- 1Department of Pediatrics, Case Western Reserve University, Cleveland, OH, United States
- 2Galleon Pharmaceuticals, Inc., Horsham, PA, United States
- 3Herman B Wells Center for Pediatric Research, Indiana University School of Medicine, Indianapolis, IN, United States
- 4Department of Biochemistry and Biophysics, University of Rochester Medical Center, Rochester, NY, United States
- 5Department of Biomedical Engineering, Case Western Reserve University, Cleveland, OH, United States
- 6Division of Pulmonary, Critical Care and Sleep Medicine, Case Western Reserve University, Cleveland, OH, United States
- 7Department of Anesthesia, University of Iowa, Iowa City, IA, United States
- 8Department of Pharmacology, Case Western Reserve University, Cleveland, OH, United States
- 9Functional Electrical Stimulation Center, Case Western Reserve University, Cleveland, OH, United States
Endogenous and exogenously administered S-nitrosothiols modulate the activities of central and peripheral systems that control breathing. We have unpublished data showing that the deleterious effects of morphine on arterial blood-gas chemistry (i.e., pH, pCO2, pO2, and sO2) and Alveolar-arterial gradient (i.e., index of gas exchange) were markedly diminished in anesthetized Sprague Dawley rats that received a continuous intravenous infusion of the endogenous S-nitrosothiol, S-nitroso-L-cysteine. The present study extends these findings by showing that unanesthetized adult male Sprague Dawley rats receiving an intravenous infusion of S-nitroso-L-cysteine (100 or 200 nmol/kg/min) markedly diminished the ability of intravenous injections of the potent synthetic opioid, fentanyl (10, 25, and 50 μg/kg), to depress the frequency of breathing, tidal volume, and minute ventilation. Our study also found that the ability of intravenously injected fentanyl (10, 25, and 50 μg/kg) to disturb eupneic breathing, which was measured as a marked increase of the non-eupneic breathing index, was substantially reduced in unanesthetized rats receiving intravenous infusions of S-nitroso-L-cysteine (100 or 200 nmol/kg/min). In contrast, the deleterious effects of fentanyl (10, 25, and 50 μg/kg) on frequency of breathing, tidal volume, minute ventilation and non-eupneic breathing index were fully expressed in rats receiving continuous infusions (200 nmol/kg/min) of the parent amino acid, L-cysteine, or the D-isomer, namely, S-nitroso-D-cysteine. In addition, the antinociceptive actions of the above doses of fentanyl as monitored by the tail-flick latency assay, were enhanced by S-nitroso-L-cysteine, but not L-cysteine or S-nitroso-D-cysteine. Taken together, these findings add to existing knowledge that S-nitroso-L-cysteine stereoselectively modulates the detrimental effects of opioids on breathing, and opens the door for mechanistic studies designed to establish whether the pharmacological actions of S-nitroso-L-cysteine involve signaling processes that include 1) the activation of plasma membrane ion channels and receptors, 2) selective intracellular entry of S-nitroso-L-cysteine, and/or 3) S-nitrosylation events. Whether alterations in the bioavailability and bioactivity of endogenous S-nitroso-L-cysteine is a key factor in determining the potency/efficacy of fentanyl on breathing is an intriguing question.
Introduction
Endogenous S-nitrosothiols (SNOs) regulate a variety of neural systems within the central (Lei et al., 1992; Lipton et al., 1993; Lipton et al., 1994; Takahashi et al., 2007; Tegeder et al., 2011; Raju et al., 2015; Tarasenko, 2015; Nakamura and Lipton, 2016) and peripheral nervous systems (Meller et al., 1990; Matsuda et al., 1995; Savidge, 2011; Lee et al., 2013; Tooker and Vigh, 2015; Gaston et al., 2020). SNOs exert their effects by mechanisms involving breakdown to nitric oxide (NO) followed by formation of dinitrosothiol-iron complexes that activate intracellular soluble guanylate cyclase and protein kinase G cell signaling (Mellion et al., 1983; Travis et al., 1996; Severina et al., 2003; Lima et al., 2010; Martínez-Ruiz et al., 2013; Marozkina and Gaston, 2015; Vanin, 2019), and by S-nitrosylation (transfer of NO+) of sulfur atoms within functional proteins (Jaffrey et al., 2001; Joksovik et al., 2007; Foster et al., 2009; Lima et al., 2010; Rudkouskaya et al., 2010; Marozkina and Gaston, 2012; Anand et al., 2014; Pires da Silva et al., 2016; Wynia-Smith and Smith, 2017; Stomberski et al., 2019; Marozkina and Gaston, 2020). S-nitroso-L-cysteine (L-CSNO) is an endogenous endothelium-derived SNO (Myers et al., 1990; Bates et al., 1991; Kukreja et al., 1993) that is synthesized and stored in vesicles of vascular endothelial cells (Seckler et al., 2020). L-CSNO may be actively/exocytotically released from the endothelium of peripheral vascular beds (Davisson et al., 1996a; Batenburg et al., 2004a; Batenburg et al., 2004b; Hashmi-Hill et al., 2007; Batenburg et al., 2009), and from neurogenic vasodilator nerves in peripheral vascular beds (Davisson et al., 1994; Davisson et al., 1996a; Davisson et al., 1996b; Davisson et al., 1997a; Possas and Lewis 1997; Possas et al., 2006).
Many of the pharmacological actions of SNOs such as L-CSNO and S-nitroso-β,β-dimethyl-L-cysteine, depend upon their stereoisomeric configuration (Davisson et al., 1996d; Lewis et al., 1996; Travis et al., 1996; Davisson et al., 1997b; Ohta et al., 1997; Travis et al., 1997; Hoque et al., 1999; Hoque et al., 2000; Travis et al., 2000; Lipton et al., 2001; Lewis et al., 2005a; Lewis et al., 2005b; Lewis et al., 2006a; Gaston et al., 2020). For instance, microinjections of L-CSNO into the nucleus tractus solitarius (NTS) lower arterial blood pressure in anesthetized rats (Ohta et al., 1997), and injections of L-CSNO into the lateral (Davisson et al., 1997b) or fourth (Lewis et al., 1996) ventricles of unanesthetized freely-moving rats elicit pronounced hemodynamic responses, however, injections of the D-isomer (D-CSNO) elicit minor responses. Potential stereoselective L-CSNO binding/recognition sites have not been fully identified, nevertheless we reported that L-CSNO, but not D-CSNO, directly activates voltage-gated K+-channels in a process that does not require the S-nitrosylation of the functional protein (Gaston et al., 2020). With respect to control of breathing, microinjections of L-CSNO into the NTS elevate minute ventilation (VE) in unanesthetized freely-moving rats (Lipton et al., 2001) by stereospecific mechanisms that are not related to L-CSNO decomposition to NO. In addition, it is evident that arterial injections of L-CSNO increases VE in unanesthetized rats by activation of stereoselective processes in the carotid bodies, since 1) the L-CSNO-induced ventilatory responses were substantially diminished in rats that previously underwent bilateral transection of the carotid sinus nerves (CSNs) and 2) the L-CSNO-induced responses were not produced by D-CSNO (Gaston et al., 2020).
The involvement of nitrosyl factors, such as NO and SNOs, in the pharmacological actions of opioids has received considerable attention. For example, there is substantial evidence for nitrosyl factors playing roles in 1) opioid receptor (OR) signaling processes (Pol, 2007; Toda et al., 2009a; Toda et al., 2009b; Rodríguez-Muñoz and Garzón, 2013), and 2) opioid effects on a) vascular function and reactivity (Sahin et al., 2005; Kaye et al., 2006), b) pain processing (Pelligrino, et al., 1996; Maegawa and Tonussi, 2003; Cury et al., 2011; Hervera et al., 2011; Mehanna et al., 2018; Ortiz et al., 2020), c) vision (Someya et al., 2017), and d) inflammatory-immunoregulatory processes (Bilfinger, et al., 1998; Jan et al., 2011). Additionally, nitrosyl factors are involved in opioid-induced catalepsy (Erkent et al., 2006), tolerance to opioids (Kissin et al., 2000; Ozdemir et al., 2011; Durmus et al., 2014), and fentanyl pre-conditioning (Lu et al., 2014). Nonetheless, only a few studies have sought evidence as to potential roles for nitrosyl factors in the ventilatory depressant effects of opioids. For instance, the ventilatory depressant responses elicited by fourth ventricular infusions of morphine in awake dogs were reduced by prior injection of the NO synthase (NOS) inhibitor, L-nitro-arginine (L-NA), whereas injection of L-NA after the injection of morphine was ineffective (Pellegrino et al., 1996). Another study found that the ventilatory depressant effects of morphine in anesthetized cats are independent of neuronal NOS (nNOS) (Teppema et al., 2000). In addition, recent studies (Seckler et al., 2022) demonstrated that the ventilatory depressant effects of fentanyl were augmented in unanesthetized rats that had received the NOS inhibitor, NG-nitro-L-arginine methyl ester (L-NAME), suggesting that the dominant role for nitrosyl factors is to ameliorate the deleterious effects of opioids on ventilation. Although the mechanisms by which nitrosyl factors exert these effects are not clear, it is known that SNOs modulate G protein-coupled receptor signaling in a receptor-specific and reversible manner (Whalen et al., 1999; Kokkola et al., 2005; Nozik-Grayck et al., 2006; Whalen et al., 2007), and importantly, in the context of the present study, that neither SNO-L-CYS or S-nitroso-L-glutathione directly interact with μ-ORs (Kokkola et al., 2005).
Recently, we determined that the detrimental effects of morphine on arterial blood-gas chemistry (i.e., pH, pCO2, pO2, and SO2), Alveolar-arterial gradient (i.e., index of alveolar gas-exchange), respiratory frequency (fR), tidal volume (VT), and minute ventilation (VE) were markedly reduced in anesthetized rats receiving a continuous intravenous infusion of L-CSNO, but not those receiving D-CSNO infusion. Moreover, the antinociceptive effects of morphine were not reduced in the rats receiving either L-CSNO or D-CSNO. While furthering our knowledge about the roles of nitrosyl factors in ventilatory control processes (Stamler et al., 1997; Lipton et al., 2001; Gaston et al., 2006; Haldar and Stamler, 2013; Palmer et al., 2013a; Gaston et al., 2014; Palmer et al., 2015; Gaston et al., 2020; Getsy et al., 2021; Seckler et al., 2022) and establishing that L-CSNO has intriguing properties directly related to modulation of OR signaling cascades, the presence of anesthesia could be viewed as problematic, and the choice of morphine does not directly address the most urgent need to develop therapies against fentanyl, an analogue driving the current opioid crisis (Han et al., 2019; Torralva and Janowsky, 2019; Lutfy, 2020). As such, the major objective of the present study was to determine whether continuous intravenous infusions of L-CSNO at 100 or 200 nmol/kg/min could modulate the problematic effects of fentanyl given by consecutive injections of 10, 25, and 50 μg/kg, IV, on ventilatory parameters, fR, VT, and VE, and ventilatory stability, as defined by the non-eupneic breathing index (NEBI) (Getsy et al., 2014), in unanesthetized and unrestrained Sprague Dawley rats. A secondary objective was to determine whether infusion of L-CSNO modulates the antinociceptive actions of fentanyl in unanesthetized rats as determined by the radiant-heat tail-flick latency (TFL) assay (Henderson et al., 2014; Gaston et al., 2021). Finally, we report surprising, but intriguing, findings related to the ability of the peripherally restricted OR antagonist, naloxone methiodide (NLXmi) (Lewanowitsch and Irvine, 2002; Lewanowitsch, et al., 2006; Henderson et al., 2014), to markedly increase fR in rats that had received prior injections of fentanyl, but not vehicle.
Materials and Methods
Permissions and Rats and Surgeries
All animal studies were carried out in accordance with the National Institutes of Health Guide for the Care and Use of Laboratory Animals (NIH Publication No. 80.23) revised in 1996. The protocols were approved by the Institutional Animal Care and Use Committees of the University of Virginia, Case Western Reserve University, and Galleon Pharmaceuticals, Inc. Adult male Sprague Dawley rats were obtained from Harlan Laboratories, Inc. (Indianapolis, IN, United States). These rats were caged in standard housing conditions in our vivaria with free access to food and water. Room temperature (22°C), humidity (48%–50%) and light-dark cycle (12:12 h) were maintained consistently in each vivarium and laboratory where the studies were performed. All protocols involved the use of rats that had been implanted with two intravenous jugular catheters exteriorized to the back of the neck as detailed previously (Davisson et al., 1996a; Davisson et al., 1996b; Davisson et al., 1996c; Davisson et al., 1996d; Davisson et al., 1997a). The jugular vein catheters were implanted under 2.5%–3.5% isoflurane anesthesia 7 days previously to be sure that the rats were free from surgical discomfort. On the day of the experiment, one catheter (PE-50 connected to PE-10, the PE-10 tubing inserted into the right jugular vein) allowed for the continuous intravenous infusion of vehicle (20 μl/min), L-CSNO (100 or 200 mol/kg/min), L-cysteine (200 nmol/kg/min) or D-CSNO (200 nmol/kg/min). The second catheter was inserted into the left jugular vein for the bolus injection of vehicle, fentanyl or NLXmi. It is important to note the infusions were maintained throughout the experiment.
Whole Body Plethysmography Protocols
Ventilatory parameters (i.e., fR, VT, VE, and NEBI) were recorded in freely-moving rats by whole body plethysmography (PLY3223; Data Sciences International, St. Paul, MN, United States) as detailed previously (Getsy et al., 2020; Gaston et al., 2021; Seckler et al., 2022). The rats were given 60 min to acclimate to the chambers, thus allowing true resting (i.e., baseline) ventilatory parameters to be established. A description of the protocols undergone by the six treatment groups of rats and their average body weights are provided in Table 1. Briefly, treatment group one (i.e., Vehicle 1) rats received a continuous infusion of phosphate-buffered saline (PBS) (i.e., vehicle) at pH 7.2. After 45 min these rats received three injections of vehicle, each given 30 min apart. Thirty minutes after the third vehicle injection, the rats received an injection of NLXmi (2.5 mg/kg, IV). Treatment group two (i.e., Vehicle 2) rats received a continuous infusion of vehicle and after 45 min, injections of fentanyl at doses 10, 25, and 50 μg/kg, each given 30 min apart. Thirty minutes after injection of the 50 μg/kg dose of fentanyl, the rats received an injection of vehicle (100 μl/kg, IV). Treatment group three (i.e., L-CSNO) rats received a continuous infusion of L-CSNO (200 nmol/kg/min) and after 45 min, injections of fentanyl at doses 10, 25, and 50 μg/kg, each given 30 min apart. Thirty minutes after injection of a 50 μg/kg dose of fentanyl, the rats received an injection of NLXmi (2.5 mg/kg, IV). Treatment group four (i.e., Vehicle 3) rats received the same protocol as Vehicle 1 rats and served as the controls for treatment group five (i.e., L-Cysteine) and treatment group six (i.e., D-CSNO). L-Cysteine and D-CSNO rats received an infusion of L-cysteine (200 nmol/kg/min, IV) or D-CSNO (200 nmol/kg/min, IV) respectively and after 45 min, injections of fentanyl (10, 25, and 50 μg/kg) each given 30 min apart. Thirty minutes after the injection of the 50 μg/kg dose of fentanyl, the rats received an injection of NLXmi (2.5 mg/kg, IV). It is important to note that the infusions of the test agents were continued throughout the entire protocol (i.e., until 15 min after the injection of NLXmi or vehicle).
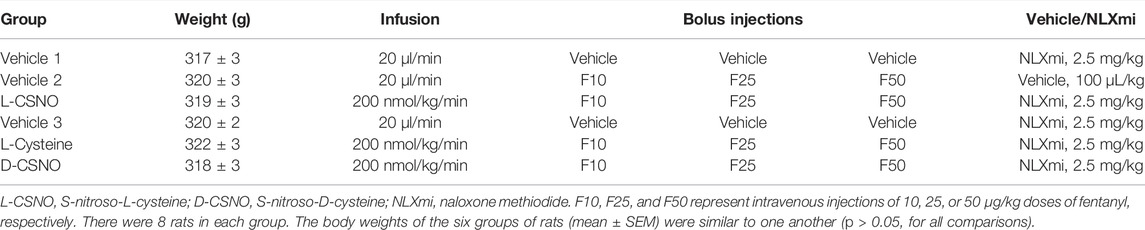
TABLE 1. Description of the six treatment groups used in the whole body plethysmography experiments.
Due to the closeness of the body weights of the six treatment groups of rats, ventilatory data, specifically VT and VE, are presented without body weight corrections. Provided software (Fine Pointe, BUXCO) constantly corrected digitized values for changes in chamber temperature and humidity. Pressure changes associated with the respiratory waveforms were then converted to volumes (i.e., VT) using the algorithm of Epstein and colleagues (Epstein and Epstein, 1978; Epstein et al., 1980). Factoring in chamber temperature and humidity, the cycle analyzers filtered acquired signals, and Fine Pointe algorithms generated an array of box flow data that identified a waveform segment as an acceptable (eupneic) breath. From that data vector, the minimum and maximum values were determined. Flows at this point were considered to be box flow signals. From this array, minimum and maximum box flow values were determined and multiplied by a compensation factor provided by the selected algorithm (Epstein and Epstein, 1978; Epstein et al., 1980), thus producing VT values that were used to determine the non-eupneic breathing events expressed as the non-eupneic breathing index (NEBI), reported as the percentage of non-eupneic breathing events per epoch (Getsy et al., 2014). All directly recorded parameters, including NEBI, were then extracted from the raw waveforms using Data Sciences International (St. Paul, MN, United States) proprietary Biosystem XA software (version 2.9.0.2) and proprietary FinePointe software (version v2.8.0), as described previously (Getsy et al., 2020; Gaston et al., 2021; Seckler et al., 2022) and as detailed in the Data Sciences International/Buxco website reference to the list of parameters provided by proprietary FinePointe Software using whole body plethysmography (https://www.datasci.com/products/buxco-respiratory-products/finepointe-whole-body-plethysmography). The BioSystem XA software extracts the waveforms that are analyzed by the FinePointe software that uses National Instruments Measurement Studio to perform the analyses (http://zone.ni.com/reference/en-XX/help/37263 6F-01/mstudiowebhelp/html/5d5b3031/).
Antinociception Protocols
Antinociception status in unanesthetized rats was determined by a radiant heat tail-flick latency (TFL) assay, as detailed previously (Lewis et al., 1991; Henderson et al., 2014; Gaston et al., 2021; Jenkins et al., 2021). Prior to administration of fentanyl, the rats were allowed to crawl inside a canvas garden glove and lightly restrained within the glove to allow for the thermal withdrawal latencies to be determined. After injection of fentanyl, placement of the rat in the glove was aided by an investigator. Each investigator performing the TFL assay was unaware of the treatments that the rats were then subjected to. The TFL testing apparatus consisted of a beam of focused radiant heat provided by a 50 W projector lamp, which was focused on the underside of the tail at 1 of 5 sites 8–10 mm apart. TFL was measured to the nearest 0.1 s as the time from onset of heating of the tail to withdrawal of the tail from the heat. The intensity of the light beam was set so that baseline TFL values were about 2.5 s. A cutoff time of 12 s was set to minimize potential damage to the tail upon repeated application of the radiant heat beam. TFL was established before and during the various stages of the experiment. The data are presented as actual TFL values (sec), arithmetic changes (sec), and as maximum possible effect (%MPE) using the formula, %MPE = [(post-injection TFL − baseline TFL)/(12 − baseline TFL)] × 100. A description of the protocols undergone by the five treatment groups of rats used and their body weights are provided in Supplementary Table S1. Group 1 rats received a continuous infusion of phosphate-buffered saline (PBS) (i.e., vehicle) at pH 7.2. After 60 min these rats received three injections of vehicle, each given 30 min apart. Thirty minutes after injection of the third injection of vehicle, the rats received an injection of NLXmi (2.5 mg/kg, IV). Group 2 rats received a continuous infusion of vehicle and after 60 min, injections of fentanyl (10, 25, and 50 μg/kg), each given 30 min apart. Thirty minutes after injection of the 50 μg/kg dose of fentanyl, the rats received an injection of vehicle (100 μl/kg, IV). Group 3–5 rats received a continuous infusion of L-CSNO (200 nmol/kg/min, IV) or L-cysteine (200 nmol/kg/min, IV) or D-CSNO (200 nmol/kg/min, IV), respectively and after 60 min, injections of fentanyl (10, 25, and 50 μg/kg) each given 30 min apart. Thirty minutes after injection of the 50 μg/kg dose of fentanyl, the rats then received an injection of NLXmi (2.5 mg/kg, IV). It is important to note that the infusions of the test agents were continued throughout the entire protocol (i.e., until 15 min after the injection of NLXmi or vehicle).
Data Analyses
All data are presented as mean ± SEM and were evaluated using one-way and two-way ANOVA followed by Bonferroni corrections for multiple comparisons between means using the error mean square terms from each ANOVA analysis (Winer, 1971; Wallenstein et al., 1980; Ludbrook, 1998; McHugh, 2011) as detailed previously (Getsy et al., 2021; Jenkins et al., 2021). A value of p < 0.05 was taken as the initial level of statistical significance (Wallenstein et al., 1980; Ludbrook, 1998; McHugh, 2011). Statistical analyses were performed using GraphPad Prism software (GraphPad Software, Inc., La Jolla, CA, United States). A detailed description of these statistical procedures is provided in the Supplementary Material under “Detailed description of Statistical Approaches.”
