- 1Shaanxi Prov Peoples Hospital, Shaanxi Prov Key Lab Infect and Immune Dis, Xian, China
- 2Shaanxi Key Laboratory of Ischemic Cardiovascular Diseases and Institute of Basic and Translational Medicine, Xi’an Medical University, Xi’an, China
- 3Department of Histology and Embryology, Xi’an Medical University, Xi’an, China
- 4Department of Pharmacology, College of Pharmacy, Shaanxi University of Chinese Medicine, Xianyang, China
Cerebralvascular diseases are the most common high-mortality diseases worldwide. Despite its global prevalence, effective treatments and therapies need to be explored. Given that oxidative stress is an important risk factor involved with cerebral vascular diseases, natural antioxidants and its derivatives can be served as a promising therapeutic strategy. Resveratrol (3, 5, 4′-trihydroxystilbene) is a natural polyphenolic antioxidant found in grape skins, red wine, and berries. As a phytoalexin to protect against oxidative stress, resveratrol has therapeutic value in cerebrovascular diseases mainly by inhibiting excessive reactive oxygen species production, elevating antioxidant enzyme activity, and other antioxidant molecular mechanisms. This review aims to collect novel kinds of literature regarding the protective activities of resveratrol on cerebrovascular diseases, addressing the potential mechanisms underlying the antioxidative activities and mitochondrial protection of resveratrol. We also provide new insights into the chemistry, sources, and bioavailability of resveratrol.
Introduction
Cerebrovascular disease (CVD) has become one of the most life-threatening diseases and represents the second leading cause of death and the main cause of serious disability, which predominantly clinically presents as acute neurological deficits (Ledbetter et al., 2021). CVD mainly includes fatal or non-fatal ischemic stroke, hemorrhagic stroke, transient ischemic attack, and vascular dementia (VaD). Due to vessel narrowing, thrombosis and emboli, these disorders may lead to reduced cerebral blood flow and even cerebrovascular rupture (Chowdhury et al., 2012). As the most common type of CVD, stroke is defined as an acute focal or global neurologic injury caused by the blockage of cerebral blood flow (ischemic stroke) and the sudden rupture of cerebral blood vessels (hemorrhage). Ischemic stroke accounts for approximately 80% of all strokes, and hemorrhagic stroke accounts for 20% (Strong, Mathers, and Bonita 2007). VaD is a cognitive disorder caused by impaired blood flow and vascular injury (Braun et al., 2019), which is the second most common type of dementia, being ranked behind Alzheimer’s disease. VaD accounts for 10–20% of all dementia, and the incidence of VaD among 65-year-old population has risen steadily over the years (Østergaard et al., 2016; Wolters and MA, 2019). Following changes in lifestyle and people getting older, the morbidity, mortality and disability rates of CVD have therefore risen sharply (Fu et al., 2021). However, there is still a lack of efficient therapy, particularly in medicine, protecting against oxidative stress.
Recently, plant-derived natural antioxidants such as resveratrol (RES), quercetin, curcumin, and naringin are extensively used to treat and prevent various types of brain damage. It has been shown that RES has better antioxidant activity than other phyto-antioxidants (Khanduja and Bhardwaj 2003; Salehi et al., 2018). RES (3,5,40- trihydroxystilbene) is a natural polyphenolic compound, which is mainly extracted from grape skin/seed, vegetables, cereals, peanut skins, red wine, and tea (Yu, Fu, and Wang 2012) (Figure 1). Both in vitro and in vivo studies have shown that RES possesses diverse biological and pharmacological properties, which may be applied through antioxidant, anti-inflammatory, anti-apoptosis, anti-cancer, cardio-cerebrovascular protection (Shankar, Singh, and Srivastava 2007; Lopez, Dempsey, and Vemuganti 2015; Yang et al., 2015; Elshaer et al., 2018). It has been suggested that RES had cerebral-protective effects whereby it reduced radicals and upregulated the expression of antioxidant-related genes, including the endothelial nitric oxide synthase (eNOS) (Wang et al., 2011), superoxide dismutase (SOD) (Kovacic and Somanathan 2010), heme oxygenase-1 (HO-1) (Ren et al., 2011) and catalase (CAT) (Khan et al., 2013). Moreover, Sirtuin1 (SIRT1) is a nicotinamide adenine dinucleotide (NAD)-dependent deacetylase involved in both cellular stress and longevity (Horio et al., 2011). RES, as a chemical SIRT1 activator, can target the SIRT1 to maintain the homeostasis between prooxidants and antioxidants in mitochondria and exerts anti-inflammatory effects (Li et al., 2013). More evidence implicates that RES can attenuate various kinds of acute CVD, suggesting that the underlying mechanism is involved with its antioxidative effects (Ma et al., 2013; Hou et al., 2018). Here, we have summarized these novel works of literature to present the pharmacological role of RES in CVD, further assessing the anti-oxidative effect of RES and its potential mechanism.
Structure and metabolism of RES
RES (C14H12O3; MW: 228.25 Da) is a natural antibacterial compound that binds to Flavin mononucleotide riboswitches and regulates gene expression (Sung and Dyck 2015). RES is a class of stilbene polyphenol molecules with dual structural isomeric forms: cis and trans (Figure 2). They possess comparable lipophilicity, but trans-resveratrol has a wider range of applications due to higher stability (Wallerath et al., 2002; Orallo 2006). Trans-resveratrol can be synthesized by pcoumaroyl coenzymes A (CoA) and malonyl CoA, whereas trans-resveratrol can also be converted into other cis-isomers after exposure to heat and ultraviolet irradiations (Shankar, Singh, and Srivastava 2007). RES is mainly transported through passive diffusion due to its small molecular weight and non-polar properties, but the oral bioavailability of RES is only 20% because of extensive metabolism in the gastrointestinal tract and poor intestinal absorption (Walle et al., 2004; Chen F et al., 2013). RES is quickly metabolized in the liver and binds to plasma albumin to promote drug uptake (Kuhnle et al., 2000). After oral and intravenous administration of RES, it has been shown that the main metabolites include sulfatesulfonylurea, 2-monosulfates, monofluorourea, 2-resveratrol monoglucuronides and dihydro resveratrol glucuronide (Calamini et al., 2010). In human urine, these metabolites were found in high concentrations (Boocock et al., 2007; Rotches-Ribalta et al., 2012). It is also reported that there was a ten folds increase in the half-life and plasma concentration of RES metabolites compared to native RES compounds in the human blood (Baur and Sinclair 2006). It suggested that even though its bioavailability was low and its metabolism and elimination were relatively rapid, RES had a relevant biological efficacy, which possibly was due to its conversion or interconversion into sulfonate and glucuronide metabolites (Vitaglione et al., 2005; Delmas et al., 2011). Besides, in animal studies, blood and serum levels of RES peaked 15 min after administration and then rapidly declined. Conversely, RES’s metabolites decreased rather slowly (Soleas et al., 2001). Above these results indicated that the bioavailability of RES metabolites was greater than that of RES (Goldberg, Yan, and Soleas 2003). It is reported that RES readily crossed the blood-brain barrier (BBB) and penetrated into brain tissue (Wang et al., 2002; Wang et al., 2004). They also demonstrated the levels of RES in the brain 4 h after intraperitoneal injection. A multicentric placebo-controlled phase II trial found that RES and its metabolites could penetrate the BBB based on the analysis of cerebrospinal fluid biomarkers (Turner et al., 2015). Besides, RES preserves BBB integrity. The study found that RES attenuated the BBB dysfunction by regulating the matrix metalloproteinase-9 (MMP-9) to limit the infiltration of leukocytes and other inflammatory mediators into the brain (Moussa et al., 2017). Nevertheless, there was no significant difference in the half-life times between the oral and intravenous administration. The half-life was 9.2 h after oral administration and 11.4 h after intravenous injection, and plasma concentrations of RES declined exponentially in a parallel of 72 h (Wang and Sang 2018).
On lipid peroxidation injury of brain synaptosomes, RES exerts the antioxidative potency. In this study, RES significantly scavenged the superoxide anion generated from rat forebrain mitochondria and inhibited ATPase activity with two EC50 values, 0.39 ± 0.15 nano-moles (nM) and 23.1 ± 6.4 micro-moles (μM) (Zini et al., 1999). Another study confirmed that the oxygen consumption of mitochondria was significantly inhibited by RES with a low EC50 of 18.34 picomole (pM) (Zini et al., 2002). Above these results showed that the RES had neuroprotection in CVDs via valuable anti-oxidant activities.
Protective effects of RES on CVD
RES and ischemic stroke
Ischemic stroke is caused by a reduction in cerebral blood flow, leading to energy dysmetabolism, neurological disorders, and cell death. Currently, the principal therapeutic treatment is to restore the cerebral blood flow whereby treatment with recombinant tissue plasminogen activator (rt-PA) destroys thrombi (clots), while rt-PA is also limited by the narrow therapeutic window (Warner et al., 2019). The main reason is that cerebral ischemia is a series of complicated pathological processes involving oxidative stress, inflammation, autophagy, mitochondrial dysfunction, and various signaling pathways (Tao et al., 2020). Chen et al. have revealed that RES extended the clinical therapeutic window of the rt-PA, suggesting that such an effect may be applied by reducing the MMP expression (Chen et al., 2016). Meanwhile, a study has demonstrated that RES preconditioning (RPC) provides a novel long-term window of cerebral ischemic tolerance for 2 weeks in mice (Khoury et al., 2019). These results provide evidence that RPC promotes a beneficial effect on cellular metabolisms such as glycolysis, mitochondrial respiration efficiency, elevated energy production (increased tricarboxylic acid cycle) as well as regulated oxidative phosphorylation and pyruvate uptake (Khoury et al., 2019). Numerous studies have shown that RES exerts neuroprotection in ischemic stroke by increasing antioxidant capacity as well as attenuating oxidative stress and inflammation (Al Dera 2017; Lei and Chen 2018). In such a process, SIRT1 is deemed as the primary molecular target of RES pharmacological actions (Borra, Smith, and Denu 2005; Della-Morte et al., 2009). In the cerebral ischemia-reperfusion-induced brain injury mice model, the mice were administered intraperitoneally with 30 mg/kg of RES and SIRT1/2 selective inhibitor before ischemia (Grewal, Singh, and Singh 2019). The results have shown that RES activates SIRT1, further promoting the improvement in neurobehavioral functions, the reduction in cerebral infarct volume, oxidative stress level, and acetylcholinestrase activity (Grewal, Singh, and Singh 2019). Other studies have also found that RES inhibited sulfonylurea receptor1 (SUR1) and aquaporin 4 (AQP4) expression by targeting specificity proteins transcription factors, thereby improving BBB damage, infarct area, cerebral edema and neurological deficit (Alquisiras-Burgos et al., 2020). In addition, Ma et al. have indicated that RES activated microglia and alleviated neuroinflammation via down-regulating the miR-155 expression (Ma et al., 2020). Even in the experimental stroke of aged female mice, the study shows that RES mitigates ischemic brain injury and inflammation (Jeong et al., 2016). The neuroprotective effects of RES on various ischemic brain injury models are shown in Tables 1, 2.
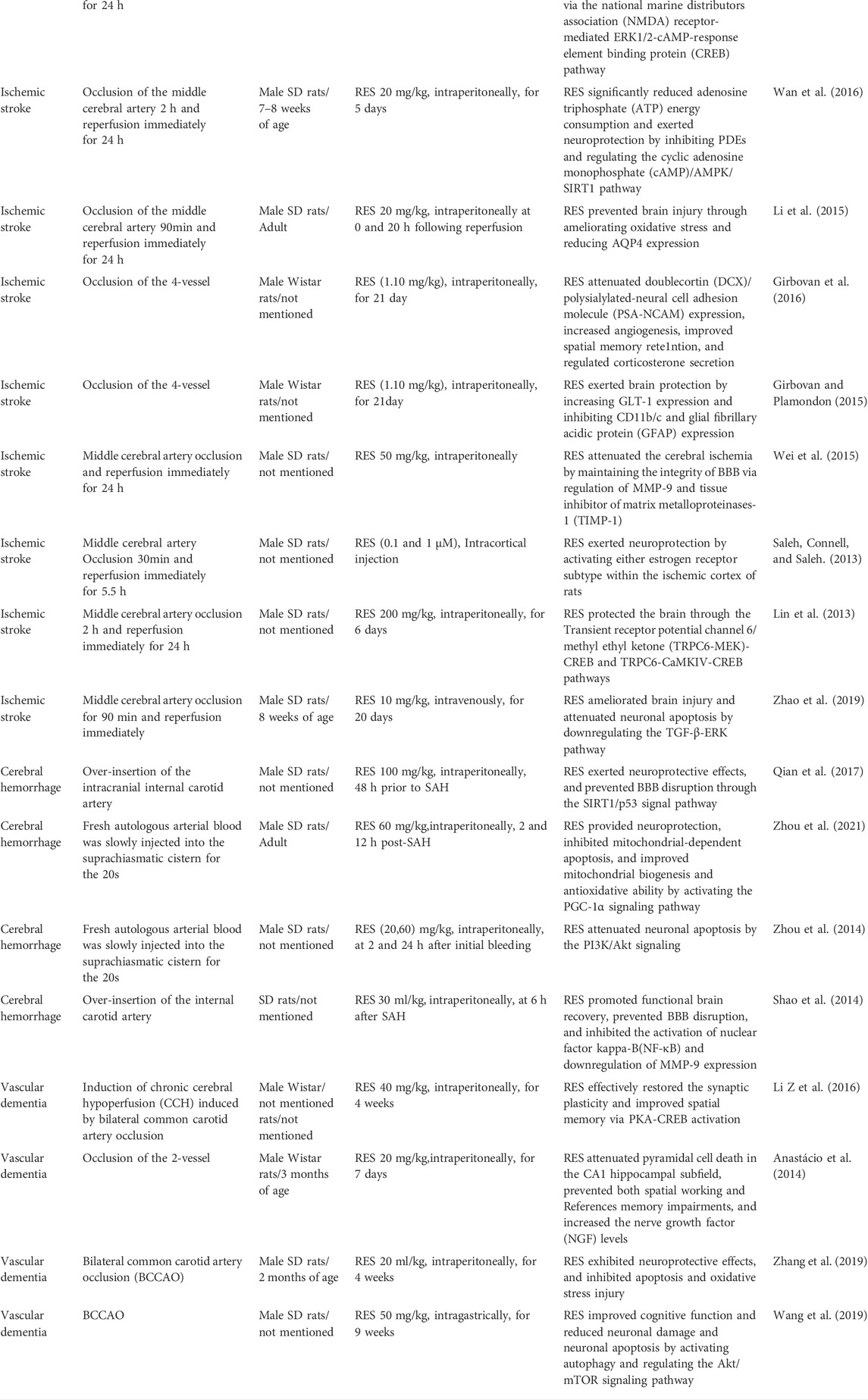
TABLE 1. Summary of the most relevant preclinical studies in vivo evaluating the effects of resveratrol administration on animals subjected to cerebrovascular diseases, including ischemic stroke, hemorrhage stroke, and vascular dementia.
