- 1Experimental and Clinical Pharmacology Unit, Centro di Riferimento Oncologico (CRO), IRCCS, Aviano, Italy
- 2Department of Life Sciences, University of Trieste, Trieste, Italy
Antibody-Drug Conjugates (ADCs) represent an innovative class of potent anti-cancer compounds that are widely used in the treatment of hematologic malignancies and solid tumors. Unlike conventional chemotherapeutic drug-based therapies, that are mainly associated with modest specificity and therapeutic benefit, the three key components that form an ADC (a monoclonal antibody bound to a cytotoxic drug via a chemical linker moiety) achieve remarkable improvement in terms of targeted killing of cancer cells and, while sparing healthy tissues, a reduction in systemic side effects caused by off-tumor toxicity. Based on their beneficial mechanism of action, 15 ADCs have been approved to date by the market approval by the Food and Drug Administration (FDA), the European Medicines Agency (EMA) and/or other international governmental agencies for use in clinical oncology, and hundreds are undergoing evaluation in the preclinical and clinical phases. Here, our aim is to provide a comprehensive overview of the key features revolving around ADC therapeutic strategy including their structural and targeting properties, mechanism of action, the role of the tumor microenvironment and review the approved ADCs in clinical oncology, providing discussion regarding their toxicity profile, clinical manifestations and use in novel combination therapies. Finally, we briefly review ADCs in other pathological contexts and provide key information regarding ADC manufacturing and analytical characterization.
1 Introduction
Cancer is a multi-stage progression that transforms normal healthy cells into malignant lesions (Cooper, 2000). It is the leading cause of death worldwide, accounting for nearly 10 million deaths in 2020, and one of the most challenging diseases to treat (Sung et al., 2021). Conventional therapeutic strategies are grouped into chemotherapy, radiotherapy, immunotherapy and surgery, with the former being the main approach for treating various cancers (Dan et al., 2018). Although most remarkable goals have been achieved with small cytotoxic drugs, they had several drawbacks that limited their efficacy, including a low therapeutic index and a high off-tumor effect (Senapati et al., 2018). Usually, the low effectiveness of chemotherapy provoked a high incidence of severe side effects in patients, mainly caused by the non-specific action of chemotherapeutic drugs on rapidly dividing normal cells (Senapati et al., 2018). Therefore, one of the hot topics in the field concerns the discovery of new chemical agents with enhanced therapeutic efficacy and that preferentially ablate tumor-derived cells, without harming the body itself (Casi and Neri, 2012). In the field of immunotherapy, several monoclonal antibodies (mAbs) have been clinically approved as they showed therapeutic benefits in both hematologic malignancies and solid tumors by selectively targeting cancer cells and by activating direct and/or indirect killing mechanisms (Weiner et al., 2009; Kimiz-Gebologlu et al., 2018; Castelli et al., 2019). However, issues including tissue accessibility, poor pharmacokinetics and lame interactions with the immune system have led to the need to exploit newer, safer and more effective targeted therapies (Chames et al., 2009; Talotta et al., 2019). In 1907, German nobel laureate Paul Erlich postulated that there could exist compounds that would selectively target pathogenic microbes, such as bacteria, while sparing normal cells, a concept gone down in history as “zauberkugel” (magic bullet) (Schwartz, 2004). From his hypothesis, an innovative therapeutic approach, known as Antibody-Drug Conjugate (ADC), has arisen in the oncology field and was firstly 40 used years ago to treat patients with advanced cancer (Ford et al., 1983). ADCs are an emerging class of pharmacological compounds that combine the potency of anti-cancer drugs (often called payloads) with the specificity of mAbs to the tumor site, thus combining chemotherapy and immunotherapy. They are composed of three portions, a mAb, an organic spacer and a cytotoxic drug. Ideally, they use the mAb targeting ability to take the cytotoxic agent, which is bound to the mAb via a stable linker, to the cancerous cells or to the cellular components of the tumor microenvironment (TME) where it can exert its anti-tumor activity and lead to cell death (Baah et al., 2021; Mckertish and Kayser, 2021; Fu et al., 2022). Compared with standard chemotherapy, this strategy offers several advantages including better drug tolerability, cytotoxicity even at low concentrations, drug stability in the bloodstream and in lysosomes, reduced off-target effects, and systemic toxicity, all features that contribute to the expansion of its therapeutic potential (Khongorzul et al., 2020; Drago et al., 2021; Mckertish and Kayser, 2021; Fu et al., 2022). Beginning with the first ADC, which was approved by the Food and Drug Administration (FDA) in 2000 (Norsworthy et al., 2018), and given the ever-evolving technology of mAbs, linkers, and payloads, by April 2023 13 different ADCs have been FDA-approved for clinical use for both solid and hematologic malignancies, setting the stage for a new era of targeted cancer therapy (Dumontet et al., 2023). In addition, a few studies have already addressed the potential use of ADCs in non-oncologic context, including infections (O’Leary et al., 2023; Tvilum et al., 2023) and auto-immune disorders (Lee et al., 2017; Yasunaga et al., 2017), with promising results showing the possibility of expanding the use of ADCs in various diseases. In this review, we aim to summarize the current knowledge of ADCs and address some key points about their molecular properties, their interaction with tumor mass and TME, their clinical use, toxicities and combinate regimens in cancer treatment. Finally, we will provide an overview on current research on ADCs in other diseases and address the main challenges and limitations in their production and characterization.
2 ADCs consist of antibodies linked to cytotoxic payloads via a linker
2.1 Antibodies form the scaffold that guides ADCs to target cells
An ADC is a synthetic molecule with pharmacological activity comprising three blocks: a selective mAb, a stable linker, and a potent cytotoxic drug (Figure 1). Together, they ensure tumor-specific targeting and efficient ablation of malignant cells by creating a “new” compound with enhanced therapeutic efficacy.
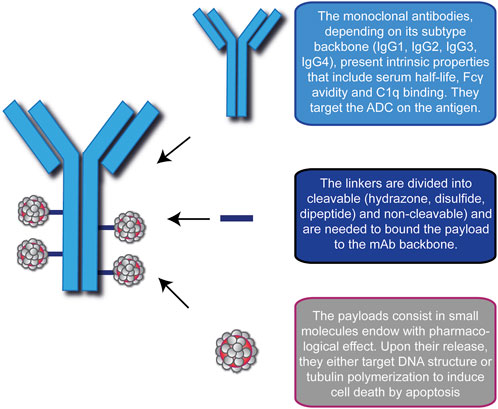
FIGURE 1. Schematic representation of the modular components of an Antibody-Drug Conjugate (ADC). ADC consists of a monoclonal antibody (blue), a linker (blue lines) and the cytotoxic drugs (gray/red). In this picture, the representative ADC on the left has a Drug-to-Antibody ratio equal to 4. A brief description of the basic function of each module is indicated on the right.
2.1.1 Full size antibodies are the most used as ADCs scaffolds
An antibody (Ab) or immunoglobulin (Ig) is a Y-shaped glycoprotein produced by plasma cells that presents an intrinsic selective ability to bind to its target (Baah et al., 2021; Pettinato, 2021). Several Abs used in oncology, upon interaction with their target antigens, possess the capacity to influence the biological activity of the tumor mass by modulating survival-related pathways and/or activating potent immune effector functions through three main mechanisms: antibody-dependent cell-mediated cytotoxicity (ADCC), in which the Fc portion of bound Ab is recognized by Natural Killer (NK) cell Fc-receptor and activates the release of lytic factors, complement-dependent cytotoxicity (CDC), in which the interaction between the Fc region and C1q triggers the classic pathway of the complement system leading to cell lysis (Macor and Tedesco, 2007; Macor et al., 2018) and antibody-dependent cellular phagocytosis (ADCP), a mechanism that relies on active macrophages to engulf tumor cells (Peters and Brown, 2015; Chen et al., 2020; Vozella et al., 2021). Similarly, tumor-targeting Abs in ADCs shall be monoclonal and ensure high target specificity and binding affinity, long half-life in plasma, minimal immunogenicity combined with low cross-reactivity, and allow efficient internalization as well as induce direct/indirect killing effects (Peters and Brown, 2015; Dean et al., 2021; Liu and Chen, 2022). According to the amino acid sequence of their heavy chain constant regions, human Igs are classified into 5 isotypes or classes (IgM, IgG, IgA, IgE, and IgD), with IgG being the most abundant in serum. Based on further amino acid variations, this isotype can be subdivided into 4 subtypes (IgG1, IgG2, IgG3, and IgG4). IgG1 consists of the variable heavy (VH) and light (VL) domains in the N-terminal portion of the antibody, the C-domains of the light chains constant region (CL) and the heavy chain constant regions (CH1, CH2, CH3) and the hinge region between the CH1 and CH2 domains (Chiu et al., 2019). To date, its backbone is the most used in ADC preparations because of its serum half-life (∼21 days), high Fcγ receptors avidity, thereby having strong immune activation of Fc-dependent pathways, and more potent complement activation (Yu et al., 2020). Beside these indirect cytotoxic mechanisms, upon the interaction with specific antigens on malignant cells, IgG1-based mAb can also exert direct killing effects by blocking pathways associated to cancer cell proliferation, metastasis and invasiveness (Natsume et al., 2009; Drago et al., 2021). As for the other subclasses, IgG2 plays a role in the response against bacterial capsular polysaccharides but exhibits low Fcγ receptor avidity, low plasma concentration and tends to form covalent dimers that likely lead to aggregation and ADC inefficacy (Zhang et al., 2018). IgG3 protects the body from a range of intracellular bacteria, parasites, and viruses. They are potent mediators of effector functions, including enhanced ADCC and CDC responses, but they are also the subtype with the shortest circulating half-life (Stapleton et al., 2011; Hoffmann et al., 2018), limiting their suitability for ADCs. The IgG4 subtype has a similar half-life to IgG1 and IgG2 but is less efficient at triggering the C1q-related pathway because it has only intermediate affinity for the Fc receptor on phagocytic cells (Spiess et al., 2013; Fu et al., 2022). However, the ability to induce a poor immune response results in a more favorable safety profile compared to the IgG1 backbone, making IgG4 ADCs suitable in cases where antibody-mediated cytotoxicity is not desired (Herbener et al., 2018).
2.1.2 Antibody-fragments represent a new and innovative approach in ADC strategy
A growing number of studies raises questions regarding the usage of full-size IgG moiety in the treatment of solid cancers and points to some critical limitations, particularly on its size (Deonarain et al., 2018; Richards, 2018). As IgG1, which is the most used in ADC synthesis, is quite large (150 kDa) it may hinder the distribution of the drug in the tumor mass and consequently affect in a negative manner ADC pharmacokinetics and the therapeutic outcome. Although this issue may find a partial solution in the tumor-associated leaky vasculature, which shall still allow sufficient pharmacological benefit due to the retention and permeability effect (EPR) (Ferl et al., 2006), its efficiency and the microdistribution of the compound in the tumor depend on many factors, such as ADC preparations and molecular and cellular signatures of the mass (Deonarain et al., 2018; Richards, 2018). Other problems related to scaffold size include systemic accumulation and slowed target-independent clearance rate (Adams et al., 2001; Jain, 2001; Thurber and Dane Wittrup, 2012). Because of these limitations, researchers are seeking new “miniaturized” versions of natural antibodies (also known as antibody-fragments) as a new and smaller drug-conjugatable alternative to expand the ADC therapeutic benefits. These fragments are produced either by proteolytic cleavage of full-size antibodies or by recombinant protein engineering and primarily retain the binding capacity of full-size IgG through the VH and VL regions (Brinkmann and Kontermann, 2017; Kholodenko et al., 2019). They present engineered scaffolds that lack the CH2 domain and Fc region and include three different formulations: the Fab format, a ∼50 kDa structure in which VL and VH are bound to CL and CH1, respectively, and linked by a disulfide bond between the chains, the single-chain variable fragment (scFv), a ∼27 kDa structure in which VH is linked to VL by a short peptide linker, and the diabody, a non-covalent ∼55 kDa dimer scFv consisting of the VH and VL regions linked by a small peptide linker (Xenaki et al., 2017; Deonarain et al., 2018; Kholodenko et al., 2019). In addition, small immunoproteins (SIPs) composed of dimerized scFvs through a CHε4 domain are a fragmentated format developed against fibronectin and other vascular antigens (Perrino et al., 2014). By preserving the targeting capacity of the full-size antibody and combining it with smaller and dynamic formats, antibody-fragments have the potential to overcome some of the major drawbacks of full-size Ig moieties and may represent an innovation in the treatment of solid tumors. To date, promising data showed a remarkable improvement in stability, tumor targeting and penetration and epitope accessibility, particularly in cancers that are still difficult to reach via conventional IgG-based ADCs (Dennis et al., 2007; Thurber et al., 2008). In addition, smaller formats should be better tolerated by the body and produce fewer adverse effects mainly because they do not show cross-reactivity caused by the interaction with Fc receptor and targeted immune cells (Cilliers et al., 2018; Gogesch et al., 2021). If these benefits should be assessed to a greater extent, significant weaknesses can be identified. For example, because of their smaller size and the absence of the Fc domain, without which they cannot trigger the neonatal Fc receptor rescue pathway, antibody-fragments are degraded more rapidly and potentially do not remain in the body long enough to exert sufficient anti-tumor activity. From this perspective, one solution might be to control the dosing regimen by administering higher and/or more frequent doses to achieve a therapeutic effect, but too little is known about the behavior of these formats in the clinic and extensive efforts are underway to improve their feasibility (Deonarain et al., 2018; Deonarain and Xue, 2020). Moreover, the absence of the Fc domain severely limits the possibility of activating the immune system, thus losing an important partner in the fight against the tumor progression. Besides these formulations, scaffolds not based on antibodies are currently being explored (Luo et al., 2022; Kaplon et al., 2023). Nevertheless, though the Ab moiety properties must be carefully considered, the choice of linker and payload are equally important to create the most suitable therapeutic ADC.
2.2 Linkers are sequences that connect antibodies to payloads by a chemical bond
In the development of the ADC strategy, linkers represent the technology that ensures a bridge between the mAb and the cytotoxic payload. They are the most modifiable part of an ADC and influence its biophysical and functional properties such as stability, potency, efficacy, and toxicity. The two main purposes of linkers are to prevent the premature release of the cytotoxic drug in the blood circulation and to ensure its efficient release at the target site (Lu et al., 2016; Bargh et al., 2019). Depending on the release mechanisms of the payloads, linkers can be broadly classified into two classes: cleavable and non-cleavable. Recent advances in linker chemistry, including formulations not currently used in clinical ADCs, such as biorthogonal, photo-responsive and Fe(II)-cleavable linkers, are discussed in Su et al., 2021.
2.2.1 Cleavable linkers are versatile and widely employed in ADCs
Cleavable linkers are most commonly used in the synthesis of ADCs and are designed to disengage the cytotoxic drug in response to changes in environmental conditions (pH, redox potential, GSH concentration, or enzymatic activity) that occur between the bloodstream, the tumor cells and the TME niche. They are stable under physiological conditions and, following the internalization of the ADC into the tumor cell, they are rapidly cleaved to ensure selective release of the cytotoxic preparation (Peters and Brown, 2015; Tsuchikama and An, 2018). In addition, these linkers are often cleaved in the TME because of its higher acidity and oxidative stress, making them the most used preparation to affect large solid masses barely impenetrable to full-size antibodies (Ponziani et al., 2020). Cleavable linkers are commonly divided into chemical (hydrazone and disulfide bonds) and enzyme (peptide bonds and glucuronide) cleavage linkers (Bargh et al., 2019). Hydrazone linkers are an example of acid-labile linkers used mainly in hematologic malignancies. They are usually stable in the physiological pH range of the blood circulation and undergo hydrolysis within the acidic microenvironment of the endosomes and lysosomes (pH 4.8–6.2) of the tumor cell (Jain et al., 2015). Similarly, linkers based on disulfide bonds are stable in the bloodstream alkaline environment, but payload release is sensitive to glutathione (GSH), a metabolite whose concentration is much higher in the cytoplasm of cancer cells (Balendiran et al., 2004; Estrela et al., 2006). However, both linkers raised concerns about their non-targeted cytotoxicity (Baah et al., 2021; Fu et al., 2022). Peptide bonds ensure that ADC remains integral in the circulation and enable the release of the cytotoxic drug upon interaction with lysosomal proteases (Tsuchikama and An, 2018), such as cathepsin B, which is generally overexpressed in several tumor cell types (Gondi and Rao, 2013). Peptide linkers are associated with improved serum stability and anticancer activity compared chemical linkers (Lu et al., 2016; Tang et al., 2019; Khongorzul et al., 2020). In addition, linkers based on glucuronide bonds, another type of enzyme-sensitive chemical bridge, are commonly used in ADCs design and rely on cleavage by β-glucuronidase, the level of which is often high in the tumor cellular microenvironment (Kostova et al., 2021).
2.2.2 Non-cleavable linkers avoid non-specific payloads release
Non-cleavable linkers include maleimidocaproyl (MC) and 4-maleimidomethyl cyclohexane-1-carboxylate (MCC) structures and consist of chemical structures that are not fragmented by enzymatic degradation. They are inert to conventional chemicals but allow the release of the cytotoxic drug once the mAb has been completely catabolized by the lysosome. In this way, they release their toxic payload into the tumor target cells without harming normal healthy cells. Due to their chemical synthesis, these linkers offer some advantages over the cleavable alternative, including lower toxicity and longer half-life in plasma (Baah et al., 2021; Fu et al., 2022). On the other hand, their limitations are mainly related to their mechanism of action as they are strongly dependent on an efficient intracellular trafficking and on cellular components with high-expression and internalizing antigens (Lu et al., 2016; Tsuchikama and An, 2018).
2.3 Payloads consist of potent cytotoxic agents against tumor cells
As described above, the linker serves as a spacer to connect the mAb to the payload, a cytotoxic drug that must be released in the tumor site to properly exert its pharmacological effects. To be suitable as payloads in ADCs, chemicals shall ideally have low molecular weight and immunogenicity, high stability in the blood circulation and endosomal/lysosomal pathways, and high cytotoxicity (Peters and Brown, 2015; Khongorzul et al., 2020; Baah et al., 2021; Mckertish and Kayser, 2021). Because intravenous administration has shown that only a very small fraction of ADC reaches the tumor (0.1%–2%) (Chari, 2008; Hughes, 2010; Beck et al., 2017), their payloads must be 100- to 1000-fold more effective than the drugs used in chemotherapeutics as free small molecules (Baah et al., 2021). Given that the goal of the ADC strategy is to achieve potent cytotoxic activity, an important attribute to consider in the design process of these compounds is the Drug-to-antibody ratio (DAR), a value that indicates the average number of chemical molecules conjugated to the mAb. For current conjugation methods based on lysine side-chain amidation or mainly on the reduction of cysteine intermediate-chain disulfide bonds, the common DAR values range from 0 (lowest value) to 8 (highest value) (Dan et al., 2018; Wagh et al., 2018; Sun Kang et al., 2021). Nevertheless, in vivo experiments have demonstrated that a high DAR value negatively correlates with Ab pharmacokinetics. Although a low DAR implies the loading of poor number of drug molecules and consequently lower therapeutic efficacy, it is worth noting that an average DAR of 2 to 4 results in an ADC with greater anti-cancer activity/efficacy compared to an ADC with higher DAR, likely because the latter is more rapidly cleared from the body when compared to the corresponding average-conjugated counterparts (Hamblett et al., 2004; Strop et al., 2015). Nowadays, cytotoxic payloads usually act as DNA-damaging agents or tubulin inhibitors, but novel potential drugs under investigation include inhibitors of B-cell lymphoma-extra large (Bcl-xL) anti-apoptotic protein, RNA and Niacinamide phosphate ribose transferase (NAMPT) inhibitors, and carmaphycins, inhibitors of proteasome activity (Wang et al., 2023).
