- 1Unidad de Farmacología y Terapéutica, Departamento Hospital y Clínicas Veterinarias, Facultad de Veterinaria, Universidad de la República, Montevideo, Uruguay
- 2Unidad de Bioestadística, Departamento de Salud Pública Veterinaria, Facultad de Veterinaria, Universidad de la República, Montevideo, Uruguay
- 3Worm Biology Laboratory, Institut Pasteur de Montevideo, Montevideo, Uruguay
- 4Departamento de Biociencias, Facultad de Química, Universidad de la República, Montevideo, Uruguay
Caenorhabditis elegans is a free-living nematode that has been validated for anthelmintic drug screening. However, this model has not been used to address anthelmintic dose-response-time and drug-drug interactions through matrix array methodology. Eprinomectin (EPM) and Ivermectin (IVM) are macrocyclic lactones widely used as anthelmintics. Despite being very similar, EPM and IVM are combined in commercial formulations or mixed by farmers, under the assumption that the combination would increase their efficacy. However, there is no data reported on the pharmacological evaluation of the combination of both drugs. In this study, we assessed the pharmacodynamics and drug-drug interactions of these two anthelmintic drugs. Since the action of these drugs causes worm paralysis, we used an infrared motility assay to measure EPM and IVM effects on worm movement over time. The results showed that EPM was slightly more potent than IVM, that drug potency increased with drug time exposure, and that once paralyzed, worms did not recover. Different EPM/IVM concentration ratios were used and synergy and combination sensitivity scores were determined at different exposure times, applying Highest Single Agent (HSA), Loewe additivity, Bliss and Zero Interaction Potency (ZIP) models. The results clearly indicate that there is neither synergy nor antagonism between both macrocyclic lactones. This study shows that it is more relevant to prioritize the exposure time of each individual drug than to combine them to improve their effects. The results highlight the utility of C. elegans to address pharmacodynamics studies, particularly for drug-drug interactions. Models in vitro can be integrated to facilitate preclinical and clinical translational studies and help researchers to understand drug-drug interactions and achieve rational therapeutic regimes.
Introduction
Drug-drug interactions may alter Pharmacokinetic-Pharmacodynamic (PK/PD) relationship. When combining two or more drugs, the main objective is to achieve positive interaction effects, that is the beneficial effect of the combined drugs compared to each one individually (Tang et al., 2015; Duarte and Vale, 2022). The concepts of synergy and antagonism have accepted definitions, they represent, respectively, greater or lesser effects for the combined drugs than the simple additive effect expected from each drug individually (Foucquier and Guedj, 2015). The altered effect is reflected by the changes in concentrations and/or exposure time in the biophase of the drugs.
Parasitic worms (helminths) constitute a prevalent sanitary and economic problem, particularly for developing countries (Molento et al., 2011). Helminth infections are the causative agents of WHO-categorized neglected diseases, and infect livestock and crops. Helminths are responsible for huge economic losses in animal breeding. Massive pharmacological treatment of livestock against helminthiasis has led producers to face the accelerated spread of anthelmintic resistance to all known classes of anthelmintics (Sangster et al., 2018). In order to delay resistance and treat more effectively helminth infections, the administration of combinations of anthelmintics with a similar spectrum of activity is used as a treatment strategy (Geary et al., 2012; Suarez et al., 2014; Lanusse et al., 2015), most frequently without previous assessment of the effect of synergism in the combination.
Macrocyclic lactones (MLs) are widely used in the treatment of humans, livestock and domestic animals infected with helminths. Eprinomectin (EPM) and ivermectin (IVM) are MLs with marked antiparasitic activity (Yilmaz et al., 2019). Both compounds are chemically related belonging to the avermectin subfamily. They target the gamma-aminobutyric acid (GABA) receptors and the glutamate-gated chloride ion channels (GluCl) and interfere with neurotransmission (Abongwa et al., 2017) (revised by Choudhary et al., 2022). This disturbance induces neuronal membrane hyperpolarization, paralysis, and ultimately the death of the parasite (Geary et al., 1993; Yates et al., 2003). Despite being very similar, they are used combined in commercial formulations or mixed by producers, under the assumption that their combined use would increase their efficacy and/or delay the spread of drug resistance. However, there is no data reported on the pharmacological evaluation of the EPM/IVM combination.
The lack of evidence for anthelmintic drug-drug interactions arises from the fact that clinical trials and field studies require a large number of animals, are costly, time-consuming, and difficult to replicate. In vitro studies with live parasites require either natural infections, which may contain mixed species populations, or artificial infections, which are costly and require ex vivo maintenance of parasites. Due to these limitations a complete drug-drug concentration matrix is not assessed in those studies, unlike the evaluation strategy that we propose in this study using the Caenorhabditis elegans model.
Many of the disadvantages mentioned above can be overcome by using the free-living nematode C. elegans. Among other advantages, C. elegans is easy and inexpensive to grow and maintain, it has a short life cycle with numerous progeny and it is amenable to genetics studies (Nigon and Félix, 2017). Despite not being a parasite, C. elegans is extremely useful for nematode parasitologists (Salinas and Risi, 2018; Hahnel et al., 2020). It belongs to nematode clade V, and therefore is a close relative to the major gastrointestinal parasitic nematodes of humans and livestock (e.g., Haemonchus contortus and Cooperia spp) (Blaxter et al., 1998). Indeed, C. elegans has been used in nematicide discovery projects (Burns et al., 2015; Partridge et al., 2018; Risi et al., 2019; Taki et al., 2021). Moreover, the mechanism of action and resistance to several anthelmintics has been elucidated using C. elegans (reviewed in Holden-Dye and Walker, 2014).
