lambert, M. P., Finch, C. E., et al. (2003). Alzheimer's Disease-Affected Brain: Presence of Oligomeric A Beta Ligands (ADDLs) Suggests a Molecular Basis for Reversible Memory Loss. Proc. Natl. Acad. Sci. U S A. 100 (18), 10417–10422. doi:10.1073/pnas.1834302100
Huang, W. J., Zhang, X., and Chen, W. W. (2016). Role of Oxidative Stress in Alzheimer's Disease. Biomed. Rep. 4 (5), 519–522. doi:10.3892/br.2016.630
Jin, L., Gao, L. F., Sun, D. S., Wu, H., Wang, Q., Ke, D., et al. (2017). Long-term Ameliorative Effects of the Antidepressant Fluoxetine Exposure on Cognitive Deficits in 3 × TgAD Mice. Mol. Neurobiol. 54 (6), 4160–4171. doi:10.1007/s12035-016-9952-910.1007/s12035-016-9952-9
Katrenčíková, B., Vaváková, M., Paduchová, Z., Nagyová, Z., Garaiova, I., Muchová, J., et al. (2021). Oxidative Stress Markers and Antioxidant Enzymes in Children and Adolescents with Depressive Disorder and Impact of Omega-3 Fatty Acids in Randomised Clinical Trial. Antioxidants 10 (8), 1256. doi:10.3390/antiox10081256
Kessing, L. V. (2012). Depression and the Risk for Dementia. Curr. Opin. Psychiatry 25 (6), 457–461. doi:10.1097/YCO.0b013e328356c368
Kessing, L. V., Søndergård, L., Forman, J. L., and Andersen, P. K. (2009). Antidepressants and Dementia. J. Affect Disord. 117 (1-2), 24–29. doi:10.1016/j.jad.2008.11.020
Klein, W. L. (2013). Synaptotoxic Amyloid-β Oligomers: a Molecular Basis for the Cause, Diagnosis, and Treatment of Alzheimer's Disease. J. Alzheimers Dis. 33 (Suppl. 1), S49–S65. doi:10.3233/jad-2012-129039
Knezevic, D., and Mizrahi, R. (2018). Molecular Imaging of Neuroinflammation in Alzheimer's Disease and Mild Cognitive Impairment. Prog. Neuropsychopharmacol. Biol. Psychiatry 80 (Pt B), 123–131. doi:10.1016/j.pnpbp.2017.05.007
Kumar, A., Sidhu, J., Goyal, A., and Tsao, J. W. (2021). “Alzheimer Disease,” in StatPearls (Treasure Island (FL): StatPearls Publishing LLC.).
Kurakazu, I., Akasaki, Y., Tsushima, H., Sueishi, T., Toya, M., Kuwahara, M., et al. (2021). TGFβ1 Signaling Protects Chondrocytes against Oxidative Stress via FOXO1-Autophagy axis. Osteoarthritis Cartilage 29 (11), 1600–1613. doi:10.1016/j.joca.2021.07.015
Ledo, J. H., Azevedo, E. P., Beckman, D., Ribeiro, F. C., Santos, L. E., Razolli, D. S., et al. (2016). Cross Talk between Brain Innate Immunity and Serotonin Signaling Underlies Depressive-like Behavior Induced by Alzheimer's Amyloid-β Oligomers in Mice. J. Neurosci. 36 (48), 12106–12116. doi:10.1523/jneurosci.1269-16.2016
Ledo, J. H., Azevedo, E. P., Clarke, J. R., Ribeiro, F. C., Figueiredo, C. P., Foguel, D., et al. (2020). Correction: Amyloid-β Oligomers Link Depressive-like Behavior and Cognitive Deficits in Mice. Mol. Psychiatry. doi:10.1038/s41380-020-00873-6
Leggio, G. M., Catania, M. V., Puzzo, D., Spatuzza, M., Pellitteri, R., Gulisano, W., et al. (2016). The Antineoplastic Drug Flavopiridol Reverses Memory Impairment Induced by Amyloid-SS1-42 Oligomers in Mice. Pharmacol. Res. 106, 10–20. doi:10.1016/j.phrs.2016.02.007
Li, Y., Sanchez, C., and Gulinello, M. (2017). Distinct Antidepressant-like and Cognitive Effects of Antidepressants with Different Mechanisms of Action in Middle-Aged Female Mice. Int. J. Neuropsychopharmacol. 20 (6), 510–515. doi:10.1093/ijnp/pyx004
Lozupone, M., La Montagna, M., D'Urso, F., Piccininni, C., Sardone, R., Dibello, V., et al. (2018). Pharmacotherapy for the Treatment of Depression in Patients with Alzheimer's Disease: a Treatment-Resistant Depressive Disorder. Expert Opin. Pharmacother. 19 (8), 823–842. doi:10.1080/14656566.2018.1471136
Lüth, H. J., Münch, G., and Arendt, T. (2002). Aberrant Expression of NOS Isoforms in Alzheimer's Disease Is Structurally Related to Nitrotyrosine Formation. Brain Res. 953 (1-2), 135–143. doi:10.1016/s0006-8993(02)03280-8
Maes, M., Mihaylova, I., Kubera, M., Uytterhoeven, M., Vrydags, N., and Bosmans, E. (2009). Lower Plasma Coenzyme Q10 in Depression: a Marker for Treatment Resistance and Chronic Fatigue in Depression and a Risk Factor to Cardiovascular Disorder in that Illness. Neuro Endocrinol. Lett. 30 (4), 462–469.