2.3.1 DNA-damaging drugs act as crosslinkers, alkylators and topoisomerase inhibitors
The available agents that induce cell death by damaging DNA can act up to picomolar concentrations (Hartley, 2011) and affect both proliferating and non-proliferating cells, so they can potentially contribute to the ablation of the tumor mass by affecting tumor-initiating cells (Cheung-Ong et al., 2013; Bornstein, 2015). From a mechanistic point of view, these chemicals alter the double helix in different ways, e.g., by inducing single/double strand breaks, alkylation and cross-linking of the DNA minor groove, or by inhibiting Topoisomerase I/II and thus replication. Some of them include amanitins (naturally byciclic octapeptides that inhibit RNA Polymerase II action and disrupt RNA and protein synthesis), calicheamicins (DNA-interactive antitumor antibiotics, that cause DNA double-strand breaks and inhibit replication), duocarmycins (natural DNA minor groove alkylating molecules), and pyrrolobenzodiazepines (highly potent DNA minor groove crosslinking agents). Two other compounds that have been used in first-generation ADCs that are worth mentioning are camptothecin (DNA topoisomerase I inhibitor at the replication bubble) and doxorubicin (antibiotic molecule that damages DNA by intercalating into it and generating free radicals) (Sau et al., 2017; Fu and Ho, 2018; Baah et al., 2021; Mckertish and Kayser, 2021).
2.3.2 Tubulin-targeting agents block the mitotic fuse formation and the cell cycle
Tubulin inhibitors block the rapid proliferation of tumor cells at the G2/M cell cycle stage by binding tubulin subunits, leading to cell death by apoptosis. This class includes maytansinoids and auristatins, a family of tubulin-inhibiting cytotoxins that arrest cells in metaphase. The auristatin derivatives monomethyl auristatin E (MMAE) and the less toxic F (MMAF) (Park et al., 2019) are commonly used as payloads in ADC design and exert their function by blocking tubulin polymerization, thereby perturbing microtubule growth and causing cell cycle arrest (Sau et al., 2017; Baah et al., 2021; Mckertish and Kayser, 2021). Looking at the mechanism in detail, microtubule formation involves either nucleation or assembly of the αβ-tubulin heterodimer into a microtubule seed in the cytoplasm (Francisco et al., 2003; Goodson and Jonasson, 2018). As auristatin, by interfering with GTP hydrolysis on the β subunit, causes an excessive and sustained growth of microtubules, they lose the ability to shorten and separate sister chromatids in anaphase, causing cells to freeze in metaphase of mitosis (Waight et al., 2016).
2.4 The development of ADC therapeutic strategy goes through three generations of compounds
In the last years the ADC development path brought into the marketplace a dozen ADCs against various hematologic and solid malignancies. Generally, these ADCs have been divided into three generations (first, second, and third) according to the type of mAb, the chemistry of the linker, the mechanism of action as well as the relationship between DAR and the conjugation method (Sau et al., 2017; Fu et al., 2022). Representing the first attempt at a novel therapeutic strategy, the first-generation ADCs caused acute adverse effects, such as hematotoxicity, and morbidity in patients, mainly due to the mAbs backbone, linker chemistry, and target non-specificity rather than the drug itself (Sau et al., 2017). Originally, these biologics utilized mouse-derived and then chimeric mAbs conjugated via unstable linkers to the few weakly potent drugs available in chemotherapy. Unfortunately, after administration, these mAbs were recognized as non-self from the body and inevitably triggered an immune response through the formation of human anti-mouse antibodies (HAMA), often leading to serious immunogenicity problems (Kim and Kim, 2015). In addition, the chemistry of acid-labile linkers, which are quite unstable at bloodstream pH, led to uncontrolled release of payloads (Beck et al., 2017), such as calicheamicin, duocarmycin, and doxorubicin, whose potency was in any case too low to cause cancer cell death. In this context, stochastic conjugation to random lysines did not allow to control DAR and resulted in heterogeneous mAbs mixtures containing unconjugated, partially conjugated, and overconjugated mAbs in unknown proportions, which negatively affected ADC efficacy, limited tumor penetration, and resulted in a narrow therapeutic window (Lucas et al., 2018). Furthermore, antigens were selected even though they lacked tumor-specific expression, resulting in severe systemic off-target effects (Sau et al., 2017; Fu et al., 2022). As expected, based on the limitations of the first-generation ADCs, the second-generation ADCs offered some implementations aimed at improving compound efficacy and largely reducing off-target toxicity. To limit potential side effects associated with the mAb backbone as much as possible, humanized mAbs were preferred over mouse-derived or chimeric mAbs due to the lower immunological response upon administration (Lambert and Chari, 2014). Cleavable linkers have been replaced by the non-cleavable alternative to ensure the stability of ADCs in the blood and to reduce the premature and dangerous release of drugs (Sau et al., 2017; Tsuchikama and An, 2018). In addition, far more potent cytotoxic chemicals have been discovered and selected to induce cell death by attacking DNA structure (disrupting its double helix conformation) and tubulin polymerization (disrupting mitotic fusion formation) (Carter and Senter, 2008; Senter, 2009). Due to the low amount of ADC in situ, the IgG1 subtype was preferred over IgG4 because it has better targeting abilities and better conjugation capabilities (Lambert and Chari, 2014). However, despite the introduction of improvements in linker stability and higher drug cytotoxicity, the main weaknesses of this generation of ADCs were still heterogeneous DAR (4–8), rapid clearance for high DAR drugs, off-target toxicity, and drug resistance effects (Sau et al., 2017). What makes the third generation of ADCs the best (so far) is based on fully human mAbs, avoiding the disadvantage of immunogenicity, and optimization in terms of linker stability, payload cytotoxicity, and site-specific conjugation. This new method consists in an evolution of previous ones and was introduced to address heterogeneous DARs and consequently improve ADC pharmacokinetics and utility (Fu et al., 2022). To this end, the synthesis of recombinant Abs bearing engineered cysteine residues enabled the precise bioconjugation of various drugs, resulting in the so-called THIOMAB drug conjugates (TDCs). The THIOMAB antibody technology platform results in a highly stable and effective ADC, whose DAR value is almost uniform (from 2 to 4) and is associated with fewer systemic side effects, improved drug activity, toxicity, and efficacy (Junutula et al., 2008). In addition, despite the potential toxicity due to the high potency of the payloads, these ADCs have lower immunogenicity and hydrophilic linkers, giving patients a more chance to counteract cancer progression (Baah et al., 2021; Fu et al., 2022).
2.5 Binding to specific tumor-related targets triggers internalization and cytotoxicity of ADCs
2.5.1 Choosing the right targeting antigen is critical for ADCs killing
Considering that one of the advantages of ADC is that a potent cytotoxic drug can be delivered specifically to cancer cells, the choice of target antigen must be the first consideration in developing this strategy. To take advantage of the maximal therapeutic index of ADCs, the ideal antigen should be a cell surface structure (such as proteins, glycoproteins, or aberrant gangliosides) that is highly or predominantly expressed on tumor cells compared to healthy, normal cells, or at least abundant on malignant, disease-associated cells (Damelin et al., 2015; Peters and Brown, 2015). Ideally, the target proteins should be tumor-specific antigens (TSAs), which are present only on cancer cell types, and/or tumor-associated antigens (TAAs), which are proteins that are highly overexpressed in tumors but rare or sparsely present in normal tissue. In conjunction with this feature, the epitope of the antigen should ideally face the extracellular matrix (rather than the internal site) to ensure easier accessibility and interaction with ADC after diffusing from blood vessels (Tipton et al., 2015; Zhao et al., 2020). In addition, to avoid undesirable systemic side effects and safety issues, the target antigen should be a protein that is anchored to the membrane and not secreted into the blood circulation. If this were the case, ADC would promote unwanted binding outside the tumor and thus reduce the anticancer effect on malignant cells (Ritchie et al., 2013; Zhao et al., 2020). Nevertheless, after the ADC interaction, the optimal antigen should ensure proper internalization of the antigen- ADC complex into the endosomal/lysosomal pathway, leading to drug release and ultimately cytotoxicity. It should be noted that the speed and efficiency of the internalization process strictly depend on the nature of the target, the type of the epitope, and the payload conjugated to ADC (Carter and Senter, 2008; Donaghy, 2016; Fu et al., 2022). Finally, since the tumor mass and its surrounding microenvironment (TME) are tightly coupled and constantly communicate with each other, TME components have been targeted as novel potential ADC targets (see below in the text) (Andersen, 2023).
2.5.2 The mechanism of action of ADCs requires internalization to exert antitumor cytotoxicity
The presence of a mAb targeting an antigen that is either specifically present on cancer cell types or highly overexpressed during carcinogenesis is a fundamental requirement for the ADC strategy (Alley et al., 2010; Damelin et al., 2015). With this in mind, the mechanism of action of ADCs is quite simple and allows for therapeutic action against the disease as well as potent on-target cytotoxicity (Figure 2) (Peters and Brown, 2015; Khongorzul et al., 2020; Drago et al., 2021; Samantasinghar et al., 2023). After administration, usually by intravenous injection to preserve drug functionality (Nolting, 2013), the mAb portion of the ADC binds to the target antigen on cancer cells, and after internalization of the antigen- ADC complex by receptor-mediated endocytosis, the newly formed early endosome matures into a late endosome that ultimately fuses with the lysosome. In this cellular compartment, acidic or redox conditions combined with the presence of proteases (cathepsin B, plasmin, etc.) allow the detachment of the cytotoxic payload from its mAb carrier, whereupon the drug diffuses into the cell and leads to cell death by attacking DNA structure or microtubule polymerization (Peters and Brown, 2015; Khongorzul et al., 2020; Drago et al., 2021; Samantasinghar et al., 2023). Of note, the IgG subtype that is widely employed in ADC synthesis can be rescued from the endosomal/lysosomal degradation pathway and recycled outside cells through interaction with the neonatal Fc receptor (FcRn), an IgG receptor involved in the regulation of IgG turnover. Therefore, this FcRn-mediated transcytosis into the extracellular space, although involving only a small percentage of the internalized ADC -complex, can potentially enhance the clearance of ADC and thus reduce its therapeutic index (Xu, 2015). On the other hand, if the small molecule is permeable to the cell membrane, it can partially diffuse back into the extracellular matrix and enter neighboring cells regardless of the expression level of the antigen, causing a “bystander effect” (Staudacher and Brown, 2017). This phenomenon, by altering components of the TME, such as neovascular endothelial cells and/or cancer-associated fibroblasts (CAFs), could further enhance the killing effect of ADC, especially in cancer lesions with high heterogeneous target expression (Gerber et al., 2009; Purcell et al., 2018; Szot et al., 2018). Moreover, the therapeutic strategy of ADCs involves other killing mechanisms to ensure efficacy against cancer. In several contexts, it has been shown that the interaction of the mAb with its specific target can directly cause potent inhibition of downstream signaling pathways triggered by antigen receptor stimulation (Albanell et al., 2003; Vu and Claret, 2012; Marei et al., 2022). While the Fab fragment of the carrier is bound to the target epitope on the malignant cell, the Fc portion of the same mAb can interact with the FcR on NK cells and macrophages, triggering ADCC and ADCP, respectively, as well as the C1q component of the complement system, triggering CDC (Junttila et al., 2011; Tai et al., 2014; Redman et al., 2015).
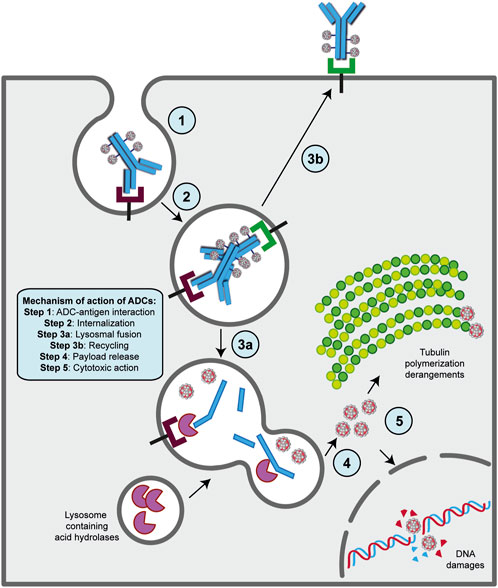
FIGURE 2. Mechanism of action of ADC strategy. The major steps of the process are indicated on the figure. Basically, following ADC-target interaction on the surface of cancer cell (step 1), this complex undergoes receptor-mediated endocytosis and enters the endosomal/lysosomal pathway until the payload is released in the cytoplasm (steps 2, 3a and 4). Then, the drug can exert its killing activity either damaging DNA structure in the nucles or derange mitotic fuse polymerization (step 5), leading to cell death by apoptosis. A fraction of ADCs binds to FcRn receptors in the early step on endosomal/lysosomal pathway and get transported out of the cell (step 2a and 3b).
2.6 The TME offers new potential targets to ADCs strategy
Most of the ADCs in preclinical and clinical development target TAAs or TSAs localized mainly on the cancer mass (Sau et al., 2017; Tong et al., 2021). Compared to hematologic malignancies, solid tumors thrive in a complex and dynamic entity called the TME, whose composition can vary widely depending on the tumor type. Key features of the TME generally include abundant extracellular matrix, stromal cells (e.g., cancer-associated fibroblasts, CAFs), new and abnormal blood vessels, and immune cells, the latter capable of infiltrating the tumor mass and exerting pro- and anti-tumor functions (Anderson and Simon, 2020; Baghban et al., 2020). Particularly in the early stages of tumor growth, a bidirectional and complex interaction exists between cancer cells and the TME components through the release of soluble factors that promote the survival of the tumor mass, its local invasion and subsequent metastatic spread (Visser and Joyce, 2023). In this sense, the TME supports the basic needs of the tumor through a neo-angiogenesis program that removes metabolic waste and, most importantly, restores oxygen and nutrient supply to the mass (Anderson and Simon, 2020; Visser and Joyce, 2023; Wang et al., 2023). Given the close relationship between these two entities, TME-associated antigens (TMAs), i.e., proteins that are dysregulated on non-malignant cells within the TME, offer new potential targets for the treatment of solid tumors as they differentiate from more traditional tumor antigens (Andersen, 2023). Major TMAs include chemokines and cytokines, transcription factors, metabolic enzymes, and checkpoint molecules. Some of their advantages lie in their overexpression on endothelial, stromal, and immune cells, whereas they are rare or very low in healthy tissues, and in their easier accessibility to ADCs when administered into the bloodstream, especially to antigens present on neo-vessels or stromal cells. Furthermore, because TME components are distinct from cancer cells, they are less susceptible to resistance mechanisms caused by inefficient DNA repair mechanisms (Agrafiotis et al., 2022; Ceci et al., 2022; Andersen, 2023). The development of agents against these antigens not only weakens the tumor mass but also provides the opportunity to modulate the TME itself, making it less immunotolerant and more susceptible to tumor ablation (Andersen, 2023). Preclinical and clinical evidence suggest that cell types/factors belonging to the tumor extracellular matrix and neo-blood vessels may be valuable choices for new ADC target antigens (Peters and Brown, 2015; Xiao and Yu, 2021). For instance, an ADC targeting stromal cells may cause cell death by altering the concentration of growth factors in the tumor niche or induce hypoxia and nutrient deprivation by binding to an antigen on the neo-vasculature (Jain, 2005; Mahadevan and Von Hoff, 2007; Xiao and Yu, 2021). In this regard, it is worth mentioning the effect of the “binding site barrier” (BSB), a phenomenon that occurs between mAb and cell populations near blood vessels and retains part of the ADC near them, reducing the penetration of Ab into the tumor mass (van Dongen, 2021). However, most TMAs have been identified on cells of the immune system and targeting them offers an innovative anti-cancer therapeutic approach achieved by promoting effector cell proliferation, the anti-cancer cytokine/chemokine production, and overall survival to create a new immune-hostile tumor niche with reduced neo angiogenesis (Gajewski et al., 2006; Labani-Motlagh et al., 2020; Andersen, 2023; Del Prete et al., 2023). To date, some TMAs targeted by novel ADCs include CD74, an MHC class chaperone II targeted by the ADC STRO-001, currently in phase I in the treatment of B-cell malignancies (NCT03424603) (Le et al., 2023) and CCR7, a chemokine receptor targeted by the novel ADC JBH492 in non-Hodgkin lymhoma and chronic lymphocytic leukemia patients (NCT04240704). In addition, Camidanlumab tesirine, also known as ADCT-301, is an ADC in phase 1/2 for the treatment of classical Hodgkin’s lymphoma (cHL) and non-HL (NCT02432235) (Hamadani et al., 2021), and CD276, an immune checkpoint overexpressed during pathologic angiogenesis and an interesting candidate target of different ADCs in advanced solid tumors (NCT04145622, NCT03729596 and NCT03595059) (Ceci et al., 2022; Ziogas et al., 2023).
2.7 Cancer creates different ways to escape the effectiveness of ADCs
A well-known feature of cancers is their ability to overcome the efficacy of therapeutic approaches, making them susceptible to various mechanisms of resistance. The evasion mechanisms developed by malignant cells can be divided into down-/high-regulation of antigen, the presence of drug efflux pumps, defects in lysosomal functions, and deregulation of signaling pathways involved in cell cycle progression and apoptotic dysregulation (Shefet-Carasso and Benhar, 2015; Loganzo et al., 2016; García-Alonso et al., 2018). In this context, an association between antigen levels and the efficacy of ADC treatment with brentuximab vedotin was observed, whose multiple treatment cycles correlated with CD30 downregulation and consequently stronger tumor resistance to MMAE (Chen et al., 2015). In another study, the cancer cell line JIMT1-TM showed long-term resistance to the drug after repeated administration of anti-human epidermal growth factor receptor 2 (anti-HER2) transtuzumab maytansinoid, as the level of HER2 protein decreased (Loganzo et al., 2015). Nevertheless, upregulation of CD33 antigen in the blood limited gemtuzumab ozogamicin penetration into the bone marrow, suggesting that elevated CD33 levels still negatively affect treatment and likely reduce drug exposure (van der Velden et al., 2004). Another non-negligible mechanism of resistance relies on a family of transmembrane proteins called ABC transporters (Zheng et al., 2021). These transmembrane proteins act as drug efflux pumps, causing various chemicals, including those used as payloads, to be excreted from the cancer cell, making it resistant or at least less susceptible to treatment (Zheng et al., 2021). This mechanism has been observed in AML cells, in which overexpression of the ABC-family member MDR1 made them resistant to gemtuzumab ozogamicin (Matsumoto et al., 2012; Senter and Sievers, 2012) and in breast carcinoma cells, in which cyclic dosing of TDM-1 induced an increase in ABCC1 transporter levels (Loganzo et al., 2015). Another escape mechanism involves lysosomal acidification. After administration of ADC and internalization, linkers are cleaved by lysosomal acid hydrolases to subsequently release the cytotoxic agent into the cytoplasm of cancer cells. However, upon persistent treatments malignant cells may acquire the ability to disrupt this process by altering the pH of the lysosomal compartment to slow the catabolic activity of their proteases, a process that has been demonstrated in HER2-positive breast cancer clones resistant to long-term T-DM1 (Ríos-Luci et al., 2017; García-Alonso et al., 2018). Nevertheless, perturbations in signaling pathways involved in cell cycle regulation and alterations in apoptotic regulation may also modulate tumor cell sensitivity to ADC (Collins et al., 2019). In T-DM1-resistant HER2-positive breast cancer cells, an increase in cyclin B levels, a protein required for the G2/M cell cycle transition, has been observed. This upregulation at the protein level could affect cell cycle dynamics by altering sensitivity to treatment with ADC (Sabbaghi et al., 2017). Moreover, in AML cells, activation of a related pathway (PI3K/Akt) was associated with lower efficacy of gemtuzumab ozogamicin and a deletion in the PTEN pathway was associated with trastuzumab low effectiveness (García-Alonso et al., 2018). Of note, in the same hematologic tumor, overexpression of members of the anti-apoptotic BCL-2 and BCL-X families plays a role in sensitivity to gemtuzumab ozogamicin (Godwin et al., 2020).