Results
SNO-L-CYS Infusion Blunts the Fentanyl-Induced Changes in fR, VT and VE
The actual fR, VT, and VE values during various stages of the L-CSNO (100 or 200 nmol/kg/min, IV) infusion experiments are presented in Figure 1. As seen in Figure 1A, the infusions of vehicle or L-CSNO at 100 (L-CSNO 100) or 200 (L-CSNO 200) nmol/kg/min did not alter resting levels of fR. In vehicle-infused rats, injection of the 10 μg/kg dose of fentanyl elicited a transient rise in fR that quickly subsided before rising again to a plateau level that was sustained for the final 5 min of the post-injection period. Subsequent injection of the 25 μg/kg dose of fentanyl caused a transient rise in fR in the vehicle-infused rats that rapidly fell to below baseline levels, and then gradually recovered to values above pre-injection between 25 and 30 min post-injection. The ensuing injection of the 50 μg/kg dose of fentanyl caused an immediate fall in fR in the vehicle-infused rats that was sustained for about 20–25 min before returning to baseline values. As is apparent from of Figure 1A, the ability of the injections of fentanyl to decrease fR was substantially diminished in rats receiving the 100 or 200 nmol/kg/min infusions of L-CSNO. The dramatic effects elicited by the subsequent injection of NLXmi will be detailed below (see section Profound effects of NLXmi in fentanyl-injected rats). As seen in Figure 1B, the infusions of vehicle or L-CSNO 100 did not alter VT, whereas infusion of L-CSNO 200 elicited a relatively minor, but sustained increase in VT. The injection of 10 μg/kg of fentanyl elicited a marked decrease in VT for about 5 min in the vehicle-infused and L-CSNO 100 rats, and that was followed by a sustained increase in VT. The injection of 10 μg/kg of fentanyl caused VT of the L-CSNO 200 rats to decrease slightly for about 5 min, and that also was followed by a sustained increase. Subsequent injections of 25 and 50 μg/kg fentanyl elicited qualitatively similar responses to the 10 μg/kg dose of fentanyl in vehicle-infused rats, although the duration of the decrease in VT was somewhat larger after injection of the 50 μg/kg dose of fentanyl in vehicle-infused rats. The 25 and 50 μg/kg injections of fentanyl elicited markedly smaller decreases in VT in rats receiving the 100 or 200 nmol/kg/min infusions of L-CSNO, and VT remained above baseline levels for most of the recording period in these two groups. As seen in of Figure 1C, infusions of vehicle or 100 nmol/kg/min of L-CSNO did not alter VE, whereas infusion of L-CSNO at 200 nmol/kg/min elicited a minor, but sustained increase in VE. The injection of the 10 μg/kg dose of fentanyl elicited a pronounced decrease in VE of about 5 min in duration in the vehicle-infused and L-CSNO 100 rats that was followed by a substantial and sustained rise in VE above baseline values. VE of L-CSNO 200 rats did not change after injection of 10 μg/kg fentanyl, but did gradually increase approximately 20 min post-injection of 10 μg/kg dose of fentanyl even higher above baseline. Injections of 25 and 50 μg/kg of fentanyl elicited qualitatively similar responses in vehicle-infused rats, although the decrease in VE lasted somewhat longer after injection of 50 μg/kg fentanyl in vehicle-infused rats. The injections of 25 and 50 μg/kg doses of fentanyl elicited smaller decreases in VE in rats that were receiving the 100 nmol/kg/min infusion of L-CSNO compared to vehicle-infused rats, and we saw no decreases in VE in rats that were receiving the 200 nmol/kg/min infusion of L-CSNO. Thus, except for the decrease in VE elicited by 10 μg/kg fentanyl in rats receiving 100 nmol/kg/min of L-CSNO, VE remained above baseline levels for the post-injection periods for the L-CSNO 100 and L-CSNO 200 groups.
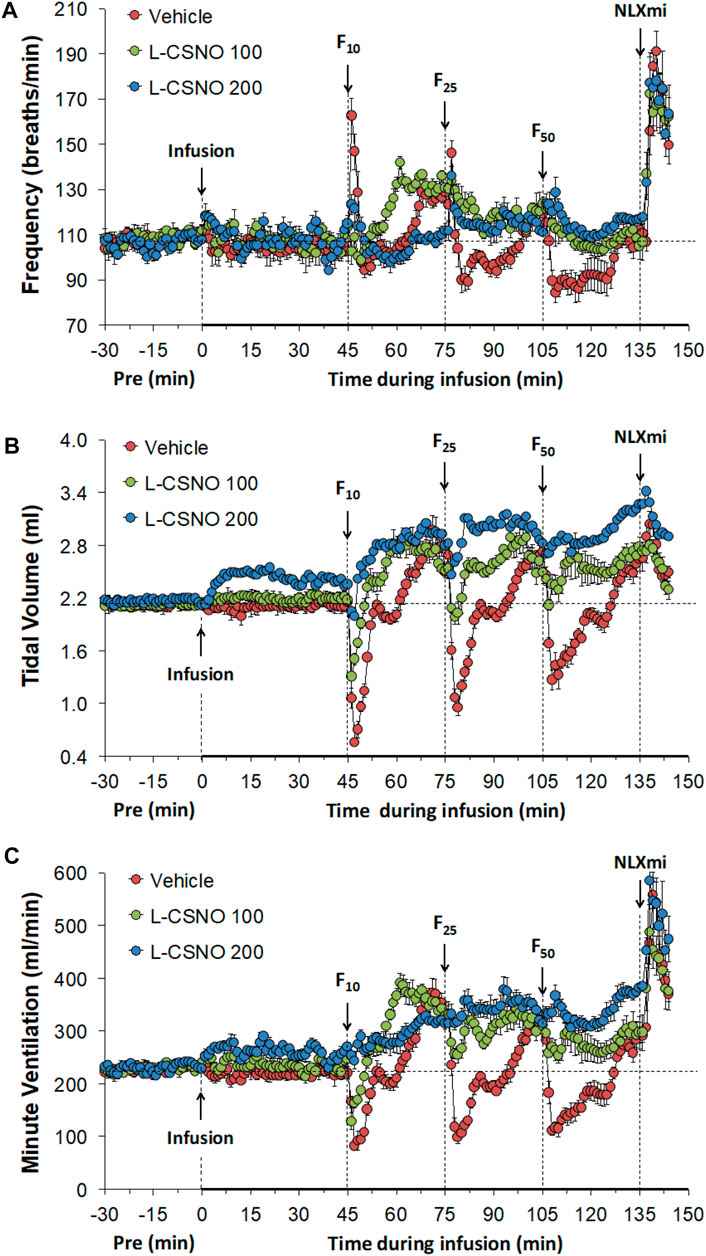
FIGURE 1. Frequency of breathing (A), tidal volume (B) and minute ventilation (C) values. The intravenous infusion of vehicle (20 μl/min, IV), L-CSNO 100 (S-nitroso-L-cysteine, 100 nmol/kg/min, IV) or L-CSNO 200 (S-nitroso-L-cysteine, 200 nmol/kg/min, IV) began at time 0. Bolus injections of fentanyl at F10 (10 μg/kg, IV), F25 (25 μg/kg, IV), and F50 (50 μg/kg, IV) were given at 45, 75, and 105 min, respectively. A bolus intravenous injection of naloxone methiodide (NLXmi, 1.5 mg/kg, IV) was given at time 135 min. Data are presented as mean ± SEM. There were 8 rats in each group.
The arithmetic changes in fR, VT, and VE (expressed as differences from the 45 min infusion timepoint) shown in Figure 2, confirm that the ability fentanyl to adversely affect fR, VT, and VE was markedly diminished in rats receiving infusions of L-CSNO 100 and L-CSNO 200. Figure 3 summarizes the total (cumulative) arithmetic changes in fR (Figure 3A), VT (Figure 3B) and VE (Figure 3C) from baseline (Pre) values during the first 5 min following injection of fentanyl at 10 μg/kg (F10), 25 μg/kg (F25), and 50 μg/kg (F50) in rats receiving infusion of vehicle (20 μl/min, IV), L-CSNO at 100 nmol/kg/min (L-CSNO 100) or 200 nmol/kg/min (L-CSNO 200). In vehicle-infused rats, injection of the 10 μg/kg dose of fentanyl caused a cumulative increase in fR, whereas the 25 and 50 μg/kg doses of fentanyl caused cumulative decreases in fR. The increase in fR elicited by the 10 μg/kg dose of fentanyl, and the decreases in fR elicited by the 25 and 50 μg/kg doses in vehicle-infused rats were diminished in rats receiving the 100 nmol/kg/min infusion of L-CSNO. The increase in fR elicited by the 10 μg/kg dose of fentanyl in vehicle-infused rats was smaller in rats receiving 200 nmol/kg/min infusion of L-CSNO, and the decreases in fR elicited by the 25 and 50 μg/kg doses of fentanyl were reversed to increases in fR in the L-CSNO 200 rats. All doses of fentanyl elicited substantial total falls in VT and VE in rats receiving the infusion of vehicle. These decreases in VT and VE were markedly smaller in the rats receiving the infusion of L-CSNO 100. In the rats receiving L-CSNO 200, the decreases in VT were even smaller than L-CSNO 100, and the VE was slightly increased over the 3 doses of fentanyl in this group due to the increases in fR.
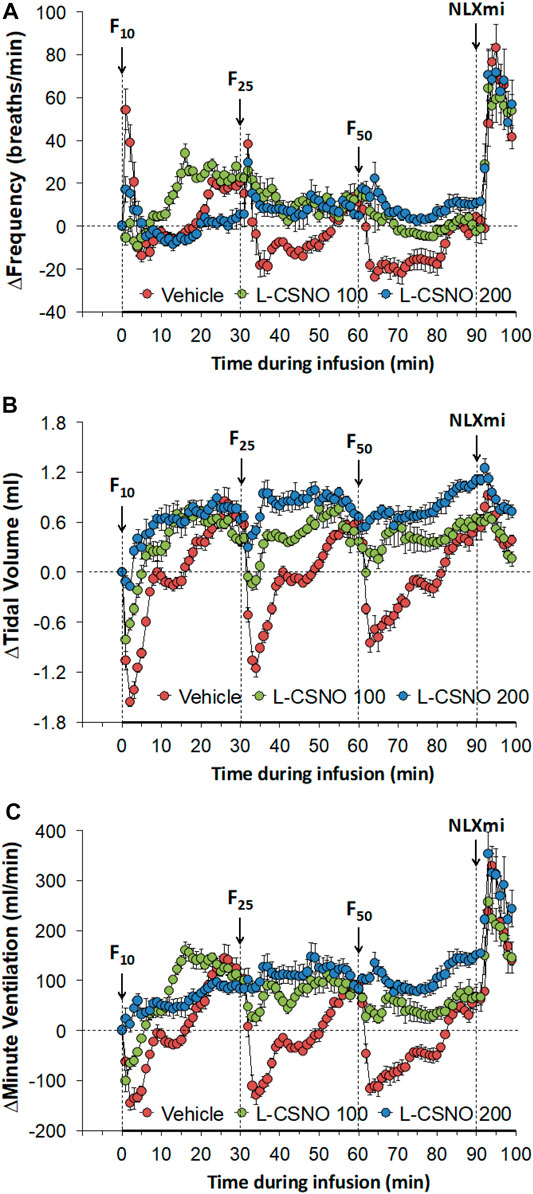
FIGURE 2. Arithmetic changes in baseline frequency of breathing (A), tidal volume (B) and minute ventilation (C) elicited by bolus injections of fentanyl at F10 (10 μg/kg, IV), F25 (25 μg/kg, IV), and F50 (50 μg/kg, IV) in rats receiving continuous infusion of vehicle (20 μl/min, IV), L-CSNO 100 (S-nitroso-L-cysteine, 100 nmol/kg/min, IV) or L-CSNO 200 (S-nitroso-L-cysteine, 200 nmol/kg/min, IV). A bolus injection of naloxone methiodide (NLXmi (2.5 mg/kg, IV) was given at the 90 min timepoint. The data are presented as mean ± SEM. There were 8 rats in each group.
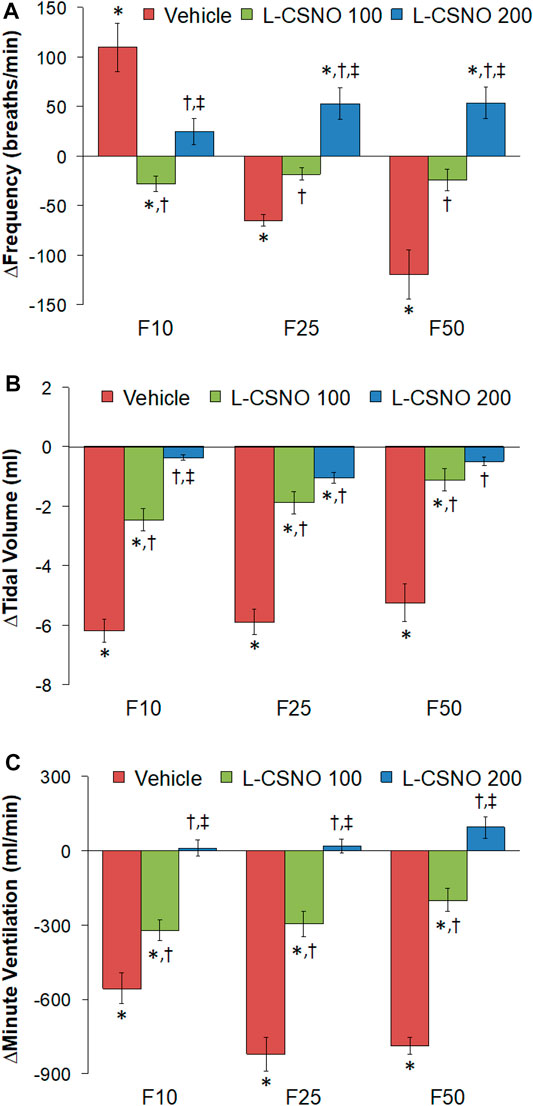
FIGURE 3. Total arithmetic changes in baseline frequency of breathing (A), tidal volume (B), and minute ventilation (C) during the first 5 min following injection of fentanyl at F10 (10 μg/kg, IV), F25 (25 μg/kg, IV), and F50 (50 μg/kg, IV) in rats receiving infusion of vehicle (20 μl/min, IV), L-CSNO 100 (S-nitroso-L-cysteine, 100 nmol/kg/min, IV) or L-CSNO 200 (S-nitroso-L-cysteine, 200 nmol/kg/min, IV). The data are presented as mean ± SEM. There were 8 rats in each group. *p < 0.05, significant change from Pre values. †p < 0.05, L-CSNO 100 or L-CSNO 200 versus vehicle. ‡p < 0.05, L-CSNO 200 versus L-CSNO 100.
Figure 4 summarizes the total arithmetic changes in fR (Figure 4A), VT (Figure 4B) and VE (Figure 4C) from baseline (Pre) values during the entire 30 min period after injection of fentanyl at 10 μg/kg (F10), 25 μg/kg (F25), and 50 μg/kg (F50) in rats receiving infusion of vehicle (20 μl/min, IV), L-CSNO at 100 nmol/kg/min (L-CSNO 100) or 200 nmol/kg/min (L-CSNO 200). The 10 μg/kg dose of fentanyl elicited a cumulative increase in fR, whereas the 25 and 50 μg/kg doses of fentanyl caused cumulative decreases in fR in the vehicle-infused rats. The increase in fR elicited by 10 μg/kg fentanyl in vehicle-infused rats was augmented, and the decreases in fR elicited by the 25 and 50 μg/kg doses of fentanyl were diminished in rats receiving the 100 nmol/kg/min infusion of L-CSNO. The changes in fR elicited by 10, 25, and 50 μg/kg fentanyl were markedly diminished or slightly increased in the rats receiving the 200 nmol/kg/min infusion of L-CSNO, respectively. The changes in VT and VE elicited by the 10 μg/kg dose of fentanyl in vehicle-infused rats were minor compared to the pronounced increases in VT and VE in rats receiving the 100 or 200 nmol/kg/min infusions of L-CSNO. The decreases in VT elicited by the 25 and 50 μg/kg doses of fentanyl in vehicle-infused rats were either increased or abolished in rats receiving the 100 or 200 nmol/kg/min infusions of L-CSNO. Additionally, the decreases in VE elicited by the 25 and 50 μg/kg doses of fentanyl in vehicle-infused rats were markedly diminished in rats receiving the 100 nmol/kg/min infusion of L-CSNO, and increased in rats receiving the 200 nmol/kg/min infusion of L-CSNO.
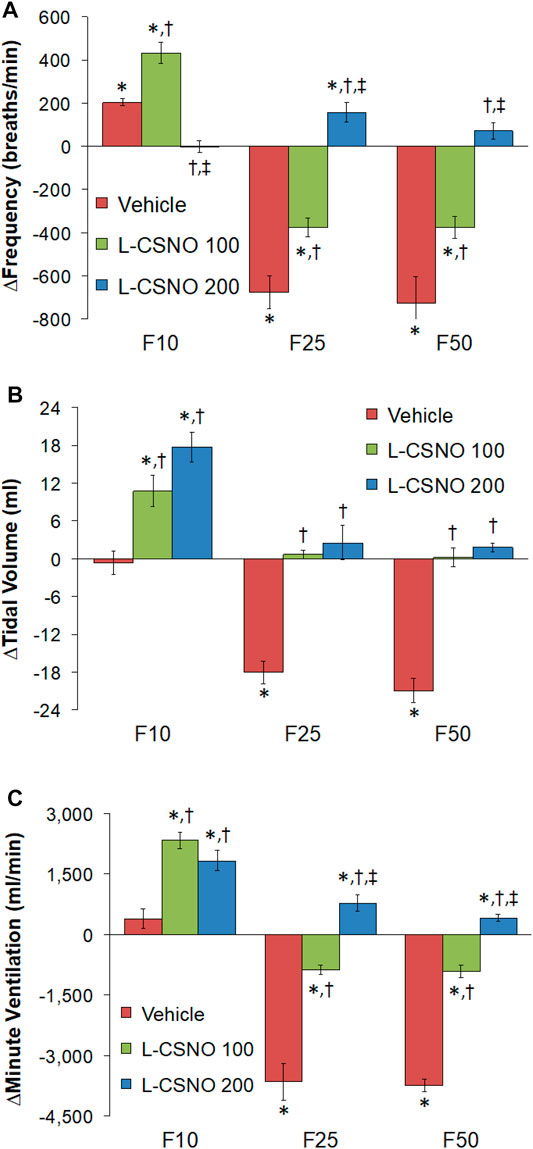
FIGURE 4. Total arithmetic changes in baseline frequency of breathing (A), tidal volume (B), and minute ventilation (C) during the 30 min period following injection of fentanyl at F10 (10 μg/kg, IV), F25 (25 μg/kg, IV) and F50 (50 μg/kg, IV) in rats receiving intravenous infusion of vehicle (20 μl/min, IV), L-CSNO 100 (S-nitroso-L-cysteine, 100 nmol/kg/min, IV) or L-CSNO 200 (S-nitroso-L-cysteine, 200 nmol/kg/min, IV). The data are presented as mean ± SEM. There were 8 rats in each group. *p < 0.05, significant change from Pre values. †p < 0.05, L-CSNO 100 or L-CSNO 200 versus vehicle. ‡p < 0.05, L-CSNO 200 versus L-CSNO 100.
Table 2 summarizes the values of ventilatory parameters at various stages of the L-CSNO infusion experiments. The baseline values (i.e., those prior to commencing the infusions) for fR, VT, and VE were similar to one another (p > 0.05, for all comparisons). The infusion of L-CSNO at 100 nmol/kg/min did not affect fR, VT, and VE compared to vehicle (Figure 1), thus the fR, VT, and VE values recorded at 45 min post start of infusion (i.e., pre-F10 values—or the values prior to the injection of the 10 μg/kg dose of fentanyl) were similar in the L-CSNO 100 group of rats compared to vehicle (Table 2). In contrast, the infusion of L-CSNO at 200 nmol/kg/min elevated VT and VE, whereas it did not change fR, for the pre-F10 values (Table 2; Figure 1). Additionally, both Table 2 and Figure 1 show that the values of fR, VT, and VE were elevated equally immediately prior to injection of the 25 and 50 μg/kg doses of fentanyl in the rats receiving the infusions of vehicle or L-CSNO (100 or 200 nmol/kg/min, IV), with the exception of fR for the L-CSNO 200 group immediately prior to injection of the 25 μg/kg dose of fentanyl, in which fR was similar to baseline. Therefore, these elevations in ventilatory parameters were evidently due to the injections of fentanyl with minimal differences occurring because of the presence of L-CSNO.
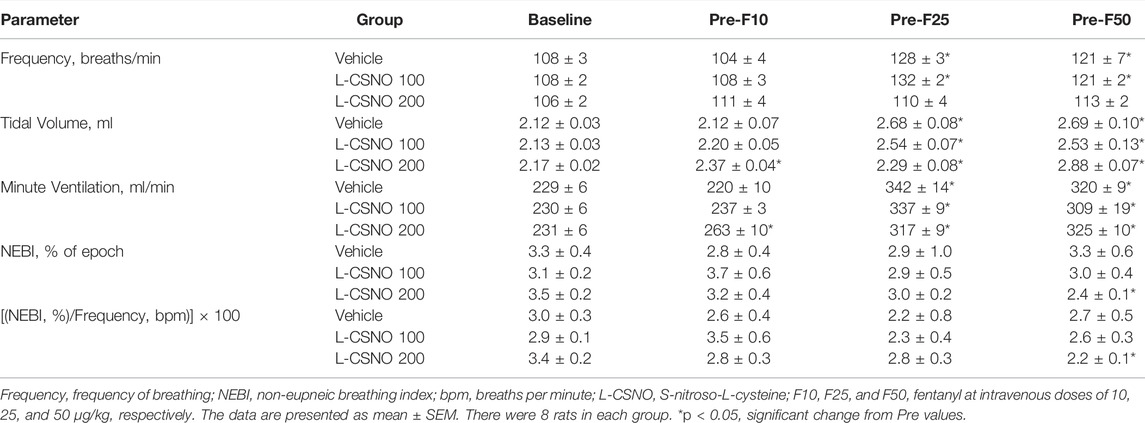
TABLE 2. Baseline parameters at the beginning of the experiments (Pre) and prior to each injection of fentanyl (F10, F25, and F50) in the L-CSNO infusion experiments.