Being a key model for anthelmintic research, very few studies have used C. elegans to address drug-drug interaction between nematicides. Ding et al. (2017) reported that an optimal combination of four anthelmintics, which is more potent than any of them at lower concentrations than their EC50 values. Hu et al. (2010) showed that when Cry5B and nAChR agonists are combined, their activities are strongly synergistic. Despite these examples, there are no previous studies that have used the entire drug-drug concentration matrix to assess anthelmintic synergism.
In this study, we use a C. elegans high throughput infrared motility assay to address EPM/IVM interaction in matrix combinations (Simonetta and Golombek, 2007; Mathew et al., 2016). Our results indicates that there is neither synergism nor antagonism for EPM and IVM at any concentration ratio at any time, whichever the model used to analyze the data. Importantly, this study highlights several advantages of this model for pharmacodynamics and drug-drug interaction: simplicity, unlimited availability of nematodes, and the possibility to follow kinetics in a high throughput assay leading to high reproducibility.
Materials and methods
Chemicals
Technical grade eprinomectin (EPM) and ivermectin (IVM) were obtained from Compañía Cibeles S.A. (Montevideo, Uruguay). EPM and IVM were prepared at a 10 mM stock concentration in DMSO (41,640, Sigma-Aldrich) and stored at −20°C.
C. elegans strains and culture methods
The C. elegans wild-type strain Bristol N2 (N2) and Escherichia coli OP50 strain were obtained from the Caenorhabditis Genomics Center (CGC, Minneapolis, MN, United States). Worms were grown on Nematode Growth Media (NGM) agar plates seeded with E. coli OP50 as a source of food, and maintained under standard conditions at 20°C. The N2 strain was cultured and maintained according to previously described procedures (Brenner, 1974; Stiernagle, 2006). Worm populations were synchronized at the stage of L1 and used in all assays at L4.
Drug combination assay
L4 C. elegans worms were removed from culture plates and washed three times with K saline (NaCl 51 mM, KCl 32 mM) by centrifugation at 1,000 g. Worms were plated in 96-well microtiter plates (Costar 3,590). Approximately 80 worms per well were seeded in 60 µl of K saline containing 0.015% BSA. Compound dilution series in DMSO (0.5%) were added into the wells in a 6 × 6 matrix array. The matrix array included a control group (DMSO 0.5% without compounds), each drug alone at 5 concentrations (0.1; 0.2; 0.4; 0.8; 1.6 µM in 0.5% DMSO) and the 25 concentration combinations of both compounds. For each experiment replicas were performed.
Motility was measured using the infrared tracking device WMicrotrackerTM ONE (PhylumTech, Santa Fe, Argentina). The method used to assess motility is described in detail in (Simonetta and Golombek, 2007). Briefly, the system detects motility through the interference to an array of infrared light microbeams, caused by worm movement. The readout motility is count events (interruption of the beam by worm movement) per unit of time (5 min).
The motility using WMicrotrackerTM ONE (Santa Fe, Argentina) was measured for 980 min (Figure 1A). We considered 240 min the optimal time to evaluate the drug effect, since the basal movement in the control group was constant during this time, but decreased markedly after 5 h (Figure 1B).
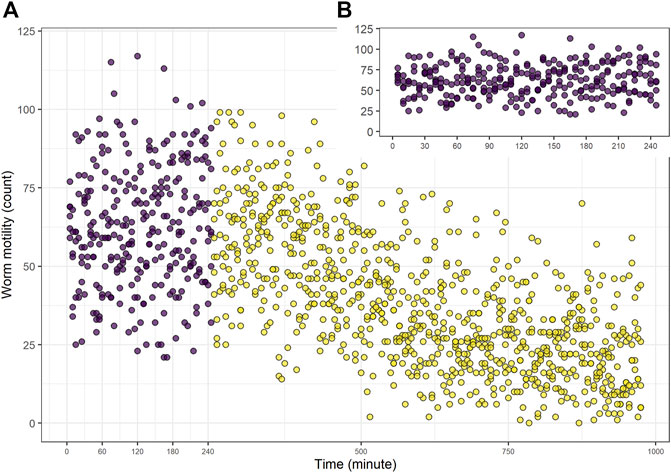
FIGURE 1. Control group worm motility. Control group (vehicle alone, DMSO 0.5%). Worm motility counts per unit of time (5 min) was measured for 980 min (A) Complete assay. Purple dots correspond to the data considered in subsequent dose-response assays (the first 240 min). (B) Inset time from 0 to 240 min only. The data shows a representative experiment.
Dose-response analysis
Worm motility of each well at 30, 60, 90, 120, 150, 180, 210, and 240 min was normalized to the median of the control wells to calculate the motility inhibition values (%). For synergy analysis, motility inhibition from replica wells was averaged and analyzed using the web-based tool SynergyFinder (Zheng et al., 2022).
The data were analyzed using a four-parameter log-logistic curve (sigmoidal shape) with variable slope and half-maximal inhibitory concentration (IC50) (Murado et al., 2002). The dose-response of a single drug and drug-drug interaction were visualized by pair heatmap and 3D surface motility inhibition [±95% confidence intervals (CI)].