2.8 Clinically approved ADCs for the treatment of hematologic and solid malignancies
The ADC strategy represents a highly successful therapeutic alternative in cancer treatment. The excellent ability to deliver a pharmacological compound in situ by drawing the mAb of choice along with various linkers and chemical alternatives represented an innovative development compared to mAb-only based therapy and traditional chemotherapy. As mentioned earlier, although the development of ADC remains challenging in terms of drug safety, efficacy, and targeting, the development of new and more precise technologies, as well as the identification of new targets and components, has led to an explosion in the use of ADC in clinical oncology (Drago et al., 2021; Dumontet et al., 2023; Fuentes-Antrás et al., 2023). To date, hundreds of ADCs are in clinical trials, and 15 of them have been approved by the FDA, the European Medicines Agency (EMA), and/or other government agencies and launched into the marketplace for the treatment of hematologic malignancies and solid tumors. Over the past 23 years, the following ADCs have been developed for the treatment of hematologic tumors: Gemtuzumab ozogamicin (Mylotarg®), Brentuximab vedotin (Adcetris®), Inotuzumab ozogamicin (Besponsa®), Polatuzumab vedotin (Polivy®), Belantamab mafodotin (Blenrep®), Loncastuximab tesirine (Zynlonta®) and Moxetumomab pasudotox (Lumoxiti®), an ADC, which uses an immunotoxin rather than a chemotherapeutic agent as a payload. The ADCs currently approved for solid tumor therapy are Ado-trastuzumab emtansine (Kadcyla®), Fam-trastuzumab deruxtecan (Enhertu®), Enfortumab vedotin (Padcev®), Sacituzumab govitecan (Trodelvy®), Tisotumab vedotin-tftv (Tivdak®), Mirvetuximab soravtansine (ELAHERE®), Disitamab vedotin (Aidixi®), and Cetuximab sarotalocan (Akalux®) (Fu et al., 2022; Kaplon et al., 2023; Yao et al., 2023). A brief description of each agent is provided below and key characteristics are listed in Table 1. In addition, Supplementary Table 1 provides the recruiting clinical trials on the use of novel ADCs being investigated for the treatment of cancer.
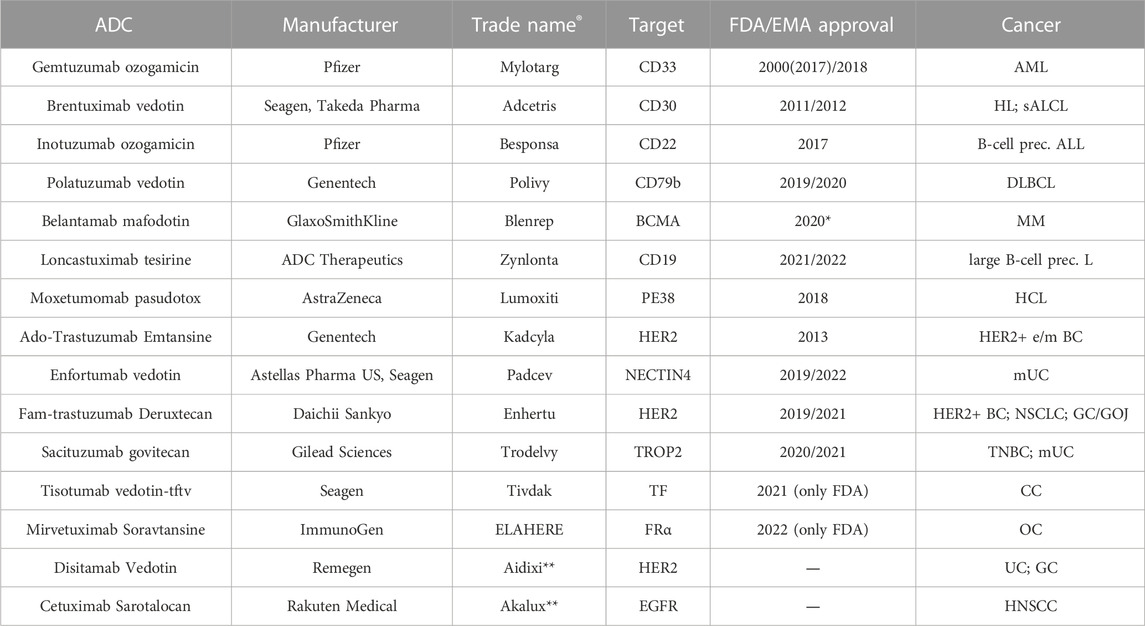
TABLE 1. FDA/EMA approved ADCs in clinical oncology. e early; m metastatic; AML acute myeloid leukemia; HL Hodgkin lymphoma; sALCL systemic Anaplastic Large Cell Lymphoma; B-cell prec. L B-cell precursor leukemia; DLBCL diffuse large B-cell lymphoma; MM multiple myeloma; HCL hairy cell leukemia; BC breast cancer; UC urothelial cancer; NSCLC non-small-cell lung cancer; GC gastric cancer; GOJ gastro-oesophageal junction cancer; TNBC triple negative breast cancer; CC cervical cancer; OC ovarian cancer; HNSCC Head and neck squamous cell carcinoma. The detailed description of the treatment for each disease is described in the text. *: withdraw from market in 2022. **: Aidixi® and Akalux® have not received FDA/EMA approval yet but Akalux® is approved by PMDA (Pharmaceuticals and Medical Devices Agency of Japan) and Aidixi® by NMPA (National Medical Products Administration of China).
2.8.1 Hematologic malignancies
2.8.1.1 Gemtuzumab ozogamicin
Gemtuzumab ozogamicin (Mylotarg®; Pfizer) was the first ADC ever developed and clinically approved by the FDA in 2000 and by the EMA in 2018. As a first-generation ADC, it was based on a humanized anti-CD33 IgG4 antibody linked to the DNA-interactive agent calicheamicin (or ozogamicin) via a hydrazone-cleavable linker bound to surface lysines (average DAR 2–3). It was indicated for the treatment of relapsed/refractory acute myeloid leukemia (AML) though it was withdrawn in 2010, but reapproved at a lower dose in 2017, because patients suffered severe toxicity problems likely due to the higher dose (Fu et al., 2022). Following administration, Mylotarg binds to the CD33 transmembrane glycoprotein on AML cells, and upon internalization, a precursor of calicheamicin is released through hydrolysis of its linker. The active form of the drug then exerts a cytotoxic activity by binding to DNA and breaking its conformation to cause cell cycle arrest and cell death. Of note, the hydrophobic nature of calicheamicin enables a bystander killing of cells in the TME that are negative for the CD33 target antigen (Coats et al., 2019; Kayser and Levis, 2022).
2.8.1.2 Brentuximab vedotin
Brentuximab vedotin (Adcetris®; Seagen, Takeda Pharma) was approved by the FDA in 2011 and by the EMA in 2012 as monotherapy for the treatment of systemic anaplastic large cell lymphoma (sALCL) and in 2018 in combination with chemotherapy for relapsed/refractory Hodgkin lymphoma (HL). It consists of a chimeric IgG1 targeting CD30, a cell membrane protein of the tumor necrosis factor receptor family, on cancer cells and is cysteine conjugated to MMAE (DAR equals 4) through a protease-cleavable linker (Mckertish and Kayser, 2021). After interacting with its target, Adcetris enters the endosomal/lysosomal pathway via clathrin-dependent endocytosis, where its linker is cleaved by acid hydrolases to release MMAE in the cytoplasm. The drug interferes with microtubule polymerization and induces apoptosis and cell death. Like calicheamicin, MMAE exerts its cytotoxic effect on neighboring CD30-negative cells using the bystander killing effect, suggesting that the efficacy of Adcetris in heterogeneous lymphomas in vivo may be related to this effect (Katz et al., 2011; Scott, 2017).
2.8.1.3 Inotuzumab ozogamicin
Inotuzumab ozogamicin (Besponsa®; Pfizer) was approved by the FDA and EMA in 2017. It targets CD22, an antigen expressed on relapsed/refractory B-cell precursors in acute lymphoblastic leukemia (ALL). It consists of a humanized IgG4 mAb linked to calicheamicin via an acid-cleavable linker attached to lysine residues. It has a DAR, ranging from 5 to 7. From a mechanistic perspective, it acts similarly to gemtuzumab ozogamicin in that it is based on the same Ab backbone and loaded with the same drug (Dahl et al., 2016; Garrett et al., 2019; Lanza et al., 2020).
2.8.1.4 Polatuzumab vedotin
Polatuzumab vedotin (Polivy®; Genentech) contains a humanized IgG1 anti-CD79b, a component of the B-cell receptor conjugated to MMAE via the same organic bridge used in the synthesis of brentuximab vedotin. The conjugation method was via engineered cysteines utilizing the THIOMAB system and has a DAR of 3.5. The clinical use of Polivy has been approved by the FDA in 2019 and by the EMA in 2020 for the treatment of adult patients with relapsed/refractory diffuse large B-cell lymphoma (DLBCL) in combination with bendamustine and rituximab (anti-CD20 mAb) (Sehn et al., 2022; Varma et al., 2022). Upon administration, this agent is internalized into cancer cells and proteolytically cleaved to release MMAE, which causes apoptotic cell death by inhibiting tubulin polymerization (Deeks, 2019; Kaplon et al., 2020).
2.8.1.5 Belantamab mafodotin
Belantamab mafodotin (Blenrep®; GlaxoSmithKline) was approved by the FDA and EMA for the treatment of refractory/relapsed multiple myeloma in 2020 (MM) but was withdrawn in 2022 because it did not meet FDA standards (https://www.myeloma.org/news-events/withdrawal-blenrep-us-market). The mAb portion of this ADC is unique in that it consists of a humanized Fc-afucosylated IgG1, a modification that enhances binding and cytotoxicity of the ADC. From the mAb backbone, a cysteine-bound, non-cleavable linker bridges the mAb with the cytotoxic payload MMAF. Its DAR is 4. The target of Blenrep is B cell maturation antigen (BCMA), a member of the tumor necrosis factor receptor family that is overexpressed in mature B lymphocytes and plasma cells (Chen et al., 2020; Yu et al., 2020). As with other ADCs, BCMA targeting internalizes ADC and degrades mAb to release MMAF, a tubulin inhibitor, into the cytoplasm, where it blocks cancer cell cycle progression and leads them to death through apoptosis (Seckinger et al., 2017; Kaplon et al., 2020).
2.8.1.6 Loncastuximab tesirine
Loncastuximab tesirine (Zynlonta®; ADC Therapeutics) targets CD19 and received accelerated approval from the FDA in 2021 and from the EMA in 2022 for relapsed/refractory B-cell lymphoma after two or more lines of systemic therapy, including DLBCL not otherwise specified, DLBCL from low-grade lymphoma, and high-grade B-cell lymphoma. Zynlonta targets CD19, a transmembrane protein commonly expressed in all B cell lineages, and consists of a humanized IgG1 mAb linked to SG3199 (a dimeric PBD alkylating agent) via an enzymatically cleavable linker (DAR of 2–3) (Lee, 2021). The payload exerts its pharmacological benefit by irreversibly binding to DNA and generating a strong adduct that inhibits DNA synthesis and causes cell death. Because SG3199 exhibits cytotoxicity in the picomolar range, it is the most toxic drug currently available on the market ADC. Currently, Zynlonta is the only anti-CD19 drug approved ADC for relapsed/refractory DLBCL as a single agent (Zammarchi et al., 2018; Fu et al., 2022).
2.8.1.7 Moxetumomab pasudotox
Moxetumomab pasudotox (Lumoxiti®; AstraZeneca) is not widely considered an ADC because its payload consists of a fragment of Pseudomonas aeruginosa exotoxin A called PE38. However, since it uses the same targeting mechanism based on mAb, we would still like to consider it as part of the ADC biocompound family. It was approved by the FDA in 2018 for patients with refractory/relapsed hairy cell leukemia (HCL) who have not received at least two systemic therapies. It was granted marketing authorization in the EU in 2021. Lumoxiti is based on an anti-CD22 mouse IgG1 mAb carrying a cleavable linker bound to the immunotoxin PE38. After interaction, internalization, and cleavage, PE38 is released into the cell cytoplasm and acts by blocking translation and inducing cell apoptosis (Kreitman and Pastan, 2011; Kreitman, 2019; Kang, 2021).
2.8.2 Solid tumors
2.8.2.1 Trastuzumab-based ADCs
Since FDA approval of rituximab for the treatment of non-HL in 1997 (Leget and Czuczman, 1998), several mAbs have been investigated and achieved approval in clinical oncology. Considering the mAbs that form the scaffold of approved ADCs, trastuzumab is an example of an anti-cancer molecule used in combination therapy or alone, and also represents the carrier motif in two preparations ADC. Trastuzumab (Herceptin®) is a humanized IgG1mAb that binds to the extracellular domain of HER2, a tyrosine kinase receptor that is upregulated in 20% of breast cancer (BC) patients, preventing its homodimerization and thereby blocking its intracellular signaling (Greenblatt and Khaddour, 2023). Because of its role in cell growth, survival, and differentiation, HER2+ breast cancers tend to grow and spread more aggressively than HER2 negative tumors (Iqbal and Iqbal, 2014). Trastuzumab was approved by the FDA in 1998 for the treatment of HER2+ BC. It improved overall survival (OS) and progression-free survival (PFS) (Albanell and Baselga, 1999; Goldenberg, 1999), but its administration was also associated with risk of cardiac toxicities, such as left ventricular ejection fraction (LVEF) decline and congestive heart failure (Bouwer et al., 2020). In the U.S. it is approved for HER2+ BC in adjuvant therapy (with anthracyclines and taxane) and for metastatic HER2+ BC in monotherapy or in combination with chemotherapeutics, tyrosine kinase inhibitors (TKIs), and immunotherapy. It is also used in a combination regimen for HER2+ gastric cancer (Dumontet et al., 2023). Trastuzumab is administered by intravenous infusion, and the dosing regimen can be adjusted depending on the stage of tumor growth (Greenblatt and Khaddour, 2023). Nowadays, the trastuzumab backbone has been used for the synthesis of two FDA- and EMA-approved ADCs, ado-trastuzumab emtansine (T-DM1, Kadcyla®; Genentech) and fam-trastuzumab deruxtecan (T-Dxd, Enhertu®, Daiichi Sankyo, Astrazeneca), which have improved the OS in the second and third-line settings and are currently used for the treatment of HER2+ early/metastatic and HER2+ low BC, respectively (Ferraro et al., 2021; Rassy et al., 2022).
2.8.2.1.1 Ado-trastuzumab emtansine
Ado-trastuzumab emtansine (Kadcyla®; Genentech) is based on a humanized IgG1 linked to emtansine via a non-cleavable linker attached to the lysine residues. Its average DAR is 3.5 (Mckertish and Kayser, 2021). After interaction with HER2 antigen, Kadcyla is internalized by endocytosis and reaches the lysosome, where IgG1 is completely proteolytically degraded. Subsequently, lysine-MCC-DM1, a DM1-containing metabolite, is released into the cytosol, where it disrupts the microtubule network and causes cell death. Interestingly, lysine-MCC-DM1 has similar toxicity to DM1 but cannot exert its pharmacological effect via the bystander killing effect due to its charge at neutral pH (Barok et al., 2014; Lambert and Chari, 2014). T-DM1 received FDA approval in 2013 for the treatment of advanced HER2+ BC based on data from the EMILIA clinical trial (Phase III). This study evaluated the efficacy of T-DM1 compared to capecitabine and lapatinib in patients with HER2+ BC previously treated with transtuzumab and taxane chemotherapy. Results based on 911 included patients were favorable for T-DM1, whose administration resulted in an improvement in objective response rate (ORR) (43.6% vs. 30.8%), median PFS (9.6 months vs. 6.4 months; p < 0.001), and median OS (29.9 vs. 25.9 months, p < 0.001) after a median follow-up of 47.8 months (Blackwell et al., 2012; Diéras et al., 2017). A few years later, positive results from the KATHERINE clinical trial (phase III) established the novel use of T-DM1 as adjuvant therapy for patients with early-stage HER2+ BC with residual disease after neoadjuvant treatment (taxane and trastuzumab). Among the 1,486 patients who met criteria, those who received T-DM1 showed a significant 50% improvement in invasive disease-free survival (IDFS) at 3 years compared with patients treated with trastuzumab alone in the control arm (88.3% vs. 77%, p < 0.001) (von Minckwitz et al., 2019; Wedam et al., 2020; Mamounas et al., 2021). From the data collected in these two studies, T-DM1 exhibits stronger therapeutic efficacy compared to chemotherapeutic agents and trastuzumab alone in HER2+ early or metastatic BC, likely because this ADC preserves the antineoplastic functions of trastuzumab and adds a novel cytotoxic effect (Ferraro et al., 2021). Remarkably, the superior benefit of T-DM1 was associated with manageable side effects, mostly grade 1 or 2, as only a small percentage of included patients reported elevations in liver enzymes aspartate transaminase (AST) and alanine transaminase (ALT) and thrombocytopenia (Diéras et al., 2017).
2.8.2.1.2 Fam-trastuzumab deruxtecan
Fam-trastuzumab Deruxtecan (Enhertu®; Daiichi Sankyo) is the second ADC HER2-targeting drug approved by the FDA. T-Dxd was approved by the FDA in 2019 and by the EMA in 2021 for the treatment of unresectable or metastatic HER2+ breast cancer (after patients have received two or more anti-HER2 therapies), non-small cell lung cancer, and for locally advanced or metastatic HER2+ gastric or gastroesophageal junction adenocarcinoma, after a trastuzumab-based therapy. It consists of a humanized IgG1-mAb carrying Dxd, a more potent DNA topoisomerase I inhibitor than SN-38, as a cytotoxic payload via an enzymatically cleavable linker. It has a DAR of 7 or 8 (Mckertish and Kayser, 2021; Fu et al., 2022; Dumontet et al., 2023). After internalization of the HER2-Enhertu complex and cleavage, Dxd blocks DNA topoisomerase I, an enzyme that controls and alters the topological state of DNA during transcription, leading to cell death. Compared to Kadcyla, which has the same target, Enhertu has several improvements related to the novel cysteine-conjugated peptide linker, higher DAR and cytotoxicity of the drug, which is more potent and hydrophobic to increase the bystander killing effect on neighboring cells (Kaplon et al., 2020; Shitara et al., 2021). This is essential for extending cytotoxic activity to cells with low or heterogeneous HER2 levels. In this sense, the bystander-killing effect achieved by T-Dxd allows for a better therapeutic response and thus greater cytotoxicity in tumors refractory to its T-DM1 counterpart (Ferraro et al., 2021). In 2019, T-Dxd received accelerated FDA approval based on positive results from the single-arm DESTINY -Breast01 trial (Phase II). In this study, a cohort of 184 female patients with HER2+ metastatic BC who had received two or more prior lines of therapy, including T-DM1, was enrolled to test the efficacy of T-Dxd. Interestingly, 60.9% of patients showed an objective response, and the median duration and median response were 16.4 months and 14.8 months, respectively, with a median time response of 1.6 months (95% CI) (Modi et al., 2020; 2021). In addition, the efficacy of T-Dxd and T-DM1 was evaluated in the DESTINY -Breast 03 trial (phase III), which enrolled 524 patients with HER2+ metastatic BC previously treated with trastuzumab and taxane. Randomization data showed superior efficacy of T-Dxd in terms of ORR (79.9% vs. 34.2%), PFS (not reached vs. 6.8 months, p < 0.001), and OS (94.1% vs. 85.9%) (Cortés et al., 2021; 2022). In general, hematotoxicity, nausea, and fatigue were the most common grade ≥3 adverse effects observed in patients treated with T-Dxd. Of note, treatment with T-Dxd was also associated with pulmonary toxicity and, in particular, interstitial lung disease (ILD), a group of respiratory diseases that require careful management and may lead to treatment discontinuation (Diéras et al., 2017; Cardoso et al., 2020). Finally, the DESTINY-Breast04 trial evaluated the use of T-Dxd versus physician’s choice chemotherapy in 577 patients with low HER2+ metastatic BC who had received prior chemotherapy in the metastatic setting or developed a recurrence within 6 months of completing adjuvant chemotherapy. The results of this study showed that administration of T-Dxd resulted in significantly longer median progression-free (9.9 months versus 5.1 months) and overall survival (23.4 months versus 16.8 months) than pharmacologic therapy in enrolled patients (Modi et al., 2022).