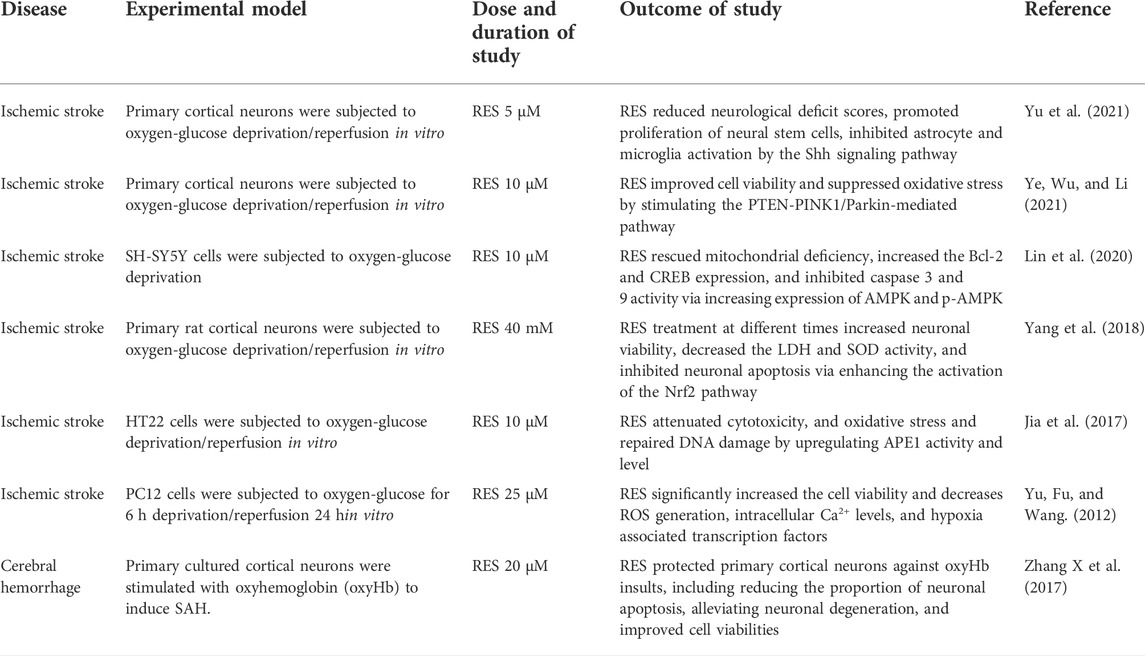
TABLE 2. Summary of the most relevant preclinical studies in vitro evaluating the effects of RES administration on animals subjected to CVD, including ischemic stroke, hemorrhage stroke and vascular dementia.
RES and hemorrhage stroke
Cerebral hemorrhage is a cause of cerebral brain vasospasm (Souissi et al., 2010). More importantly, subarachnoid hemorrhage (SAH) results in long-term cognitive and neurological outcomes (Bederson et al., 2009; Suzuki 2015). Studies have revealed that oxidative damage and endoplasmic reticulum (ER) stress lead to the early stage of brain injury (Ayer and Zhang 2008; Nakka, Prakash-Babu, and Vemuganti 2016). In recent years, there has been a functional link between oxidative stress and ER stress (Ilieva et al., 2007; Zhang 2010). He et al. showed that oxidative stress increased the accumulation of reactive oxygen species (ROS) in the ER stress, and upregulated the expression of Glucose-regulated protein 78 (GRP78) (He et al., 2008). Zhang et al. also proposed that ROS can target ER-based calcium channels, leading to the release of calcium from the ER to the cytosol (Zhang 2010). Increased cytosolic calcium can form a positive feedback loop to produce more ROS. GRP78 is a molecular chaperone with important functions at the cellular level, including the regulation of intracellular calcium, protein folding, and ER stress (Chiang et al., 2011). As a monitor of ER stress, GRP78 is associated with oxidative stress and stabilization of calcium homeostasis (Kudo et al., 2008). It is reported that reduction of GRP78 expression inhibited the ER stress induced by oxidative stress (Pan et al., 2010). Xie et al. have revealed that RES ameliorated SAH-induced brain injury by attenuating oxidative damage, ER stress, and neuroinflammation (Xie et al., 2019). Regarded rats were treated with 60 mg/kg RES after SAH-induced brain injury. And they showed that it alleviated neurological deficits and brain edema, and reduced ROS and malondialdehyde (MDA) levels, indicating that the NF-E2-related factor 2 (Nrf2)/HO-1 pathway and suppressed GRP78 may involve with these effects (Xie et al., 2019). Another study demonstrated that RES significantly prevented the brain injury in hypertension-induced cerebral microhemorrhages, diminished hypertension-induced oxidative stress, and inhibited vascular MMP activation (Toth et al., 2015). Moreover, Post-SAH administration of RES attenuated neurological deficits, cerebral vasospasm, and microvessel thrombi through SIRT1-dependent activation (Diwan et al., 2021). A number of studies suggested that RES prevented BBB disruption, inhibited mitochondrial-dependent apoptosis, and was correlated with transforming growth factor-β-extracellular-signal regulated kinases (TGF-β-ERK), peroxisome proliferator-activated receptor-gamma coactivator (PGC)-1α, phosphatidylinositol three kinase/protein kinase B (PI3K/Akt) and et al. (Tables 1, 2).
RES and vascular dementia
VaD is the second largest cause of dementia in the elderly and is mainly associated with cerebrovascular lesions as well as cognitive decline (Kovacic and Somanathan 2010). The primary pathological cause of VaD is the reduction of blood flow in cerebrovascular (Enciu et al., 2011). Chronic cerebral hypoperfusion is one of the most significant risk factors, which can lead to cerebrovascular degeneration, energy loss, oxidative stress, and inflammation (Jellinger 2007). Ma et al. showed that RES improved learning and memory ability in VaD rats. Specifically, RES exerted an anti-oxidative role via diminishing the MDA but increasing the SOD and glutathione (GSH) levels (Ma et al., 2013). Gocmez et al. established a streptozotocin-induced diabetic VaD rat model, and RES (20 mg/kg) was administrated intraperitoneally for 4 weeks, showing that RES prevented endothelial dysfunction, inflammation, and impairment of neurotrophin expression (Gocmez et al., 2019). Studies showed that a significant reduction of estrogens and progesterone was related to the onset of Alzheimer’s disease (Resnick and Henderson 2002; Jankowska 2017). Note, in the chronic cerebral hypoperfused and ovariectomized-female rats model, RES significantly alleviated brain injury by reducing astrocyte activation and its antioxidant and antiapoptotic effects. Above these findings may provide new insight into the potential clinical application in postmenopausal elderly women who suffer from VaD (Ozacmak, Sayan-Ozacmak, and Barut 2016). In addition, as shown in Table 1, RES regulates various proteins and signal pathways involved in the pathogenesis of VaD.
Molecular mechanisms of RES on CVD
Antioxidant actions of RES on CVD
A major mechanism of CVD may be ascribed to oxidative stress, which is mainly caused by the excessive accumulation of ROS and depleted activities of antioxidant enzymes (Orellana-Urzúa et al., 2020). Excess ROS includes superoxide anion, hydrogen peroxide (H2O2), and nitric oxide (NO), resulting in severe damage to cellular DNA, proteins, and lipids. It has been reported that the MDA and 4-hydroxynonenal were products of lipid peroxidation (Adibhatla, Hatcher, and Dempsey 2006). On the another hand, a variety of antioxidants such as SOD, CAT, GSH, and glutathione peroxidase (GSH-Pxs) protect from brain injury by eliminating ROS. Of note, the levels and activities of these antioxidants were markedly reduced in the animal model of CVD (Bharti and Garg 2019). Therefore, an increase in antioxidant defenses is an important strategy for blunting oxidative damage. Studies have shown that RES directly attenuates oxidative stress by inhibiting lipid peroxidation and upregulating the SOD and GSH activity in brain injury (Ma et al., 2013; Toth et al., 2015; Ozacmak, Sayan-Ozacmak, and Barut 2016; Zhao et al., 2019). Given that extensive ROS inhibits the generation of endothelial NOS (eNOS) and causes vascular endothelial damage, RES can prevent endothelial dysfunction by reducing oxidative stress and inflammation (Gocmez et al., 2019).
In addition, RES regulates trace elements to avoid ROS-induced oxidative damage. A variety of trace elements are important for maintaining normal brain functions. It is reported that Mg depressed oxidative stress and stabilizes the cell membrane (de Baaij, Hoenderop, and Bindels 2012). Zn is a part of the SOD structure and involves antioxidant production (Fang et al., 2013). Se has various beneficial effects, including protecting neurons, increasing antioxidant enzyme activity, and repairing DNA damage (Van Eersel et al., 2010; Song et al., 2014). Besides, increased Fe and Cu levels produce toxic hydroxyl radicals, leading to deleterious lipid peroxidation and oxidative injury (Allen et al., 2008; Murphy and Oudit 2010). Al and As are also considered to induce oxidative stress (Good et al., 1992; Miller et al., 2002; Cohen et al., 2006; Jomova and Valko 2011; Jaishankar et al., 2014). In a recent report, researchers have discovered that RES diminished the overload of Fe, Cu, As and Al, but increased Mg, Zn and Se levels to exert antioxidant effects in cerebral ischemic injury (Lin et al., 2021; Ro, Liu, and Lin 2021).
Nrf2 is a molecular target of oxidative stress through regulating abundant antioxidant enzymes and defense proteins (Vomund et al., 2017). Under normal physiological conditions, Nrf2 forms a Kelch-like ECH-associated protein l (Keap1)-Nrf2 complex in the cytoplasm. After stressful or inflammatory responses, Nrf2 is released from the Keap1/Nrf2 complex and translocates into the nucleus to combine with the antioxidant response element (ARE), initiating the transcription of downstream target genes (Kobayashi et al., 2004; Hayes et al., 2010). Many target genes are involved in the regulation of cellular redox homeostasis, such as NAD(P)H quinone oxidoreductase 1 (NQO1), HO-1, glutathione-s-transferase (GST) (Kumar and Mittal 2017). A recent study suggested that RES inhibited oxidative stress by upregulating the Nrf2 and HO-1 mRNA, thus protecting brain function (Yang et al., 2018).
Noteworthy, DJ-1 is activated during oxidative stress, which is therefore deemed as a crucial antioxidant regulator in brain science (Taira et al., 2004). Indeed, DJ-1 is an important modulator of Nrf2 and negatively regulates PI3K/Akt signal pathway (McNally et al., 2011). A number of studies have revealed that PI3K/Akt activation is apparently associated with antioxidative stress and anti-apoptosis (Liu et al., 2017; Yang et al., 2020). In contrast, PI3K activity is negatively regulated by phosphatase and tensin homolog deleted on chromosome ten (PTEN) (Almazari et al., 2012). In the central nervous system, PTEN has been considered a mediator of ROS production and cell apoptosis (Shang et al., 2022). At present, some studies have reported that DJ-1 activates the PI3K/Akt signal pathway by inhibiting PTEN to avert damage due to oxidative stress (Kim et al., 2005; Kim et al., 2009). In another study, RES reduced ROS generation and enhanced antioxidant enzyme activity. This effect is clearly linked to the reduction in oxidized levels of DJ-1, inhibiting PTEN activity and PI3K/Akt survival pathway activation (Abdel-Aleem et al., 2016).