The arithmetic changes in Pre values (i.e., those prior to any drug administration) from values just before injection of fentanyl at F10 (10 μg/kg, IV), F25 (25 μg/kg, IV), and F50 (50 μg/kg, IV) in rats receiving infusion of vehicle (20 μl/min, IV), L-CSNO 100 (S-nitroso-L-cysteine, 100 nmol/kg/min, IV) or L-CSNO 200 (S-nitroso-L-cysteine, 200 nmol/kg/min, IV) are provided in Figure 5. We see significant arithmetic changes for fR, VT, and VE with the vehicle and L-CSNO 100 infusions for F25 and F50. We see no significant changes for fR with the L-CSNO 200 infusion for F25 and F50, and no significant changes for VE with the L-CSNO 200 infusion for F10. Nevertheless, we do see significant arithmetic changes for VT with the L-CSNO 200 infusion for F10, F25, and F50, and significant arithmetic changes for VE with the L-CSNO 200 infusion for F25 and F50. Additionally, we see no significant changes for fR, VT and VE values for F10 across all three continuous infusions, except VT values with the L-CSNO 200 infusion. The levels of fR prior to injections of the 25 and 50 μg/kg doses of fentanyl were higher than baseline infusion values in rats receiving the 100, but not the 200 nmol/kg/min continuous infusions of L-CSNO. In contrast, the levels of VT and VE prior to the injections of the 25 and 50 μg/kg doses of fentanyl were higher than baseline infusion values in the rats receiving both the 100 or 200 nmol/kg/min infusions of L-CSNO. The cumulative changes in ventilatory parameters elicited by continuous intravenous infusion of vehicle or L-CSNO (L-CSNO 100 and L-CSNO 200) during the 45 min of infusion are shown in Table 3. The values confirm that the infusion of L-CSNO at 200 nmol/kg/min, but not the 100 nmol/kg/min, caused significant cumulative increases in VT and VE, but not fR.
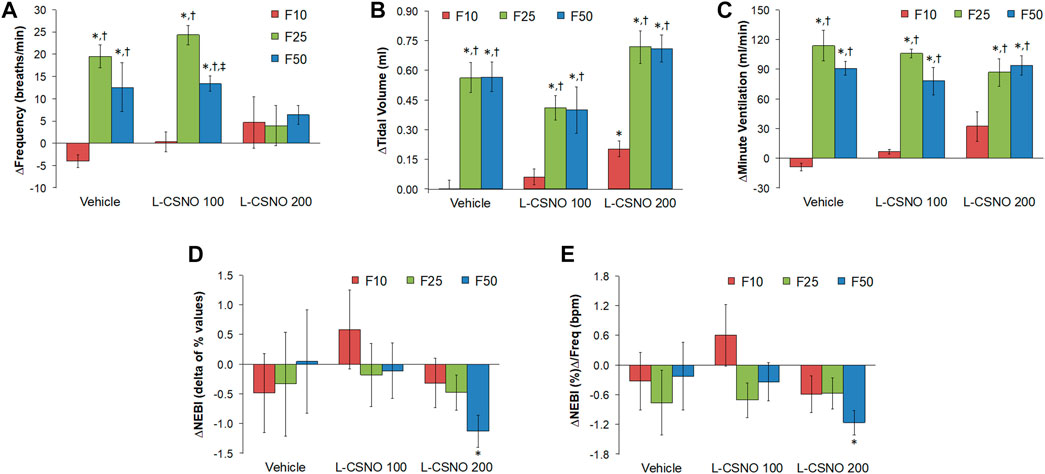
FIGURE 5. Arithmetic changes in Pre values (i.e., those prior to any drug administration) from values just before injection of fentanyl at F10 (10 μg/kg, IV), F25 (25 μg/kg, IV), and F50 (50 μg/kg, IV) in rats that were receiving infusion of vehicle (20 μl/min, IV), L-CSNO 100 (S-nitroso-L-cysteine, 100 nmol/kg/min, IV) or L-CSNO 200 (S-nitroso-L-cysteine, 200 nmol/kg/min, IV). (A) Frequency of breathing. (B) Tidal volume. (C) Minute ventilation. (D) Non-eupneic breathing index (NEBI). (E) NEBI/Frequency of breathing (NEBI/Freq). The data are presented as mean ± SEM. There were 8 rats in each group. *p < 0.05, significant change from Pre values. †p < 0.05, F25 or F50 versus F10. ‡p < 0.05, L-CSNO 200 versus L-CSNO 100.
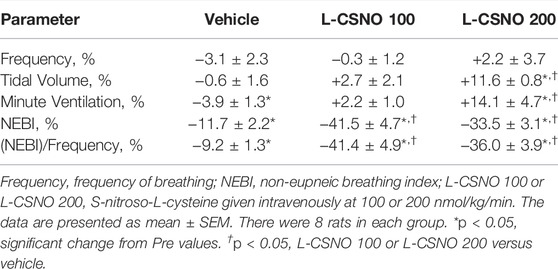
TABLE 3. Cumulative changes in Pre ventilatory parameters elicited by the continuous intravenous infusion of vehicle or S-nitroso-L-cysteine (L-CSNO) during the 45 min of infusion.
Infusions of L-Cysteine or D-CSNO Do Not Blunt the Fentanyl-Induced Changes in fR, VT, and VE
Figure 6 shows the values for fR (Figure 6A), VT (Figure 6B), and VE (Figure 6C) during studies involving injections of fentanyl (10, 25, and 50 μg/kg, IV) in rats receiving infusions of vehicle (20 μl/min, IV), L-cysteine (200 nmol/kg/min, IV) or D-CSNO (200 nmol/kg/min, IV). The infusions of L-cysteine or D-CSNO did not alter baseline values. The injections of fentanyl at 10 μg/kg (F10), 25 μg/kg (F25), and 50 μg/kg (F50) elicited very similar qualitative and quantitative responses to those of vehicle-infused rats described in Figure 1. Thus, the fentanyl responses at 10, 25, and 50 μg/kg are not affected by continuous infusion of L-cysteine or D-CSNO. The arithmetic changes in fR, VT, and VE (expressed as changes from the 45 min infusion timepoint) shown in Figure 7, also confirm that the continuous infusions of L-cysteine or D-CSNO do not change the effects of fentanyl on fR, VT, and VE compared to vehicle-infused rats. Figure 8 summarizes the total (cumulative) arithmetic changes from Pre values during the first 5 min and entire 30 min recording periods in fR (Figures 8A,B, respectively), VT (Figures 8C,D, respectively), and VE (Figures 8E,F, respectively) after injection of fentanyl at F10 (10 μg/kg, IV), F25 (25 μg/kg, IV), and F50 (50 μg/kg, IV) in rats receiving infusion of vehicle (20 μl/min, IV), or L-cysteine or D-CSNO at 200 nmol/kg/min. The total increases in fR over the first 5 min elicited by the 10 μg/kg dose of fentanyl was similar in the three groups as were the total decreases in fR elicited by the 25 and 50 μg/kg doses of fentanyl. The total decreases in VT and VE over the first 5 min elicited by the 10, 25, and 50 μg/kg doses of fentanyl were similar in all three groups. Additionally, similar cumulative changes in fR, VT, and VE were observed over the entire 30 min recording period in the three groups at the 25 and 50 μg/kg doses of fentanyl. At the 10 μg/kg dose of fentanyl, changes in fR, VT, and VE were increased or not changed from Pre values for the three groups. Supplementary Table S2 summarizes the values of ventilatory parameters during the L-cysteine and D-CSNO infusion studies. Baseline values (Pre—those before commencing the infusions) for fR, VT, and VE were similar to one another (p > 0.05, for all comparisons). The infusion of L-cysteine or D-CSNO at 200 nmol/kg/min did not affect fR, VT, and VE at the 45 min infusion timepoint (Pre-F10 values—those prior to injection of the 10 μg/kg dose of fentanyl). The fR, VT, and VE values prior to the injection of the 25 and 50 μg/kg doses of fentanyl (Pre-F25 and Pre-F50) in the rats receiving infusions of vehicle, L-cysteine or D-CSNO were elevated to a similar degree, except the fR values prior to the injection of the 50 μg/kg dose of fentanyl in the rats receiving infusions of vehicle, L-cysteine or D-CSNO. Therefore, these elevations in ventilatory parameters were evidently due to the injections of fentanyl with minimal differences occurring because of the presence of L-cysteine or D-CSNO.
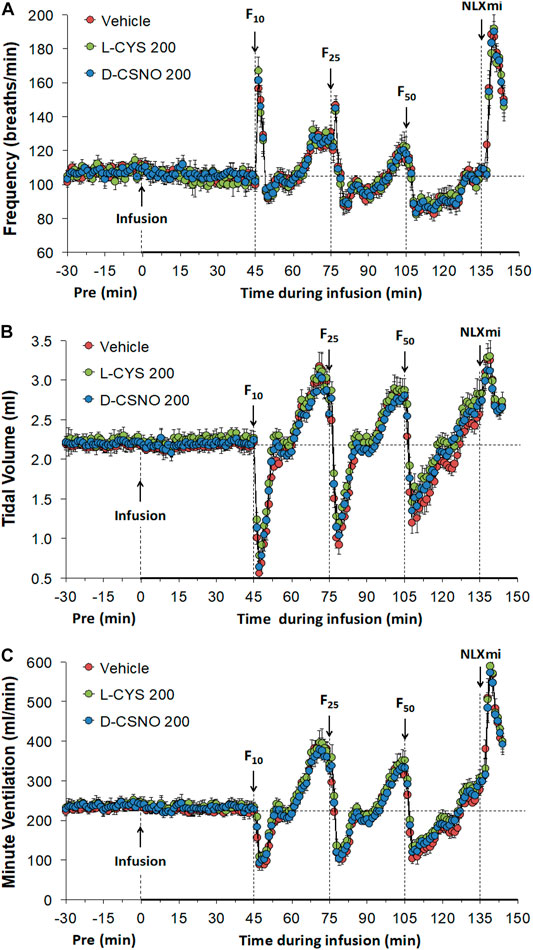
FIGURE 6. Frequency of breathing (A), tidal volume (B) and minute ventilation (C) values. The infusion of vehicle (20 μl/min, IV), L-cysteine (200 nmol/kg/min, IV) or S-nitroso-D-cysteine (D-CSNO, 200 nmol/kg/min, IV) began at time 0. Bolus injections of fentanyl at F10 (10 μg/kg, IV), F25 (25 μg/kg, IV), and F50 (50 μg/kg, IV) were given at 45, 75, and 105 min, respectively. A bolus intravenous injection of naloxone methiodide (NLXmi, 1.5 mg/kg, IV) was given at time 135 min. Data are presented as mean ± SEM. There were 8 rats in each group.
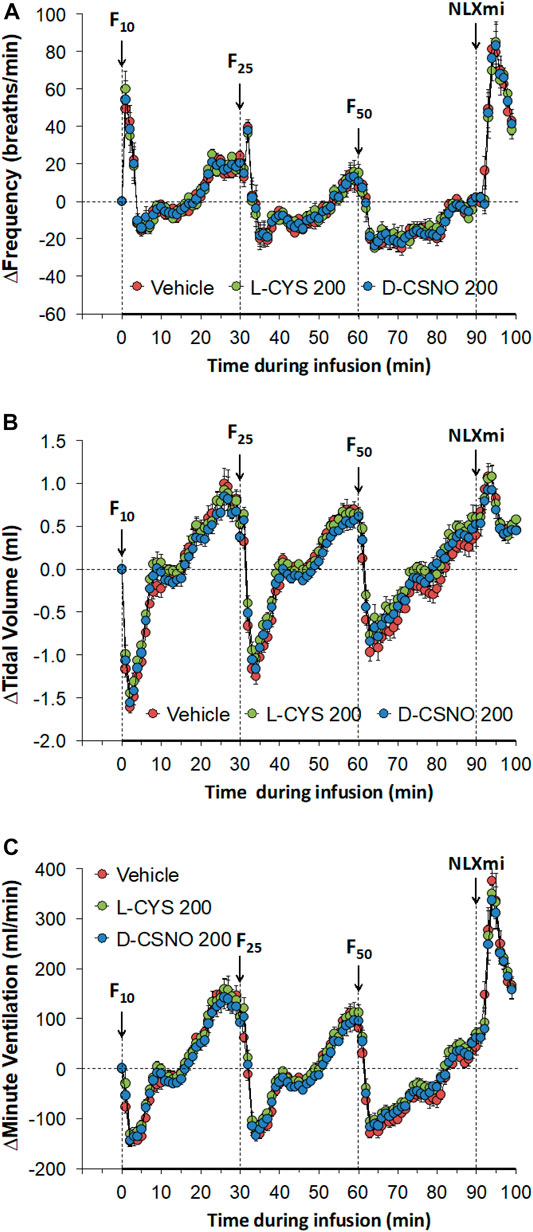
FIGURE 7. Arithmetic changes in baseline frequency of breathing (A), tidal volume (B) and minute ventilation (C) elicited by injections of fentanyl at F10 (10 μg/kg, IV), F25 (25 μg/kg, IV), and F50 (50 μg/kg, IV) in rats receiving continuous infusion of vehicle (20 μl/min, IV), L-cysteine (200 nmol/kg/min, IV) or S-nitroso-D-cysteine (D-CSNO, 200 nmol/kg/min, IV). The data are presented as mean ± SEM. There were 8 rats in each group.
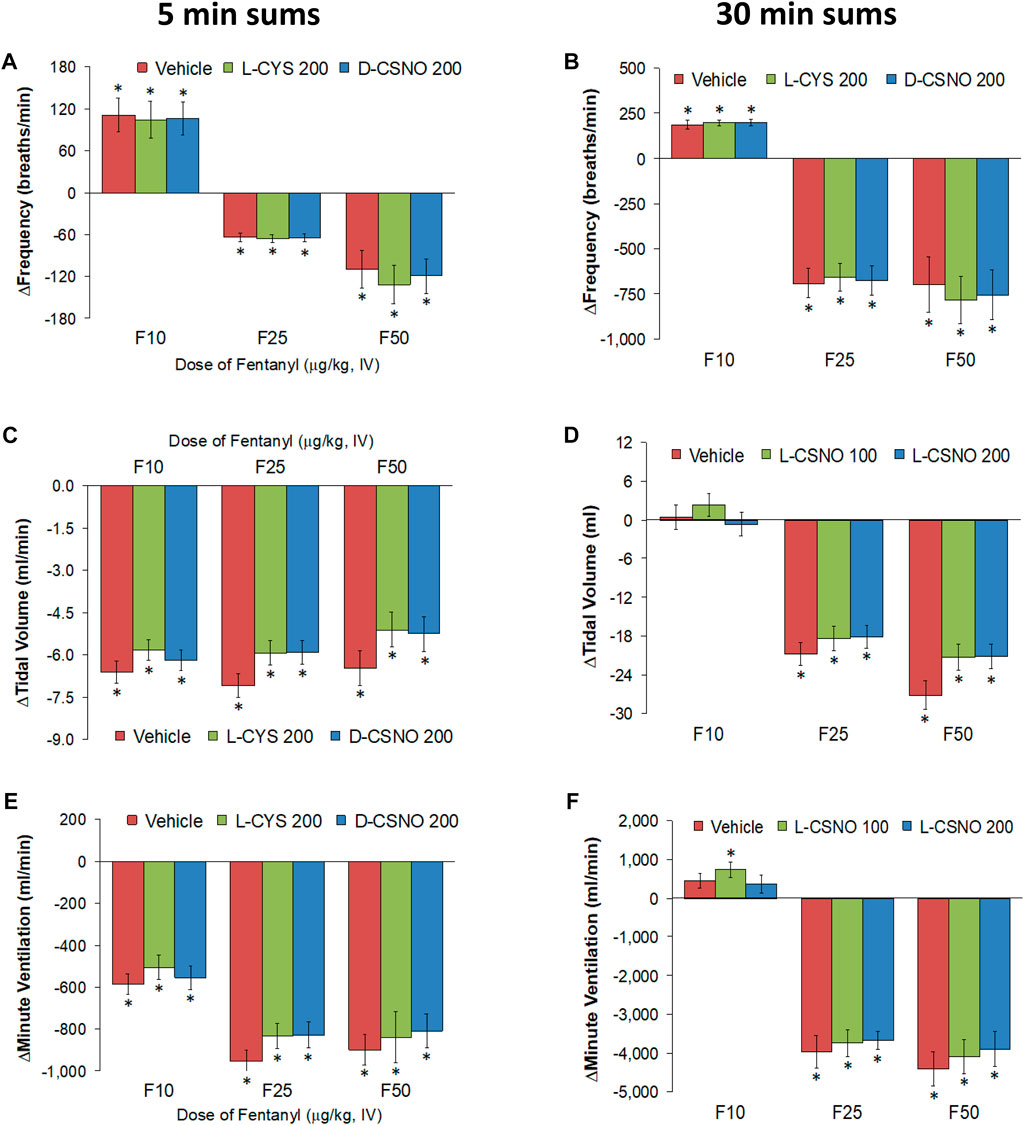
FIGURE 8. Total (cumulative) arithmetic changes in Pre values during the first 5 min and entire 30 min periods in frequency of breathing (A,B), tidal volume (C,D), and minute ventilation (E,F) after injection of fentanyl at F10 (10 μg/kg, IV), F25 (25 μg/kg, IV), and F50 (50 μg/kg, IV) in rats receiving infusion of vehicle (20 μl/min, IV), L-cysteine (200 nmol/kg/min) or D-CSNO (200 nmol/kg/min). The data are presented as mean ± SEM. There were 8 rats in each group. *p < 0.05, significant change from Pre values.
SNO-L-CYS Infusion Blunts the Negative Effects of Fentanyl on Eupneic Breathing
The actual NEBI and NEBI/fR values during various stages of the L-CSNO (100 or 200 nmol/kg/min, IV) infusion studies are presented in Figure 9. Figure 9A shows that the baseline NEBI values in all rats were very low, suggesting that most breaths were eupneic in nature. The infusions of vehicle or L-CSNO at 100 or 200 nmol/kg/min minimally affected resting NEBI levels. In vehicle-infused rats, the injection of the 10, 25, and 50 μg/kg doses of fentanyl elicited transient, but substantial, increases in NEBI of 5–10 min in duration. These detrimental effects of fentanyl were virtually absent in rats receiving 100 or 200 nmol/kg/min infusions of L-CSNO. Figure 9B shows that even when corrected for the levels of fR, the pattern of effects elicited by fentanyl in rats receiving infusions of vehicle or L-CSNO are qualitatively similar to those shown in Figure 9A. The arithmetic changes in NEBI and NEBI/fR (expressed as differences from the 45 min infusion timepoint) shown in Supplementary Figure S1, confirm that the ability of fentanyl to deleteriously affect NEBI and NEBI/fR are diminished in rats receiving infusions of L-CSNO 100 or L-CSNO 200. Supplementary Figure S2 summarizes the total (cumulative) arithmetic changes in NEBI and NEBI/fR recorded 5 and 30 min after injection of fentanyl (10, 25, and 50 μg/kg doses, IV) in rats receiving continuous infusions of vehicle (20 μl/min, IV), or L-CSNO (100 or 200 nmol/kg/min, IV). The data clearly shows that L-CSNO 100 and 200 dramatically reduce the ability of fentanyl to destabilize breathing. As shown in Supplementary Figures S3, S4 the ability of fentanyl (10, 25, and 50 μg/kg doses, IV) to deleteriously affect NEBI and NEBI/fR was not altered by infusions of L-cysteine (200 nmol/kg/min, IV) or D-CSNO (200 nmol/kg/min, IV).
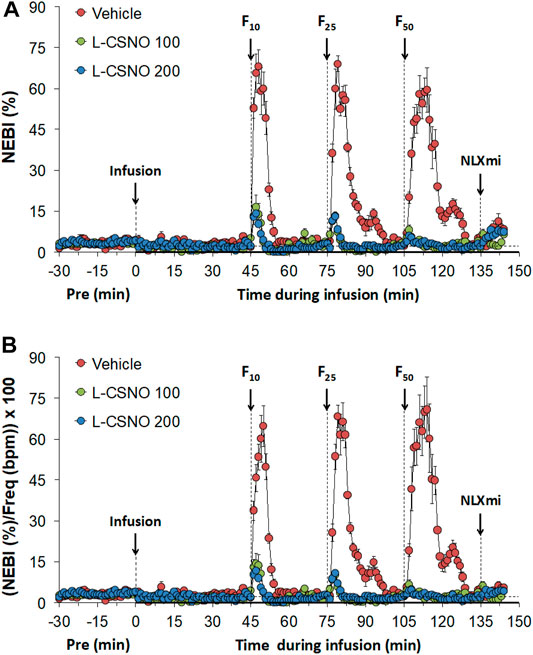
FIGURE 9. Non-eupneic breathing index (NEBI) (A) and NEBI/Frequency of breathing (NEBI/Freq) (B) values. The infusion of vehicle (20 μl/min, IV), L-CSNO 100 (S-nitroso-L-cysteine, 100 nmol/kg/min, IV) or L-CSNO 200 (S-nitroso-L-cysteine, 200 nmol/kg/min, IV) commenced at time 0. Bolus intravenous injections of fentanyl at F10 (10 μg/kg, IV), F25 (25 μg/kg, IV), and F50 (50 μg/kg, IV) were given at 45, 75, and 105 min, respectively. A bolus intravenous injection of naloxone methiodide (NLXmi, 1.5 mg/kg, IV) was given at time 135 min. The data are presented as mean ± SEM. There were 8 rats in each group.