2.8.2.2 Enfortumab vedotin
Enfortumab vedotin (Padcev®; Astellas Pharma US, Seagen) is a fully human IgG1 conjugated to the microtubule inhibitor MMAE via a protease-cleavable linker on cysteine residues and has a DAR of 3.8 (Joubert et al., 2020). It targets nectin-4, a transmembrane protein involved in multiple cellular signaling pathways, including cell adhesion, proliferation, and migration, and is overexpressed in several malignancies. in 2019, Padcev was approved by the FDA for locally advanced or metastatic urothelial carcinoma following Pt-containing therapy and a PD -1 or PD -L1 inhibitor (Alt et al., 2020; Chang et al., 2021) and received EU-wide marketing approval in 2022.
2.8.2.3 Sacituzumab govitecan
Sacituzumab govitecan (Trodelvy®; Gilead Sciences) is an ADC consisting of a humanized IgG1 mAb targeting Tumor-associated calcium signal transducer 2 (TROP2), a transmembrane glycoprotein involved in cell self-renewal, proliferation, invasion, and survival, and plays an important role in intracellular calcium signaling. It is generally overexpressed in most solid tumors, including triple negative breast cancer (TNBC) (Furlanetto et al., 2022). The mAb harnesses to malignant cells SN-38, a DNA topoisomerase I inhibitor that causes DNA breaks and ultimately cell death. IgG1 and payload are connected via an acid-cleavable linker bound to cysteine residues with a DAR between 7 and 8 (Tong et al., 2021). Trodelvy was approved by the FDA in 2020 and by the EMA in 2021 for the treatment of locally advanced or metastatic TNBC in patients who have received at least two prior therapies and in locally advanced or metastatic urothelial carcinoma following Pt-containing therapy and a PD -1 or PD -L1 inhibitor (Mehanna et al., 2019; Bardia et al., 2021; Tong et al., 2021).
2.8.2.4 Tisotumab vedotin-tftv
Tisotumab vedotin-tftv (Tivdak®; Seagen) consists of a fully human IgG1, an enzymatically cleavable linker, MMAE as a payload, and a DAR equal to 4. It targets tissue factor (TF), a membrane protein related to cancer metastasis and invasiveness that is highly expressed in various solid tumors. Being the last ADC on the market, it was approved by the FDA in 2021 for the treatment of relapsed/refractory metastatic cervical cancer with disease progression during or after chemotherapy (Liu et al., 2011; Alley et al., 2019; Heitz et al., 2023).
2.8.2.5 Mirvetuximab soravtansine
Mirvetuximab soravtansine (ELAHERE®; ImmunoGen) was approved by the FDA in 2022 for the treatment of adult patients with Folate Receptor α (FRα)-positive, platinum-resistant epithelial ovarian cancer who have previously received 1–3 systemic therapies. It targets FRα, a member of the folate receptor family that is overexpressed on several epithelial-derived cancer cells (Macor et al., 2006). It consists of a chimeric mAb bound via a cleavable linker to DM4, a potent tubulin targeting agent that belongs to the maytansinoids. Like other ADC, the drug exerts its cytotoxic effect after internalization into cancer cells, leading to their death by blocking their mitotic fuse formation (Dilawari et al., 2023; Heo, 2023; Kaplon et al., 2023).
2.8.2.6 Disitamab vedotin
Disitamab vedotin (Aidixi®; RemeGen) was approved by the NMPA (National Medical Products Administration of China) in 2021 as a second-line treatment for patients with HER2-expressing, locally advanced or metastatic urothelial carcinoma (mUC) who have previously received Pt-containing chemotherapy (Fu et al., 2022), and approved for patients with HER2-overexpressing locally advanced or metastatic gastric cancer who have received at least two systemic chemotherapy regimens (Deeks, 2021). It delivers HER2+ cancer cell MMAE (DAR equals 4) via a cleavable linker bound to a humanized mAb. Interestingly, Aidixi showed high specific antigenic activity and stronger tumor activity compared to other HER2+-targeted ADCs in preclinical experiments and in animal models (Shi et al., 2022).
2.8.2.7 Cetuximab sarotalocan
Cetuximab sarotalocan (Akalux®; Rakuten Medical) received PMDA (Pharmaceuticals and Medical Devices Agency of Japan) approval in 2020 (Gomes-da-Silva et al., 2020). It is comprised of a chimeric IgG1 mAb specifically targeting epidermal growth factor receptor (EGFR), the triggering of which is involved in cell proliferation, angiogenesis, and invasion/metastasis. The conjugation of Akalux is not with a small molecule, but with a light-activatable near-infrared dye called 700DX (DAR 1.3–3.8) (Fu et al., 2022). In this case, we would also like to consider it as ADC given its mechanism of action against cancer cells. After interaction with ADC-EGFR, this ADC inhibits EGFR signaling pathway and achieves high anticancer effect by laser activation of 700DX dye. In this way, malignant cells are targeted and rapidly eliminated while healthy cells surrounding the tumor mass are spared. It has been approved for the treatment of unresectable locally advanced or recurrent head and neck squamous cell carcinoma (HNSCC) (Kitamura et al., 2020; Omura et al., 2023).
2.9 The therapeutic strategy of ADC reveals challenges and limitations and can be combined in combinatorial regimens
2.9.1 Payloads are the main cause of ADCs toxicity
In 2 decades, ADCs have achieved remarkable results in the treatment of hematologic malignancies and solid tumors and represent a valid alternative in the field of clinical oncology. Although they have been designed to release cytotoxic agents by targeting selective cell populations, a striking number of clinical trials have shown that ADCs are not free of adverse effects, sometimes leading to toxicities commonly observed with conventional chemotherapy (Dumontet et al., 2023; Tarantino et al., 2023). In general, a significant proportion of patients suffered various toxicities, sometimes so severe (or fatal) to require dose reduction or interruption, treatment delays, and supportive medications (Dumontet et al., 2023; Tarantino et al., 2023). Each of the blocks that form an ADC can result in significant side effects when these compounds are administered to the human body. Even if the nature of mAbs is responsible for moderate/severe immunogenic side effects, especially in ADCs preparations using murine and chimeric mAb scaffolds (Kim and Kim, 2015), the primary manifestation of a toxicity profile is highly dependent on the type of payload (Zahavi and Weiner, 2020). Most ADCs used in the clinic are loaded with tubulin inhibitors or DNA-interacting agents that exert their cytotoxic effects in the range of nano- or picomolar concentrations and are highly toxic when used in unconjugated form (Mecklenburg, 2018). Unfortunately, because only a very small percentage of ADCs reach their targets and a part of the payload is released prematurely, a significant portion of the dose is virtually free to interact with numerous non-target healthy cells and cause unconventional systemic or local side effects (Dahlgren and Lennernäs, 2020). The out-of-target toxicities are closely related to the linker. Non-cleavable linkers exhibit greater stability in plasma and the ADCs are better tolerated by the body. However, in the majority of ADCs used in the clinic and foremost to the ones use in solid tumors, cleavable linkers are preferred as they have shown more benefits, probably due to the bystander side effect. This out-of-cell toxicity has two sides. On the one hand, it may extend the efficacy of treatment to antigen-negative cells found in the tumor niche, to cells that form the core of the tumor mass being less accessible to the ADC (Jain, 2005; Mahadevan and Von Hoff, 2007; Xiao and Yu, 2021), and to cells that have low/heterogeneous expression of the antigen. On the other hand, due to the non-specific effect of the conventional small drugs, normal cells can also suffer severe damages with potential unpredictable consequences (Donaghy, 2016; Staudacher and Brown, 2017). Other limitations relate to the pharmacokinetic properties of ADCs. Rapid clearance and aggregation represent two important aspects that may negatively impact the therapeutic activity of these compounds (Lucas et al., 2018; Mahmood, 2021; Pettinato, 2021). To solve complications and improve the body compatibility of ADC, the mAb component and linker can be chemically modified by glycosylation or PEGylation (Mahmood, 2021; Edwards et al., 2022). While the former has not been studied in detail within this platform, the latter allows overcoming some drawbacks (Pettinato, 2021). PEGylation involves the addition of polyethylene glycol (PEG) to specific amino acid residues. In general, it has been shown that the use of PEG as a linker can improve the solubility of ADCs and reduce aggregation, improving their pharmacokinetic by enhancing stability and distribution in the body (Verhoef and Anchordoquy, 2013; Mahmood, 2021).
2.9.2 Clinical manifestations of ADCs include major toxicities
As ADCs are developed to limit their exposure to healthy tissues, they are associated with quite manageable toxicities, with nausea, vomiting, diarrhea and fatigue being among the most frequent ones. Unfortunately, the large amount of data provided by several clinical trials also highlights the presence of severe toxicities (grade 3 or higher) that include peripheral neuropathy and hematotoxicity, often dose-limiting (Masters et al., 2018; Professional Committee on Clinical Research of Oncology Drugs et al., 2022). Peripheral neuropathy includes tingling, pain in the extremities, numbness, and rarely muscle weakness and is commonly associated with ADCs carrying cleavable linkers bound to tubulin inhibitors (i.e., all ADCs loaded with MMAE) and Mirvetuximab soravtansine, carrying DM4 (Nguyen et al., 2023). As cleavable linkers are associated to the premature drug release and that these compounds block tubulin polymerization, this common side effect is not unexpected as microtubules are deeply involved in the axonal transport, an essential process to the growth and maturation of neurons (Yogev et al., 2016). Hematologic side effects include anemia, neutropenia, thrombocytopenia, leukopenia and are mainly due to the off-target Fc receptor-mediated uptake of ADCs into immune cells, with neutropenia being the most prominent toxicity in ADC-based monotherapy (Mahalingaiah et al., 2019). Besides, major toxicities responsible for dose limitations are related to drug classes and mainly include hepatotoxicity (for MMAF, DM1, and calicheamicin), skin toxicity (for MMAE and PBD), and ocular toxicity (for MMAF and DM4) (Zhao et al., 2020; Hurwitz et al., 2023). Of note, the mechanism underlying corneal toxicity has not been solved yet but occurred in a relevant percentage of patients treated with Belantamab mafotodin, Trastuzumab emtansine and Mirvetuximab soravtansine, all loaded with tubulin inhibitors (Aschauer et al., 2022; Domínguez-Llamas et al., 2023). Other relevant clinical manifestations include gastrointestinal side effects upon administration of Sacituzumab govitecan and Trastuzumab deruxtecan, two ADC used in the treatment of breast cancer and loaded with Topoisomerase inhibitors and serosal effusion for duocarmycin and PBD, the latter responsible of nephrotic toxicity in Loncastuzimab tesirine preparation (Nguyen et al., 2023). In addition, Trastuzumab emtansine and Trastuzumab deruxtecan, both targeting HER2+ breast cancer, are associated with an increased risk of interstitial lung disease (ILD)/pneumonitis, which must be carefully managed with dose adjustment and supportive care recommendations to avoid fatal outcomes (Ma et al., 2018; Hackshaw et al., 2020). Like other strategies based on small molecules and immunotherapy, ADCs show some challenges that need to be carefully addressed to improve their efficacy and reduce systemic side effects. A variety of approaches is being taken into consideration in clinics to deeply counteract the manifestation of side effects, and many of them focus on an individual basis. Extensive efforts are being made to identify patients who have potentially life-threatening toxicities at an early stage and offer them supportive measures, such as dose and schedule adjustments. The investigation of patients’ history and data on previous treatments, comorbidities, or genetic profiles, including pharmacogenetic analysis to identify SNPs or potential mutations in key genes, will undoubtedly play a critical role in the determination of the best strategy to improve the tolerability and efficacy of ADCs (Lambert and Morris, 2017; Dumontet et al., 2023; Tarantino et al., 2023).
2.9.1 ADCs can be used in combination therapies
In addition to the use of ADCs as monotherapy, recent preclinical and clinical studies have also focused on ADCs combinations with chemo-immunotherapics. Ideally, concomitant administration of ADCs with antiangiogenic agents, i.e., agents that damage neo-blood vessels to facilitate ADC penetration into the tumor niche, or immunomodulatory drugs that promote immune surveillance should amplify the body’s anti-neoplastic response to the tumor mass and its TME, without or with limited severe toxicities and safety issues (Dumontet et al., 2023; Fuentes-Antrás et al., 2023). Regarding ADC combination with chemotherapy, promising data include the combination of Brentuximab vedotin and CHP (cyclophosphamide, doxorubicin, and prednisone) and Polatuzumab vedotin and Rituximab-CHP in CD30+ peripheral T cell lymphoma (Herrera et al., 2021) and in DLBCL (Tilly et al., 2022), respectively. Similarly, the combination therapy based on Trastuzumab emtansine and the selective anti-HER2 tyrosine kinase inhibitor (TKI) tucatinib achieved remarkable benefits in metastatic breast cancer (Nader-Marta et al., 2022) and the administration of bevacizumab, the mAb targeting the Vascular-Endothelial Growth Factor (VEGF), with Mirvetuximab soravtansine obtained similar benefits in preclinal models of ovarian cancer (Ponte et al., 2016). Combinatorial approaches using ADCs and immunotherapeutic agents have been recently explored in several types of cancers (Fuentes-Antrás et al., 2023). Immune checkpoint inhibitors (ICIs) are immunotherapy that enhance antineoplastic immune responses by converting exhausted T-cells into activated ones and bypassing the pathways that cause the tumor escape from the immune system (Shiravand et al., 2022; von Arx et al., 2023). ICIs targeting Cytotoxic T-Lymphocyte Antigen 4 (CTLA-4) and the Programmed Cell Death Protein 1/its ligand (PD-1/PD-L1) axis have demonstrated robust clinical activity in several malignancies but only a fraction of patients experience long-term benefits with monotherapy (Wojtukiewicz et al., 2021). From this perspective, an effort could come from ADCs, capable to enhance antitumor immune responses by inducing tumor-specific adaptive immunity through increasing T cell infiltration into the TME, while ICIs revitalize exhausted T cells (Nicolò et al., 2022). Accordingly, encouraging results come from the combination of ICIs and HER2-targeted trastuzumab emtansine in the treatment of PD-L1+, HER2+ advanced breast cancer (Emens et al., 2020).
2.10 ADC strategy shows application in non-oncology indications
In recent years, research groups have investigated the use of ADCs in non-oncologic contexts, mainly focusing on the treatment of bacterial infections and autoimmune diseases. Tvilum et al. achieved antimicrobial efficacy by developing an Antibody-Antibiotic Conjugate (AAC), an antibody directed against bacteria conjugated to the antimicrobial molecule mitomycin C, for the treatment of implant-associated biofilm infections caused by Staphylococcus aureus, the most common causative agent in prosthetic joint infections (Tvilum et al., 2023). In another study, O-Leary et al. proposed the development of an Antibody-Bactericide Conjugate (ABC), an antibody conjugated to an antimicrobial peptide that exerts its effect by binding to the cell surface of P. aeruginosa, providing another interesting example of ADC activity against bacterial infections (O'Leary et al., 2023). Regarding inflammatory diseases, Yasunaga et al. developed an ADC targeting IL-7 receptor (IL-7R) conjugated with MMAE and showed that ADC-mediated immunoregulation of the IL-7R, the upregulation of which is a common mechanism in the pathogenesis of autoimmunity, specifically depleted IL-7R-positive cells in the inflammation site of a mouse model of autoimmune arthritis and abrogated disease progression (Yasunaga et al., 2017). In the same year, Lee et al. demonstrated in a model of rheumatoid arthritis (RA) the immunosuppressive efficacy of TCZ-ALD, an ADC that targets the IL-6 receptor (IL-6R) and is conjugated to the small molecule alendronate. TCZ-ALD blocks the IL-6R activity and macrophages activity in the manifestation of RA symptoms and joint inflammation (Lee et al., 2017). A few years later, Gillard et al. achieved immune reset of disease-causing reactive T cells by a single administration of CD45-ADC and bone marrow transplantation, resulting in significant disease-modifying effect in mouse models of autoimmune disease (Gillard et al., 2020). In addition, a few studies also addressed the use of ADCs in cardiovascular (Lim et al., 2015) and renal diseases (Kvirkvelia et al., 2015), highlighting the potential application of this therapeutic strategy in other pathological conditions.
2.11 Challenges and solutions in the manufacturing of ADCs
2.11.1 Process development consists of several steps
The main goal in the manufacture of ADCs by companies is to produce a pure and bioburden-free compound that is safe for the human body for clinical use. Since the preparations of ADC consist of mixtures of mAbs with multiple conjugation sites and small organic molecules, several analytical steps are required in the development of these compounds, and several challenges must be overcome. Overall, the production of ADCs can be divided into three steps: production of the antibody, synthesis of the drug-linker complex, and conjugation to form the final ADC (Hutchinson et al., 2018; Bulger et al., 2023). The conjugation step is of fundamental importance as it determines the therapeutic efficacy of the biomolecule. Conventional conjugation methods based on lysine side-chains or reduced cysteines result in heterogeneous ADC preparations whose safety and therapeutic efficacy are difficult to predict. To overcome this crucial limitation, site-selective conjugation methods have recently been developed. In this way, a known number of drug molecules are constantly bound to selected sites on mAbs, resulting in a more homogeneous mixture with improved batch-to-batch consistency and therapeutic efficacy (Cao et al., 2019; Nejadmoghaddam et al., 2019). After the conjugation step, ADC proceeds to purification and filling into aseptic vials, all following the sterile pipeline of current Good Manufacturing Practices (cGMP). Quality is controlled throughout the development process. Production requires a biological manufacturing environment that allows for the safe handling of sensitive structures, such as various powders and chemicals, purified mAbs, and high potency drugs, to minimize potential pollution and losses that may occur at various stages of production. To ensure product purity and sterility, synthesis and bioconjugation reactions are performed in aseptic rooms and conditions, with regular documentation of instrument accuracy in accordance with cGMP standards. To reduce potential contamination and exposure from the use of hazardous substances, each step of the experimental process is achieved by providing in-depth expertise and specialized equipment to personnel and operators (Hutchinson et al., 2018; Schmidhalter et al., 2019). In addition, the production of ADC usually involves several departments/services and, in this sense, an extremely low temperature supply chain and secure packaging must be ensured to reduce possible variations, damage and leakage during long-term storage and transportation (Ducry, 2012). Considering the complexity and number of processes involved, the production of ADCs is quite laborious, time-consuming and economically expensive. Large capital investments are required to cover a multitude of steps ranging from the design of the experiment, the invention and development of new drugs to product innovation, differentiation, and safety monitoring, all at a cost that must make the finished drug marketable (www.cbo.gov). To overcome these barriers, contract development and manufacturing organizations (CDMOs), companies that provide drug development and manufacturing services to the pharmaceutical industry, are turning to single-use technologies (SUTs) and automated end-to-end systems (Schmidhalter et al., 2019). SUTs are sterile, single-use items made of various plastics, most of which can be used in the same way as their stainless-steel counterparts without the need for sterilization and recycling after use. As a result, these technologies offer significant advantages over traditional reusable systems by reducing the risk of cross-contamination, better ensuring sterility, allowing more flexibility throughout the process and, most importantly, improving cost efficiency and reducing time to market (Schmidhalter et al., 2019). In these terms, continuous improvement in technology and investments by major biopharmaceutical companies in extensive research programs will drive the growth of the ADC market (valued at approximately $7 billion in June 2022 but expected to reach $22.4 billion in 2030 (www.researchandmarkets.com) and increase the number of available ADC-based therapies in new medical areas.
2.11.2 Analytical characterization startegies
Another challenge in the production of ADCs is the evaluation of their biochemical attributes to obtain a safe and effective product. Critical quality attributes of ADCs that must be carefully controlled during the manufacturing process include determination of DAR and distribution of drug, residual non-conjugated species, especially in terms of mAb and payload, and evaluation of size and charge variants in the final preparations. Nowadays, various in vitro instruments and techniques are used either alone or in combination to perform comprehensive analytical characterization of ADCs, including spectroscopic, chromatographic, and mass spectrometric methods (Wagh et al., 2018; Liu et al., 2022).