PGC-1α is a transcription co-activator involved in mitochondrial biogenesis and function (Wareski et al., 2009). In addition, increasing evidence suggests that PGC-1α upregulates a serious of oxidative stress protective genes such as manganese SOD (Mn-SOD) to remove the ROS production (Valle et al., 2005; Orallo 2006). This remarkable antioxidant mechanism was also observed in the protective role of RES in the SAH model. In this study, RES increased PGC-1α expression and promoted PGC-1α nuclear translocation. Moreover, RES can apply to scavenge excess ROS, increasing the activity of SOD and improving the mitochondrial function and ATP levels to prevent neurological impairment after SAH. These results indicate that RES promotes mitochondrial biogenesis and decreases oxidative stress by activation of the PGC-1α signaling pathway in SAH (Zhou et al., 2021).
Oxidative stress commonly causes DNA peroxidation, which gives rise to DNA damage (Chen et al., 1997; Lan et al., 2003; Chen et al., 2011). APE1 is a ubiquitous and multifunctional DNA repair enzyme and participates in protein redox regulation (Dyrkheeva, Lebedeva, and Lavrik 2016; Laev, Salakhutdinov, and Lavrik 2017). Moreover, regulation of APE1 in neurons protects against ischemia-induced neuronal death (Ludwig et al., 1998; Vasko, Guo, and Kelley 2005). It has been reported that endogenous upregulation of APE1 reduced white matter infarct, promoting neurologic functional recovery after stroke (Stetler et al., 2016). Leak et al. have also revealed that the upregulation of APE1, either endogenously or through transgene overexpression, reduces oxidative DNA damage and protects neurons against acute ischemic damage (Leak et al., 2015). Consistent with prior studies, Jia et al. have found that RES obviously elevated the activity and level of APE1 (Jia et al., 2017). On the other hand, previous research has shown that strand breaks of apurinic/apyrimidinic sites are considered a marker of oxidative DNA damage, and 8-OHdG is also the specific product of DNA oxidative damage (Chen et al., 1997; Lan et al., 2003). Notedly, this study also demonstrated that RES reduced the level of 8-OHdG and AP sites in OGD/R-induced cell damage. In addition, the knockdown of APE1 blocked the neuroprotective and antioxidant effects of RES. The above results further showed that RES exerted a neuroprotective role in ischemic stroke, which was related to the APE1-induced oxidative DNA damage reduction and antioxidant defense system enhancement (Jia et al., 2017) (Figure 3).
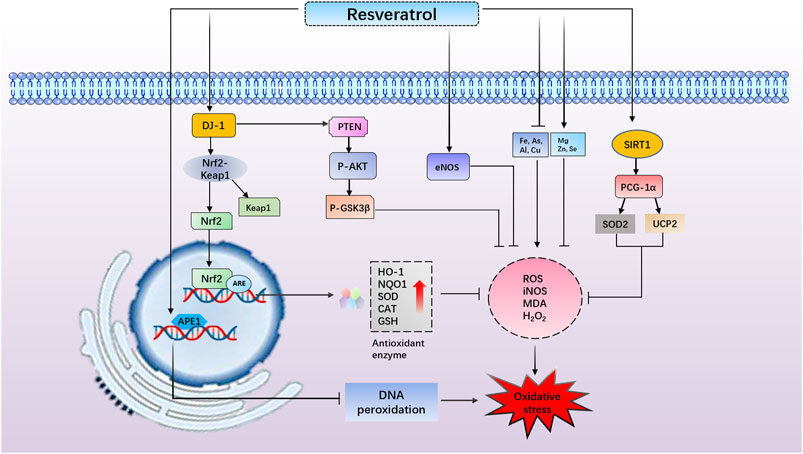
FIGURE 3. Resveratrol (RES) reduces apurinic/apyrimidinic endonuclease 1–induced oxidative DNA damage. RES increases DJ-1 protein expression, promotes NF-E2-related factor 2 (Nrf2) dissociating from Kelch-like ECH- associated protein l and induces Nrf2/antioxidant response element–dependent antioxidant enzyme (heme oxygenase-1 and NAD(P)H quinone oxidoreductase 1) transcriptions, thus exerting antioxidant effects. RES reduces reactive oxygen species generation and enhances antioxidant enzyme activity through reduction in oxidized levels of DJ-1, inhibiting phosphatase and tensin homolog deleted on chromosome ten activity and phosphatidylinositol 3 kinase/protein kinase B survival pathway activation. RES increases the generation of endothelial nitric oxide synthase to prevent oxidative stress. RES diminishes the overload of Fe, Cu, As, and Al but increases Mg, Zn, and Se levels to exert an antioxidant role. RES activates peroxisome proliferator–activated receptor–gamma coactivator–1alpha and increases superoxide dismutase 2 and UCP2 levels to promote mitochondrial biogenesis and thus decreases oxidative stress.
Mitochondrial protective effects of RES on CVD
Mitochondria are the major organelles involved in the synthesis of ATP in mammalian cells, which are essential for maintaining cellular homeostasis and function (Papa et al., 2012; Chaban, Boekema, and Dudkina 2014). Mitochondrial dysfunction plays an important role in the pathogenesis of CVD (Correia et al., 2010; Li et al., 2012; Chen et al., 2020; Han et al., 2020). Mitochondrial biogenesis is the process responsible for the synthesis of new mitochondria, which is the main component of the mitochondrial mass control system. Mammalian mitochondrial biogenesis and function require the coordinated action of both nuclear and mitochondrial genomes (Scarpulla 2008; Jornayvaz and Shulman 2010; Ungvari et al., 2011). PGC-1α regulates mitochondrial biogenesis by activating the nuclear respiratory factors 1 and 2 (NRF-1 and NRF-2) and increases the expression of mitochondrial proteins encoded by nuclear DNA (Virbasius, Virbasius, and Scarpulla 1993; Schreiber et al., 2004; Anderson and Prolla 2009; Scarpulla 2011). Among these proteins, Transcription factor A mitochondrial (TFAM) is an essential mitochondria transcriptor playing both a transcription and replication role in mitochondrial DNA (mtDNA) (Canugovi et al., 2010). Additionally, TFAM has been viewed as an important modulator of mtDNA homeostasis and repair (Picca and Lezza 2015; Filograna et al., 2021). The D-loop (mitochondrial displacement loop) was an mtDNA noncoding region, and it was the major control region for the regulation of mitochondrial genome replication and expression. The expression of the D-loop was used in evaluating mtDNA copy number and mitochondrial biogenesis (Shuwen, Xi, and Yuefen 2017). Recent evidence implicates the increasing mitochondrial biogenesis is thought to prevent mitochondrial dysfunction as well as attenuate CVD progression (Yin et al., 2008; Chen et al., 2010). Lin et al. have evidenced that RES rescued SH-SY5Y cells from OGD-mediated mitochondrial lower D-loop level and mitochondrial mass dimension. RES also rescued the transcript expression levels of PGC1-α, and mitochondrial genes (NRF-1 and TFAM) in OGD-treated SH-SY5Y cells (Lin et al., 2020). Besides, AMPK activity is important for maintaining mitochondrial biogenesis homeostasis (Zhang J et al., 2017). These studies have shown that activation of AMPK protected neurons against mitochondrial dysfunction, and oxidative stress, further suggesting that AMPK may be critically important in preventing neuronal cell damage (Zhang J et al., 2017; Nanjaiah and Vallikannan 2019). Lin et al. also have found that RES improved the expression of AMPK and p-AMPK in OGD-exposed SH-SY5Y cells. It implies that RES reverses OGD-induced SH-SY5Y cell damage caused by mitochondrial dysfunction in an AMPK-dependant manner (Lin et al., 2020).
Mitochondria also have crucial functions in a number of essential cellular processes, such as the regulation of ROS production, tricarboxylic acid cycle (TAC) and respiratory chain regulation (Madamanchi and Runge 2007; Peng et al., 2019). In the brain, oxidative damage decreases enzyme activity of the respiratory chain, resulting in mitochondrial dysfunction (Szeto 2008; Huang et al., 2013; Han et al., 2020). The respiratory chain dysfunction promotes electrons leakage and excess ROS production (Murphy 2009). ROS forms a positive feedback loop, which can cause damage to the inner membrane integrity of the mitochondrial, mitochondrial depolarization, and the opening of mitochondrial permeability transition pore (MPTP) (Szeto 2008). ROS mainly consists of H2O2 and superoxide anion radical. H2O2 and superoxide anion radical lead to alterations in the function of the respiratory chain (Turrens 2003). There are several antioxidant defense mechanisms in mitochondria, including enzyme and non-enzymatic defenses. Among the enzymatic antioxidant defenses, Mn-SOD takes a crucial role in the converting superoxide anion radical into H2O2 through reacting with glutathione peroxidase (GPx) or CAT, generating water (Flynn and Melov 2013; Lu 2013; Morris et al., 2014; Barrera et al., 2016). Reduced GSH is the main nonenzymatic antioxidant defense (Lu 2013). GSH is produced in the cytosol and is necessary to attenuate redox impairment through the reactions of Mn-SOD (Flynn and Melov 2013) and from mitochondrial complexes (Zorov, Juhaszova, and Sollott 2014). Therefore, protective intervention in the mitochondrial function might contribute to the amelioration of ischemia-related brain damage. Studies have shown that RES and mitochondrial function have also been linked to ischemia-reperfusion injury (Zini et al., 1999; Kipp and Ramirez 2001; Zini et al., 2002). The RES can preserve the function of brain mitochondria by reducing the generation of ROS in the mitochondria after hypoxia-reoxygenation (Morin et al., 2003). The mitochondrial respiratory enzyme COX was also examined to evaluate the mitochondrial function (Ortiz-Ruiz et al., 2021). In the present study, it is very likely that RES significantly rescues SH-SY5Y cells from OGD-mediated mitochondrial deficiency (maximal respiratory function, ATP content, COX activity, and mitochondrial membrane potential) (Yousuf et al., 2009; Lin et al., 2020). Remarkably, Khoury et al. reported that RPC promoted an increase in glycolysis and mitochondrial respiration efficiency. Additionally, genes involved in pyruvate uptake, TAC cycle, and oxidative phosphorylation were more highly expressed, indicating protection on mitochondrial energy-producing pathways during cerebral ischemia (Khoury et al., 2019). For the treatment of SAH, RES resulted in the activation of Sirt5. The activation restored mitochondrial metabolism dysfunction and alleviated early brain injury as well as desuccinylation of citrate synthase and ATP synthase (Xiao et al., 2021). There is also evidence that in the VaD model, RES significantly reduces mitochondrial ROS generation, lipid peroxidation, and protein carbonyls. Beyond this, significant improvements in redox ratio and Mn-SOD activity were observed with RES (Yadav et al., 2018).
The adverse reactions and clinical efficacy of RES
Although RES intake has potent pleiotropic effects in humans and is well tolerated (Ramírez-Garza et al., 2018), others have reported toxic effects of RES in vitro and in vivo (Calabrese, Mattson, and Calabrese 2010; Cottart et al., 2010; Salehi et al., 2018). It is known that RES has beneficial antioxidant activity, but it appears that RES intake has negative effects on metabolic function, endothelial health, inflammation, and cardiovascular markers in human patients (Ramírez-Garza et al., 2018). In one study, healthy volunteers were given RES doses of 25 mg, 50 mg, 100 mg, and 150 mg at intervals of 4 hours for 48 h. Finally, in some participants, mild adverse effects were reported, such as headaches, dizziness, and epididymitis (Almeida et al., 2009). In obese postmenopausal women, administration of 1 g RES for 12 weeks resulted in negative effects. The liver enzyme levels of one of the 34 subjects were elevated. In addition, 30% of the subjects experienced diarrhea, and 27% had increased total cholesterol (Chow et al., 2014). It is reported that a 450 mg/day dose of RES was a safe dose for a 60 kg person (Moon et al., 2004). RES (1,000 mg/day) was found to inhibit cytochrome P450 isoenzymes such as CYP1A2 and CYP2C9, resulting in interactions with many other drugs (Detampel et al., 2012). In most cases, these interactions could diminish the activity of these drugs. It indicates that RES administration (over 1000 mg/day) causes differences in the pharmacokinetics of drugs administered concurrently (Detampel et al., 2012). Moreover, intracellular redox imbalance in endothelial cells can lead to endothelial dysfunction, which is an important step in the progression of CVD (Cai and Harrison 2000). Numerous studies have demonstrated RES provided protection via its antioxidant impact on the endothelium (Cao and Li 2004; Chen M et al., 2013). However, RES has been shown to increase intracellular oxidative state at tissue-achievable doses, according to Posadino et al. It leads to mitochondrial damage and endothelial cell death (Posadino et al., 2015). Finally, the researchers concluded RES affected endothelial cells biphasically depending on the concentration. In vitro, RES at low concentrations reduced the oxidative state of endothelial cells. RES (≥10 µM) increased the status of oxidative stress at higher concentrations in vitro (Posadino et al., 2015). Overall, the adverse effects associated with RES use correlate with drug dose. Compared with the overwhelming benefits of RES, its side effects appear mild and sporadic.