Profound Effects of NLXmi in Fentanyl-Injected Rats
Rather remarkably, the injection of NLXmi (1.5 mg/kg, IV) elicited a prompt and substantial rise in fR in vehicle-infused rats (Figure 1). This effect of NLXmi was strictly related to administration of prior injections of fentanyl, since the injection of NLXmi (1.5 mg/kg, IV) elicited minimal changes in fR, VT, and VE values in rats receiving the infusions of vehicle, but which received injections of vehicle instead of fentanyl (Supplementary Table S3). As can be seen in Figure 1, the increases in fR elicited by NLXmi in rats receiving the 100 or 200 nmol/kg/min infusions of L-CSNO were similar to those in vehicle-infused rats (Figure 1A). Due to the more dramatic effect of NLXmi on fR than VT (Figure 1B), the net effect of the administration of NLXmi was a marked increase in VE of similar magnitude in all three groups (Figure 1C). The differences in the Pre values (i.e., those prior to any drug administration) for fR, VT, and VE compared to those values prior to the administration of NLXmi in rats receiving vehicle, L-CSNO 100 and L-CSNO 200 are summarized in Supplementary Table S4. As can be seen, fR values were not changed in rats receiving infusions of vehicle or 100 nmol/kg/min of L-CSNO, whereas fR was elevated in rats receiving the 200 nmol/kg/min infusion of L-CSNO. In addition, VT and VE values were elevated in all three of the groups, but substantially more so in the rats receiving the 200 nmol/kg/min infusion of L-CSNO. The total (cumulative) changes in fR, VT, and VE elicited by the injection of NLXmi are summarized in Figure 10. The injection of NLXmi elicited pronounced increases in VE (Figure 10C) that were entirely due to the increases in fR (Figure 10A), but not in VT (Figure 10B). Conclusively, it is readily apparent that the NLXmi-induced responses were not modified by the infusions of L-CSNO at either 100 or 200 nmol/kg/min infusions (Figure 10).
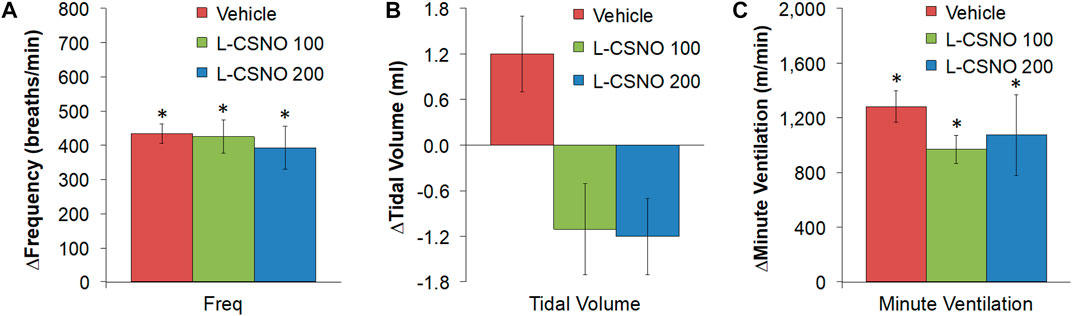
FIGURE 10. Total arithmetic changes in frequency of breathing (A), tidal volume (B), and minute ventilation (C) over the 9-min period following the injection of naloxone methiodide (1.5 mg/kg, IV) in rats receiving infusion of vehicle (20 μl/min, IV), L-CSNO 100 (S-nitroso-L-cysteine, 100 nmol/kg/min, IV), or L-CSNO 200 (S-nitroso-L-cysteine, 200 nmol/kg/min, IV). The data are shown as mean ± SEM. There were 8 rats in each group. *p < 0.05, significant change from Pre values. †p < 0.05, L-CSNO 100 or L-CSNO 200 versus vehicle.
The differences in the Pre values (i.e., those prior to any drug administration) for fR, VT, and VE compared to those values prior to the administration of NLXmi in rats receiving vehicle, L-cysteine or D-CSNO are summarized in Supplementary Table S5. As can be seen, VT and VE, but not fR were elevated prior to injection of NLXmi in the three groups (p > 0.05 for all between group responses). As seen in Figure 6, the responses were qualitatively similar in all three groups, and the injection of NLXmi elicited prompt and substantial increases in fR that were accompanied by smaller increases in VT, which together produced substantial increases in VE. Supplementary Figure S5, shows that the total (cumulative) responses recorded during the 9 min period following injection of NLXmi were similar in all groups for fR and VE, whereas the total responses for VT were smaller in rats receiving infusions of L-cysteine or D-CSNO than those receiving vehicle infusion. As seen in Supplementary Figure S6, the total changes in NEBI elicited by the injection of NLXmi in rats receiving the 100 or 200 nmol/kg/min infusions of L-CSNO (Supplementary Figure S6A) or the 200 nmol/kg/min infusions of L-cysteine or D-CSNO (Supplementary Figure S6C) were not different from their respective vehicle-infusion groups. However, upon correcting the changes in NEBI for the levels of fR, it was evident that the NLXmi-induced changes in NEBI/fR were somewhat greater in rats receiving the 200 nmol/kg/min infusions of L-CSNO (Supplementary Figure S6B) or infusion of L-cysteine (Supplementary Figure S6D).
L-CSNO, but Not L-Cysteine or D-CSNO Promotes the Antinociceptive Actions of Fentanyl
The tail-flick latency (TFL) values of the five treatment groups for the entire experimental protocol are summarized in Supplementary Table S6. The baseline values of each treatment group were similar to one another (p > 0.05, for all comparisons). In addition, none of the infusions altered TFL values as recorded at 60 min of infusion, which was immediately preceding injection of the 10 μg/kg dose of fentanyl (p > 0.05, for all comparisons based on arithmetic changes). Nonetheless, the arithmetic changes because of all the five treatments are summarized in Supplementary Table S7. The changes in TFL expressed as percent of maximum possible effect (%MPE) are summarized in Figure 11. As seen in Figure 11A, the antinociceptive effects of the 10, 25, and 50 μg/kg doses of fentanyl recorded 15 min after injection were similar in all groups that received fentanyl (i.e., all those except for Vehicle 1, which received injections of vehicle instead). As seen in Figure 11B, the antinociceptive effects of the 10 and 25 μg/kg doses of fentanyl recorded 30 min after injection were clearly less than those recorded at 15 min for all groups except the group of rats receiving infusion of L-CSNO. The antinociceptive effect of the 50 μg/kg dose of fentanyl recorded 30 min after injection was clearly similar to the effect recorded at 15 min for all groups, except Vehicle 1 (Figure 11B). Therefore, it appears that the antinociceptive actions of fentanyl were augmented by L-CSNO at the lower doses of fentanyl, whereas they were not affected by L-cysteine or D-CSNO at either the low or high doses of fentanyl. As summarized in Figure 12, the bolus injection of NLXmi did not affect TFL in rats that received an infusion of vehicle plus three bolus injections of vehicle (Vehicle 1). In contrast, the injection of NLXmi elicited a significant reduction in TFL in the rats that received the injections of fentanyl, namely, the Vehicle 2 rats, L-CSNO rats, L-cysteine rats and D-CSNO rats, as recorded 15 and 30 min post-NLXmi injection. The decrease in TFL at 15 min was less in the L-CSNO rats than in the Vehicle 2, L-cysteine or D-CSNO rats.
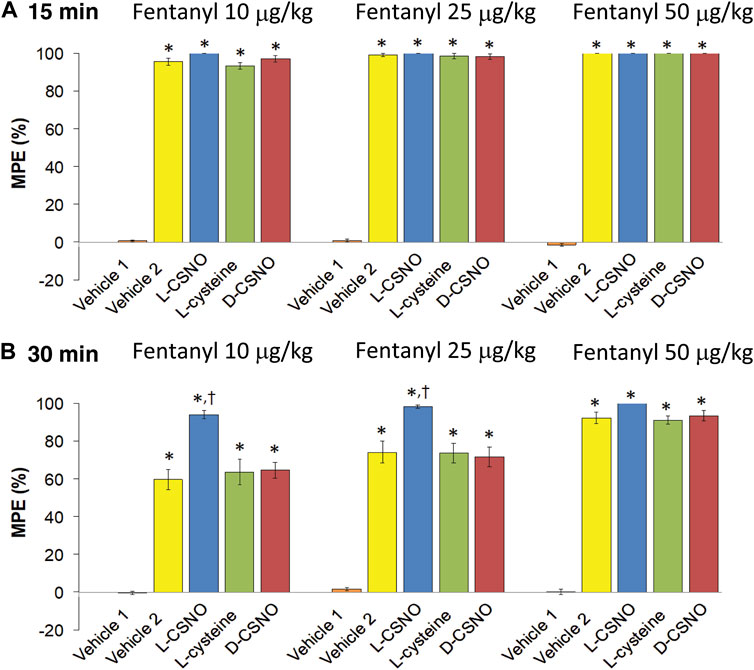
FIGURE 11. Changes in tail-flick latencies 15 min (A) and 30 min (B) following injection of fentanyl (10, 25, and 50 μg/kg, IV) expressed as maximal possible effect (MPE, %). Vehicle 1 rats received an infusion of vehicle, three injections of vehicle and then an injection of NLXmi (2.5 mg/kg, IV) 30 min after the third injection of vehicle. Vehicle 2 rats received an infusion of vehicle for 60 min before receiving injections of 10, 25, or 50 μg/kg doses of fentanyl given 30 min apart and then injection of vehicle, 30 min after injection of 50 μg/kg dose of fentanyl. The groups denoted as L-CSNO, L-cysteine or D-CSNO received infusions at 200 nmol/kg/min for 60 min before receiving injections of 10, 25, or 50 μg/kg doses of fentanyl given 30 min apart and an injection of NLXmi (2.5 mg/kg, IV) 30 min after injection of 50 μg/kg dose of fentanyl. The data are presented as mean ± SEM. There were 6 rats in each group. *p < 0.05, significant change from Pre values. †p < 0.05, L-CSNO versus Vehicle 2, L-cysteine and D-CSNO.
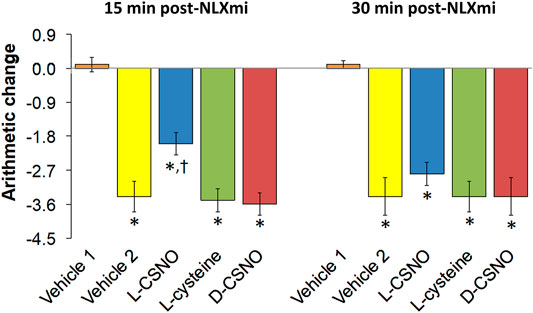
FIGURE 12. Changes in tail-flick latencies 15 min (A) and 30 min (B) following injection of naloxone methiodide (NLXmi, 2.5 mg/kg, IV) given 30 min after the injection of vehicle (Vehicle 1) or fentanyl (50 μg/kg, IV). The group denoted Vehicle 1 received an infusion of vehicle, three injections of vehicle and then NLXmi (2.5 mg/kg, IV) 30 min after the third injection of vehicle. The group denoted Vehicle 2 received an infusion of vehicle for 60 min before injection with 10, 25, or 50 μg/kg doses of fentanyl given 30 min apart and then an injection of vehicle 30 min after injection of 50 μg/kg dose of fentanyl. Infusion groups denoted as L-CSNO (S-nitroso-L-cysteine, 200 nmol/kg/min), L-cysteine (200 nmol/kg/min) or D-CSNO (S-nitroso-D-cysteine, 200 nmol/kg/min) were infused for 60 min before receiving injections of 10, 25, or 50 μg/kg doses of fentanyl given 30 min apart and then an injection of NLXmi (2.5 mg/kg, IV) given 30 min after the injection of 50 μg/kg dose of fentanyl. The data are presented as mean ± SEM. There were 6 rats in each group. *p < 0.05, significant change from Pre values. †p < 0.05, L-CSNO versus Vehicle 2, L-cysteine and D-CSNO.
Discussion
Pharmacological Effects of Fentanyl in Rats Receiving an Infusion of Vehicle
This study demonstrates that consecutive injections of 10, 25, and 50 μg/kg doses of the synthetic opioid, fentanyl (Suzuki and El-Haddad, 2017; Armenian et al., 2018), elicit pronounced biphasic effects on fR, VT, and VE in unanesthetized male Sprague Dawley rats receiving an infusion of vehicle. The first injection of fentanyl (10 μg/kg) elicited a rapid short-lasting increase in fR that, after return to pre-injection levels (i.e., frequency values before injection of a 10 μg/kg dose fentanyl), began to rise steadily to a plateau level that was 15% higher than the pre-injection levels. This plateau took 15 min to occur. Subsequent injections of 25 and 50 μg/kg doses of fentanyl elicited a transient rise in fR followed by a sustained decrease in fR below pre-injection levels. The changes in VT elicited by fentanyl (10, 25, and 50 μg/kg) consisted of a pronounced initial decrease followed by a period of recovery, with levels being close to pre-injection, and then a gradual rise in VT of about 25% above pre-injection levels, after 15 min. Due to similar changes in fR and VT, each dose of fentanyl produced qualitatively similar changes in VE consisting of rapid and large decreases followed by a recovery period and then a rise to about 50% above pre-injection values. While the ability of opioids, such as morphine, to depress breathing and the mechanisms by which this occurs have been extensively studied (Lalley, 2008; Pattinson, 2008; Dahan et al., 2010; Pattinson and Wise, 2016; Baby et al., 2018; Dahan et al., 2018; Bateman et al., 2021; Baby et al., 2021a; Baby et al., 2021b; Ramirez et al., 2021), the sites/mechanisms of action by which fentanyl exerts its cardiorespiratory and antinociceptive effects are less well understood (Laubie et al., 1977; Fone and Wilson, 1986; Mayer et al., 1989; Yamakura et al., 1999; Lalley, 2003; Maegawa and Tonussi, 2003; Griffioen et al., 2004; Mastronicola et al., 2004; Nikolaishvili et al., 2004; Hajiha et al., 2009; Tschirhart et al., 2019; Webster and Rauck, 2021; Ramos-Matos et al., 2022). Mechanisms of action of fentanyl involve activation of μ-ORs in brainstem nuclei controlling cardiorespiratory and nociceptive functions (Laubie et al., 1977; Fone and Wilson, 1986; Lalley, 2003; Griffioen et al., 2004; Nikolaishvili, et al., 2004; Hajiha, et al., 2009; Webster and Rauck, 2021; Ramos-Matos et al., 2022), and in peripheral structures, including the carotid body (Mayer et al., 1989; Henderson et al., 2014; Tschirhart et al., 2019; Ramos-Matos et al., 2022).
Consistent with previous reports (Jenkins et al., 2021; Seckler et al., 2022), each injection of fentanyl in vehicle-infused rats elicited substantial, relatively short-lived increases in occurrence of non-eupneic breaths, as demonstrated by the increases in NEBI and NEBI/fR. Based on the known effects of opioids on breathing patterns (Zutler and Holty, 2011; Nagappa et al., 2017), it is likely that most non-eupneic breathing consists of breath-holds (apneas) although other events, such as type 1 and type 2 sighs, may have occurred. There is conflicting data showing that fentanyl has a strong potential for relief of dyspnea/refractory breathlessness in humans (Simon et al., 2013; Campbell, 2017; Higgins et al., 2020). Nonetheless, the ability of fentanyl to produce apneas is well documented (Willette et al., 1987; Yeadon and Kitchen, 1990; Ren et al., 2009; Zhang et al., 2012a,b; Zhuang et al., 2012; Haouzi et al., 2020; Saunders and Levitt, 2020) by mechanisms including activation of μ-OR in 1) ventrolateral medulla (Willette et al., 1987), 2) the Kölliker-Fuse/parabrachial nucleus complex (Saunders and Levitt, 2020), and 3) the NTS (Zhang et al., 2012a; Zhuang et al., 2012). Additionally, there is evidence for μ-OR-mediated activation of pulmonary C-fiber afferents (Zhang et al., 2012a; Zhang et al., 2012b; Zhuang et al., 2012). Moreover, opioids depress ventilatory responses to hypoxic, hypercapnic, and hypoxic-hypercapnic challenges (Berkenbosch, et al., 1997; May et al., 2013a; May et al., 2013b). Accordingly, the ability of fentanyl to depress breathing, and perhaps to elicit non-eupneic breathing, may also involve the suppression of ventilatory adaptations that occur in response to elevations of arterial blood concentrations of protons, and increases in blood CO2 and decreases in blood O2 (Henderson et al., 2014; Jenkins et al., 2021). These actions of fentanyl may involve activation of μ-ORs in the brainstem, since microinjections of μ-OR agonists into sites in the caudal medullary raphe region inhibit the ventilatory responses to hypercapnic challenges (Zhang et al., 2007), whereas direct application of these agonists in sites in the medullary raphe (Zhang et al., 2009) or commissural NTS (Zhang et al., 2011) reduce ventilatory responses to hypoxic challenges. The ability of the 10, 25, and 50 μg/kg doses of fentanyl in vehicle-infused rats to elicit an increased TFL in unanesthetized rats is consistent with its ability to produce antinociception in rats and other species (Henderson et al., 2014), and to bring pain relief in animals and humans (Hansen et al., 2012; Henderson et al., 2014; Wiffen et al., 2017).
L-CSNO Markedly Diminishes the Negative Effects of Fentanyl on Breathing
L-CSNO is an endogenous SNO (Myers et al., 1990; Bates et al., 1991; Seckler et al., 2020) with an array of activities (Seth and Stamler, 2011; Marozkina and Gaston, 2012; Stomberski et al., 2019; Marozkina and Gaston, 2020), including modulation of ventilatory control systems (Lipton et al., 2001; Gaston et al., 2006; Gaston et al., 2014; Gaston et al., 2020). The infusion concentrations of L-CSNO (100 and 200 nmol/kg/min) were designed to minimally affect baseline ventilatory parameters to see whether cell-signaling events triggered by L-CSNO prevent fentanyl depression of breathing independently of direct effects of L-CSNO on ventilatory control processes that manifest in changes in baseline ventilation. Our preliminary data found that infusions of SNO-L-CYS at 250 nmol/kg/min elevated VE to plateau levels of +48 ± 6% of baseline values (n = 6 rats, p < 0.05), which is consistent with our recent evidence that systemic administration of SNO-L-CYS increases VE (Gaston et al., 2020) via mechanisms including activation of carotid body chemoafferents (Gaston et al., 2020). This increase in baseline VE would have complicated our interpretation of alterations in the efficacy of fentanyl, and so we used lower concentrations of L-CSNO in the hope that despite minimally changes in baseline values, these concentrations would be efficacious against fentanyl. A key finding was that the ventilatory depressant effects and increases in non-eupneic breathing elicited by fentanyl were markedly reduced in rats receiving L-CSNO at 100 and 200 nmol/kg/min, whereas they were not reduced by 200 nmol/kg/min infusions of D-CSNO, or the parent thiol, L-cysteine. The efficacy of the 100 nmol/kg/min infusion of L-CSNO is noteworthy since this infusion did not alter baseline fR, VT or VE. It appears that this low concentration of L-CSNO prevented OR-mediated signaling processes independent of baseline changes in ventilation that this SNO can exert.
Our finding that the antinociceptive actions of fentanyl were augmented in rats receiving L-CSNO, taken together with knowledge that SNOs do not directly interact with μ-ORs (Kokkola et al., 2005), support the possibility that L-CSNO does not block ORs, but that it modulates mechanisms and signaling pathways that mediate the respiratory depressant and antinociceptive actions of opioids (Imam et al., 2018; Stein, 2018; Birdsong and Williams, 2020). Processes by which L-CSNO differentially modulates respiratory depressant and antinociceptive actions of fentanyl are not understood, although the inability of L-cysteine or D-CSNO to exert similar effects suggests that the SNO moiety is essential for activity, and its activity relies upon its stereoselective configuration. L-CSNO stereoselectivity in cardiorespiratory systems has been heavily described (Davisson et al., 1996a; Lewis et al., 1996; Davisson et al., 1997a; Ohta et al., 1997; Hoque et al., 1999; Hoque et al., 2000; Lipton et al., 2001; Lewis et al., 2005a; Lewis et al., 2005b, Lewis et al., 2006a; Gaston et al., 2020) and these activities may involve interactions with membrane-bound proteins, including T-type Ca2+-channels (Joksovic et al., 2007), glutamate (N-methyl-D-aspartate) ion-channel receptors (Travis and Lewis, 2000), and voltage-gated Kv1.1-channels (Gaston et al., 2020). Although stereoselectivity has not been established, L-CSNO activity may involve interactions with Ca2+-activated K+-channels (Bolotina et al., 1994; George and Shibata, 1995; Forrester et al., 2009; Foster et al., 2009; Lima et al., 2010) and Kv1.2- and Kv1.3-channels (Garcia et al., 1995). Stereoselectivity of effects of L-CSNO may involve its ability to enter cells via L-amino acid transporter (L-AT) systems, which do not transport D-CSNO (Nemoto et al., 2003; Li and Whorton, 2007). Intracellular entry of L-CSNO via L-AT would allow it to modulate the activities/functions of signaling pathways (Matsumoto et al., 2003; Burwell et al., 2006; Whalen et al., 2007; Forrester et al., 2009; Broniowska and Hogg, 2010; Iwakiri, 2011; Seth and Stamler, 2011; Piantadosi, 2012; Dejanovic and Schwarz, 2014; Tarasenko, 2015; Lin et al., 2018).
We have reported that NADPH diaphorase histochemistry visualizes S-nitrosylated proteins in peripheral and central structures (Seckler et al., 2020). The finding that morphine administration results in a marked decrease in NADPH diaphorase staining in the brain (Dyuizen et al., 2001; Diuĭzen, et al., 2003; Dyuizen et al., 2004) suggests that opioids may promote the use and/or denitrosylation of S-nitrosylated proteins in the brain, and by analogy in peripheral structures that express NADPH diaphorase staining, such as the carotid body-carotid sinus nerve terminal complex (Atanasova et al., 2020). Establishing higher levels of S-nitrosylated proteins may countermand the ability of opioids to reduce the S-nitrosylation status of cells and this might be an important factor in how L-CSNO infusion diminishes the ability of fentanyl to deleteriously affect breathing. Enhancement of endogenous S-nitrosylation status, by L-CSNO infusion, may enhance the antinociceptive effects of the opioid acting at pain modulation sites.