2.11.2.1 Determination of purity
Purity of compound is a fundamental goal in any biopharmaceutical manufacturing process. One of the techniques used to evaluate the purity of ADC preparations is size exclusion chromatography followed by ultraviolet detection (SEC-UV), a method that separates molecules by size and, in some cases, molecular weight, and is used to fractionate large macromolecules such as proteins (e.g., mAbs) or protein complexes (de Mel et al., 2019). In ADC synthesis, separation of macromolecules by size allows purification of the mAb from potential fragments, aggregates, and particles, three examples of undesirable species that affect the efficacy of the final ADC as well as its safety when administered to patients (Jiang et al., 2019). In general, coupling SEC with multi-angle laser light scattering (SEC-MALS) offers some advantages for biopharmaceutical applications. In MALS detection, a laser beam passes through a sample solution containing the target molecule, and depending on the size of the molecules, the intensity of the scattered light is measured at specific angles (Some et al., 2019). Compared to SEC itself, SEC-MALS requires high-purity columns but provides additional information by increasing the sensitivity for detecting impurities in the preparation (Beck et al., 2019). Other techniques for determining the presence of aggregation or fragmentation include dynamic light scattering (DLS), sedimentation velocity analytical ultracentrifugation (SV-AUC) (Ducry, 2012), and capillary electrophoresis followed by sodium dodecyl sulfate analysis (CE-SDS), a valuable method commonly used in the biopharmaceutical industry for mAbs and ADC preparations to determine batch consistency and overall protein purity (Wagner et al., 2020). In addition, various residual species in ADC preparations may pose a potential safety risk to patients and are a critical quality attribute that must be carefully evaluated. The levels of these unconjugated forms can be monitored by measuring the charge on the molecules (Wakanar, 2011). Since the bioconjugation reaction between the mAb and the payload can significantly alter the electrostatic profile of the newly formed ADC, charge-based separation techniques such as ion exchange chromatography (IEC), isoelectric focusing gel electrophoresis (IEF), and capillary isoelectric focusing (cIEF) can provide information on charge heterogeneity, drug distribution pattern, and overall preparation quality (Ducry, 2012). To date, imaged capillary isoelectric focusing (iCIEF) is considered a robust method in biopharmaceutical quality control because it can quantitatively separate samples based on the isoelectric point (pI) of individual variants (Wagh et al., 2018; Abbood, 2023). Therefore, iCIEF can be used to rapidly measure the content of free mAb in an ADC mixture according to the different pI of the mAb and its conjugated form. However, the drawbacks of this assay are that conjugates cannot be distinguished from reaction intermediates and other impurities, and it can only be used for conjugation chemistries that result in significant changes in charge and net pI (Wagh et al., 2018).
2.11.2.2 Determination of DAR and drug distribution
Probably the hottest topic in the ADC manufacturing process is the ability to achieve bioconjugation reactions that lead to the synthesis of a homogeneous mixture and thus a controlled DAR and payload. Various analytical methods have been used to determine these parameters, including ultraviolet/visible spectroscopy (UV/Vis) (Andris et al., 2018), hydrophobic interaction chromatography (HIC) (Andris and Hubbuch, 2020), reversed-phase liquid chromatography (RPLC) (Chen et al., 2019), and mass spectrometry (MS). As for MS, its extended use in characterization of ADCs is discussed in other works (Debaene et al., 2014; Zmolek et al., 2016; Liu and Chen, 2022; Barbero et al., 2023). UV/Vis spectrometry is relatively easy to measure DAR compared to the other techniques, although it requires sufficiently different absorption profiles between the mAb and the payload and a UV/Vis chromophore on the payload (Wagh et al., 2018). Among liquid chromatographic methods, HIC and RPLC are routinely used to measure average DAR and drug distribution (Ouyang, 2013; D’atri et al., 2019). HIC is a method that allows determination of species distribution based on differences in their hydrophobic properties and uniquely preserves the native structures and activity of ADCs. Because the analysis is performed under mild, non-denaturing conditions, ADCs can be studied in their native conformation, which is an advantage because isolation of chromatographically pure species allows their further characterization in subsequent analysis. This method is commonly used to analyze cysteine-conjugated ADCs and other site-specific conjugations but cannot be applied to ADCs obtained by lysine conjugation because the greater heterogeneity of these preparations complicates chromatographic separation (D’atri et al., 2019; Liu and Chen, 2022). Nevertheless, HIC requires a large amount of starting material, shows low efficiency for randomly conjugated ADCs, and cannot separate positional isomers from cysteine-conjugated ADCs or determine the chain, H or L, to which the drug was conjugated (Becker et al., 2020; Fleming, 2020; Liu and Chen, 2022). The RPLC method also separates the components of a mixture according to their hydrophobicity, but this approach requires denaturation of the proteins, resulting in the loss of some information about the distribution of some ADCs and the loss of certain DAR species (Liu and Chen, 2022).
2.12 Conclusion and perspectives
Nowadays, ADC represents a solid strategy to treat different types of malignancies. The design of ADCs provides an exceptional opportunity to selectively deliver an effective anti-tumor chemical to the target cell and eliminate it without severe toxic off-target effects. The main advantage of ADC lies in its mechanism of action, as it offers the potential to overcome some major limitations of conventional small molecule-based therapies, such as low therapeutic index and high off-tumor toxicity The possibility of killing neighboring antigen-negative cells, through the bystander effect, in the mass and in the TME and the potential activation of direct and indirect anti-cancer mechanisms via mAb-antigen interaction and immune cell activation, respectively, argue for their use in the clinic (Figure 3). In this context, it is worth noting that the benefits associated with the activity of ADCs when administered as monotherapy may encounter consistent clinical limitations due to the presence of tumor-derived resistance mechanisms and several manageable and few severe adverse effects mainly caused by the payload of the ADC (Fuentes-Antrás et al., 2023). Despite the promising results obtained so far, the ADC technology is still under investigation and has some limitations in terms of its pharmacokinetic properties and biological efficacy in different tumor contexts. To develop a more suitable generation of ADCs, future prospects in this field are based on the optimization of available technologies and especially on the discovery of new methodologies. Accordingly, profound improvements in the validation of newly developed mAbs, in the synthesis of less immunogenic and more stable linkers, and in the discovery of more effective payloads are being actively explored by scientists in this field, and similar advance are also focused on the development of more appropriate formulations as well as on the identification of new target antigens. Given the central role of the TME in solid tumor progression and spread, targeting novel candidates upregulated in stromal cells, blood vessels and most importantly immune cells within the TME could lead to greater inhibition of tumor escape mechanisms and metabolic dysfunctions to achieve long-lasting therapeutic effects. Moreover, combinatorial therapies with different drug classes have already shown synergistic and promising results in the treatment of hematologic and solid tumors by enhancing the anticancer efficacy and therapeutic index of ADCs. Based on these observations, all these efforts are aimed at developing a new generation of ADCs that will undoubtedly show significant improvements in terms of pharmacological properties, therapeutic efficacy and safety in the field of oncology and in other pathological conditions.
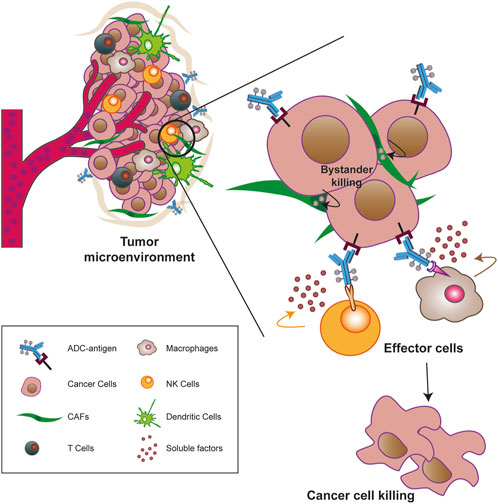
FIGURE 3. Overview on the tumor-targeting ADCs. Taking advantage of blood circulation, ADCs reach the tumor microenvironment (TME), which is composed by cancer-associated fibroblasts (CAFs) and other cell types and interact with malignant cells that exposed the tumor associated/specific antigen on their surface. In addition to their canonical mechanism of action, ADCs can potentiate their positive response against the tumor mass. To this aim, ADCs hydrophobic payloads may diffuse through the cell membrane inducing the killing of neighborhood antigen-negative cells via bystander killing effect (black arrows) and/or the ADCs antibody Fc fragment may elicit anti-tumor immunity (ADCC, CDC, and ADCP) by engaging immune effector mechanisms, such as complement system, macrophages and NK cells. All together, these mechanisms aim to induce the death of the cellular component of the tumor mass via apoptosis.
Author contributions
FR: Writing–original draft. MDB: Writing–review and editing. PM: Writing–review and editing. GT: Writing–review and editing.
Funding
The authors declare financial support was received for the research, authorship, and/or publication of this article. The research leading to these results has received funding from the European Union—NextGenerationEU through the Italian Ministry of University and Research under PNRR—M4C2-I1.3 Project PE_00000019 “HEAL ITALIA” to MDB (Spoke 5) and GT (Spoke 6) (CUP J33C22002930006 of Centro di Riferimento Oncologico di Aviano, IRCCS). This research was supported in part by the Italian Ministry of Health (Ricerca Corrente).
Conflict of interest
The authors declare that the research was conducted in the absence of any commercial or financial relationships that could be construed as a potential conflict of interest.
Publisher’s note
All claims expressed in this article are solely those of the authors and do not necessarily represent those of their affiliated organizations, or those of the publisher, the editors and the reviewers. Any product that may be evaluated in this article, or claim that may be made by its manufacturer, is not guaranteed or endorsed by the publisher.
Author disclaimer
The views and opinions expressed are those of the authors only and do not necessarily reflect those of the European Union or the European Commission. Neither the European Union nor the European Commission can be held responsible for them.
Supplementary material
The Supplementary Material for this article can be found online at: https://www.frontiersin.org/articles/10.3389/fphar.2023.1274088/full#supplementary-material
References
Abbood, A. (2023). Optimization of the imaged cIEF method for monitoring the charge heterogeneity of antibody-maytansine conjugate. J. Anal. Methods Chem. 2023, 8150143. doi:10.1155/2023/8150143
Abrahams, C. L., Li, X., Embry, M., Yu, A., Krimm, S., Krueger, S., et al. (2018). Targeting CD74 in multiple myeloma with the novel, site-specific antibody-drug conjugate STRO-001. Oncotarget 9, 37700–37714. doi:10.18632/oncotarget.26491
Adams, G. P., Schier, R., McCall, A. M., Simmons, H. H., Horak, E. M., Alpaugh, R. K., et al. (2001). High affinity restricts the localization and tumor penetration of single-chain fv antibody molecules. Cancer Res. 61, 4750–4755.
Agrafiotis, A. C., Siozopoulou, V., Hendriks, J. M. H., Pauwels, P., Koljenovic, S., and Van Schil, P. E. (2022). Tumor microenvironment in thymic epithelial tumors: A narrative review. Cancers (Basel) 14, 6082. doi:10.3390/cancers14246082
Albanell, J., and Baselga, J. (1999). Trastuzumab, a humanized anti-HER2 monoclonal antibody, for the treatment of breast cancer. Drugs Today (Barc) 35, 931–946. doi:10.1358/dot.1999.35.12.564040
Albanell, J., Codony, J., Rovira, A., Mellado, B., and Gascón, P. (2003). Mechanism of action of anti-HER2 monoclonal antibodies: scientific update on trastuzumab and 2C4. Adv. Exp. Med. Biol. 532, 253–268. doi:10.1007/978-1-4615-0081-0_21
Alley, S. C., Harris, J. R., Cao, A., Heuvel, E. G., Velayudhan, J., Satijn, D., et al. (2019). Abstract 221: Tisotumab vedotin induces anti-tumor activity through MMAE-mediated, Fc-mediated, and Fab-mediated effector functions in vitro. Cancer Res. 79, 221. doi:10.1158/1538-7445.AM2019-221
Alley, S. C., Okeley, N. M., and Senter, P. D. (2010). Antibody-drug conjugates: targeted drug delivery for cancer. Curr. Opin. Chem. Biol. 14, 529–537. doi:10.1016/j.cbpa.2010.06.170
Alt, M., Stecca, C., Tobin, S., Jiang, D. M., and Sridhar, S. S. (2020). Enfortumab Vedotin in urothelial cancer. Ther. Adv. Urol. 12, 1756287220980192. doi:10.1177/1756287220980192
Andersen, M. H. (2023). Tumor microenvironment antigens. Semin. Immunopathol. 45, 253–264. doi:10.1007/s00281-022-00966-0
Anderson, N. M., and Simon, M. C. (2020). The tumor microenvironment. Curr. Biol. 30, R921–R925. doi:10.1016/j.cub.2020.06.081
Andris, S., and Hubbuch, J. (2020). Modeling of hydrophobic interaction chromatography for the separation of antibody-drug conjugates and its application towards quality by design. J. Biotechnol. 317, 48–58. doi:10.1016/j.jbiotec.2020.04.018
Andris, S., Rüdt, M., Rogalla, J., Wendeler, M., and Hubbuch, J. (2018). Monitoring of antibody-drug conjugation reactions with UV/Vis spectroscopy. J. Biotechnol. 288, 15–22. doi:10.1016/j.jbiotec.2018.10.003
Aschauer, J., Donner, R., Lammer, J., Roberts, P., Funk, M., Agis, H., et al. (2022). Corneal toxicity associated with Belantamab mafodotin is not restricted to the epithelium: Neuropathy studied with confocal microscopy. Am. J. Ophthalmol. 242, 116–124. doi:10.1016/j.ajo.2022.06.009
Baah, S., Laws, M., and Rahman, K. M. (2021). Antibody–drug conjugates—a tutorial review. Molecules 26, 2943. doi:10.3390/molecules26102943
Baghban, R., Roshangar, L., Jahanban-Esfahlan, R., Seidi, K., Ebrahimi-Kalan, A., Jaymand, M., et al. (2020). Tumor microenvironment complexity and therapeutic implications at a glance. Cell Commun. Signal. 18, 59. doi:10.1186/s12964-020-0530-4
Balendiran, G. K., Dabur, R., and Fraser, D. (2004). The role of glutathione in cancer. Cell Biochem. Funct. 22, 343–352. doi:10.1002/cbf.1149
Barbero, L. M., Ianni, A. D., Molinaro, F., Cowan, K. J., and Sirtori, F. R. (2023). Hybrid liquid chromatography high resolution and accuracy mass spectrometry approach for quantification of antibody-drug conjugates at the intact protein level in biological samples. Eur. J. Pharm. Sci. 188, 106502. doi:10.1016/j.ejps.2023.106502
Bardia, A., Hurvitz, S. A., Tolaney, S. M., Loirat, D., Punie, K., Oliveira, M., et al. (2021). Sacituzumab govitecan in metastatic triple-negative breast cancer. N. Engl. J. Med. 384, 1529–1541. doi:10.1056/NEJMoa2028485
Bargh, J. D., Isidro-Llobet, A., Parker, J. S., and Spring, D. R. (2019). Cleavable linkers in antibody-drug conjugates. Chem. Soc. Rev. 48, 4361–4374. doi:10.1039/c8cs00676h
Barok, M., Joensuu, H., and Isola, J. (2014). Trastuzumab emtansine: mechanisms of action and drug resistance. Breast Cancer Res. 16, 209. doi:10.1186/bcr3621
Beck, A., D’Atri, V., Ehkirch, A., Fekete, S., Hernandez-Alba, O., Gahoual, R., et al. (2019). Cutting-edge multi-level analytical and structural characterization of antibody-drug conjugates: present and future. Expert Rev. Proteomics 16, 337–362. doi:10.1080/14789450.2019.1578215
Beck, A., Goetsch, L., Dumontet, C., and Corvaïa, N. (2017). Strategies and challenges for the next generation of antibody-drug conjugates. Nat. Rev. Drug Discov. 16, 315–337. doi:10.1038/nrd.2016.268
Becker, C. L., Duffy, R. J., Gandarilla, J., and Richter, S. M. (2020). Purification of ADCs by hydrophobic interaction chromatography. Methods Mol. Biol. 2078, 273–290. doi:10.1007/978-1-4939-9929-3_19
Blackwell, K. L., Miles, D., Gianni, L., Krop, I. E., Welslau, M., Baselga, J., et al. (2012). Primary results from EMILIA, a phase III study of trastuzumab emtansine (T-DM1) versus capecitabine (X) and lapatinib (L) in HER2-positive locally advanced or metastatic breast cancer (MBC) previously treated with trastuzumab (T) and a taxane. JCO 30, LBA1. doi:10.1200/jco.2012.30.18_suppl.lba1
Bornstein, G. G. (2015). Antibody drug conjugates: Preclinical considerations. AAPS J. 17, 525–534. doi:10.1208/s12248-015-9738-4
Bouwer, N. I., Jager, A., Liesting, C., Kofflard, M. J. M., Brugts, J. J., Kitzen, J. J. E. M., et al. (2020). Cardiac monitoring in HER2-positive patients on trastuzumab treatment: A review and implications for clinical practice. Breast 52, 33–44. doi:10.1016/j.breast.2020.04.005
Brinkmann, U., and Kontermann, R. E. (2017). The making of bispecific antibodies. MAbs 9, 182–212. doi:10.1080/19420862.2016.1268307
Bulger, P. G., Conlon, D. A., Cink, R. D., Fernandez-Cerezo, L., Zhang, Q., Thirumalairajan, S., et al. (2023). Drug-linkers in antibody–drug conjugates: Perspective on current industry Practices. Org. Process Res. Dev. 27, 1248–1257. doi:10.1021/acs.oprd.3c00136
Cao, M., De Mel, N., Jiao, Y., Howard, J., Parthemore, C., Korman, S., et al. (2019). Site-specific antibody-drug conjugate heterogeneity characterization and heterogeneity root cause analysis. MAbs 11, 1064–1076. doi:10.1080/19420862.2019.1624127
Cardoso, F., Paluch-Shimon, S., Senkus, E., Curigliano, G., Aapro, M. S., André, F., et al. (2020). 5th ESO-ESMO international consensus guidelines for advanced breast cancer (ABC 5). Ann. Oncol. 31, 1623–1649. doi:10.1016/j.annonc.2020.09.010
Carter, P. J., and Senter, P. D. (2008). Antibody-drug conjugates for cancer therapy. Cancer J. 14, 154–169. doi:10.1097/PPO.0b013e318172d704
Casi, G., and Neri, D. (2012). Antibody-drug conjugates: basic concepts, examples and future perspectives. J. Control Release 161, 422–428. doi:10.1016/j.jconrel.2012.01.026
Castelli, M. S., McGonigle, P., and Hornby, P. J. (2019). The pharmacology and therapeutic applications of monoclonal antibodies. Pharmacol. Res. Perspect. 7, e00535. doi:10.1002/prp2.535
Ceci, C., Lacal, P. M., and Graziani, G. (2022). Antibody-drug conjugates: Resurgent anticancer agents with multi-targeted therapeutic potential. Pharmacol. Ther. 236, 108106. doi:10.1016/j.pharmthera.2021.108106
Chames, P., Van Regenmortel, M., Weiss, E., and Baty, D. (2009). Therapeutic antibodies: successes, limitations and hopes for the future. Br. J. Pharmacol. 157, 220–233. doi:10.1111/j.1476-5381.2009.00190.x
Chang, E., Weinstock, C., Zhang, L., Charlab, R., Dorff, S. E., Gong, Y., et al. (2021). FDA approval summary: Enfortumab vedotin for locally advanced or metastatic urothelial carcinoma. Clin. Cancer Res. 27, 922–927. doi:10.1158/1078-0432.CCR-20-2275
Chari, R. V. J. (2008). Targeted cancer therapy: conferring specificity to cytotoxic drugs. Acc. Chem. Res. 41, 98–107. doi:10.1021/ar700108g
Chen, R., Hou, J., Newman, E., Kim, Y., Donohue, C., Liu, X., et al. (2015). CD30 downregulation, MMAE resistance, and MDR1 upregulation are all associated with resistance to brentuximab vedotin. Mol. Cancer Ther. 14, 1376–1384. doi:10.1158/1535-7163.MCT-15-0036
Chen, T.-H., Yang, Y., Zhang, Z., Fu, C., Zhang, Q., Williams, J. D., et al. (2019). Native reversed-phase liquid chromatography: A technique for lcms of intact antibody-drug conjugates. Anal. Chem. 91, 2805–2812. doi:10.1021/acs.analchem.8b04699
Chen, T., Chen, Y., Stella, C., Medley, C. D., Gruenhagen, J. A., and Zhang, K. (2016). Antibody-drug conjugate characterization by chromatographic and electrophoretic techniques. J. Chromatogr. B 1032, 39–50. doi:10.1016/j.jchromb.2016.07.023
Chen, W., Yuan, Y., and Jiang, X. (2020). Antibody and antibody fragments for cancer immunotherapy. J. Control Release 328, 395–406. doi:10.1016/j.jconrel.2020.08.021
Cheung-Ong, K., Giaever, G., and Nislow, C. (2013). DNA-Damaging agents in cancer chemotherapy: serendipity and chemical biology. Chem. Biol. 20, 648–659. doi:10.1016/j.chembiol.2013.04.007
Cilliers, C., Menezes, B., Nessler, I., Linderman, J., and Thurber, G. M. (2018). Improved tumor penetration and single-cell targeting of antibody drug conjugates increases anticancer efficacy and host survival. Cancer Res. 78, 758–768. doi:10.1158/0008-5472.CAN-17-1638
Coats, S., Williams, M., Kebble, B., Dixit, R., Tseng, L., Yao, N.-S., et al. (2019). Antibody-drug conjugates: Future directions in clinical and translational strategies to improve the therapeutic index. Clin. Cancer Res. 25, 5441–5448. doi:10.1158/1078-0432.CCR-19-0272
Collins, D. M., Bossenmaier, B., Kollmorgen, G., and Niederfellner, G. (2019). Acquired resistance to antibody-drug conjugates. Cancers (Basel) 11, 394. doi:10.3390/cancers11030394
Cooper, G. M. (2000). “The development and causes of cancer,” in The cell: A molecular approach. 2nd edition (Sunderland: Sinauer Associates). Available at: https://www.ncbi.nlm.nih.gov/books/NBK9963/ (Accessed July 12, 2023).