Currently, there are many clinical cases that support the use of RES in the prevention and treatment of nervous system disorders. In patients with acute ischemic stroke, rt-PA is one of the most successful treatment options. However, their narrow therapeutic window limits their therapeutic benefits (Lansberg et al., 2012). As is reported, co-administration of RES and rt-PA could extend the time-bound therapeutic window in cerebral stroke patients (Chen et al., 2016). Additionally, they also suggest a potential role for the combination of RES and rt-PA treatment to improve BBB integrity. There has also been researching on the effects of RES on neurological and cognitive disorders in overweight but otherwise healthy individuals as well. Memory performance was improved by RES by increasing hippocampal functional connectivity and improving glucose metabolism (Witte et al., 2014). Interestingly, it was found that RES altered cerebral blood flow in healthy adults. During this study, a dose-dependent increase in cerebral blood flow was observed following two doses of trans-resveratrol (250 and 500 mg) (Kennedy et al., 2010). While RES has been reported to be beneficial for a number of human diseases, it is currently not prescribed for them. Many clinical trials still ongoing. As knowledge of the health benefits of RES increases, the clinical utility is likely to become more apparent and widely accepted.
Conclusion and future direction
CVD severely impacts the patient’s quality of life. Treatments of cerebrovascular disorders have developed significantly, such as intravenous (IV) tissue plasminogen activator (IV-tPA), intra-arterial therapy (IAT) for ischemic stroke, and endovascular embolization of vascular malformations. However, thrombolysis has a relatively narrow therapeutic time window (4.5 h) and the risk of severe adverse effects such as hemorrhagic transformation. These treatments are effective, but it is limited to use in a small proportion of patients. Therefore, developing new therapeutic agents are necessary for the treatment of CVD.
Natural products and their derivatives are widely used as treatments for many diseases. RES has various properties like low cytotoxicity, low cost, and extensive sources. More importantly, oxidative stress also becomes a prominent target of RES. Taken together, RES has therapeutic value in CVD mainly by inhibiting excessive ROS production and elevating antioxidant enzyme activity. Despite that, RES is limitedly used because of its low aqueous solubility and bioavailability. To overcome these defects, cyclodextrins and nanoparticles are developed as drug carriers, which can be applied for enhancement of the solubility, stability, and absorption rate. In addition, an effective sustained-release drug delivery system may decrease the early degradation in the intestine and liver. These innovative approaches have significantly improved the pharmacokinetics of RES, providing a promising treatment for CVD.
Author contributions
QW and QY contributed to the design of the review. MW revised the manuscript. All the authors read and approved the final version of the manuscript.
Funding
The present work was supported by the National Natural Science of China (81400328 and 81773795), Innovation Support Plan and Key Research and Development Program of Shaanxi Province of China (2020PT-003) and Research Found of Xi’an Medical University (2018XNRC02 and 2018PT23).
Conflict of interest
The authors declare that the research was conducted in the absence of any commercial or financial relationships that could be construed as a potential conflict of interest.
Publisher’s note
All claims expressed in this article are solely those of the authors and do not necessarily represent those of their affiliated organizations, or those of the publisher, the editors and the reviewers. Any product that may be evaluated in this article, or claim that may be made by its manufacturer, is not guaranteed or endorsed by the publisher.
Supplementary material
The Supplementary Material for this article can be found online at: https://www.frontiersin.org/articles/10.3389/fphar.2022.948889/full#supplementary-material
References
Abdel-Aleem, G. A., Khaleel, E. F., Mostafa, D. G., and Elberier, L. K. (2016). Neuroprotective effect of resveratrol against brain ischemia reperfusion injury in rats entails reduction of DJ-1 protein expression and activation of PI3K/Akt/GSK3b survival pathway. Arch. Physiol. Biochem. 122, 200–213. doi:10.1080/13813455.2016.1182190
Adibhatla, R. M., Hatcher, J. F., and Dempsey, R. J. (2006). Lipids and lipidomics in brain injury and diseases. Aaps J. 8, E314–E321. doi:10.1007/BF02854902
Aguilar, G. F., Alquisiras-Burgos, I., Franco-Pérez, J., Pineda-Ramírez, N., Ortiz-Plata, A., Torres, I., et al. (2020). Resveratrol prevents GLUT3 up-regulation induced by middle cerebral artery occlusion. Brain Sci. 10, E651. doi:10.3390/brainsci10090651
Al Dera, H. (2017). Neuroprotective effect of resveratrol against late cerebral ischemia reperfusion induced oxidative stress damage involves upregulation of osteopontin and inhibition of interleukin-1beta. J. Physiol. Pharmacol. 68, 47–56.
Allen, K. J., Gurrin, L. C., Constantine, C. C., Osborne, N. J., Delatycki, M. B., Nicoll, A. J., et al. (2008). Iron-overload-related disease in HFE hereditary hemochromatosis. N. Engl. J. Med. 358, 221–230. doi:10.1056/NEJMoa073286
Almazari, I., Park, J. M., Park, S. A., Suh, J. Y., Na, H. K., Cha, Y. N., et al. (2012). Guggulsterone induces heme oxygenase-1 expression through activation of Nrf2 in human mammary epithelial cells: PTEN as a putative target. Carcinogenesis 33, 368–376. doi:10.1093/carcin/bgr259
Almeida, L., Vaz-da-Silva, M., Falcão, A., Soares, E., Costa, R., Loureiro, A. I., et al. (2009). Pharmacokinetic and safety profile of trans-resveratrol in a rising multiple-dose study in healthy volunteers. Mol. Nutr. Food Res. 53 (1), S7–S15. doi:10.1002/mnfr.200800177
Alquisiras-Burgos, I., Ortiz-Plata, A., Franco-Pérez, J., Millán, A., and Aguilera, P. (2020). Resveratrol reduces cerebral edema through inhibition of de novo SUR1 expression induced after focal ischemia. Exp. Neurol. 330, 113353. doi:10.1016/j.expneurol.2020.113353
Anastácio, J. R., Netto, C. A., Castro, C. C., Sanches, E. F., Ferreira, D. C., Noschang, C., et al. (2014). Resveratrol treatment has neuroprotective effects and prevents cognitive impairment after chronic cerebral hypoperfusion. Neurol. Res. 36, 627–633. doi:10.1179/1743132813Y.0000000293
Anderson, R., and Prolla, T. (2009). PGC-1alpha in aging and anti-aging interventions. Biochim. Biophys. Acta 1790, 1059–1066. doi:10.1016/j.bbagen.2009.04.005
Ayer, R. E., and Zhang, J. H. (2008). Oxidative stress in subarachnoid haemorrhage: Significance in acute brain injury and vasospasm. Acta Neurochir. Suppl. 104, 33–41. doi:10.1007/978-3-211-75718-5_7
Barrera, G., Gentile, F., Pizzimenti, S., Canuto, R. A., Daga, M., Arcaro, A., et al. (2016). Mitochondrial dysfunction in cancer and neurodegenerative diseases: Spotlight on fatty acid oxidation and lipoperoxidation products. Antioxidants (Basel) 5, 7. doi:10.3390/antiox5010007
Baur, J. A., and Sinclair, D. A. (2006). Therapeutic potential of resveratrol: The in vivo evidence. Nat. Rev. Drug Discov. 5, 493–506. doi:10.1038/nrd2060
Bederson, J. B., Connolly, E. S., Batjer, H. H., Dacey, R. G., Dion, J. E., Diringer, M. N., et al. (2009). Guidelines for the management of aneurysmal subarachnoid hemorrhage: A statement for healthcare professionals from a special writing group of the stroke council. Am. Heart Assoc. 40, 994–1025. doi:10.1161/STROKEAHA.108.191395
Bharti, A., and Garg, N. (2019). SA and AM symbiosis modulate antioxidant defense mechanisms and asada pathway in chickpea genotypes under salt stress. Ecotoxicol. Environ. Saf. 178, 66–78. doi:10.1016/j.ecoenv.2019.04.025
Boocock, D. J., Patel, K. R., Faust, G. E., Normolle, D. P., Marczylo, T. H., Crowell, J. A., et al. (2007). Quantitation of trans-resveratrol and detection of its metabolites in human plasma and urine by high performance liquid chromatography. J. Chromatogr. B Anal. Technol. Biomed. Life Sci. 848, 182–187. doi:10.1016/j.jchromb.2006.10.017
Borra, M. T., Smith, B. C., and Denu, J. M. (2005). Mechanism of human SIRT1 activation by resveratrol. J. Biol. Chem. 280, 17187–17195. doi:10.1074/jbc.M501250200
Cai, H., and Harrison, D. G. (2000). Endothelial dysfunction in cardiovascular diseases: The role of oxidant stress. Circ. Res. 87, 840–844. doi:10.1161/01.res.87.10.840
Calabrese, E. J., Mattson, M. P., and Calabrese, V. (2010). Resveratrol commonly displays hormesis: Occurrence and biomedical significance. Hum. Exp. Toxicol. 29, 980–1015. doi:10.1177/0960327110383625
Calamini, B., Ratia, K., Malkowski, M. G., Cuendet, M., Pezzuto, J. M., Santarsiero, B. D., et al. (2010). Pleiotropic mechanisms facilitated by resveratrol and its metabolites. Biochem. J. 429, 273–282. doi:10.1042/BJ20091857
Canugovi, C., Maynard, S., Bayne, A. C., Sykora, P., Tian, J., de Souza-Pinto, N. C., et al. (2010). The mitochondrial transcription factor A functions in mitochondrial base excision repair. DNA Repair (Amst) 9, 1080–1089. doi:10.1016/j.dnarep.2010.07.009
Cao, Z., and Li, Y. (2004). Potent induction of cellular antioxidants and phase 2 enzymes by resveratrol in cardiomyocytes: Protection against oxidative and electrophilic injury. Eur. J. Pharmacol. 489, 39–48. doi:10.1016/j.ejphar.2004.02.031
Chaban, Y., Boekema, E. J., and Dudkina, N. V. (2014). Structures of mitochondrial oxidative phosphorylation supercomplexes and mechanisms for their stabilisation. Biochim. Biophys. Acta 1837, 418–426. doi:10.1016/j.bbabio.2013.10.004
Chang, C., Zhao, Y., Song, G., and She, K. (2018). Resveratrol protects hippocampal neurons against cerebral ischemia-reperfusion injury via modulating JAK/ERK/STAT signaling pathway in rats. J. Neuroimmunol. 315, 9–14. doi:10.1016/j.jneuroim.2017.11.015
Chen, F., Qian, L. H., Deng, B., Liu, Z. M., Zhao, Y., and Le, Y. Y. (2013). Resveratrol protects vascular endothelial cells from high glucose-induced apoptosis through inhibition of NADPH oxidase activation-driven oxidative stress. CNS Neurosci. Ther. 19, 675–681. doi:10.1111/cns.12131
Chen, H., Yoshioka, H., Kim, G. S., Jung, J. E., Okami, N., Sakata, H., et al. (2011). Oxidative stress in ischemic brain damage: Mechanisms of cell death and potential molecular targets for neuroprotection. Antioxid. Redox Signal. 14, 1505–1517. doi:10.1089/ars.2010.3576
Chen, J., Bai, Q., Zhao, Z., Sui, H., and Xie, X. (2016). Resveratrol improves delayed r-tPA treatment outcome by reducing MMPs. Acta Neurol. Scand. 134, 54–60. doi:10.1111/ane.12511
Chen, J., Jin, K., Chen, M., Pei, W., Kawaguchi, K., Greenberg, D. A., et al. (1997). Early detection of DNA strand breaks in the brain after transient focal ischemia: Implications for the role of DNA damage in apoptosis and neuronal cell death. J. Neurochem. 69, 232–245. doi:10.1046/j.1471-4159.1997.69010232.x