L-CSNO prevents down regulation/desensitization of G-protein-coupled β-adrenoceptors (Whalen et al., 2000; Whalen et al., 2006), pituitary adenylate-cyclase-activating polypeptide (PACAP) (Whalen et al., 1999), and ligand-gated 5-HT3 ion-channel receptors (Owen et al., 2005) by mechanisms that are independent of the NO-cGMP-protein kinase G signaling pathway. β-adrenoceptors are linked mainly to Gs proteins and to a lesser degree, Gi proteins (Frielle et al., 1989; Rubenstein et al., 1991; Rockman et al., 2002; Mori et al., 2020), whereas PACAP receptors are linked mainly to Gs, but also to Gq and Gi/Go proteins (Van Rampelbergh et al., 1997; Martin et al., 2005). μ-, δ-, and κ-ORs couple to Gi/o-proteins (Roerig et al., 1992; Laugwitz et al., 1993; Prather et al., 1995; Law et al., 2000) with signaling effects including, inhibition of adenylate cyclase (Sharma et al., 1977; Law et al., 2000), inhibition of Ca2+ channels (Hescheler et al., 1987), activation of 1) G protein-coupled inwardly-rectifying K+ channels (North et al., 1987; Henry et al., 1995), and 2) mitogen-activated protein kinase (Fukuda et al., 1996), and stimulation of phospholipase C (Spencer et al., 1997). S-nitrosylation signaling events triggered by L-CSNO directly modulate activities of signaling proteins (George and Shibata, 1995; Gaston et al., 2003; Gonzalez et al., 2009; Lima et al., 2010; Kayki-Mutlu and Koch, 2021). We are examining the literature about S-nitrosylation targets for L-CSNO (Matsumoto et al., 2003; Burwell et al., 2006; Forrester et al., 2009; Foster et al., 2009; Lima et al., 2010; Numajiri et al., 2011; Seth and Stamler, 2011; Haldar and Stamler, 2013; Shao et al., 2016; Stomberski et al., 2019) to design studies to better understand molecular mechanisms by which L-CSNO affects the actions of fentanyl.
L-CSNO Augments Fentanyl-Induced Antinociception
SNOs modulate G protein-coupled receptor signaling in a receptor-specific and reversible manner (Whalen et al., 1999; Whalen et al., 2007; Kokkola et al., 2005; Nozik-Grayck et al., 2006), whereas neither L-CSNO nor S-nitroso-L-glutathione directly interact with μ-ORs (Kokkola et al., 2005). As such, the ability of L-CSNO infusion to augment the duration of fentanyl-induced analgesia (particularly evident for the 10 and 25 μg/kg doses) may involve modulation of the signaling pathways controlling pain perception that are activated by fentanyl (Hansen et al., 2012; Henderson et al., 2014; Wiffen et al., 2017). The mechanisms by which L-CSNO infusion promotes fentanyl analgesia may involve the release NO because of evidence that peripheral antinociceptive actions of fentanyl are mediated via the NO/cyclic-GMP/protein kinase G pathway (Maegawa and Tonussi, 2003), and that opioids, such as morphine, induce pain relief via the NO/cyclic-GMP/protein kinase G pathway (Ferreira et al., 1991; Leánez et al., 2009; Cunha et al., 2010; Cury et al., 2011; Gomes et al., 2020). There is also substantial evidence that NO is a cGMP/protein kinase G-dependent pronociceptive agent that drives nociceptive hyper-sensitivity (Tegeder et al., 2011), and that NOS inhibitors reduce nociceptive behaviors (Hao and Xu, 1996; Aley et al., 1998; Levy and Zochodne, 1998; Chen and Levine, 1999). The ability of L-CSNO to augment fentanyl antinociception may involve L-CSNO activation and/or S-nitrosylation of functional proteins. S-nitrosylation of nociceptive signaling proteins include 1) transient receptor potential channels, 2) voltage-gated channels, 3) G-protein-coupled receptors, 4) glutamate receptors, 5) redoxins, and 6) pro-inflammatory enzymes (Tegeder et al., 2011). S-nitrosylation of these proteins requires a permissive redox state of sulfur atoms, and includes changes of ion channel gating properties, modulation of membrane fusion and fission processes that regulate insertion/removal of receptors/ion-channels into plasma membranes, and alteration of protein ubiquitination status and protein degradation. The roles of NO and SNO-dependent signaling events in the ability of L-CSNO to augment fentanyl-induced antinociception awaits further study.
Novel Effects of NLXmi in Fentanyl-Treated Rats
The novel finding that NLXmi elicited a pronounced increase in fR, whereas it decreased VT in vehicle-infused rats that received injections of fentanyl (10, 25, and 50 μg/kg), but not injections of vehicle, raises important questions. First, injections of fentanyl elicited excitatory and inhibitory effects on breathing via peripheral and/or central pathways that sub-serve the opposing actions of fentanyl. While the adverse effects of opioids on breathing have been extensively characterized (Dahan et al., 2010; Dahan et al., 2018; Gaston et al., 2020), there is also evidence that lower doses of opioids, including fentanyl, morphine, methadone, dermorphin, and other μ- and δ-OR agonists, stimulate ventilation (Metcalfe et al., 1980; Hassen et al., 1982; Haddad et al., 1984; Hurle et al., 1985; Schaeffer and Haddad, 1985; Szeto et al., 1988; Paakkari et al., 1990; Szeto et al., 1991; Paakkari et al., 1993; Henderson et al., 2013; Henderson et al., 2014). Respiratory stimulation occurred upon many sites/methods of administration (Metcalfe et al., 1980; Paakkari et al., 1993; Henderson et al., 2013; Henderson et al., 2014), including NTS (Hassen et al., 1982), ventral medullary and dorsal pontine surfaces (Hurle et al., 1985), lateral ventricles (Paakkari et al., 1990; Paakkari et al., 1993) and fourth ventricle (Haddad et al., 1984; Schaeffer and Haddad, 1985) of the brain, and fetal inferior vena cava (Szeto et al., 1988; Szeto et al., 1991).
NLXmi increased fR in fentanyl-injected rats at 30 min post-fentanyl 50 μg/kg injection when resting fR was back to baseline. An explanation for the effects of NLXmi in fentanyl-treated rats is that repeated injections of fentanyl activate opposing pathways, one that promotes and one that depresses breathing, and that these opposing drives result in a normal fR. The inhibitory pathway that controls fR may involve NLXmi-sensitive peripheral OR signaling processes, and the effects of this excitatory pathway expressed after injection of NLXmi on fR may be due to non-OR-driven pathways in the periphery and/or to central OR and/or non-OR-driven systems. In contrast to fR, VT was elevated at the time NLXmi was given to fentanyl-injected rats receiving vehicle infusion. The decrease in VT elicited by NLXmi suggests that tonically active peripheral OR-driven systems were involved in the increases in VT after injections of fentanyl. It is difficult to speculate on peripheral mechanisms involved in responses to NLXmi, but differential effects occur via OR signaling cascades in structures without blood-brain barriers, including the area postrema (Guan et al., 1999), anteroventral region of the third ventricle (Feuerstein et al., 1985), subfornical organ (Atwah and Kuhar, 1977), and carotid bodies (McQueen and Ribeiro, 1980; Zimpfer et al., 1983; Kirby and McQueen, 1986; Mayer et al., 1989; Sarton et al., 1999), noting that sex differences in morphine-induced ventilatory depression reside in peripheral chemoreflex loops (Sarton et al., 1999). Despite the effects of L-CSNO on immediate changes in fR, VT, and VE elicited by fentanyl, the ventilatory responses elicited by NLXmi in rats receiving L-CSNO infusion were similar to those in vehicle-infused rats. Whatever mechanisms are involved in recruiting fentanyl-induced tonically active excitatory/inhibitory ventilatory control pathways, they may not be subject to control by L-CSNO or downstream events, including S-nitrosylation (Lipton, et al., 1993; Matsumoto et al., 2003; Forrester, et al., 2009). Despite a robust increase in fR, NLXmi elicited minor changes in NEBI and NEBI/fR in fentanyl-injected rats receiving vehicle or L-CSNO. As such, fentanyl did not initiate NLXmi-sensitive control processes that perturb eupneic breathing.
Study Limitations
The main finding that continuous infusion of L-CSNO in unanesthetized adult male Sprague Dawley rats diminishes the detrimental effects fentanyl has on breathing should be confirmed in female rats. There is a major gap in understanding of sex differences in 1) ventilatory control processes and the roles of SNOs in these processes (Palmer et al., 2013a; Getsy et al., 2021), 2) S-nitrosylation events (Brown-Steinke et al., 2010; Shao et al., 2016; Casin and Kohr, 2020), and 3) ventilatory responses to opioids in naïve rats or those subjected to interventions such as, inhibition of NOS (Dahan et al., 1998; Sarton et al., 1999; Hosseini et al., 2011; Gaulden et al., 2021). A more circumspect set of studies in which a single higher dose of fentanyl (e.g., 75 μg/kg) is injected into rats receiving an infusion of L-CSNO may provide more straight-forward results to allow additional interventions (e.g., injection of enzyme inhibitors) aimed at elucidating mechanisms by which L-CSNO has profound effects on the ability of fentanyl to deleteriously affect breathing and NEBI. Studies using centrally active μ-OR antagonists (Dahan et al., 2010) or δ-OR antagonists (Young et al., 2013) will help to define the roles of peripheral and central OR systems associated with the effects of NLXmi in fentanyl-injected rats. Moreover, it would seem essential to establish the potency and efficacy of L-CSNO in dose-response studies to see whether even lower infusion concentrations of L-CSNO will be efficacious against fentanyl. As mentioned above, a limitation of this study is the lack of understanding of molecular mechanisms by which L-CSNO modulates the ventilatory and antinociceptive actions of fentanyl. Therefore, we are currently determining whether systemic injections of fentanyl generate SNOs in central or peripheral structures in rats using capacitative sensor technology (Seckler et al., 2017), and whether infusions of fentanyl change the S-nitrosylation status of central/peripheral structures by NADPH diaphorase histochemistry, which visualizes S-nitrosylated proteins (Seckler et al., 2020).
Conclusion
This study reports that the deleterious effects of fentanyl on fR, VT, VE, and ventilatory, stability, as defined by the increase in NEBI, were diminished in unanesthetized rats receiving a continuous intravenous infusion of L-CSNO, but not in those receiving continuous intravenous infusion of L-cysteine or D-CSNO. Additionally, the antinociceptive actions of fentanyl were augmented by L-CSNO, but not L-cysteine or D-CSNO. As such, we conclude that the ability of L-CSNO to exert these therapeutically relevant responses against the detrimental effects of fentanyl may involve the activation of stereoselective signal transduction processes, which may include the activation of membrane signaling proteins (Travis and Lewis, 2000; Joksovic et al., 2007; Gaston et al., 2020) and/or intracellular entry via the L-amino acid transporter (Li et al., 2000; Nemoto et al., 2003; Li and Whorton, 2007), which allows for modulation of intracellular signaling pathways. Taking into consideration the findings that the peripherally-restricted μ-OR antagonist, NLXmi, substantially reduces ventilatory and antinociceptive responses elicited by fentanyl (Henderson et al., 2014), we conclude that L-CSNO modulation of the effects of fentanyl involve interactions with both peripheral and central μ-OR signaling pathways.
Data Availability Statement
The raw data supporting the conclusion of this article will be made available by the authors, without undue reservation.
Ethics Statement
The animal study was reviewed and approved by the Institutional Animal Care and Use Committees of the University of Virginia, Case Western Reserve University, and Galleon Pharmaceuticals, Inc.
Author Contributions
The study was originated and constructed by PG, SB, RG, BG, Y-HH, JB, AG, JS, and SL. The experiments were performed by PG, RG, JB, TL, and SL. Data sets were collated and statistically analyzed by PG, JB, TL, Y-HH, and SL. The figures and tables were prepared by PG, JB, SB, AG, JS, TL, and SL. All authors contributed to writing of the original version of the manuscript and revision of the final document that was then submitted for publication with the approval of all authors.
Funding
These studies were supported in part by grants from Galleon Pharmaceuticals, Inc. (to SL), NIH PO1HL101871 (to BG and SL) and NIDA U01DA051373 (to SL).
Conflict of Interest
The co-authors SB and RG were employed by Galleon Pharmaceuticals, Inc. The authors declare that this study received funding from Galleon Pharmaceuticals, Inc. All authors state that the leadership of the company was not directly involved in this study as a commercial entity. Only the principal scientists of the company were involved in the study design, collection, analysis, interpretation of data, the writing of this article and the decision to submit it for publication.
The remaining authors declare that the research was conducted in the absence of any commercial or financial relationships that could be construed as a potential conflict of interest.
Publisher’s Note
All claims expressed in this article are solely those of the authors and do not necessarily represent those of their affiliated organizations, or those of the publisher, the editors and the reviewers. Any product that may be evaluated in this article, or claim that may be made by its manufacturer, is not guaranteed or endorsed by the publisher.
Acknowledgments
The authors wish to thank the staff at the animal care facilities at the University of Virginia, Case Western Reserve University and Galleon Pharmaceuticals, Inc., for their expert assistance with the care of the animals. The authors also wish to thank Mr. David Kalergis (CEO, Atelerix Life Sciences) for his help with clinical perspectives related to the present findings and for providing text.
Supplementary Material
The Supplementary Material for this article can be found online at: https://www.frontiersin.org/articles/10.3389/fphar.2022.892307/full#supplementary-material
Abbreviations
SNO, S-nitrosothiol; L-CSNO, S-nitroso-L-cysteine; D-CSNO, S-nitroso-D-cysteine; fR, frequency of breathing; VT, tidal volume; VE, minute ventilation; TFL, tail-flick withdrawal latency; L-NAME, NG-nitro-L-arginine methyl ester; NTS, nucleus tractus solitarius; NO, nitric oxide; NOS, nitric oxide synthase; nNOS, neuronal nitric oxide synthase.
References
Aley, K. O., McCarter, G., and Levine, J. D. (1998). Nitric Oxide Signaling in Pain and Nociceptor Sensitization in the Rat. J. Neurosci. 18, 7008–7014. doi:10.1523/JNEUROSCI.18-17-07008.1998
Anand, P., Hausladen, A., Wang, Y. J., Zhang, G. F., Stomberski, C., Brunengraber, H., et al. (2014). Identification of S-Nitroso-CoA Reductases that Regulate Protein S-Nitrosylation. Proc. Natl. Acad. Sci. U. S. A. 111, 18572–18577. doi:10.1073/pnas.1417816112
Armenian, P., Vo, K. T., Barr-Walker, J., and Lynch, K. L. (2018). Fentanyl, Fentanyl Analogs and Novel Synthetic Opioids: A Comprehensive Review. Neuropharmacology 134, 121–132. doi:10.1016/j.neuropharm.2017.10.016
Atanasova, D. Y., Dandov, A. D., Dimitrov, N. D., and Lazarov, N. E. (2020). Histochemical and Immunohistochemical Localization of Nitrergic Structures in the Carotid Body of Spontaneously Hypertensive Rats. Acta histochem. 122, 151500. doi:10.1016/j.acthis.2019.151500
Atweh, S. F., and Kuhar, M. J. (1977). Autoradiographic Localization of Opiate Receptors in Rat Brain. III. The Telencephalon. Brain Res. 134 (3), 393–405. doi:10.1016/0006-8993(77)90817-4
Baby, S., Gruber, R., Discala, J., Puskovic, V., Jose, N., Cheng, F., et al. (2021a). Systemic Administration of Tempol Attenuates the Cardiorespiratory Depressant Effects of Fentanyl. Front. Pharmacol. 12, 690407. doi:10.3389/fphar.2021.690407
Baby, S. M., Discala, J. F., Gruber, R., Getsy, P. M., Cheng, F., Damron, D. S., et al. (2021b). Tempol Reverses the Negative Effects of Morphine on Arterial Blood-Gas Chemistry and Tissue Oxygen Saturation in Freely-Moving Rats. Front. Pharmacol. 12, 749084. doi:10.3389/fphar.2021.749084
Baby, S. M., Gruber, R. B., Young, A. P., MacFarlane, P. M., Teppema, L. J., and Lewis, S. J. (2018). Bilateral Carotid Sinus Nerve Transection Exacerbates Morphine-Induced Respiratory Depression. Eur. J. Pharmacol. 834, 17–29. doi:10.1016/j.ejphar.2018.07.018
Bateman, J. T., Saunders, S. E., and Levitt, E. S. (2021). Understanding and Countering Opioid‐induced Respiratory Depression. Br. J Pharmacol. doi:10.1111/bph.15580
Batenburg, W. W., de Vries, R., Saxena, P. R., and Danser, A. H. (2004b). L-S-nitrosothiols: Endothelium-Derived Hyperpolarizing Factors in Porcine Coronary Arteries? J. Hypertens. 22, 1927–1936. doi:10.1097/00004872-200410000-00015
Batenburg, W. W., Kappers, M. H., Eikmann, M. J., Ramzan, S. N., de Vries, R., and Danser, A. H. (2009). Light-induced vs. Bradykinin-Induced Relaxation of Coronary Arteries: Do S-Nitrosothiols Act as Endothelium-Derived Hyperpolarizing Factors? J. Hypertens. 27, 1631–1640. doi:10.1097/HJH.0b013e32832bff54
Batenburg, W. W., Popp, R., Fleming, I., de Vries, R., Garrelds, I. M., Saxena, P. R., et al. (2004a). Bradykinin-induced Relaxation of Coronary Microarteries: S-Nitrosothiols as EDHF? Br. J. Pharmacol. 142, 125–135. doi:10.1038/sj.bjp.0705747
Bates, J. N., Harrison, D. G., Myers, P. R., and Minor, R. L. (1991). EDRF: Nitrosylated Compound or Authentic Nitric Oxide. Basic Res. Cardiol. 86 (Suppl. 2), 17–26. doi:10.1007/978-3-642-72461-9_3
Berkenbosch, A., Teppema, L. J., Olievier, C. N., and Dahan, A. (1997). Influences of Morphine on the Ventilatory Response to Isocapnic Hypoxia. Anesthesiology 86, 1342–1349. doi:10.1097/00000542-199706000-00016
Bilfinger, T. V., Fimiani, C., and Stefano, G. B. (1998). Morphine's Immunoregulatory Actions Are Not Shared by Fentanyl. Int. J. Cardiol. 64 (Suppl. 1), S61–S66. doi:10.1016/s0167-5273(98)00037-0
Birdsong, W. T., and Williams, J. T. (2020). Recent Progress in Opioid Research from an Electrophysiological Perspective. Mol. Pharmacol. 98, 401–409. doi:10.1124/mol.119.119040
Bolotina, V. M., Najibi, S., Palacino, J. J., Pagano, P. J., and Cohen, R. A. (1994). Nitric Oxide Directly Activates Calcium-dependent Potassium Channels in Vascular Smooth Muscle. Nature 368, 850–853. doi:10.1038/368850a0
Broniowska, K. A., and Hogg, N. (2010). Differential Mechanisms of Inhibition of Glyceraldehyde-3-Phosphate Dehydrogenase by S-Nitrosothiols and NO in Cellular and Cell-free Conditions. Am. J. Physiol. Heart Circ. Physiol. 299, H1212–H1219. doi:10.1152/ajpheart.00472.2010
Brown-Steinke, K., deRonde, K., Yemen, S., and Palmer, L. A. (2010). Gender Differences in S-Nitrosoglutathione Reductase Activity in the Lung. PLoS One 5, e14007. doi:10.1371/journal.pone.0014007
Burwell, L. S., Nadtochiy, S. M., Tompkins, A. J., Young, S., and Brookes, P. S. (2006). Direct Evidence for S-Nitrosation of Mitochondrial Complex I. Biochem. J. 394, 627–634. doi:10.1042/BJ20051435
Campbell, M. L. (2017). Dyspnea. Crit. Care Nurs. Clin. N. Am. 29, 461–470. doi:10.1016/j.cnc.2017.08.006
Casin, K. M., and Kohr, M. J. (2020). An Emerging Perspective on Sex Differences: Intersecting S-Nitrosothiol and Aldehyde Signaling in the Heart. Redox Biol. 31, 101441. doi:10.1016/j.redox.2020.101441
Chen, X., and Levine, J. D. (1999). NOS Inhibitor Antagonism of PGE2-Induced Mechanical Sensitization of Cutaneous C-Fiber Nociceptors in the Rat. J. Neurophysiol. 81, 963–966. doi:10.1152/jn.1999.81.3.963
Cunha, T. M., Roman-Campos, D., Lotufo, C. M., Duarte, H. L., Souza, G. R., Verri, W. A., et al. (2010). Morphine Peripheral Analgesia Depends on Activation of the PI3Kgamma/AKT/nNOS/NO/KATP Signaling Pathway. Proc. Natl. Acad. Sci. U. S. A. 107, 4442–4447. doi:10.1073/pnas.0914733107
Cury, Y., Picolo, G., Gutierrez, V. P., and Ferreira, S. H. (2011). Pain and Analgesia: The Dual Effect of Nitric Oxide in the Nociceptive System. Nitric Oxide 25, 243–254. doi:10.1016/j.niox.2011.06.004
Dahan, A., Aarts, L., and Smith, T. W. (2010). Incidence, Reversal, and Prevention of Opioid-Induced Respiratory Depression. Anesthesiology 112, 226–238. doi:10.1097/ALN.0b013e3181c38c25
Dahan, A., Sarton, E., Teppema, L., and Olievier, C. (1998). Sex-related Differences in the Influence of Morphine on Ventilatory Control in Humans. Anesthesiology 88, 903–913. doi:10.1097/00000542-199804000-00009
Dahan, A., van der Schrier, R., Smith, T., Aarts, L., van Velzen, M., and Niesters, M. (2018). Averting Opioid-Induced Respiratory Depression without Affecting Analgesia. Anesthesiology 128, 1027–1037. doi:10.1097/ALN.0000000000002184
Davisson, R. L., Bates, J. N., Johnson, A. K., and Lewis, S. J. (1996a). Use-dependent Loss of Acetylcholine- and Bradykinin-Mediated Vasodilation after Nitric Oxide Synthase Inhibition. Evidence for Preformed Stores of Nitric Oxide-Containing Factors in Vascular Endothelial Cells. Hypertension 28, 354–360. doi:10.1161/01.hyp.28.3.354
Davisson, R. L., Johnson, A. K., and Lewis, S. J. (1994). Nitrosyl Factors Mediate Active Neurogenic Hindquarter Vasodilation in the Conscious Rat. Hypertension 23, 962–966. doi:10.1161/01.hyp.23.6.962
Davisson, R. L., Possas, O. S., Murphy, S. P., and Lewis, S. J. (1997a). Neurogenically Derived Nitrosyl Factors Mediate Sympathetic Vasodilation in the Hindlimb of the Rat. Am. J. Physiol. 272, H2369–H2376. doi:10.1152/ajpheart.1997.272.5.H2369
Davisson, R. L., Shaffer, R. A., Johnson, A. K., and Lewis, S. J. (1996c). Stimulation of Lumbar Sympathetic Nerves May Produce Hindlimb Vasodilation via the Release of Pre-formed Stores of Nitrosyl Factors. Neuroscience 72, 881–887. doi:10.1016/0306-4522(96)00090-5
Davisson, R. L., Shaffer, R. A., Johnson, A. K., and Lewis, S. J. (1996b). Use-dependent Loss of Active Sympathetic Neurogenic Vasodilation after Nitric Oxide Synthase Inhibition in Conscious Rats. Evidence for the Presence of Preformed Stores of Nitric Oxide-Containing Factors. Hypertension 28, 347–353. doi:10.1161/01.hyp.28.3.347
Davisson, R. L., Travis, M. D., Bates, J. N., Johnson, A. K., and Lewis, S. J. (1997b). Stereoselective Actions of S-Nitrosocysteine in Central Nervous System of Conscious Rats. Am. J. Physiol. 272, H2361–H2368. doi:10.1152/ajpheart.1997.272.5.H2361
Davisson, R. L., Travis, M. D., Bates, J. N., and Lewis, S. J. (1996d). Hemodynamic Effects of L- and D-S-Nitrosocysteine in the Rat. Stereoselective S-Nitrosothiol Recognition Sites. Circ. Res. 79, 256–262. doi:10.1161/01.res.79.2.256
Dejanovic, B., and Schwarz, G. (2014). Neuronal Nitric Oxide Synthase-dependent S-Nitrosylation of Gephyrin Regulates Gephyrin Clustering at GABAergic Synapses. J. Neurosci. 34, 7763–7768. doi:10.1523/JNEUROSCI.0531-14.2014
Diuĭzen, I. V., Deridovich, I. I., Kurbatskiĭ, R. A., and Shorin, V. V. (2003). NO-ergic Rat Brain Commissural Neurons in the Norm and during Opiate Administration. Morfologiia 123, 24–29.