Cortés, J., Kim, S.-B., Chung, W.-P., Im, S.-A., Park, Y. H., Hegg, R., et al. (2021). LBA1 Trastuzumab deruxtecan (T-DXd) vs trastuzumab emtansine (T-DM1) in patients (Pts) with HER2+ metastatic breast cancer (mBC): Results of the randomized phase III DESTINY-Breast03 study. Ann. Oncol. 32, S1287–S1288. doi:10.1016/j.annonc.2021.08.2087
Cortés, J., Kim, S.-B., Chung, W.-P., Im, S.-A., Park, Y. H., Hegg, R., et al. (2022). Trastuzumab deruxtecan versus trastuzumab emtansine for breast cancer. N. Engl. J. Med. 386, 1143–1154. doi:10.1056/NEJMoa2115022
Dahl, J., Marx, K., and Jabbour, E. (2016). Inotuzumab ozogamicin in the treatment of acute lymphoblastic leukemia. Expert Rev. Hematol. 9, 329–334. doi:10.1586/17474086.2016.1143771
Dahlgren, D., and Lennernäs, H. (2020). Antibody-drug conjugates and targeted treatment strategies for hepatocellular carcinoma: A drug-delivery perspective. Molecules 25, 2861. doi:10.3390/molecules25122861
Damelin, M., Zhong, W., Myers, J., and Sapra, P. (2015). Evolving strategies for target selection for antibody-drug conjugates. Pharm. Res. 32, 3494–3507. doi:10.1007/s11095-015-1624-3
Dan, N., Setua, S., Kashyap, V. K., Khan, S., Jaggi, M., Yallapu, M. M., et al. (2018). Antibody-drug conjugates for cancer therapy: Chemistry to clinical implications. Pharm. (Basel) 11, 32. doi:10.3390/ph11020032
D’Atri, V., Pell, R., Clarke, A., Guillarme, D., and Fekete, S. (2019). Is hydrophobic interaction chromatography the most suitable technique to characterize site-specific antibody-drug conjugates? J. Chromatogr. A 1586, 149–153. doi:10.1016/j.chroma.2018.12.020
de Mel, N., Mulagapati, S. H. R., Cao, M., and Liu, D. (2019). A method to directly analyze free-drug–related species in antibody-drug conjugates without sample preparation. J. Chromatogr. B 1116, 51–59. doi:10.1016/j.jchromb.2019.04.012
Dean, A. Q., Luo, S., Twomey, J. D., and Zhang, B. (2021). Targeting cancer with antibody-drug conjugates: Promises and challenges. MAbs 13, 1951427. doi:10.1080/19420862.2021.1951427
Debaene, F., Boeuf, A., Wagner-Rousset, E., Colas, O., Ayoub, D., Corvaïa, N., et al. (2014). Innovative native MS methodologies for antibody drug conjugate characterization: High resolution native MS and IM-MS for average DAR and DAR distribution assessment. Anal. Chem. 86, 10674–10683. doi:10.1021/ac502593n
Deeks, E. D. (2021). Disitamab vedotin: First approval. Drugs 81, 1929–1935. doi:10.1007/s40265-021-01614-x
Deeks, E. D. (2019). Polatuzumab vedotin: First global approval. Drugs 79, 1467–1475. doi:10.1007/s40265-019-01175-0
Del Prete, A., Salvi, V., Soriani, A., Laffranchi, M., Sozio, F., Bosisio, D., et al. (2023). Dendritic cell subsets in cancer immunity and tumor antigen sensing. Cell Mol. Immunol. 20, 432–447. doi:10.1038/s41423-023-00990-6
Dennis, M. S., Jin, H., Dugger, D., Yang, R., McFarland, L., Ogasawara, A., et al. (2007). Imaging tumors with an albumin-binding Fab, a novel tumor-targeting agent. Cancer Res. 67, 254–261. doi:10.1158/0008-5472.CAN-06-2531
Deonarain, M. P., and Xue, Q. (2020). Tackling solid tumour therapy with small-format drug conjugates. Antib. Ther. 3, 237–245. doi:10.1093/abt/tbaa024
Deonarain, M. P., Yahioglu, G., Stamati, I., Pomowski, A., Clarke, J., Edwards, B. M., et al. (2018). Small-format drug conjugates: A viable alternative to ADCs for solid tumours? Antibodies (Basel) 7, 16. doi:10.3390/antib7020016
Diéras, V., Miles, D., Verma, S., Pegram, M., Welslau, M., Baselga, J., et al. (2017). Trastuzumab emtansine versus capecitabine plus lapatinib in patients with previously treated HER2-positive advanced breast cancer (EMILIA): a descriptive analysis of final overall survival results from a randomised, open-label, phase 3 trial. Lancet Oncol. 18, 732–742. doi:10.1016/S1470-2045(17)30312-1
Dilawari, A., Shah, M., Ison, G., Gittleman, H., Fiero, M. H., Shah, A., et al. (2023). FDA approval summary: Mirvetuximab soravtansine-gynx for FRα-positive, platinum-resistant ovarian cancer. Clin. Cancer Res. 22, OF1–OF6. CCR-23-0991. doi:10.1158/1078-0432.CCR-23-0991
Domínguez-Llamas, S., Caro-Magdaleno, M., Mataix-Albert, B., Avilés-Prieto, J., Romero-Barranca, I., and Rodríguez-de-la-Rúa, E. (2023). Adverse events of antibody–drug conjugates on the ocular surface in cancer therapy. Clin. Transl. Oncol. 2023, 03261. doi:10.1007/s12094-023-03261-y
Donaghy, H. (2016). Effects of antibody, drug and linker on the preclinical and clinical toxicities of antibody-drug conjugates. MAbs 8, 659–671. doi:10.1080/19420862.2016.1156829
Drago, J. Z., Modi, S., and Chandarlapaty, S. (2021). Unlocking the potential of antibody–drug conjugates for cancer therapy. Nat. Rev. Clin. Oncol. 18, 327–344. doi:10.1038/s41571-021-00470-8
Ducry, L. (2012). Challenges in the development and manufacturing of antibody-drug conjugates. Methods Mol. Biol. 899, 489–497. doi:10.1007/978-1-61779-921-1_29
Dumontet, C., Reichert, J. M., Senter, P. D., Lambert, J. M., and Beck, A. (2023). Antibody–drug conjugates come of age in oncology. Nat. Rev. Drug Discov. 22, 641–661. doi:10.1038/s41573-023-00709-2
Edwards, E., Livanos, M., Krueger, A., Dell, A., Haslam, S. M., Mark Smales, C., et al. (2022). Strategies to control therapeutic antibody glycosylation during bioprocessing: Synthesis and separation. Biotechnol. Bioeng. 119, 1343–1358. doi:10.1002/bit.28066
Emens, L. A., Esteva, F. J., Beresford, M., Saura, C., Laurentiis, M. D., Kim, S.-B., et al. (2020). Trastuzumab emtansine plus atezolizumab versus trastuzumab emtansine plus placebo in previously treated, HER2-positive advanced breast cancer (KATE2): a phase 2, multicentre, randomised, double-blind trial. Lancet Oncol. 21, 1283–1295. doi:10.1016/S1470-2045(20)30465-4
Estrela, J. M., Ortega, A., and Obrador, E. (2006). Glutathione in cancer biology and therapy. Crit. Rev. Clin. Lab. Sci. 43, 143–181. doi:10.1080/10408360500523878
Fleming, R. (2020). ADC analysis by hydrophobic interaction chromatography. Methods Mol. Biol. 2078, 147–161. doi:10.1007/978-1-4939-9929-3_10
Ferl, G. Z., Kenanova, V., Wu, A. M., and DiStefano, J. J. (2006). A two-tiered physiologically based model for dually labeled single-chain Fv-Fc antibody fragments. Mol. Cancer Ther. 5, 1550–1558. doi:10.1158/1535-7163.MCT-06-0072
Ferraro, E., Drago, J. Z., and Modi, S. (2021). Implementing antibody-drug conjugates (ADCs) in HER2-positive breast cancer: state of the art and future directions. Breast Cancer Res. 23, 84. doi:10.1186/s13058-021-01459-y
Flynn, M. J., Zammarchi, F., Tyrer, P. C., Akarca, A. U., Janghra, N., Britten, C. E., et al. (2016). ADCT-301, a pyrrolobenzodiazepine (PBD) dimer-containing antibody-drug conjugate (ADC) targeting CD25-expressing hematological malignancies. Mol. Cancer Ther. 15, 2709–2721. doi:10.1158/1535-7163.MCT-16-0233
Ford, C. H., Newman, C. E., Johnson, J. R., Woodhouse, C. S., Reeder, T. A., Rowland, G. F., et al. (1983). Localisation and toxicity study of a vindesine-anti-CEA conjugate in patients with advanced cancer. Br. J. Cancer 47, 35–42. doi:10.1038/bjc.1983.4
Francisco, J. A., Cerveny, C. G., Meyer, D. L., Mixan, B. J., Klussman, K., Chace, D. F., et al. (2003). cAC10-vcMMAE, an anti-CD30-monomethyl auristatin E conjugate with potent and selective antitumor activity. Blood 102, 1458–1465. doi:10.1182/blood-2003-01-0039
Fu, Y., and Ho, M. (2018). DNA damaging agent-based antibody-drug conjugates for cancer therapy. Antib. Ther. 1, 33–43. doi:10.1093/abt/tby007
Fu, Z., Li, S., Han, S., Shi, C., and Zhang, Y. (2022). Antibody drug conjugate: the “biological missile” for targeted cancer therapy. Sig Transduct. Target Ther. 7, 93. doi:10.1038/s41392-022-00947-7
Fuentes-Antrás, J., Genta, S., Vijenthira, A., and Siu, L. L. (2023). Antibody–drug conjugates: in search of partners of choice. Trends Cancer 9, 339–354. doi:10.1016/j.trecan.2023.01.003
Fukuda, Y., Bustos, M. A., Cho, S.-N., Roszik, J., Ryu, S., Lopez, V. M., et al. (2022). Interplay between soluble CD74 and macrophage-migration inhibitory factor drives tumor growth and influences patient survival in melanoma. Cell Death Dis. 13, 117. doi:10.1038/s41419-022-04552-y
Furlanetto, J., Marmé, F., and Loibl, S. (2022). Sacituzumab govitecan: past, present and future of a new antibody-drug conjugate and future horizon. Future Oncol. 18, 3199–3215. doi:10.2217/fon-2022-0407
Gajewski, T. F., Meng, Y., and Harlin, H. (2006). Immune suppression in the tumor microenvironment. J. Immunother. 29, 233–240. doi:10.1097/01.cji.0000199193.29048.56
García-Alonso, S., Ocaña, A., and Pandiella, A. (2018). Resistance to antibody–drug conjugates. Cancer Res. 78, 2159–2165. doi:10.1158/0008-5472.CAN-17-3671
Garrett, M., Ruiz-Garcia, A., Parivar, K., Hee, B., and Boni, J. (2019). Population pharmacokinetics of inotuzumab ozogamicin in relapsed/refractory acute lymphoblastic leukemia and non-Hodgkin lymphoma. J. Pharmacokinet. Pharmacodyn. 46, 211–222. doi:10.1007/s10928-018-9614-9
Gerber, H.-P., Senter, P. D., and Grewal, I. S. (2009). Antibody drug-conjugates targeting the tumor vasculature: Current and future developments. MAbs 1, 247–253. doi:10.4161/mabs.1.3.8515
Gillard, G. O., Proctor, J. L., Brooks, M. L., Lamothe, T. L., Hyzy, S. L., McDonough, S. M., et al. (2020). A novel targeted approach to achieve immune system reset: CD45-Targeted antibody drug conjugates enable autologous HSCT and ameliorate disease in preclinical autoimmune disease models. Biol. Blood Marrow Transplant. 26, S307–S308. doi:10.1016/j.bbmt.2019.12.407
Godwin, C. D., Bates, O. M., Jean, S. R., Laszlo, G. S., Garling, E. E., Beddoe, M. E., et al. (2020). Anti-apoptotic BCL-2 family proteins confer resistance to calicheamicin-based antibody-drug conjugate therapy of acute leukemia. Leuk. Lymphoma 61, 2990–2994. doi:10.1080/10428194.2020.1786553
Gogesch, P., Dudek, S., van Zandbergen, G., Waibler, Z., and Anzaghe, M. (2021). The role of Fc receptors on the effectiveness of therapeutic monoclonal antibodies. Int. J. Mol. Sci. 22, 8947. doi:10.3390/ijms22168947
Goldenberg, M. M. (1999). Trastuzumab, a recombinant DNA-derived humanized monoclonal antibody, a novel agent for the treatment of metastatic breast cancer. Clin. Ther. 21, 309–318. doi:10.1016/S0149-2918(00)88288-0
Gomes-da-Silva, L. C., Kepp, O., and Kroemer, G. (2020). Regulatory approval of photoimmunotherapy: photodynamic therapy that induces immunogenic cell death. Oncoimmunology 9, 1841393. doi:10.1080/2162402X.2020.1841393
Gondi, C. S., and Rao, J. S. (2013). Cathepsin B as a cancer target. Expert Opin. Ther. Targets 17, 281–291. doi:10.1517/14728222.2013.740461
Goodson, H. V., and Jonasson, E. M. (2018). Microtubules and microtubule-associated proteins. Cold Spring Harb. Perspect. Biol. 10, a022608. doi:10.1101/cshperspect.a022608
Greenblatt, K., and Khaddour, K. (2023). Trastuzumab, in StatPearls (treasure island (FL): StatPearls publishing). Available at: http://www.ncbi.nlm.nih.gov/books/NBK532246/ [Accessed August 29, 2023].