Chen, M. L., Yi, L., Jin, X., Xie, Q., Zhang, T., Zhou, X., et al. (2013). Absorption of resveratrol by vascular endothelial cells through passive diffusion and an SGLT1-mediated pathway. J. Nutr. Biochem. 24, 1823–1829. doi:10.1016/j.jnutbio.2013.04.003
Chen, S. D., Lin, T. K., Lin, J. W., Yang, D. I., Lee, S. Y., Shaw, F. Z., et al. (2010). Activation of calcium/calmodulin-dependent protein kinase IV and peroxisome proliferator-activated receptor γ coactivator-1α signaling pathway protects against neuronal injury and promotes mitochondrial biogenesis in the hippocampal CA1 subfield after transient global ischemia. J. Neurosci. Res. 88, 3144–3154. doi:10.1002/jnr.22469
Chen, W., Guo, C., Jia, Z., Wang, J., Xia, M., Li, C., et al. (2020). Inhibition of mitochondrial ROS by MitoQ alleviates white matter injury and improves outcomes after intracerebral haemorrhage in mice. Oxid. Med. Cell. Longev. 2020, 8285065. doi:10.1155/2020/8285065
Chiang, C. K., Hsu, S. P., Wu, C. T., Huang, J. W., Cheng, H. T., Chang, Y. W., et al. (2011). Endoplasmic reticulum stress implicated in the development of renal fibrosis. Mol. Med. 17, 1295–1305. doi:10.2119/molmed.2011.00131
Chow, H. H., Garland, L. L., Heckman-Stoddard, B. M., Hsu, C. H., Butler, V. D., Cordova, C. A., et al. (2014). A pilot clinical study of resveratrol in postmenopausal women with high body mass index: Effects on systemic sex steroid hormones. J. Transl. Med. 12, 223. doi:10.1186/s12967-014-0223-0
Chowdhury, R., Stevens, S., Gorman, D., Pan, A., Warnakula, S., Chowdhury, S., et al. (2012). Association between fish consumption, long chain omega 3 fatty acids, and risk of cerebrovascular disease: Systematic review and meta-analysis. Bmj 345, e6698. doi:10.1136/bmj.e6698
Cohen, S. M., Arnold, L. L., Eldan, M., Lewis, A. S., and Beck, B. D. (2006). Methylated arsenicals: The implications of metabolism and carcinogenicity studies in rodents to human risk assessment. Crit. Rev. Toxicol. 36, 99–133. doi:10.1080/10408440500534230
Correia, S. C., Carvalho, C., Cardoso, S., Santos, R. X., Santos, M. S., Oliveira, C. R., et al. (2010). Mitochondrial preconditioning: A potential neuroprotective strategy. Front. Aging Neurosci. 2, 138. doi:10.3389/fnagi.2010.00138
Cottart, C. H., Nivet-Antoine, V., Laguillier-Morizot, C., and Beaudeux, J. L. (2010). Resveratrol bioavailability and toxicity in humans. Mol. Nutr. Food Res. 54, 7–16. doi:10.1002/mnfr.200900437
de Baaij, J. H., Hoenderop, J. G., and Bindels, R. J. (2012). Regulation of magnesium balance: Lessons learned from human genetic disease. Clin. Kidney J. 5, i15–i24. doi:10.1093/ndtplus/sfr164
Della-Morte, D., Dave, K. R., DeFazio, R. A., Bao, Y. C., Raval, A. P., and Perez-Pinzon, M. A. (2009). Resveratrol pretreatment protects rat brain from cerebral ischemic damage via a sirtuin 1-uncoupling protein 2 pathway. Neuroscience 159, 993–1002. doi:10.1016/j.neuroscience.2009.01.017
Delmas, D., Aires, V., Limagne, E., Dutartre, P., Mazué, F., Ghiringhelli, F., et al. (2011). Transport, stability, and biological activity of resveratrol. Ann. N. Y. Acad. Sci. 1215, 48–59. doi:10.1111/j.1749-6632.2010.05871.x
Detampel, P., Beck, M., Krähenbühl, S., and Huwyler, J. (2012). Drug interaction potential of resveratrol. Drug Metab. Rev. 44, 253–265. doi:10.3109/03602532.2012.700715
Diwan, D., Vellimana, A. K., Aum, D. J., Clarke, J., Nelson, J. W., Lawrence, M., et al. (2021). Sirtuin 1 mediates protection against delayed cerebral ischemia in subarachnoid hemorrhage in response to hypoxic postconditioning. J. Am. Heart Assoc. 10, e021113. doi:10.1161/JAHA.121.021113
Dou, Z., Rong, X., Zhao, E., Zhang, L., and Lv, Y. (2019). Neuroprotection of resveratrol against focal cerebral ischemia/reperfusion injury in mice through a mechanism targeting gut-brain Axis. Cell. Mol. Neurobiol. 39, 883–898. doi:10.1007/s10571-019-00687-3
Dyrkheeva, N. S., Lebedeva, N. A., and Lavrik, O. I. (2016). AP endonuclease 1 as a Key enzyme in repair of apurinic/apyrimidinic sites. Biochemistry. 81, 951–967. doi:10.1134/S0006297916090042
Elshaer, M., Chen, Y., Wang, X. J., and Tang, X. (2018). Resveratrol: An overview of its anti-cancer mechanisms. Life Sci. 207, 340–349. doi:10.1016/j.lfs.2018.06.028
Enciu, A. M., Constantinescu, S. N., Popescu, L. M., Mureşanu, D. F., and Popescu, B. O. (2011). Neurobiology of vascular dementia. J. Aging Res. 2011, 401604. doi:10.4061/2011/401604
Fang, K. M., Cheng, F. C., Huang, Y. L., Chung, S. Y., Jian, Z. Y., and Lin, M. C. (2013). Trace element, antioxidant activity, and lipid peroxidation levels in brain cortex of gerbils after cerebral ischemic injury. Biol. Trace Elem. Res. 152, 66–74. doi:10.1007/s12011-012-9596-1
Filograna, R., Mennuni, M., Alsina, D., and Larsson, N. G. (2021). Mitochondrial DNA copy number in human disease: The more the better? FEBS Lett. 595, 976–1002. doi:10.1002/1873-3468.14021
Flynn, J. M., and Melov, S. (2013). SOD2 in mitochondrial dysfunction and neurodegeneration. Free Radic. Biol. Med. 62, 4–12. doi:10.1016/j.freeradbiomed.2013.05.027
Fu, M., Guo, J., Zhao, Y., Zhang, Y., Zhang, Y., Wang, Z., et al. (2021). Characteristics of fall-related fractures in older adults with cerebrovascular disease: A cross-sectional study. Clin. Interv. Aging 16, 1337–1346. doi:10.2147/CIA.S316739
Girbovan, C., Kent, P., Merali, Z., and Plamondon, H. (2016). Dose-related effects of chronic resveratrol administration on neurogenesis, angiogenesis, and corticosterone secretion are associated with improved spatial memory retention following global cerebral ischemia. Nutr. Neurosci. 19, 352–368. doi:10.1179/1476830515Y.0000000020
Girbovan, C., and Plamondon, H. (2015). Resveratrol downregulates type-1 glutamate transporter expression and microglia activation in the hippocampus following cerebral ischemia reperfusion in rats. Brain Res. 1608, 203–214. doi:10.1016/j.brainres.2015.02.038
Gocmez, S. S., Şahin, T. D., Yazir, Y., Duruksu, G., Eraldemir, F. C., Polat, S., et al. (2019). Resveratrol prevents cognitive deficits by attenuating oxidative damage and inflammation in rat model of streptozotocin diabetes induced vascular dementia. Physiol. Behav. 201, 198–207. doi:10.1016/j.physbeh.2018.12.012
Goldberg, D. M., Yan, J., and Soleas, G. J. (2003). Absorption of three wine-related polyphenols in three different matrices by healthy subjects. Clin. Biochem. 36, 79–87. doi:10.1016/s0009-9120(02)00397-1
Good, P. F., Perl, D. P., Bierer, L. M., and Schmeidler, J. (1992). Selective accumulation of aluminum and iron in the neurofibrillary tangles of alzheimer's disease: A laser microprobe (LAMMA) study. Ann. Neurol. 31, 286–292. doi:10.1002/ana.410310310
Grewal, A. K., Singh, N., and Singh, T. G. (2019). Effects of resveratrol postconditioning on cerebral ischemia in mice: Role of the sirtuin-1 pathway. Can. J. Physiol. Pharmacol. 97, 1094–1101. doi:10.1139/cjpp-2019-0188
Han, B., Jiang, W., Liu, H., Wang, J., Zheng, K., Cui, P., et al. (2020). Upregulation of neuronal PGC-1α ameliorates cognitive impairment induced by chronic cerebral hypoperfusion. Theranostics 10, 2832–2848. doi:10.7150/thno.37119
Hayes, J. D., McMahon, M., Chowdhry, S., and Dinkova-Kostova, A. T. (2010). Cancer chemoprevention mechanisms mediated through the Keap1-Nrf2 pathway. Antioxid. Redox Signal. 13, 1713–1748. doi:10.1089/ars.2010.3221
He, Q., Li, Z., Wang, Y., Hou, Y., Li, L., and Zhao, J. (2017). Resveratrol alleviates cerebral ischemia/reperfusion injury in rats by inhibiting NLRP3 inflammasome activation through Sirt1-dependent autophagy induction. Int. Immunopharmacol. 50, 208–215. doi:10.1016/j.intimp.2017.06.029
He, S., Yaung, J., Kim, Y. H., Barron, E., Ryan, S. J., and Hinton, D. R. (2008). Endoplasmic reticulum stress induced by oxidative stress in retinal pigment epithelial cells. Graefes Arch. Clin. Exp. Ophthalmol. 246, 677–683. doi:10.1007/s00417-008-0770-2
Horio, Y., Hayashi, T., Kuno, A., and Kunimoto, R. (2011). Cellular and molecular effects of sirtuins in health and disease. Clin. Sci. 121, 191–203. doi:10.1042/CS20100587
Hou, Y., Wang, K., Wan, W., Cheng, Y., Pu, X., and Ye, X. (2018). Resveratrol provides neuroprotection by regulating the JAK2/STAT3/PI3K/AKT/mTOR pathway after stroke in rats. Genes Dis. 5, 245–255. doi:10.1016/j.gendis.2018.06.001
Huang, L., Wan, J., Chen, Y., Wang, Z., Hui, L., Li, Y., et al. (2013). Inhibitory effects of p38 inhibitor against mitochondrial dysfunction in the early brain injury after subarachnoid hemorrhage in mice. Brain Res. 1517, 133–140. doi:10.1016/j.brainres.2013.04.010
Ilieva, E. V., Ayala, V., Jové, M., Dalfó, E., Cacabelos, D., Povedano, M., et al. (2007). Oxidative and endoplasmic reticulum stress interplay in sporadic amyotrophic lateral sclerosis. Brain 130, 3111–3123. doi:10.1093/brain/awm190
Jaishankar, M., Tseten, T., Anbalagan, N., Mathew, B. B., and Beeregowda, K. N. (2014). Toxicity, mechanism and health effects of some heavy metals. Interdiscip. Toxicol. 7, 60–72. doi:10.2478/intox-2014-0009
Jankowska, K. (2017). Premature ovarian failure. Przeglad menopauzalny = Menopause Rev. 16, 51–56. doi:10.5114/pm.2017.68592
Jellinger, K. A. (2007). The enigma of vascular cognitive disorder and vascular dementia. Acta Neuropathol. 113, 349–388. doi:10.1007/s00401-006-0185-2
Jeong, S. I., Shin, J. A., Cho, S., Kim, H. W., Lee, J. Y., Kang, J. L., et al. (2016). Resveratrol attenuates peripheral and brain inflammation and reduces ischemic brain injury in aged female mice. Neurobiol. Aging 44, 74–84. doi:10.1016/j.neurobiolaging.2016.04.007
Jia, J. Y., Tan, Z. G., Liu, M., and Jiang, Y. G. (2017). Apurinic/apyrimidinic endonuclease 1 (APE1) contributes to resveratrol-induced neuroprotection against oxygen-glucose deprivation and re-oxygenation injury in HT22 cells: Involvement in reducing oxidative DNA damage. Mol. Med. Rep. 16, 9786–9794. doi:10.3892/mmr.2017.7799
Jomova, K., and Valko, M. (2011). Advances in metal-induced oxidative stress and human disease. Toxicology 283, 65–87. doi:10.1016/j.tox.2011.03.001
Jornayvaz, F. R., and Shulman, G. I. (2010). Regulation of mitochondrial biogenesis. Essays Biochem. 47, 69–84. doi:10.1042/bse0470069
Kennedy, D. O., Wightman, E. L., Reay, J. L., Lietz, G., Okello, E. J., Wilde, A., et al. (2010). Effects of resveratrol on cerebral blood flow variables and cognitive performance in humans: A double-blind, placebo-controlled, crossover investigation. Am. J. Clin. Nutr. 91, 1590–1597. doi:10.3945/ajcn.2009.28641
Khan, M. A., Chen, H. C., Wan, X. X., Tania, M., Xu, A. H., Chen, F. Z., et al. (2013). Regulatory effects of resveratrol on antioxidant enzymes: A mechanism of growth inhibition and apoptosis induction in cancer cells. Mol. Cells 35, 219–225. doi:10.1007/s10059-013-2259-z
Khanduja, K. L., and Bhardwaj, A. (2003). Stable free radical scavenging and antiperoxidative properties of resveratrol compared in vitro with some other bioflavonoids. Indian J. biochem. Biophys. 40, 416–422.