Durmus, N., Bagcivan, I., Ozdemir, E., Altun, A., and Gursoy, S. (2014). Soluble Guanylyl Cyclase Activators Increase the Expression of Tolerance to Morphine Analgesic Effect. Bratisl. Lek. Listy. 115, 334–339. doi:10.4149/bll_2014_066
Dyuizen, I. V., Deridovich, I. I., Kurbatskii, R. A., and Shorin, V. V. (2004). NO-ergic Neurons of the Cervical Nucleus of the Rat Brain in Normal Conditions and after Administration of Opiates. Neurosci. Behav. Physiol. 34, 621–626. doi:10.1023/b:neab.0000028295.15502.2b
Dyuizen, I. V., Motavkin, P. A., and Shorin, V. V. (2001). Dynamics of NADPH Diaphorase Activity in Raphe Neurons during Chronic Treatment with Opiates. Bull. Exp. Biol. Med. 132, 918–920. doi:10.1023/a:1013151625135
Epstein, M. A., and Epstein, R. A. (1978). A Theoretical Analysis of the Barometric Method for Measurement of Tidal Volume. Respir. Physiol. 32, 105–120. doi:10.1016/0034-5687(78)90103-2
Epstein, R. A., Epstein, M. A., Haddad, G. G., and Mellins, R. B. (1980). Practical Implementation of the Barometric Method for Measurement of Tidal Volume. J. Appl. Physiol. Respir. Environ. Exerc Physiol. 49, 1107–1115. doi:10.1152/jappl.1980.49.6.1107
Erkent, U., Iskit, A. B., Onur, R., and Ilhan, M. (2006). The Effect of Nitric Oxide on Fentanyl and Haloperidol-Induced Catalepsy in Mice. Eur. J. Anaesthesiol. 23, 580–585. doi:10.1017/S0265021506000226
Ferreira, S. H., Duarte, I. D., and Lorenzetti, B. B. (1991). The Molecular Mechanism of Action of Peripheral Morphine Analgesia: Stimulation of the cGMP System via Nitric Oxide Release. Eur. J. Pharmacol. 201, 121–122. doi:10.1016/0014-2999(91)90333-l
Feuerstein, G., Powell, E., and Faden, A. I. (1985). Central Effects of Mu, Delta, and Kappa Receptor Agonists in Hemorrhagic Shock. Peptides 6 (Suppl. 1), 11–13. doi:10.1016/0196-9781(85)90005-1
Fone, K. C., and Wilson, H. (1986). The Effects of Alfentanil and Selected Narcotic Analgesics on the Rate of Action Potential Discharge of Medullary Respiratory Neurones in Anaesthetized Rats. Br. J. Pharmacol. 89, 67–76. doi:10.1111/j.1476-5381.1986.tb11121.x
Forrester, M. T., Thompson, J. W., Foster, M. W., Nogueira, L., Moseley, M. A., and Stamler, J. S. (2009). Proteomic Analysis of S-Nitrosylation and Denitrosylation by Resin-Assisted Capture. Nat. Biotechnol. 27, 557–559. doi:10.1038/nbt.1545
Foster, M. W., Hess, D. T., and Stamler, J. S. (2009). Protein S-Nitrosylation in Health and Disease: a Current Perspective. Trends Mol. Med. 15, 391–404. doi:10.1016/j.molmed.2009.06.007
Foster, M. W., Pawloski, J. R., Singel, D. J., and Stamler, J. S. (2005). Role of Circulating S-Nitrosothiols in Control of Blood Pressure. Hypertension 45, 15–17. doi:10.1161/01.HYP.0000150160.41992.71
Frielle, T., Caron, M. G., and Lefkowitz, R. J. (1989). Properties of the Beta 1- and Beta 2-adrenergic Receptor Subtypes Revealed by Molecular Cloning. Clin. Chem. 35, 721–725. doi:10.1093/clinchem/35.5.721
Fukuda, K., Kato, S., Morikawa, H., Shoda, T., and Mori, K. (1996). Functional Coupling of the δ-, μ-, and κ-opioid Receptors to Mitogen-Activated Protein Kinase and Arachidonate Release in Chinese Hamster Ovary Cells. J. Neurochem. 67, 1309–1316.
Garcia, M. L., Knaus, H. G., Munujos, P., Slaughter, R. S., and Kaczorowski, G. J. (1995). Charybdotoxin and its Effects on Potassium Channels. Am. J. Physiol. 269, C1–C10. doi:10.1152/ajpcell.1995.269.1.C1
Gaston, B., Baby, S. M., May, W. J., Young, A. P., Grossfield, A., Bates, J. N., et al. (2021). D-Cystine di(m)ethyl ester reverses the deleterious effects of morphine on ventilation and arterial blood gas chemistry while promoting antinociception. Sci. Rep. 11, 10038. doi:10.1038/s41598-021-89455-2
Gaston, B., May, W. J., Sullivan, S., Yemen, S., Marozkina, N. V., Palmer, L. A., et al. (2014). Essential Role of Hemoglobin Beta-93-Cysteine in Posthypoxia Facilitation of Breathing in Conscious Mice. J. Appl. Physiol. (1985) 116, 1290–1299. doi:10.1152/japplphysiol.01050.2013
Gaston, B., Singel, D., Doctor, A., and Stamler, J. S. (2006). S-nitrosothiol Signaling in Respiratory Biology. Am. J. Respir. Crit. Care Med. 173, 1186–1193. doi:10.1164/rccm.200510-1584PP
Gaston, B., Smith, L., Bosch, J., Seckler, J., Kunze, D., Kiselar, J., et al. (2020). Voltage-gated Potassium Channel Proteins and Stereoselective S-Nitroso-L-Cysteine Signaling. JCI Insight 5, e134174. doi:10.1172/jci.insight.134174
Gaston, B. M., Carver, J., Doctor, A., and Palmer, L. A. (2003). S-nitrosylation Signaling in Cell Biology. Mol. Interv. 3, 253–263. doi:10.1124/mi.3.5.253
Gaulden, A. D., Burson, N., Sadik, N., Ghosh, I., Khan, S. J., Brummelte, S., et al. (2021). Effects of Fentanyl on Acute Locomotor Activity, Behavioral Sensitization, and Contextual Reward in Female and Male Rats. Drug Alcohol. Depend. 229, 109101. doi:10.1016/j.drugalcdep.2021.109101
George, M. J., and Shibata, E. F. (1995). Regulation of Calcium-Activated Potassium Channels by S-Nitrosothiol Compounds and Cyclic Guanosine Monophosphate in Rabbit Coronary Artery Myocytes. J. Investig. Med. 43, 451–458.
Getsy, P. M., Coffee, G. A., and Lewis, S. J. (2020). The Role of Carotid Sinus Nerve Input in the Hypoxic-Hypercapnic Ventilatory Response in Juvenile Rats. Front. Physiol.11, 613786. doi:10.3389/fphys.2020.613786
Getsy, P. M., Davis, J., Coffee, G. A., May, W. J., Palmer, L. A., Strohl, K. P., et al. (2014). Enhanced Non-eupneic Breathing Following Hypoxic, Hypercapnic or Hypoxic-Hypercapnic Gas Challenges in Conscious Mice. Respir. Physiol. Neurobiol. 204, 147–159. doi:10.1016/j.resp.2014.09.006
Getsy, P. M., Sundararajan, S., May, W. J., von Schill, G. C., McLaughlin, D. K., Palmer, L. A., et al. (2021). Short-term Facilitation of Breathing upon Cessation of Hypoxic Challenge Is Impaired in Male but Not Female Endothelial NOS Knock-Out Mice. Sci. Rep. 11, 18346. doi:10.1038/s41598-021-97322-3
Gomes, F. I. F., Cunha, F. Q., and Cunha, T. M. (2020). Peripheral Nitric Oxide Signaling Directly Blocks Inflammatory Pain. Biochem. Pharmacol. 176, 113862. doi:10.1016/j.bcp.2020.113862
Gonzalez, D. R., Treuer, A., Sun, Q. A., Stamler, J. S., and Hare, J. M. (2009). S-nitrosylation of Cardiac Ion Channels. J. Cardiovasc. Pharmacol. 54, 188–195. doi:10.1097/FJC.0b013e3181b72c9f
Graves, J. E., Bates, J. N., Kooy, N. W., and Lewis, S. J. (2005). Vasodilator Actions of the Endothelium-Derived Relaxing Factor L-S-Nitrosocysteine in Anaesthetized Rats Are Markedly Diminished by Peroxynitrite. Clin. Exp. Pharmacol. Physiol. 32, 1137–1141. doi:10.1111/j.1440-1681.2005.04310.x
Griffioen, K. J., Venkatesan, P., Huang, Z. G., Wang, X., Bouairi, E., Evans, C., et al. (2004). Fentanyl Inhibits GABAergic Neurotransmission to Cardiac Vagal Neurons in the Nucleus Ambiguus. Brain Res. 1007, 109–115. doi:10.1016/j.brainres.2004.02.010
Guan, J. L., Wang, Q. P., and Nakai, Y. (1999). Electron Microscopic Observation of Mu-Opioid Receptor in the Rat Area Postrema. Peptides 20, 873–880. doi:10.1016/s0196-9781(99)00075-3
Haddad, G. G., Schaeffer, J. I., and Chang, K. J. (1984). Opposite Effects of the Delta- and Mu-Opioid Receptor Agonists on Ventilation in Conscious Adult Dogs. Brain Res. 323, 73–82. doi:10.1016/0006-8993(84)90266-x
Hajiha, M., DuBord, M. A., Liu, H., and Horner, R. L. (2009). Opioid Receptor Mechanisms at the Hypoglossal Motor Pool and Effects on Tongue Muscle Activity In Vivo. J. Physiol. 587, 2677–2692. doi:10.1113/jphysiol.2009.171678
Haldar, S. M., and Stamler, J. S. (2013). S-nitrosylation: Integrator of Cardiovascular Performance and Oxygen Delivery. J. Clin. Invest. 123, 101–110. doi:10.1172/JCI62854
Han, Y., Yan, W., Zheng, Y., Khan, M. Z., Yuan, K., and Lu, L. (2019). The Rising Crisis of Illicit Fentanyl Use, Overdose, and Potential Therapeutic Strategies. Transl. Psychiatry 9, 282. doi:10.1038/s41398-019-0625-0
Hansen, M. S., Mathiesen, O., Trautner, S., and Dahl, J. B. (2012). Intranasal Fentanyl in the Treatment of Acute Pain-Aa Systematic Review. Acta Anaesthesiol. Scand. 56, 407–419. doi:10.1111/j.1399-6576.2011.02613.x
Hao, J. X., and Xu, X. J. (1996). Treatment of a Chronic Allodynia-like Response in Spinally Injured Rats: Effects of Systemically Administered Nitric Oxide Synthase Inhibitors. Pain 66, 313–319. doi:10.1016/0304-3959(96)03039-4
Haouzi, P., Mellen, N., McCann, M., Sternick, M., Guck, D., and Tubbs, N. (2020). Evidence for the Emergence of an Opioid-Resistant Respiratory Rhythm Following Fentanyl Overdose. Respir. Physiol. Neurobiol. 277, 103428. doi:10.1016/j.resp.2020.103428
Hashmi-Hill, M. P., Sandock, K., Bates, J. N., Robertson, T. P., and Lewis, S. J. (2007). Flavin Adenine Dinucleotide May Release Preformed Stores of Nitrosyl Factors from the Vascular Endothelium of Conscious Rats. J. Cardiovasc. Pharmacol. 50, 142–154. doi:10.1097/FJC.0b013e31805c1646
Hassen, A. H., Feuerstein, G., and Faden, A. I. (1982). Mu Receptors and Opioid Cardiovascular Effects in the NTS of Rat. Peptides 3, 1031–1037. doi:10.1016/0196-9781(82)90074-2
Henderson, F., May, W. J., Gruber, R. B., Discala, J. F., Puskovic, V., Young, A. P., et al. (2014). Role of Central and Peripheral Opiate Receptors in the Effects of Fentanyl on Analgesia, Ventilation and Arterial Blood-Gas Chemistry in Conscious Rats. Respir. Physiol. Neurobiol. 191, 95–105. doi:10.1016/j.resp.2013.11.005
Henderson, F., May, W. J., Gruber, R. B., Young, A. P., Palmer, L. A., Gaston, B., et al. (2013). Low-dose Morphine Elicits Ventilatory Excitant and Depressant Responses in Conscious Rats: Role of Peripheral μ-opioid Receptors. Open J. Mol. Integr. Physiol. 3, 111–124. doi:10.4236/ojmip.2013.33017
Henry, D. J., Grandy, D. K., Lester, H. A., Davidson, N., and Chavkin, C. (1995). Kappa-opioid Receptors Couple to Inwardly Rectifying Potassium Channels when Coexpressed by Xenopus Oocytes. Mol. Pharmacol. 47, 551–557.