Additionally, relative inhibition (RI) was determined considering the ratio of the area under the dose-response curve (adjusted by a four-parameter logistic model), with respect to the maximum area that a drug can reach in the same dose range.
Drug synergy analysis
Data were analyzed using SynergyFinder Plus software (Zheng et al., 2022) considering four reference drug-drug interaction models: Highest Single Agent (HSA), Bliss Independence (Bliss), Loewe model (Loewe) and Zero Interaction Potency (ZIP). The models make different assumptions regarding the expected effect. In summary, the degree of synergy is quantified as the excess over the maximum single drug response (HSA); the multiplicative effect of single drugs as if they act independently (Bliss), the expected response corresponding to an additive effect as if the single drugs were the same compound (Loewe), or the expected response corresponding to the effect as if the single drugs did not affect the potency of each other (ZIP model) (Tang et al., 2015; Lanevski et al., 2020; Zheng et al., 2022). The drug-drug interaction models were visualized by pair heatmap and 3D surface of Synergy Score (±95% confidence intervals (CI)) and the average synergy score value in the whole combination matrix was determined. Synergy Score (SS) > 5 was categorized as strong synergy, whilst <5 was categorized as strong antagonism, according to (Yadav et al., 2015).
To determine the sensitivity of the drug pair, we used the combination sensitivity score (CSS) model that calculates the relative inhibition of a drug combination based on the area under the log10 scaled dose-response curves at the IC50 doses of the constituent drugs (Malyutina et al., 2019).
To visualize the maximal synergy and sensitivity scores we performed two-dimensional plots of these scores for all the analyzed models, as suggested by heng et al. (Malyutina et al., 2019; Zheng et al., 2022).
Statistical analysis
Statistical analyses were performed with RStudio (Version 2022.2.3.492) (RStudio Team, 2022). The dose-response and synergy analysis were calculated using the package SynergyFinder of the R software fitting a dose-response sigmoid curve (four-parameter logistic). CSS of a drug combination was calculated such that each of the compounds (background drug) at a fixed concentration (its relative IC50) and the other (foreground drug) at varying concentrations resulting in two CSS values, which are then averaged (Malyutina et al., 2019; Zheng et al., 2022). Graphs were plotted using ggplot2 (Wickham, 2016)in R (Version 4.2.1) (R Core Team, 2022). All compound combination ratio drugs were randomized using random codes on plates to prevent experimental bias.
Results
Worm motility for single-drug treatment
Locomotive activity for worms treated with EPM, IVM (range of 0.1–1.6 µM) and control group (without drugs) are shown in Figure 2. The activity distribution trends were similar for EPM and IVM, both drugs clearly differ from the control group (Figure 2). Inhibition of worm motility was observed at all concentrations examined. At the highest concentration (1.6 µM) the maximal effect (100% inhibition) was observed in the first 30 min. Below 1 µM inhibition with EPN was faster than with IVM. Sporadic movement was observed. We verified that worms in wells in which no motility was registered at the end of the experiment were dead (did not exhibit movement or touch response on plates).
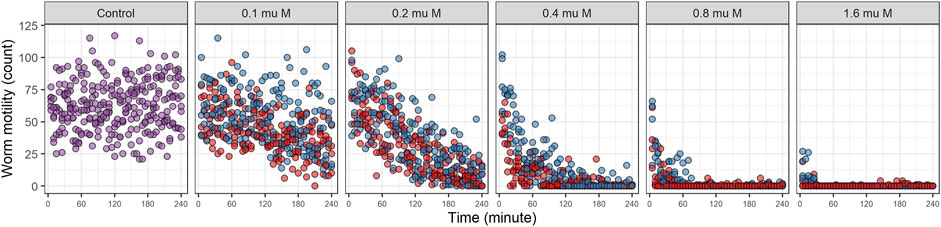
FIGURE 2. Locomotive activity for drugs alone. Worm motility over time was registered for 240 min. Different drug dose was used (range of 0.1–1.6 µM). Eprinomectin is depicted in red, Ivermectin in blue and Control in purple.
Dose-response curve
Figure 3 shows the variation in IC50 (Panel A) and RI (Panel B) to single drugs in the dose-response model from 30 to 240 min. For both drugs potency (IC50) and RI increased as a function of exposure time reaching similar maximum values after 120–150 min. Nevertheless, in the first 90 min of the assay, EPM showed higher potency (lower IC50 values) than IVM (Panel A, left graph). The IC50 EPM/IVM ratios at 30, 60, and 90 min were 0.69, 0.74, and 0.59, respectively (Panel A, right graph). Similarly, EPM showed higher RI values than IVM at these times (Panel B, left graph). The EPM/IVM ratios 1.27, 1.30, and 1.34 at 30, 60, and 90 min, respectively (Panel B, right graph).