Khoury, N., Xu, J., Stegelmann, S. D., Jackson, C. W., Koronowski, K. B., Dave, K. R., et al. (2019). Resveratrol preconditioning induces genomic and metabolic adaptations within the long-term window of cerebral ischemic tolerance leading to bioenergetic efficiency. Mol. Neurobiol. 56, 4549–4565. doi:10.1007/s12035-018-1380-6
Kim, R. H., Peters, M., Jang, Y., Shi, W., Pintilie, M., Fletcher, G. C., et al. (2005). DJ-1, a novel regulator of the tumor suppressor PTEN. Cancer Cell 7, 263–273. doi:10.1016/j.ccr.2005.02.010
Kim, Y. C., Kitaura, H., Taira, T., Iguchi-Ariga, S. M., and Ariga, H. (2009). Oxidation of DJ-1-dependent cell transformation through direct binding of DJ-1 to PTEN. Int. J. Oncol. 35, 1331–1341. doi:10.3892/ijo_00000451
Kipp, J. L., and Ramirez, V. D. (2001). Effect of estradiol, diethylstilbestrol, and resveratrol on F0F1-ATPase activity from mitochondrial preparations of rat heart, liver, and brain. Endocrine 15, 165–175. doi:10.1385/ENDO:15:2:165
Kobayashi, A., Kang, M. I., Okawa, H., Ohtsuji, M., Zenke, Y., Chiba, T., et al. (2004). Oxidative stress sensor Keap1 functions as an adaptor for Cul3-based E3 ligase to regulate proteasomal degradation of Nrf2. Mol. Cell. Biol. 24, 7130–7139. doi:10.1128/MCB.24.16.7130-7139.2004
Kovacic, P., and Somanathan, R. (2010). Multifaceted approach to resveratrol bioactivity: Focus on antioxidant action, cell signaling and safety. Oxid. Med. Cell. Longev. 3, 86–100. doi:10.4161/oxim.3.2.11147
Kudo, T., Kanemoto, S., Hara, H., Morimoto, N., Morihara, T., Kimura, R., et al. (2008). A molecular chaperone inducer protects neurons from ER stress. Cell Death Differ. 15, 364–375. doi:10.1038/sj.cdd.4402276
Kuhnle, G., Spencer, J. P., Chowrimootoo, G., Schroeter, H., Debnam, E. S., Srai, S. K., et al. (2000). Resveratrol is absorbed in the small intestine as resveratrol glucuronide. Biochem. Biophys. Res. Commun. 272, 212–217. doi:10.1006/bbrc.2000.2750
Kumar, A., and Mittal, R. (2017). Nrf2: A potential therapeutic target for diabetic neuropathy. Inflammopharmacology 25, 393–402. doi:10.1007/s10787-017-0339-y
Laev, S. S., Salakhutdinov, N. F., and Lavrik, O. I. (2017). Inhibitors of nuclease and redox activity of apurinic/apyrimidinic endonuclease 1/redox effector factor 1 (APE1/Ref-1). Bioorg. Med. Chem. 25, 2531–2544. doi:10.1016/j.bmc.2017.01.028
Lan, J., Li, W., Zhang, F., Sun, F. Y., Nagayama, T., O'Horo, C., et al. (2003). Inducible repair of oxidative DNA lesions in the rat brain after transient focal ischemia and reperfusion. J. Cereb. Blood Flow. Metab. 23, 1324–1339. doi:10.1097/01.WCB.0000091540.60196.F2
Lansberg, M. G., O'Donnell, M. J., Khatri, P., Lang, E. S., Nguyen-Huynh, M. N., Schwartz, N. E., et al. (2012). Antithrombotic and thrombolytic therapy for ischemic stroke: Antithrombotic therapy and prevention of thrombosis, 9th ed: American college of chest physicians evidence-based clinical practice guidelines. Chest 141, e601S–e636S. doi:10.1378/chest.11-2302
Leak, R. K., Li, P., Zhang, F., Sulaiman, H. H., Weng, Z., Wang, G., et al. (2015). Apurinic/apyrimidinic endonuclease 1 upregulation reduces oxidative DNA damage and protects hippocampal neurons from ischemic injury. Antioxid. Redox Signal. 22, 135–148. doi:10.1089/ars.2013.5511
Ledbetter, L. N., Burns, J., Shih, R. Y., Ajam, A. A., Brown, M. D., Chakraborty, S., et al. (2021). ACR appropriateness Criteria® cerebrovascular diseases-aneurysm, vascular malformation, and subarachnoid hemorrhage. J. Am. Coll. Radiol. 18, S283–S304. doi:10.1016/j.jacr.2021.08.012
Lei, J., and Chen, Q. (2018). Resveratrol attenuates brain damage in permanent focal cerebral ischemia via activation of PI3K/Akt signaling pathway in rats. Neurol. Res. 40, 1014–1020. doi:10.1080/01616412.2018.1509826
Lei, J. R., Tu, X. K., Wang, Y., Tu, D. W., and Shi, S. S. (2019). Resveratrol downregulates the TLR4 signaling pathway to reduce brain damage in a rat model of focal cerebral ischemia. Exp. Ther. Med. 17, 3215–3221. doi:10.3892/etm.2019.7324
Li, H., Wang, J., Wang, P., Rao, Y., and Chen, L. (2016). Resveratrol reverses the synaptic plasticity deficits in a chronic cerebral hypoperfusion rat model. J. Stroke Cerebrovasc. Dis. 25, 122–128. doi:10.1016/j.jstrokecerebrovasdis.2015.09.004
Li, J., Ma, X., Yu, W., Lou, Z., Mu, D., Wang, Y., et al. (2012). Reperfusion promotes mitochondrial dysfunction following focal cerebral ischemia in rats. PLoS One 7, e46498. doi:10.1371/journal.pone.0046498
Li, W., Tan, C., Liu, Y., Liu, X., Wang, X., Gui, Y., et al. (2015). Resveratrol ameliorates oxidative stress and inhibits aquaporin 4 expression following rat cerebral ischemia-reperfusion injury. Mol. Med. Rep. 12, 7756–7762. doi:10.3892/mmr.2015.4366
Li, Y. G., Zhu, W., Tao, J. P., Xin, P., Liu, M. Y., Li, J. B., et al. (2013). Resveratrol protects cardiomyocytes from oxidative stress through SIRT1 and mitochondrial biogenesis signaling pathways. Biochem. Biophys. Res. Commun. 438, 270–276. doi:10.1016/j.bbrc.2013.07.042
Li, Z., Fang, F., Wang, Y., and Wang, L. (2016). Resveratrol protects CA1 neurons against focal cerebral ischemic reperfusion-induced damage via the ERK-CREB signaling pathway in rats. Pharmacol. Biochem. Behav. 146-147, 21–27. doi:10.1016/j.pbb.2016.04.007
Lin, C. H., Nicol, C. J. B., Cheng, Y. C., Yen, C., Wang, Y. S., and Chiang, M. C. (2020). Neuroprotective effects of resveratrol against oxygen glucose deprivation induced mitochondrial dysfunction by activation of AMPK in SH-SY5Y cells with 3D gelatin scaffold. Brain Res. 1726, 146492. doi:10.1016/j.brainres.2019.146492
Lin, M. C., Liu, C. C., Lin, Y. C., and Liao, C. S. (2021). Resveratrol protects against cerebral ischemic injury via restraining lipid peroxidation, transition elements, and toxic metal levels, but enhancing anti-oxidant activity. Antioxidants (Basel) 10, 1515. doi:10.3390/antiox10101515
Lin, Y., Chen, F., Zhang, J., Wang, T., Wei, X., Wu, J., et al. (2013). Neuroprotective effect of resveratrol on ischemia/reperfusion injury in rats through TRPC6/CREB pathways. J. Mol. Neurosci. 50, 504–513. doi:10.1007/s12031-013-9977-8
Liu, L., Huang, W., Wang, J., Song, H., Cen, J., and Ji, B. (2017). Anthraquinone derivative exerted hormetic effect on the apoptosis in oxygen-glucose deprivation-induced PC12 cells via ERK and Akt activated Nrf2/HO-1 signaling pathway. Chem. Biol. Interact. 262, 1–11. doi:10.1016/j.cbi.2016.12.001
Lopez, M. S., Dempsey, R. J., and Vemuganti, R. (2015). Resveratrol neuroprotection in stroke and traumatic CNS injury. Neurochem. Int. 89, 75–82. doi:10.1016/j.neuint.2015.08.009
Lu, S. C. (2013). Glutathione synthesis. Biochim. Biophys. Acta 1830, 3143–3153. doi:10.1016/j.bbagen.2012.09.008
Ludwig, D. L., MacInnes, M. A., Takiguchi, Y., Purtymun, P. E., Henrie, M., Flannery, M., et al. (1998). A murine AP-endonuclease gene-targeted deficiency with post-implantation embryonic progression and ionizing radiation sensitivity. Mutat. Res. 409, 17–29. doi:10.1016/s0921-8777(98)00039-1
Ma, S., Fan, L., Li, J., Zhang, B., and Yan, Z. (2020). Resveratrol promoted the M2 polarization of microglia and reduced neuroinflammation after cerebral ischemia by inhibiting miR-155. Int. J. Neurosci. 130, 817–825. doi:10.1080/00207454.2019.1707817
Ma, X., Sun, Z., Liu, Y., Jia, Y., Zhang, B., and Zhang, J. (2013). Resveratrol improves cognition and reduces oxidative stress in rats with vascular dementia. Neural Regen. Res. 8, 2050–2059. doi:10.3969/j.issn.1673-5374.2013.22.004
Madamanchi, N. R., and Runge, M. S. (2007). Mitochondrial dysfunction in atherosclerosis. Circ. Res. 100, 460–473. doi:10.1161/01.RES.0000258450.44413.96
McNally, R. S., Davis, B. K., Clements, C. M., Accavitti-Loper, M. A., Mak, T. W., and Ting, J. P. (2011). DJ-1 enhances cell survival through the binding of Cezanne, a negative regulator of NF-kappaB. J. Biol. Chem. 286, 4098–4106. doi:10.1074/jbc.M110.147371
Miller, W. H., Schipper, H. M., Lee, J. S., Singer, J., and Waxman, S. (2002). Mechanisms of action of arsenic trioxide. Cancer Res. 62, 3893–3903.
Moon, R. T., Kohn, A. D., De Ferrari, G. V., and Kaykas, A. (2004). WNT and beta-catenin signalling: Diseases and therapies. Nat. Rev. Genet. 5, 691–701. doi:10.1038/nrg1427
Morin, C., Zini, R., Albengres, E., Bertelli, A. A., Bertelli, A., and Tillement, J. P. (2003). Evidence for resveratrol-induced preservation of brain mitochondria functions after hypoxia-reoxygenation. Drugs Exp. Clin. Res. 29, 227–233.