Hervera, A., Negrete, R., Leánez, S., Martín-Campos, J. M., and Pol, O. (2011). Peripheral Effects of Morphine and Expression of μ-opioid Receptors in the Dorsal Root Ganglia during Neuropathic Pain: Nitric Oxide Signaling. Mol. Pain 7, 25. doi:10.1186/1744-8069-7-25
Hescheler, J., Rosenthal, W., Trautwein, W., and Schultz, G. (1987). The GTP-Binding Protein, Go, Regulates Neuronal Calcium Channels. Nature 325, 445–447. doi:10.1038/325445a0
Higgins, E. A., Young, A. M., Cain, J., Dulin, J. D., Miller, M. M., Overstreet, A. N., et al. (2020). Nebulized Fentanyl for Dyspnea: A Retrospective Chart Review. J. Pain Palliat. Care Pharmacother. 34, 77–81. doi:10.1080/15360288.2019.1708529
Hoque, A., Bates, J. N., and Lewis, S. J. (1999). In Vivo evidence that L-S-Nitrosocysteine May Exert its Vasodilator Effects by Interaction with Thiol Residues in the Vasculature. Eur. J. Pharmacol. 384, 169–172. doi:10.1016/s0014-2999(99)00686-x
Hoque, A., Bates, J. N., and Lewis, S. J. (2000). Redox Regulation of S-Nitrosocysteine-Mediated Vasodilation In Vivo. Eur. J. Pharmacol. 408, 195–198. doi:10.1016/s0014-2999(00)00779-2
Hosseini, M., Taiarani, Z., Hadjzadeh, M. A., Salehabadi, S., Tehranipour, M., and Alaei, H. A. (2011). Different Responses of Nitric Oxide Synthase Inhibition on Morphine-Induced Antinociception in Male and Female Rats. Pathophysiology 18, 143–149. doi:10.1016/j.pathophys.2010.05.004
Hurlé, M. A., Mediavilla, A., and Flórez, J. (1985). Differential Respiratory Patterns Induced by Opioids Applied to the Ventral Medullary and Dorsal Pontine Surfaces of Cats. Neuropharmacology 24, 597–606. doi:10.1016/0028-3908(85)90100-5
Imam, M. Z., Kuo, A., Ghassabian, S., and Smith, M. T. (2018). Progress in Understanding Mechanisms of Opioid-Induced Gastrointestinal Adverse Effects and Respiratory Depression. Neuropharmacology 131, 238–255. doi:10.1016/j.neuropharm.2017.12.032
Iwakiri, Y. (2011). S-nitrosylation of Proteins: a New Insight into Endothelial Cell Function Regulated by eNOS-Derived NO. Nitric Oxide 25, 95–101. doi:10.1016/j.niox.2011.04.014
Jaffrey, S. R., Erdjument-Bromage, H., Ferris, C. D., Tempst, P., and Snyder, S. H. (2001). Protein S-Nitrosylation: a Physiological Signal for Neuronal Nitric Oxide. Nat. Cell Biol. 3, 193–197. doi:10.1038/35055104
Jan, W. C., Chen, C. H., Hsu, K., Tsai, P. S., and Huang, C. J. (2011). L-type Calcium Channels and μ-opioid Receptors Are Involved in Mediating the Anti-inflammatory Effects of Naloxone. J. Surg. Res. 167, e263–72. doi:10.1016/j.jss.2010.03.039
Jenkins, M. W., Khalid, F., Baby, S. M., May, W. J., Young, A. P., Bates, J. N., et al. (2021). Glutathione Ethyl Ester Reverses the Deleterious Effects of Fentanyl on Ventilation and Arterial Blood-Gas Chemistry while Prolonging Fentanyl-Induced Analgesia. Sci. Rep. 11, 6985. doi:10.1038/s41598-021-86458-x
Joksovic, P. M., Doctor, A., Gaston, B., and Todorovic, S. M. (2007). Functional Regulation of T-type Calcium Channels by S-Nitrosothiols in the Rat Thalamus. J. Neurophysiol. 97, 2712–2721. doi:10.1152/jn.00926.2006
Kaye, A. D., Hoover, J. M., Ibrahim, I. N., Phelps, J., Baluch, A., Fields, A., et al. (2006). Analysis of the Effects of Fentanyl in the Feline Pulmonary Vascular Bed. Am. J. Ther. 13, 478–484. doi:10.1097/01.mjt.0000178338.43545.3a
Kayki-Mutlu, G., and Koch, W. J. (2021). Nitric Oxide and S-Nitrosylation in Cardiac Regulation: G Protein-Coupled Receptor Kinase-2 and β-Arrestins as Targets. Ijms 22, 521. doi:10.3390/ijms22020521
Kirby, G. C., and McQueen, D. S. (1986). Characterization of Opioid Receptors in the Cat Carotid Body Involved in Chemosensory Depression In Vivo. Br. J. Pharmacol. 88, 889–898. doi:10.1111/j.1476-5381.1986.tb16263.x
Kissin, I., Bright, C. A., and Bradley, E. L. (2000). Acute Tolerance to Continuously Infused Alfentanil: the Role of Cholecystokinin and N-Methyl-D-Aspartate-Nitric Oxide Systems. Anesth. Analg. 91, 110–116. doi:10.1097/00000539-200007000-00021
Kokkola, T., Savinainen, J. R., Mönkkönen, K. S., Retamal, M. D., and Laitinen, J. T. (2005). S-nitrosothiols Modulate G Protein-Coupled Receptor Signaling in a Reversible and Highly Receptor-specific Manner. BMC Cell Biol. 6, 21. doi:10.1186/1471-2121-6-21
Kukreja, R. C., Wei, E. P., Kontos, H. A., and Bates, J. N. (1993). Nitric Oxide and S-Nitroso-L-Cysteine as Endothelium-Derived Relaxing Factors from Acetylcholine in Cerebral Vessels in Cats. Stroke 24, 2010–2015. doi:10.1161/01.str.24.12.2010
Lalley, P. M. (2003). Mu-opioid Receptor Agonist Effects on Medullary Respiratory Neurons in the Cat: Evidence for Involvement in Certain Types of Ventilatory Disturbances. Am. J. Physiol. Regul. Integr. Comp. Physiol. 285, R1287–R1304. doi:10.1152/ajpregu.00199.2003
Lalley, P. M. (2008). Opioidergic and Dopaminergic Modulation of Respiration. Respir. Physiol. Neurobiol. 164, 160–167. doi:10.1016/j.resp.2008.02.004
Laubie, M., Schmitt, H., and Drouillat, M. (1977). Central Sites and Mechanisms of the Hypotensive and Bradycardic Effects of the Narcotic Analgesic Agent Fentanyl. Naunyn Schmiedeb. Arch. Pharmacol. 296, 255–261. doi:10.1007/BF00498691
Laugwitz, K. L., Offermanns, S., Spicher, K., and Schultz, G. (1993). Mu and Delta Opioid Receptors Differentially Couple to G Protein Subtypes in Membranes of Human Neuroblastoma SH-Sy5y Cells. Neuron 10, 233–242. doi:10.1016/0896-6273(93)90314-h
Law, P. Y., Wong, Y. H., and Loh, H. H. (2000). Molecular Mechanisms and Regulation of Opioid Receptor Signaling. Annu. Rev. Pharmacol. Toxicol. 40, 389–430. doi:10.1146/annurev.pharmtox.40.1.389
Leánez, S., Hervera, A., and Pol, O. (2009). Peripheral Antinociceptive Effects of Mu- and Delta-Opioid Receptor Agonists in NOS2 and NOS1 Knockout Mice during Chronic Inflammatory Pain. Eur. J. Pharmacol. 602, 41–49. doi:10.1016/j.ejphar.2008.11.019
Lee, J., Nelson, M. T., Rose, K. E., and Todorovic, S. M. (2013). Redox Mechanism of S-Nitrosothiol Modulation of Neuronal CaV3.2 T-type Calcium Channels. Mol. Neurobiol. 48, 274–280. doi:10.1007/s12035-013-8493-8
Lei, S. Z., Pan, Z. H., Aggarwal, S. K., Chen, H. S., Hartman, J., Sucher, N. J., et al. (1992). Effect of Nitric Oxide Production on the Redox Modulatory Site of the NMDA Receptor-Channel Complex. Neuron 8, 1087–1099. doi:10.1016/0896-6273(92)90130-6
Levy, D., and Zochodne, D. W. (1998). Local Nitric Oxide Synthase Activity in a Model of Neuropathic Pain. Eur. J. Neurosci. 10, 1846–1855. doi:10.1046/j.1460-9568.1998.00186.x
Lewanowitsch, T., and Irvine, R. J. (2002). Naloxone Methiodide Reverses Opioid-Induced Respiratory Depression and Analgesia without Withdrawal. Eur. J. Pharmacol. 445, 61–67. doi:10.1016/s0014-2999(02)01715-6
Lewanowitsch, T., Miller, J. H., and Irvine, R. J. (2006). Reversal of Morphine, Methadone and Heroin Induced Effects in Mice by Naloxone Methiodide. Life Sci. 78, 682–688. doi:10.1016/j.lfs.2005.05.062
Lewis, S. J., Graves, J. E., Bates, J. N., and Kooy, N. W. (2005b). Peroxynitrite Elicits Dysfunction of Stereoselective S-Nitrosocysteine Recognition Sites. J. Cardiovasc. Pharmacol. 46, 637–645. doi:10.1097/01.fjc.0000181717.87204.2f
Lewis, S. J., Hashmi-Hill, M. P., Owen, J. R., Sandock, K., Robertson, T. P., and Bates, J. N. (2006c). ACE Inhibition Restores the Vasodilator Potency of the Endothelium-Derived Relaxing Factor, L-S-Nitrosocysteine, in Conscious Spontaneously Hypertensive Rats. Vasc. Pharmacol. 44, 491–507. doi:10.1016/j.vph.2006.03.003
Lewis, S. J., Hashmi-Hill, M. P., Owen, J. R., Sandock, K., Robertson, T. P., and Bates, J. N. (2006b). The Vasodilator Potency of the Endothelium-Derived Relaxing Factor, L-S-Nitrosocysteine, Is Impaired in Conscious Spontaneously Hypertensive Rats. Vasc. Pharmacol. 44, 476–490. doi:10.1016/j.vph.2006.03.013
Lewis, S. J., Hoque, A., and Bates, J. N. (2005a). Differentiation of L- and D-S-Nitrosothiol Recognition Sites In Vivo. J. Cardiovasc. Pharmacol. 46, 660–671. doi:10.1097/01.fjc.0000181714.94827.5d
Lewis, S. J., Meller, S. T., Brody, M. J., and Gebhart, G. F. (1991). Reduced Nociceptive Effects of Intravenous Serotonin (5-HT) in the Spontaneously Hypertensive Rat. Clin. Exp. Hypertens. A 13, 849–857. doi:10.3109/10641969109042089
Lewis, S. J., Owen, J. R., and Bates, J. N. (2006a). S-nitrosocysteine Elicits Hemodynamic Responses Similar to Those of the Bezold-Jarisch Reflex via Activation of Stereoselective Recognition Sites. Eur. J. Pharmacol. 531, 254–258. doi:10.1016/j.ejphar.2005.11.027
Lewis, S. J., Travis, M. D., and Bates, J. N. (1996). Stereoselective S-Nitrosocysteine Recognition Sites in Rat Brain. Eur. J. Pharmacol. 312, R3–R5. doi:10.1016/0014-2999(96)00607-3
Li, S., and Whorton, A. R. (2007). Functional Characterization of Two S-Nitroso-L-Cysteine Transporters, Which Mediate Movement of NO Equivalents into Vascular Cells. Am. J. Physiol. Cell Physiol. 292, C1263–C1271. doi:10.1152/ajpcell.00382.2006
Li, X., Rose, G., Dongre, N., Pan, H. L., Tobin, J. R., and Eisenach, J. C. (2000). S-nitroso-l-cysteine Releases Norepinephrine in Rat Spinal Synaptosomes. Brain Res. 872, 301–307. doi:10.1016/s0006-8993(00)02551-8
Lima, B., Forrester, M. T., Hess, D. T., and Stamler, J. S. (2010). S-nitrosylation in Cardiovascular Signaling. Circ. Res. 106, 633–646. doi:10.1161/CIRCRESAHA.109.207381
Lin, L., Xu, C., Carraway, M. S., Piantadosi, C. A., Whorton, A. R., and Li, S. (2018). RhoA Inactivation by S-Nitrosylation Regulates Vascular Smooth Muscle Contractive Signaling. Nitric Oxide 74, 56–64. doi:10.1016/j.niox.2018.01.007
Lipton, A. J., Johnson, M. A., Macdonald, T., Lieberman, M. W., Gozal, D., and Gaston, B. (2001). S-nitrosothiols Signal the Ventilatory Response to Hypoxia. Nature 413, 171–174. doi:10.1038/35093117
Lipton, S. A., Choi, Y. B., Pan, Z. H., Lei, S. Z., Chen, H. S., Sucher, N. J., et al. (1993). A Redox-Based Mechanism for the Neuroprotective and Neurodestructive Effects of Nitric Oxide and Related Nitroso-Compounds. Nature 364, 626–632. doi:10.1038/364626a0
Lipton, S. A., Singel, D. J., and Stamler, J. S. (1994). Nitric Oxide in the Central Nervous System. Prog. Brain Res. 103, 359–364. doi:10.1016/s0079-6123(08)61149-8
Liu, L., Yan, Y., Zeng, M., Zhang, J., Hanes, M. A., Ahearn, G., et al. (2004). Essential Roles of S-Nitrosothiols in Vascular Homeostasis and Endotoxic Shock. Cell 116, 617–628. doi:10.1016/s0092-8674(04)00131-x
Liu, T., Schroeder, H. J., Wilson, S. M., Terry, M. H., Romero, M., Longo, L. D., et al. (2016). Local and Systemic Vasodilatory Effects of Low Molecular Weight S-Nitrosothiols. Free Radic. Biol. Med. 91, 215–223. doi:10.1016/j.freeradbiomed.2015.12.009
Lu, Y., Hu, J., Zhang, Y., and Dong, C. (2014). Spinal Neuronal NOS Activation Mediates Intrathecal Fentanyl Preconditioning Induced Remote Cardioprotection in Rats. Int. Immunopharmacol. 19, 127–131. doi:10.1016/j.intimp.2014.01.013
Ludbrook, J. (1998). Multiple Comparison Procedures Updated. Clin. Exp. Pharmacol. Physiol. 25, 1032–1037. doi:10.1111/j.1440-1681.1998.tb02179.x
Lutfy, K. (2020). Opioid Crisis-An Emphasis on Fentanyl Analogs. Brain Sci. 10, 485. doi:10.3390/brainsci10080485
Maegawa, F. A., and Tonussi, C. R. (2003). The L-Arginine/nitric Oxide/cyclic-GMP Pathway Apparently Mediates the Peripheral Antihyperalgesic Action of Fentanyl in Rats. Braz. J. Med. Biol. Res. 36, 1701–1707. doi:10.1590/s0100-879x2003001200012
Marozkina, N., and Gaston, B. (2020). An Update on Thiol Signaling: S-Nitrosothiols, Hydrogen Sulfide and a Putative Role for Thionitrous Acid. Antioxidants (Basel) 9, 225. doi:10.3390/antiox9030225
Marozkina, N. V., and Gaston, B. (2015). Nitrogen Chemistry and Lung Physiology. Annu. Rev. Physiol. 77, 431–452. doi:10.1146/annurev-physiol-021113-170352
Marozkina, N. V., and Gaston, B. (2012). S-nitrosylation Signaling Regulates Cellular Protein Interactions. Biochim. Biophys. Acta 1820, 722–729. doi:10.1016/j.bbagen.2011.06.017
Martin, B., Lopez de Maturana, R., Brenneman, R., Walent, T., Mattson, M. P., and Maudsley, S. (2005). Class II G Protein-Coupled Receptors and Their Ligands in Neuronal Function and Protection. Neuromolecular Med. 7, 3–36. doi:10.1385/nmm:7:1-2:003
Martínez-Ruiz, A., Araújo, I. M., Izquierdo-Álvarez, A., Hernansanz-Agustín, P., Lamas, S., and Serrador, J. M. (2013). Specificity in S-Nitrosylation: a Short-Range Mechanism for NO Signaling? Antioxid. Redox Signal 19, 1220–1235. doi:10.1089/ars.2012.5066
Mastronicola, D., Arcuri, E., Arese, M., Bacchi, A., Mercadante, S., Cardelli, P., et al. (2004). Morphine but Not Fentanyl and Methadone Affects Mitochondrial Membrane Potential by Inducing Nitric Oxide Release in Glioma Cells. Cell. Mol. Life Sci. 61, 2991–2997. doi:10.1007/s00018-004-4371-x
Matsuda, T., Bates, J. N., Lewis, S. J., Abboud, F. M., and Chapleau, M. W. (1995). Modulation of Baroreceptor Activity by Nitric Oxide and S-Nitrosocysteine. Circ. Res. 76, 426–433. doi:10.1161/01.res.76.3.426
Matsumoto, A., Comatas, K. E., Liu, L., and Stamler, J. S. (2003). Screening for Nitric Oxide-dependent Protein-Protein Interactions. Science 301, 657–661. doi:10.1126/science.1079319
May, W. J., Gruber, R. B., Discala, J. F., Puskovic, V., Henderson, F., Palmer, L. A., et al. (2013a). Morphine Has Latent Deleterious Effects on the Ventilatory Responses to a Hypoxic Challenge. Open J. Mol. Integr. Physiol. 3, 166–180. doi:10.4236/ojmip.2013.34022
May, W. J., Henderson, F., Gruber, R. B., Discala, J. F., Young, A. P., Bates, J. N., et al. (2013b). Morphine Has Latent Deleterious Effects on the Ventilatory Responses to a Hypoxic-Hypercapnic Challenge. Open. J. Mol. Integr. Physiol. 3, 134–145. doi:10.4236/ojmip.2013.33019
Mayer, N., Zimpfer, M., Raberger, G., and Beck, A. (1989). Fentanyl Inhibits the Canine Carotid Chemoreceptor Reflex. Anesth. Analg. 69, 756–762. doi:10.1213/00000539-198912000-00012
McHugh, M. L. (2011). Multiple Comparison Analysis Testing in ANOVA. Biochem. Med. Zagreb. 21, 203–209. doi:10.11613/bm.2011.029
McQueen, D. S., and Ribeiro, J. A. (1980). Inhibitory Actions of Methionine-Enkephalin and Morphine on the Cat Carotid Chemoreceptors. Br. J. Pharmacol. 71, 297–305. doi:10.1111/j.1476-5381.1980.tb10939.x
Mehanna, M. M., Domiati, S., Nakkash Chmaisse, H., and El Mallah, A. (2018). Antinociceptive Effect of Tadalafil in Various Pain Models: Involvement of Opioid Receptors and Nitric Oxide Cyclic GMP Pathway. Toxicol. Appl. Pharmacol. 352, 170–175. doi:10.1016/j.taap.2018.05.013
Meller, S. T., Lewis, S. J., Bates, J. N., Brody, M. J., and Gebhart, G. F. (1990). Is There a Role for an Endothelium-Derived Relaxing Factor in Nociception? Brain Res. 531, 342–345. doi:10.1016/0006-8993(90)90798-g
Mellion, B. T., Ignarro, L. J., Myers, C. B., Ohlstein, E. H., Ballot, B. A., Hyman, A. L., et al. (1983). Inhibition of Human Platelet Aggregation by S-Nitrosothiols. Heme-dependent Activation of Soluble Guanylate Cyclase and Stimulation of Cyclic GMP Accumulation. Mol. Pharmacol. 23, 653–664.
Metcalfe, J., Dunham, M. J., Olsen, G. D., and Krall, M. A. (1980). Respiratory and Hemodynamic Effects of Methadone in Pregnant Women. Respir. Physiol. 42, 383–393. doi:10.1016/0034-5687(80)90127-9
Mori, A., Taniai, A., Hasegawa, M., Sakamoto, K., and Nakahara, T. (2020). Involvement of Gi Protein-dependent BKCa Channel Activation in β2-adrenoceptor-mediated Dilation of Retinal Arterioles in Rats. Naunyn Schmiedeb. Arch. Pharmacol. 393, 2043–2052. doi:10.1007/s00210-020-01895-1
Myers, P. R., Minor, R. L., Guerra, R., Bates, J. N., and Harrison, D. G. (1990). Vasorelaxant Properties of the Endothelium-Derived Relaxing Factor More Closely Resemble S-Nitrosocysteine Than Nitric Oxide. Nature 345, 161–163. doi:10.1038/345161a0
Nagappa, M., Weingarten, T. N., Montandon, G., Sprung, J., and Chung, F. (2017). Opioids, Respiratory Depression, and Sleep-Disordered Breathing. Best. Pract. Res. Clin. Anaesthesiol. 31, 469–485. doi:10.1016/j.bpa.2017.05.004
Nakamura, T., and Lipton, S. A. (2016). Protein S-Nitrosylation as a Therapeutic Target for Neurodegenerative Diseases. Trends Pharmacol. Sci. 37, 73–84. doi:10.1016/j.tips.2015.10.002
Nemoto, T., Shimma, N., Horie, S., Saito, T., Okuma, Y., Nomura, Y., et al. (2003). Involvement of the System L Amino Acid Transporter on Uptake of S-Nitroso-L-Cysteine, an Endogenous S-Nitrosothiol, in PC12 Cells. Eur. J. Pharmacol. 458, 17–24. doi:10.1016/s0014-2999(02)02699-7
Nikolaishvili, L. S., Gobechiya, L. S., and Mitagvariya, N. P. (2004). The Effects of Fentanyl and Morphine on Local Blood Flow and Oxygen Tension in the Frontoparietal Cortex and Nucleus Accumbens of the Brain in White Rats. Neurosci. Behav. Physiol. 34, 467–471. doi:10.1023/b:neab.0000022631.46176.6b
North, R. A., Williams, J. T., Surprenant, A., and Christie, M. J. (1987). Mu and Delta Receptors Belong to a Family of Receptors that Are Coupled to Potassium Channels. Proc. Natl. Acad. Sci. U. S. A. 84, 5487–5491. doi:10.1073/pnas.84.15.5487
Nozik-Grayck, E., Whalen, E. J., Stamler, J. S., McMahon, T. J., Chitano, P., and Piantadosi, C. A. (2006). S-nitrosoglutathione Inhibits Alpha1-Adrenergic Receptor-Mediated Vasoconstriction and Ligand Binding in Pulmonary Artery. Am. J. Physiol. Lung Cell. Mol. Physiol. 290, L136–L143. doi:10.1152/ajplung.00230.2005
Numajiri, N., Takasawa, K., Nishiya, T., Tanaka, H., Ohno, K., Hayakawa, W., et al. (2011). On-off System for PI3-Kinase-Akt Signaling through S-Nitrosylation of Phosphatase with Sequence Homology to Tensin (PTEN). Proc. Natl. Acad. Sci. U. S. A. 108, 10349–10354. doi:10.1073/pnas.1103503108
Ohta, H., Bates, J. N., Lewis, S. J., and Talman, W. T. (1997). Actions of S-Nitrosocysteine in the Nucleus Tractus Solitarii Are Unrelated to Release of Nitric Oxide. Brain Res. 746, 98–104. doi:10.1016/s0006-8993(96)01188-2
Ortiz, M. I., Cariño-Cortés, R., and Castañeda-Hernández, G. (2020). Participation of the Opioid Receptor - nitric oxide - cGMP - K+ Channel Pathway in the Peripheral Antinociceptive Effect of Nalbuphine and Buprenorphine in Rats. Can. J. Physiol. Pharmacol. 98, 753–762. doi:10.1139/cjpp-2020-0104
Owen, J. A., Bates, J. N., and Lewis, S. J. (2005). Endogenous Nitrosyl Factors May Inhibit the Desensitization of 5-HT3 Receptors on Vagal Cardiopulmonary Afferents. Brain Res. 1059, 167–172. doi:10.1016/j.brainres.2005.08.023
Ozdemir, E., Bagcivan, I., Durmus, N., Altun, A., and Gursoy, S. (2011). The Nitric Oxide-cGMP Signaling Pathway Plays a Significant Role in Tolerance to the Analgesic Effect of Morphine. Can. J. Physiol. Pharmacol. 89, 89–95. doi:10.1139/y10-109
Paakkari, P., Paakkari, I., Sirén, A. L., and Feuerstein, G. (1990). Respiratory and Locomotor Stimulation by Low Doses of Dermorphin, a Mu1 Receptor-Mediated Effect. J. Pharmacol. Exp. Ther. 252, 235–240.
Paakkari, P., Paakkari, I., Vonhof, S., Feuerstein, G., and Sirén, A. L. (1993). Dermorphin Analog Tyr-D-Arg2-Phe-Sarcosine-Induced Opioid Analgesia and Respiratory Stimulation: the Role of Mu 1-receptors? J. Pharmacol. Exp. Ther. 266, 544–550.
Palmer, L. A., Kimberly deRonde, K., Brown-Steinke, K., Gunter, S., Jyothikumar, V., Forbes, M. S., et al. (2015). Hypoxia-induced Changes in Protein S-Nitrosylation in Female Mouse Brainstem. Am. J. Respir. Cell. Mol. Biol. 52, 37–45. doi:10.1165/rcmb.2013-0359OC
Palmer, L. A., May, W. J., deRonde, K., Brown-Steinke, K., Bates, J. N., Gaston, B., et al. (2013a). Ventilatory Responses during and Following Exposure to a Hypoxic Challenge in Conscious Mice Deficient or Null in S-Nitrosoglutathione Reductase. Respir. Physiol. Neurobiol. 185, 571–581. doi:10.1016/j.resp.2012.11.009
Palmer, L. A., May, W. J., deRonde, K., Brown-Steinke, K., Gaston, B., and Lewis, S. J. (2013b). Hypoxia-induced Ventilatory Responses in Conscious Mice: Gender Differences in Ventilatory Roll-Off and Facilitation. Respir. Physiol. Neurobiol. 185, 497–505. doi:10.1016/j.resp.2012.11.010
Pattinson, K. T. (2008). Opioids and the Control of Respiration. Br. J. Anaesth. 100, 747–758. doi:10.1093/bja/aen094
Pattinson, K. T., and Wise, R. G. (2016). Imaging the Respiratory Effects of Opioids in the Human Brain. Adv. Exp. Med. Biol. 903, 145–156. doi:10.1007/978-1-4899-7678-9_10
Pelligrino, D. A., Laurito, C. E., and VadeBoncouer, T. R. (1996). Nitric Oxide Synthase Inhibition Modulates the Ventilatory Depressant and Antinociceptive Actions of Fourth Ventricular Infusions of Morphine in the Awake Dog. Anesthesiology 85, 1367–1377. doi:10.1097/00000542-199612000-00018
Piantadosi, C. A. (2012). Regulation of Mitochondrial Processes by Protein S-Nitrosylation. Biochim. Biophys. Acta 1820, 712–721. doi:10.1016/j.bbagen.2011.03.008
Pires da Silva, M., de Almeida Moraes, D. J., Mecawi, A. S., Rodrigues, J. A., and Varanda, W. A. (2016). Nitric Oxide Modulates HCN Channels in Magnocellular Neurons of the Supraoptic Nucleus of Rats by an S-nitrosylation-dependent Mechanism. J. Neurosci. 36, 11320–11330. doi:10.1523/JNEUROSCI.1588-16.2016
Pol, O. (2007). The Involvement of the Nitric Oxide in the Effects and Expression of Opioid Receptors during Peripheral Inflammation. Curr. Med. Chem. 14, 1945–1955. doi:10.2174/092986707781368469
Possas, O. S., Johnson, A. K., and Lewis, S. J. (2006). Role of Nitrosyl Factors in the Hindlimb Vasodilation Elicited by Baroreceptor Afferent Nerve Stimulation. Am. J. Physiol. Regul. Integr. Comp. Physiol. 290, R741–R748. doi:10.1152/ajpregu.00660.2005
Possas, O. S., and Lewis, S. J. (1997). NO-containing Factors Mediate Hindlimb Vasodilation Produced by Superior Laryngeal Nerve Stimulation. Am. J. Physiol. 273, H234–H243. doi:10.1152/ajpheart.1997.273.1.H234
Prather, P. L., McGinn, T. M., Claude, P. A., Liu-Chen, L. Y., Loh, H. H., and Law, P. Y. (1995). Properties of a Kappa-Opioid Receptor Expressed in CHO Cells: Interaction with Multiple G-Proteins Is Not Specific for Any Individual G Alpha Subunit and Is Similar to that of Other Opioid Receptors. Brain Res. Mol. Brain Res. 29, 336–346. doi:10.1016/0169-328x(94)00264-f
Raju, K., Doulias, P. T., Evans, P., Krizman, E. N., Jackson, J. G., Horyn, O., et al. (2015). Regulation of Brain Glutamate Metabolism by Nitric Oxide and S-Nitrosylation. Sci. Signal 8, ra68. doi:10.1126/scisignal.aaa4312
Ramirez, J. M., Burgraff, N. J., Wei, A. D., Baertsch, N. A., Varga, A. G., Baghdoyan, H. A., et al. (2021). Neuronal Mechanisms Underlying Opioid-Induced Respiratory Depression: Our Current Understanding. J. Neurophysiol. 125, 1899–1919. doi:10.1152/jn.00017.2021
Ramos-Matos, C. F., Bistas, K. G., and Lopez-Ojeda, W. (2022). “Fentanyl,” in StatPearls [Internet] (Treasure Island (FL): StatPearls Publishing).