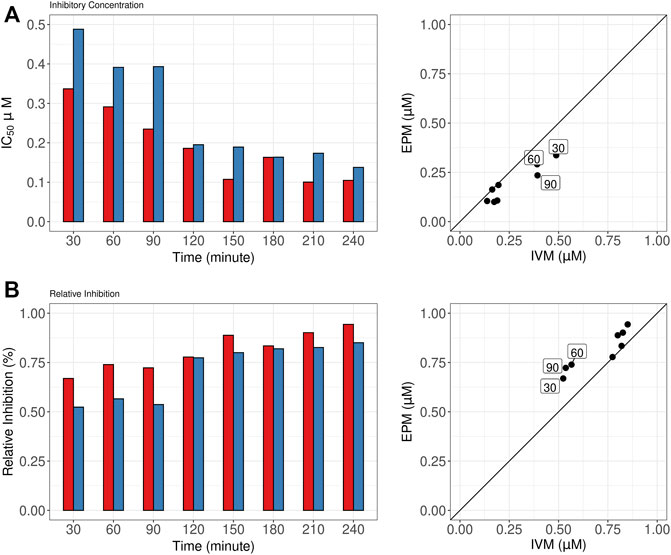
FIGURE 3. Half-maximal inhibitory concentration (IC50) and relative inhibition (RI) for single drugs. (A). Eprinomectin (EPM) and Ivermectin (IVM) IC50 (left) and IC50 EPM/IVM ratios (right). 30–240 min exposure times are shown (B). EPM and IVM RI (left) and EPM/IVM RI ratios. 30–240 min exposure time are shown. The squares highlight the higher potency of EPM than IVM at 30, 60, and 90 min.
Synergy effect and combination sensitivity score for EPM and IVM
3D surface of Synergy Scores (SS) for EPM and IVM drug-drug interaction for the four models (HSA, Bliss, Loewe and ZIP) were derived from the data. The different models used to study drug-drug interactions make different assumptions and define synergy and antagonism differently (BLISS, 1939; Loewe, 1953; Berenbaum, 1989; Yadav et al., 2015). In any case, none of the models suggest synergy or antagonism between both drugs. The 3D surface of SS at minute 90 is shown in Figure 4, but the same pattern was observed at all timepoints (Supplementary Figures S1–S8). In this representation, synergy has a positive value and is depicted in red and green has a negative value and is depicted in green. Values above 5 or below −5 are considered the threshold to define a synergic or antagonist effect, respectively (Malyutina et al., 2019).
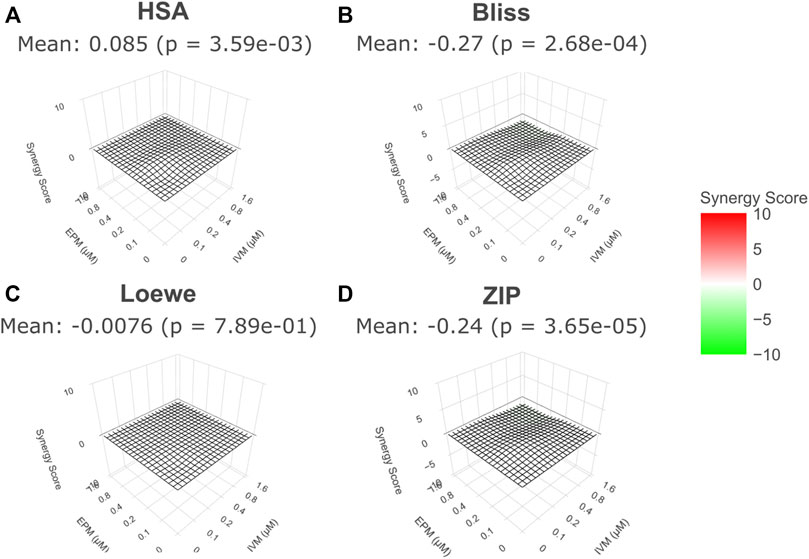
FIGURE 4. 3D surface of Synergy Scores for EPM and IVM drug-drug interaction. For all models, EPM and IVM interaction landscapes are shown in 3D (A). Highest Single Agent model (HAS) (B). Bliss Independence model (BLISS) (C). Loewe model (LOEWE) (D). Zero Interaction Potency model (ZIP). 90 min exposure time is shown in all cases. The mean value of Synergy Score is indicated in the SD surface (p value compared to 0% inhibition). No significant synergy (red) or antagonism (green) between EPM and IVM at all concentrations.
Pair heatmaps of the dose-response matrix for EPM and IVM at 30 and 90 min are shown in Figure 5. The same pattern was observed at all times (Supplementary Figures S1–S8). These times were selected since they showed minimal and maximal Combination Sensitivity Score (CSS). The heatmaps revealed that there was an additive effect, but neither significant synergism nor antagonism was observed.
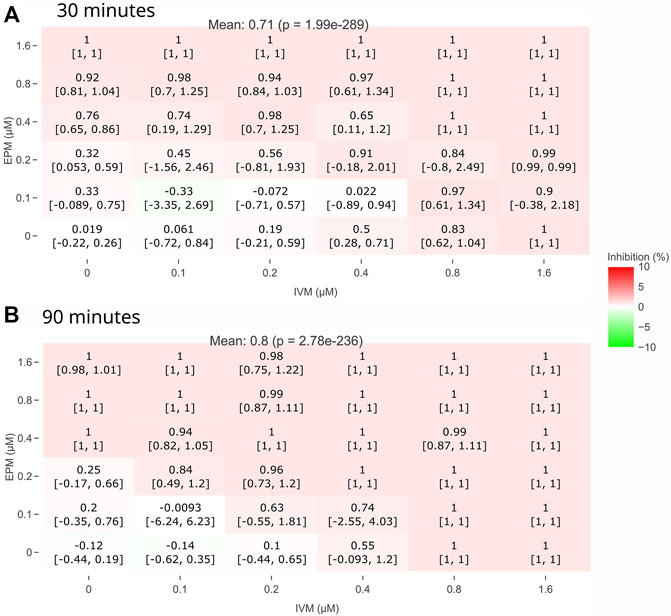
FIGURE 5. Heatmaps of the dose-response matrix for EPM and IVM drug-drug interaction. Exposure time at 30 min (A) and 90 min (B). Mean value of the dose-response matrix is indicated at the top (p value compared to 0% inhibition). No significant change in inhibition (red or green) between EPM and IVM at all concentrations.