Hackshaw, M. D., Danysh, H. E., Singh, J., Ritchey, M. E., Ladner, A., Taitt, C., et al. (2020). Incidence of pneumonitis/interstitial lung disease induced by HER2-targeting therapy for HER2-positive metastatic breast cancer. Breast Cancer Res. Treat. 183, 23–39. doi:10.1007/s10549-020-05754-8
Hamadani, M., Collins, G. P., Caimi, P. F., Samaniego, F., Spira, A., Davies, A., et al. (2021). Camidanlumab tesirine in patients with relapsed or refractory lymphoma: a phase 1, open-label, multicentre, dose-escalation, dose-expansion study. Lancet Haematol. 8, e433–e445. doi:10.1016/S2352-3026(21)00103-4
Hamblett, K. J., Senter, P. D., Chace, D. F., Sun, M. M. C., Lenox, J., Cerveny, C. G., et al. (2004). Effects of drug loading on the antitumor activity of a monoclonal antibody drug conjugate. Clin. Cancer Res. 10, 7063–7070. doi:10.1158/1078-0432.CCR-04-0789
Hartley, J. A. (2011). The development of pyrrolobenzodiazepines as antitumour agents. Expert Opin. Investig. Drugs 20, 733–744. doi:10.1517/13543784.2011.573477
Herbener, P., Schönfeld, K., König, M., Germer, M., Przyborski, J. M., Bernöster, K., et al. (2018). Functional relevance of in vivo half antibody exchange of an IgG4 therapeutic antibody-drug conjugate. PLoS One 13, e0195823. doi:10.1371/journal.pone.0195823
Heitz, N., Greer, S. C., and Halford, Z. (2023). A review of Tisotumab vedotin-tftv in recurrent or metastatic cervical cancer. Ann. Pharmacother. 57, 585–596. doi:10.1177/10600280221118370
Heo, Y.-A. (2023). Mirvetuximab soravtansine: First approval. Drugs 83, 265–273. doi:10.1007/s40265-023-01834-3
Herrera, A. F., Zain, J., Savage, K. J., Feldman, T. A., Brammer, J. E., Chen, L., et al. (2021). Brentuximab vedotin plus cyclophosphamide, doxorubicin, etoposide, and prednisone (CHEP-BV) followed by BV consolidation in patients with CD30-expressing peripheral T-cell lymphomas. Blood 138, 133. doi:10.1182/blood-2021-151105
Hoffmann, R. M., Coumbe, B. G. T., Josephs, D. H., Mele, S., Ilieva, K. M., Cheung, A., et al. (2018). Antibody structure and engineering considerations for the design and function of Antibody Drug Conjugates (ADCs). Oncoimmunology 7, e1395127. doi:10.1080/2162402X.2017.1395127
Hughes, B. (2010). Antibody-drug conjugates for cancer: poised to deliver? Nat. Rev. Drug Discov. 9, 665–667. doi:10.1038/nrd3270
Hurwitz, J., Haggstrom, L. R., and Lim, E. (2023). Antibody–drug conjugates: Ushering in a new era of cancer therapy. Pharmaceutics 15, 2017. doi:10.3390/pharmaceutics15082017
Hutchinson, M. H., Hendricks, R. S., Lin, X. X., and Olson, D. A. (2018). “Chapter 40 - process development and manufacturing of antibody-drug conjugates,” in Biopharmaceutical processing. Editors G. Jagschies, E. Lindskog, K. Łącki, and P. Galliher (Amsterdam: Elsevier), 813–836. doi:10.1016/B978-0-08-100623-8.00041-4
Iqbal, N., and Iqbal, N. (2014). Human epidermal growth factor receptor 2 (HER2) in cancers: Overexpression and therapeutic implications. Mol. Biol. Int. 2014, 852748. doi:10.1155/2014/852748
Jain, N., Smith, S. W., Ghone, S., and Tomczuk, B. (2015). Current ADC linker chemistry. Pharm. Res. 32, 3526–3540. doi:10.1007/s11095-015-1657-7
Jain, R. K. (2001). Delivery of molecular and cellular medicine to solid tumors. Adv. Drug Deliv. Rev. 46, 149–168. doi:10.1016/s0169-409x(00)00131-9
Jain, R. K. (2005). Normalization of tumor vasculature: an emerging concept in antiangiogenic therapy. Science 307, 58–62. doi:10.1126/science.1104819
Jiang, Q., Patel, B., Jin, X., Di Grandi, D., Bortell, E., Czapkowski, B., et al. (2019). Structural characterization of the aggregates of gemtuzumab ozogamicin. ACS Omega 4, 6468–6475. doi:10.1021/acsomega.8b03627
Joubert, N., Beck, A., Dumontet, C., and Denevault-Sabourin, C. (2020). Antibody–drug conjugates: The last decade. Pharmaceuticals 13, 245. doi:10.3390/ph13090245
Junttila, T. T., Li, G., Parsons, K., Phillips, G. L., and Sliwkowski, M. X. (2011). Trastuzumab-DM1 (T-DM1) retains all the mechanisms of action of trastuzumab and efficiently inhibits growth of lapatinib insensitive breast cancer. Breast Cancer Res. Treat. 128, 347–356. doi:10.1007/s10549-010-1090-x
Junutula, J. R., Raab, H., Clark, S., Bhakta, S., Leipold, D. D., Weir, S., et al. (2008). Site-specific conjugation of a cytotoxic drug to an antibody improves the therapeutic index. Nat. Biotechnol. 26, 925–932. doi:10.1038/nbt.1480
Kang, C. (2021). Moxetumomab pasudotox in hairy cell leukaemia: A profile of its use. Clin. Drug Investig. 41, 829–834. doi:10.1007/s40261-021-01069-8
Kaplon, H., Crescioli, S., Chenoweth, A., Visweswaraiah, J., and Reichert, J. M. (2023). Antibodies to watch in 2023. MAbs 15, 2153410. doi:10.1080/19420862.2022.2153410
Kaplon, H., Muralidharan, M., Schneider, Z., and Reichert, J. M. (2020). Antibodies to watch in 2020. MAbs 12, 1703531. doi:10.1080/19420862.2019.1703531
Katz, J., Janik, J. E., and Younes, A. (2011). Brentuximab vedotin (SGN-35). Clin. Cancer Res. 17, 6428–6436. doi:10.1158/1078-0432.CCR-11-0488
Kayser, S., and Levis, M. J. (2022). Updates on targeted therapies for acute myeloid leukaemia. Br. J. Haematol. 196, 316–328. doi:10.1111/bjh.17746
Kholodenko, R. V., Kalinovsky, D. V., Doronin, I. I., Ponomarev, E. D., and Kholodenko, I. V. (2019). Antibody fragments as potential biopharmaceuticals for cancer therapy: Success and limitations. CMC 26, 396–426. doi:10.2174/0929867324666170817152554
Khongorzul, P., Ling, C. J., Khan, F. U., Ihsan, A. U., and Zhang, J. (2020). Antibody–drug conjugates: A comprehensive review. Mol. Cancer Res. 18, 3–19. doi:10.1158/1541-7786.MCR-19-0582
Kim, E. G., and Kim, K. M. (2015). Strategies and advancement in antibody-drug conjugate optimization for targeted cancer therapeutics. Biomol. Ther. Seoul. 23, 493–509. doi:10.4062/biomolther.2015.116
Kimiz-Gebologlu, I., Gulce-Iz, S., and Biray-Avci, C. (2018). Monoclonal antibodies in cancer immunotherapy. Mol. Biol. Rep. 45, 2935–2940. doi:10.1007/s11033-018-4427-x
Kitamura, N., Sento, S., Yoshizawa, Y., Sasabe, E., Kudo, Y., and Yamamoto, T. (2020). Current trends and future prospects of molecular targeted therapy in head and neck squamous cell carcinoma. Int. J. Mol. Sci. 22, 240. doi:10.3390/ijms22010240
Kostova, V., Désos, P., Starck, J.-B., and Kotschy, A. (2021). The chemistry behind ADCs. Pharm. (Basel) 14, 442. doi:10.3390/ph14050442
Kreitman, R. J. (2019). Hairy cell leukemia: present and future directions. Leuk. Lymphoma 60, 2869–2879. doi:10.1080/10428194.2019.1608536
Kreitman, R. J., and Pastan, I. (2011). Antibody fusion proteins: anti-CD22 recombinant immunotoxin moxetumomab pasudotox. Clin. Cancer Res. 17, 6398–6405. doi:10.1158/1078-0432.CCR-11-0487
Kvirkvelia, N., McMenamin, M., Gutierrez, V. I., Lasareishvili, B., and Madaio, M. P. (2015). Human anti-α3(IV)NC1 antibody drug conjugates target glomeruli to resolve nephritis. Am. J. Physiol. Ren. Physiol. 309, F680–F684. doi:10.1152/ajprenal.00289.2015
Labani-Motlagh, A., Ashja-Mahdavi, M., and Loskog, A. (2020). The tumor microenvironment: A milieu hindering and obstructing antitumor immune responses. Front. Immunol. 11, 940. doi:10.3389/fimmu.2020.00940
Lambert, J. M., and Chari, R. V. J. (2014). Ado-trastuzumab emtansine (T-DM1): an antibody-drug conjugate (ADC) for HER2-positive breast cancer. J. Med. Chem. 57, 6949–6964. doi:10.1021/jm500766w
Lambert, J. M., and Morris, C. Q. (2017). Antibody–drug conjugates (ADCs) for personalized treatment of solid tumors: A review. Adv. Ther. 34, 1015–1035. doi:10.1007/s12325-017-0519-6
Lanza, F., Maffini, E., Rondoni, M., Massari, E., Faini, A. C., and Malavasi, F. (2020). CD22 expression in B-cell acute lymphoblastic leukemia: Biological significance and implications for inotuzumab therapy in adults. Cancers (Basel) 12, 303. doi:10.3390/cancers12020303
Le, Q., Tang, T., Leonti, A., Castro, S., McKay, C. N., Perkins, L., et al. (2023). Preclinical studies targeting CD74 with STRO-001 antibody-drug conjugate in acute leukemia. Blood Adv. 7, 1666–1670. doi:10.1182/bloodadvances.2022008303
Lee, A. (2021). Loncastuximab tesirine: First approval. Drugs 81, 1229–1233. doi:10.1007/s40265-021-01550-w
Lee, H., Bhang, S. H., Lee, J. H., Kim, H., and Hahn, S. K. (2017). Tocilizumab-alendronate conjugate for treatment of rheumatoid arthritis. Bioconjug Chem. 28, 1084–1092. doi:10.1021/acs.bioconjchem.7b00008
Leget, G. A., and Czuczman, M. S. (1998). Use of rituximab, the new FDA-approved antibody. Curr. Opin. Oncol. 10, 548–551. doi:10.1097/00001622-199811000-00012
Lim, R. K. V., Yu, S., Cheng, B., Li, S., Kim, N.-J., Cao, Y., et al. (2015). Targeted delivery of LXR agonist using a site-specific antibody-drug conjugate. Bioconjug Chem. 26, 2216–2222. doi:10.1021/acs.bioconjchem.5b00203
Lin, J., and Lazar, A. C. (2013). Determination of charge heterogeneity and level of unconjugated antibody by imaged cIEF. Methods Mol. Biol. 1045, 295–302. doi:10.1007/978-1-62703-541-5_19
Lindley, C., McCune, J. S., Thomason, T. E., Lauder, D., Sauls, A., Adkins, S., et al. (1999). Perception of chemotherapy side effects cancer versus noncancer patients. Cancer Pract. 7, 59–65. doi:10.1046/j.1523-5394.1999.07205.x
Liu, L., and Chen, J. (2022). Therapeutic antibodies for precise cancer immunotherapy: current and future perspectives. Med. Rev. 2, 555–569. doi:10.1515/mr-2022-0033
Liu, Y., Jiang, P., Capkova, K., Xue, D., Ye, L., Sinha, S. C., et al. (2011). Tissue factor-activated coagulation cascade in the tumor microenvironment is critical for tumor progression and an effective target for therapy. Cancer Res. 71, 6492–6502. doi:10.1158/0008-5472.CAN-11-1145
Loganzo, F., Sung, M., and Gerber, H.-P. (2016). Mechanisms of resistance to antibody–drug conjugates. Mol. Cancer Ther. 15, 2825–2834. doi:10.1158/1535-7163.MCT-16-0408
Loganzo, F., Tan, X., Sung, M., Jin, G., Myers, J. S., Melamud, E., et al. (2015). Tumor cells chronically treated with a trastuzumab-maytansinoid antibody-drug conjugate develop varied resistance mechanisms but respond to alternate treatments. Mol. Cancer Ther. 14, 952–963. doi:10.1158/1535-7163.MCT-14-0862
Lu, J., Jiang, F., Lu, A., and Zhang, G. (2016). Linkers having a crucial role in antibody-drug conjugates. Int. J. Mol. Sci. 17, 561. doi:10.3390/ijms17040561
Lucas, A. T., Price, L. S. L., Schorzman, A. N., Storrie, M., Piscitelli, J. A., Razo, J., et al. (2018). Factors affecting the pharmacology of antibody-drug conjugates. Antibodies (Basel) 7, 10. doi:10.3390/antib7010010
Luo, R., Liu, H., and Cheng, Z. (2022). Protein scaffolds: antibody alternatives for cancer diagnosis and therapy. RSC Chem. Biol. 3, 830–847. doi:10.1039/D2CB00094F
Ma, Z., Wang, H., Cai, Y., Wang, H., Niu, K., Wu, X., et al. (2018). Epigenetic drift of H3K27me3 in aging links glycolysis to healthy longevity in Drosophila. eLife 7, e35368. doi:10.7554/eLife.35368
Macor, P., Capolla, S., and Tedesco, F. (2018). Complement as a biological tool to control tumor growth. Front. Immunol. 9, 2203. doi:10.3389/fimmu.2018.02203
Macor, P., Mezzanzanica, D., Cossetti, C., Alberti, P., Figini, M., Canevari, S., et al. (2006). Complement activated by chimeric anti-folate receptor antibodies is an efficient effector system to control ovarian carcinoma. Cancer Res. 66, 3876–3883. doi:10.1158/0008-5472.CAN-05-3434
Macor, P., and Tedesco, F. (2007). Complement as effector system in cancer immunotherapy. Immunol. Lett. 111, 6–13. doi:10.1016/j.imlet.2007.04.014
Mahadevan, D., and Von Hoff, D. D. (2007). Tumor-stroma interactions in pancreatic ductal adenocarcinoma. Mol. Cancer Ther. 6, 1186–1197. doi:10.1158/1535-7163.MCT-06-0686
Mahalingaiah, P. K., Ciurlionis, R., Durbin, K. R., Yeager, R. L., Philip, B. K., Bawa, B., et al. (2019). Potential mechanisms of target-independent uptake and toxicity of antibody-drug conjugates. Pharmacol. Ther. 200, 110–125. doi:10.1016/j.pharmthera.2019.04.008
Mahmood, I. (2021). Clinical pharmacology of antibody-drug conjugates. Antibodies (Basel) 10, 20. doi:10.3390/antib10020020
Malhotra, V., and Perry, M. C. (2003). Classical chemotherapy: mechanisms, toxicities and the therapeutic window. Cancer Biol. Ther. 2, S2–S4.
Mamounas, E. P., Untch, M., Mano, M. S., Huang, C.-S., Geyer, C. E., von Minckwitz, G., et al. (2021). Adjuvant T-DM1 versus trastuzumab in patients with residual invasive disease after neoadjuvant therapy for HER2-positive breast cancer: subgroup analyses from KATHERINE. Ann. Oncol. 32, 1005–1014. doi:10.1016/j.annonc.2021.04.011
Marei, H. E., Cenciarelli, C., and Hasan, A. (2022). Potential of antibody–drug conjugates (ADCs) for cancer therapy. Cancer Cell Int. 22, 255. doi:10.1186/s12935-022-02679-8
Masters, J. C., Nickens, D. J., Xuan, D., Shazer, R. L., and Amantea, M. (2018). Clinical toxicity of antibody drug conjugates: a meta-analysis of payloads. Invest. New Drugs 36, 121–135. doi:10.1007/s10637-017-0520-6
Matsumoto, T., Jimi, S., Hara, S., Takamatsu, Y., Suzumiya, J., and Tamura, K. (2012). Importance of inducible multidrug resistance 1 expression in HL-60 cells resistant to gemtuzumab ozogamicin. Leuk. Lymphoma 53, 1399–1405. doi:10.3109/10428194.2012.656102
Mckertish, C. M., and Kayser, V. (2021). Advances and limitations of antibody drug conjugates for cancer. Biomedicines 9, 872. doi:10.3390/biomedicines9080872
Mecklenburg, L. (2018). A brief introduction to antibody-drug conjugates for toxicologic pathologists. Toxicol. Pathol. 46, 746–752. doi:10.1177/0192623318803059
Mehanna, J., Haddad, F. G., Eid, R., Lambertini, M., and Kourie, H. R. (2019). Triple-negative breast cancer: current perspective on the evolving therapeutic landscape. Int. J. Womens Health 11, 431–437. doi:10.2147/IJWH.S178349
Modi, S., Jacot, W., Yamashita, T., Sohn, J., Vidal, M., Tokunaga, E., et al. (2022). Trastuzumab deruxtecan in previously treated HER2-low advanced breast cancer. N. Engl. J. Med. 387, 9–20. doi:10.1056/NEJMoa2203690
Modi, S., Saura, C., Yamashita, T., Park, Y. H., Kim, S.-B., Tamura, K., et al. (2021). Abstract PD3-06: Updated results from DESTINY-breast01, a phase 2 trial of trastuzumab deruxtecan (T-DXd) in HER2 positive metastatic breast cancer. Cancer Res. 81, PD3–06. doi:10.1158/1538-7445.SABCS20-PD3-06
Modi, S., Saura, C., Yamashita, T., Park, Y. H., Kim, S.-B., Tamura, K., et al. (2020). Trastuzumab deruxtecan in previously treated HER2-positive breast cancer. N. Engl. J. Med. 382, 610–621. doi:10.1056/NEJMoa1914510
Nader-Marta, G., Martins-Branco, D., Agostinetto, E., Bruzzone, M., Ceppi, M., Danielli, L., et al. (2022). Efficacy of tyrosine kinase inhibitors for the treatment of patients with HER2-positive breast cancer with brain metastases: a systematic review and meta-analysis. ESMO Open 7, 100501. doi:10.1016/j.esmoop.2022.100501
Natsume, A., Niwa, R., and Satoh, M. (2009). Improving effector functions of antibodies for cancer treatment: Enhancing ADCC and CDC. Drug Des. Devel Ther. 3, 7–16. doi:10.2147/dddt.s4378
Nejadmoghaddam, M.-R., Minai-Tehrani, A., Ghahremanzadeh, R., Mahmoudi, M., Dinarvand, R., and Zarnani, A.-H. (2019). Antibody-drug conjugates: Possibilities and challenges. Avicenna J. Med. Biotechnol. 11, 3–23.