Ren, J., Ding, X., Funk, G. D., and Greer, J. J. (2009). Ampakine CX717 Protects against Fentanyl-Induced Respiratory Depression and Lethal Apnea in Rats. Anesthesiology 110, 1364–1370. doi:10.1097/ALN.0b013e31819faa2a
Rockman, H. A., Koch, W. J., and Lefkowitz, R. J. (2002). Seven-transmembrane-spanning Receptors and Heart Function. Nature 415, 206–212. doi:10.1038/415206a
Rodríguez-Muñoz, M., and Garzón, J. (2013). Nitric Oxide and Zinc-Mediated Protein Assemblies Involved in Mu Opioid Receptor Signaling. Mol. Neurobiol. 48, 769–782. doi:10.1007/s12035-013-8465-z
Roerig, S. C., Loh, H. H., and Law, P. Y. (1992). Identification of Three Separate Guanine Nucleotide-Binding Proteins that Interact with the Delta-Opioid Receptor in NG108-15 Neuroblastoma X Glioma Hybrid Cells. Mol. Pharmacol. 41, 822–831.
Rubenstein, R. C., Linder, M. E., and Ross, E. M. (1991). Selectivity of the Beta-Adrenergic Receptor Among Gs, Gi's, and Go: Assay Using Recombinant Alpha Subunits in Reconstituted Phospholipid Vesicles. Biochemistry 30, 10769–10777. doi:10.1021/bi00108a023
Rudkouskaya, A., Sim, V., Shah, A. A., Feustel, P. J., Jourd'heuil, D., and Mongin, A. A. (2010). Long-lasting Inhibition of Presynaptic Metabolism and Neurotransmitter Release by Protein S-Nitrosylation. Free Radic. Biol. Med. 49, 757–769. doi:10.1016/j.freeradbiomed.2010.05.032
Sahin, A. S., Duman, A., Atalik, E. K., Ogün, C. O., Sahin, T. K., Erol, A., et al. (2005). The Mechanisms of the Direct Vascular Effects of Fentanyl on Isolated Human Saphenous Veins In Vitro. J. Cardiothorac. Vasc. Anesth. 19, 197–200. doi:10.1053/j.jvca.2005.01.031
Sarton, E., Teppema, L., and Dahan, A. (1999). Sex Differences in Morphine-Induced Ventilatory Depression Reside within the Peripheral Chemoreflex Loop. Anesthesiology 90, 1329–1338. doi:10.1097/00000542-199905000-00017
Saunders, S. E., and Levitt, E. S. (2020). Kölliker-Fuse/Parabrachial Complex Mu Opioid Receptors Contribute to Fentanyl-Induced Apnea and Respiratory Rate Depression. Respir. Physiol. Neurobiol. 275, 103388. doi:10.1016/j.resp.2020.103388
Savidge, T. C. (2011). S-nitrosothiol Signals in the Enteric Nervous System: Lessons Learnt from Big Brother. Front. Neurosci. 5, 31. doi:10.3389/fnins.2011.00031
Schaeffer, J. I., and Haddad, G. G. (1985). Regulation of Ventilation and Oxygen Consumption by Delta- and Mu-Opioid Receptor Agonists. J. Appl. Physiol. 59, 959–968. doi:10.1152/jappl.1985.59.3.959
Seckler, J. M., Grossfield, A., May, W. J., Getsy, P. M., and Lewis, S. J. (2022). Nitrosyl Factors Play a Vital Role in the Ventilatory Depressant Effects of Fentanyl in Unanesthetized Rats. Biomed. Pharmacother. 146, 112571. doi:10.1016/j.biopha.2021.112571
Seckler, J. M., Meyer, N. M., Burton, S. T., Bates, J. N., Gaston, B., and Lewis, S. J. (2017). Detection of Trace Concentrations of S-Nitrosothiols by Means of a Capacitive Sensor. PLoS One 12, e0187149. doi:10.1371/journal.pone.0187149
Seckler, J. M., Shen, J., Lewis, T. H. J., Abdulameer, M. A., Zaman, K., Palmer, L. A., et al. (2020). NADPH Diaphorase Detects S-Nitrosylated Proteins in Aldehyde-Treated Biological Tissues. Sci. Rep. 10, 21088. doi:10.1038/s41598-020-78107-6
Seth, D., and Stamler, J. S. (2011). The SNO-Proteome: Causation and Classifications. Curr. Opin. Chem. Biol. 15, 129–136. doi:10.1016/j.cbpa.2010.10.012
Severina, I. S., Bussygina, O. G., Pyatakova, N. V., Malenkova, I. V., and Vanin, A. F. (2003). Activation of Soluble Guanylate Cyclase by NO Donors-SNnitrosothiols, and Dinitrosyl-Iron Complexes with Thiol-Containing Ligands. Nitric Oxide 8, 155–163. doi:10.1016/s1089-8603(03)00002-8
Shao, Q., Fallica, J., Casin, K. M., Murphy, E., Steenbergen, C., and Kohr, M. J. (2016). Characterization of the Sex-dependent Myocardial S-Nitrosothiol Proteome. Am. J. Physiol. Heart Circ. Physiol. 310, H505–H515. doi:10.1152/ajpheart.00681.2015
Sharma, S. K., Klee, W. A., and Nirenberg, M. (1977). Opiate-Dependent Modulation of Adenylate Cyclase. Proc. Natl. Acad. Sci. USA 74, 3365–3369. doi:10.1073/pnas.74.8.3365
Simon, S. T., Köskeroglu, P., Gaertner, J., and Voltz, R. (2013). Fentanyl for the Relief of Refractory Breathlessness: a Systematic Review. J. Pain Symptom Manage. 46, 874–886. doi:10.1016/j.jpainsymman.2013.02.019
Someya, E., Mori, A., Sakamoto, K., Ishii, K., and Nakahara, T. (2017). Stimulation of μ-opioid Receptors Dilates Retinal Arterioles by Neuronal Nitric Oxide Synthase-Derived Nitric Oxide in Rats. Eur. J. Pharmacol. 803, 124–129. doi:10.1016/j.ejphar.2017.03.043
Spencer, R. J., Jin, W., Thayer, S. A., Chakrabarti, S., Law, P. Y., and Loh, H. H. (1997). Mobilization of Ca2+ from Intracellular Stores in Transfected Neuro2a Cells by Activation of Multiple Opioid Receptor Subtypes. Biochem. Pharmacol. 54, 809–818. doi:10.1016/s0006-2952(97)00243-8
Stamler, J. S., Jia, L., Eu, J. P., McMahon, T. J., Demchenko, I. T., Bonaventura, J., et al. (1997). Blood Flow Regulation by S-Nitrosohemoglobin in the Physiological Oxygen Gradient. Science 276, 2034–2037. doi:10.1126/science.276.5321.2034
Stein, C. (2018). New Concepts in Opioid Analgesia. Expert Opin. Investig. Drugs 27, 765–775. doi:10.1080/13543784.2018.1516204
Stomberski, C. T., Hess, D. T., and Stamler, J. S. (2019). Protein S-Nitrosylation: Determinants of Specificity and Enzymatic Regulation of S-Nitrosothiol-Based Signaling. Antioxid. Redox Signal. 30, 1331–1351. doi:10.1089/ars.2017.7403
Suzuki, J., and El-Haddad, S. (2017). A Review: Fentanyl and Non-pharmaceutical Fentanyls. Drug Alcohol. Depend. 171, 107–116. doi:10.1016/j.drugalcdep.2016.11.033
Szeto, H. H., Cheng, P. Y., Dwyer, G., Decena, J. A., Wu, D. L., and Cheng, Y. (1991). Morphine-induced Stimulation of Fetal Breathing: Role of Mu 1-receptors and Central Muscarinic Pathways. Am. J. Physiol. 261, R344–R350. doi:10.1152/ajpregu.1991.261.2.R344
Szeto, H. H., Zhu, Y. S., Umans, J. G., Dwyer, G., Clare, S., and Amione, J. (1988). Dual Action of Morphine on Fetal Breathing Movements. J. Pharmacol. Exp. Ther. 245, 537–542.
Takahashi, H., Shin, Y., Cho, S. J., Zago, W. M., Nakamura, T., Gu, Z., et al. (2007). Hypoxia Enhances S-Nitrosylation-Mediated NMDA Receptor Inhibition via a Thiol Oxygen Sensor Motif. Neuron 53, 53–64. doi:10.1016/j.neuron.2006.11.023
Tarasenko, A. S. (2015). The Effect of Nitric Oxide on Synaptic Vesicle Proton Gradient and Mitochondrial Potential of Brain Nerve Terminals. Ukr. Biochem. J. 87, 64–75. doi:10.15407/ubj87.06.064
Tegeder, I., Scheving, R., Wittig, I., and Geisslinger, G. (2011). SNO-ing at the Nociceptive Synapse? Pharmacol. Rev. 63, 366–389. doi:10.1124/pr.110.004200
Teppema, L., Sarton, E., Dahan, A., and Olievier, C. N. (2000). The Neuronal Nitric Oxide Synthase Inhibitor 7-nitroindazole (7-NI) and Morphine Act Independently on the Control of Breathing. Br. J. Anaesth. 84, 190–196. doi:10.1093/oxfordjournals.bja.a013402
Toda, N., Kishioka, S., Hatano, Y., and Toda, H. (2009b). Interactions between Morphine and Nitric Oxide in Various Organs. J. Anesth. 23, 554–568. doi:10.1007/s00540-009-0793-9
Toda, N., Kishioka, S., Hatano, Y., and Toda, H. (2009a). Modulation of Opioid Actions by Nitric Oxide Signaling. Anesthesiology 110, 166–181. doi:10.1097/ALN.0b013e31819146a9
Tooker, R. E., and Vigh, J. (2015). Light-evoked S-Nitrosylation in the Retina. J. Comp. Neurol. 523, 2082–2110. doi:10.1002/cne.23780
Torralva, R., and Janowsky, A. (2019). Noradrenergic Mechanisms in Fentanyl-Mediated Rapid Death Explain Failure of Naloxone in the Opioid Crisis. J. Pharmacol. Exp. Ther. 371, 453–475. doi:10.1124/jpet.119.258566
Travis, M. D., Davisson, R. L., Bates, J. N., and Lewis, S. J. (1997). Hemodynamic Effects of L- and D-S-Nitroso-Beta,beta-Dimethylcysteine in Rats. Am. J. Physiol. 273, H1493–H1501. doi:10.1152/ajpheart.1997.273.3.H1493
Travis, M. D., Hoque, A., Bates, J. N., and Lewis, S. J. (2000). Blockade of Voltage-Sensitive Ca(2+)-Channels Markedly Diminishes Nitric Oxide- but Not L-S-Nitrosocysteine- or Endothelium-dependent Vasodilation In Vivo. Eur. J. Pharmacol. 408, 289–298. doi:10.1016/s0014-2999(00)00792-5
Travis, M. D., and Lewis, S. J. (2000). Apparent Association of MK-801-Sensitive Ion Channels with L-S-Nitrosocysteine Recognition Sites in the Hindlimb Vasculature of the Rat. Eur. J. Pharmacol. 407, 309–312. doi:10.1016/s0014-2999(00)00710-x
Travis, M. D., Stoll, L. L., Bates, J. N., and Lewis, S. J. (1996). L- and D-S-Nitroso-Beta,beta-Dimethylcysteine Differentially Increase cGMP in Cultured Vascular Smooth Muscle Cells. Eur. J. Pharmacol. 318, 47–53. doi:10.1016/s0014-2999(96)00719-4
Tschirhart, J. N., Li, W., Guo, J., and Zhang, S. (2019). Blockade of the Human Ether A-Go-Go-Related Gene (hERG) Potassium Channel by Fentanyl. Mol. Pharmacol. 95, 386–397. doi:10.1124/mol.118.114751
Van Rampelbergh, J., Poloczek, P., Françoys, I., Delporte, C., Winand, J., Robberecht, P., et al. (1997). The Pituitary Adenylate Cyclase Activating Polypeptide (PACAP I) and VIP (PACAP II VIP1) Receptors Stimulate Inositol Phosphate Synthesis in Transfected CHO Cells through Interaction with Different G Proteins. Biochim. Biophys. Acta. 1357, 249–255. doi:10.1016/s0167-4889(97)00028-1
Vanin, A. F. (2019). What Is the Mechanism of Nitric Oxide Conversion into Nitrosonium Ions Ensuring S-Nitrosating Processes in Living Organisms. Cell biochem. Biophys. 77, 279–292. doi:10.1007/s12013-019-00886-1
Wallenstein, S., Zucker, C. L., and Fleiss, J. L. (1980). Some Statistical Methods Useful in Circulation Research. Circ. Res. 47, 1–9. doi:10.1161/01.res.47.1.1
Webster, L., and Rauck, R. L. (2021). Atypical Opioids and Their Effect on Respiratory Drive. J. Opioid Manag. 17, 109–118. doi:10.5055/jom.2021.0648
Whalen, E. J., Bates, J. N., Johnson, A. K., and Lewis, S. J. (2006). Downregulation of Propranolol-Sensitive Beta-Adrenoceptor Signaling after Inhibition of Nitric Oxide Synthesis. Br. J. Pharmacol. 147, 755–764. doi:10.1038/sj.bjp.0706675
Whalen, E. J., Foster, M. W., Matsumoto, A., Ozawa, K., Violin, J. D., Que, L. G., et al. (2007). Regulation of Beta-Adrenergic Receptor Signaling by S-Nitrosylation of G-Protein-Coupled Receptor Kinase 2. Cell 129, 511–522. doi:10.1016/j.cell.2007.02.046
Whalen, E. J., Johnson, A. K., and Lewis, S. J. (2000). Beta-Adrenoceptor Dysfunction After Inhibition of NO Synthesis. Hypertension 36, 376–382. doi:10.1161/01.hyp.36.3.376
Whalen, E. J., Travis, M. D., Johnson, A. K., and Lewis, S. J. (1999). Rapid Tachyphylaxis to Hemodynamic Effects of PACAP-27 after Inhibition of Nitric Oxide Synthesis. Am. J. Physiol. 276, H2117–H2126. doi:10.1152/ajpheart.1999.276.6.h2117
Wiffen, P. J., Cooper, T. E., Anderson, A. K., Gray, A. L., Grégoire, M. C., Ljungman, G., et al. (2017). Opioids for Cancer-Related Pain in Children and Adolescents. Cochrane Database Syst. Rev. 7, CD012564. doi:10.1002/14651858.CD012564.pub2
Willette, R. N., Doorley, B. M., and Sapru, H. N. (1987). Activation of Cholinergic Mechanisms in the Medulla Oblongata Reverse Intravenous Opioid-Induced Respiratory Depression. J. Pharmacol. Exp. Ther. 240, 352–358.
Wynia-Smith, S. L., and Smith, B. C. (2017). Nitrosothiol Formation and S-Nitrosation Signaling through Nitric Oxide Synthases. Nitric Oxide 63, 52–60. doi:10.1016/j.niox.2016.10.001
Yamakura, T., Sakimura, K., and Shimoji, K. (1999). Direct Inhibition of the N-Methyl-D-Aspartate Receptor Channel by High Concentrations of Opioids. Anesthesiology 91, 1053–1063. doi:10.1097/00000542-199910000-00026
Yeadon, M., and Kitchen, I. (1990). Multiple Opioid Receptors Mediate the Respiratory Depressant Effects of Fentanyl-like Drugs in the Rat. Gen. Pharmacol. 21, 655–664. doi:10.1016/0306-3623(90)91013-h
Young, A. P., Gruber, R. B., Discala, J. F., May, W. J., McLaughlin, D., Palmer, L. A., et al. (2013). Co-activation of μ- and δ-opioid Receptors Elicits Tolerance to Morphine-Induced Ventilatory Depression via Generation of Peroxynitrite. Respir. Physiol. Neurobiol. 186, 255–264. doi:10.1016/j.resp.2013.02.028
Zhang, Z., Xu, F., Zhang, C., and Liang, X. (2007). Activation of Opioid Mu Receptors in Caudal Medullary Raphe Region Inhibits the Ventilatory Response to Hypercapnia in Anesthetized Rats. Anesthesiology 107, 288–297. doi:10.1097/01.anes.0000270760.46821.67
Zhang, Z., Xu, F., Zhang, C., and Liang, X. (2009). Opioid Mu-Receptors in Medullary Raphe Region Affect the Hypoxic Ventilation in Anesthetized Rats. Respir. Physiol. Neurobiol. 168, 281–288. doi:10.1016/j.resp.2009.07.015
Zhang, Z., Zhang, C., Zhuang, J., and Xu, F. (2012a). Contribution of Central μ-receptors to Switching Pulmonary C-Fibers-Mediated Rapid Shallow Breathing into an Apnea by Fentanyl in Anesthetized Rats. Brain Res. 1469, 73–81. doi:10.1016/j.brainres.2012.06.028
Zhang, Z., Zhuang, J., Zhang, C., and Xu, F. (2011). Activation of Opioid μ-receptors in the Commissural Subdivision of the Nucleus Tractus Solitarius Abolishes the Ventilatory Response to Hypoxia in Anesthetized Rats. Anesthesiology 115, 353–363. doi:10.1097/ALN.0b013e318224cc1f
Zhang, Z., Zhang, C., Zhou, M., and Xu, F. (2012b). Activation of Opioid μ-receptors, but Not δ- or κ-receptors, Switches Pulmonary C-Fiber-Mediated Rapid Shallow Breathing into an Apnea in Anesthetized Rats. Respir. Physiology Neurobiol. 183, 211–217. doi:10.1016/j.resp.2012.06.032
Zhuang, J., Zhang, Z., Zhang, C., and Xu, F. (2012). 8-OH-DPAT Abolishes the Pulmonary C-Fiber-Mediated Apneic Response to Fentanyl Largely via Acting on 5HT1A Receptors in the Nucleus Tractus Solitarius. Am. J. Physiol. Regul. Integr. Comp. Physiol. 303, R449–R458. doi:10.1152/ajpregu.00016.2012
Zimpfer, M., Beck, A., Mayer, N., Raberger, G., and Steinbereithner, K. (1983). Effects of Morphine on the Control of the Cardiovascular System by the Carotid-Sinus-Reflex and by the Carotid Chemoreflex. Anaesthesist 32, 60–66.
Keywords: S-nitrosothiol, fentanyl, frequency of breathing, tidal volume, minute ventilation, noneupneic breathing index, Sprague Dawley rats
Citation: Getsy PM, Baby SM, Gruber RB, Gaston B, Lewis THJ, Grossfield A, Seckler JM, Hsieh Y-H, Bates JN and Lewis SJ (2022) S-Nitroso-L-Cysteine Stereoselectively Blunts the Deleterious Effects of Fentanyl on Breathing While Augmenting Antinociception in Freely-Moving Rats. Front. Pharmacol. 13:892307. doi: 10.3389/fphar.2022.892307
Received: 08 March 2022; Accepted: 26 April 2022;
Published: 26 May 2022.
Edited by:
Nick Andrews, Salk Institute for Biological Studies, United StatesReviewed by:
Victor Ruiz-Velasco, The Pennsylvania State University, United StatesMichael Morgan, The University of Melbourne, Australia
Copyright © 2022 Getsy, Baby, Gruber, Gaston, Lewis, Grossfield, Seckler, Hsieh, Bates and Lewis. This is an open-access article distributed under the terms of the Creative Commons Attribution License (CC BY). The use, distribution or reproduction in other forums is permitted, provided the original author(s) and the copyright owner(s) are credited and that the original publication in this journal is cited, in accordance with accepted academic practice. No use, distribution or reproduction is permitted which does not comply with these terms.
*Correspondence: Paulina M. Getsy, pxg55@case.edu
†Present address: Santhosh M. Baby, Translational Sciences Treatment Discovery, Galvani Bioelectronics, Inc., Collegeville, PA, United States