SS and the CSS for the entire combination matrix EPM/IVM are shown in Figure 6. The SS values were lower than ±0.5 for all experimental times, revealing the absence of synergism or antagonism, since the threshold for drug-drug interaction is ±5% (Malyutina et al., 2019). Indeed, all mean SS values were close to zero in the four reference models (HSA, Loewe, Bliss and ZIP), indicating additive interactions in all the concentration range. The CSS in motility reduction at different times varied marginally (from 0.02 to 0.1). The lowest and highest CSS were observed at exposure times of 30 and 90 min, respectively.
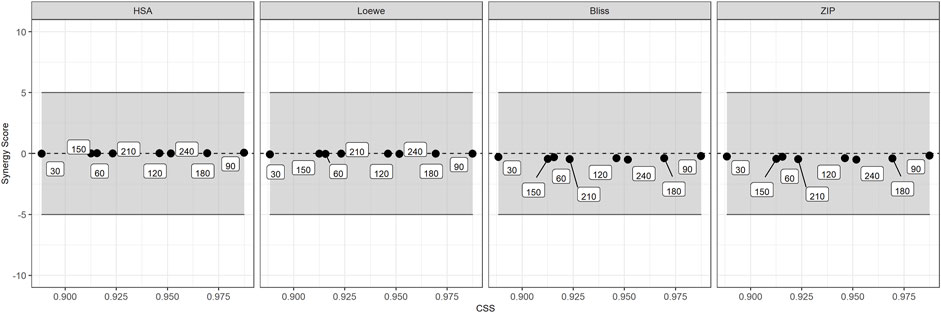
FIGURE 6. Synergy Scores (SS) and the overall Combination Sensitivity Score (CSS) for the combination EPM/IVM matrix. Each panel represents a different reference model of Synergy Scoring models with different assumptions regarding the expected effect (HSA = Highest Single Agent; BLISS = Bliss Independence; LOEWE = Loewe model and ZIP = Zero Interaction Potency). The gray zone indicates additive effect (Malyutina et al., 2019). Squares indicate the different times.
Discussion
In this study we used the free-living nematode C. elegans, the simplest animal model, to study drug-drug interaction of widely used anthelmintics against parasitic nematodes. C. elegans is a firmly established model, but it has not been used before for thorough drug-drug interactions. Our results showed that this animal model is very useful since it can easily provide a large amount of data, which would be very difficult to obtain with nematode parasites in vitro, or with animal infections in vivo.
The results showed that there is a clear dose effect for both drugs. A time-dependent response was observed for both drugs (i.e., the longer the time the greater the effect, as evidenced by the lower IC50 with increased time). Furthermore, at the concentration range used, the effect was not reversible: once the drug paralyzed the worm, motility did not recover (the sporadic motility measured can be considered as a minor intrinsic variability, as observed for the control group). This is consistent with original reports for the IVM mode of action, which described its effect as persistent paralysis on nematode pharyngeal (Brownlee et al., 1997; Pemberton et al., 2001) and body wall musculature (Kass et al., 1980, 1982).
The exposure time of parasites to the active drug concentration is a critical factor that affects the efficacy and/or persistence of activity of most anthelmintics used in ruminants and determines the anthelmintic activity (Lanusse et al., 2018). The C. elegans motility assay is ideally suited to study time exposure to nematicides in vitro. Our results clearly reinforce the concept that nematicide activity increases with time at a constant concentration. The comparison of the time-dependent IC50 for EPM and IVM showed that EPM is slightly more potent than IVM, particularly before the first 2 h. This was confirmed by the relative inhibition, which was also greater for EPM than for IVM. These results contribute to visualize the importance of adequate anthelmintic dosage and exposure time, which may be relevant factors in delaying anthelmintic resistance.
C. elegans was also found valuable to reliably study drug-drug interactions in vitro. Taken together, the CSS and SS data show that EPM and IVM act through an additive mode. The lack of synergism supports previous assumptions that both macrocyclic lactones are supposed to act on the same target on nematodes (Martin et al., 2021; Choudhary et al., 2022). The structure of EPM and IVM would suggest that they act through the same mechanism (Lespine et al., 2012; David et al., 2016).
In view that the combined action of EPM and IVM is not superior to their individual actions, our results discouraged the combined use of these drugs, which are sometimes used not only by farmers but also in commercial formulations. Furthermore, our results suggest that EPM would be a better active principle than IVM for nematodes. EPM has a better dose-response time and efficacy than IVM (this study), less prolonged residual concentration in milk (Imperiale et al., 2021), and both drugs have similar toxicity to animals (Salman et al., 2022).