Marcus, D. L., Thomas, C., Rodriguez, C., Simberkoff, K., Tsai, J. S., Strafaci, J. A., et al. (1998). Increased Peroxidation and Reduced Antioxidant Enzyme Activity in Alzheimer's Disease. Exp. Neurol. 150 (1), 40–44. doi:10.1006/exnr.1997.6750
McIntyre, R. S., Harrison, J., Loft, H., Jacobson, W., and Olsen, C. K. (2016). The Effects of Vortioxetine on Cognitive Function in Patients with Major Depressive Disorder: A Meta-Analysis of Three Randomized Controlled Trials. Int. J. Neuropsychopharmacol. 19 (10), pyw055. doi:10.1093/ijnp/pyw055
Medeiros, R., Prediger, R. D., Passos, G. F., Pandolfo, P., Duarte, F. S., Franco, J. L., et al. (2007). Connecting TNF-Alpha Signaling Pathways to iNOS Expression in a Mouse Model of Alzheimer's Disease: Relevance for the Behavioral and Synaptic Deficits Induced by Amyloid Beta Protein. J. Neurosci. 27 (20), 5394–5404. doi:10.1523/jneurosci.5047-06.2007
Mehrpooya, M., Yasrebifar, F., Haghighi, M., Mohammadi, Y., and Jahangard, L. (2018). Evaluating the Effect of Coenzyme Q10 Augmentation on Treatment of Bipolar Depression: A Double-Blind Controlled Clinical Trial. J. Clin. Psychopharmacol. 38 (5), 460–466. doi:10.1097/jcp.0000000000000938
Metto, E. C., Evans, K., Barney, P., Culbertson, A. H., Gunasekara, D. B., Caruso, G., et al. (2013). An Integrated Microfluidic Device for Monitoring Changes in Nitric Oxide Production in Single T-Lymphocyte (Jurkat) Cells. Anal. Chem. 85 (21), 10188–10195. doi:10.1021/ac401665u
Minati, L., Edginton, T., Bruzzone, M. G., and Giaccone, G. (2009). Current Concepts in Alzheimer's Disease: a Multidisciplinary Review. Am. J. Alzheimers Dis. Other Demen. 24 (2), 95–121. doi:10.1177/1533317508328602
Moretti, M., Colla, A., de Oliveira Balen, G., dos Santos, D. B., Budni, J., de Freitas, A. E., et al. (2012). Ascorbic Acid Treatment, Similarly to Fluoxetine, Reverses Depressive-like Behavior and Brain Oxidative Damage Induced by Chronic Unpredictable Stress. J. Psychiatr. Res. 46 (3), 331–340. doi:10.1016/j.jpsychires.2011.11.009
Murphy, S. (2000). Production of Nitric Oxide by Glial Cells: Regulation and Potential Roles in the CNS. Glia 29 (1), 1–13. doi:10.1002/(sici)1098-1136(20000101)29:1<1:aid-glia1>3.0.co;2-n
Park, S. J., Ciccone, S. L., Beck, B. D., Hwang, B., Freie, B., Clapp, D. W., et al. (2004). Oxidative Stress/damage Induces Multimerization and Interaction of Fanconi Anemia Proteins. J. Biol. Chem. 279 (29), 30053–30059. doi:10.1074/jbc.M403527200
Petersen, R. C., Caracciolo, B., Brayne, C., Gauthier, S., Jelic, V., and Fratiglioni, L. (2014). Mild Cognitive Impairment: a Concept in Evolution. J. Intern. Med. 275 (3), 214–228. doi:10.1111/joim.12190
Scapagnini, G., Davinelli, S., Drago, F., De Lorenzo, A., and Oriani, G. (2012). Antioxidants as Antidepressants: Fact or Fiction. CNS Drugs 26 (6), 477–490. doi:10.2165/11633190-000000000-00000