Nguyen, T. D., Bordeau, B. M., and Balthasar, J. P. (2023). Mechanisms of ADC toxicity and strategies to increase ADC tolerability. Cancers (Basel) 15, 713. doi:10.3390/cancers15030713
Nicolò, E., Giugliano, F., Ascione, L., Tarantino, P., Corti, C., Tolaney, S. M., et al. (2022). Combining antibody-drug conjugates with immunotherapy in solid tumors: current landscape and future perspectives. Cancer Treat. Rev. 106, 102395. doi:10.1016/j.ctrv.2022.102395
Nolting, B. (2013). Linker technologies for antibody-drug conjugates. Methods Mol. Biol. 1045, 71–100. doi:10.1007/978-1-62703-541-5_5
Norsworthy, K. J., Ko, C.-W., Lee, J. E., Liu, J., John, C. S., Przepiorka, D., et al. (2018). FDA approval summary: Mylotarg for treatment of patients with relapsed or refractory CD33-positive acute myeloid leukemia. Oncologist 23, 1103–1108. doi:10.1634/theoncologist.2017-0604
Ouyang, J. (2013). Drug-to-antibody ratio (DAR) and drug load distribution by hydrophobic interaction chromatography and reversed phase high-performance liquid chromatography. Methods Mol. Biol. 1045, 275–283. doi:10.1007/978-1-62703-541-5_17
O’Leary, M. K., Ahmed, A., and Alabi, C. A. (2023). Development of host-cleavable antibody-bactericide conjugates against extracellular pathogens. ACS Infect. Dis. 9, 322–329. doi:10.1021/acsinfecdis.2c00492
Omura, G., Honma, Y., Matsumoto, Y., Shinozaki, T., Itoyama, M., Eguchi, K., et al. (2023). Transnasal photoimmunotherapy with cetuximab sarotalocan sodium: Outcomes on the local recurrence of nasopharyngeal squamous cell carcinoma. Auris Nasus Larynx 50, 641–645. doi:10.1016/j.anl.2022.06.004
Park, M.-H., Lee, B. I., Byeon, J.-J., Shin, S.-H., Choi, J., Park, Y., et al. (2019). Pharmacokinetic and metabolism studies of monomethyl auristatin F via liquid chromatography-quadrupole-time-of-flight mass spectrometry. Molecules 24, 2754. doi:10.3390/molecules24152754
Perrino, E., Steiner, M., Krall, N., Bernardes, G. J. L., Pretto, F., Casi, G., et al. (2014). Curative properties of noninternalizing antibody-drug conjugates based on maytansinoids. Cancer Res. 74, 2569–2578. doi:10.1158/0008-5472.CAN-13-2990
Peters, C., and Brown, S. (2015). Antibody–drug conjugates as novel anti-cancer chemotherapeutics. Biosci. Rep. 35, e00225. doi:10.1042/BSR20150089
Pettinato, M. C. (2021). Introduction to antibody-drug conjugates. Antibodies 10, 42. doi:10.3390/antib10040042
Ponte, J. F., Ab, O., Lanieri, L., Lee, J., Coccia, J., Bartle, L. M., et al. (2016). Mirvetuximab soravtansine (IMGN853), a folate receptor alpha-targeting antibody-drug conjugate, potentiates the activity of standard of care therapeutics in ovarian cancer models. Neoplasia 18, 775–784. doi:10.1016/j.neo.2016.11.002
Ponziani, S., Di Vittorio, G., Pitari, G., Cimini, A. M., Ardini, M., Gentile, R., et al. (2020). Antibody-drug conjugates: The new frontier of chemotherapy. Int. J. Mol. Sci. 21, 5510. doi:10.3390/ijms21155510
Professional Committee on Clinical Research of Oncology Drugs, Expert Committee for Monitoring the Clinical Application of Antitumor Drugs, Chinese Anti-Cancer Association, and Cancer Chemotherapy Quality Control Expert Committee of Beijing Cancer Treatment Quality Control and Improvement Center (2022). Expert consensus on the clinical application of antibody-drug conjugates in the treatment of malignant tumors (2021 edition). Cancer Innov. 1, 3–24. doi:10.1002/cai2.8
Purcell, J. W., Tanlimco, S. G., Hickson, J., Fox, M., Sho, M., Durkin, L., et al. (2018). LRRC15 is a novel mesenchymal protein and stromal target for antibody–drug conjugates. Cancer Res. 78, 4059–4072. doi:10.1158/0008-5472.CAN-18-0327
Ritchie, M., Tchistiakova, L., and Scott, N. (2013). Implications of receptor-mediated endocytosis and intracellular trafficking dynamics in the development of antibody drug conjugates. MAbs 5, 13–21. doi:10.4161/mabs.22854
Rassy, E., Rached, L., and Pistilli, B. (2022). Antibody drug conjugates targeting HER2: Clinical development in metastatic breast cancer. Breast 66, 217–226. doi:10.1016/j.breast.2022.10.016
Redman, J. M., Hill, E. M., AlDeghaither, D., and Weiner, L. M. (2015). Mechanisms of action of therapeutic antibodies for cancer. Mol. Immunol. 67, 28–45. doi:10.1016/j.molimm.2015.04.002
Richards, D. A. (2018). Exploring alternative antibody scaffolds: Antibody fragments and antibody mimics for targeted drug delivery. Drug Discov. Today Technol. 30, 35–46. doi:10.1016/j.ddtec.2018.10.005
Ríos-Luci, C., García-Alonso, S., Díaz-Rodríguez, E., Nadal-Serrano, M., Arribas, J., Ocaña, A., et al. (2017). Resistance to the antibody-drug conjugate T-DM1 is based in a reduction in lysosomal proteolytic activity. Cancer Res. 77, 4639–4651. doi:10.1158/0008-5472.CAN-16-3127
Sabbaghi, M., Gil-Gómez, G., Guardia, C., Servitja, S., Arpí, O., García-Alonso, S., et al. (2017). Defective cyclin B1 induction in trastuzumab-emtansine (T-DM1) acquired resistance in HER2-positive breast cancer. Clin. Cancer Res. 23, 7006–7019. doi:10.1158/1078-0432.CCR-17-0696
Salem, A., Alotaibi, M., Mroueh, R., Basheer, H. A., and Afarinkia, K. (2021). CCR7 as a therapeutic target in Cancer. Biochim. Biophys. Acta Rev. Cancer 1875, 188499. doi:10.1016/j.bbcan.2020.188499
Samantasinghar, A., Sunildutt, N. P., Ahmed, F., Soomro, A. M., Salih, A. R. C., Parihar, P., et al. (2023). A comprehensive review of key factors affecting the efficacy of antibody drug conjugate. Biomed. Pharmacother. 161, 114408. doi:10.1016/j.biopha.2023.114408
Sau, S., Alsaab, H. O., Kashaw, S. K., Tatiparti, K., and Iyer, A. K. (2017). Advances in antibody-drug conjugates: A new era of targeted cancer therapy. Drug Discov. Today 22, 1547–1556. doi:10.1016/j.drudis.2017.05.011
Schmidhalter, D. R., Elzner, S., and Schmid, R. (2019). “Progress in the development of single-use solutions in antibody–drug conjugate (ADC) manufacturing,” in Single-use technology in biopharmaceutical manufacture (New Jersey: John Wiley & Sons, Ltd), 303–310. doi:10.1002/9781119477891.ch27
Schwartz, R. S. (2004). Paul Ehrlich’s magic bullets. N. Engl. J. Med. 350, 1079–1080. doi:10.1056/NEJMp048021
Scott, L. J. (2017). Brentuximab vedotin: A review in CD30-positive Hodgkin lymphoma. Drugs 77, 435–445. doi:10.1007/s40265-017-0705-5
Seaman, S., Zhu, Z., Saha, S., Zhang, X. M., Yang, M. Y., Hilton, M. B., et al. (2017). Eradication of tumors through simultaneous ablation of CD276/B7-H3-positive tumor cells and tumor vasculature. Cancer Cell 31, 501–515. doi:10.1016/j.ccell.2017.03.005
Seckinger, A., Delgado, J. A., Moser, S., Moreno, L., Neuber, B., Grab, A., et al. (2017). Target expression, generation, preclinical activity, and pharmacokinetics of the BCMA-T cell bispecific antibody EM801 for multiple myeloma treatment. Cancer Cell 31, 396–410. doi:10.1016/j.ccell.2017.02.002
Sehn, L. H., Hertzberg, M., Opat, S., Herrera, A. F., Assouline, S., Flowers, C. R., et al. (2022). Polatuzumab vedotin plus bendamustine and rituximab in relapsed/refractory DLBCL: survival update and new extension cohort data. Blood Adv. 6, 533–543. doi:10.1182/bloodadvances.2021005794
Senapati, S., Mahanta, A. K., Kumar, S., and Maiti, P. (2018). Controlled drug delivery vehicles for cancer treatment and their performance. Signal Transduct. Target Ther. 3, 7. doi:10.1038/s41392-017-0004-3
Senter, P. D. (2009). Potent antibody drug conjugates for cancer therapy. Curr. Opin. Chem. Biol. 13, 235–244. doi:10.1016/j.cbpa.2009.03.023
Senter, P. D., and Sievers, E. L. (2012). The discovery and development of brentuximab vedotin for use in relapsed Hodgkin lymphoma and systemic anaplastic large cell lymphoma. Nat. Biotechnol. 30, 631–637. doi:10.1038/nbt.2289
Shefet-Carasso, L., and Benhar, I. (2015). Antibody-targeted drugs and drug resistance--challenges and solutions. Drug Resist Updat 18, 36–46. doi:10.1016/j.drup.2014.11.001
Shi, F., Liu, Y., Zhou, X., Shen, P., Xue, R., and Zhang, M. (2022). Disitamab vedotin: a novel antibody-drug conjugates for cancer therapy. Drug Deliv. 29, 1335–1344. doi:10.1080/10717544.2022.2069883
Shiravand, Y., Khodadadi, F., Kashani, S. M. A., Hosseini-Fard, S. R., Hosseini, S., Sadeghirad, H., et al. (2022). Immune checkpoint inhibitors in cancer therapy. Curr. Oncol. 29, 3044–3060. doi:10.3390/curroncol29050247
Shitara, K., Baba, E., Fujitani, K., Oki, E., Fujii, S., and Yamaguchi, K. (2021). Discovery and development of trastuzumab deruxtecan and safety management for patients with HER2-positive gastric cancer. Gastric Cancer 24, 780–789. doi:10.1007/s10120-021-01196-3
Some, D., Amartely, H., Tsadok, A., and Lebendiker, M. (2019). Characterization of proteins by size-exclusion chromatography coupled to multi-angle light scattering (SEC-MALS). J. Vis. Exp. 2019, 59615. doi:10.3791/59615
Spiess, C., Bevers, J., Jackman, J., Chiang, N., Nakamura, G., Dillon, M., et al. (2013). Development of a human IgG4 bispecific antibody for dual targeting of interleukin-4 (IL-4) and interleukin-13 (IL-13) cytokines. J. Biol. Chem. 288, 26583–26593. doi:10.1074/jbc.M113.480483
Staudacher, A. H., and Brown, M. P. (2017). Antibody drug conjugates and bystander killing: is antigen-dependent internalisation required? Br. J. Cancer 117, 1736–1742. doi:10.1038/bjc.2017.367
Stapleton, N. M., Andersen, J. T., Stemerding, A. M., Bjarnarson, S. P., Verheul, R. C., Gerritsen, J., et al. (2011). Competition for FcRn-mediated transport gives rise to short half-life of human IgG3 and offers therapeutic potential. Nat. Commun. 2, 599. doi:10.1038/ncomms1608
Strop, P., Delaria, K., Foletti, D., Witt, J. M., Hasa-Moreno, A., Poulsen, K., et al. (2015). Site-specific conjugation improves therapeutic index of antibody drug conjugates with high drug loading. Nat. Biotechnol. 33, 694–696. doi:10.1038/nbt.3274
Su, Z., Xiao, D., Xie, F., Liu, L., Wang, Y., Fan, S., et al. (2021). Antibody–drug conjugates: Recent advances in linker chemistry. Acta Pharm. Sin. B 11, 3889–3907. doi:10.1016/j.apsb.2021.03.042
Sun Kang, M., See Kong, T. W., Xin Khoo, J. Y., and Loh, T.-P. (2021). Recent developments in chemical conjugation strategies targeting native amino acids in proteins and their applications in antibody–drug conjugates. Chem. Sci. 12, 13613–13647. doi:10.1039/D1SC02973H
Sung, H., Ferlay, J., Siegel, R. L., Laversanne, M., Soerjomataram, I., Jemal, A., et al. (2021). Global cancer statistics 2020: GLOBOCAN estimates of incidence and mortality worldwide for 36 cancers in 185 countries. CA Cancer J. Clin. 71, 209–249. doi:10.3322/caac.21660
Szot, C., Saha, S., Zhang, X. M., Zhu, Z., Hilton, M. B., Morris, K., et al. (2018). Tumor stroma–targeted antibody-drug conjugate triggers localized anticancer drug release. J. Clin. Invest. 128, 2927–2943. doi:10.1172/JCI120481
Tai, Y.-T., Mayes, P. A., Acharya, C., Zhong, M. Y., Cea, M., Cagnetta, A., et al. (2014). Novel anti-B-cell maturation antigen antibody-drug conjugate (GSK2857916) selectively induces killing of multiple myeloma. Blood 123, 3128–3138. doi:10.1182/blood-2013-10-535088
Talotta, R., Rucci, F., Canti, G., and Scaglione, F. (2019). Pros and cons of the immunogenicity of monoclonal antibodies in cancer treatment: a lesson from autoimmune diseases. Immunotherapy 11, 241–254. doi:10.2217/imt-2018-0081
Tang, H., Liu, Y., Yu, Z., Sun, M., Lin, L., Liu, W., et al. (2019). The analysis of key factors related to ADCs structural design. Front. Pharmacol. 10, 373. doi:10.3389/fphar.2019.00373
Tarantino, P., Ricciuti, B., Pradhan, S. M., and Tolaney, S. M. (2023). Optimizing the safety of antibody–drug conjugates for patients with solid tumours. Nat. Rev. Clin. Oncol. 20, 558–576. doi:10.1038/s41571-023-00783-w
Thurber, G. M., and Dane Wittrup, K. (2012). A mechanistic compartmental model for total antibody uptake in tumors. J. Theor. Biol. 314, 57–68. doi:10.1016/j.jtbi.2012.08.034
Thurber, G. M., Schmidt, M. M., and Wittrup, K. D. (2008). Antibody tumor penetration: transport opposed by systemic and antigen-mediated clearance. Adv. Drug Deliv. Rev. 60, 1421–1434. doi:10.1016/j.addr.2008.04.012
Tilly, H., Morschhauser, F., Sehn, L. H., Friedberg, J. W., Trněný, M., Sharman, J. P., et al. (2022). Polatuzumab vedotin in previously untreated diffuse large B-cell lymphoma. N. Engl. J. Med. 386, 351–363. doi:10.1056/NEJMoa2115304
Tipton, T. R. W., Roghanian, A., Oldham, R. J., Carter, M. J., Cox, K. L., Mockridge, C. I., et al. (2015). Antigenic modulation limits the effector cell mechanisms employed by type I anti-CD20 monoclonal antibodies. Blood 125, 1901–1909. doi:10.1182/blood-2014-07-588376
Tong, J. T. W., Harris, P. W. R., Brimble, M. A., and Kavianinia, I. (2021). An insight into FDA approved antibody-drug conjugates for cancer therapy. Molecules 26, 5847. doi:10.3390/molecules26195847
Tsuchikama, K., and An, Z. (2018). Antibody-drug conjugates: recent advances in conjugation and linker chemistries. Protein Cell 9, 33–46. doi:10.1007/s13238-016-0323-0
Tvilum, A., Johansen, M. I., Glud, L. N., Ivarsen, D. M., Khamas, A. B., Carmali, S., et al. (2023). Antibody-drug conjugates to treat bacterial biofilms via targeting and extracellular drug release. Adv. Sci. (Weinh) 10, e2301340. doi:10.1002/advs.202301340
van der Velden, V. H. J., Boeckx, N., Jedema, I., te Marvelde, J. G., Hoogeveen, P. G., Boogaerts, M., et al. (2004). High CD33-antigen loads in peripheral blood limit the efficacy of gemtuzumab ozogamicin (Mylotarg) treatment in acute myeloid leukemia patients. Leukemia 18, 983–988. doi:10.1038/sj.leu.2403350
van Dongen, G. A. M. S. (2021). Improving tumor penetration of antibodies and antibody-drug conjugates: Taking away the barriers for trojan horses. Cancer Res. 81, 3956–3957. doi:10.1158/0008-5472.CAN-21-0952
Varma, G., Wang, J., and Diefenbach, C. (2022). Polatuzumab vedotin in relapsed/refractory aggressive B-cell lymphoma. Expert Rev. Anticancer Ther. 22, 795–803. doi:10.1080/14737140.2022.2093191
Verhoef, J. J. F., and Anchordoquy, T. J. (2013). Questioning the use of PEGylation for drug delivery. Drug Deliv. Transl. Res. 3, 499–503. doi:10.1007/s13346-013-0176-5
Vidarsson, G., Dekkers, G., and Rispens, T. (2014). IgG subclasses and allotypes: from structure to effector functions. Front. Immunol. 5, 520. doi:10.3389/fimmu.2014.00520
Visser, K. E. de, and Joyce, J. A. (2023). The evolving tumor microenvironment: From cancer initiation to metastatic outgrowth. Cancer Cell 41, 374–403. doi:10.1016/j.ccell.2023.02.016
von Arx, C., De Placido, P., Caltavituro, A., Di Rienzo, R., Buonaiuto, R., De Laurentiis, M., et al. (2023). The evolving therapeutic landscape of trastuzumab-drug conjugates: Future perspectives beyond HER2-positive breast cancer. Cancer Treat. Rev. 113, 102500. doi:10.1016/j.ctrv.2022.102500
von Minckwitz, G., Huang, C.-S., Mano, M. S., Loibl, S., Mamounas, E. P., Untch, M., et al. (2019). Trastuzumab emtansine for residual invasive HER2-positive breast cancer. N. Engl. J. Med. 380, 617–628. doi:10.1056/NEJMoa1814017
Vozella, F., Fazio, F., Lapietra, G., Petrucci, M. T., Martinelli, G., and Cerchione, C. (2021). Monoclonal antibodies in multiple myeloma. Panminerva Med. 63, 21–27. doi:10.23736/S0031-0808.20.04149-X
Vu, T., and Claret, F. X. (2012). Trastuzumab: updated mechanisms of action and resistance in breast cancer. Front. Oncol. 2, 62. doi:10.3389/fonc.2012.00062
Wagh, A., Song, H., Zeng, M., Tao, L., and Das, T. K. (2018). Challenges and new frontiers in analytical characterization of antibody-drug conjugates. MAbs 10, 222–243. doi:10.1080/19420862.2017.1412025
Wagner, E., Colas, O., Chenu, S., Goyon, A., Murisier, A., Cianferani, S., et al. (2020). Determination of size variants by CE-SDS for approved therapeutic antibodies: Key implications of subclasses and light chain specificities. J. Pharm. Biomed. Anal. 184, 113166. doi:10.1016/j.jpba.2020.113166
Waight, A. B., Bargsten, K., Doronina, S., Steinmetz, M. O., Sussman, D., and Prota, A. E. (2016). Structural basis of microtubule destabilization by potent auristatin anti-mitotics. PLoS One 11, e0160890. doi:10.1371/journal.pone.0160890
Wang, Q., Shao, X., Zhang, Y., Zhu, M., Wang, F. X. C., Mu, J., et al. (2023). Role of tumor microenvironment in cancer progression and therapeutic strategy. Cancer Med. 12, 11149–11165. doi:10.1002/cam4.5698
Wang, Z., Li, H., Gou, L., Li, W., and Wang, Y. (2023). Antibody–drug conjugates: Recent advances in payloads. Acta Pharm. Sin. B 2023, 15. doi:10.1016/j.apsb.2023.06.015
Wedam, S., Fashoyin-Aje, L., Gao, X., Bloomquist, E., Tang, S., Sridhara, R., et al. (2020). FDA approval summary: Ado-trastuzumab emtansine for the adjuvant treatment of HER2-positive early breast cancer. Clin. Cancer Res. 26, 4180–4185. doi:10.1158/1078-0432.CCR-19-3980
Weiner, L. M., Dhodapkar, M. V., and Ferrone, S. (2009). Monoclonal antibodies for cancer immunotherapy. Lancet 373, 1033–1040. doi:10.1016/S0140-6736(09)60251-8
Wojtukiewicz, M. Z., Rek, M. M., Karpowicz, K., Górska, M., Polityńska, B., Wojtukiewicz, A. M., et al. (2021). Inhibitors of immune checkpoints—PD-1, PD-L1, CTLA-4—New opportunities for cancer patients and a new challenge for internists and general practitioners. Cancer Metastasis Rev. 40, 949–982. doi:10.1007/s10555-021-09976-0
Xiao, Y., and Yu, D. (2021). Tumor microenvironment as a therapeutic target in cancer. Pharmacol. Ther. 221, 107753. doi:10.1016/j.pharmthera.2020.107753
Xu, S. (2015). Internalization, trafficking, intracellular processing and actions of antibody-drug conjugates. Pharm. Res. 32, 3577–3583. doi:10.1007/s11095-015-1729-8
Yao, P., Zhang, Y., Zhang, S., Wei, X., Liu, Y., Du, C., et al. (2023). Knowledge atlas of antibody-drug conjugates on CiteSpace and clinical trial visualization analysis. Front. Oncol. 12, 1039882. doi:10.3389/fonc.2022.1039882
Yasunaga, M., Manabe, S., and Matsumura, Y. (2017). Immunoregulation by IL-7R-targeting antibody-drug conjugates: overcoming steroid-resistance in cancer and autoimmune disease. Sci. Rep. 7, 10735. doi:10.1038/s41598-017-11255-4
Yogev, S., Cooper, R., Fetter, R., Horowitz, M., and Shen, K. (2016). Microtubule organization determines axonal transport dynamics. Neuron 92, 449–460. doi:10.1016/j.neuron.2016.09.036
Yu, J., Song, Y., and Tian, W. (2020). How to select IgG subclasses in developing anti-tumor therapeutic antibodies. J. Hematol. Oncol. 13, 45. doi:10.1186/s13045-020-00876-4
Zahavi, D., and Weiner, L. (2020). Monoclonal antibodies in cancer therapy. Antibodies (Basel) 9, 34. doi:10.3390/antib9030034
Zammarchi, F., Corbett, S., Adams, L., Tyrer, P. C., Kiakos, K., Janghra, N., et al. (2018). ADCT-402, a PBD dimer-containing antibody drug conjugate targeting CD19-expressing malignancies. Blood 131, 1094–1105. doi:10.1182/blood-2017-10-813493
Zhang, J., Woods, C., He, F., Han, M., Treuheit, M. J., and Volkin, D. B. (2018). Structural changes and aggregation mechanisms of two different dimers of an IgG2 monoclonal antibody. Biochemistry 57, 5466–5479. doi:10.1021/acs.biochem.8b00575
Zhao, P., Zhang, Y., Li, W., Jeanty, C., Xiang, G., and Dong, Y. (2020). Recent advances of antibody drug conjugates for clinical applications. Acta Pharm. Sin. B 10, 1589–1600. doi:10.1016/j.apsb.2020.04.012
Zheng, Y., Ma, L., Sun, Q., and Tian, L. (2021). Clinically-relevant ABC transporter for anti-cancer drug resistance. Front. Pharmacol. 12, 648407. doi:10.3389/fphar.2021.648407
Ziogas, D. C., Theocharopoulos, C., Lialios, P.-P., Foteinou, D., Koumprentziotis, I.-A., Xynos, G., et al. (2023). Beyond CTLA-4 and PD-1 inhibition: Novel immune checkpoint molecules for melanoma treatment. Cancers (Basel) 15, 2718. doi:10.3390/cancers15102718
Keywords: antibody-drug conjugate, antibodies, linkers, payloads, target therapy, cancer treatment
Citation: Riccardi F, Dal Bo M, Macor P and Toffoli G (2023) A comprehensive overview on antibody-drug conjugates: from the conceptualization to cancer therapy. Front. Pharmacol. 14:1274088. doi: 10.3389/fphar.2023.1274088
Received: 07 August 2023; Accepted: 07 September 2023;
Published: 18 September 2023.
Edited by:
Ayaz Shahid, Western University of Health Sciences, United StatesReviewed by:
Jifa Zhang, Sutro Biopharma, Inc., United StatesMarco Cavaco, Universidade de Lisboa, Portugal
Copyright © 2023 Riccardi, Dal Bo, Macor and Toffoli. This is an open-access article distributed under the terms of the Creative Commons Attribution License (CC BY). The use, distribution or reproduction in other forums is permitted, provided the original author(s) and the copyright owner(s) are credited and that the original publication in this journal is cited, in accordance with accepted academic practice. No use, distribution or reproduction is permitted which does not comply with these terms.
*Correspondence: Paolo Macor, cG1hY29yQHVuaXRzLml0