Morris, G., Anderson, G., Dean, O., Berk, M., Galecki, P., Martin-Subero, M., et al. (2014). The glutathione system: A new drug target in neuroimmune disorders. Mol. Neurobiol. 50, 1059–1084. doi:10.1007/s12035-014-8705-x
Moussa, C., Hebron, M., Huang, X., Ahn, J., Rissman, R. A., Aisen, P. S., et al. (2017). Resveratrol regulates neuro-inflammation and induces adaptive immunity in Alzheimer's disease. J. Neuroinflammation 14, 1. doi:10.1186/s12974-016-0779-0
Murphy, C. J., and Oudit, G. Y. (2010). Iron-overload cardiomyopathy: Pathophysiology, diagnosis, and treatment. J. Card. Fail. 16, 888–900. doi:10.1016/j.cardfail.2010.05.009
Murphy, M. P. (2009). How mitochondria produce reactive oxygen species. Biochem. J. 417, 1–13. doi:10.1042/BJ20081386
Nakka, V. P., Prakash-Babu, P., and Vemuganti, R. (2016). Crosstalk between endoplasmic reticulum stress, oxidative stress, and autophagy: Potential therapeutic targets for acute CNS injuries. Mol. Neurobiol. 53, 532–544. doi:10.1007/s12035-014-9029-6
Nanjaiah, H., and Vallikannan, B. (2019). Enhanced phosphorylation of AMPK by lutein and oxidised lutein that lead to mitochondrial biogenesis in hyperglycemic HepG2 cells. J. Cell. Biochem. 120, 15255–15267. doi:10.1002/jcb.28793
Orallo, F. (2006). Comparative studies of the antioxidant effects of cis- and trans-resveratrol. Curr. Med. Chem. 13, 87–98. doi:10.2174/092986706775197962
Orellana-Urzúa, S., Rojas, I., Líbano, L., and Rodrigo, R. (2020). Pathophysiology of ischemic stroke: Role of oxidative stress. Curr. Pharm. Des. 26, 4246–4260. doi:10.2174/1381612826666200708133912
Ortiz-Ruiz, A., Ruiz-Heredia, Y., Morales, M. L., Aguilar-Garrido, P., García-Ortiz, A., Valeri, A., et al. (2021). Myc-related mitochondrial activity as a novel target for multiple myeloma. Cancers (Basel) 13, 1662. doi:10.3390/cancers13071662
Østergaard, L., Engedal, T. S., Moreton, F., Hansen, M. B., Wardlaw, J. M., Dalkara, T., et al. (2016). Cerebral small vessel disease: Capillary pathways to stroke and cognitive decline. J. Cereb. Blood Flow. Metab. 36, 302–325. doi:10.1177/0271678X15606723
Ozacmak, V. H., Sayan-Ozacmak, H., and Barut, F. (2016). Chronic treatment with resveratrol, a natural polyphenol found in grapes, alleviates oxidative stress and apoptotic cell death in ovariectomized female rats subjected to chronic cerebral hypoperfusion. Nutr. Neurosci. 19, 176–186. doi:10.1179/1476830515Y.0000000027
Pan, C., Giraldo, G. S., Prentice, H., and Wu, J. Y. (2010). Taurine protection of PC12 cells against endoplasmic reticulum stress induced by oxidative stress. J. Biomed. Sci. 17 (1), S17. doi:10.1186/1423-0127-17-S1-S17
Papa, S., Martino, P. L., Capitanio, G., Gaballo, A., De Rasmo, D., Signorile, A., et al. (2012). The oxidative phosphorylation system in mammalian mitochondria. Adv. Exp. Med. Biol. 942, 3–37. doi:10.1007/978-94-007-2869-1_1
Park, D. J., Kang, J. B., Shah, F. A., and Koh, P. O. (2019). Resveratrol modulates the Akt/GSK-3β signaling pathway in a middle cerebral artery occlusion animal model. Lab. Anim. Res. 35, 18. doi:10.1186/s42826-019-0019-8
Peng, W., Cai, G., Xia, Y., Chen, J., Wu, P., Wang, Z., et al. (2019). Mitochondrial dysfunction in atherosclerosis. DNA Cell Biol. 38, 597–606. doi:10.1089/dna.2018.4552
Picca, A., and Lezza, A. M. (2015). Regulation of mitochondrial biogenesis through TFAM-mitochondrial DNA interactions: Useful insights from aging and calorie restriction studies. Mitochondrion 25, 67–75. doi:10.1016/j.mito.2015.10.001
Pineda-Ramírez, N., Alquisiras-Burgos, I., Ortiz-Plata, A., Ruiz-Tachiquín, M. E., Espinoza-Rojo, M., and Aguilera, P. (2020). Resveratrol activates neuronal autophagy through AMPK in the ischemic brain. Mol. Neurobiol. 57, 1055–1069. doi:10.1007/s12035-019-01803-6
Posadino, A. M., Cossu, A., Giordo, R., Zinellu, A., Sotgia, S., Vardeu, A., et al. (2015). Resveratrol alters human endothelial cells redox state and causes mitochondrial-dependent cell death. Food Chem. Toxicol. 78, 10–16. doi:10.1016/j.fct.2015.01.017
Qian, C., Jin, J., Chen, J., Li, J., Yu, X., Mo, H., et al. (2017). SIRT1 activation by resveratrol reduces brain edema and neuronal apoptosis in an experimental rat subarachnoid hemorrhage model. Mol. Med. Rep. 16, 9627–9635. doi:10.3892/mmr.2017.7773
Ramírez-Garza, S. L., Laveriano-Santos, E. P., Marhuenda-Muñoz, M., Storniolo, C. E., Tresserra-Rimbau, A., Vallverdú-Queralt, A., et al. (2018). Health effects of resveratrol: Results from human intervention trials. Nutrients 10, E1892. doi:10.3390/nu10121892
Ren, J., Fan, C., Chen, N., Huang, J., and Yang, Q. (2011). Resveratrol pretreatment attenuates cerebral ischemic injury by upregulating expression of transcription factor Nrf2 and HO-1 in rats. Neurochem. Res. 36, 2352–2362. doi:10.1007/s11064-011-0561-8
Resnick, S. M., and Henderson, V. W. (2002). Hormone therapy and risk of alzheimer disease: A critical time. Jama 288, 2170–2172. doi:10.1001/jama.288.17.2170
Ro, J. H., Liu, C. C., and Lin, M. C. (2021). Resveratrol mitigates cerebral ischemic injury by altering levels of trace elements, toxic metal, lipid peroxidation, and antioxidant activity. Biol. Trace Elem. Res. 199, 3718–3727. doi:10.1007/s12011-020-02497-x
Rotches-Ribalta, M., Andres-Lacueva, C., Estruch, R., Escribano, E., and Urpi-Sarda, M. (2012). Pharmacokinetics of resveratrol metabolic profile in healthy humans after moderate consumption of red wine and grape extract tablets. Pharmacol. Res. 66, 375–382. doi:10.1016/j.phrs.2012.08.001
Saleh, M. C., Connell, B. J., and Saleh, T. M. (2013). Resveratrol induced neuroprotection is mediated via both estrogen receptor subtypes, ER(α) and ER(β). Neurosci. Lett. 548, 217–221. doi:10.1016/j.neulet.2013.05.057
Salehi, B., Mishra, A. P., Nigam, M., Sener, B., Kilic, M., Sharifi-Rad, M., et al. (2018). Resveratrol: A double-edged sword in health benefits. Biomedicines 6, E91. doi:10.3390/biomedicines6030091
Scarpulla, R. C. (2011). Metabolic control of mitochondrial biogenesis through the PGC-1 family regulatory network. Biochim. Biophys. Acta 1813, 1269–1278. doi:10.1016/j.bbamcr.2010.09.019
Scarpulla, R. C. (2008). Transcriptional paradigms in mammalian mitochondrial biogenesis and function. Physiol. Rev. 88, 611–638. doi:10.1152/physrev.00025.2007
Schreiber, S. N., Emter, R., Hock, M. B., Knutti, D., Cardenas, J., Podvinec, M., et al. (2004). The estrogen-related receptor alpha (ERRalpha) functions in PPARgamma coactivator 1alpha (PGC-1alpha)-induced mitochondrial biogenesis. Proc. Natl. Acad. Sci. U. S. A. 101, 6472–6477. doi:10.1073/pnas.0308686101
Shang, Y., Xue, W., Kong, J., Chen, Y., Qiu, X., An, X., et al. (2022). Ultrafine black carbon caused mitochondrial oxidative stress, mitochondrial dysfunction and mitophagy in SH-SY5Y cells. Sci. Total Environ. 813, 151899. doi:10.1016/j.scitotenv.2021.151899
Shankar, S., Singh, G., and Srivastava, R. K. (2007). Chemoprevention by resveratrol: Molecular mechanisms and therapeutic potential. Front. Biosci. 12, 4839–4854. doi:10.2741/2432
Shao, A. W., Wu, H. J., Chen, S., Ammar, A. B., Zhang, J. M., and Hong, Y. (2014). Resveratrol attenuates early brain injury after subarachnoid hemorrhage through inhibition of NF-κB-dependent inflammatory/MMP-9 pathway. CNS Neurosci. Ther. 20, 182–185. doi:10.1111/cns.12194
Shuwen, H., Xi, Y., and Yuefen, P. (2017). Can mitochondria DNA provide a novel biomarker for evaluating the risk and prognosis of colorectal cancer? Dis. Markers 2017, 5189803. doi:10.1155/2017/5189803
Soleas, G. J., Angelini, M., Grass, L., Diamandis, E. P., and Goldberg, D. M. (2001). Absorption of trans-resveratrol in rats. Methods Enzymol. 335, 145–154. doi:10.1016/s0076-6879(01)35239-4
Song, E., Su, C., Fu, J., Xia, X., Yang, S., Xiao, C., et al. (2014). Selenium supplementation shows protective effects against patulin-induced brain damage in mice via increases in GSH-related enzyme activity and expression. Life Sci. 109, 37–43. doi:10.1016/j.lfs.2014.05.022
Souissi, R., Boubakker, A., Souissi, H., Abdelrazek, A., Badri, M., Bziouech, S., et al. (2010). Prediction of cerebral vasospasm in patients with aneurysmal subarachnoid hemorrhage using jugular bulb oximetry monitoring: Preliminary results. Crit. Care (Houten). 14, 343. doi:10.1186/cc8575
Stetler, R. A., Gao, Y., Leak, R. K., Weng, Z., Shi, Y., Zhang, L., et al. (2016). APE1/Ref-1 facilitates recovery of gray and white matter and neurological function after mild stroke injury. Proc. Natl. Acad. Sci. U. S. A. 113, E3558–E3567. doi:10.1073/pnas.1606226113
Strong, K., Mathers, C., and Bonita, R. (2007). Preventing stroke: Saving lives around the world. Lancet. Neurol. 6, 182–187. doi:10.1016/S1474-4422(07)70031-5
Sung, M. M., and Dyck, J. R. (2015). Therapeutic potential of resveratrol in heart failure. Ann. N. Y. Acad. Sci. 1348, 32–45. doi:10.1111/nyas.12839
Suzuki, H. (2015). What is early brain injury? Transl. Stroke Res. 6, 1–3. doi:10.1007/s12975-014-0380-8
Szeto, H. H. (2008). Mitochondria-targeted cytoprotective peptides for ischemia-reperfusion injury. Antioxid. Redox Signal. 10, 601–619. doi:10.1089/ars.2007.1892
Taira, T., Saito, Y., Niki, T., Iguchi-Ariga, S. M., Takahashi, K., and Ariga, H. (2004). DJ-1 has a role in antioxidative stress to prevent cell death. EMBO Rep. 5, 213–218. doi:10.1038/sj.embor.7400074
Tao, T., Liu, M., Chen, M., Luo, Y., Wang, C., Xu, T., et al. (2020). Natural medicine in neuroprotection for ischemic stroke: Challenges and prospective. Pharmacol. Ther. 216, 107695. doi:10.1016/j.pharmthera.2020.107695
Toth, P., Tarantini, S., Springo, Z., Tucsek, Z., Gautam, T., Giles, C. B., et al. (2015). Aging exacerbates hypertension-induced cerebral microhemorrhages in mice: Role of resveratrol treatment in vasoprotection. Aging Cell 14, 400–408. doi:10.1111/acel.12315
Turner, R. S., Thomas, R. G., Craft, S., van Dyck, C. H., Mintzer, J., Reynolds, B. A., et al. (2015). A randomized, double-blind, placebo-controlled trial of resveratrol for Alzheimer disease. Neurology 85, 1383–1391. doi:10.1212/WNL.0000000000002035
Turrens, J. F. (2003). Mitochondrial formation of reactive oxygen species. J. Physiol. 552, 335–344. doi:10.1113/jphysiol.2003.049478
Ungvari, Z., Sonntag, W. E., de Cabo, R., Baur, J. A., and Csiszar, A. (2011). Mitochondrial protection by resveratrol. Exerc. Sport Sci. Rev. 39, 128–132. doi:10.1097/JES.0b013e3182141f80
Valle, I., Alvarez-Barrientos, A., Arza, E., Lamas, S., and Monsalve, M. (2005). PGC-1alpha regulates the mitochondrial antioxidant defense system in vascular endothelial cells. Cardiovasc. Res. 66, 562–573. doi:10.1016/j.cardiores.2005.01.026
Van Eersel, J., Ke, Y. D., Liu, X., Delerue, F., Kril, J. J., Götz, J., et al. (2010). Sodium selenate mitigates tau pathology, neurodegeneration, and functional deficits in Alzheimer's disease models. Proc. Natl. Acad. Sci. U. S. A. 107, 13888–13893. doi:10.1073/pnas.1009038107
Vasko, M. R., Guo, C., and Kelley, M. R. (2005). The multifunctional DNA repair/redox enzyme Ape1/Ref-1 promotes survival of neurons after oxidative stress. DNA Repair (Amst) 4, 367–379. doi:10.1016/j.dnarep.2004.11.006
Virbasius, C. A., Virbasius, J. V., and Scarpulla, R. C. (1993). NRF-1, an activator involved in nuclear-mitochondrial interactions, utilizes a new DNA-binding domain conserved in a family of developmental regulators. Genes Dev. 7, 2431–2445. doi:10.1101/gad.7.12a.2431
Vitaglione, P., Sforza, S., Galaverna, G., Ghidini, C., Caporaso, N., Vescovi, P. P., et al. (2005). Bioavailability of trans-resveratrol from red wine in humans. Mol. Nutr. Food Res. 49, 495–504. doi:10.1002/mnfr.200500002
Vomund, S., Schäfer, A., Parnham, M. J., Brüne, B., and von Knethen, A. (2017). Nrf2, the master regulator of anti-oxidative responses. Int. J. Mol. Sci. 18, E2772. doi:10.3390/ijms18122772
Walle, T., Hsieh, F., DeLegge, M. H., Oatis, J. E., and Walle, U. K. (2004). High absorption but very low bioavailability of oral resveratrol in humans. Drug Metab. Dispos. 32, 1377–1382. doi:10.1124/dmd.104.000885