Schiavone, S., Tucci, P., Mhillaj, E., Bove, M., Trabace, L., and Morgese, M. G. (2017). Antidepressant Drugs for Beta Amyloid-Induced Depression: A New Standpoint. Prog. Neuropsychopharmacol. Biol. Psychiatry 78, 114–122. doi:10.1016/j.pnpbp.2017.05.004
Setti, S. E., Hunsberger, H. C., and Reed, M. N. (2017). Alterations in Hippocampal Activity and Alzheimer's Disease. Transl Issues Psychol. Sci. 3 (4), 348–356. doi:10.1037/tps0000124
Shimohama, S., Tanino, H., Kawakami, N., Okamura, N., Kodama, H., Yamaguchi, T., et al. (2000). Activation of NADPH Oxidase in Alzheimer's Disease Brains. Biochem. Biophys. Res. Commun. 273 (1), 5–9. doi:10.1006/bbrc.2000.2897
Siegel, J. M., Schilly, K. M., Wijesinghe, M. B., Caruso, G., Fresta, C. G., and Lunte, S. M. (2019). Optimization of a Microchip Electrophoresis Method with Electrochemical Detection for the Determination of Nitrite in Macrophage Cells as an Indicator of Nitric Oxide Production. Anal. Methods 11 (2), 148–156. doi:10.1039/c8ay02014k
Solleiro-Villavicencio, H., and Rivas-Arancibia, S. (2018). Effect of Chronic Oxidative Stress on Neuroinflammatory Response Mediated by CD4+T Cells in Neurodegenerative Diseases. Front Cel Neurosci 12, 114. doi:10.3389/fncel.2018.00114
Sowa-Kućma, M., Styczeń, K., Siwek, M., Misztak, P., Nowak, R. J., Dudek, D., et al. (2018). Lipid Peroxidation and Immune Biomarkers Are Associated with Major Depression and its Phenotypes, Including Treatment-Resistant Depression and Melancholia. Neurotox Res. 33 (2), 448–460. doi:10.1007/s12640-017-9835-5
Sultan, C. S., Saackel, A., Stank, A., Fleming, T., Fedorova, M., Hoffmann, R., et al. (2018). Impact of Carbonylation on Glutathione Peroxidase-1 Activity in Human Hyperglycemic Endothelial Cells. Redox Biol. 16, 113–122. doi:10.1016/j.redox.2018.02.018
Szałach, Ł. P., Lisowska, K. A., and Cubała, W. J. (2019). The Influence of Antidepressants on the Immune System. Arch. Immunol. Ther. Exp. (Warsz) 67 (3), 143–151. doi:10.1007/s00005-019-00543-8
Talmon, M., Rossi, S., Pastore, A., Cattaneo, C. I., Brunelleschi, S., and Fresu, L. G. (2018). Vortioxetine Exerts Anti-inflammatory and Immunomodulatory Effects on Human Monocytes/macrophages. Br. J. Pharmacol. 175 (1), 113–124. doi:10.1111/bph.14074
Thase, M. E., Mahableshwarkar, A. R., Dragheim, M., Loft, H., and Vieta, E. (2016). A Meta-Analysis of Randomized, Placebo-Controlled Trials of Vortioxetine for the Treatment of Major Depressive Disorder in Adults. Eur. Neuropsychopharmacol. 26 (6), 979–993. doi:10.1016/j.euroneuro.2016.03.007
Tin, G., Mohamed, T., Shakeri, A., Pham, A. T., and Rao, P. P. N. (2019). Interactions of Selective Serotonin Reuptake Inhibitors with β-Amyloid. ACS Chem. Neurosci. 10 (1), 226–234. doi:10.1021/acschemneuro.8b00160
Torrisi, S. A., Geraci, F., Tropea, M. R., Grasso, M., Caruso, G., Fidilio, A., et al. (2019). Fluoxetine and Vortioxetine Reverse Depressive-like Phenotype and Memory Deficits Induced by Aβ1-42 Oligomers in Mice: A Key Role of Transforming Growth Factor-Β1. Front. Pharmacol. 10, 693. doi:10.3389/fphar.2019.00693