A note of caution is needed. There are limitations to this kind of study. C. elegans is a free-living nematode, and therefore valuable results should be further addressed using parasitic nematodes. Secondly, it is a reductionist approach that does not consider what happens within the host, in which there are other relevant interactions. Indeed, whether the results can be extended to infer what happens within the host is not simple. PKPD studies for each drug and for a selected combination complemented by efficacy studies would help to validate the model. Another approach that could contribute to reliable inferences is to determine the concentrations of drugs inside and outside the worm in vitro and in vivo conditions, in the worm environment. However, this reductionist approach has the enormous advantage to evaluate numerous different combinations to select a promising specific combination.
Drug combinations are widely used in parasitology to delay the development of drug resistance. However, in contrast to the field of antibiotics or antitumor drugs, there is a lack of models to assess synergy or antagonism in helminths. Our results show that C. elegans is a valuable nematode model for addressing antihelmintic drug-drug interactions in vitro, which is important before using drug combinations without field studies or proper assessment. Due to their simplicity, reproducibility and low cost, it could be a reference model to rationalize the use of different active principles, particularly against nematodes.
Data availability statement
The original contributions presented in the study are included in the article/Supplementary Materials, further inquiries can be directed to the corresponding authors.
Author contributions
GSu and GSa conceived the project. GSu conceived the pharmacological model. GSu and GSa performed the experiments. GSu and IA created the statistical model. GSu, IA, and GSa analyzed results. GSu and GSa wrote the manuscript. All authors read, edited, and approved the final manuscript.
Funding
This work was supported by: Programa para el Desarrollo de las Ciencias Básicas (PEDECIBA) and Universidad de la República, Uruguay; FOCEM - Fondo para la Covergencia Estructural del Mercosur (COF 03/11).
Acknowledgments
We wish to thank the Caenorhabditis Genetics Centre (CGC, University of Minnesota, United States) for provision of C. elegans Bristol wild-type strain (N2) and the bacterial strain Escherichia coli OP50. We thank Romanelli for assistance with C. elegans.
Conflict of interest
The authors declare that the research was conducted in the absence of any commercial or financial relationships that could be construed as a potential conflict of interest.
Publisher’s note
All claims expressed in this article are solely those of the authors and do not necessarily represent those of their affiliated organizations, or those of the publisher, the editors and the reviewers. Any product that may be evaluated in this article, or claim that may be made by its manufacturer, is not guaranteed or endorsed by the publisher.
Supplementary material
The Supplementary Material for this article can be found online at: https://www.frontiersin.org/articles/10.3389/fphar.2022.984905/full#supplementary-material
References
Abongwa, M., Martin, R. J., and Robertson, A. P. (2017). A brief review on the mode of action of antinematodal drugs. Acta Vet. 67, 137–152. doi:10.1515/ACVE-2017-0013
Blaxter, M. L., de Ley, P., Garey, J. R., Llu, L. X., Scheldeman, P., Vierstraete, A., et al. (1998). A molecular evolutionary framework for the phylum Nematoda. Nature 392, 71–75. doi:10.1038/32160
Bliss, C. I. (1939). The toxicity of poisons applied jointly. Ann. Appl. Biol. 26, 585–615. doi:10.1111/J.1744-7348.1939.TB06990.X
Brenner, S. (1974). The genetics of Caenorhabditis elegans. Genetics 77, 71–94. doi:10.1093/GENETICS/77.1.71
Brownlee, D. J. A., Holden-Dye, L., and Walker, R. J. (1997). Actions of the anthelmintic ivermectin on the pharyngeal muscle of the parasitic nematode, Ascaris suum. Parasitology 115, 553–561. doi:10.1017/S0031182097001601
Burns, A. R., Luciani, G. M., Musso, G., Bagg, R., Yeo, M., Zhang, Y., et al. (2015). Caenorhabditis elegans is a useful model for anthelmintic discovery. Nat. Commun. 6, 7485. doi:10.1038/NCOMMS8485
Choudhary, S., Kashyap, S. S., Martin, R. J., and Robertson, A. P. (2022). Advances in our understanding of nematode ion channels as potential anthelmintic targets. Int. J. Parasitol. Drugs Drug Resist. 18, 52–86. doi:10.1016/J.IJPDDR.2021.12.001
David, M. A. M. A., Orlowski, S., Prichard, R. K. R. K., Hashem, S., André, F., and Lespine, A. (2016). In silico analysis of the binding of anthelmintics to Caenorhabditis elegans P-glycoprotein 1. Int. J. Parasitol. Drugs Drug Resist. 6, 299–313. doi:10.1016/J.IJPDDR.2016.09.001
Ding, X., Njus, Z., Kong, T., Su, W., Ho, C. M., and Pandey, S. (2017). Effective drug combination for Caenorhabditis elegans nematodes discovered by output-driven feedback system control technique. Sci. Adv. 3, eaao1254–10. doi:10.1126/sciadv.aao1254
Duarte, D., and Vale, N. (2022). Evaluation of synergism in drug combinations and reference models for future orientations in oncology. Curr. Res. Pharmacol. Drug Discov. 3, 100110. doi:10.1016/J.CRPHAR.2022.100110