Wallerath, T., Deckert, G., Ternes, T., Anderson, H., Li, H., Witte, K., et al. (2002). Resveratrol, a polyphenolic phytoalexin present in red wine, enhances expression and activity of endothelial nitric oxide synthase. Circulation 106, 1652–1658. doi:10.1161/01.cir.0000029925.18593.5c
Wan, D., Zhou, Y., Wang, K., Hou, Y., Hou, R., and Ye, X. (2016). Resveratrol provides neuroprotection by inhibiting phosphodiesterases and regulating the cAMP/AMPK/SIRT1 pathway after stroke in rats. Brain Res. Bull. 121, 255–262. doi:10.1016/j.brainresbull.2016.02.011
Wang, N., He, J., Pan, C., Wang, J., Ma, M., Shi, X., et al. (2019). Resveratrol activates autophagy via the AKT/mTOR signaling pathway to improve cognitive dysfunction in rats with chronic cerebral hypoperfusion. Front. Neurosci. 13, 859. doi:10.3389/fnins.2019.00859
Wang, P., and Sang, S. (2018). Metabolism and pharmacokinetics of resveratrol and pterostilbene. Biofactors 44, 16–25. doi:10.1002/biof.1410
Wang, Q., Xu, J., Rottinghaus, G. E., Simonyi, A., Lubahn, D., Sun, G. Y., et al. (2002). Resveratrol protects against global cerebral ischemic injury in gerbils. Brain Res. 958, 439–447. doi:10.1016/s0006-8993(02)03543-6
Wang, Q., Yu, S., Simonyi, A., Rottinghaus, G., Sun, G. Y., and Sun, A. Y. (2004). Resveratrol protects against neurotoxicity induced by kainic acid. Neurochem. Res. 29, 2105–2112. doi:10.1007/s11064-004-6883-z
Wang, S., Qian, Y., Gong, D., Zhang, Y., and Fan, Y. (2011). Resveratrol attenuates acute hypoxic injury in cardiomyocytes: Correlation with inhibition of iNOS-NO signaling pathway. Eur. J. Pharm. Sci. 44, 416–421. doi:10.1016/j.ejps.2011.08.029
Wareski, P., Vaarmann, A., Choubey, V., Safiulina, D., Liiv, J., Kuum, M., et al. (2009). PGC-1{alpha} and PGC-1{beta} regulate mitochondrial density in neurons. J. Biol. Chem. 284, 21379–21385. doi:10.1074/jbc.M109.018911
Warner, J. J., Harrington, R. A., Sacco, R. L., and Elkind, M. S. V. (2019). Guidelines for the early management of patients with acute ischemic stroke: 2019 update to the 2018 guidelines for the early management of acute ischemic stroke. Stroke 50, 3331–3332. doi:10.1161/STROKEAHA.119.027708
Wei, H., Wang, S., Zhen, L., Yang, Q., Wu, Z., Lei, X., et al. (2015). Resveratrol attenuates the blood-brain barrier dysfunction by regulation of the MMP-9/TIMP-1 balance after cerebral ischemia reperfusion in rats. J. Mol. Neurosci. 55, 872–879. doi:10.1007/s12031-014-0441-1
Witte, A. V., Kerti, L., Margulies, D. S., and Flöel, A. (2014). Effects of resveratrol on memory performance, hippocampal functional connectivity, and glucose metabolism in healthy older adults. J. Neurosci. 34, 7862–7870. doi:10.1523/JNEUROSCI.0385-14.2014
Wolters, F. J., and Ma, I. (2019). Epidemiology of vascular dementia. Arterioscler. Thromb. Vasc. Biol. 39, 1542–1549. doi:10.1161/ATVBAHA.119.311908
Xiao, Z. P., Lv, T., Hou, P. P., Manaenko, A., Liu, Y., Jin, Y., et al. (2021). Sirtuin 5-mediated lysine desuccinylation protects mitochondrial metabolism following subarachnoid hemorrhage in mice. Stroke 52, 4043–4053. doi:10.1161/STROKEAHA.121.034850
Xie, Y. K., Zhou, X., Yuan, H. T., Qiu, J., Xin, D. Q., Chu, X. L., et al. (2019). Resveratrol reduces brain injury after subarachnoid hemorrhage by inhibiting oxidative stress and endoplasmic reticulum stress. Neural Regen. Res. 14, 1734–1742. doi:10.4103/1673-5374.257529
Yadav, A., Sunkaria, A., Singhal, N., and Sandhir, R. (2018). Resveratrol loaded solid lipid nanoparticles attenuate mitochondrial oxidative stress in vascular dementia by activating Nrf2/HO-1 pathway. Neurochem. Int. 112, 239–254. doi:10.1016/j.neuint.2017.08.001
Yang, H., Zhang, A., Zhang, Y., Ma, S., and Wang, C. (2016). Resveratrol pretreatment protected against cerebral ischemia/reperfusion injury in rats via expansion of T regulatory cells. J. Stroke Cerebrovasc. Dis. 25, 1914–1921. doi:10.1016/j.jstrokecerebrovasdis.2016.04.014
Yang, J., Huang, J., Shen, C., Cheng, W., Yu, P., Wang, L., et al. (2018). Resveratrol treatment in different time-attenuated neuronal apoptosis after oxygen and glucose deprivation/reoxygenation via enhancing the activation of nrf-2 signaling pathway in vitro. Cell Transpl. 27, 1789–1797. doi:10.1177/0963689718780930
Yang, T., Wang, L., Zhu, M., Zhang, L., and Yan, L. (2015). Properties and molecular mechanisms of resveratrol: A review. Trans. Tianjin Univ. 70, 501–506. doi:10.1007/s12209-015-2629-z
Yang, Y., Ding, Z., Zhong, R., Xia, T., Wang, W., Zhao, H., et al. (2020). Cardioprotective effects of a Fructus Aurantii polysaccharide in isoproterenol-induced myocardial ischemic rats. Int. J. Biol. Macromol. 155, 995–1002. doi:10.1016/j.ijbiomac.2019.11.063
Ye, M., Wu, H., and Li, S. (2021). Resveratrol alleviates oxygen/glucose deprivation/reoxygenation-induced neuronal damage through induction of mitophagy. Mol. Med. Rep. 23, 73. doi:10.3892/mmr.2020.11711
Yin, W., Signore, A. P., Iwai, M., Cao, G., Gao, Y., and Chen, J. (2008). Rapidly increased neuronal mitochondrial biogenesis after hypoxic-ischemic brain injury. Stroke 39, 3057–3063. doi:10.1161/STROKEAHA.108.520114
Yousuf, S., Atif, F., Ahmad, M., Hoda, N., Ishrat, T., Khan, B., et al. (2009). Resveratrol exerts its neuroprotective effect by modulating mitochondrial dysfunctions and associated cell death during cerebral ischemia. Brain Res. 1250, 242–253. doi:10.1016/j.brainres.2008.10.068
Yu, P., Wang, L., Tang, F., Guo, S., Liao, H., Fan, C., et al. (2021). Resveratrol-mediated neurorestoration after cerebral ischemic injury - sonic Hedgehog signaling pathway. Life Sci. 280, 119715. doi:10.1016/j.lfs.2021.119715
Yu, W., Fu, Y. C., and Wang, W. (2012). Cellular and molecular effects of resveratrol in health and disease. J. Cell. Biochem. 113, 752–759. doi:10.1002/jcb.23431
Zhang, J., Wang, Y., Liu, X., Dagda, R. K., and Zhang, Y. (2017). How AMPK and PKA interplay to regulate mitochondrial function and survival in models of ischemia and diabetes. Oxid. Med. Cell Longev. 2017, 4353510. doi:10.1155/2017/4353510
Zhang, K. (2010). Integration of ER stress, oxidative stress and the inflammatory response in health and disease. Int. J. Clin. Exp. Med. 3, 33–40.
Zhang, X., Wu, Q., Zhang, Q., Lu, Y., Liu, J., Li, W., et al. (2017). Resveratrol attenuates early brain injury after experimental subarachnoid hemorrhage via inhibition of NLRP3 inflammasome activation. Front. Neurosci. 11, 611. doi:10.3389/fnins.2017.00611
Zhang, Y., Li, Y., Wang, Y., Wang, G., Mao, L., Zhang, D., et al. (2019). Effects of resveratrol on learning and memory in rats with vascular dementia. Mol. Med. Rep. 20, 4587–4593. doi:10.3892/mmr.2019.10723
Zhao, R., Zhao, K., Su, H., Zhang, P., and Zhao, N. (2019). Resveratrol ameliorates brain injury via the TGF-β-mediated ERK signaling pathway in a rat model of cerebral hemorrhage. Exp. Ther. Med. 18, 3397–3404. doi:10.3892/etm.2019.7939
Zhou, J., Yang, Z., Shen, R., Zhong, W., Zheng, H., Chen, Z., et al. (2021). Resveratrol improves mitochondrial biogenesis function and activates PGC-1α pathway in a preclinical model of early brain injury following subarachnoid hemorrhage. Front. Mol. Biosci. 8, 620683. doi:10.3389/fmolb.2021.620683
Zhou, X. M., Zhou, M. L., Zhang, X. S., Zhuang, Z., Li, T., Shi, J. X., et al. (2014). Resveratrol prevents neuronal apoptosis in an early brain injury model. J. Surg. Res. 189, 159–165. doi:10.1016/j.jss.2014.01.062
Zini, R., C Morin, A. B., Bertelli, A. A., and Tillement, J. P. (1999). Effects of resveratrol on the rat brain respiratory chain. Drugs Exp. Clin. Res. 25, 87–97.
Zini, R., Morin, C., Bertelli, A., Bertelli, A. A., and Tillement, J. P. (2002). Resveratrol-induced limitation of dysfunction of mitochondria isolated from rat brain in an anoxia-reoxygenation model. Life Sci. 71, 3091–3108. doi:10.1016/s0024-3205(02)02161-6
Zorov, D. B., Juhaszova, M., and Sollott, S. J. (2014). Mitochondrial reactive oxygen species (ROS) and ROS-induced ROS release. Physiol. Rev. 94, 909–950. doi:10.1152/physrev.00026.2013
Glossary
Al: Aluminum
AMPK: Adenosine 5′-monophosphate (AMP)-activated protein kinase
APE1: Apurinic/apyrimidinic endonuclease one
AQP4: Aquaporin four
ARE: Antioxidant response element
ATP: Adenosine triphosphate
BBB: Blood-brain barrier disruption
BCCAO: Bilateral common carotid artery occlusion
CaMKIV: Calmodulin kinase IV
cAMP: Cyclic adenosine monophosphate
CAT: Catalase
CCH: Chronic cerebral hypoperfusion
CoA: CoenzymeA
COX2: Cyclo-oxygenase two
CREB: cAMP-response element binding protein
Cu: Copper
CVD: Cerebrovascular diseases
DCX: Doublecortin
DNA: Deoxyribonucleic acid
eNOS: Endothelial nitric oxide synthase
ER: Endoplasmic reticulum
Fe: Ferrum
GPx: Glutathione peroxidase
GFAP: Glial fibrillary acidic protein
GLUT3: Glucose transporter3
GRP78: Glucose-regulated protein 78
GSH: Glutathione
GSH-Pxs: Glutathione peroxidase
GST: Glutathione-s-transferase
H2O2: Hydrogen peroxide
HO-1: Heme oxygenase-1
I/R: Ischemic reperfusion
IL-1β: Interleukin-1β
JAK: Janus kinase
Keap1: Kelch-like ECH- associated protein l
LDH: Lactate dehydrogenase
MCAO/R: Middle cerebral artery occlusion/reperfusion
MDA: Malondialdehyde
Mg: Magnesium
MMP: Matrix metalloproteinase
MnSOD: Manganese superoxide dismutase
MPO: Myeloperoxidase
MPTP: Mitochondrial permeability transition pore
mTOR: Mechanistic target of rapamycin
mtDNA: Mitochondrial DNA
NF-κB: Nuclear factor kappa-B
NGF: Nerve growth factor
NLRP3: NOD-like receptor protein three inflammasome
NMDA: National Marine Distributors Association
NO: Nitric oxide
NQO1: NAD(P)H qui-none oxidoreductase one
NRF-1: Respiratory factors 1
Nrf2: NF-E2-related factor 2
OGD/R: Oxygen-glucose deprivation/reperfusion
PGC-1α: Peroxisome proliferator-activated receptor-gamma coactivator (PGC)-1alpha
PI3K/Akt: Phosphatidylinositol three kinase/Protein Kinase B
PINK1: PTEN-induced putative kinase protein one
PKA: Protein kinase A
PSA-NCAM: Polysialylated-neural cell adhesion molecule
PTEN: Phosphatase and tensin homolog deleted on chromosome ten
RCCA: Right common carotid artery
RES: Resveratrol
RMCA: Right Middle Cerebral Artery
ROS: Reactive oxygen species
RPC: RES preconditioning
rt-PA: Recombinant tissue plasminogen activator
SAH: Subarachnoid hemorrhage
Se: Selenium
SIRT1: Sirtuin1
SOD: Superoxide dismutase
STAT3: Signal transducer and activator of transcription3
SUR1: Sulfonylurea Receptor1
TAC: tricarboxylic acid cycle
TFAM: Transcription factor A mitochondrial
TGF-β-ERK: Transforming growth factor-β-Extracellular-signal-regulated kinases
TIMP-1: Tissue inhibitor of matrix metalloproteinases
TLR4: Toll-like receptor four
TNF-α: Tumor necrosis factor-α
TRPC6-MEK: Transient receptor potential channel 6/methyl ethyl ketone
VaD: Vascular dementia
VO: Vessel occlusion
Zn: Zinc
Keywords: resveratrol, anti-oxidant, neuroprotection, stroke, vascular dementia
Citation: Wang Q, Yu Q and Wu M (2022) Antioxidant and neuroprotective actions of resveratrol in cerebrovascular diseases. Front. Pharmacol. 13:948889. doi: 10.3389/fphar.2022.948889
Received: 20 May 2022; Accepted: 01 August 2022;
Published: 05 September 2022.
Edited by:
Li-Nan Zhang, Hebei Medical University, ChinaReviewed by:
Tom L Broderick, Midwestern University, United StatesLetizia Giampietro, University G.d'Annunzio, Italy
Félix Javier Jiménez-Jiménez, Hospital Universitario del Sureste, Spain
Wladyslaw - Lason, Maj Institute of Pharmacology, Poland
Copyright © 2022 Wang, Yu and Wu. This is an open-access article distributed under the terms of the Creative Commons Attribution License (CC BY). The use, distribution or reproduction in other forums is permitted, provided the original author(s) and the copyright owner(s) are credited and that the original publication in this journal is cited, in accordance with accepted academic practice. No use, distribution or reproduction is permitted which does not comply with these terms.
*Correspondence: Min Wu, V3VtaW4xNzVAMTI2LmNvbQ==
†These authors have contributed equally to this work