Varadarajan, S., Yatin, S., Aksenova, M., and Butterfield, D. A. (2000). Review: Alzheimer's Amyloid Beta-Peptide-Associated Free Radical Oxidative Stress and Neurotoxicity. J. Struct. Biol. 130 (2-3), 184–208. doi:10.1006/jsbi.2000.4274
Villa, R. F., Ferrari, F., Gorini, A., Brunello, N., and Tascedda, F. (2016). Effect of Desipramine and Fluoxetine on Energy Metabolism of Cerebral Mitochondria. Neuroscience 330, 326–334. doi:10.1016/j.neuroscience.2016.05.051
Wang, J., Zhang, Y., Xu, H., Zhu, S., Wang, H., He, J., et al. (2014). Fluoxetine Improves Behavioral Performance by Suppressing the Production of Soluble β-amyloid in APP/PS1 Mice. Curr. Alzheimer Res. 11 (7), 672–680. doi:10.2174/1567205011666140812114715
Wang, W., Yang, L., Liu, T., Ma, Y., Huang, S., He, M., et al. (2020). Corilagin Ameliorates Sleep Deprivation-Induced Memory Impairments by Inhibiting NOX2 and Activating Nrf2. Brain Res. Bull. 160, 141–149. doi:10.1016/j.brainresbull.2020.03.010
Wu, J. Q., Kosten, T. R., and Zhang, X. Y. (2013). Free Radicals, Antioxidant Defense Systems, and Schizophrenia. Prog. Neuropsychopharmacol. Biol. Psychiatry 46, 200–206. doi:10.1016/j.pnpbp.2013.02.015
Yuste, J. E., Tarragon, E., Campuzano, C. M., and Ros-Bernal, F. (2015). Implications of Glial Nitric Oxide in Neurodegenerative Diseases. Front. Cel Neurosci 9, 322. doi:10.3389/fncel.2015.00322
Zekry, D., Epperson, T. K., and Krause, K. H. (2003). A Role for NOX NADPH Oxidases in Alzheimer's Disease and Other Types of Dementia. IUBMB Life 55 (6), 307–313. doi:10.1080/1521654031000153049
Keywords: oxidative stress, Alzheimer’s disease, depression, amyloid-β, vortioxetine, fluoxetine, TGF-β1, neuroprotection
Citation: Caruso G, Grasso M, Fidilio A, Torrisi SA, Musso N, Geraci F, Tropea MR, Privitera A, Tascedda F, Puzzo D, Salomone S, Drago F, Leggio GM and Caraci F (2021) Antioxidant Activity of Fluoxetine and Vortioxetine in a Non-Transgenic Animal Model of Alzheimer’s Disease. Front. Pharmacol. 12:809541. doi: 10.3389/fphar.2021.809541
Received: 05 November 2021; Accepted: 17 November 2021;
Published: 24 December 2021.
Edited by:
Maria Grazia Morgese, University of Foggia, ItalyCopyright © 2021 Caruso, Grasso, Fidilio, Torrisi, Musso, Geraci, Tropea, Privitera, Tascedda, Puzzo, Salomone, Drago, Leggio and Caraci. This is an open-access article distributed under the terms of the Creative Commons Attribution License (CC BY). The use, distribution or reproduction in other forums is permitted, provided the original author(s) and the copyright owner(s) are credited and that the original publication in this journal is cited, in accordance with accepted academic practice. No use, distribution or reproduction is permitted which does not comply with these terms.
*Correspondence: Giuseppe Caruso, Zm9yZ2l1c2VwcGVjYXJ1c29AZ21haWwuY29t
†These authors share first authorship
‡These authors share last authorship