Foucquier, J., and Guedj, M. (2015). Analysis of drug combinations: Current methodological landscape. Pharmacol. Res. Perspect. 3, e00149. doi:10.1002/prp2.149
Geary, T. G., Hosking, B. C., Skuce, P. J., von Samson-Himmelstjerna, G., Maeder, S., Holdsworth, P., et al. (2012). World Association for the Advancement of Veterinary Parasitology (W.A.A.V.P.) Guideline: Anthelmintic combination products targeting nematode infections of ruminants and horses. Veterinary Parasitol. 190, 306–316. doi:10.1016/j.vetpar.2012.09.004
Geary, T. G., Sims, S. M., Thomas, E. M., Vanover, L., Davis, J. P., Winterrowd, C. A., et al. (1993). Haemonchus contortus: Ivermectin-induced paralysis of the pharynx. Exp. Parasitol. 77, 88–96. doi:10.1006/EXPR.1993.1064
Hahnel, S. R., Dilks, C. M., Heisler, I., Andersen, E. C., and Kulke, D. (2020). Caenorhabditis elegans in anthelmintic research – old model, new perspectives. Int. J. Parasitol. Drugs Drug Resist. 14, 237–248. doi:10.1016/J.IJPDDR.2020.09.005
Holden-Dye, L., and Walker, R. J. (2014). Anthelmintic drugs and nematicides: Studies in Caenorhabditis elegans. WormBook, 1–29. doi:10.1895/WORMBOOK.1.143.2
Hu, Y., Platzer, E. G., Bellier, A., and Aroian, R. v. (2010). Discovery of a highly synergistic anthelmintic combination that shows mutual hypersusceptibility. Proc. Natl. Acad. Sci. U. S. A. 107, 5955–5960. doi:10.1073/PNAS.0912327107
Imperiale, F., Lanusse, C., Sahagun, A. M., and Diez, R. (2021). The pattern of blood-milk exchange for antiparasitic drugs in dairy ruminants. Anim. (Basel) 11, 2758. doi:10.3390/ani11102758
Kass, I. S., Larsen, D. A., Wang, C. C., and Stretton, A. O. W. (1982). Ascaris suum: Differential effects of Avermectin B1a on the intact animal and neuromuscular strip preparations. Exp. Parasitol. 54, 166–174. doi:10.1016/0014-4894(82)90123-0
Kass, I. S., Wang, C. C., Walrond, J. P., and Stretton, A. O. W. (1980). Avermectin B(1a), a paralyzing anthelmintic that affects interneurons and inhibitory motoneurons in Ascaris. Proc. Natl. Acad. Sci. U. S. A. 77, 6211–6215. doi:10.1073/PNAS.77.10.6211
Lanevski, A., Giri, A. K., and Aittokallio, T. (2020). SynergyFinder 2.0: Visual analytics of multi-drug combination synergies. Nucleic Acids Res. 48, W488–W493. doi:10.1093/NAR/GKAA216
Lanusse, C., Canton, C., Virkel, G., Alvarez, L., Costa-Junior, L., and Lifschitz, A. (2018). Strategies to optimize the efficacy of anthelmintic drugs in ruminants. Trends Parasitol. 34, 664–682. doi:10.1016/j.pt.2018.05.005
Lanusse, C., Lifschitz, A., and Alvarez, L. (2015). Basic and clinical pharmacology contribution to extend anthelmintic molecules lifespan. Vet. Parasitol. 212, 35–46. doi:10.1016/j.vetpar.2015.07.015
Lespine, A., Ménez, C., Bourguinat, C., and Prichard, R. K. (2012). P-glycoproteins and other multidrug resistance transporters in the pharmacology of anthelmintics: Prospects for reversing transport-dependent anthelmintic resistance. Int. J. Parasitol. Drugs Drug Resist. 2, 58–75. doi:10.1016/j.ijpddr.2011.10.001
Loewe, S. (1953). The problem of synergism and antagonism of combined drugs. Arzneimittelforschung. 3, 285–290.
Malyutina, A., Majumder, M. M. M., Wang, W., Pessia, A., Heckman, C. A., Tang, J., et al. (2019). Drug combination sensitivity scoring facilitates the discovery of synergistic and efficacious drug combinations in cancer. PLoS Comput. Biol. 15 (5), e1006752. doi:10.1371/journal.pcbi.1006752
Martin, R. J., Robertson, A. P., and Choudhary, S. (2021). Ivermectin: An anthelmintic, an insecticide, and much more. Trends Parasitol. 37, 48–64. doi:10.1016/J.PT.2020.10.005
Mathew, M. D., Mathew, N. D., Miller, A., Simpson, M., Au, V., Garland, S., et al. (2016). Using C. elegans forward and reverse genetics to identify new compounds with anthelmintic activity. PLoS Negl. Trop. Dis. 10, e0005058. doi:10.1371/JOURNAL.PNTD.0005058
Molento, M. B., Fortes, F. S., Pondelek, D. A. S., Borges, F. de A., Chagas, A. C. de S., Torres-Acosta, J. F. de J., et al. (2011). Challenges of nematode control in ruminants: Focus on Latin America. Vet. Parasitol. 180, 126–132. doi:10.1016/j.vetpar.2011.05.033
Murado, M. A., González, M. P., and Vázquez, J. A. (2002). Dose–response relationships: An overview, a generative model and its application to the verification of descriptive models. Enzyme Microb. Technol. 31, 439–455. doi:10.1016/S0141-0229(02)00109-6
Nigon, V. M., and Félix, M. A. (2017). History of research on C. elegans and other free-living nematodes as model organisms. WormBook 2017, 1–84. doi:10.1895/WORMBOOK.1.181.1
Partridge, F. A., Brown, A. E., Buckingham, S. D., Willis, N. J., Wynne, G. M., Forman, R., et al. (2018). An automated high-throughput system for phenotypic screening of chemical libraries on C. elegans and parasitic nematodes. Int. J. Parasitol. Drugs Drug Resist. 8, 8–21. doi:10.1016/J.IJPDDR.2017.11.004
Pemberton, D. J., Franks, C. J., Walker, R. J., and Holden-Dye, L. (2001). Characterization of glutamate-gated chloride channels in the pharynx of wild-type and mutant Caenorhabditis elegans delineates the role of the subunit GluCl-alpha2 in the function of the native receptor. Mol. Pharmacol. 59, 1037–1043. doi:10.1124/MOL.59.5.1037
R Core Team (2022). R: A language and environment for statistical computing. Available at: https://www.R-project.org/(Accessed June 29, 2022).
Risi, G., Aguilera, E., Ladós, E., Suárez, G., Carrera, I., Álvarez, G., et al. (2019). Caenorhabditis elegans infrared-based motility assay identified new hits for nematicide drug development. Vet. Sci. 6, 29. doi:10.3390/vetsci6010029
RStudio Team (2022). RStudio: Integrated development environment for R. Available at: http://www.rstudio.com/(Accessed July 1, 2022).
Salinas, G., and Risi, G. (2018). Caenorhabditis elegans: Nature and nurture gift to nematode parasitologists. Parasitology 145, 979–987. doi:10.1017/S0031182017002165
Salman, M., Abbas, R. Z. R. Z., Mehmood, K., Hussain, R., Shah, S., Faheem, M., et al. (2022). Assessment of avermectins-induced toxicity in animals. Pharmaceuticals 15, 332. doi:10.3390/ph15030332
Sangster, N. C., Cowling, A., and Woodgate, R. G. (2018). Ten events that defined anthelmintic resistance research. Trends Parasitol. 34, 553–563. doi:10.1016/J.PT.2018.05.001
Simonetta, S. H., and Golombek, D. A. (2007). An automated tracking system for Caenorhabditis elegans locomotor behavior and circadian studies application. J. Neurosci. Methods 161, 273–280. doi:10.1016/J.JNEUMETH.2006.11.015
Suarez, G., Alvarez, L., Castells, D., Moreno, L., Fagiolino, P., and Lanusse, C. (2014). Evaluation of pharmacological interactions after administration of a levamisole, albendazole and ivermectin triple combination in lambs. Vet. Parasitol. 201, 110–119. doi:10.1016/j.vetpar.2013.12.015
Taki, A. C., Byrne, J. J., Boag, P. R., Jabbar, A., and Gasser, R. B. (2021). Practical high-throughput method to screen compounds for anthelmintic activity against Caenorhabditis elegans. Molecules 26, 4156. doi:10.3390/MOLECULES26144156
Tang, J., Wennerberg, K., and Aittokallio, T. (2015). What is synergy? The saariselkä agreement revisited. Front. Pharmacol. 6, 181. doi:10.3389/fphar.2015.00181
Wickham, H. (2016). ggplot2: Elegant graphics for data analysis. New York: Springer-Verlang. doi:10.1007/978-3-319-24277-4
Yadav, B., Wennerberg, K., Aittokallio, T., and Tang, J. (2015). Searching for drug synergy in complex dose-response landscapes using an interaction potency model. Comput. Struct. Biotechnol. J. 13, 504–513. doi:10.1016/J.CSBJ.2015.09.001
Yates, D. M., Portillo, V., and Wolstenholme, A. J. (2003). The avermectin receptors of Haemonchus contortus and Caenorhabditis elegans. Int. J. Parasitol. 33, 1183–1193. doi:10.1016/S0020-7519(03)00172-3
Yilmaz, E., Gerst, B., McKay-Demeler, J., and Krücken, J. (2019). Minimal modulation of macrocyclic lactone susceptibility in Caenorhabditis elegans following inhibition of cytochrome P450 monooxygenase activity. Exp. Parasitol. 200, 61–66. doi:10.1016/J.EXPPARA.2019.03.017
Keywords: drug combination, antiparasitic resistance, EC50, pharmacology, synergism, nematode, helminth
Citation: Suárez G, Alcántara I and Salinas G (2022) Caenorhabditis elegans as a valuable model for the study of anthelmintic pharmacodynamics and drug-drug interactions: The case of ivermectin and eprinomectin. Front. Pharmacol. 13:984905. doi: 10.3389/fphar.2022.984905
Received: 02 July 2022; Accepted: 03 October 2022;
Published: 19 October 2022.
Edited by:
Firzan Nainu, Hasanuddin University, IndonesiaReviewed by:
Talha Bin Emran, Begum Gulchemonara Trust University, BangladeshAbu Montakim Tareq, University of Houston, United States
Copyright © 2022 Suárez, Alcántara and Salinas. This is an open-access article distributed under the terms of the Creative Commons Attribution License (CC BY). The use, distribution or reproduction in other forums is permitted, provided the original author(s) and the copyright owner(s) are credited and that the original publication in this journal is cited, in accordance with accepted academic practice. No use, distribution or reproduction is permitted which does not comply with these terms.
*Correspondence: Gonzalo Suárez, c3VhcmV6dmVpcmFub0BnbWFpbC5jb20=; Gustavo Salinas, Z3NhbGluQGZxLmVkdS51eQ==